Plant-Soil Feedbacks and Facilitation Influence the Demography of Herbaceous Alpine Species in Response to Woody Plant Range Expansion
- 1Department of Botany and Plant Sciences, University of California, Riverside, Riverside, CA, United States
- 2Institute of Arctic and Alpine Research, University of Colorado, Boulder, Boulder, CO, United States
Plant species migrations, or range shifts, in response to changing climate are one of many interacting factors influencing plant population and community dynamics in an era of global change. Range shifts may cause novel assemblages of competing species because species may respond to changing climate at different rates. Range-expanding species may directly influence resident species through resource competition or indirectly by modifying the local environment both aboveground and belowground. Further, range-expanding plant species can create novel plant-soil feedbacks (PSFs) by altering soil microbial community structure and function and the interactions of resident plant species with microbial symbionts. These changes can have important implications for resident plant population dynamics and their ability to coexist with novel competitors. Here we test the impacts of competitive interactions and plant-soil feedbacks (PSFs) of a range-expanding sagebrush species (Artemisia rothrockii) on the demography and population growth rates of two resident alpine plant species (Koeleria macrantha and Eriogonum ovalifolium). We use an experimental, multi-year field approach combined with integral projection modeling to determine how PSFs and competition influence species coexistence in both the historic and range expansion zone of A. rothrockii. We find that sagebrush has an overall net negative effect on herbaceous plant demography, primarily due to negative PSFs for plants growing in sagebrush-conditioned soil. However, these negative soil effects are partially buffered via facilitation effects for herbs growing under or nearby sagebrush canopies. In general, population growth rates were more sensitive to survival than other demographic rates, furthermore this sensitivity to survival was higher for herbaceous species in sagebrush soils. Identifying the major drivers of plant population dynamics and species interactions remains an important and unresolved question in ecology. PSFs are a central mechanism influencing plant species interactions, yet the majority of PSF research has made little direct connection between plant population dynamics and PSFs in situ. We believe that utilizing a field-based approach, focusing on multiple components of plant demography, is an important next step in understanding the role of PSFs and species interactions in a changing world.
Introduction
Changes in climate and land use are creating novel communities of organisms around the globe (Tylianakis et al., 2008; Lurgi et al., 2012). In terrestrial ecosystems, species migrations or range shifts, often upwards in latitude or elevation, are an important mechanism driving these changes (Parmesan et al., 2003; Valéry et al., 2008). Species ranges may become larger (expansion), smaller (contraction), or simply shift in their distribution along a climate or land use gradient (Sexton et al., 2009; Chen et al., 2011). Range shifts are limited by both environmental and biotic filters as well as species dispersal abilities (HilleRisLambers et al., 2012) and many species are unable to migrate (Zhu et al., 2012). Together, these changes in species distributions are “reshuffling” the composition of plant communities, and often have significant community and ecosystem consequences, such as altered nutrient cycling and net primary production (Wardle et al., 2011; Alexander et al., 2016; Manrubia et al., 2019).
Competitive interactions between local and range-expanding plant species will influence both the ability of the range-expanding species to successfully establish and the capacity of resident plant species to persist within their historic distribution (Körner et al., 2008; Alexander et al., 2016; Fadrique and Feeley, 2016). Successful range shifts may require strong competitive abilities, while lack thereof may limit a species' ability to colonize a new area (Krapek and Buma, 2018; Neuschulz et al., 2018). In fact, novel plant competitors were equally or more influential than warming on plant performance in plant community transplants across an alpine elevation gradient (Alexander et al., 2015). The outcomes of species interactions are determined by coexistence mechanisms including the balance between inter- and intraspecific competition and negative density dependence (Callaway et al., 1997; Chesson, 2000; Mangan et al., 2010; Piao et al., 2014). Assessing the influence of range-expanding competitors on the demography and population dynamics of resident plant species will be critical to predicting whether resident and range-expanding species will successfully coexist.
In addition to altered competitive interactions, species range shifts may have indirect effects on resident plants through altering the local environment or trophic interactions. For example, range-expanding plant species can modify local resource pools, microclimate conditions, densities of species-specific herbivores or pollinators and interactions with soil organisms (Tylianakis et al., 2008; Metcalfe et al., 2011). Plant-soil-feedbacks (PSFs) are plant-induced changes to the soil which feedback to affect plant performance (Van der Putten et al., 2013). PSFs can play an important role in shaping plant species interactions and promoting species coexistence (Bever et al., 1997, 2012; Bever, 2003). For example, PSFs can help maintain species diversity by enhancing negative soil feedbacks on conspecific individuals via the accumulation species-specific soil pathogens (Bever, 2003). On the other hand, positive PSFs can lead to competitive exclusion and species dominance, thereby reducing overall diversity (Bever, 2003). Non-native invasive species often create PSFs which further promote their invasion, including reducing the diversity of mycorrhizal fungi or soil mutualists of resident species (Hawkes et al., 2006), enhancing native soil pathogens (Eppinga et al., 2006), or selecting for microbes which preferentially degrade their own litter (Austin et al., 2014). Range-shifting plant species can also influence resident plant species via PSFs (Dostálek et al., 2016) and changes in mycorrhizal dominance (Williams et al., 2013), however further information is necessary to determine under which range-expansion scenarios this will occur (Tomiolo and Ward, 2018).
Range-expanding species that are functionally dissimilar to the native plant community may create strong PSFs, as plant origin alone (native vs. range expanding) does not necessarily predict impacts on soil microbial communities (Manrubia et al., 2019; Ramirez et al., 2019). These PSFs may arise through multiple mechanisms, including changes in the quantity or chemistry of leaf and root litter entering soil organic matter pools, changes to soil hydrology via rooting depth and structure, or association with novel microbial mutualists or pathogens (Klironomos, 2002; Wardle et al., 2004). For example, Mesquite trees expanding into desert grasslands associate with N-fixing bacteria and have deep taproots, thus altering soil nutrient pools, microbial communities and water availability for resident grasses (Wilson et al., 2001). Novel secondary compounds in litter of range-expanding species can also alter interactions of other plants with mycorrhizal fungi and free-living soil microbes (Weaver and Klarich, 1977; Nilsson et al., 1993; Wardle et al., 1998), creating potentially positive or negative PSFs.
Finally, PSFs can alter many components of the plant life cycle, including growth, survival, and reproduction, however the majority of PSF research has only considered effects on plant growth or biomass (Hovatter et al., 2013; Dudenhöffer et al., 2017). For example, seed germination may be limited by species-specific pathogens, particularly in close proximity to conspecific individuals (Mangan et al., 2010) and flower production can be enhanced by spatial heterogeneity of PSFs (Burns et al., 2017). Additionally, PSFs may cause contrasting responses across distinct phases of the plant life cycle, such as increased growth or vegetative biomass but decreased seed germination or flowering (Mehrabi et al., 2015; Dudenhöffer et al., 2017) creating an overall neutral effect on plant fitness. Therefore, all demographic life stages need to be simultaneously considered for a complete picture of how PSFs influence plant population dynamics (Dudenhöffer et al., 2017).
Woody plant range shifts are occurring in mountainous regions globally due to a variety of global change drivers including warming temperatures, increased CO2, altered precipitation, and changes in fire and grazing regimes (Myers-Smith et al., 2011). In the White Mountains of California, climate and land use change has led to an upward range expansion of a dominant subalpine shrub species, Artemisia rothrockii A. Gray (Rothrock sagebrush) into alpine grasslands over the last 60 years (Kopp and Cleland, 2014). This range expansion has coincided with decreased abundance of a native bunchgrass [Koeleria macrantha (Ledeb.) Schult] and cushion plant (Eriogonum ovalifolium Nutt.), however the mechanism(s) of these species' declines are unknown (Kopp and Cleland, 2014). We sought to determine the relative importance of direct competition with sagebrush vs. indirect soil effects, a form of apparent competition, for driving the decline in abundance of K. macrantha and E. ovalifolium in the White Mountains.
Specifically, we asked: Does sagebrush range expansion influence the demography of native alpine plant species in the White Mountains? Are sagebrush influences on demography and population growth rates (lambdas) via direct competition and/or apparent competition via PSFs, and what are the relative strengths of these mechanisms? We hypothesized that sagebrush creates negative PSFs for K. macrantha and E. ovalifolium, which manifest in lower demographic and population growth rates for plants growing in sagebrush soil. Inducing negative PSFs is a common mechanism by which non-native invasive plants gain a competitive advantage over resident species (Suding et al., 2013), and we extend this line of reasoning to a native range-expanding species. We predicted that the negative effects of PSFs will be stronger than the effects of direct competition with sagebrush because competitive interactions can be weak or shift to facilitation in stressful abiotic conditions, such as alpine environments (Callaway et al., 2002; Maestre et al., 2009).
Materials and Methods
Location
This study takes place in the subalpine to alpine zones of the White Mountains of California, which lie on the western edge of the Great Basin in the rain shadow of the Sierra Nevada range. The climate is cold and dry, receiving between 327 and 456 mm of precipitation annually and mean annual temperatures span from 0.9 to −1.7°C (Hall, 1991). These mountains have extremely diverse soil histories (Mooney and Zavaleta, 2016) but this study was confined only to granitic soils (Colluvium derived from granite) and east-/south-east-facing slopes to control for edaphic and topographic variation. Abiotic soil characteristics across the elevation gradient and plant communities of this area are summarized in Collins et al. (2016), but in general, soils have low levels of organic matter (~1.7–2.6 mg/L TOC, 0.8–0.34 mg/L TON) and low soil moisture (~1.9–10.3% VWC), which both increase with elevation. Soil pH is slightly acidic (~6) across the study area.
Study Species
We conducted this study across a gradient of A. rothrockii range expansion from subalpine (<3,500 m) to alpine (>3,500 m) zones that has been documented in the White Mountains over the last 50 years (Kopp and Cleland, 2014). In 1961, A. rothrockii was not present at the 3,800 m site, was found in moderate to low densities at the 3,500 m site, and high densities at the 3,200 m site (Mooney et al., 1962; Kopp and Cleland, 2014). Currently, it is found at high densities, but with more spacing between individuals (~1–2 m) at the low and middle elevation sites, and in isolated circular patches ~10 m wide but with shrubs more closely clustered at high elevation sites (Kopp and Cleland, 2018, personal observation). Therefore, this gradient spans from the “historic range” of A. rothrockii at low elevations to the leading edge of the “expansion range” at high elevations where A. rothrockii transitions from an almost continuous population to isolated patches.
Artemisia rothrockii is a California endemic shrub, while K. macrantha and E. ovalifolium are more widespread throughout the western United States and span a wide habitat range from pinyon juniper woodlands to subalpine forests, and alpine fellfield (Calflora, 2019). Sagebrush, primarily A. tridentata, is known to strongly compete with herbaceous plant species for water and nutrients, particularly phosphorus, across the inter-mountain west (Robertson, 1947; Caldwell et al., 1985; Fowler, 1986; Ryel et al., 2004). Sagebrush also directly alters the abiotic soil environment including enhancing water and nutrients below its canopies as compared to herbaceous soils (Collins et al., 2016). Additionally, sagebrush alters the soil microbial community structure and function, including the diversity and community composition of soil bacteria and fungi, substrate induced respiration (CO2 flux) and extracellular enzyme activity (Collins et al., 2016, 2018, and unpublished data).
These changes are likely to have important feedbacks on herbaceous plant species by altering the relative abundances of microbial taxa, such as species-specific soil mutualists and pathogens. In addition, secondary compounds in sagebrush litter may alter how herbaceous plants (grasses, forbs etc.) interact with mycorrhizal fungi and free-living soil microbes (Weaver and Klarich, 1977; Nilsson et al., 1993; Wardle et al., 1998). Aboveground sagebrush removal led to the re-establishment of herbaceous cover (including K. macrantha and E. ovalifolium) after 4 years in the White Mountains suggesting potentially high levels of interspecific competition (Kopp and Cleland, 2018). However, indirect soil effects of sagebrush on alpine plant growth, a form of apparent competition, may be as strong or stronger than the direct effects of competition with sagebrush (Allen et al., 2018).
Experimental Design
We monitored populations of K. macrantha and E. ovalifolium at three elevations described previously: 3,200, 3,500, and 3,800 m (3,200 m site: UTM: 396148 E; 4151156 N; 3,500 m site: UTM: 390629 E124157248 N; 3,800 m site: UTM: 390445 E; 4159559 N-UTM Zone 11). In July 2015, we established 30 experimental blocks, each with four 0.5 × 0.5 m plots (treatments) and each block was repeated 5 times for each species at each elevation (site). Each plot has one of the following 4 treatments: shrub competition, shrub removal, herbaceous competition and herbaceous removal (Figure 1). Shrub plots (competition and removal) were selected where individuals of K. macrantha and/or E. ovalifolium were growing directly under or very nearby (<0.25 m) a sagebrush canopy. Herbaceous plots (competition and removal) were selected in the interspaces of sagebrush between 1 and 5 m away from the nearest shrub canopy, based on the sagebrush density at each site. For competition plots, the entire plant community was left intact. For shrub removal plots, aboveground sagebrush biomass was removed by cutting down stems at the base. For herb removal plots, aboveground biomass of all non-target herbaceous plant species was removed by manually clipping with scissors. For both removal plots, only aboveground biomass was removed to prevent significant disturbance to soil structure. All treatments were maintained annually, and any regrowth trimmed back.
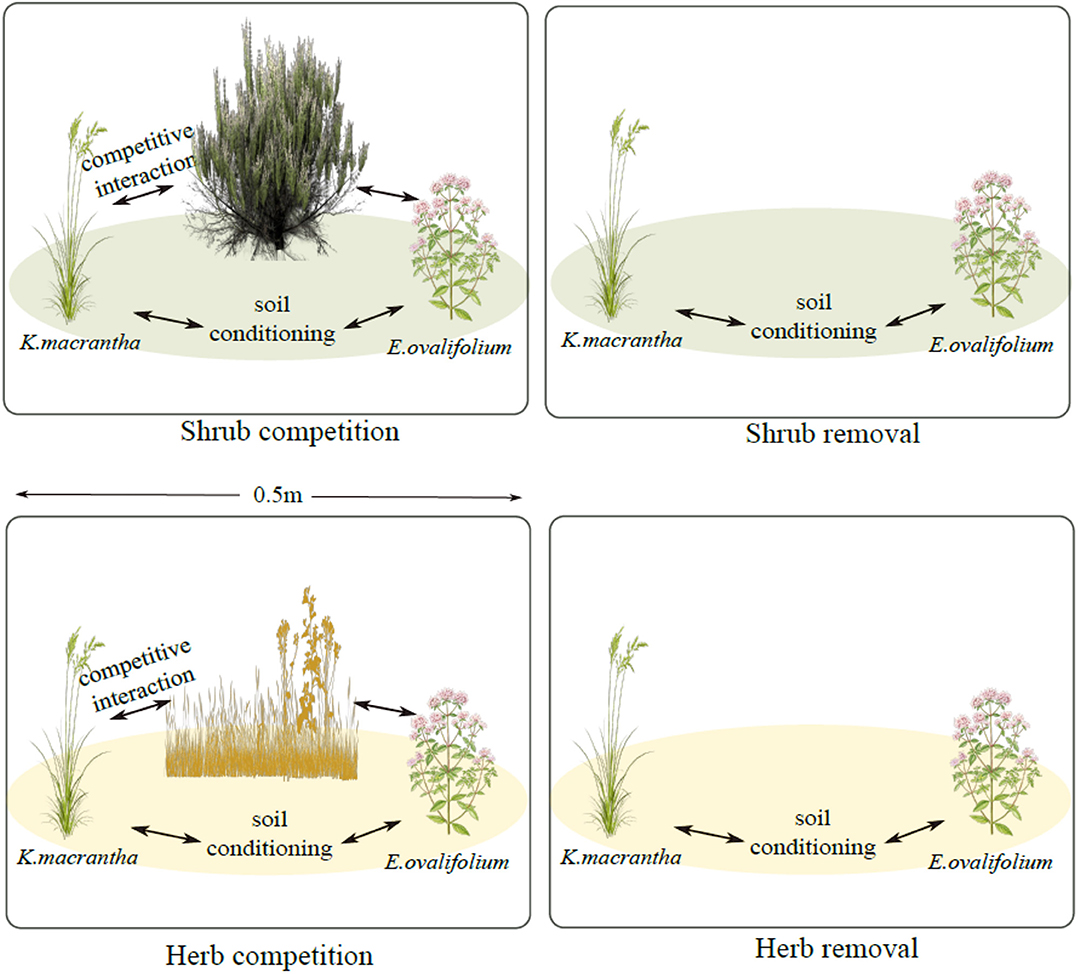
Figure 1. Experimental plot design for field demographic measurements. Green ovals represent sagebrush soil conditioning and yellow ovals represent soil conditioning by the herbaceous community.
Our experiment was designed to disentangle above- and belowground influences of sagebrush on herbaceous plant demography under natural field conditions. Plants growing in removal plots are experiencing soil legacies (both biotic and abiotic) of either the shrub or the herbaceous community, but without aboveground competition from the removed species. In the non-removal plots (competition), plants are experiencing both the soil conditioning and competition from shrubs and/or herbs. By comparing performance of the focal species in the shrub plots and the shrub removal plots, we can therefore isolate the effect of shrub competition (Figure 1). Similarly, by comparing the herbaceous plots to the herb-removal plots, we estimated the effects of herb competition. To estimate the effects of shrub PSFs, in the absence of shrub competition, we compared the shrub removal plots to the herbaceous removal plots, because the primary difference between those plots was the identity of plants conditioning the soil. Sagebrush, soil conditioning overwhelms that of herbaceous plants in its litter chemistry and biomass, and creates a distinct soil environment underneath its crown (Welch, 2005; Collins et al., 2016) and therefore we attribute soil effects to sagebrush directly. However, we do not estimate PSFs of the herbaceous community, as the species composition was more variable across sites, and soil conditioning is much less concentrated than under shrub canopies.
Field-based approaches for measuring plant-soil feedbacks have been used successfully in other studies (Kulmatiski, 2006; Mccarthy-Neumann and Ibáñez, 2012). Nonetheless, there are trade-offs to this approach relative to more traditional controlled experiments in a greenhouse or common garden. A strength of this approach is that soils are conditioned in the field, under natural climate conditions and over longer time periods, creating a more realistic soil environment (Kulmatiski and Kardol, 2008; Pernilla Brinkman et al., 2010). Additionally, this approach does not risk spurious effects that can occur with soil sterilization (Bonanomi et al., 2005). A limitation of a field-based approach, however, is that is not possible to disentangle the influences of soil microbial communities and the physical soil environment in the field (Kulmatiski and Kardol, 2008; Pernilla Brinkman et al., 2010). Thus, our estimated PSFs include all physio-chemical and microbial changes caused by sagebrush soil conditioning to be plant-soil feedbacks potentially influencing plant demography.
Demographic Measurements
Within each of the four plots (treatments) in all blocks, we tagged up to five adult individuals, depending on species density at the site, of either K. macrantha or E. ovalifolium and took initial demographic measurements in July 2015. For K. macrantha, plant area was calculated by multiplying height of the tallest leaf (cm) by width of the tussock (cm). For E. ovalifolium, plant area was calculated through digital image analysis. Photos of each individual plant were taken with a ruler for scale in the field and were then analyzed in ImageJ (Version 1.51 J8) based on the methodology of Jarou (2009). For both species, we measured flowering status (Y/N), and number of inflorescences of each flowering individual. Seed production per inflorescence was calculated as a single value for each species on 100 additional inflorescences which were counted in the laboratory using a dissecting microscope to ensure seed maturity/viability. Beginning in 2016, mortality was also recorded as alive (Y/N) for each individual. Plots were re-sampled yearly in mid-July to early August (depending on snow melt) and all measurements taken for three subsequent years, for a total of four years of measurements (2015, 2016, 2017, 2018).
Recruitment probabilities were estimated using seed germination trials for each species. In September 2017, mature seeds from both species were collected from 10 individuals at each elevation. Seeds were placed in 12 × 12 cm mesh bags and then deployed in the field by fixing them to the upper soil surface using metal stakes. Each bag contained 10 seeds and for each species, 12 bags were deployed at each elevation site, six under sagebrush canopies and six in shrub interspace. Bags were collected in mid-July 2018, and total number of germinated seeds in each bag were recorded. Probabilities were calculated as the total of germinated seeds/sum seeds deployed. Due to low overall germination, single probabilities were calculated for each species and were not elevation- or treatment-specific. In addition, due to low germination percentages and slow growth of alpine plants, we were unable to measure recruit sizes in the field. For K. macrantha, we estimated recruit size distribution from the seedling dataset of Chu and Adler (2014). For E. ovalifolium, due to the lack of available information on this species, we simulated seedling size data based on the smallest 2.5% of adults in the dataset, which produced a size distribution of 0.001–2.5 cm2 and a mean of 0.6 cm2. While this modeling choice could affect the magnitude of estimated population growth rates, it should not bias the analysis of treatment effects.
Population Modeling
We calculated size-dependent demographic rates (growth, survival probability, flowering probability, and seed production) using 229 and 224 individuals of K. macrantha and E. ovalifolium, respectively. Plant size was logged in all models for normality and seed number was logged to transform from count data to continuous. Germination probability was estimated as a single value for each species based on seed germination trials, and recruit size was estimated using an intercept only linear model using the dataset from Chu and Adler (2014) for K. macrantha and a simulated dataset of realistic recruit sizes for E. ovalifolium as described above.
We used mixed effects models for each demographic rate, including fixed effects of size, treatment and elevation, and a random effect of year. We fit these as Bayesian models using the brms package (Bürkner, 2018) in R (R Core Team, 2015), and using the default non-informative, improper priors for all models. We used a “nested” model structure with elevation effects nested within treatments. Importantly, we fit this model with elevation nested within treatments in order to allow for “partial pooling” of information across elevations within each treatment. Partial pooling allowed separate estimates of demographic rates at each treatment × elevation combination, but the data from different elevations, within a treatment, informed each other. This approach is therefore a compromise between complete pooling of data across elevations and independent estimates for each elevation × treatment. This was a conservative modeling decision based on the observation that mortality events in particular were sparse in the dataset; the partial pooling prevents biases from sparse data, such as the chance event that an elevation has no mortality (see Figure S3 for comparison of the “partial pooling” model with a “no pooling” model). We tested for the treatment and elevation effects on each demographic rate by calculating pairwise contrasts using the posterior distributions and computing the probabilities that the difference between each pair was different from zero.
Using the posterior distributions from the demographic rate models, we constructed integral projection models (IPMs) to calculate population growth rates (lambdas) for each species within each treatment × elevation combination. These population models and estimated lambdas were used as a way to integrate the effects of sagebrush across multiple phases of the plant life cycle, rather than accurate projections of population growth rates. Thus, we consider lambda to be an estimate of the relative fitness of each species among the different plot treatments, and do not suggest they will accurately predict changes in population sizes over time. The effects of shrub and herbaceous competition, as well as shrub PSFs, on lambdas were calculated using a-priori contrasts between the lambdas estimated in the different treatments: Shrub Competition = (Shrub competition) – (Shrub removal); Herb Competition = (Herb competition) – (Herb removal); Shrub PSF = (Shrub removal) – (Herb removal) (Figure 1). These contrasts were calculated from the posterior distributions of estimated lambdas within each treatment × elevation.
Finally, we conducted elasticity analyses to assess the contributions of different demographic rates to lambdas, and the effects of treatments on demographic rate sensitivities. The elasticity analyses on growth, survival, and probability of reproduction determine the sensitivity of lambda to changes in specific demographic rates. For all IPM analyses, we used modified R code from Ellner et al. (2016).
Results
Demographic Rate Models
All demographic rates varied by treatment and elevation for both species. Pairwise contrasts for all demographic rates among plot treatments within each elevation and the probabilities that each treatment is greater (or less) than another treatment are listed in Tables S1 and S2. Here we report contrasts where the probability was >0.75 (Table 1).
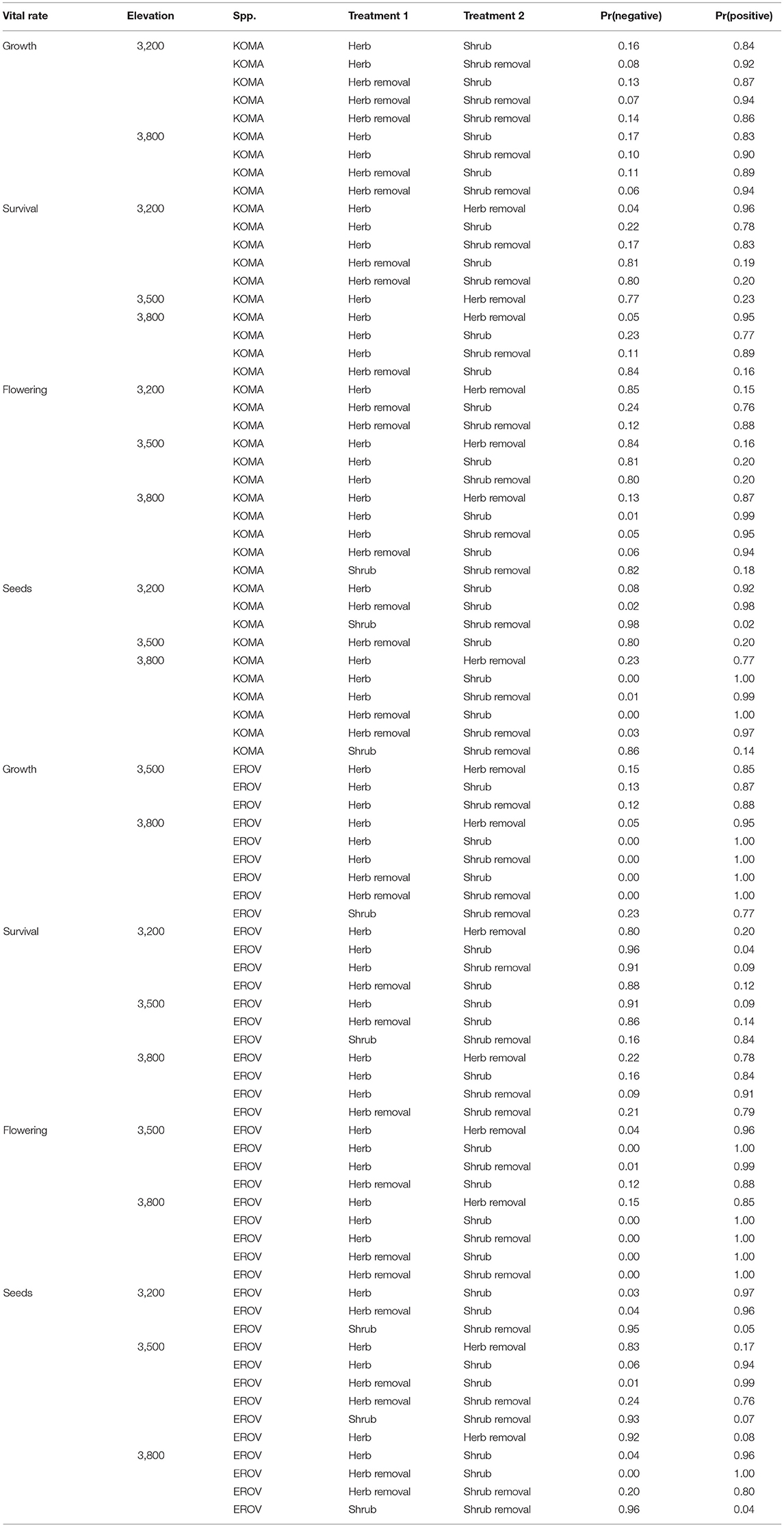
Table 1. Contrasts among vital rates for Koeleria macrantha (KOMA) and Eriogonom ovalifolium (EROV). Pr(negative) represents the probability that the effect of Treatment 1 < Treatment 2, whereas Pr(positive) is the probability that the effect of Treatment 1 > Treatment 2 [and is equal to 1 – Pr(negative)]. Only contrasts with probabilities >0.75 are displayed.
We find in general that demographic rates tended to be highest in herbaceous and herbaceous removal plots for both species, and this pattern was strongest at the high and low elevation sites. For K. macrantha, growth was highest in herbaceous plots at the low elevation site and in herbaceous removal plots at the middle and high elevation sites. Growth in herbaceous and herbaceous removal plots was higher in than in shrub and shrub removal plots which suggests that sagebrush has a negative effect on Koeleria growth (Table 1, Figure S1). Survival varied greatly by treatment and elevation and overall was highest herbaceous removal plots at the middle elevation site and herbaceous plots at the low and high elevation sites (Table 1, Figure 2, Figure S1). Probability of flowering was greatest in herbaceous and herbaceous removal plots particularly at high elevation, however was higher in shrub plots at the middle elevation site (Table 1, Figure S1). Similarly seed production was higher in shrub plots at the middle elevation site which suggests that sagebrush may have a positive effect on reproduction at this site which counters the negative effects on growth and survival (Table 1, Figure S1). At low and high elevations flowering and seed production were greater in herbaceous and herbaceous removal plots than in shrub and shrub removal plots, which follows the general pattern we observe for the other demographic rates (Table 1, Figure S1).
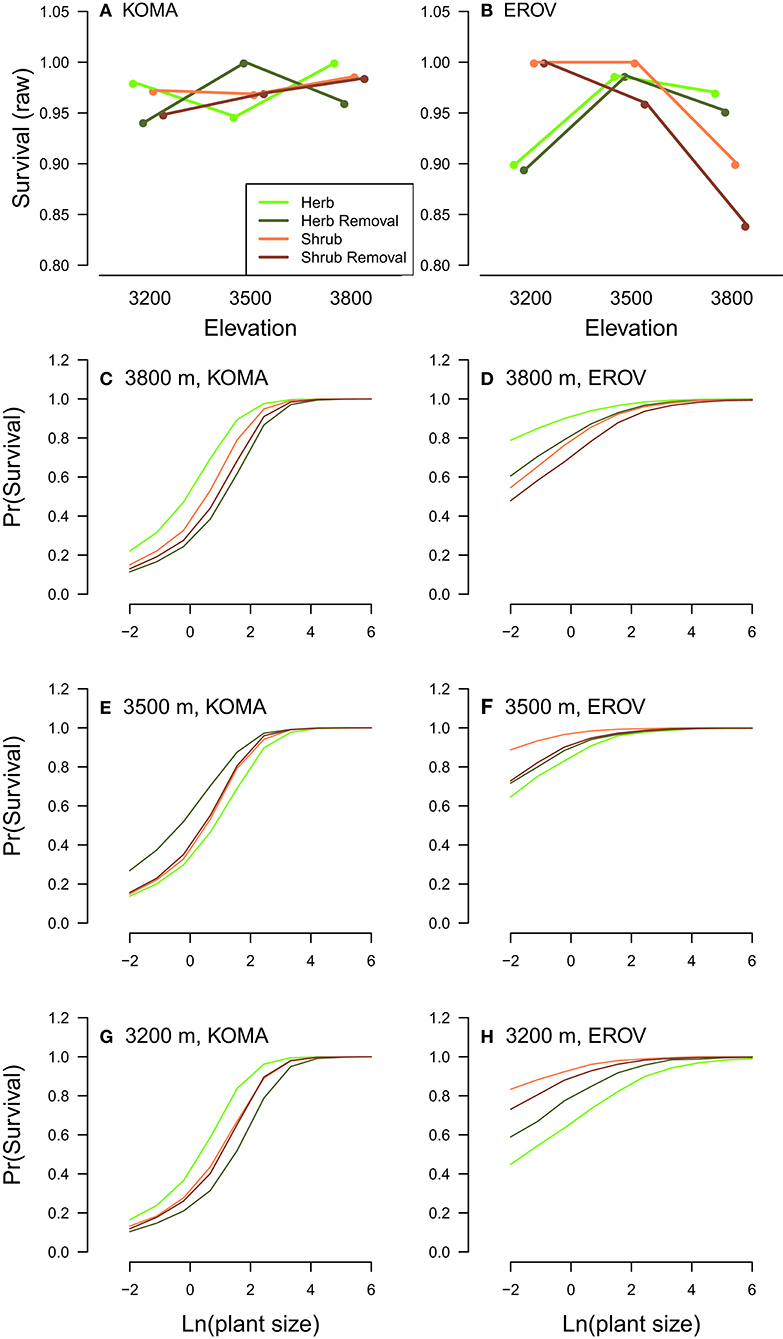
Figure 2. Plot treatment effects on survival for K. macrantha (KOMA, left column) and E. ovalifolium (EROV, right column). Raw survival data is shown in the top row (A,B) plotted by elevation (m) on the x-axis and by plot treatments in different colors. Modeled probability of survival is shown in the bottom figures (C–H) and plotted separately for each elevation by species combination, with individual plant size on the x-axis and colored lines signifying different plot treatments.
Many patterns observed in K. macrantha demographic rates were similar for E. ovalifolium. Growth was highest in herbaceous and herbaceous removal plots at the middle and high elevation sites and no treatments were different at the low elevation site. Growth in herbaceous and herbaceous removal plots was higher in than in shrub and shrub removal plots which suggests that sagebrush also has a negative effect on Eriogonum growth (Table 1, Figure S2). Survival also varied by treatment and elevation and overall was highest shrub and shrub removal plots at the low and middle elevation site, but then dropped significantly at the high elevation site, and fell below both herbaceous and herbaceous removal plots (Table 1, Figure 2, Figure S2). Probability of flowering was greatest in herbaceous and herbaceous removal plots, particularly at high elevation, and was higher than shrub and shrub removal plots except at low elevation where no treatments differed (Table 1, Figure S2). Seed production was highest in herbaceous and herbaceous removal plots across all elevations but especially at the high elevation site. This suggests that sagebrush has a more negative influence on Eriogonum than Koeleria reproduction, particularly in the range expansion zone (Table 1, Figure S2).
Population Growth Rates (Lambdas)
Lambda values were higher overall for E. ovalifolium than K. macrantha, while the differences among lambdas across treatments and elevations were distinct for each species. Herb and herb removal plots had higher median lambda values than shrub and shrub removal plots for K. macrantha at low and high elevations and for E. ovalifolium at high elevation (Figures 3A,B). This reflects the general pattern observed in the demographic rate models where negative effects of sagebrush were strongest at the two ends of the elevation gradient. At 3,500 m elevation, lambdas did not differ among the plot treatments except for a slight increase in herbaceous removal plots for K. macrantha (Figure 3A). Lambdas in shrub and shrub removal plots were higher than in herb and herb removal plots for E. ovalifolium at 3,200 m elevation (Figure 3B). This is likely due to the fact that survival was higher in shrub and shrub removal plots at this site, as survival has a very large contribution to lambda in this system (see elasticity analyses).
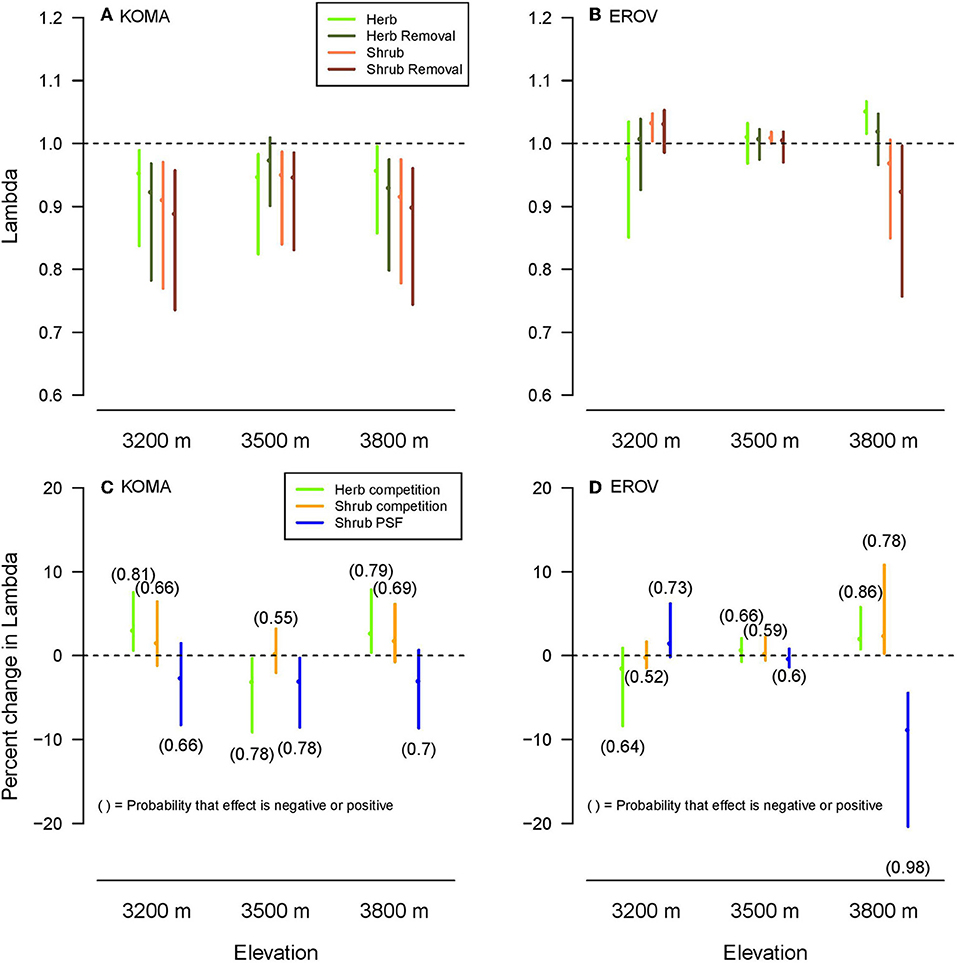
Figure 3. Population lambdas (A,B) by plot treatment and elevation for K. macrantha (KOMA, left column) and E. ovalifolium (EROV, right column). Change in lambda (C,D) plotted for each a-priori contrast (Herb competition: Herb–Herb removal, Shrub competition: Shrub–Shrub removal, Shrub PSF: Shrub removal-Herb removal) shown in colors. Numbers above or below line, respectively signify the probability that the effect of the contrast on lambda is positive or negative.
For the a-priori contrasts, Herb competition (herb competition-herb removal) had a positive effect (probability >0.75) on lambda for K. macrantha at 3,200 and 3,800 m elevations and E. ovalifolium at 3,800 m elevation, and a negative effect on lambda for K. macrantha at 3,500 m elevation (Figures 3C,D). Shrub competition (shrub competition – shrub removal) had a positive effect on lambda for E. ovalifolium at 3,800 m elevation, and slightly positive to neutral effect at other elevations (Figures 3C,D). Shrub PSFs (shrub removal – herb removal) had a negative effect on lambda for E. ovalifolium at 3,800 m elevation and K. macrantha at 3,500 m elevation and slightly negative to neutral effect on lambda at other elevations except for E. ovalifolium at 3,200 m where the effect was slightly positive (Figures 3C,D).
Elasticity Analyses
Survival had the highest impact on population lambdas of all demographic rates (max elasticity values ~0.35–0.45) for both species however E. ovalifolium had slightly higher elasticity than K. macrantha, particularly at the high elevation site in shrub and shrub removal plots (Figure S4). Growth had the next highest impact on lambda (max elasticity values ~0.16–0.25) for both species however K. macrantha, had higher elasticities than E. ovalifolium, which signifies that growth contributed more to population lambdas in the grass species. Additionally, the influence of plant growth on lambdas was more important at larger size class transitions [moving from size class ~z(log) 4 to ~z(log) 5] for Koeleria vs. smaller size class transitions [~z(log) 2 to ~z(log) 3] for Eriogonum (Figure S5). This shows that changes in the growth rate of larger, smaller individuals are more important for population lambdas in the grass, cushion plant, respectively. Growth elasticities did not vary noticeably by elevation or treatment. Probability of reproduction contributed the least to population lambdas, however had a much more significant contribution for Eriogonum (max elasticities ~0.005–0.016) than Koeleria (max elasticities ~0–0.0025) (Figure S4).
Discussion
Understanding the broader implications of species range shifts will be crucial as climate change continues to promote differential species migration and novel species interactions (Midgley et al., 2007; Tomiolo and Ward, 2018). In this study, we sought to tease apart the potential above- and belowground mechanisms by which a range-expanding sagebrush species, Artemisia rothrockii, affected the demography of two herbaceous alpine plant species. We found that sagebrush most often had a net negative effect on the demographic rates and population lambdas of herbaceous species. Our results suggest that these effects were driven by negative PSFs for plants growing in sagebrush conditioned soil, counteracting facilitative effects of sagebrush aboveground. However, we found variation among species and across elevations suggesting that the effects of sagebrush PSFs vary based on abiotic conditions of the site and for herbaceous species identity. Overall these results show for the first time, using a manipulative field experiment and demographic modeling, that shrubs may have both positive and negative impacts on herbaceous plant demography due to distinct aboveground and belowground mechanisms.
Demographic Rates and Lambdas
Sagebrush affected the demography and population lambdas of both E. ovalifolium and K. macrantha across an elevation gradient in the White Mountains. Specifically, plants growing with sagebrush, or in its conditioned soils, tended to have slower growth, reduced probability of flowering and lower seed production than those growing in herbaceous dominated soils. Thus, population lambdas that were highest in herbaceous and herbaceous removal plots and lowest in shrub and shrub removal plots, respectively (Figure 3). This supports the hypothesis that sagebrush would have a negative effect on the demography of native alpine plants. These differences were particularly pronounced at the high elevation site and were consistent for both species of interest, suggesting the strong influence of sagebrush on herbaceous plant population dynamics in the range expansion zone.
Similar to our findings, an herbaceous forb species growing in association with four Mediterranean montane shrubs had reduced reproductive output including lower number of seeds and reproductive stems, and lower infructescence volume compared to individuals growing in open areas (Macek et al., 2016). In our study system, however, previous work showed that A. rothrockii slowed the phenology and reduced the flower production of a Trifolium cushion plant via shading, thus decreasing the benefits of climate warming on reproduction (Kopp and Cleland, 2015). This contrasts somewhat with our finding of reduced reproductive output (flowering and seeds) in both shrub and shrub removal plots, suggesting that the relative importance of shading vs. belowground effects may vary among herbaceous species.
Survival of E. ovalifolium was a notable exception to this overall pattern, being significantly higher in shrub and shrub removal plots at low and middle elevations. However, survival sharply declined in shrub and shrub removal plots at the high elevation site for E. ovalifolium (Figure 3B). For K. macrantha, survival was intermediate in shrub and shrub removal plots depending on elevation. These treatment effects on survival were critical because overall mortality was low across the observation period (~2% Koeleria, ~2.5% Eriogonum). Low mortality rates are common for slow-growing alpine species that are well-adapted to stressful abiotic conditions (Körner, 2003). Therefore, when mortality events do occur, they can strongly impact population growth rates (see Elasticity analyses).
The differences in how E. ovalifolium and K. macrantha responded to the experimental treatments reflect the large differences in their life history characteristics. E. ovalifolium is a slow growing, long lived, cushion plant while, K. macrantha is a/perennial bunchgrass with an average lifespan between 7 and 10 years (Dixon, 2000; Rundel et al., 2005; Anderson, 2006). Koeleria is solely wind pollinated, while Eriogonum is wind pollinated, but also largely insect and bird pollinated, and produces many fewer seeds per inflorescence. Finally, Koeleria senesces most of its aboveground biomass annually, while Eriogonum retains green leaves throughout the winter. Despite these strong differences, overall patterns in lambda were relatively similar, particularly at high elevation sites, where sagebrush establishment is most recent and therefore demographic rates will potentially be most responsive.
Elasticity Analyses
We used elasticity analyses to understand which demographic rates contributed most to the observed patterns in population lambdas and how robust lambda values were to changes in demographic rates. Survival had the largest contribution to population lambdas, followed by growth and then probability of reproduction. Elasticities also varied among the two species, treatments and by elevation. Eriogonum had higher elasticities in response to changes in survival, particularly at high elevation in shrub and shrub removal plots. This suggest that in areas of recent sagebrush establishment, population growth may be particularly affected by mortality events for this species, and more so than when growing in the herbaceous plant community.
Although survival was the most important demographic rate for both species, changes in growth were more important for Koeleria than Eriogonum, likely due to faster growth and a shorter lifespan of this grass species. Finally, the probability of reproduction had a very minor influence on lambda but was significantly more important for E. ovalifolium than K. macrantha. Again, this likely reflects differences in the life history characteristics of these species in that Eriogonum produces fewer seeds per inflorescence and has a more complex pollinator strategy, making reproduction a more important component of its overall population growth. Overall, lower lambda values suggest that K. macrantha is more likely than E. ovalifolium to decline in the future (Figures 3A,B), however, more years of data are needed to confirm this trend.
Above and Belowground Effects on Lambdas
We found support for the hypothesis that the effects of sagebrush PSFs on lambda would be more negative than the effects of sagebrush competition. The effects of sagebrush presence on lambda were neutral to slightly positive suggesting weak competition to facilitation, with the strongest positive effect for E. ovalifolium at the high elevation site. This supports the well-known hypothesis that species interactions will become more positive (facilitative) as abiotic stress increases (Callaway et al., 2002; Maestre et al., 2009).
Nurse plant facilitation of herbs commonly occurs through enhanced resources, such as water and nutrients and by buffering effects of extreme temperatures, wind or snow in the understory (Körner, 2003). Indeed, A. rothrockii has increased soil moisture and higher soil organic matter content below its canopies as compared to shrub interspace areas in the White Mountains (Collins et al., 2016); however, the effect of sagebrush facilitation on herbaceous demography was not consisitent across elevations and herbaceous species. Facilitation intensity can increase with functional dissimilarity among species at the cold and wet end of a stress gradient (Gallien et al., 2018) suggesting that shrubs may most strongly facilitate herbs at high elevations. Despite this, overall lambda values for shrub plots tended to be lower than herbaceous plots regardless of treatment, suggesting that the benefits do not outweigh the costs of growing in association with this shrub species.
As predicted, the effects of shrub PSFs on lambda were generally negative, implying that in the absence of competition, plants growing in shrub-conditioned soils had lower growth, survival and reproduction than those growing in herbaceous-conditioned soils. The one exception to this pattern was a slightly positive effect on lambda for E. ovalifolium at the low elevation. PSFs are therefore a potentially strong form of apparent competition by which sagebrush negatively impacts resident plant species. Many factors can determine the strength of PSFs of range expanding species on native communities and whether they are positive or negative. For example a range-expanding forb species had positive PSFs that enhanced the growth of a co-occurring native grass species, but only in the expansion zone (Dostálek et al., 2016). Here we find accordingly that sagebrush PSFs were stronger in the range expansion zone than in the native range (high vs. low elevation), but unlike the previous study, effects on resident plants were negative rather than positive. This may be due to differences in the time of soil conditioning between the historic and range expansion zones, as sagebrush is more recently established at high elevation sites. Range expanding species may also impose different PSFs depending on their relatedness to the resident community. Koorem et al. (2018) found that range expanders that were unrelated to resident plant species reduced the biomass production of the resident plant community, whereas related range expanding species did not. In our study, PSFs may be enhanced because sagebrush is not closely related (congeneric) with either herbaceous species.
Due to our experimental design however, we can only speculate whether the PSFs of sagebrush on resident plant species are due to changes in soil microbial communities, abiotic soil conditions, or both. One potential PSF mechanism is through secondary compounds (e.g., terpenes, jasmonic acid) in aromatic shrubs, such as Artemisia that can enter the soil through leaf litter and root exudates and have strong negative effects on plant growth, metabolism, and seed germination (Weaver and Klarich, 1977; Kelsey et al., 1978; Karban, 2007). These classes of chemicals can also strongly influence soil microbial community structure and function including microbial biomass C and N, respiration, nitrogen fixation, soil faunal substrate choice, and mycorrhizal networks of co-occurring plant species (Weston and Putnam, 1985; Wardle et al., 1998; Asensio et al., 2012; Austin et al., 2014). For example, organic compounds in the dwarf shrub Empetrum hermaphroditum greatly reduced Ectomycorrhizal infection of root tips and mycorrhizal uptake of soil Nitrogen for pine seedlings (Nilsson et al., 1993). Labile C in these compounds may also stimulate free-living (saprotrophic) microbial growth and nutrient immobilization, thus increasing resident plant-microbial competition for limiting soil nutrients. This was proposed as a mechanism by which Betula, Empetrum, and Cassiope shrub species inhibited the growth of nearby graminoid species in arctic soils (Michelsen et al., 1995). Therefore, via secondary chemicals, sagebrush may similarly alter plant-microbe competition in ways that enhance their own growth and nutrient acquisition to the detriment of co-occurring herbaceous plant species.
In previous work, we found that soils under sagebrush had higher bacterial diversity but lower fungal diversity than soils under herbaceous plants, and this corresponded with a decrease in both pathogenic and mutualistic fungi (Collins et al., 2016, 2018, and unpublished data). A change in soil mutualist to pathogen ratios has been shown to facilitate both inter- and intracontinental range expansions, as plants may benefit from decreased species-specific pathogens, while utilizing more generalist soil mutualists (van der Putten et al., 2016). However, the patterns we observe in this study suggest the opposite may be true for herbaceous plants growing in association with the range expander (sagebrush), which may still be experiencing negative effects of their own soil pathogens, but also a decreased abundance of soil mutualists. These effects may strengthen over time in the range expansion zone with more sagebrush soil conditioning, or they may attenuate as herbaceous plants become adapted to the sagebrush soil community (Rout and Callaway, 2012).
We acknowledge that the experimental plot treatments do not completely isolate the effects of sagebrush soil conditioning, and there may be other factors contributing to the changes in lambdas in the shrub PSF contrasts. It is possible, for example, that removal of shrub facilitation could negatively impact lambdas in shrub removal plots. However, we find that shrubs are weaker facilitators than the herbaceous community, and yet the difference between shrub removal and herbaceous removal plots is mostly negative, suggesting that lack of shrub facilitation is not driving the negative PSFs. It is also possible that some belowground competition may still occur between herbaceous and shrub roots after aboveground shrub removal, however we expect these interactions to be minor and short term, while soil legacy effects left by sagebrush can last many years after shrub removal or death (Collins et al., 2016, 2018). Therefore, the demographic patterns observed in shrub removal plots are very likely attributable to sagebrush soil conditioning, although we cannot rule out some remnant belowground competition. Additionally, our experiment lacks distinct “conditioning” and “feedbacks” phases, but instead measures feedbacks over a longer, continuous time frame. While this has the strength of being more relevant to population dynamics, which unfold over longer time periods than most discrete PSF experiments, it also may dampen the ability to disentangle PSFs during the transition period after aboveground biomass removal.
Overall the patterns we observed were variable and sometimes weak, however this is to be expected due to the due to slow growth, low mortality and high stress tolerance of plants in alpine environments, and the relatively short observation period (4 years) given these species' lifespans. Nonetheless, the data show that the effects of sagebrush on lambdas were more negative than the effects of the herbaceous community, particularly in the absence of aboveground competition and at middle and high elevation sites, providing a potential PSF mechanism for the observed declines in cover of K. macrantha and E. ovalifolium in areas of sagebrush expansion. While stronger effect sizes may have been observed in a more controlled greenhouse setting, estimating longer-term population dynamics in a field setting is more indicative of likely consequences for the species in nature.
Conclusions
Alpine landscapes are characterized by heterogeneous microclimates, resource availability and species interactions which can have large impacts on plant fitness (Körner, 2003). The movement of woody shrubs upwards in elevation, occurring in alpine ecosystems across the globe due to climate and land use change, may therefore alter these landscapes and affect the persistence of rare and endemic plant species. Here we find that shrubs can have both positive and negative impacts on herbaceous alpine plants simultaneously, and through both above and belowground mechanisms. Shrubs may facilitate herbs growing under or nearby their canopies particularly at high elevations, likely by shielding the effects of extreme temperatures, wind or snow. At the same time, shrubs may cause negative effects on herbaceous plants through PSFs predictably from changes in litter chemistry and their influences on plant growth and soil microbial community structure and function. These changes created by shrubs may impact multiple components of the plant life cycle, including growth, survival, and reproduction, and these components can have divergent responses which together determine the net outcomes for population growth.
The majority of PSF research has been carried out in controlled environments, particularly greenhouse experiments, while little direct connection has been made between plant population dynamics and PSFs in situ (Kulmatiski and Kardol, 2008; Kulmatiski et al., 2008; Heinze et al., 2016). In addition, while conceptually well-developed, attempts to disentangle the effects of direct competition and PSFs on population dynamics and species coexistence have been rare (Bever et al., 1997; Revilla et al., 2013). The experimental field approach used here can help isolate direct competitive interactions vs. influences of soil conditioning on plant demography. By coupling these experiments to demographic modeling, it is possible to scale the aboveground and belowground effects of a range-expanding species to the effects on population dynamics and species coexistence over time. We believe this approach of combining estimates of PSFs in the field with species-specific population modeling is an important next step in understanding plant community dynamics in a changing world.
Data Availability Statement
The datasets generated by this study are available on request to the corresponding author.
Author Contributions
CC developed the research questions, designed and set up the experimental plots, collected the field data, and wrote the first draft of the manuscript. JD and TB analyzed the data and ran the statistical models. All authors created the graphics, wrote the sections of the manuscript, contributed to the manuscript revision, read and approved the submitted version.
Funding
Research funding was provided by the University of California Riverside Department of Botany and Plant Sciences and a White Mountain Research station mini-grant. CC was supported by a University of California President's Dissertation Year Fellowship. Publication of this article was funded by the University of Colorado Boulder Libraries Open Access Fund.
Conflict of Interest
The authors declare that the research was conducted in the absence of any commercial or financial relationships that could be construed as a potential conflict of interest.
Acknowledgments
We thank Christopher Karunos and Soren Weber for field assistance and help with setup experimental plot treatments. We thank Michele Slaton, Dylan Neubauer, and members of the CNPS Bristlecone chapter for helpful botanical knowledge of White Mountain flora. We thank the Andrew Tran and Amy Hsu for assistance with digital image analyses.
Supplementary Material
The Supplementary Material for this article can be found online at: https://www.frontiersin.org/articles/10.3389/fevo.2019.00417/full#supplementary-material
References
Alexander, J. M., Diez, J. M., Hart, S. P., and Levine, J. M. (2016). When climate reshuffles competitors: a call for experimental macroecology. Trends Ecol. Evol. 31, 831–841. doi: 10.1016/j.tree.2016.08.003
Alexander, J. M., Diez, J. M., and Levine, J. M. (2015). Novel competitors shape species' responses to climate change. Nature 525, 515–518. doi: 10.1038/nature14952
Allen, W. J., Meyerson, L. A., Flick, A. J., and Cronin, J. T. (2018). Intraspecific variation in indirect plant–soil feedbacks influences a wetland plant invasion. Ecology 99, 1430–1440. doi: 10.1002/ecy.2344
Anderson, D.G. (2006). Eriogonum Exilifolium Reveal (Dropleaf Buckwheat): A Technical Conservation Assessment. [Online]. USDA Forest Service, Rocky Mountain Region. Available online at: http://www.fs.fed.us/r2/projects/scp/assessments/eriogonumexilifolium.pdf (accessed July 15, 2019).
Asensio, D., Yuste, J. C., Mattana, S., Ribas, À., Llusià, J., and Peñuelas, J. (2012). Litter VOCs induce changes in soil microbial biomass C and N and largely increase soil CO2 efflux. Plant Soil 360, 163–174. doi: 10.1007/s11104-012-1220-9
Austin, A. T., Vivanco, L., González-Arzac, A., and Pérez, L. I. (2014). There's no place like home? An exploration of the mechanisms behind plant litter-decomposer affinity in terrestrial ecosystems. New Phytol. 204, 307–314. doi: 10.1111/nph.12959
Bever, J., Westover, K., and Antonovics, J. (1997). Incorporating the soil community into plant population dynamics: the utility of the feedback approach. J. Ecol. 85, 561–573. doi: 10.2307/2960528
Bever, J. D. (2003). Soil community feedback and the coexistence of competitors: conceptual frameworks and empirical tests. New Phytol. 157, 465–473. doi: 10.1046/j.1469-8137.2003.00714.x
Bever, J. D., Platt, T. G., and Morton, E. R. (2012). Microbial population and community dynamics on plant roots and their feedbacks on plant communities. Annu. Rev. Microbiol. 66, 265–283. doi: 10.1146/annurev-micro-092611-150107
Bonanomi, G., Giannino, F., and Mazzoleni, S. (2005). Negative plant–soil feedback and species coexistence. Oikos 111, 311–321. doi: 10.1111/j.0030-1299.2005.13975.x
Bürkner, P.-C. (2018). Advanced Bayesian multilevel modeling with the R package brms. R J. 10:395. doi: 10.32614/RJ-2018-017
Burns, J. H., Brandt, A. J., Murphy, J. E., Kaczowka, A. M., and Burke, D. J. (2017). Spatial heterogeneity of plant–soil feedbacks increases per capita reproductive biomass of species at an establishment disadvantage. Oecologia 183, 1077–1086. doi: 10.1007/s00442-017-3828-1
Caldwell, M. M., Eissenstat, D. M., Richards, J. H., and Allen, M. F. (1985). Competition for phosphorus: differential uptake from dual-isotope-labeled soil interspaces between shrub and grass. Science 229, 384–386. doi: 10.1126/science.229.4711.384
Calflora (2019). Information on California Plants for Education, Research and Conservation. Berkeley, CA: The Calflora Database. Available online at: https://www.calflora.org/ (accessed September 18, 2019).
Callaway, R. M., Brooker, R. W., Choler, P., Kikvidze, Z., Lortie, C. J., Michalet, R., et al. (2002). Positive interactions among alpine plants increase with stress. Nature 417, 844–8. doi: 10.1038/nature00812
Callaway, R. M., Walker, L. R., Callaway, R. M., and Walker, L. R. (1997). Competition and facilitation: a synthetic approach to interactions in plant communities. Source Ecol. Ecol. 78, 1958–1965. doi: 10.1890/0012-9658(1997)078[1958:CAFASA]2.0.CO;2
Chen, I., Hill, J. K., Ohlemüller, R., Roy, D. B., and Thomas, C. D. (2011). Rapid range shifts of species of climate warming. Science 333, 1024–1026. doi: 10.1126/science.1206432
Chesson, P. (2000). Mechanisms of maintenance of species diversity. Annu. Rev. Ecol. Syst. 31, 343–366. doi: 10.1146/annurev.ecolsys.31.1.343
Chu, C., and Adler, P. B. (2014). When should plant population models include age structure? J. Ecol. 102, 531–543. doi: 10.1111/1365-2745.12212
Collins, C. G., Carey, C. J., Aronson, E. L., Kopp, C. W., and Diez, J. M. (2016). Direct and indirect effects of native range expansion on soil microbial community structure and function. J. Ecol. 104, 1271–1283. doi: 10.1111/1365-2745.12616
Collins, C. G., Stajich, J. E., Weber, S. E., Pombubpa, N., and Diez, J. M. (2018). Shrub range expansion alters diversity and distribution of soil fungal communities across an alpine elevation gradient. Mol. Ecol. 27, 2461–2476. doi: 10.1111/mec.14694
Dixon, J. M. (2000). Koeleria macrantha (Ledeb.) schultes (K. alpigena Domin, K. cristata (L.) Pers. pro parte, K. gracilis Pers., K. albescens auct. non DC.). J. Ecol. 88, 709–726. doi: 10.1046/j.1365-2745.2000.00483.x
Dostálek, T., Münzbergová, Z., Kladivová, A., and Macel, M. (2016). Plant–soil feedback in native vs. invasive populations of a range expanding plant. Plant Soil 399, 209–220. doi: 10.1007/s11104-015-2688-x
Dudenhöffer, J.-H., Ebeling, A., Klein, A.-M., and Wagg, C. (2017). Beyond biomass: soil feedbacks are transient over plant life-stages and alter fitness. J. Ecol. 106, 230–241. doi: 10.1111/1365-2745.12870
Ellner, S. P., Childs, D. Z., and Rees, M. (2016). Data-Driven Modelling of Structured Populations. Cham: Springer International Publishing
Eppinga, M. B., Rietkerk, M., Dekker, S. C., De Ruiter, P. C., Van der Putten, W. H., and Van der Putten, W. H. (2006). Accumulation of local pathogens: a new hypothesis to explain exotic plant invasions. Oikos 114, 168–176. doi: 10.1111/j.2006.0030-1299.14625.x
Fadrique, B., and Feeley, K. J. (2016). Commentary: novel competitors shape species' responses to climate change. Front. Ecol. Evol. 4:33. doi: 10.3389/fevo.2016.00033
Fowler, N. (1986). The role of competition in plant communities in arid and semiarid regions. Annu. Rev. Ecol. Syst. 17, 89–110. doi: 10.1146/annurev.es.17.110186.000513
Gallien, L., Zurell, D., and Zimmermann, N. E. (2018). Frequency and intensity of facilitation reveal opposing patterns along a stress gradient. Ecol. Evol. 8, 2171–2181. doi: 10.1002/ece3.3855
Hall, C. A. (1991). Natural History of the White-Inyo Range, Eastern California. University of California Press. Available online at: https://books.google.com/books?id=jMqaigYWKLoC
Hawkes, C. V., Belnap, J., D'Antonio, C., and Firestone, M. K. (2006). Arbuscular mycorrhizal assemblages in native plant roots change in the presence of invasive exotic grasses. Plant Soil 281, 369–380. doi: 10.1007/s11104-005-4826-3
Heinze, J., Sitte, M., Schindhelm, A., Wright, J., and Joshi, J. (2016). Plant-soil feedbacks: a comparative study on the relative importance of soil feedbacks in the greenhouse versus the field. Oecologia 181, 559–569. doi: 10.1007/s00442-016-3591-8
HilleRisLambers, J., Adler, P. B., Harpole, W. S., Levine, J. M., and Mayfield, M. M. (2012). Rethinking community assembly through the lens of coexistence theory. Annu. Rev. Ecol. Evol. Syst. 43, 227–248. doi: 10.1146/annurev-ecolsys-110411-160411
Hovatter, S., Blackwood, C. B., and Case, A. L. (2013). Conspecific plant-soil feedback scales with population size in Lobelia siphilitica (Lobeliaceae). Oecologia 173, 1295–1307. doi: 10.1007/s00442-013-2710-z
Jarou, Z. J. (2009). Measuring Leaf Area with Adobe Photoshop CS3. YouTube Tutor. Available online at: http://www.youtube.com/watch?v=E3O-V6WLw0g
Karban, R. (2007). Experimental clipping of sagebrush inhibits seed germination of neighbours. Ecol. Lett. 10, 791–797. doi: 10.1111/j.1461-0248.2007.01068.x
Kelsey, R. G., Stevenson, T. I., Scholl, J. P., Watson, T. J., and Shafizadeh, F. (1978). The chemical composition of the litter and soil in a community of Artemisia tridentata ssp. vaseyana. Biochem. Syst. Ecol. 6, 193–200. doi: 10.1016/0305-1978(78)90007-8
Klironomos, J. N. (2002). Feedback with soil biota contributes to plant rarity and invasiveness in communities. Nature 417, 67–70. doi: 10.1038/417067a
Koorem, K., Kostenko, O., Snoek, L. B., Weser, C., Ramirez, K. S., Wilschut, R. A., et al. (2018). Relatedness with plant species in native community influences ecological consequences of range expansions. Oikos 127, 981–990. doi: 10.1111/oik.04817
Kopp, C. W., and Cleland, E. E. (2014). Shifts in plant species elevational range limits and abundances observed over nearly five decades in a western North America mountain range. J. Veg. Sci. 25, 135–146. doi: 10.1111/jvs.12072
Kopp, C. W., and Cleland, E. E. (2015). A range-expanding shrub species alters plant phenological response to experimental warming. PLoS ONE 10:e0139029. doi: 10.1371/journal.pone.0139029
Kopp, C. W., and Cleland, E. E. (2018). Plant community response to Artemisia rothrockii (sagebrush) encroachment and removal along an arid elevational gradient. J. Veg. Sci. 29, 859–866. doi: 10.1111/jvs.12669
Körner, C. (2003). Alpine Plant Life: Functional Plant Ecology of High Mountain Ecosystems. Springer Berlin Heidelberg. Available online at: https://books.google.com/books?id=uFE2_-Hr6IoC
Körner, C., Stöcklin, J., Reuther-Thiébaud, L., and Pelaez-Riedl, S. (2008). Small differences in arrival time influence composition and productivity of plant communities. New Phytol. 177, 698–705. doi: 10.1111/j.1469-8137.2007.02287.x
Krapek, J., and Buma, B. (2018). Limited stand expansion by a long-lived conifer at a leading northern range edge, despite available habitat. J. Ecol. 106, 911–924. doi: 10.1111/1365-2745.12885
Kulmatiski, A. (2006). Exotic plants establish persistent communities. Plant Ecol. 187, 261–275. doi: 10.1007/s11258-006-9140-5
Kulmatiski, A., Beard, K. H., Stevens, J. R., and Cobbold, S. M. (2008). Plant-soil feedbacks: a meta-analytical review. Ecol. Lett. 11, 980–92. doi: 10.1111/j.1461-0248.2008.01209.x
Kulmatiski, A., and Kardol, P. (2008). “Getting plant — soil feedbacks out of the greenhouse : experimental and getting plant – soil feedbacks out of the greenhouse : experimental and conceptual approaches,” in Progress in Botany, eds U. Lüttge, W. Beyschlag, and J. Murata (Berlin; Heidelberg: Springer-Verlag), 449–472.
Lurgi, M., Lopez, B. C., and Montoya, J. M. (2012). Novel communities from climate change. Philos. Trans. R. Soc. B Biol. Sci. 367, 2913–2922. doi: 10.1098/rstb.2012.0238
Macek, P., Prieto, I., Macková, J., Pistón, N., and Pugnaire, F. I. (2016). Functional plant types drive plant interactions in a Mediterranean mountain range. Front. Plant Sci. 7:662. doi: 10.3389/fpls.2016.00662
Maestre, F. T., Callaway, R. M., Valladares, F., and Lortie, C. J. (2009). Refining the stress-gradient hypothesis for competition and facilitation in plant communities. J. Ecol. 97, 199–205. doi: 10.1111/j.1365-2745.2008.01476.x
Mangan, S. A., Schnitzer, S. A., Herre, E. A., Mack, K. M. L., Valencia, M. C., Sanchez, E. I., et al. (2010). Negative plant – soil feedback predicts tree-species relative abundance in a tropical forest. Nature 466, 752–755. doi: 10.1038/nature09273
Manrubia, M., Snoek, L. B., Weser, C., Veen, G. F., and van der Putten, W. H. (2019). Belowground consequences of intracontinental range-expanding plants and related natives in novel environments. Front. Microbiol. 10:505. doi: 10.3389/fmicb.2019.00505
Mccarthy-Neumann, S., and Ibáñez, I. (2012). Tree range expansion may be enhanced by escape from negative plant-soil feedbacks. Ecology 93, 2637–2649. doi: 10.1890/11-2281.1
Mehrabi, Z., Bell, T., and Lewis, O. T. (2015). Plant-soil feedbacks from 30-year family-specific soil cultures: Phylogeny, soil chemistry and plant life stage. Ecol. Evol. 5, 2333–2339. doi: 10.1002/ece3.1487
Metcalfe, D. B., Fisher, R. A., and Wardle, D. A. (2011). Plant communities as drivers of soil respiration: Pathways, mechanisms, and significance for global change. Biogeosciences 8, 2047–2061. doi: 10.5194/bg-8-2047-2011
Michelsen, A., Schmidt, I. K., Jonasson, S., Dighton, J., Jones, H. E., and Callaghan, T. V. (1995). Inhibition of growth, and effects on nutrient uptake of arctic graminoids by leaf extracts–allelopathy or resource competition between plants and microbes? Oecologia 103, 407–418. doi: 10.1007/BF00328678
Midgley, G. F., Thuiller, W., and Higgins, S. I. (2007). “Plant species migration as a key uncertainty in predicting future impacts of climate change on ecosystems: progress and challenges BT,” in Terrestrial Ecosystems in A Changing World, eds J. G. Canadell, D. E. Pataki, and L. F. Pitelka (Berlin; Heidelberg: Springer), 129–137. doi: 10.1007/978-3-540-32730-1_11
Mooney, H., Andre, G., and Wright, R. (1962). Alpine and subalpine vegetation patterns in the white mountains of California. Am. Midl. Nat. 68, 257–273. doi: 10.2307/2422731
Mooney, H., and Zavaleta, E. (2016). Ecosystems of California. University of California Press. Available online at: https://books.google.com/books?id=HkT_CgAAQBAJ
Myers-Smith, I. H., Forbes, B. C., Wilmking, M., Hallinger, M., Lantz, T., Blok, D., et al. (2011). Shrub expansion in tundra ecosystems: dynamics, impacts and research priorities. Environ. Res. Lett. 6:045509. doi: 10.1088/1748-9326/6/4/045509
Neuschulz, E. L., Merges, D., Bollmann, K., Gugerli, F., and Böhning-Gaese, K. (2018). Biotic interactions and seed deposition rather than abiotic factors determine recruitment at elevational range limits of an alpine tree. J. Ecol. 106, 948–959. doi: 10.1111/1365-2745.12818
Nilsson, M.-C., Högberg, P., Zackrisson, O., and Fengyou, W. (1993). Allelopathic effects by Empetrum hermaphroditum on development and nitrogen uptake by roots and mycorrhizae of Pinus silvestris. Can. J. Bot. 71, 620–628. doi: 10.1139/b93-071
Parmesan, C., Parmesan, C., Yohe, G., and Yohe, G. (2003). A globally coherent fingerprint of climate change impacts across natural systems. Nature 421, 37–42. doi: 10.1038/nature01286
Pernilla Brinkman, E., Van der Putten, W. H., Bakker, E.-J., and Verhoeven, K. J. F. (2010). Plant-soil feedback: experimental approaches, statistical analyses and ecological interpretations. J. Ecol. 98, 1063–1073. doi: 10.1111/j.1365-2745.2010.01695.x
Piao, T., Chun, J. H., Yang, H. M., and Cheon, K. (2014). Negative density dependence regulates two tree species at later life stage in a temperate forest. PLoS ONE 9:e0103344. doi: 10.1371/journal.pone.0103344
R Core Team (2015). R: A Language and Environment for Statistical Computing. Vienna: R Foundation for Statistical Computing.
Ramirez, K. S., Snoek, L. B., Koorem, K., Geisen, S., Bloem, L. J., ten Hooven, F., et al. (2019). Range-expansion effects on the belowground plant microbiome. Nat. Ecol. Evol. 3, 604–611. doi: 10.1038/s41559-019-0828-z
Revilla, T. A., Veen, G. F., Eppinga, M. B., and Weissing, F. J. (2013). Plant-soil feedbacks and the coexistence of competing plants. Theor. Ecol. 6, 99–113. doi: 10.1007/s12080-012-0163-3
Robertson, J. H. (1947). Responses of range grasses to different intensities of competition with sagebrush (Artemisia tridentata Nutt.). Ecology 28, 1–16. doi: 10.2307/1932913
Rout, M. E., and Callaway, R. M. (2012). Interactions between exotic invasive plants and soil microbes in the rhizosphere suggest that “everything is not everywhere.” Ann. Bot. 110, 213–222. doi: 10.1093/aob/mcs061
Rundel, P. W., Gibson, A. C., and Sharifi, M. R. (2005). Plant functional groups in alpine fellfield habitats of the White Mountains, California. Arctic Antarct. Alp. Res. 37, 358–365. doi: 10.1657/1523-0430(2005)037[0358:PFGIAF]2.0.CO;2
Ryel, R. J., Leffler, A. J., Peek, M. S., Ivans, C. Y., and Caldwell, M. M. (2004). Water conservation in Artemisia tridentata through redistribution of precipitation. Oecologia 141, 335–345. doi: 10.1007/s00442-003-1421-2
Sexton, J. P., McIntyre, P. J., Angert, A. L., and Rice, K. J. (2009). Evolution and ecology of species range limits. Annu. Rev. Ecol. Evol. Syst. 40, 415–436. doi: 10.1146/annurev.ecolsys.110308.120317
Suding, K. N., Stanley Harpole, W., Fukami, T., Kulmatiski, A., MacDougall, A. S., Stein, C., et al. (2013). Consequences of plant-soil feedbacks in invasion. J. Ecol. 101, 298–308. doi: 10.1111/1365-2745.12057
Tomiolo, S., and Ward, D. (2018). Species migrations and range shifts: a synthesis of causes and consequences. Perspect. Plant Ecol. Evol. Syst. 33, 62–77. doi: 10.1016/j.ppees.2018.06.001
Tylianakis, J. M., Didham, R. K., Bascompte, J., and Wardle, D. A. (2008). Global change and species interactions in terrestrial ecosystems. Ecol. Lett. 11, 1351–1363. doi: 10.1111/j.1461-0248.2008.01250.x
Valéry, L., Fritz, H., Lefeuvre, J.-C., and Simberloff, D. (2008). In search of a real definition of the biological invasion phenomenon itself. Biol. Invasions 10, 1345–1351. doi: 10.1007/s10530-007-9209-7
Van der Putten, W. H., Bardgett, R. D., Bever, J. D., Bezemer, T. M., Casper, B. B., Fukami, T., et al. (2013). Plant-soil feedbacks: the past, the present and future challenges. J. Ecol. 101, 265–276. doi: 10.1111/1365-2745.12054
van der Putten, W. H., Bradford, M. A., Pernilla Brinkman, E., van de Voorde, T. F. J., and Veen, G. F. (2016). Where, when and how plant–soil feedback matters in a changing world. Funct. Ecol. 30, 1109–1121. doi: 10.1111/1365-2435.12657
Wardle, D. a, Bardgett, R. D., Klironomos, J. N., Setälä, H., van der Putten, W. H., and Wall, D. H. (2004). Ecological linkages between aboveground and belowground biota. Science 304, 1629–1633. doi: 10.1126/science.1094875
Wardle, D. a, Nilsson, M. C., Gallet, C., and Zackrisson, O. (1998). An ecosystem-level perspective of allelopathy. Biol. Rev. 73, 305–319. doi: 10.1017/S0006323198005192
Wardle, D. A., Bardgett, R. D., Callaway, R. M., and Expansion, R. (2011). Terrestrial ecosystem responses to species gains and losses. Science 332, 1273–1277. doi: 10.1126/science.1197479
Weaver, T. W., and Klarich, D. (1977). Allelopathic effects of volatile substances from Artemisia tridentata Nutt. Am. Midl. Nat. 97, 508–512. doi: 10.2307/2425118
Welch, B. L. (2005). Big Sagebrush: A Sea Fragmented Into Lakes, Ponds, and Puddles. Gen. Tech Rep. RMRS-GTR-144. Fort Collins, CO: U.S. Department of Agriculture, Forest Service, Rocky Mountain Research Station, 210.
Weston, L. A., and Putnam, A. R. (1985). Inhibition of growth, nodulation, and nitrogen fixation of legumes by quackgrass. Crop Sci. 25, 561–565. doi: 10.2135/cropsci1985.0011183X002500030031x
Williams, R. J., Hallgren, S. W., Wilson, G. W. T., and Palmer, M. W. (2013). Juniperus virginiana encroachment into upland oak forests alters arbuscular mycorrhizal abundance and litter chemistry. Appl. Soil Ecol. 65, 23–30. doi: 10.1016/j.apsoil.2012.12.020
Wilson, T. B., Webb, R. H., and Thompson, T. L. (2001). Mechanisms of Range Expansion and Removal of Mesquite in Desert Grasslands of the Southwestern United States. Gen. Tech. Ogden, UT: U.S. Department of Agriculture, Forest Service, Rocky Mountain Research Station, 1–24.
Keywords: plant-soil (belowground) feedbacks, range expansion, alpine, global change, demography, population dynamics, woody plant encroachment, integral projection model (IPM)
Citation: Collins CG, Bohner TF and Diez JM (2019) Plant-Soil Feedbacks and Facilitation Influence the Demography of Herbaceous Alpine Species in Response to Woody Plant Range Expansion. Front. Ecol. Evol. 7:417. doi: 10.3389/fevo.2019.00417
Received: 20 July 2019; Accepted: 17 October 2019;
Published: 08 November 2019.
Edited by:
Johannes Heinze, University of Potsdam, GermanyReviewed by:
Stephanie N. Kivlin, The University of Tennessee, United StatesJon Bates, Oregon State University, United States
Conrad Schittko, University of Potsdam, Germany
Copyright © 2019 Collins, Bohner and Diez. This is an open-access article distributed under the terms of the Creative Commons Attribution License (CC BY). The use, distribution or reproduction in other forums is permitted, provided the original author(s) and the copyright owner(s) are credited and that the original publication in this journal is cited, in accordance with accepted academic practice. No use, distribution or reproduction is permitted which does not comply with these terms.
*Correspondence: Courtney G. Collins, ccoll009@ucr.edu