Positive Effects on Alfalfa Productivity and Soil Nutrient Status in Coastal Wetlands Driven by Biochar and Microorganisms Mixtures
- Shandong Key Laboratory of Eco-Environmental Science for the Yellow River Delta, Binzhou University, Binzhou, China
Biochar application in reclaiming degraded soils and improving plant productivity has been recognized as a promising technology. Yet, the impacts of biochar and mixtures with compound effective microorganisms (CEM) on alfalfa growth and soil quality in coastal wetlands are poorly understood. A greenhouse experiment was set to systematically reveal the impacts of biochar and biochar combined with CEM on alfalfa growth traits, nutrient uptake, biomass, soil quality, and enzyme activities. Eight treatments were included: (1) control (CK−CEM), (2) 10-g/kg biochar (B10−CEM); (3) 20-g/kg biochar (B20−CEM); (4) 30-g/kg biochar (B30−CEM), (5) CEM without biochar (CK + CEM); (6) 10-g/kg biochar with CEM (B10 + CEM), (7) 20-g/kg biochar with CEM (B20 + CEM), (8) 30-g/kg biochar with CEM (B30 + CEM). The utilization of biochar promoted seed germination, height, and tissue nutrient contents of alfalfa, and the combined biochar with CEM showed greater effects. Alfalfa biomass showed the maximum value in the B20 + CEM treatment, and the biomass of root, shoot, leaf in the B20 + CEM treatment increased by 200, 117.3, 144.6%, respectively, relative to the CK−CEM treatment. Alfalfa yield in the CK + CEM, B10 + CEM, B20 + CEM, B30 + CEM treatments was 71.91, 84.11, 138.5, and 120.5% higher than those in the CK−CEM treatment. The use of biochar and CEM decreased soil salinity and elevated soil nutrient content effectively. Biochar elevated soil organic carbon (SOC) and microbial biomass carbon (MBC), NH4+, NO3–, and enzymatic activities, and the positive impacts of biochar combined with CEM were additive. The combined addition of 20-g/kg biochar with CEM showed the pronounced improvement effects on improving soil fertility and nutrient availability as well as soil enzyme activities. Path analysis indicated that the application of biochar mixture with CEM promoted alfalfa biomass by regulating plant nutrient uptake, soil quality (soil nitrogen, SOC, MBC, NH4+, NO3–), and soil enzymatic activities (sucrase, urease, and alkaline phosphatases). Thus, incorporation of suitable biochar and CEM can serve as an effective measure to promote alfalfa productivity and restore coastal wetlands soils.
HIGHLIGHTS
- Biochar addition decreased soil salinity and elevated soil organic carbon, NH4+ and sucrase activity effectively.
- Combined use of 20 g/kg biochar with microorganisms mixtures performed well in improving alfalfa growth.
- Alfalfa total biomass increased depend on the rise of plant potassium uptake, soil organic carbon, NH4+, and NO3–.
- An appropriate dosage of biochar combined with microorganisms mixtures is effective to ameliorate coastal wetlands.
Introduction
Soil salinization with potentially nutrient deficiency has become a global issue concerning the sustainable development of food security and human livelihoods (Saifullah et al., 2018; Hassani et al., 2020). As a typical part of saline-alkali soil, coastal wetlands are considered as one of the most productive ecosystems on Earth and contributors to valuable service (Kirwan and Megonigal, 2013; Zhao et al., 2018). Recently, anthropogenic activities and seawater encroachment have resulted in high salinization, shallow groundwater, and soil function deterioration, which would cause soil degradation and threaten crop productivity in coastal wetlands (Stagg et al., 2017; Liu et al., 2018). Severe soil depletion, low nutrient deficiency, and poor vegetation coverage occur on coastal wetlands, aggravating plant productivity and unsustainable land use (Huang et al., 2012; Li et al., 2021). Consequently, the restoration of coastal wetlands is particularly critical, and soil amendments with the advantages of being effective, sustainable, and environmentally friendly are urgently needed for improving soil health and rehabilitating vegetation (Luo et al., 2017).
Biochar is a carbonous coproduct generated by pyrolysis organic biomass that has received growing attentions in pieces of research of ecological issues, soil restoration, and soil carbon sequestration (Lehmann, 2007; Chávez-García and Siebe, 2019; Cooper et al., 2020). Biochar has been advocated as a suitable conditioner that can remediate salt-affected soils by reducing salinity stress and promoting soil nutrient status (Kim et al., 2016; Ali et al., 2017; Gunarathne et al., 2020). Biochar has been proved to increase soil aggregate stability and water retention (Palansooriya et al., 2019; Caban et al., 2020), stimulate nutrient utilization efficiency (Biederman and Harpole, 2013; Chen et al., 2018), boost soil microbial proliferation and activities (Pokharel et al., 2020), and improve plant nutrient uptake and productivity (Lashari et al., 2015). Biochar can diminish sodium (Na+) absorption by plants and mitigate salt stress to plant growth and physiology in salt-affected soils (Akhtar et al., 2015; Saifullah et al., 2018). Furthermore, biochar application can stimulate the plant nutrients absorption ability via promoting biochar-root interactions and increasing the efficiency of nutrient absorption by the roots (Olmo et al., 2016; Jeffery et al., 2017). However, inappropriate use of biochar to salt-affected soils may increase salinity and alkalinity and inhibit soil nutrient supply, which have an adverse impact on plant performance (Sigua et al., 2016; Luo et al., 2017). Thus, the effect of biochar on soil and plant quality under salinity conditions depends on soil type, plant feature, biochar feedstock type, biochar pyrolysis terms, and biochar addition rate.
Recently, compound effective microorganisms (CEM), a wide range of beneficial and non-pathogenic microorganisms, has been adopted as microbial fertilizers to improve soil quality (Talaat et al., 2015). The benefits of CEM have been highlighted in salt-affects soils for diminishing the detrimental impact of saline stress, enhancing beneficial microbial activities and soil nutrient cycling, accelerating decay of soil organic matter, which are favorable for plant productivity (Hu and Qi, 2013; Olle and Williams, 2013; Talaat, 2019). The amendment of biochar within microorganism mixtures showed encouraging results in promoting plant yield under saline conditions than single addition of biochar or other soil amendments (Akhtar et al., 2015; Talaat et al., 2015; Wang et al., 2021). For example, El-Mageed et al. (2020) found that the combined use of biochar and CEM maximized pepper growth and production more by improving favorable soil characteristics, photosynthetic availability, and nutritional status than biochar addition alone. Wang et al. (2021) reported that biochar mixture with plant growth-promoting rhizobacteria improved microbial diversity, enzyme activity, and nitrogen availability from tomato soil. In general, biochar mixture with microorganisms provides a favorable environment for microorganisms and produces remarkable effects on soil quality and plant growth. Thus, the combination of biochar with microorganisms is considered as a promising and effective measure for improving plant yield and remediating nutrient-poor soil.
Adding soil conditioner is an effective and eco-friendly approach to remediate soil degradation and improve salinity soil function of coastal wetlands (Sun et al., 2016). The additive positive effects on soil nutrient status and plant growth have been proved in salt-affected soils amended with the mixture of biochar and other fertilizer or microorganisms (Luo et al., 2017; Saifullah et al., 2018). Furthermore, planting halophytes in coastal wetlands is a bioremediation strategy to restore the degraded soil and improve the efficiency of land usage (Cai et al., 2021). Alfalfa is a preferred halophyte to adapt to saline environment and develop grass and livestock breeding in salt-affected regions. Alfalfa is a considerable important forage crop and has many prominent advantages, including high yield, nutritional quality, drought tolerance, and adaptation to infertile habitat (Cao et al., 2012; Zhang et al., 2019). In addition, combinations of biochar with microorganism mixtures or other amendments applied to soil showed effective effects on alfalfa yield (Liu et al., 2020; Raklami et al., 2021). However, previous reviews on restoring coastal wetlands soils have focused on sole biochar addition or combined with other organic materials (Kim et al., 2016; Luo et al., 2017). It is still unclear if the use of biochar with microorganism mixtures affects soil nutrient status and plant yield in coastal wetlands. Here, we hypothesize that biochar combined with CEM would improve soil nutrient availability, enhance soil enzyme activities, and promote plant nutrient uptake and alfalfa growth. The main objectives of this study were: (1) to clarify the changes in plant growth traits, plant nutrient content, and soil nutrient content, following biochar and CEM inputs, and (2) to evaluate the potential mechanisms affecting alfalfa growth in coastal wetlands.
Materials and Methods
Soil Sample and Experiment Preparation
The soil was randomly sampled from topsoil samples (0–20 cm) of the coastal wetlands in the Nature Reserve of the Yellow River Delta (37°45′N, 118°59′E) in the Shandong Province, China. Soil samples were transferred to a laboratory, and stones and roots were removed. The soil samples were air-dried and passed through a 2-mm sieve; then, all the samples were homogenized thoroughly. The soil had a salt content of 0.6%, pH of 7.49, soil organic matter of 9.23 g/kg, total nitrogen (TN) of 0.42 g/kg, available phosphate (AP) of 3.9 mg/kg.
Phragmites australis was chosen as a raw material for biochar mainly due to well-developed aerenchym and high carbon fixation capacity, which is suitable for the requirement of biochar (Liang et al., 2019). Biochar was produced from Phragmites australis shoot by combustion anaerobically for 2 h at 400°C in a muffle furnace (Leibo Terry Equipment Co., Ltd., Tianjin, China). The pyrolysis temperature of 400°C was selected due to its highest yield and relatively stable properties. The biochar sample was passed through a 2-mm sieve and homogenized completely before addition. Biochar contains 40.5% yield, 58.34% total carbon (TC), 1.18% TN, 4.11% hydrogen (H), 36.75% oxygen (O), 14.19 C/H, 0.70 (O + H)/C, an 8.65 m2/g specific surface area, 1.83-nm average pore width, with a pH of 7.65. CEM contains a series of composite strains, and the main strains are photosynthetic bacteria, lactic acid bacteria, fermenting fungi, yeast, and actinomycetes. The quantity of colony forming units (CFU) units in each strain exceeded 104/ml.
Experiment Design and Measurements
This pot experiment comprised eight treatments in five replicates. These treatments were: (1) soil alone (CK−CEM); (2) 10-g/kg biochar (B10−CEM, w/w); (3) 20-g/kg biochar (B20−CEM); (4) 30-g/kg biochar (B30−CEM); (5) CEM without biochar (CK + CEM); (6) 10-g/kg biochar with CEM (B10 + CEM); (7) 20-g/kg biochar with CEM (B20 + CEM); (8) 30-g/kg biochar with CEM (B30 + CEM). Soils samples with or without biochar addition were weighed (450-g dry mass) into a series of plastic pots (15 cm × 13 cm × 10 cm) with a hole and a tray in the bottom. Each pot was filled with deionized water to adjust the soil moisture to 60–70% of its water-holding capacity during the experiment. The CEM solution was diluted with deionized water to a concentration of 0.5 and then added to each pot. This diluted concentration was chosen because the alfalfa seed germination rate showed the highest in the pre-experiment. The dosage of the CEM was 4 ml/pot from the CEM solution in the treatments with CEM at the age of irrigation, while the treatments without CEM were added with double distilled water.
This experiment was conducted in a greenhouse. Alfalfa seeds were surface sterilized with 10% H2O2 for 0.5 h and rinsed. Fifteen alfalfa seeds were sown and then thinned to five plants per pot after short-term growth (3- to 5-cm height aboveground). The alfalfa grew in May 2019 and harvested in September 2019. The alfalfa was watered daily, and weeds in each pot were eradicated to ensure the growth of alfalfa. The number of seed germination was counted after the seeds were germinated. The height of alfalfa was measured in each plastic pot before harvesting. After harvesting, alfalfas aboveground parts and roots were rinsed, wiped with absorbent paper, and oven-dried at 70°C for 48 h. The soil sample in each pot was collected, mixed, and stored at 4°C for the measurement of soil property.
Soil pH was measured by deionized water at a 1:5 ratio (soil/water) with a pH meter, and soil salt content was measured using the gravimetric method. An elemental analyzer was used to analyze soil TC and TN. The Olsen method was conducted to measure AP, and the NH4Ac extraction plus flame photometry method was used to analyze available potassium (AK). Soil organic carbon (SOC) was analyzed by the elemental analyzer after being treated with hydrochloric acid (HCl, 1 mol/L) for removal of inorganic carbon. The chloroform fumigation-extraction method was adopted to determine microbial biomass carbon (MBC; Vance et al., 1987). After the soil samples were extracted with potassium chloride (KCl) solution, an AA3 automated flow injection analysis (Auto Analyzer III, Seal, Germany) was used to determine soil NH4+ and NO3–. Soil sucrase, urease, and alkaline phosphatase activities were measured based on these methods and procedures (Tan et al., 2014). An elemental analyzer was used to analyze plant TN, the molybdenum-antimony colorimetry method was used to test plant total phosphorus (TP), and the flame photometer was used to determine plant total potassium (TK).
Statistical Analysis
All statistical analyses were conducted via SPSS 19.0. The influences of biochar, CEM, and their interactions on alfalfa seed germination, height, biomass, nutrient contents, and soil fertility change were tested by two-way ANOVA. The significance of differences in alfalfa growth parameters, soil properties, and soil enzymatic activities was analyzed via one-way ANOVA. Pearson correlation analysis was used to explore the relationship of plant parameters with soil quality. Path analysis was performed to ascertain hypothetical pathways by which soil quality and plant nutrient might explain plant biomass. Path analysis was conducted using the AMOS 21.0 version (IBM SPSS Amos 21.0 software). The data were fit to the model using maximum likelihood estimation. We selected the χ2 test and the root mean square error of approximation to assess the fitness of the model.
Results
Plant Growth, Biomass, and Nutrient Status
Compared with the CK−CEM treatment, seed germination significantly increased by 50–81.25% and 56.25–118.7% under −CEM treatments and +CEM treatments, respectively (p < 0.05, Figure 1A). The application of biochar and CEM increased alfalfa height among different treatments, and the highest plant height in the B20 + CEM treatment reached to 34.33 ± 2.96 cm (p < 0.05, Figure 1B). The influences of biochar and CEM on plant biomass (root, shoot, leaf, aboveground, and total) varied with a biochar level (Figure 2). Alfalfa biomass enhanced with the increase of the biochar level in −CEM treatments, and the alfalfa biomass elevated and then declined with the increase of the biochar level in +CEM treatments. The B20 + CEM treatment showed the highest biomass among all the treatments, and the biomass of root, shoot, leaf, aboveground, and total was significantly higher than the CK−CEM treatment by 200, 117.3, 144.6, 123.2, and 138.5%, respectively (p < 0.05).
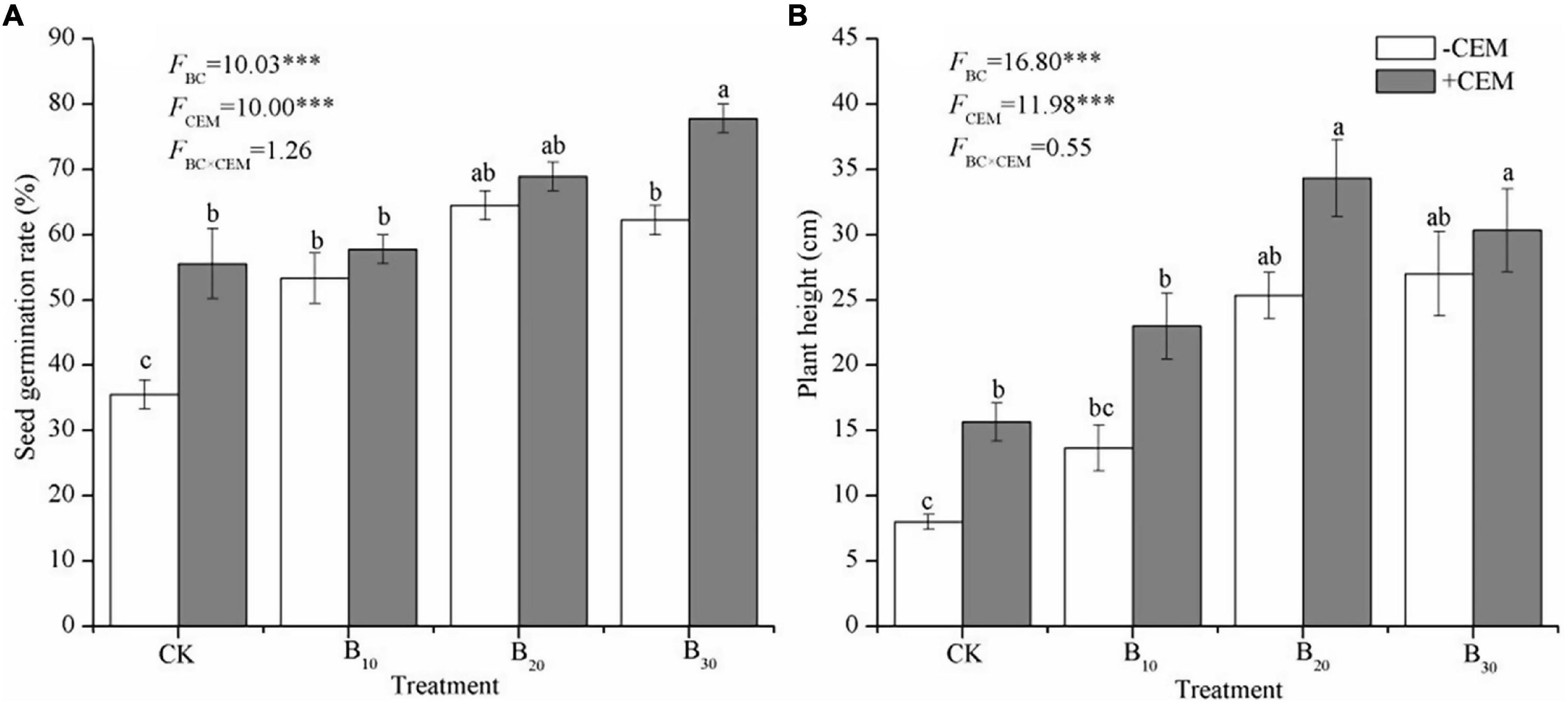
Figure 1. Alfalfa seed germination rate (A) and alfalfa plant height (B) under different treatments. Bars and error bars show means ± SE (n = 5). Different lowercase letters referred to significant difference between treatments (p < 0.05). The FBC, FCEM, and FBC × CEM are F and the degree of freedom of two-way ANOVAs. ***p < 0.001.
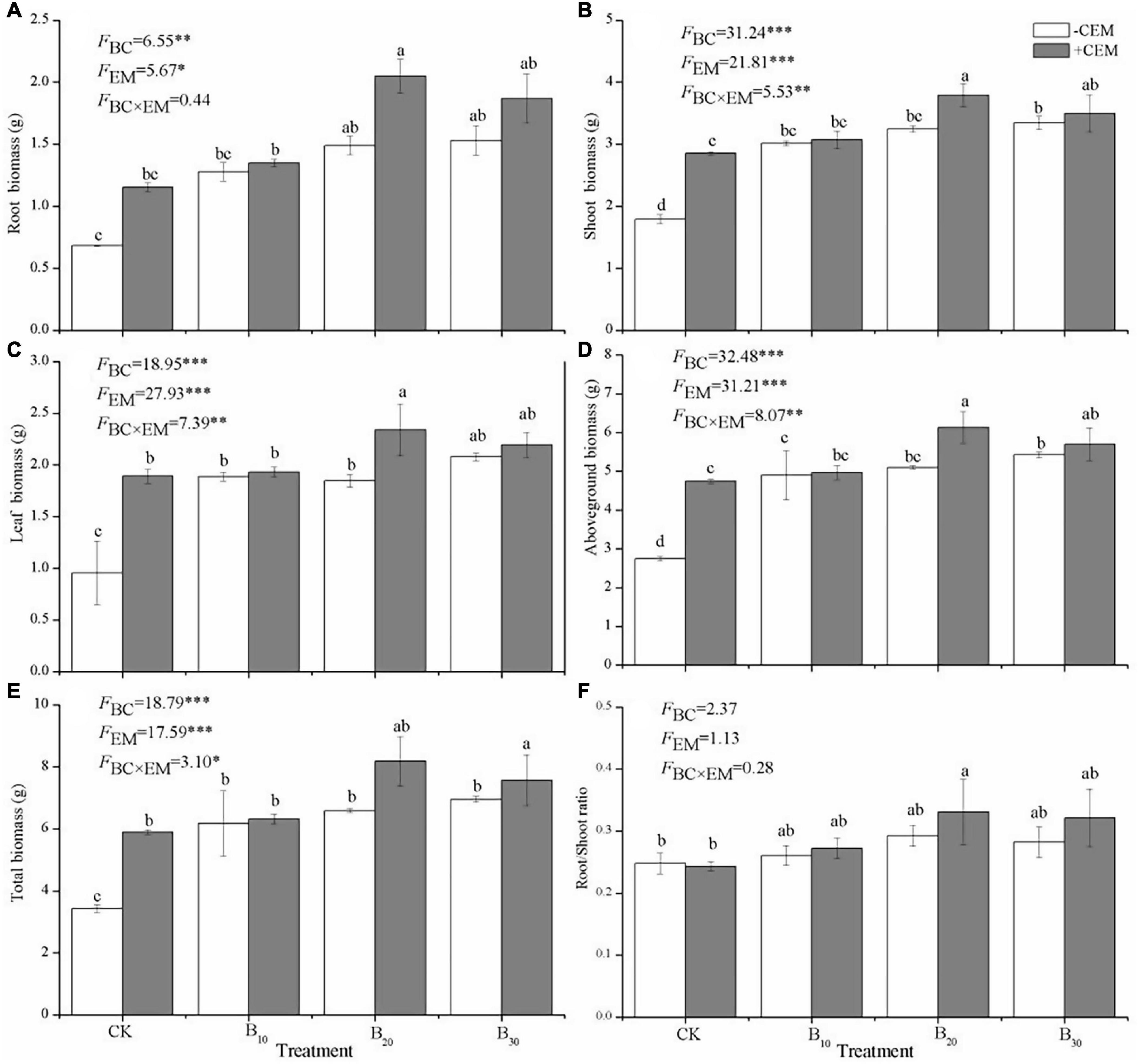
Figure 2. Root biomass (A), shoot biomass (B), leaf biomass (C), aboveground biomass (D), total biomass (E), and root/shoot ratio (F) under different treatments. Different lowercase letters referred to significant difference between treatments (p < 0.05). The FBC, FCEM, and FBC × CEM are F and the degree of freedom of two-way ANOVAs. ***p < 0.001, **p < 0.01, *p < 0.05.
The contents of root TN, shoot TN, and leaf TN in other treatments were 60.24–226.6%, 46.46–143.5%, and 33.98–93.73% higher, respectively, than those in the CK−CEM treatment (p < 0.05, Figures 3A–C). Single biochar amendment or mixture with CEM significantly increased root TP concentrations by 57.58–155.3%, shoot TP concentrations by 36.89–117.6%, leaf TP concentrations by 31.81–108.8%, root TK concentrations by 41.28–138.9%, shoot TK concentrations by 64.55–209.8%, and leaf TK concentrations by 20.85–98.32%, respectively (p < 0.05, Figures 3D–I), compared with the CK−CEM treatment. Biochar elevated the contents of plant TN, TP, and TK in the −CEM treatments, while biochar increased the concentrations of plant TN, TP, and TK first and then decreased in +CEM treatments. The maximum TN, TP, and TK contents in plant tissues were detected in the B20 + CEM treatment. The interactive application of biochar and CEM led to more improved performance on alfalfa growth and nutrient absorption than the individual addition of either biochar or CEM.
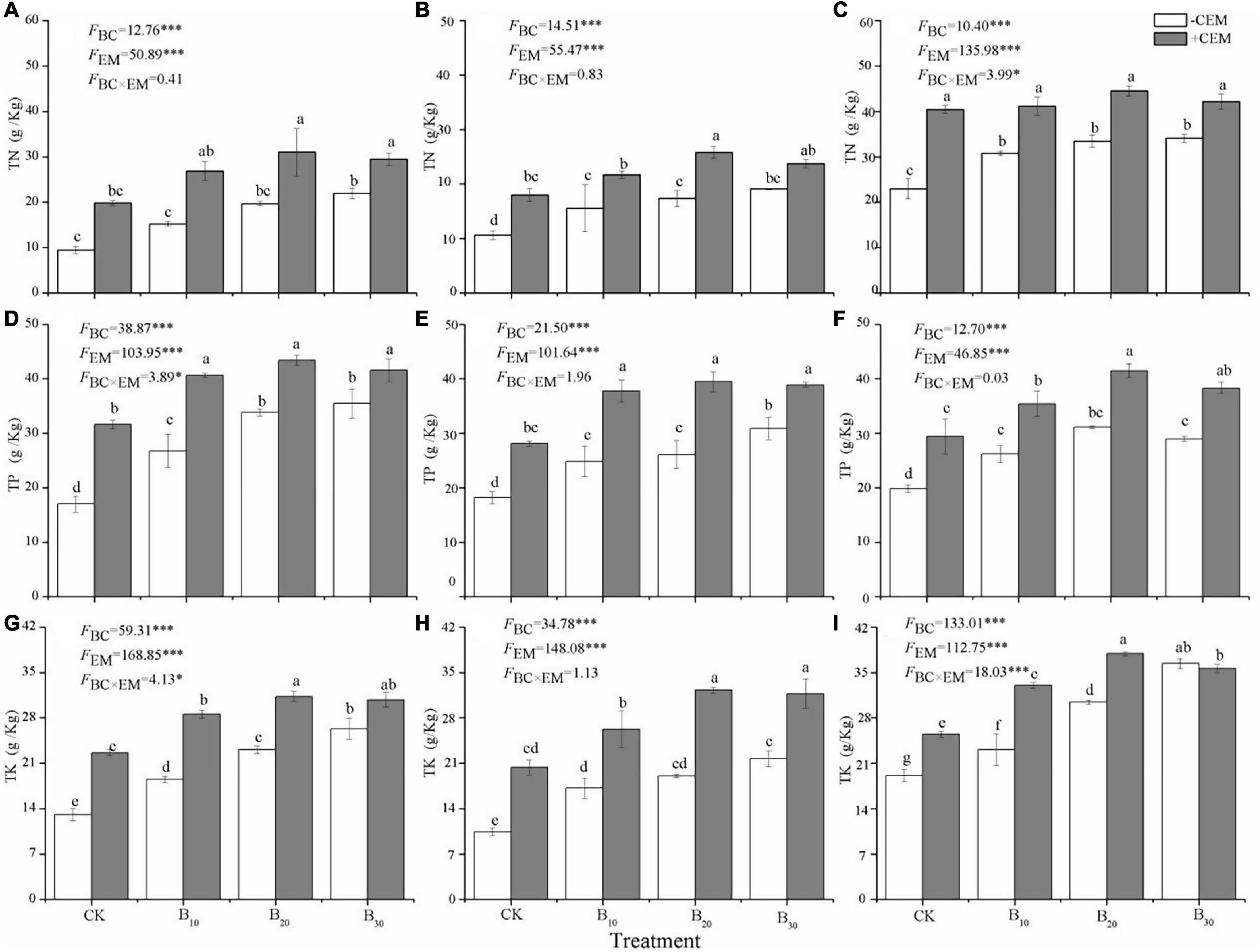
Figure 3. Root TN (A), shoot TN (B), leaf TN (C), root TP (D), shoot TP (E), leaf TP (F), root TK (G), shoot TK (H), and leaf TK (I) under different treatments. TN, total nitrogen; TP, total phosphorus; TK, total potassium. Different lowercase letters referred to significant difference between treatments (p < 0.05). The FBC, FCEM, and FBC × CEM are F and the degree of freedom of two-way ANOVAs. ***p < 0.001 and *p < 0.05.
Soil Properties and Fertility
Biochar addition with or without CEM had no significant influence on soil pH (Table 1). The soil salinity in the B10 + CEM, B20 + CEM, and B30 + CEM treatments was significantly reduced by 38.76, 44.14, and 45.48% than the CK−CEM treatment (p < 0.05, Table 1). The use of biochar and CEM improved soil TC, and the highest value in the B20 + CEM treatment was 50.13% higher than the CK−CEM treatment. Compared with that of CK−CEM treatment, soil TN treated with biochar application alone increased by 17.89–70.36%, while soil TN treated with biochar combined with CEM enhanced by 60.12–114.7% (p < 0.05, Table 1). Soil AP and AK in the B20 + CEM treatment significantly elevated by 44.06 and 18.35% (p < 0.05), respectively, compared with the CK−CEM treatment. Biochar amendment alone enhanced soil fertility, whereas the use of 30-g/kg biochar combined with CEM exerted a negative influence on soil fertility.
Soil Nutrient Availability and Enzyme Activities
Compared with the CK−CEM treatment, the B10−CEM, B20−CEM, B30−CEM, B10 + CEM, B20 + CEM, B30 + CEM treatments enhanced SOC by 15.58, 65.07, 135.9, 12.65, 34.95, 157.94, and 140.3%, respectively (Figure 4A). Likewise, biochar addition alone significantly enhanced soil MBC by 41.44–86.50%, while biochar mixture with CEM enhanced soil MBC by 43.31–97.33% (p < 0.05, Figure 4B). Under the −CEM and +CEM treatments, soil NH4+ and NO3– were significantly increased by 44.95–198.1% and 36.42–104.7% relative to the CK−CEM treatment, and the enhanced effects on soil NH4+ were greater than soil NO3– (p < 0.05, Figures 4C,D). Biochar, CEM, and their interaction enhanced soil sucrase activity by 23.64–125.7%, urease activity by 19.35–74.45%, and alkaline phosphatase activity by 13.86–113.8%, as compared to the CK−CEM treatment, respectively (Figure 5). Notably, the increase amplitude of soil sucrase activity was more pronounced than alkaline phosphatase and urease activity, and the B20 + CEM treatment emerged the highest value among all the treatments.
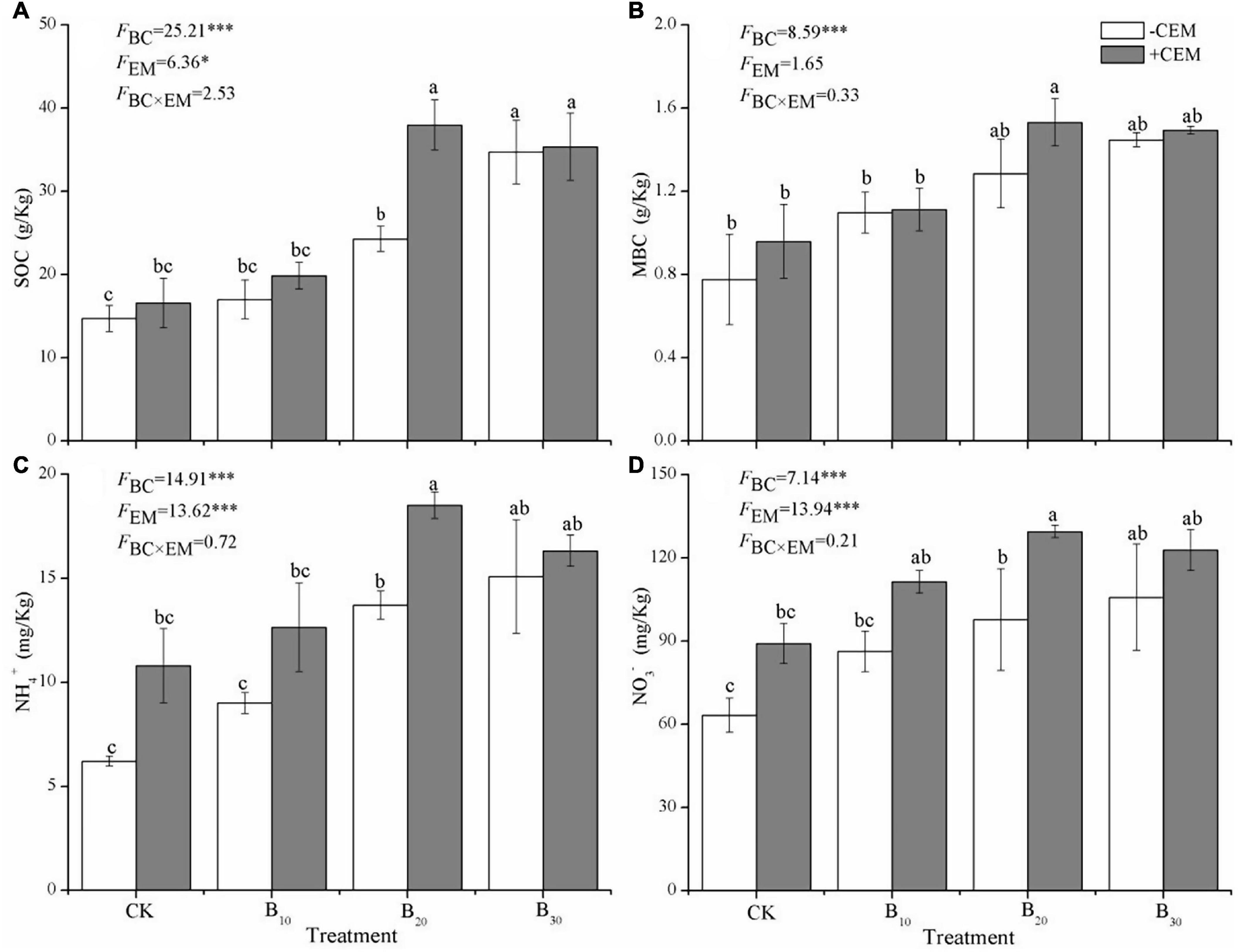
Figure 4. SOC (A), MBC (B), NH4+ (C), and NO3– (D) under different treatments. SOC, soil organic carbon; MBC, microbial biomass carbon; NH4+, ammonium nitrogen; NO3–, nitrate nitrogen. Different lowercase letters referred to significant difference between treatments (p < 0.05). The FBC, FCEM, and FBC × CEM are F and the degree of freedom of two-way ANOVAs. ***p < 0.001 and *p < 0.05.
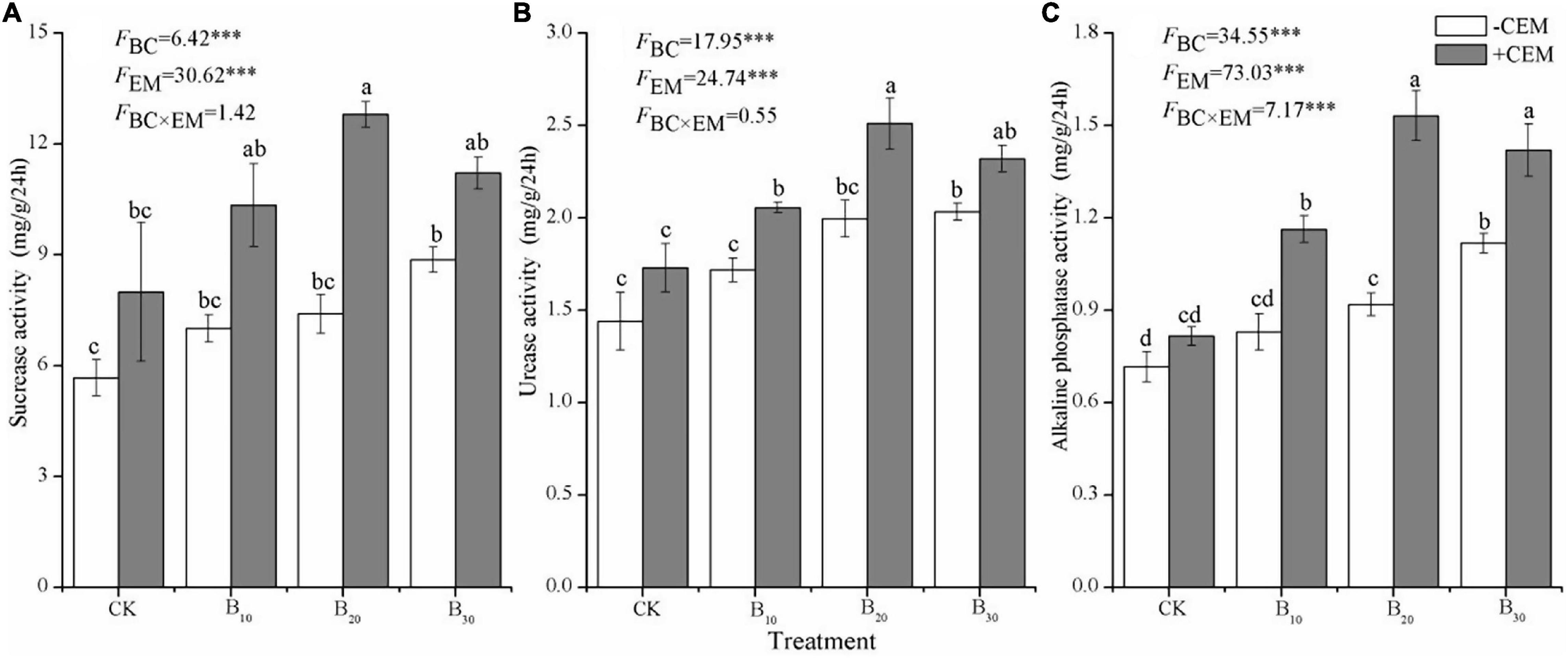
Figure 5. Soil sucrase activity (A), soil urease activity (B), and soil alkaline phosphatase activity (C) under different treatments. Different lowercase letters referred to significant difference between treatments (p < 0.05). The FBC, FCEM, and FBC × CEM are F and the degree of freedom of two-way ANOVAs. ***p < 0.001.
Linkages Between Soil Nutrient Status and Plant Biomass
Significant positive correlations between plant biomass with nutrient contents of plant tissue, soil TN, SOC, MBC, NH4+, and NO3– were detected in this experiment (Table 2). The various pathways of biochar and CEM addition on soil nutrient and further on alfalfa biomass were quantified by SEM. The root mass, shoot mass, leaf mass, and total mass were explained by 54, 62, 70, and 72% using the path analysis (Figure 6). The amendment of biochar and CEM enhanced the contents of soil enzyme activities, TN, SOC, MBC, NH4+, and NO3–, and then positively promoted plant tissue nutrient absorption, and hence improved plant biomass. Soil TN, SOC, MBC, NH4+, and NO3– positively affected root TN and TK, and subsequently, root TN and TK strongly impacted root mass (Figure 6A). Likewise, soil NH4+, NO3–, and SOC were positively correlated with shoot TK, and hence, shoot TK mainly affected shoot mass (Figure 6B). Soil TN, MBC, NH4+, and NO3– positively influenced leaf TN, and SOC, NH4+, and NO3– positively affected leaf TK, and thus, leaf mass was positively driven by leaf TN and TK (Figure 6C). With regard to total biomass, soil NH4+, NO3–, and SOC positively affected total TK, and hence, total mass was explained by total TK (Figure 6D).
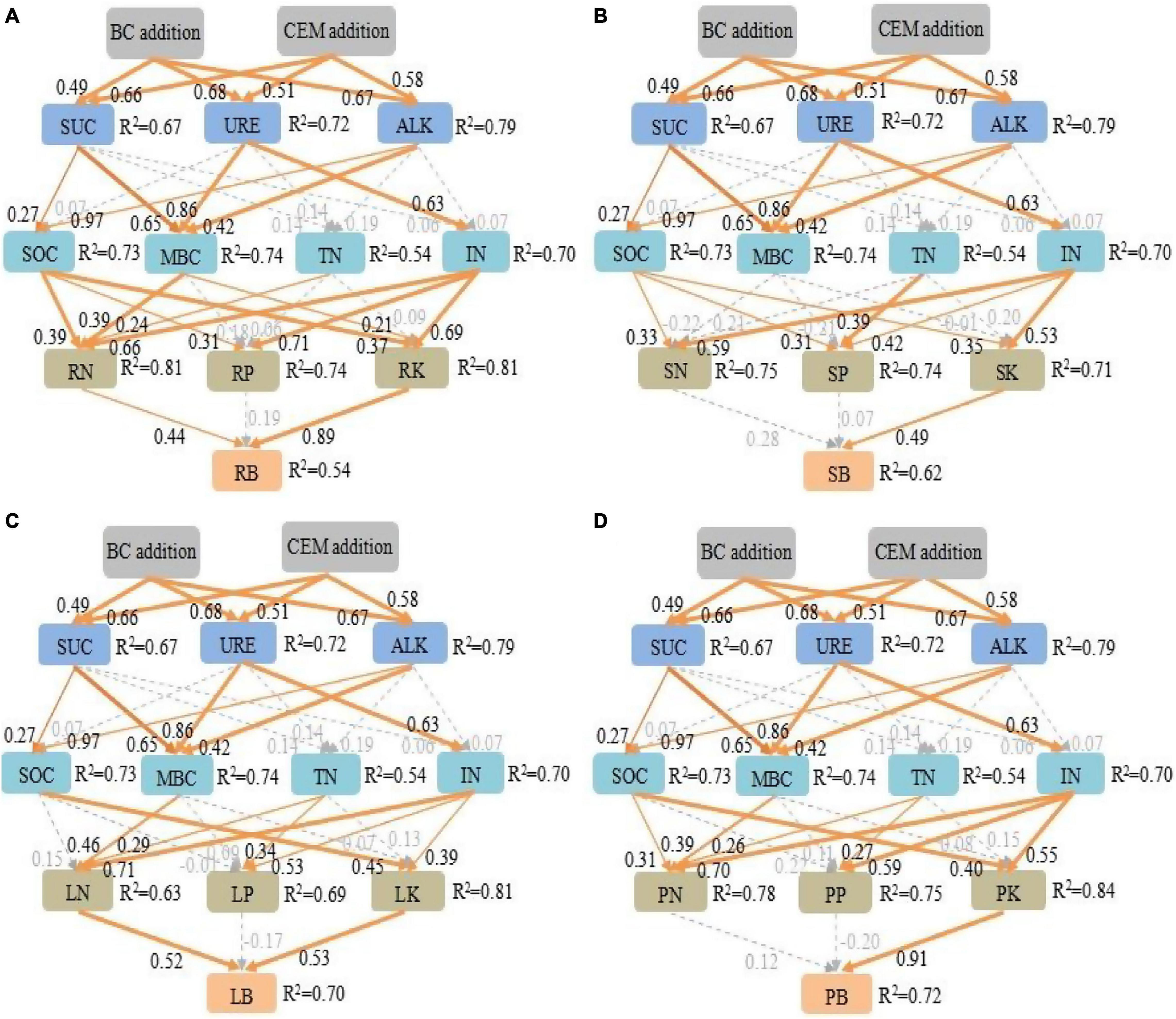
Figure 6. The structure equation model investigates the plausible pathways by which biochar addition and CEM addition affected root biomass (A), shoot biomass (B), leaf biomass (C), and plant total biomass (D). Goodness-of-fit statistics for the model: root biomass: χ2 = 118, df = 45, p < 0.05, root mean square error of approximation (RMSEA) = 0.26. Shoot biomass: χ2 = 127, df = 45, p < 0.05, shoot mean square error of approximation (RMSEA) = 0.28. Leaf biomass: χ2 = 113.6, df = 45, p < 0.05, leaf mean square error of approximation (RMSEA) = 0.25. Plant total biomass: χ2 = 153.9, df = 45, p < 0.05, total mean square error of approximation (RMSEA) = 0.32. Orange solid arrows from thick to thin indicate positive pathways (p < 0.001, p < 0.01, and p < 0.05) and light dashed gray arrows indicate non-significant relationships (p > 0.05). The numbers next to arrows are standardized path coefficients, and the arrow width represents the strength of the relationship. The proportion of the variation (R2) values occurs aside each response variable in the model. SUC, sucrase activity; URE, urease activity; ALK, alkaline phosphatase activity; SOC, soil organic carbon; MBC, microbial biomass carbon; TN, total nitrogen; IN, NH4+ and NO3–; RN, root total nitrogen; RP, root total phosphorus; RK, root total potassium; SN, shoot total nitrogen; SP, shoot total phosphorus; SK, shoot total potassium; LN, leaf total nitrogen; LP, leaf total phosphorus; LK, leaf total potassium; PN, plant total nitrogen; PP, plant total phosphorus; PK, plant total potassium; RB, root biomass; SB, shoot biomass; LB, leaf biomass; PB, plant total biomass.
Discussion
Promotion of Alfalfa Growth and Nutrient Absorption
In accordance with our hypothesis, appropriate application of biochar and CEM in coastal wetland soils resulted in an increase in alfalfa seed germination, height and biomass, indicating that improved alfalfa growth was regulated by many benefits from the presence of biochar (Zhang et al., 2019; Liu et al., 2020). These results were similar to previous studies, which documented that biochar promoted plant growth, yield, and soil quality in salinity soils (Kim et al., 2016; Saifullah et al., 2018). The improved soil physicochemical and biological properties and elevated soil nutrient induced by biochar may be responsible for these results, which can provide nutrient supply with ongoing alfalfa growth (Agegnehu et al., 2017; Ali et al., 2017; Mehmood et al., 2020). As revealed by the path analysis, the improvement of plant biomass may be due to the stimulated nutrient cycling of alfalfa caused by the enhanced soil quality and enzyme activities. These following factors dominated the promotion of biochar on alfalfa growth: (i) improved soil TN, NH4+, NO3–, SOC, MBC, soil sucrase, urease, and alkaline phosphatase activities (El-Naggar et al., 2019); (ii) stimulated plant TN, TP, and TK concentration (Agegnehu et al., 2017); (iii) decreased soil salinity stress to alfalfa (Lashari et al., 2015).
The promotion impacts of biochar mixture with CEM on alfalfa growth were stronger than sole biochar addition, implying that biochar combined with CEM can have a stronger cooperative effect. These profound lifting effects could be ascribed to the increased beneficial microorganism activity induced by CEM, thus facilitating alfalfa growth through accelerating decomposition of soil organic matter (Talaat, 2019). Moreover, the interior of biochar could offer suitable habitats for the survival of microorganisms, leading to boosted microbial activities and nutrient release. The combined use of biochar and CEM can further promote alfalfa growth through alleviating salt stress and increasing photosynthetic capacity in salted-affected soils (Akhtar et al., 2015; El-Mageed et al., 2020). However, the CEM + B20 treatment accelerated alfalfa growth, while the CEM + B30 treatment inhibited alfalfa growth. Therefore, the combined use of biochar with CEM should be kept at an appropriate level, which may generate greater noticeable effects.
The amendment of biochar and CEM caused a significant increase in concentrations of alfalfa tissue nutrient (TN, TP, and TK), identifying the positive response of biochar in contributing to plant nutrient uptake as proved by several findings (Biederman and Harpole, 2013; Sigua et al., 2016). Improved soil properties, soil nutrient availability, and plant photosynthetic parameter after biochar is applied may contribute to the boosted plant nutrient uptake (Palansooriya et al., 2019). Notably, biochar combined with CEM was more effective in stimulating alfalfa nutrient uptake compared to single biochar application. The CEM could provide photosynthetic bacteria for soil to promote photosynthesis and endogenous phytohormones synthesis (Talaat, 2019). Thus, the EM combined with biochar could alleviate salinity stress and elevate the contents of plant TN, TP, and TK. Hence, biochar combined with CEM could provide a survival advantage for alfalfa growth by acquiring more nutrients in soil and improving plant absorption (Agegnehu et al., 2017). In general, nutrient contents of alfalfa tissues could generate dramatically active effect on improving alfalfa growth by modulating nutrient supply (Zhang et al., 2019).
Amelioration of Soil Salinity and Fertility
As an excellent soil conditioner, biochar plays a crucial role in reducing soil salinity via improving leaching of soluble salts and declining salt accumulation of surface soils (Saifullah et al., 2018). Biochar addition or combined with CEM reduced soil salinity by 12.97–45.48%, suggesting that biochar addition can alleviate salt stress and offer a suitable environment for alfalfa growth. Moreover, the adsorption of salts on the biochar surfaces or fine pores might be a reason for reducing soil salinity. Another possible explanation for decreased soil salinity could be the cover of biochar that helped downward saline water movement, contributing to the falling soil salinity in topsoil (Hammer et al., 2015; Gunarathne et al., 2020). Biochar addition could facilitate salts leaching by meliorating soil hydraulic conductivity and porosity (Chaganti et al., 2015). Moreover, extensive results carried out confirmed that planting alfalfa was an effective plant-assisted strategy to prevent salinization and promote soil quality in inland regions (Qadir et al., 2001; Cao et al., 2012). Thus, alfalfa planting combined with the amendment of biochar and CEM could also help inhibit salt accumulation in coastal wetlands.
Biochar makes a critical difference in improving soil fertility and rehabilitating salt-affected soils via the improvement in soil functions, such as aggregation formation, a nutrient cycle, and microorganism development (El-Naggar et al., 2019; Gunarathne et al., 2020). Our analysis confirmed that biochar application elevated soil content of TC, TN, AP, and AK, and the enhanced effects become even more significant when biochar is combined with CEM. The high content of nutrients in biochar may elevate soil nutrient status and promote nutrient transformation considerably (Kim et al., 2016; Gunarathne et al., 2020). Besides, the porous structure and super absorption of biochar may enhance soil fertility through reserving nutrients and releasing nutrient slowly (Ding et al., 2016). The increased soil aggregation and water holding ability induced by biochar addition were responsible for stabilizing soil structure, which was conducive to decreasing nutrient leaching and increasing nutrient contents (Agegnehu et al., 2017; El-Naggar et al., 2019; Zhao et al., 2020). In addition, the more pronounced improvement of soil fertility caused by the addition of biochar with CEM may also ascribe the beneficial microorganisms that boost soil nutrient cycling and organic matter decomposition, thereby improving soil nutrient supply (Talaat, 2019; El-Mageed et al., 2020).
Enhancement of Soil Organic Carbon, Microbial Biomass Carbon, NH4+, and NO3– Availability
Considerable reviews from field or laboratory experiments confirmed that biochar could be used to reduce carbon mineralization and sequestrate soil carbon (Lehmann, 2007; Agegnehu et al., 2016; Liu et al., 2021). We showed that SOC and MBC were facilitated under the application of biochar and CEM, as supported by a meta-analysis study by Liu et al. (2016), who revealed biochar addition elevated SOC and MBC by 40 and 18%, respectively. The increased soil SOC and MBC following biochar and CEM addition may be explained by several reasons: (i) the inertness and rich polychromatic acids of biochar may store carbon in soil and increase the mineralization of SOC via activating soil microbial activity and preserving labile soil organic matter (Cooper et al., 2020); (ii) the amendment of biochar and CEM would be more beneficial for accelerating soil carbon decomposition through improving soil aeration and modulating microbial diversity (Talaat, 2019; Liu et al., 2021); (iii) the promoted plant biomass and endogenous carbon formation or exogenous carbon input induced by biochar and CEM would increase soil aggregation formation and strengthen fertility conservation (Liu et al., 2016; Caban et al., 2020).
Amendment of biochar and CEM led to improvements in soil NH4+ and NO3– availabilities, which may be closely connected with stimulated soil N recycling in coastal wetland soil. Increases in soil NH4+ and NO3– may have been linked to the alteration of soil N mineralization to immobilization and the negatively charged biochar surface, in turn influencing plants N absorption (Jones et al., 2012; Lentz et al., 2014; Agegnehu et al., 2017). The improvement of N immobilization and the reduction of N leaching following biochar addition may also be responsible for altering the nitrification rate and enhancing NH4+ content (Sun et al., 2017). The CEM supplement modified the diversity and abundance of beneficial microorganisms in salt-affected soils, which, in turn, stimulated soil organic matter turnover and N availability (Olle and Williams, 2013; Talaat, 2019). The path analysis suggested that soil NH4+ and NO3– were closely correlated with enzyme activities, indicating that biochar and CEM addition may accelerate N transformations by enhancing soil enzyme and microbial activities (Lehmann et al., 2011; Ameloot et al., 2015). Furthermore, the stimulated soil inorganic N availability after biochar and CEM addition was beneficial for alfalfa growth and productivity.
Stimulation of Soil Enzyme Activities
In this study, the mixture of biochar and CEM exerted a beneficial impact on soil sucrase, urease, and alkaline phosphatase activities. Biochar application directly drives changes in soil enzyme activities by targeting diverse soil substrates for the decomposition of SOC (Sinsabaugh, 2010). The priming effect on soil microbial and enzyme activities induced by biochar may stimulate microbial growth by increasing the SOC bioavailability (Pokharel et al., 2020). Significantly, the most likely mechanisms promoting enzyme activities included the following parts: (i) improved soil aggregate formation and water retention (Palansooriya et al., 2019); (ii) enhanced nutrients supply for microbes through improving flows of air, water, and nutrients within soil substrates (Liu et al., 2016); (iii) decreased salinity and provided sufficient carbon sources for microorganisms (Mehmood et al., 2020; Liu et al., 2021). In addition, CEM as a microbial inoculant contained many species of microorganisms and produced biologically active agents that can stimulate the decomposition of organic materials and soil enzyme activities (Talaat, 2019). Soil enzymes are good indicators of soil quality due to the crucial role in influencing microbial activity and nutrient cycling (Pokharel et al., 2020). In our case, soil sucrase, urease, and alkaline phosphatase activities were directly associated with SOC, MBC, and inorganic N, implying that the elevated soil enzyme activities and nutrient availability would be helpful to ameliorate soil quality and promote alfalfa growth (Chávez-García and Siebe, 2019; El-Naggar et al., 2019). Thus, the use of biochar combined with CEM in coastal wetlands soils showed encouraging results, resulting in increased soil enzyme activities, ameliorated soil quality, and improved alfalfa nutrient absorption and growth.
Conclusion
Biochar addition alone and combined with CEM decreased soil salt and improved alfalfa growth, nutrient uptake, soil fertility, SOC, MBC, NH4+, NO3–, and enzyme activities in coastal wetlands. As compared with the CK−CEM treatment, alfalfa mass in the B10−CEM, B20−CEM, B30−CEM, CK + CEM, B10 + CEM, B20 + CEM, B30 + CEM treatment enhanced by 20.01, 91.93, 102.8, 71.91, 84.11, 138.5, and 120.5%, respectively. The B20 + CEM treatment could achieve the best performance in terms of elevating soil quality and enzyme activities, contributing to the highest nutrient concentration and growth of alfalfa. Alfalfa biomass was regulated by elevated plant nutrient uptake through promoting soil quality, such as SOC, MBC, TN, NH4+, NO3–, soil sucrase, urease, and alkaline phosphatase activities. Together, applying biochar compositing with CEM into coastal saline-alkali soils could take as an efficient management practice to accelerate soil nutrient cycling and improve alfalfa productivity. This study could provide effective coastal wetlands restoration approach, and further research should be confirmed in the field.
Data Availability Statement
The original contributions presented in the study are included in the article/supplementary material, further inquiries can be directed to the corresponding author.
Author Contributions
QC contributed to data curation, formal analysis, methodology, and writing of the original draft. JX contributed to data curation and supervision. LP contributed to visualization, methodology, and investigation. XZ contributed to conceptualization and formal analysis. FQ contributed to review and editing. All authors reviewed, edited, and approved the manuscript.
Funding
This study was funded by the Joint Funds of the National Natural Science Foundation of China (U2006215), the National Natural Science Foundation of China (41901118), Natural Science Foundation of Shandong Province (ZR2019PD012), Shandong Key Laboratory of Coastal Environmental Processes, YICCAS (2019SDHADKFJJ10), and Taishan Scholars Program of Shandong Province, China (No. TSQN201909152).
Conflict of Interest
The authors declare that the research was conducted in the absence of any commercial or financial relationships that could be construed as a potential conflict of interest.
Publisher’s Note
All claims expressed in this article are solely those of the authors and do not necessarily represent those of their affiliated organizations, or those of the publisher, the editors and the reviewers. Any product that may be evaluated in this article, or claim that may be made by its manufacturer, is not guaranteed or endorsed by the publisher.
References
Agegnehu, G., Bass, A. M., Nelson, P. N., and Bird, M. I. (2016). Benefits of biochar, compost and biochar-compost for soil quality, maize yield and greenhouse gas emissions in a tropical agricultural soil. Sci. Total Environ. 543, 295–306. doi: 10.1016/j.scitotenv.2015.11.054
Agegnehu, G., Srivastava, A. K., and Bird, M. I. (2017). The role of biochar and biochar-compost in improving soil quality and crop performance: a review. Appl. Soil Ecol. 119, 156–170. doi: 10.1016/j.apsoil.2017.06.008
Akhtar, S. S., Andersen, M. N., Naveed, M., Zahir, Z. A., and Liu, F. (2015). Interactive effect of biochar and plant growth-promoting bacterial endophytes on ameliorating salinity stress in maize. Funct. Plant Biol. 42, 770–781. doi: 10.1071/FP15054
Ali, S., Rizwan, M., Qayyum, M. F., Ok, Y. S., Ibrahim, M., Riaz, M., et al. (2017). Biochar soil amendment on alleviation of droughtand salt stress in plants: a critical review. Environ. Sci. Pollut. Res. 24, 12700–12712. doi: 10.1007/s11356-017-8904-x
Ameloot, N., Sleutel, S., Das, K. C., Kanagaratnam, J., and De Neve, S. (2015). Biochar amendment to soils with contrasting organic matter level: effects on N mineralization and biological soil properties. Glob. Change Biol. Bioenergy 7, 135–144. doi: 10.1111/gcbb.12119
Biederman, L. A., and Harpole, W. S. (2013). Biochar and its effects on plant productivity and nutrient cycling: a meta-analysis. Glob. Change Biol. Bioenergy 5, 202–214. doi: 10.1111/gcbb.12037
Caban, M., Folentarska, A., Lis, H., Kobylis, P., Bielicka-Giełdon, A., Kumirska, J., et al. (2020). Critical study of crop-derived biochars for soil amendment and pharmaceutical ecotoxicity reduction. Chemosphere 248:125976. doi: 10.1016/j.chemosphere.2020.125976
Cai, J. F., Jiang, F., Liu, X. S., Sun, K., Wang, W., Zhang, M. X., et al. (2021). Biochar-amended coastal wetland soil enhances growth of Suaeda salsa and alters rhizosphere soil nutrients and microbial communities. Sci. Total Environ. 788:147707. doi: 10.1016/j.scitotenv.2021.147707
Cao, J., Li, X., Kong, X., Zed, R., and Dong, L. (2012). Using alfalfa (Medicago sativa) to ameliorate salt-affected soils in Yingda irrigation district in Northwest China. Acta Ecol. Sin. 32, 68–73. doi: 10.1016/j.chnaes.2011.12.001
Chaganti, V. N., Crohn, D. M., and Šimůnek, J. (2015). Leaching and reclamation of a biochar and compost amended saline-sodic soil with moderate SAR reclaimed water. Agric. Water Manag. 158, 255–265. doi: 10.1016/j.agwat.2015.05.016
Chávez-García, E., and Siebe, C. (2019). Rehabilitation of a highly saline-sodic soil using a rubblebarrier and organic amendments. Soil Tillage Res. 189, 176–188. doi: 10.1016/j.still.2019.01.003
Chen, M., Alim, N., Zhang, Y. T., Xu, N., and Cao, X. D. (2018). Contrasting effects of biochar nanoparticles on the retention and transport of phosphorus in acidic and alkaline soils. Environ. Pollut. 239, 562–570. doi: 10.1016/j.envpol.2018.04.050
Cooper, J., Greenberg, I., Ludwig, B., Hippich, L., Ficher, D., Glaser, B., et al. (2020). Effect of biochar and compost on soil properties and organic matter in aggregate size fractions under field conditions. Agric. Ecosyst. Environ. 295:106882. doi: 10.1016/j.agee.2020.106882
Ding, Y., Liu, Y. G., Liu, S. B., Li, Z. W., Tan, X. F., and Huang, X. X. (2016). Biochar to improve soil fertility: a review. Agron. Sustain. Dev. 36, 35–52. doi: 10.1007/s13593-016-0372-z
El-Mageed, T. A. A., Rady, M. M., Taha, R. S., El Azeam, S. A., Simpson, C. R., and Semida, W. M. (2020). Effects of integrated use of residual sulfur-enhanced biochar with effective microorganisms on soil properties, plant growth and short-term productivity of Capsicum annuum under salt stress. Sci. Hortic. 261:108930. doi: 10.1016/j.scienta.2019.108930
El-Naggar, A., Lee, S. S., Rinklebe, J., Farooq, M., Song, H., Sarmah, A. K., et al. (2019). Biochar application to low fertility soils: a review of current status, and future prospects. Geoderma 337, 536–554. doi: 10.1016/j.geoderma.2018.09.034
Gunarathne, V., Senadeera, A., Gunarathne, U., Biswas, J. K., Almaroai, Y. A., and Vithanage, M. (2020). Potential of biochar and organic amendments for reclamation of coastal acidic-salt affected soil. Biochar 2, 107–120. doi: 10.1007/s42773-020-00036-4
Hammer, E. C., Forstreuter, M., Rillig, M. C., and Kohler, J. (2015). Biochar increases arbuscular mycorrhizal plant growth enhancement and ameliorates salinity stress. Appl. Soil Ecol. 96, 114–121. doi: 10.1016/j.apsoil.2015.07.014
Hassani, A., Azapagic, A., and Shokri, N. (2020). Predicting long-term dynamics of soil salinity and sodicity on a global scale. Proc. Natl. Acad. Sci. U.S.A. 117, 33017–33027. doi: 10.1073/pnas.2013771117
Hu, C., and Qi, Y. C. (2013). Long-term effective microorganisms application promote growth and increase yields and nutrition of wheat in China. Eur. J. Agron. 46, 63–67. doi: 10.1016/j.eja.2012.12.003
Huang, L. B., Bai, J. H., Chen, B., Zhang, K. J., Huang, C., and Liu, P. P. (2012). Two-decade wetland cultivation and its effects on soil properties in salt marshes in the Yellow River Delta, China. Ecol. Inform. 10, 49–55. doi: 10.1016/j.ecoinf.2011.11.001
Jeffery, S., Memelink, I., Hodgson, E., Jones, S., van de Voorde, T. F., Bezemer, T. M., et al. (2017). Initial biochar effects on plant productivityderive from N fertilization. Plant Soil 13, 1–4. doi: 10.1007/s11104-016-3171-z
Jones, D. L., Rousk, J., Edwards-Jones, G., DeLuca, T. H., and Murphy, D. V. (2012). Biochar-mediated changes in soil quality and plant growth in a three year field trial. Soil Biol. Biochem. 45, 113–124. doi: 10.1016/j.soilbio.2011.10.012
Kim, H. S., Kim, K. R., Yang, J. E., Ok, Y. S., Owens, G., Nehls, T., et al. (2016). Effect of biochar on reclaimed tidal land soil properties and maize (Zea mays L.) response. Chemosphere 142, 153–159. doi: 10.1016/j.chemosphere.2015.06.041
Kirwan, M. L., and Megonigal, J. P. (2013). Tidal wetland stability in the face of human impactsand sea-level rise. Nature 504, 53–60. doi: 10.1038/nature12856
Lashari, M. S., Ye, Y., Ji, H., Li, L., Kibue, G. W., Lu, H., et al. (2015). Biochar-manure compost in conjunction with pyroligneous solution alleviated salt stress and improved leaf bioactivity of maize in a saline soil from central China: a 2-year field experiment. J. Sci. Food Agric. 95, 1321–1327. doi: 10.1002/jsfa.6825
Lehmann, J., Rillig, M. C., Thies, J., Masiello, C. A., Hockaday, W. C., and Crowley, D. (2011). Biochar effects on soil biota-a review. Soil. Biol. Biochem. 43, 1812–1836. doi: 10.1016/j.soilbio.2011.04.022
Lentz, R. D., Ippolito, J. A., and Spokas, K. A. (2014). Biochar and manure effects on net nitrogen mineralization and greenhouse gas emissions from calcareous soil under corn. Soil Sci. Soc. Am. J. 78, 1641–1655. doi: 10.2136/sssaj2014.05.0198
Li, K. S., Li, Q. X., Geng, Y. H., and Liu, C. X. (2021). An evaluation of the effects of microstructural characteristics and frost heave on the remediation of saline-alkali soils in the Yellow River Delta, China. Land Degrad. Dev. 32, 1325–1337. doi: 10.1002/ldr.3801
Liang, J. F., An, J., Gao, J. Q., Zhang, X. Y., Song, M. H., and Yu, F. H. (2019). Interactive effects of biochar and AMF on plant growth and greenhouse gas emissions from wetland microcosms. Geoderma 346, 11–17. doi: 10.1016/j.geoderma.2019.03.033
Liu, J., Jiang, B. S., Shen, J. L., Zhu, X., Yi, W. Y., Li, Y., et al. (2021). Contrasting effects of straw and straw-derived biochar applications on soil carbon accumulation and nitrogen use efficiency in double-rice cropping systems. Agric. Ecosyst. Environ. 311:107286. doi: 10.1016/j.agee.2020.107286
Liu, M. H., Zhuo, Z. J., Chen, L., Wang, L. Q., Ji, L. Z., and Xiao, Y. (2020). Influences of arbuscular mycorrhizae, phosphorus fertiliser and biochar on alfalfa growth, nutrient status and cadmium uptake. Ecotoxicol. Environ. Saf. 196:110537. doi: 10.1016/j.ecoenv.2020.110537
Liu, S. L., Hou, X. Y., Yang, M., Cheng, F. Y., and Coxixo, A. (2018). Factors driving the relationships between vegetation and soil properties in the Yellow River Delta, China. Catena 165, 279–285. doi: 10.1016/j.catena.2018.02.004
Liu, S. W., Zhang, Y. J., Zong, Y. J., Hu, Z. Q., Wu, S., Zhou, J., et al. (2016). Response of soil carbon dioxide fluxes, soil organic carbon and microbial biomass carbon to biochar amendment: a meta-analysis. Glob. Change Biol. Bioenergy 8, 392–406. doi: 10.1111/gcbb.12265
Luo, X. X., Liu, G. C., Xia, Y., Chen, L., and Jiang, Z. X. (2017). Use of biochar-compost to improve properties and productivity of the degraded coastal soil in the Yellow River Delta, China. J. Soils Sediments 17, 780–789. doi: 10.1007/s11368-016-1361-1
Mehmood, I., Qiao, L., Chen, H. Q., Tang, Q. Y., Woolf, D., and Fam, M. S. (2020). Biochar addition leads to more soil organic carbon sequestration under a maize-rice cropping system than continuous flooded rice. Agric. Ecosyst. Environ. 298:106965. doi: 10.1016/j.agee.2020.106965
Olle, M., and Williams, I. H. (2013). Effective microorganisms and their influence on vegetable production-a review. J. Hortic. Sci. Biotechnol. 88, 380–386. doi: 10.1080/14620316.2013.11512979
Olmo, M., Villar, R., Salazar, P., and Alburquerque, J. A. (2016). Changes in soil nutrient availability explain biochar’s impact on wheat root development. Plant Soil 399, 333–343. doi: 10.1007/s11104-015-2700-5
Palansooriya, K. N., Ok, Y. S., Awad, Y. M., Lee, S. S., Sung, J. K., Koutsospyros, A., et al. (2019). Impacts of biochar application on upland agriculture: a review. J. Environ. Manage. 234, 52–64. doi: 10.1016/j.jenvman.2018.12.085
Pokharel, P., Ma, Z., and Chang, S. X. (2020). Biochar increases soil microbial biomass with changes in extra-and intracellular enzyme activities: a global meta-analysis. Biochar 2, 65–79. doi: 10.1007/s42773-020-00039-1
Qadir, M., Schubert, S., Ghafoor, A., and Murtaza, G. (2001). Amelioration strategies for sodic soils: a review. Land Degrad. Dev. 12, 357–386. doi: 10.1002/ldr.458
Raklami, A., Tahiri, A. I., Bechtaoui, N., Abdelhay, E. G., Pajuelo, E., Baslam, M., et al. (2021). Restoring the plant productivity of heavy metal-contaminated soil using phosphate sludge, marble waste, and beneficial microorganisms. J. Environ. Sci. 99, 210–221. doi: 10.1016/j.jes.2020.06.032
Saifullah, Dahlawi, S., Naeem, A., Rengel, Z., and Naidu, R. (2018). Biochar application for the remediation of salt-affected soils: challenges and opportunities. Sci. Total Environ. 625, 320–335.
Sigua, G. C., Novak, J. M., Watts, D. W., Johnson, M. G., and Spokas, K. (2016). Efficacies of designer biochars in improving biomass and nutrient uptake of winter wheat grown in a hard setting subsoil layer. Chemosphere 142, 176–183. doi: 10.1016/j.chemosphere.2015.06.015
Sinsabaugh, R. L. (2010). Phenol oxidase, peroxidase and organic matter dynamics of soil. Soil. Biol. Biochem. 42, 391–404. doi: 10.1016/j.soilbio.2009.10.014
Stagg, C. L., Schoolmaster, D. R., Krauss, K. W., Cormier, N., and Conner, W. H. (2017). Causal mechanisms of soil organic matter decomposition:deconstructing salinity and flooding impacts in coastal wetlands. Ecology 98, 2003–2018. doi: 10.1002/ecy.1890
Sun, H. J., Lu, H. Y., Chu, L., Shao, H. B., and Shi, W. M. (2017). Biochar applied with appropriate rates can reduce N leaching, keep N retention and not increase NH3 volatilization in a coastal saline soil. Sci. Total Environ. 575, 820–825. doi: 10.1016/j.scitotenv.2016.09.137
Sun, J., He, F., Shao, H. B., Zhang, Z. H., and Xu, G. (2016). Effects of biochar application on Suaeda salsa growth and saline soil properties. Environ. Earth Sci. 75:630. doi: 10.1007/s12665-016-5440-9
Talaat, N. B. (2019). Effective microorganisms: an innovative tool for inducing common bean (Phaseolus vulgaris L.) salt-tolerance by regulating photosynthetic rate and endogenous phytohormones production. Sci. Hortic. 250, 254–265. doi: 10.1016/j.scienta.2019.02.052
Talaat, N. B., Ghoniem, A. E., Abdelhamid, M. T., and Shawky, B. T. (2015). Effective microorganisms improve growth performance, alter nutrients acquisition and induce compatible solutes accumulation in common bean (Phaseolus vulgaris L.) plants subjected to salinity stress. Plant Growth Regul. 75, 281–295. doi: 10.1007/s10725-014-9952-6
Tan, X., Kong, L., Yan, H., Wang, Z., He, W., and Wei, G. (2014). Influence of soil factors on the soil enzyme inhibition by Cd. Acta Agric. Scand. Sect. B Soil Plant Sci. 64, 666–674. doi: 10.1080/09064710.2014.953985
Vance, E. D., Brookes, P. C., and Jenkinson, D. S. (1987). An extraction method for measuring soil microbial biomass C. Soil. Biol. Biochem. 19, 703–707. doi: 10.1016/0038-0717(87)90052-6
Wang, Y., Li, W. Q., Du, B. H., and Li, H. H. (2021). Effect of biochar applied with plant growth-promoting rhizobacteria (PGPR) on soil microbial community composition and nitrogen utilization in tomato. Pedosphere 31, 872–881. doi: 10.1016/S1002-0160(21)60030-9
Zhang, F. G., Liu, M. H., Li, Y., Che, Y. Y., and Xiao, Y. (2019). Effects of arbuscular mycorrhizal fungi, biochar and cadmium on the yield and element uptake of Medicago sativa. Sci. Total Environ. 655, 1150–1158. doi: 10.1016/j.scitotenv.2018.11.317
Zhao, Q. Q., Bai, J. H., Zhang, G. L., Jia, J., Wang, W., and Wang, X. (2018). Effects of water and salinity regulation measures on soil carbon sequestration in coastal wetlands of the Yellow River Delta. Geoderma 319, 219–229. doi: 10.1016/j.geoderma.2017.10.058
Keywords: biochar, alfalfa growth, alfalfa nutrient uptake, soil quality, soil enzyme activities
Citation: Cui Q, Xia J, Peng L, Zhao X and Qu F (2022) Positive Effects on Alfalfa Productivity and Soil Nutrient Status in Coastal Wetlands Driven by Biochar and Microorganisms Mixtures. Front. Ecol. Evol. 9:798520. doi: 10.3389/fevo.2021.798520
Received: 20 October 2021; Accepted: 22 December 2021;
Published: 27 January 2022.
Edited by:
Zhenguo Niu, Aerospace Information Research Institute, Chinese Academy of Sciences (CAS), ChinaReviewed by:
Mingxiang Zhang, Beijing Forestry University, ChinaQingxue Guo, Hangzhou Normal University, China
Pifu Cong, National Marine Environmental Monitoring Center, China
Shouzheng Tong, Northeast Institute of Geography and Agroecology, Chinese Academy of Sciences (CAS), China
Copyright © 2022 Cui, Xia, Peng, Zhao and Qu. This is an open-access article distributed under the terms of the Creative Commons Attribution License (CC BY). The use, distribution or reproduction in other forums is permitted, provided the original author(s) and the copyright owner(s) are credited and that the original publication in this journal is cited, in accordance with accepted academic practice. No use, distribution or reproduction is permitted which does not comply with these terms.
*Correspondence: Jiangbao Xia, xiajb@163.com