Patch-level processes of vegetation underlying site-level restoration patterns in a megatidal salt marsh
- Department of Biology, University of New Brunswick, Fredericton, NB, Canada
Vegetation patterns during salt marsh restoration reflect underlying processes related to colonization, reproduction, and interactions of halotolerant plants. Examining both pattern and process during recovery is valuable for understanding and managing salt marsh restoration projects. We present a decade of vegetation dynamics during salt marsh restoration (2011–2020) at a study site in the Bay of Fundy with megatidal amplitudes, strong currents, cold winter temperatures, and ice. We mainly investigated reproduction (asexual and sexual) and associated spread rates of Spartina grasses, and their health-related states (stem density, canopy height, and percent flowering) which help inform the probability of processes occurring. We also estimated modes of colonization and began quantifying the effects of interspecific interactions and environmental conditions on plant state. Spartina pectinata was the only pastureland plant to survive dike-breaching and saltwater intrusion in 2010; however, it was stunted compared to reference plants. Spartina pectinata patches remained consistent initially, before decreasing in size, and disappearing by the fifth year (2015). This early dynamic may provide initial protection to a developing salt marsh before Spartina alterniflora becomes established. Spartina alterniflora first colonized the sites in year 2 (2012), likely via deposition of rhizomal material, and then spread asexually before seedlings (sexual reproduction) appeared in year 4 (2014). Vegetation cover subsequently increased greatly until near-complete in year 9 (2019). The early successional dynamics of S. pectinata and S. alterniflora occurred spatially independently of each other, and likely contributed to sediment retention, creating an improved environment for S. patens, the dominant high marsh species in our region. Spartina patens have been slowly spreading into restoration sites from high elevation areas since year 6 (2016). We expect that competition between S. alterniflora and S. patens will result in the typical distinct zonation between high and low marsh zones. A next study will use the quantified processes for spatial-explicit modeling to simulate patterns of vegetation recovery, and to evaluate different salt marsh restoration strategies for the Bay of Fundy and elsewhere. Thus, proper identification and quantification of pattern-building processes in salt marsh vegetation recovery, the focus of our present study, was an essential step.
Introduction
Community patterns are the result of underlying, patch-level processes of individual species (Levin, 1992; Tilman and Kareiva, 1997; Liu et al., 2016). Patch-level processes can be generally defined as small-scale ecological changes in species that, when acting in unison with others and through time, affect larger-scale distributions of the communities in a defined area (Wu and Loucks, 1995). During ecological restoration, changes in vegetation community patterns reflect changes in species abundance and distribution (Radeloff et al., 2001; Peng et al., 2012; Virgin et al., 2020), and quantitatively analyzing processes related to colonization, reproduction, and interactions of these species can elucidate the trajectory (structure and rate of change) of the patterns (Hupp, 1992; Bergen et al., 2000). In addition, monitoring health-related states (live stem density, canopy height, and percent of live stems flowering) of vegetation at the patch-level informs the probability of processes occurring (Briske et al., 2005). Ecological studies that examine pattern, process, and state are valuable for understanding and managing ecosystems (Levin, 1999).
After tidal flow to salt marsh restoration sites is reestablished, the vegetation community undergoes substantial changes in its pattern, including extirpation of terrestrial or freshwater vegetation, decreased abundance of brackish vegetation, and increased abundance of halotolerant vegetation, which is typically led by those most tolerant of saltwater inundation and followed by those that are superior competitors at higher elevation (Sinicrope et al., 1990; van Proosdij et al., 2010; Virgin et al., 2020). The rate of vegetation recovery (i.e., rate of change of the vegetation community patterns) of salt marshes varies depending on several conditions including sediment deposition and edaphic characteristics; the species of terrestrial, freshwater, and semi-halotolerant vegetation present prior to restoration; proximity to sources of reproductive material of halotolerant vegetation; and patch-level processes and states of vegetation (Broome et al., 1988; Warren et al., 2002). For the present salt marsh restoration study (Aulac, New Brunswick, Canada), community patterns following saltwater intrusion after breaching an agriculture dike (formerly protecting pasture land from tidal influence) and die-off of pastureland vegetation were: decline of the on-site semi-halotolerant Spartina pectinata (freshwater cordgrass, syn. Sporobolus michauxianus), establishment and spread of Spartina alterniflora (saltwater cordgrass, syn. Sporobolus alterniflorus; Peterson et al., 2014; Bortolus et al., 2019), and establishment of a high marsh (dominated by salt marsh hay Spartina patens, syn. Sporobolus pumilus), resulting in a salt marsh with distinct zonation (Boone et al., 2017; Virgin et al., 2020).
Processes contributing to site-level community patterns observed during the Aulac salt marsh restoration project may have been driven by physiological thresholds, ecological interactions, or a combination of both. Spartina pectinata is a semi-halotolerant species with a salinity tolerance up to 0.2 M NaCl (12 ppt; Warren et al., 1985). Although spread rates of S. pectinata have been measured in a wet prairie ecosystem (up to 3 m y−1, Fraser and Kindscher, 2005), they have not been rigorously measured within the context of salt marsh restoration. In contrast, rates of S. alterniflora spread have been well studied in salt marshes throughout the world because of its capacity as an invasive species (Taylor and Hastings, 2004; Zhang et al., 2017). It is well-adapted to frequent flooding by saltwater, being tolerant of high salinities including 0.6 M NaCl (35 ppt; Vasquez et al., 2006) and above (Webb, 1983). Furthermore, it displays tall and short phenotypes, with the short phenotype likely reflecting less favorable environmental conditions (Anderson and Treshow, 1980; Zerebecki et al., 2021). Spartina alterniflora’s asexual spread rates when colonizing intertidal soft sediments can be vigorous (134 ± 28 m y−1; Callaway and Josselyn, 1992), and its seeds can disperse up to hundreds of km via tidal currents (Morgan and Sytsma, 2013). Seedlings typically need more than one growing season to become as tall as mature plants (Redfield, 1972), and in appropriate elevations and edaphic conditions, they are more successful growing in bare spaces than under mature canopies (Metcalfe et al., 1986). Spartina alterniflora can tolerate low elevation marsh conditions (i.e., longer inundation) that other species cannot, which leads to strong vegetation zonation with S. patens. Spartina patens typically occupy high elevation areas in mature salt marshes. This zonation is controlled by abiotic stress on the seaward side and competitive stress on the landward side (Bertness, 1991). Spartina patens displays dense, clonal morphology, has a very high salinity tolerance (up to 60 ppt, Hester et al., 1996), and spreads slower than S. alterniflora (~1 m y−1 estimated from Ayres et al., 2004). Frenkel and Boss (1988) (Oregon, United States) reported that S. patens did not begin to spread at an apparent exponential rate until three decades after its colonization. Overall, substantial research on processes affecting site-level patterns in salt marshes has been conducted (see citations included earlier in this paragraph), but more is needed to better understand them during restoration. Research on recovery dynamics of salt marshes is essential, because of historical high losses of salt marshes due to human activity (Gedan et al., 2009) and the relatively recent realization of their contribution to important ecosystem services, including protection of coastal infrastructure (Costanza et al., 2008), carbon sequestration (Mcleod et al., 2011), and providing habitat for fish, birds, and invertebrates (Minello et al., 2003).
The objective of the present study was to identify and quantitatively analyze patch-level processes of vegetation community change that explain the site-level patterns observed during salt marsh restoration on a megatidal (semi-diurnal tidal amplitudes in the study area reach ~14 m; Fisheries and Oceans Canada, 2022a) and ice-influenced coastline. Our study examined plant dynamics annually from 2011 to 2020, following a managed realignment that started in Fall 2010 in the upper Bay of Fundy. We investigated the health-related states and spread rates of Spartina grasses (S. pectinata, S. alterniflora, and S. patens), and modes of reproduction and seedling performance of S. alterniflora. Vegetation reproduction (mostly asexual) was the process that was quantified in the most detail during our study, but we also provided our best estimate regarding colonization, and began quantifying the effects of biotic and abiotic interactions on health-related vegetation states. We first present patterns of vegetation community change at the site level and then describe, in detail, patch-level dynamics including plant health-related states (stem density, canopy height, and percent flowering) and spread rates. We subsequently discuss ecological insights and restoration implications and describe how our process-related results can be used to model spatial dynamics of vegetation.
Materials and methods
Study area
The sites of the salt marsh restoration project are located in Aulac, New Brunswick (Figure 1). The restoration project began in 2009 and is led by Ducks Unlimited Canada (DUC) and partners (Millard et al., 2013; Boone et al., 2017). Before the old dike was breached in fall 2010, the geomorphology of the site was assessed, and hydrodynamic modeling was done to encourage the reestablishment of salt marsh. The project consists of two restoration sites (Restoration East and West) and two established salt marsh sites (Reference East and West). The restoration is a managed realignment, where the agricultural dike bordering pastoral land was no longer feasible to maintain due to wave action, erosion, and sea level rise (Boone et al., 2017; Virgin et al., 2020). A new dike was constructed ~100 m landward of the old agricultural dike; the latter was later breached to encourage the development of salt marsh in front of the new dike which should prolong its lifespan. Two breaches were excavated from the seaward dike of Restoration East and one breach for Restoration West. The Reference sites are mainly high elevation salt marsh with S. patens dominating (typical of Bay of Fundy salt marshes; Virgin et al., 2020) and S. alterniflora restricted to the creek and seaward edges. The initial elevation disparity between Restoration and Reference sites was as much as 2 m in some areas, and Restoration West (~11.0 ha in size) was ~0.43 m higher than Restoration East (~5.5 ha in size; Virgin et al., 2020). The Restoration sites are typically inundated twice in a 24-h period, following the tidal regime of the region (Desplanque and Mossman 2004), with inundation times varying depending on the tidal amplitudes (Higher High Water Mean Tide is 11.66 m for nearby tidal station Pecks Point; Fisheries and Oceans Canada, 2022b). In the pre-breach sampling of the sites to be restored (summer 2010), the vegetation community was terrestrial with some semi-aquatic plants, notably S. pectinata (Virgin et al., 2020). Spartina pectinata was the only plant species that survived tidal inundation following breaching.
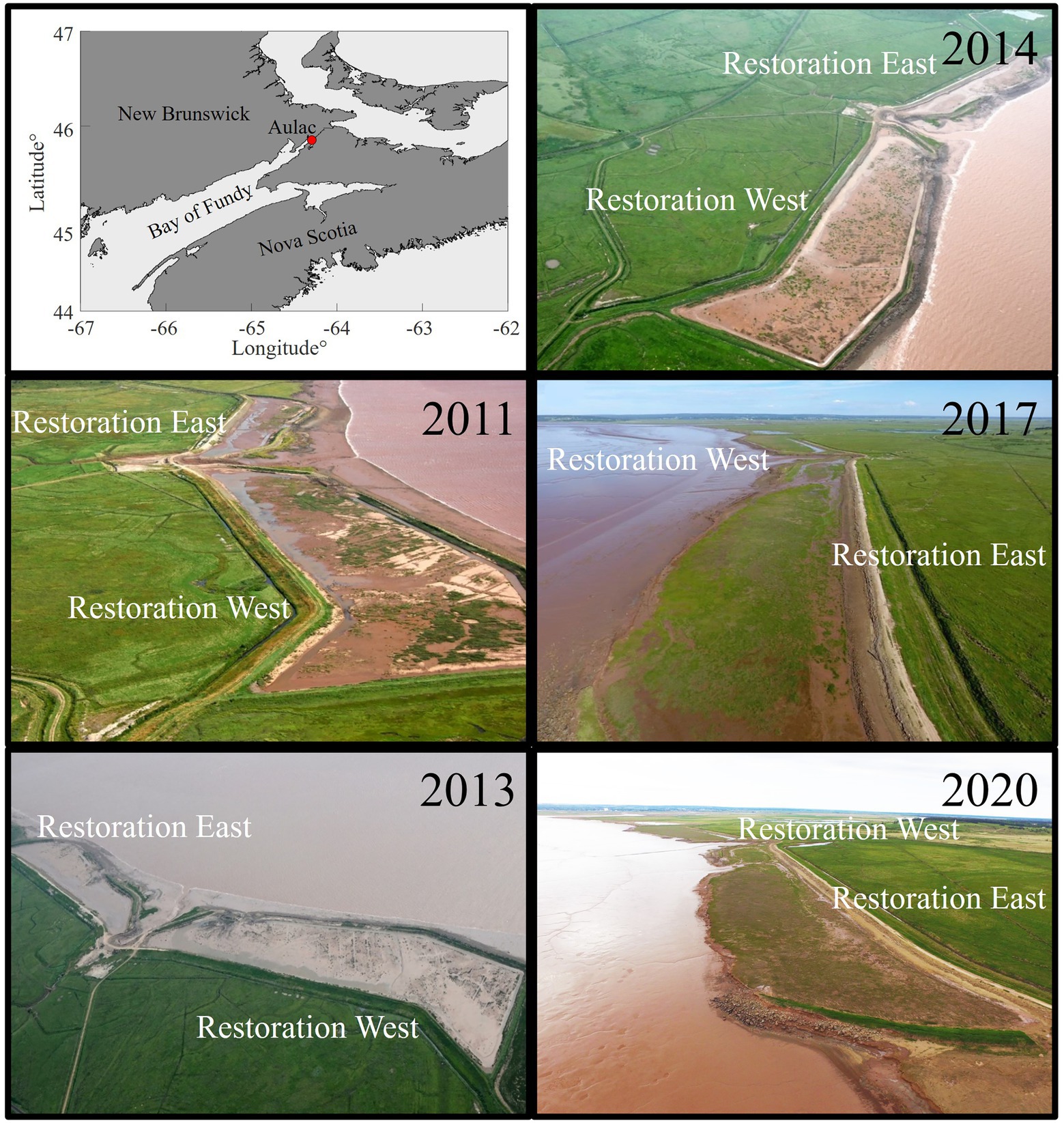
Figure 1. Location of the Aulac (Fort Beauséjour) salt marsh restoration project in New Brunswick, Canada (top left); the study area is in Cumberland Basin in the upper Bay of Fundy. Aerial photographs to show changes in vegetation cover in the two Restoration sites (see also Virgin et al., 2020). Photographs courtesy of Ducks Unlimited Canada (August 2011, July 2013, July 2014), Sebastian Richard (July 2017), and GSN (June 2020).
Vegetation dynamics at the site level
To determine large-scale vegetation patterns in Restoration sites, we subdivided each site into five strata and visually estimated and sketched percent cover of vegetation and the spatial distribution of the different sized plant patches (monospecific groups of stems; see Figure 1, panels 2011–2014) in July. The strata dividing the Restoration sites ran perpendicular to the shoreline and were 100–180 m long and 135–160 m wide. Vegetation cover was estimated visually by standing on the new landward dike midway through each stratum. From 2011 to 2014, before patches started merging, the number of patches per stratum was counted per size class. There were five size classes depending on patch diameter; they were < 0.1 (1), 0.1–0.5 (2), 0.5–1 (3), 1–2 (4), and > 2 m (5). In 2013–2014, the species of Spartina (either S. pectinata or S. alterniflora) in these patches was recorded in three randomly selected half-strata censused on foot.
Dynamics of Spartina patches in restoration sites
To determine the details of plant spread and state (stem density, canopy height, and percent flowering), we haphazardly selected and monitored five replicate patches per species (S. pectinata and S. alterniflora) per site, every 2–4 weeks from mid-June until fall 2011–2020. Note that after winter, the plants first appear above ground in late May–early June. A patch selected for study was initially defined as a monospecific group of five or more stems that was at least 2 m away from the next closest patch; in 2014, this separation distance was reduced to 50 cm (the length of a rhizome; Bertness, 1991). From 2011 to 2014, new patches were selected, and their GPS location recorded at the beginning of each growing season. In contrast, from 2015 to 2020, the locations of patches selected in 2015 were monitored across subsequent years because the vegetation had largely coalesced into a meadow of Spartina. Patches were classified based on their attributes at the time of selection, hereafter referred to as patch type. Patch types were: S. pectinata (0.24–4.25 m starting diameter); Large S. alterniflora (8–20 m starting diameter) and Small S. alterniflora (0.28–2.06 m starting diameter); Seedling S. alterniflora (young-of-the-year), first observed onsite in 2014; and S. patens located along dike edges or found within the Restoration sites (first observed in 2016 in Restoration West; see Supplementary Figure 1.1 for example year of patch locations). For each patch, the number of live and flowering stems were counted, and the first and fifth tallest plant heights were measured; the tallest stem was canopy height, and the fifth tallest was measured to detect possible presence of tall outliers. When patch diameter was larger than 1 m or if it merged with other patches, plant counts and height measurements were done within a quadrat (0.5 m × 0.5 m) near the middle of the patch. Patch area was estimated as an ellipse, for S. pectinata and S. alterniflora from 2011 to 2015 and for within-site S. patens from 2017 to 2020, using the longest patch diameter and the diameter perpendicular to the longest. Each patch was photographed on each monitoring date with a ruler as a scale to complement field data.
Spartina in reference sites
In the Reference sites, Spartina spp. “patches” (i.e., GPS locations marked with a survey flag, Supplementary Figure 1 .1) were monitored for plant counts and heights as in the section “Dynamics of Spartina patches in restoration sites” using a 0.25-m2 quadrat. Three to five replicate “patches” per site for each patch type included both phenotypes of S. alterniflora, the tall form bordering protected creeks within the marsh (Creek type) and the short form located along the exposed seaward edge of the marsh (Edge type); S. pectinata near the terrestrial border in Reference East (none present in Reference West); and S. patens in the high marsh zone. Note that the S. alterniflora edge patches in Reference East were omitted from analysis, because new patches (which were of mixed species composition and stressed) needed to be selected yearly due to the high erosion of that shoreline (Virgin et al., 2020).
Spartina patens encroachment onto restoration sites proper
To record the spread of S. patens from the new dike onto the Restoration sites, we monitored six S. patens patches (n = 3 Restoration site−1) per year from 2017 to 2020. These patches were selected based on their stem density and canopy height (approaching that of Reference sites: e.g., 418 ± 40 live stems m−2 and 29 ± 2 cm tall; mean ± SE, n = 10 reference patches on 23 June 2017), size (>1 m longest diameter), and integrity. A gridded 1.0 m × 0.6 m quadrat, divided into 375 4.0 cm × 4.0 cm cells using monofilament line, was positioned such that ~10 cm of the seaward edge of the S. patens patch was within the quadrat on the first deployment date and its spread seaward could be recorded (see Supplementary Figure 2 .1 for photograph of quadrat). Permanent bamboo stakes were inserted into sediment under the corners of the quadrat so that it could be placed into the same position at every sampling date. On each date, the seaward edge of the S. patens patches was carefully drawn on a gridded datasheet, as well as photographed. The distance advanced of the patches’ edge onto the Restoration sites proper was measured for each from the drawings, with the first drawing in mid-June representing a start distance of 0 cm.
Data analysis
To evaluate large-scale patterns, percent vegetation cover in Restoration sites was analyzed using ANOVA (Minitab 18 Statistical Software, 2015) with Year (10 levels: 2011–2020) and Site (two levels: Restoration East and West) as fixed factors, and Stratum (five levels, nested in Site) as a random factor. Dynamics of the distribution of patch sizes (five size classes, with resemblance matrix constructed using Euclidian distance) were analyzed using Permutation Multivariate ANOVA (PRIMER with PERMANOVA add-on, v. 6; Anderson et al., 2008) with Year (four levels: 2011–2014) and Site as fixed factors, and Stratum as a random factor.
To determine change in Spartina patch area over a growing season, we first estimated area of each patch after a standardized period of 90 days by using simple linear regression of patch area versus days since the first sampling date (usually mid-June). Percent areal growth was then calculated by dividing by initial patch area and multiplying by 100. This was done for each patch of S. pectinata in 2011–2014, S. alterniflora in 2012–2015, and non-dike S. patens in 2017–2020. For S. pectinata and S. alterniflora, percent areal growth was analyzed using ANOVA with Year (three levels: 2012–2014) and Species (two levels) as fixed factors. Data obtained in 2011 for S. pectinata and 2015 for S. alterniflora were graphed but not included in the analysis.
To estimate encroachment of S. patens in dike patches onto the Restoration sites, we quantified incremental distance advanced after each sampling round, standardized for 2-week periods, during the growing seasons in 2017–2020. Specifically, using the grid drawings mentioned above, we selected (in a stratified random manner) five measures of the leading patch edge per drawing, converted them to standardized incremental advance, and used ANOVA, with Year (four levels: 2017–2020) and Round (six levels: June–September) as fixed factors, and Patch (six replicates; nested in Year) as a random factor. Note that we assumed edaphic conditions within Restoration sites were suitable for S. patens when S. patens patches had positive annual spread.
To examine differences in plant states of S. pectinata and S. alterniflora, live stem density, canopy height, and percent of live stems flowering were analyzed using ANOVA or PERMANOVA (which can be used for univariate analysis; Anderson et al., 2008) using Euclidian distance. For S. pectinata in 2013–2014, which is when we had a complete dataset, Year (two levels) and Site type (two levels: Restoration and Reference) were fixed factors, and Patch (3–10 replicates) was a random factor (the error term). For S. alterniflora in 2015–2020, which is when we repeatedly monitored the same patches, Year (when used, six levels) and Patch type (four or eight levels, described in the section Spartina in reference sites) were fixed factors, and Patch (5–10 replicates; nested in Patch type) was a random factor. More explicitly, the analyses that spanned 6 years (2015–2020, ~third week of August for each year) included the S. alterniflora patch types: Restoration Large, Restoration Small, Reference Creek, and Reference Edge; while the analyses conducted for an example year (namely 22 August 2017) to compare all patch types included the aforementioned patch types as well as young-of-the-year patches from 2014 to 2017. Planned contrasts in the PERMANOVAs focused on differences in vegetation states of the patch types sampled on 22 August 2017 to determine how patch types in Restoration sites compared to patch types in Reference sites, as well as how many years it took for patches established by seedlings to be similar to mature patches.
For univariate analyses, assumptions of homogeneity of variance and normality of residuals were assessed using Cochran’s C test and visual examination of residuals, respectively. Dependent variables were transformed as necessary using logit for percent, square root for counts, and log10 for measurements (indicated in the tables). Construction of F-ratios and Pseudo F-ratios were as per Underwood (1997). Tukey’s post hoc comparisons were conducted where appropriate to help interpretation and are presented as Supplementary material. Means ± SE are presented throughout the results, unless indicated otherwise.
Results
Site dynamics
Mean vegetation cover in Restoration sites was low to moderate in the first-year post breach (July 2011: Restoration East: 25 ± 6% and Restoration West: 51 ± 5%, n = 5 strata) and decreased (by 22% in Restoration East and 28% in Restoration West) from 2012 to 2013. From 2014 to 2019 however, vegetation cover rapidly increased (by 93% in Restoration East and 71% in Restoration West) before plateauing in 2019–2020; Figures 1, 2; Supplementary Table 1 .1. Furthermore, the two Restoration sites differed (significant Year*Site interaction, p < 0.001; Table 1; Supplementary Table 1 .1) in that the East site typically had less vegetation cover than the West site, but by 2019–2020 both sites had essentially complete cover (~95%; Figure 2).
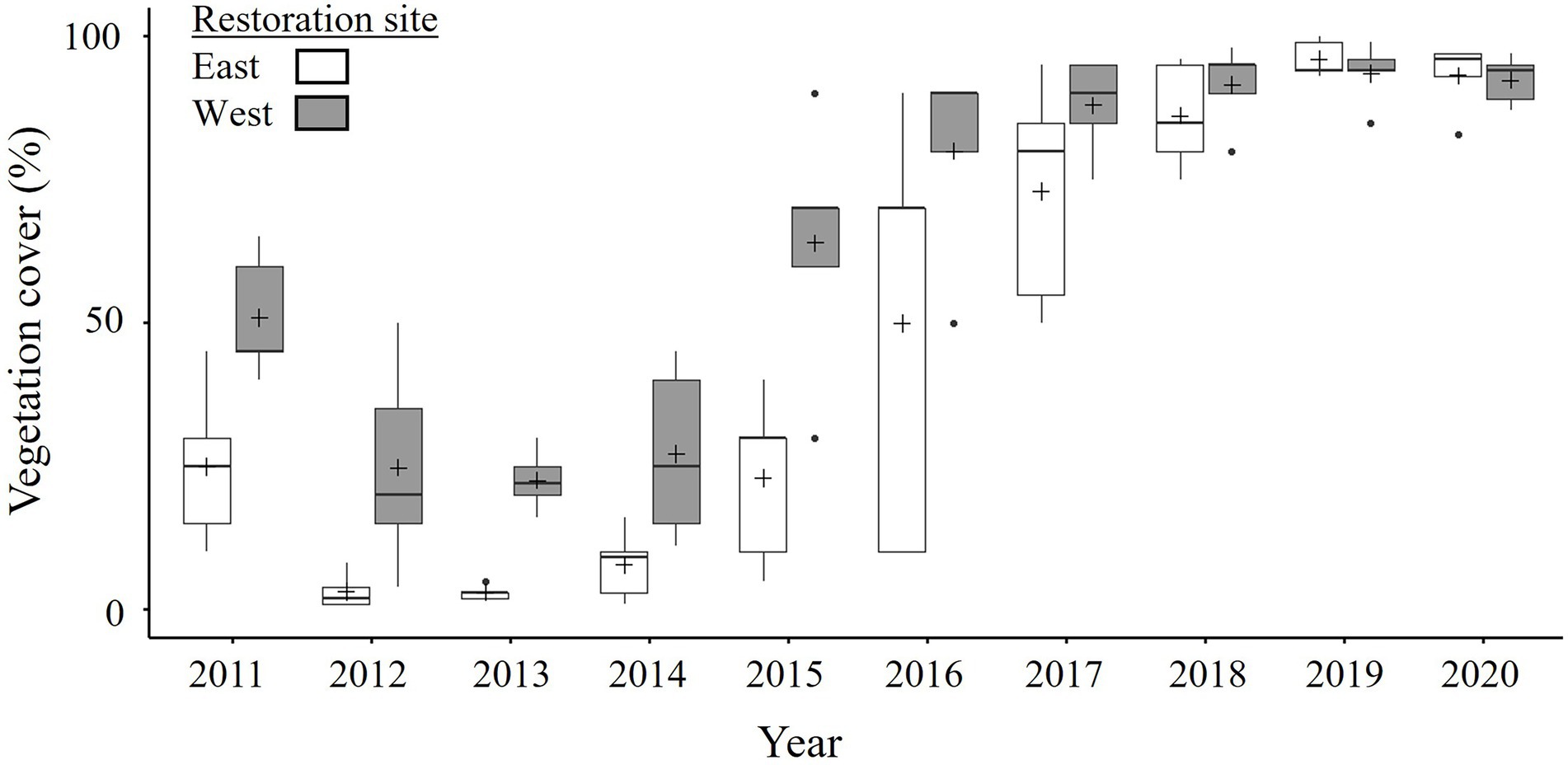
Figure 2. Estimated percent cover of vegetation in the Restoration sites in late July from 2011 to 2020 for the Aulac salt marsh complex. n = 5 strata. For boxplots, midline represents the median, + the mean, box edges the first and third quartiles, whiskers ± 1.5*interquartile range, and dots outliers. See Table 1 for ANOVA and Supplementary Table 1 .1 for pairwise comparisons.
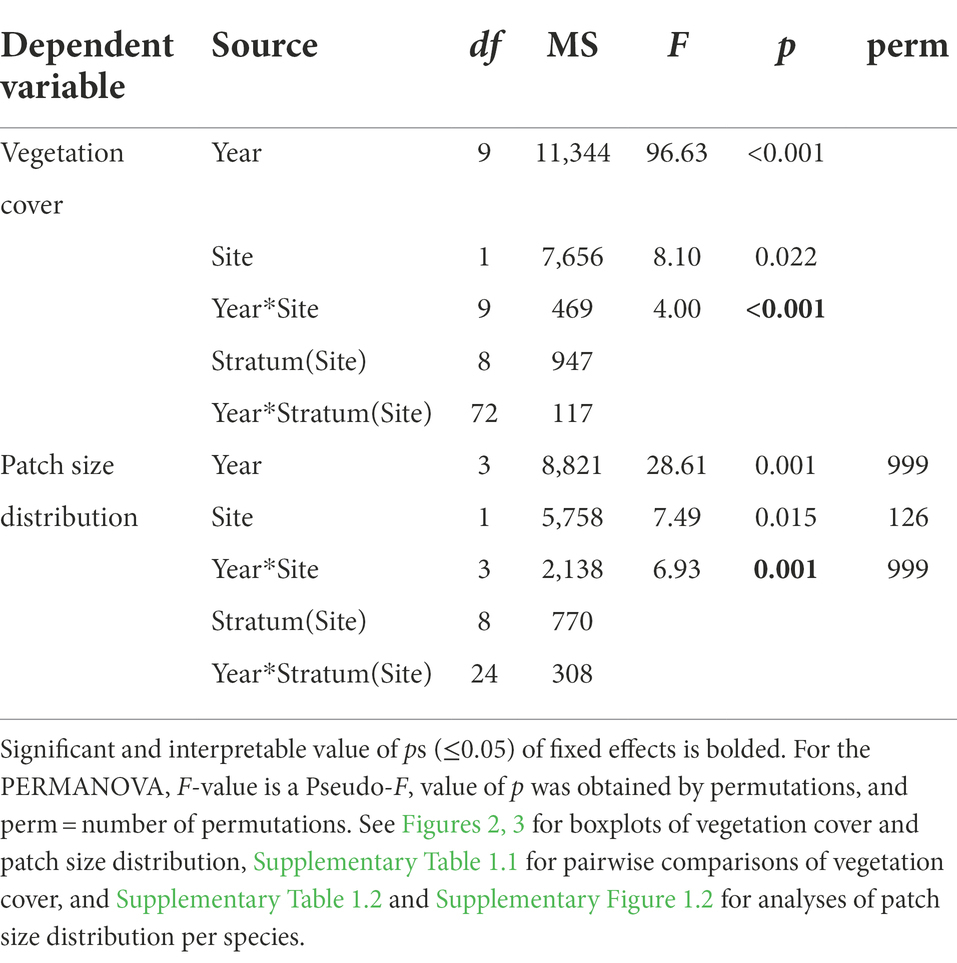
Table 1. Results for percent vegetation cover (ANOVA) and size distribution of plant patches (<0.1, 0.1–0.5, 0.5–1, 1–2, and >2 m in diameter; PERMANOVA) of Spartina spp. in the Restoration sites (East, West; each with five replicate strata) of the Aulac salt marsh complex from 2011–2020 and 2011–2014, respectively.
Initially, Spartina vegetation cover was in the form of almost circular patches. Patches of S. alterniflora expanded rapidly (see the section Spartina spread rates) before eventually merging and empty space was mainly in-filled by S. alterniflora seedlings. The patch size distribution was dominated by small patches (<0.5 m diameter) in the early years (particularly 2012; Figure 3), with a few large S. pectinata patches (>1 m diameter) in the higher elevation areas in the sites. Patch size distribution shifted to mid and large sized patches in 2013, large patches (mostly S. alterniflora) dominated in 2014, and there was widespread merging of patches (all S. alterniflora) in 2015 (Table 1; Figure 2; Supplementary Table 1.2; Supplementary Figure 1.2). Very small patches (<0.1 m) were abundant in 2014, reflecting en masse appearance of S. alterniflora seedlings that year. The two sites again showed differences (significant Year*Site interaction, p = 0.001; Table 1), with the West site having relatively more of certain sized patches than the East site in some years (Figure 3).
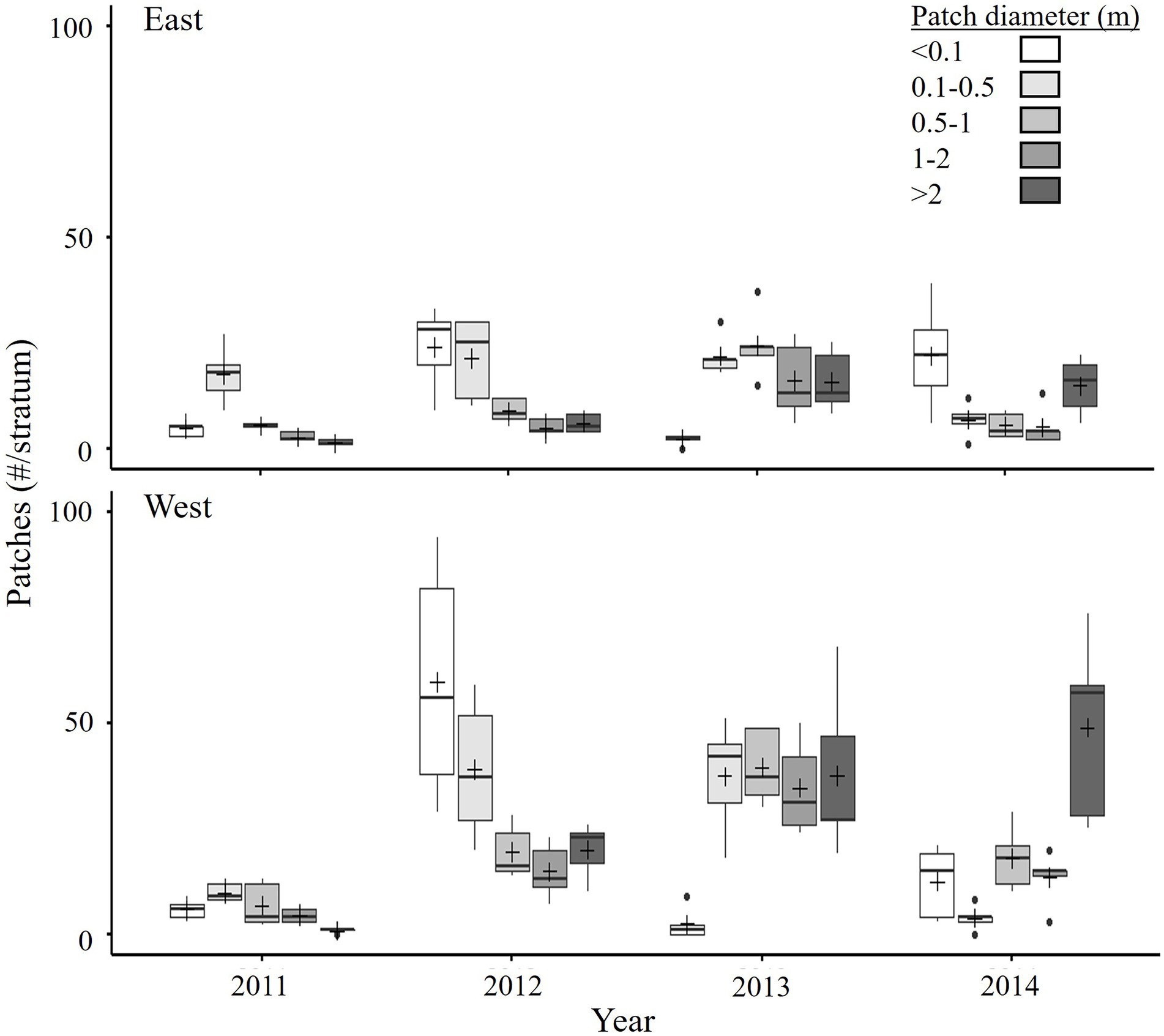
Figure 3. Estimated number of different sized patches of Spartina spp. in late July from 2011 to 2014 in the Restoration sites (East and West) of the Aulac salt marsh complex. n = 5 strata. See Figure 2 for explanation of boxplots and Table 1 for permutation Multivariate ANOVA (PERMANOVA).
Spartina spread rates
Focal Spartina pectinata patches, which survived the dike-breaching, were small in the first year of restoration (1.3 ± 0.4 m2 on 13 July 2011; n = 10 patches). In subsequent years (2012–2014), annually selected patches were 4.3 ± 1.2 m2 in mid-June. Spartina pectinata patches moderately increased in area (mean change: 75 and 45% over 90 days) in the early years’ growing seasons (2011–2012), and mostly decreased (mean change: −65 and −35%) afterwards, becoming extirpated from Restoration sites by 2015 (p = 0.001 for Year effect; Table 2; Figure 4; Supplementary Table 2.1; Supplementary Figure 2.2).
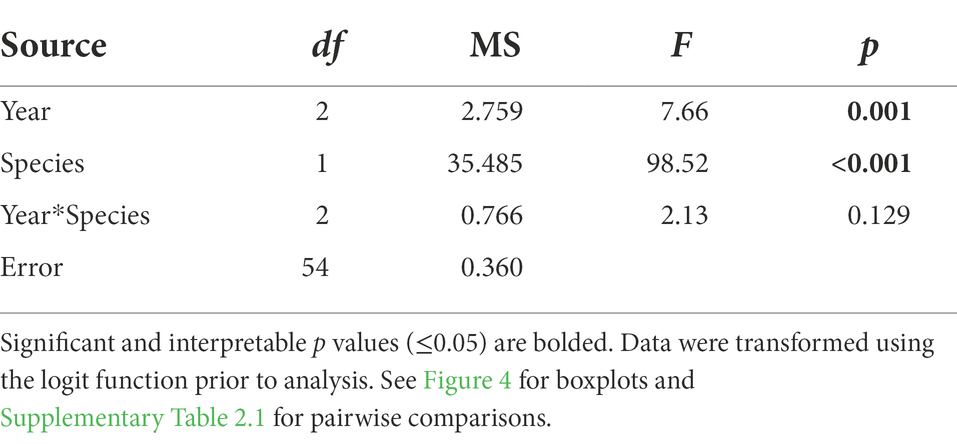
Table 2. ANOVA results for asexual growth of plant patches (% change in area) of Spartina pectinata and S alterniflora over a 90-day growing period in the Restoration sites of the Aulac salt marsh complex in June–August 2012–2014.
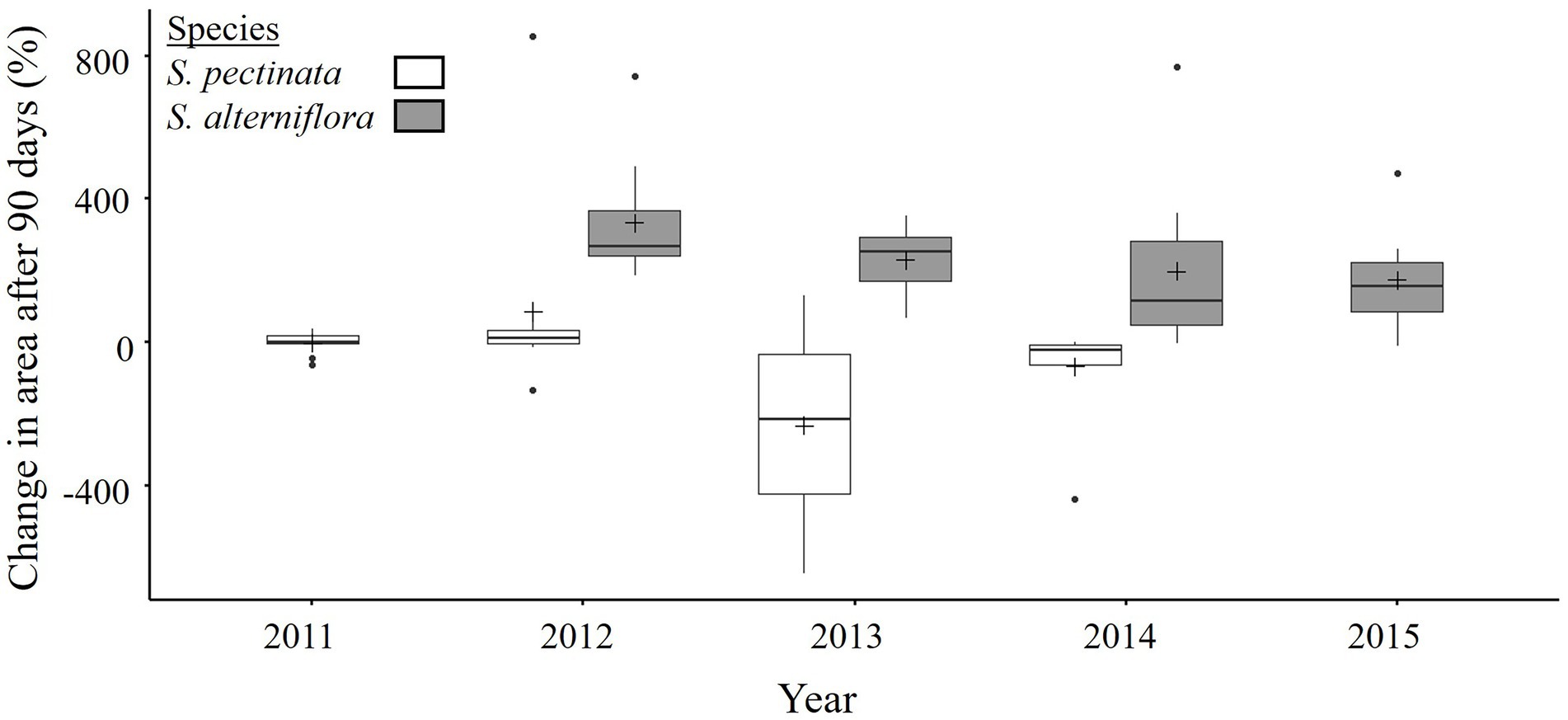
Figure 4. Change in patch area of Spartina pectinata (2011–2014) and S. alterniflora (2012–2015) in the Restoration sites of the Aulac salt marsh complex. n = 10 patches. See Figure 2 for explanation of boxplots, Table 2 for ANOVA, and Supplementary Table 2 .1 for pairwise comparisons.
Spartina alterniflora appeared in the Restoration sites in the second-year post breach (2012) as small patches (1.7 ± 0.2 m2 in mid-June 2012, Figure 3). In subsequent years (2013–2015), annually selected patches were 3.3 ± 0.5 m2 in mid-June. In contrast to S. pectinata, Spartina alterniflora patches greatly increased in area during every growing season from 2012 to 2015 (p < 0.001 for Species effect; Table 2; Figure 4). Specifically, the increase in patch area for S. alterniflora was initially very high (mean change: ~330% in 2012) then lower in subsequent years (mean change: ~230% in 2013, ~200% in 2014, and ~ 175% in 2015) as space became limited and patches began merging in 2015 (p = 0.001 for Year effect, Table 2; Figure 4; Supplementary Table 2.1; Supplementary Figure 2.2).
Spartina patens appeared on the side of the dike in 2012 (Supplement 4 in Virgin et al., 2020) but did not start to spread onto the Restoration sites proper until 2016. During the 2017–2020 growing seasons, incremental encroachment rate of dike patches of S. patens varied among years and sampling rounds (significant Year*Round interaction, p < 0.001, Table 3; Figure 5; Supplementary Table 2.2; Supplementary Figure 2.1 for photographs). The annual spread rate was highest and most consistent in 2017 (5.2 ± 1.3 cm 14 days−1, n = 36 patch-round combinations). In other years, dike patches slowly advanced (0.6 ± 1.1 cm 14 days−1 in 2018, 2.3 ± 1.2 cm 14 days−1 in 2019, and 4.2 ± 2.5 cm 14 days−1 in 2020).
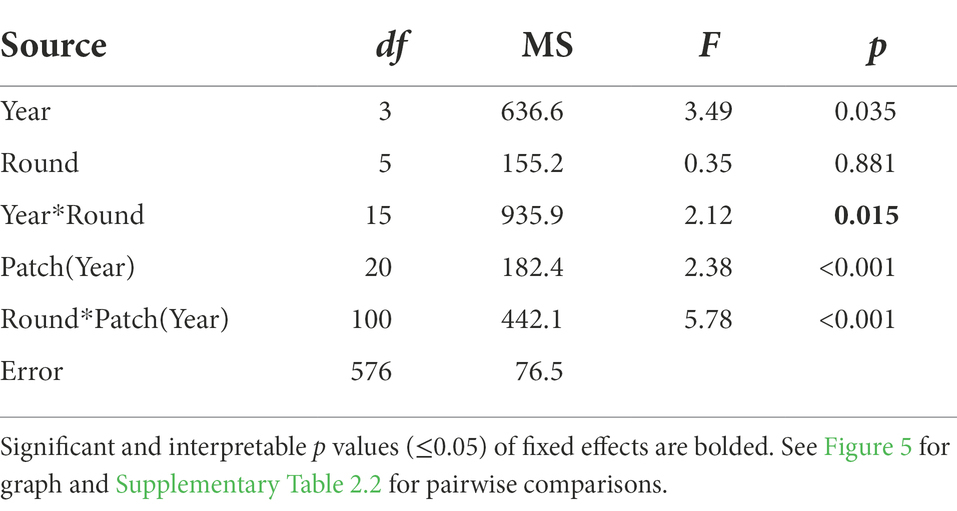
Table 3. ANOVA results for incremental distance advanced (cm 14 day−1) by dike patches of Spartina patens into Restoration sites of the Aulac salt marsh complex from June–September in 2017–2020.
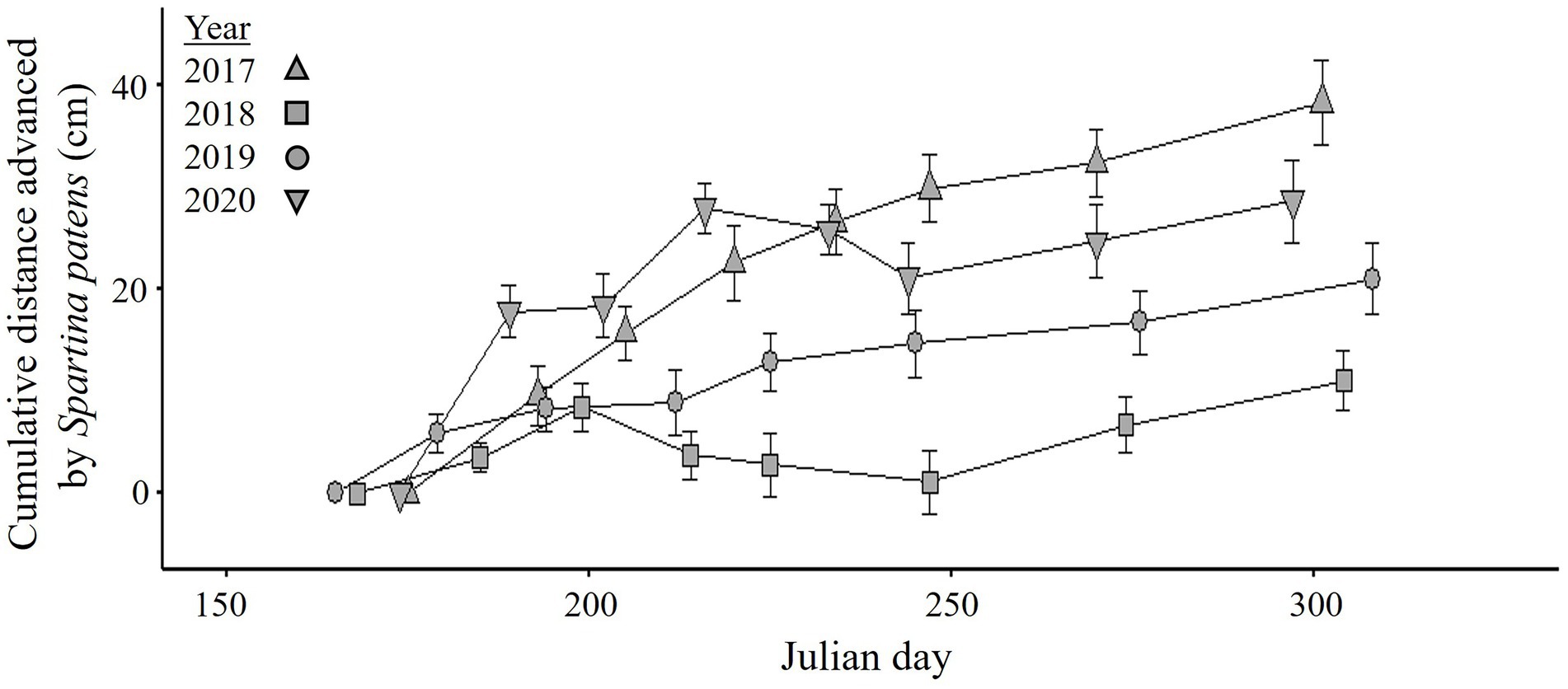
Figure 5. Mean (± SE, n = 6 patches) cumulative distance advanced of Spartina patens from the landward (new) dike onto Restoration sites of the Aulac salt marsh complex from 2017 to 2020. See Table 3 for ANOVA and Supplementary Table 2.2 for pairwise comparisons.
In 2016–2017, two isolated S. patens patches were discovered within Restoration West proper (none in Restoration East), which were ~ 2.3 m2 in mid-June 2017. One isolated S. patens patch was discovered in Restoration East in Fall 2020, and many more patches were found in Restoration West in 2021. During the 2017–2022 growing seasons, isolated patches moderately increased in area by 50 ± 11% over 90 days (mean ± SE, n = 15 patch-year combinations; range-15–149%; Supplementary Figure 2.3).
Spartina pectinata plant dynamics
In the first year post-breach, Spartina pectinata plants in Restoration sites had live stem densities (303 ± 54 stems m−2 measured on September 8, 2011, n = 10 patches) and percent flowering (25 ± 7%) similar to those generally observed in the Reference site [e.g., 210 ± 14 stems m−2 and 15 ± 3%, n = 4 patches, measured a later year (on 19 August 2014); Figure 6] but were stunted at ~50 cm height (MA Barbeau, personal observation). In subsequent years, live stem density and percent flowering of restoration S. pectinata greatly decreased [e.g., 61 ± 10 stems m−2 (p < 0.001 for year effect) and 4 ± 1% (p = 0.023), respectively, on August 19, 2014], being lower than in the Reference site in 2013–2014 (Table 4, Figure 6, Supplementary Tables 3.1–3.5, Supplementary Figure 3.1). Canopy height of S. pectinata remained stunted at ~1/3 that in reference locations (e.g., 40 ± 3 cm vs. 111 ± 7 cm tall on August 19, 2014). Spartina pectinata in the Restoration sites were exposed to much higher soil salinity (51 ± 3 mg salt cm−3 dry sediment; estimated at 43 ppt based on water content of collected sediment cores, n = 24 patches) than those growing in the Reference sites (11 ± 2 mg salt cm−3 dry sediment; estimated at 7 ppt, n = 6 patches; measured in 2013, p < 0.001 for Patch type effect, Supplement 5), likely contributing to their stressed condition. In addition, examination of S. pectinata root masses in 2013 indicated more dead roots per unit volume of sediment, and so a lower live to dead root ratio, for patches in Restoration sites (3.6 ± 0.8 mg dead roots cm−3, and a ratio of 1.3 ± 0.4:1, n = 6 patches) than in the Reference site [2.6 ± 0.7 mg dead roots cm−3 (p = 0.007 for Patch type effect) and a ratio of 5.2 ± 2.5:1 (p < 0.001); Supplement 6].
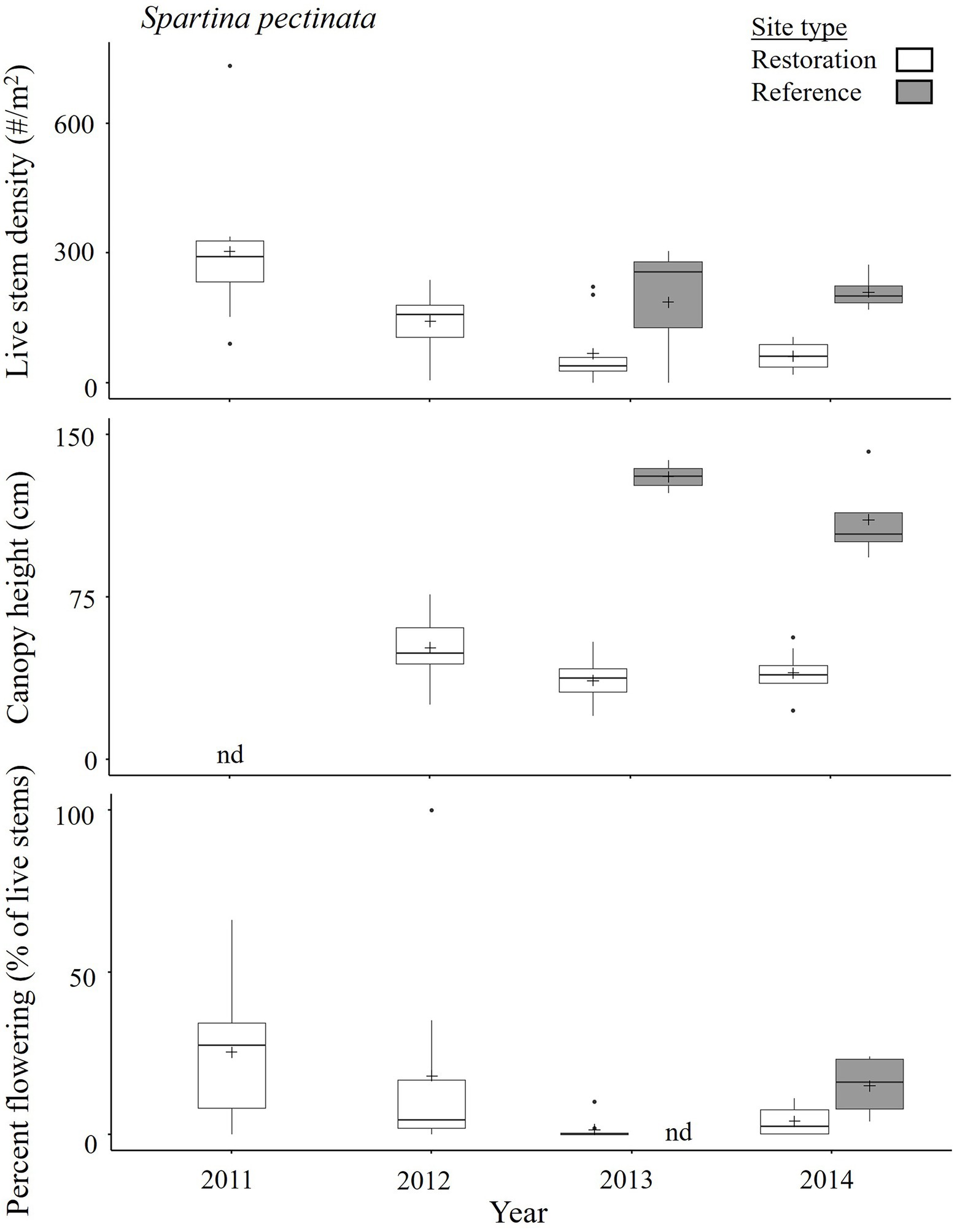
Figure 6. State of Spartina pectinata in late August/early September in the Restoration and Reference sites of the Aulac salt marsh complex from 2011–2014. In Restoration sites, n = 8–10 patches; for Reference sites, n = 3–4 patches; nd = no data. Spartina pectinata was not monitored in the Reference sites in 2011–2012, and canopy height was not recorded in the Restoration sites in 2011. See Figure 2 for explanation of boxplots, Table 4 for ANOVAs, and Supplementary Table 3.3 for pairwise comparisons.
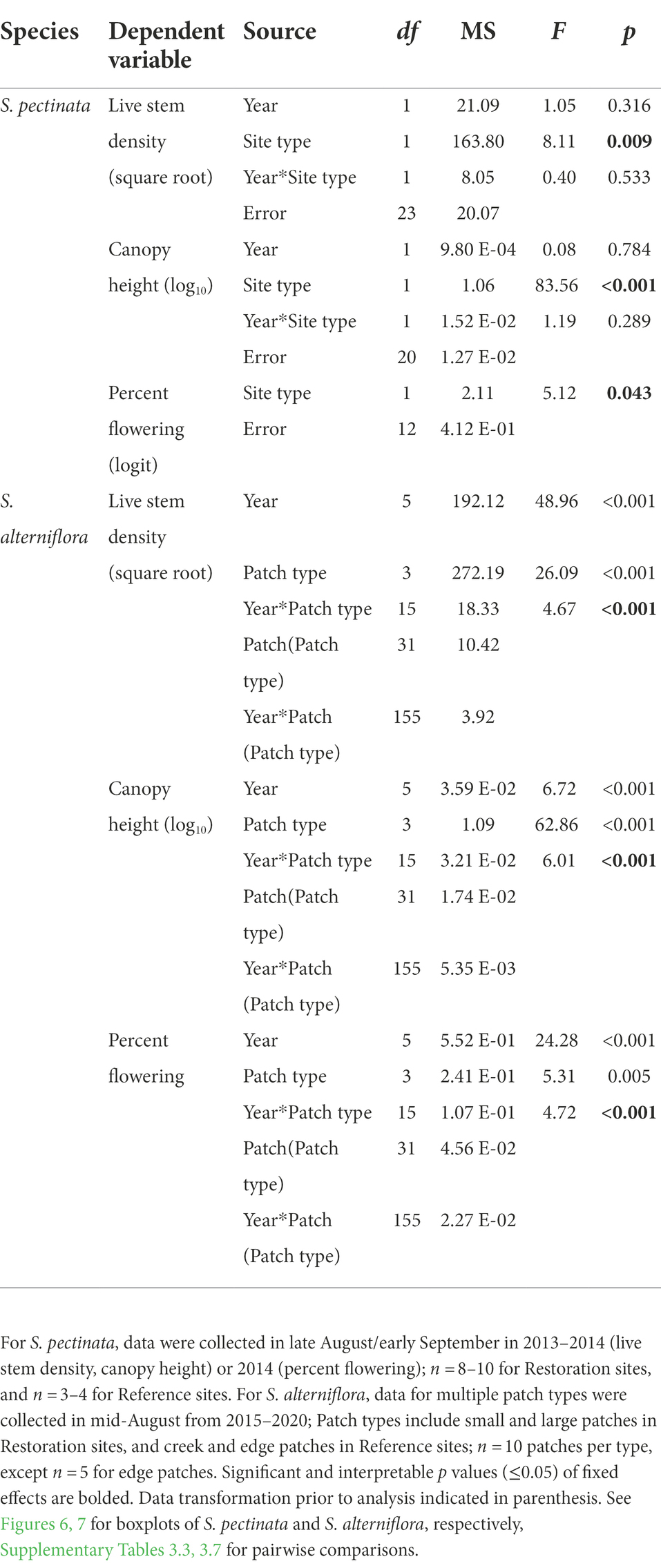
Table 4. ANOVA results for live stem density (# stems m−2), canopy height (cm), and percent flowering (% of live stems flowering) of Spartina pectinata and S. alterniflora in Restoration and Reference sites (i.e., two site types) in the Aulac salt marsh complex over years.
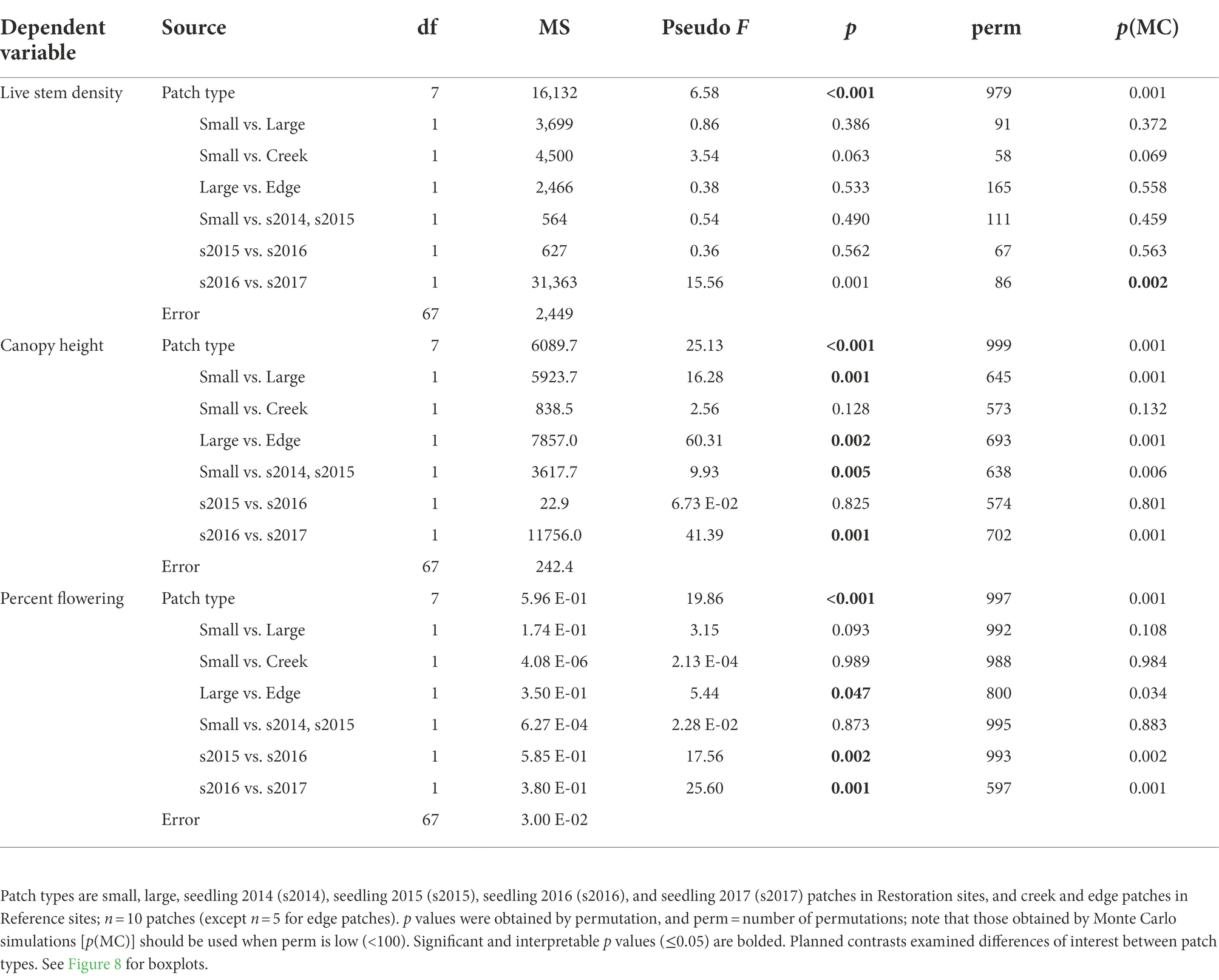
Table 5. PERMANOVA results for effect of patch type on each of live stem density (# stems m−2), canopy height (cm), and percent flowering (% of live stems flowering) of Spartina alterniflora in Restoration and Reference sites in the Aulac salt marsh complex on 22 August 2017.
Spartina alterniflora plant dynamics
In the earlier years of restoration (2012–2015), small patches of S. alterniflora had lower live stem densities (e.g., 202 ± 91 stems m−2 on August 12, 2014, n = 10 patches) and lower or similar percent flowering (11 ± 3%) than reference plants [whether creek: 471 ± 38 stems m−2 and 12 ± 2% (n = 8 patches), or edge patches: 544 ± 51 stems m−2 and 35 ± 14% (n = 4 patches), p = 0.048 for Year*Patch type interaction], and a canopy height (80 ± 6 cm) that was intermediate between the reference creek plants (tall-form, 107 ± 6 cm) and edge plants (short-form, 50 ± 7 cm; p < 0.001 for Patch type effect; Supplementary Tables 3.1, 3.6; Supplementary Figure 3.2). In later years (2016–2020), live stem density (e.g., 210 ± 10 stems m−2 on August 22, 2017, n = 10 patches) and canopy height (115 ± 8 cm) of restoration patches that were small in 2015 reached the density (180 ± 12 stems m−2, n = 10 patches) and canopy height (102 ± 3 cm) of reference tall-form plants (significant Year*Patch type interaction for all response variables, p < 0.001, Table 4; Figure 7; Supplementary Table 3.9; Supplementary Figure 3.2). The reference short-form plants had higher live stem densities (264 ± 30 stems m−2, n = 5 patches) and shorter canopy heights (32 ± 4 cm). Large patches, which appeared and became common in the mid-years (2014–2015), within the Restoration sites were similar to reference tall-form plants in 2015–2016 (e.g., 237 ± 9 stems m−2 and 94 ± 6 cm on August 13, 2015). However, they started to approach the short-form state as live stem density increased (e.g., 254 ± 23 stems m−2 on August 12, 2019) and canopy height decreased (57 ± 3 cm) in the later years (2017–2020), which is associated with shorter distances from the seaward edge of the marsh (Supplement 7). Note that the large patches tended to be located more seaward on the Restoration sites than small patches, and so more exposed to wave and wind stress since the almost complete erosion of the old dike in 2016. Percent flowering of small and large restoration patches in 2016–2020 showed variation but were within the range of reference plants (restoration: 32 ± 4 and 29 ± 3% flowering for small and large patches, respectively, averaged over mid-to late August 2016–2020; reference: 35 ± 3 and 15 ± 2% flowering for creek and edge patches, respectively).
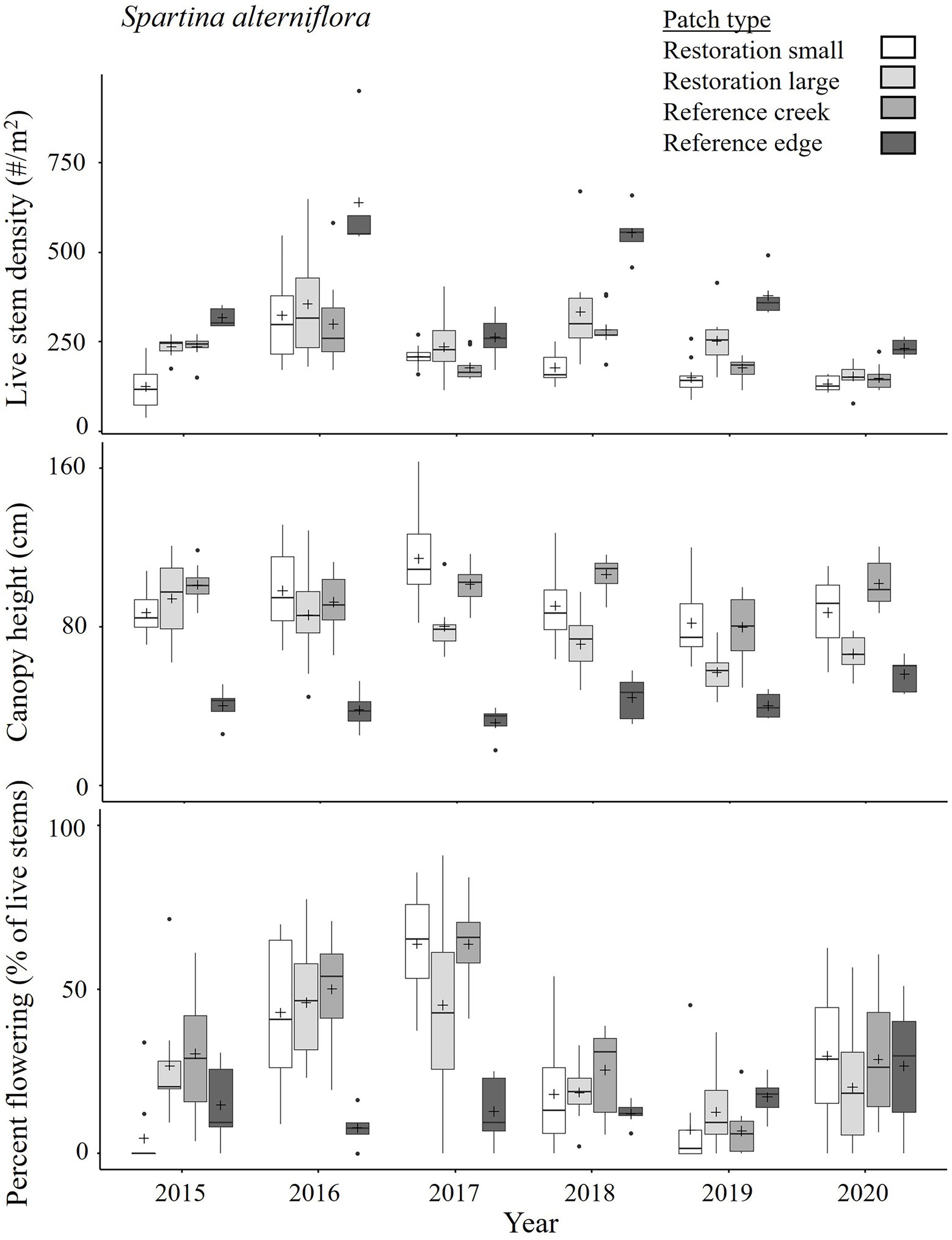
Figure 7. State of Spartina alterniflora in mid-August in established patches in Reference and Restoration sites of the Aulac salt marsh complex from 2015 to 2020. n = 10, except n = 5 for Reference edge patches. See Figure 2 for explanation of boxplots, Table 4 for ANOVAs, Supplementary Table 3.7 for pairwise comparisons, and Supplementary Figure 3.2 for more detailed plant dynamics.
Spartina alterniflora seedlings (Supplementary Figure 3.3) first appeared en masse in 2014 in the Restoration sites, reflecting increased seed supply from locally established patches, and availability of suitable space (with appropriate marsh surface elevation, Supplement 8). Seedings continued to appear in good numbers until 2017, when availability of free space greatly diminished (Figures 1, 2). Young-of-the-year S. alterniflora had lower live stem densities (1/3–2/3 lower, e.g., 114 ± 14 stems m−2 on August 22, 2017, n = 10 patches; Figure 8) during the growing season than established restoration patches and reference patches (p < 0.001 for Round*Patch type interaction, Supplementary Tables 3.4, 3.8; p < 0.001 for Year*Patch type interaction, Supplementary Tables 3.9, 3.10; Supplementary Figures 3.2, 3.4). Canopy height of seedling patches near the end of the growing season (e.g., 49 ± 4 cm on August 22, 2017) was similar to reference short-form plants, and about half that of established restoration patches and of reference tall-form plants. Furthermore, young-of-the-year plants did not flower. By the fall, collected specimens indicated that most started to reproduce asexually (Supplementary Figure 3.3). Early in their second year (June), the young plants are easily distinguishable from new seedlings and from older plants, because they are both robust-looking and singlets (Supplementary Figure 3.3). They had a live stem density (e.g., 193 ± 15 stems m−2 on August 22, 2017) and canopy height (98 ± 6 cm) approaching that of established restoration patches and reference tall-form plants, but their percent flowering was on average lower (1/3–1/2 lower; 28 ± 5% on 22 August 2017; Figure 8; Supplementary Tables 3.4, 3.8–3.10; Supplementary Figures 3.2, 3.4). By their third year, former seedling plants were similar in stem density (e.g., 204 ± 11 stems m−2 on August 22, 2017), canopy height (100 ± 5 cm), and flowering (62 ± 6%) to established restoration patches and reference tall-form plants.
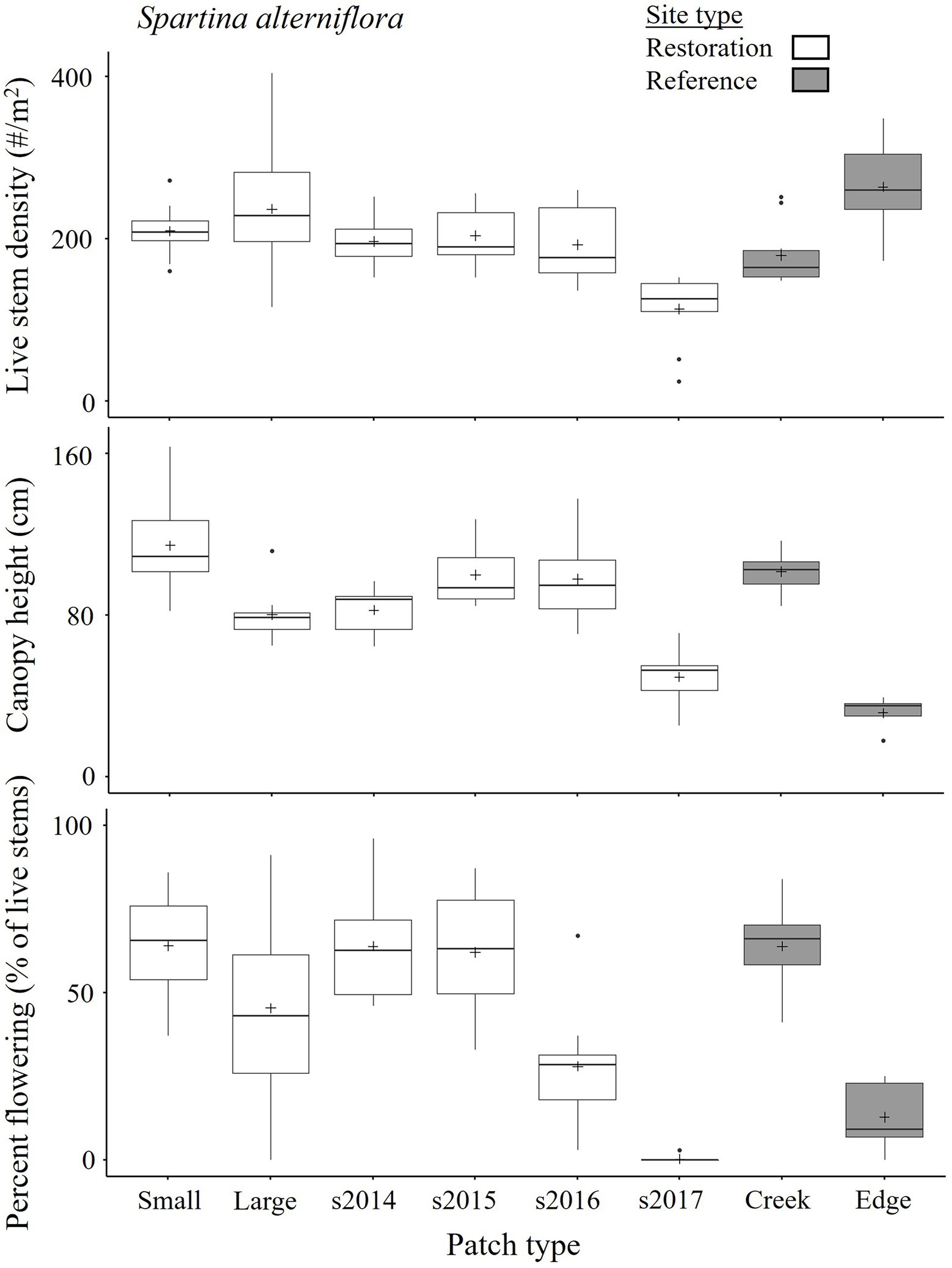
Figure 8. State of S. alterniflora in established and seedling patches in the Restoration and Reference sites of the Aulac salt marsh complex on August 22, 2017. Patch types labeled as a year (e.g., “s2014”–“s2017”) are young-of-the-year from said year. n = 10 patches, except n = 5 for Reference edge patches. See Figure 2 for explanation of boxplots, Table 5 for PERMANOVAs, and Supplementary Figures 3.2, 3.4 for more detailed plant dynamics.
Dynamics over a growing season (presented for 2016 and 2017 as examples) showed that live stem density for plants that were not young-of-the-year started relatively high in mid-June (e.g., 613 ± 135 and 649 ± 114 stems m−2 for restoration small and large patches, respectively, measured in June 2016, n = 10 patches:), decreased over the growing season (325 ± 42 and 357 ± 53 stems m−2 for restoration small and large patches measured on August 24, 2016), likely due to self-thinning (Roderick and Barnes, 2004), and had a small peak in early fall (338 ± 32 and 396 ± 52 stems m−2 for restoration large and small patches measured on 24 September 2016) with appearance of small stems that were produced asexually (significant Round*Patch type interaction, p < 0.001; Supplementary Table 3.4; Supplementary Figure 3.4). Young-of-the-year began the growing season with low live density (e.g., 90 ± 18 stems m−2 measured in mid-June 2016) and increased over the growing season as new seedlings continued to emerge in the summer (164 ± 24 stems m−2 measured on 24 August 2016), and as asexually produced stems started to appear in early fall (302 ± 23 stems m−2 measured on 24 September 2016; Supplementary Figure 3.3). The stems and leaves of all plant types senesced and died in mid to late fall (see also Baerlocher and Moulton, 1999). Canopy height for plants that were not young-of-the-year rapidly increased from June (e.g., 36 ± 4 cm and 33 ± 3 cm for restoration small and large patches in 2016) to July (72 ± 6 cm and 59 ± 3 cm of restoration small and large patches) and leveled off in August (98 ± 7 cm and 86 ± 8 cm for restoration small and large patches; significant Round*Patch type interaction, p < 0.001; Supplementary Tables 3.4, 3.8; Supplementary Figure 3.4). Young-of-the-year grew sigmoidally (6 ± 0.5 cm in mid-June 2016), surpassed the height of reference short-form plants by end of August (47 ± 6 cm measured), and leveled off in September (55 ± 4 cm measured). All plant types showed a decreased canopy height in mid-fall as taller shoots died and remaining live shoots were measured. Flowering typically started toward the end of July, peaked in late-August/early-September (e.g., 60 ± 7 and 52 ± 10% of live stems flowering of small and large patches measured in early September 2016), and was finished by end of September. Two-year old plants started to flower in August and flowered less than more established plants (~130% less than four-year-old plants in August 2017). Note that reference short-form (edge) patches had high yearly variation in percent flowering (Supplementary Figure 3.4); in 2016 and 2017, percent flowering was relatively low.
Spartina alterniflora root masses in 2013 had a higher live to dead root ratio in Restoration sites (12.9 ± 4.5:1 on August 20, 2013, n = 6 patches; at the time, S. alterniflora patches were all small) than reference creek (1.0 ± 0.1:1) and edge (2.1 ± 0.4:1) patches (p < 0.001 for Patch type effect; Supplementary Tables 6.1, 6.2). Restoration live root mass per unit volume of sediment (2.7 ± 0.8 mg cm−3) was equivalent to reference tall-form (creek) plants (2.7 ± 0.8 mg cm−3), and dead root mass was lower (0.4 ± 0.1 mg cm−3) than both reference patch types (2.3 ± 0.6 and 5.9 ± 2.0 mg cm−3 for creek and edge, respectively; p = 0.002 and 0.007 for Patch type effect when testing differences in live and dead root masses, respectively; Supplementary Tables 6.1, 6.2). Spartina alterniflora was growing in sediments (measured in 2013) with somewhat higher salt content in the restoration patches (50 ± 4 mg salt cm−3 dry sediment; estimated at 41 ppt; n = 12 patches) than in reference creek patches (40 ± 3 mg salt cm−3 dry sediment, estimated at 29 ppt; though the salinity difference was not significant, Supplementary Tables 5.1, 5.2).
Spartina patens plant dynamics and possible interaction with Spartina alterniflora
Spartina patens growing along the dike (observed since 2012) were well-established and resembled reference S. patens in live stem density (e.g., 3,376 ± 537 and 3,275 ± 217 stems m−2, respectively, n = 6–10 patches, measured in August 2018), canopy height (66 ± 6 cm and 54 ± 2 cm), and percent flowering (3 ± 2 and 4 ± 1%) when measured in August 2017–2020 (p > 0.30 for Patch type effect on stem density and percent flowering; post-hoc p > 0.05 for Patch type effect on canopy height; Supplementary Tables 3.11, 3.12; Supplementary Figure 3.5). The isolated S. patens patches discovered growing within Restoration West (in 2016–2017) tended to have lower live stem density (e.g., 2,360 ± 584 stems m−2 in August 2018, n = 2 patches, though not significant) and had shorter canopy height (37 ± 9 cm; p < 0.001 for Patch type effect) for plants growing in their center than S. patens growing on the dikes and in Reference sites (Supplementary Tables 3.11, 3.12; Supplementary Figure 3.5).
Shoots of S. patens growing along the inner edges of S. patens dike patches and isolated patches, where they could be interacting with S. alterniflora, had similar live stem densities [1,179 ± 388 and 1,616 ± 688 stems m−2 in August 2018 for inner edges of dike patches (n = 3) and inner edges of isolated patches (n = 2), respectively], canopy height (58 ± 7 cm and 43 ± 9 cm), or percent flowering (1 ± 1 and 4 ± 4%) compared to shoots growing more centrally in the patches (3,056 ± 371 and 2,360 ± 584 stems m−2, 58 ± 5 and 37 ± 9 cm, and 0 ± 0 and 16 ± 16%, for dike and isolated patches, respectively; Supplementary Tables 4.1, 4.2; Supplementary Figure 4.1). The S. patens shoots growing just beyond the outside edges of the isolated patches, compared to shoots growing on the inside edges mentioned above, had lower live stem density (216 ± 64 stems m−2), were shorter (35 ± 7 cm), and did not flower (p = 0.005 and p < 0.001 for Round*Patch type for live stem density and canopy height, respectively; Supplementary Tables 4.1, 4.2; Supplementary Figure 4.1). Spartina alterniflora plants growing along the edges of the dike patches and isolated patches of S. patens generally had lower live stem densities (89 ± 8, 148 ± 60, and 164 ± 28 stems m−2), shorter canopy height (52 ± 13 cm, 67 ± 6 cm, and 58 ± 4 cm), and less percent flowering (0 ± 0, 8 ± 1, and 9 ± 3% for patches on the dike edges, the inner edges of isolated patches, and the outer edges of isolated patches, respectively, in August 2018) than those growing in monoculture S. alterniflora areas (183 ± 20 and 292 ± 40 stems m−2, 92 ± 6 and 70 ± 7 cm, and 26 ± 9 and 14 ± 4% percent of live stems flowering, for restoration small and large patches in Restoration West, respectively; n = 10 patches; p = 0.006, p = 0.003, and p < 0.001 for Round*Patch type for live stem density, canopy height, and percent flowering, respectively; Supplementary Tables 4.1, 4.3; Supplementary Figure 4.2).
Discussion
We collected detailed measurements over 10 years of Spartina plant states and patch dynamics that underlie vegetation recovery patterns at two sites in the Aulac salt marsh restoration project in the upper Bay of Fundy. We estimated modes of colonization of S. alterniflora and S. patens, measured asexual spread of S. pectinata, S. alterniflora, and S. patens; monitored seedling production of S. alterniflora; began quantifying interactions between S. alterniflora and S. patens; and began exploring how spatial distribution within the sites affected S. alterniflora health-related states. In the following discussion, we first briefly examine the observed site-level patterns, and then discuss the patch-level processes in terms of dynamics, ecological insights, restoration implications, and planned research.
Recovery patterns of the salt marsh vegetation community in Aulac
Following breaching of the old agriculture dike in 2010 (Boone et al., 2017; Virgin et al., 2020), site-level vegetation patterns reflected initial persistence, then decline, and disappearance of S. pectinata in under 5 years, followed by spread of S. alterniflora in the mid years, and of S. patens in the later years (Virgin et al., 2020). Vegetation recovery has followed secondary, progressive succession where site-level community changes are expected to eventually result in spatial distributions of the vegetation communities observed in the reference sites (i.e., climax condition of salt marshes in the region). During other salt marsh restoration projects in New England and the Bay of Fundy, freshwater and brackish vegetation typically disappears quickly (<6 years; Burdick et al., 1996; van Proosdij et al., 2010; Smith and Warren, 2012). Increasing cover of halotolerant vegetation during salt marsh restoration typically begins with species that are more tolerant to inundation and salinity (e.g., S. alterniflora, but also succulent forbs including Suaeda and Salicornia spp.; Virgin et al., 2020), followed by species less tolerant to both (e.g., S. patens; Redfield, 1972; Mossman et al., 2012). In Aulac, S. alterniflora most likely colonized the restoration sites via rhizomal material brought into the sites by the tide and ice blocks during winter (based on observations of ice blocks and their content; van Proosdij et al., 2006; Boone et al., 2017) and lack of observed seedlings in the early years. After the sites were colonized, S. alterniflora spread asexually before seedlings were observed in the project’s fourth year (2014). Asexual spread was rapid during our project (nearing complete cover after 9 y) as has been reported in regions where S. alterniflora is considered native (Trilla et al., 2009) and invasive (Taylor and Hastings, 2004; Zhang et al., 2017). While recovery of vegetation communities was rapid in both Aulac restoration sites, differences in site dynamics likely reflected an initial lower surface elevation of the East site (Boone et al., 2017) and differing (though high) rates of sediment deposition (Virgin et al., 2020); this demonstrates that subtle differences in site conditions can affect vegetation recovery dynamics. A restoration project in Maine, United States, reached 70% of halotolerant vascular plant cover in 6 years (Burdick et al., 1996), and another in the Bay of Fundy reached 100% cover in 3 years (van Proosdij et al., 2010). Attaining high salt marsh vegetation percent cover is quite fast in the Bay of Fundy (<6 years), likely due to high deposition of sediment and vegetation propagules (van Proosdij et al., 2010; Roman and Burdick, 2012; Virgin et al., 2020) resulting from large tidal amplitudes. Furthermore, in this and other restoration projects to date in the Bay of Fundy (Bowron et al., 2012; Norris et al., 2020), it has been observed that recovery of vegetation appears most rapid if the tidal waters move over established marsh before entering a site to be restored, likely because this is a direct source of vegetation propagules. In other geographic locations, especially in areas with small tidal amplitudes, high vegetation percent cover may take more than 10 years (Burdick et al., 1996; Garbutt et al., 2006; Weinstein et al., 2019). Currently, we are observing a gradual spread of S. patens into the restoration sites from high elevation areas, including the dike edge and isolated patches within the sites. We forecast continued spread and eventual dominance of S. patens, coinciding with ongoing sediment deposition and elevation increase, which will competitively displace S. alterniflora to lower elevation areas (Bertness, 1991) and lead to the expected plant zonation of S. alterniflora occupying low elevation marsh areas and S. patens dominating higher elevations.
Processes underlying the early phases of salt marsh restoration and their implications
Development of vegetation patterns during salt marsh recovery can be described in phases, which is useful when examining underlying patch-level processes. The first and second phases of salt marsh restoration in Aulac were the high initial sediment deposition occurring immediately after breaching (phase 1, year 1) and the loss of S. pectinata and colonization and spread of S. alterniflora (phase 2, years 2–5; see Virgin et al., 2020). Competition between brackish and halotolerant species (e.g., Phragmites sp. and Typha sp. vs. S. alterniflora; Roman et al., 1984; Barrett and Niering, 1993) has played a substantial role in successional dynamics of vegetation communities during previous salt marsh restorations. During the second phase of our salt marsh restoration project, the disappearance of S. pectinata and proliferation of S. alterniflora was the probable result of inappropriate environmental conditions for the former species, and ample suitable habitat for the latter, rather than interspecific competition. Soil water salinity of the sites was considered lethal for S. pectinata (>0.2 M NaCl or 12 ppt; Warren et al., 1985), but suitable for S. alterniflora (Webb, 1983; Betzen et al., 2019). Furthermore, the availability of empty and suitable habitat would have minimized competition between S. pectinata and S. alterniflora (Craine and Dybzinski, 2013). The two species were located far apart in the restoration sites, with surviving S. pectinata occupying the high elevation areas of the sites (see year 1 aerial photograph in Figure 1; marsh surface elevation profiles presented in Supplementary Figure S1.1 of Virgin et al., 2020), and S. alterniflora colonizing bare areas at intermediate elevations. Thus, conversion from S. pectinata to a S. alterniflora-dominated area was driven by interactions with their environment (or edaphic conditions), and not by competitive interactions between species.
We initially expected the colonization and spread of S. alterniflora would be from high elevation dike edges, because S. alterniflora grows most successfully in non-halophytic conditions with little competition (Bertness, 1991). Instead, we observed circular colonies (patches) of S. alterniflora dotting the sites, which had the tall-form phenotype when they matured. The vigorous spread by S. alterniflora was possible likely due to appropriate elevational range of most of the sites’ surface (Proffitt et al., 2003) and high nutrient content of the soil (Keizer et al., 1989; Langley et al., 2013). The S. alterniflora seedlings in 2014 (year 4) must have been produced by the healthy-looking mature plants in the restoration sites in 2013, which we observed bearing seeds in addition to dense batches of seeds on the mud surface. Other studies have reported seedling colonization in the first-year post-restoration (van Proosdij et al., 2010), although seedling establishment of Spartina spp. can be erratic (Callaway and Josselyn, 1992). Clonal species are more often thought to transition from mostly sexual to asexual reproduction when establishing in a new site (Silvertown, 2008), which is opposite to what we observed during the Aulac restoration. In contrast to sites that are up tidal channels or are landward of established salt marshes, the Aulac sites face the open Bay of Fundy (Cumberland Basin), and we suspect that seeds would not have been readily brought in by tidal water. Furthermore, the Aulac sites’ surface elevation may not have been suitable for the successful germination of seeds until the fourth year of the project. Indeed, we observed that seedlings in 2014 occurred within a narrow range of elevation (Supplement 8). Also, very high sedimentation rates in the early years [as much as 50 cm in the first year, averaging 18.3 ± 3.8 cm (mean ± SE, n = 20 plots) in Restoration East and 10.8 ± 1.4 cm (n = 27 plots) in Restoration West; Virgin et al., 2020] may have contributed to lack of germination if seeds were present; S. alterniflora germination rates decrease when buried under too much sediment (Zhao et al., 2020). In addition, our later monitoring of S. alterniflora patches that started from young plants indicated that it takes 2–3 years for the plants to flower substantially, supporting the claim that onsite plants would have started producing substantial amounts of seeds only at the end of the 2013 growing season. As the typical ecosystem engineer species of salt marsh on the east coast of North America, the colonization and initial asexual and sexual spreads of S. alterniflora were considered crucial for the successful development of salt marsh habitat during our restoration project.
Our process-related observations and attendant ecological insights during the early phases (particularly phase 2, Virgin et al., 2020) of the restoration suggest three restoration implications. First, the initial presence of S. pectinata onsite (present before and after the breaching), along with S. alterniflora which appeared a year later, likely aided in binding sediments (Neumeier and Ciavola, 2004) and preventing erosion, which has been reported during failed restoration projects where retrogressive succession results in the failure of salt marsh establishment (French et al., 2000). Spartina pectinata has not previously been thought of as a good temporary species facilitating the initial development of a salt marsh. Due to its potential value as a facilitator and lack of competition with S. alterniflora, we propose that S. pectinata is a good temporary plant when initiating a salt marsh restoration and assists in salt marsh development in the first 3–4 years. This idea should be tested in a field experiment conducted concurrently with a planned restoration, in which presence/absence of S. pectinata is manipulated. Second, the appearance of S. alterniflora at our Aulac sites was delayed compared to other salt marsh restoration projects in the Bay of Fundy. When considering where to breach an old dike, we suggest that the presence of an established salt marsh seaward of the restoration site should be included in the list of criteria of the breach (see Boone et al., 2017). This would provide an immediate source of vegetation propagules brought in by tidal water. This idea could be tested further by studying the effect of breach location relative to surrounding established salt marsh on initial vegetation recovery, as well as in a spatial modeling exercise examining the importance of input rate of S. alterniflora propagules relative to other processes in the vegetation recovery. Third, at north temperate latitudes, ice blocks are a vector of colonization for various organisms as well as a source of sediment transport (van Proosdij et al., 2006; Macfarlane et al., 2013; Lundholm et al., 2021). The importance of ice blocks for sediment transport had been considered in planning the breaches in Aulac (i.e., by making the breaches large enough to allow ice in; Boone et al., 2017). However, we had not originally considered ice blocks to be a primary mode of S. alterniflora colonization for our sites. This idea could be assessed by quantifying density of ice blocks appearing on site and proportion of ice blocks with marsh plant rhizomal material. This information could then be used to parameterize the input rate of S. alterniflora propagules in a spatial model. The size distribution of plant patches outputted from the model and observed in the field could then be compared. To summarize, three implications were suggested during the early restoration phases (particularly phase 2, the decline and eventual disappearance of S. pectinata and colonization and spread of S. alterniflora) which, if considered while planning future restoration projects, could speed the appearance of the later phases.
Processes underlying the later phases of salt marsh restoration and their implications
The third phase (years 6–10) of salt marsh restoration in Aulac was characterized by the dominance of S. alterniflora with the continued merging of patches and infilling by seedlings, resulting in decreased spatial variation and homogenization of vegetation density and diversity (Supplementary Figure 1.3; Virgin et al., 2020). The production of S. alterniflora seeds and subsequent germination into seedlings were important processes underlying the spatial vegetation pattern up until year 8 (2018), at which time free space became vanishingly small, and very low densities of seedlings were observed, mostly interspersed among mature plants. We stopped establishing and monitoring new seedling patches that year (2018) because there were almost none. Mature plants were still producing seeds, but sexual reproduction was no longer an important process affecting spatial patterns. Seeds likely had low germination success because of light and space limitations, i.e., intraspecific competition with established plants (Metcalfe et al., 1986). Our results suggest that successful spread by sexual reproduction of S. alterniflora was related to availability of space, in addition to appropriate edaphic conditions. Any spread of S. alterniflora in the later part of the third phase appeared mostly through asexual production of shoots either from plants in the older established patches or from past seedlings which were creating shoots asexually starting in their second year of life. Thus, the two modes of reproduction (asexual and sexual) were highly complementary with their relative importance changing as the recovery proceeded and led to the rapid spread and domination of S. alterniflora throughout the sites. A restoration implication of this is that transplanting S. alterniflora tillers or plugs of seedlings (e.g., grown in greenhouse) upon initiating a restoration may not contribute much to the recovery of vegetation cover in site situations like ours, i.e., within an elevation range of a low marsh zone, and where sediment deposition is high and passive establishment of the primary ecosystem engineer species (S. alterniflora in our case) is prompt. In other situations, such as in a high marsh zone (even in the Bay of Fundy; Rabinowitz et al., 2022) or other geographic locations (Travis et al., 2002; Novy et al., 2010), transplanting tillers and/or seedlings has been shown to be worthwhile.
Aside from their influence on site-level spatial patterns, which was presented as part of our current study, a potential restoration implication and likely consequence of the high number of seedlings appearing during recovery (from 2014 to 2017, or end of phase 2 and start of phase 3) that requires investigation is the potential increase in S. alterniflora genetic diversity. Indeed, we did collect plant samples for genetic analysis in 2016–2017, but still need to process them. Restoration projects elsewhere did observe that natural levels of genetic diversity in S. alterniflora can develop quickly through passive colonization if a ready source of propagules is available (Louisiana; Travis et al., 2002). Genetic diversity in S. alterniflora can influence many aspects affecting recovery dynamics, including plant performance and spread rates (Seliskar et al., 2002; Wang et al., 2012), germination response (Seneca, 1974; Travis et al., 2002), flowering phenology (Somers and Grant, 1981), adaptability (Travis et al., 2002), and interactions with other species, such as competition, facilitation, and resistance to consumer pressure and fungal infection (Travis et al., 2002; Proffitt et al., 2005; Zerebecki et al., 2017). With the return to mostly asexual reproduction in S. alterniflora once a marsh site is fully vegetated, genetic diversity is expected to decrease as certain competitively superior genotypes begin to dominate the maturing marsh (Travis et al., 2004); this should be tested at our sites in the future. Overall, the third phase of restoration was defined by the natural proliferation and success of S. alterniflora until its near complete cover of the restoration sites.
The Aulac salt marsh restoration is now just starting the fourth phase (years 10+), distinguished by the development of the S. alterniflora short phenotype, spread of S. patens, and likely interaction between S. alterniflora and S. patens (Virgin et al., 2020). During earlier phases, mature S. alterniflora plants throughout the restoration sites were the tall phenotype (similar in canopy height to those growing in creeks of reference sites), indicating that they were healthy and not stressed by intraspecific competition, nutrient limitation, or abiotic conditions (Anderson and Treshow, 1980; Zerebecki et al., 2021). However, at the beginning of the fourth phase, S. alterniflora growing close to the seaward edge of the restoration sites began to resemble the short-form plants of the reference marshes. Bay of Fundy salt marshes typically have a scarped seaward edge, which is elevated compared to the sides of the creeks within the marshes where tall-form S. alterniflora grows. This seaward edge is exposed to environmental stress (including tidal, wind, and wave action), and is occupied by short-form S. alterniflora. Our recent observations of spatial heterogeneity in canopy height in the restoration sites, with short S. alterniflora plants seaward in more elevated and older vegetated areas, and tall S. alterniflora plants landward in the lower elevation, protected and more newly vegetated areas (including the borrow pits), are approaching the reference condition. The transition in phenotype from tall form to short form in the seaward area of our sites may be due to increasing intraspecific competition among these older S. alterniflora plants (Wang et al., 2005), decreasing edaphic conditions with accreting marsh surface elevation and presumably less tidal flushing (resulting in the delivery of less fresh sediment and nutrients; Mendelssohn and Seneca, 1980), and/or environmental stress in the form of mechanical action by high tidal, wave, and wind energy (e.g., causing high rates of plant mass loss, particularly in apical sections of leaves; Baerlocher and Moulton, 1999). We suspect that a combination of the above is involved because the old seaward dike (breached in 2010 in demarcated locations) protected the developing marsh until it was essentially fully eroded after winter 2016 (Virgin et al., 2020); this resulted in much higher environmental stress on the young marsh. However, we only started detecting the short phenotype in 2019 (Figure 7). Targeted research is needed to disentangle the cause(s) of the change in phenotype, since proximity to the seaward marsh edge and age of the S. alterniflora patches are confounded in our study, and this is further confounded by the effect of the yearly increased accretion of the marsh surface (mean accretion ± SE between 2010 and 2020: 0.57 ± 0.05 m, n = 45 GPS locations, range: 0.13–1.25 m). Note that although our restoration sites have accreted substantially, their surface elevation in 2020 (mean ± SE: 5.92 ± 0.04 m CGVD28, n = 45 locations) still has much accretion to do to reach the elevation of reference sites (6.83 ± 0.07 m CGVD28; n = 7 locations; data from J. Ollerhead). Two restoration implications arise from our above observations. First, dike-breaching plans need to consider the duration of protection provided to the developing young marsh. This duration partly depends on the state of the old dike when breached. For the Aulac project, although a new (managed realignment) dike was built in 2006, dedicated plans for breaching were done in 2009, and the breaching occurred in 2010, when the old dike had already started to erode (Boone et al., 2017). Implementing the breaching sooner would have given the developing marsh more time to develop while being protected. Second, conversion of S. alterniflora patches from tall form to short form is indicative of the increased maturity of the restoring marsh, heading toward the reference condition, and is an easy metric to monitor. Overall, development of distinct S. alterniflora phenotypes is a key indicator of the fourth phase of restoration in Aulac, as is the spread of S. patens.
Just before and at the start of the fourth phase, S. patens began spreading into the restoration sites from the higher elevation dike areas as well as from individual patches within the restoration sites proper. We predicted spread from dike edges would be the primary mode of spread because S. patens performs best in high elevation salt marsh areas (Bertness, 1991). Like S. alterniflora, we did not expect that S. patens would colonize and begin spreading in relatively high elevation areas in the middle of the restoration sites. We suspect that these isolated, circular, within-site, S. patens patches originated from rhizome material or turf deposited by ice blocks during the winter in a similar manner to S. alterniflora (Lundholm et al., 2021), since the isolated S. patens patches were already substantial in size (1–2 m in diameter) when we found them (one in 2016 and one in 2017 in Restoration West, one in fall 2020 in Restoration East, and ~ 20 in 2021 in Restoration West). Based on the morphologies of the grasses, namely the long rhizomes of S. alterniflora and dense clonal morphology of S. patens, we think that S. alterniflora mostly colonized as rhizome material and S. patens as turf, but this needs to be investigated by inspecting ice blocks in winter. Thus far, we have only detected asexual colonization and spread of S. patens, and not by sexual reproduction although S. patens patches in restoration and reference sites do flower. There is reduced need for planting tillers or seedlings in sites like ours since plant material readily colonizes a restoration area passively. After colonizing the restoration sites, the increase in S. patens has been relatively slow compared to that of S. alterniflora earlier in the restoration.
Spartina patens encroachment has been slow likely due to the plant’s dense clonal morphology, gradually improving edaphic conditions, and possible interactions with S. alterniflora (Bertness, 1991; Pennings et al., 2005). Based on S. patens dynamics to date and as mentioned above, only recently has the marsh surface likely reached high enough elevation (and consequent appropriate inundation conditions) that enabled S. patens to spread. More years of monitoring are needed to determine if an increasing rate of spread will occur (Frenkel and Boss, 1988). In the upper Bay of Fundy (such as our Aulac sites), appropriate edaphic conditions for S. patens develop naturally since sedimentation rates are very high (Virgin et al., 2020). Other regions may require anthropogenic intervention to improve edaphic conditions for high elevation marsh species including S. patens (Fearnley, 2008), which when not appropriate, negatively influence plant performance (Anastasiou and Brooks, 2003; Merino et al., 2010) and prevent colonization (Gleason and Zieman, 1981). In addition, other regions may require planting of tillers or seedlings if plant material does not readily colonize a restoration area passively as it does in the upper Bay of Fundy. As edaphic conditions for S. patens continue to improve in Aulac, we expect that S. patens will competitively displace S. alterniflora (Bertness, 1991). Recently, S. alterniflora plants adjacent to S. patens patches appeared to be experiencing competitive stress, based on their observed lower stem densities and canopy heights than further away from these patches. It is not clear if S. patens are experiencing stress from adjacent S. alterniflora. Continued monitoring as well as experimentation on interspecific interactions between S. patens and S. alterniflora are needed to quantify plant states and spread rates in this situation, and to better understand processes underlying the fourth phase of salt marsh restoration during our project.
Conclusion
We conducted a decade-long study to quantify plant states and patch-level processes that lead to changes in vegetation community patterns during salt marsh restoration in the upper Bay of Fundy. Our focus on processes enabled us to better understand the phases of salt marsh restoration pattern change identified by Virgin et al. (2020). We found that S. pectinata, surviving after the dike breaching, was stressed, growing in sediment of high salinity, and declined because of these environmental conditions rather than competition with S. alterniflora. We also suggest that S. pectinata has potential in protecting a new restoration site, facilitating sediment retention. Spartina alterniflora initially colonized the restoration sites 2 years post-breach (in 2012), we think via deposited vegetative material, and soon after displayed the tall phenotype and rapid asexual spread. After the appearance of S. alterniflora seedlings in 2014 as well as continued asexual spread, the percent cover of vegetation in the restoration sites became greater than 90% by 2019. Seedlings took 2 years to reach mature live stem density and canopy height, and 3 years to reach mature percent flowering. As the restoration site aged, we began observing evidence that the short form phenotype of S. alterniflora was forming near the seaward scarped edges of the sites. We also observed S. patens slowly spreading from high elevation dike areas into the restoration sites and outward from isolated patches within-site. We expect these processes to continue until the restoration sites resemble established salt marshes by displaying distinct zonation between high and low marsh zones, and with tall and short phenotypes of S. alterniflora. In a future study, we plan to use our patch-level process information to estimate parameters for spatial-explicit modeling to examine patterns of percent vegetation cover and patch size distributions at the site level and to compare against observed patterns (complemented with recent and on-going remote sensing of salt marsh vegetation at our sites; Norris et al., 2022). Once developed, the modeling exercise will be used to evaluate different restoration strategies and help plan future restoration projects in the Bay of Fundy. Aside from contributing to a future modeling exercise, our study also provided relevant, detailed-oriented information and insight for salt marsh restoration strategies.
Data availability statement
The raw data supporting the conclusions of this article will be made available by the authors, without undue reservation.
Author contributions
MB and DS conceived the main study design. MB oversaw the research. GN, SV, EM, DS, JW, KM, LH, and MH led year-specific sampling designs, data collection, processing, and reporting of the project. GN analyzed the overall dataset. GN and MB wrote and edited the manuscript. SV and DS also edited the manuscript. All authors contributed to the article and approved the submitted version.
Funding
This work was funded by Mprime Network (MB and James Watmough), MITACS ACCELERATE Internships (IT09293 and IT11110 to SV and MB, and IT14070 and IT18318 to GN and MB), MITACS ACCELERATE Fellowship (IT25726 to GN, MB, and Brigitte Leblon), and Ducks Unlimited Canada; Natural Sciences and Engineering Research Council of Canada (Discovery grants 227554, RGPIN-2015-04825, and RGPIN-2020-04106 to MB); Science Horizons Youth Internship Program (Clean Tech or Natural Resources Internships, funded by Environment and Climate Change Canada or Natural Resources Canada), New Brunswick Environmental Trust Fund (160133 to MB, Jeff Ollerhead, and Nic R. McLellan, and 200133 and 210235 to Armand LaRocque, Brigitte Leblon, MB, and GN), New Brunswick Student Employment Experience Development Program (New Brunswick Post-Secondary Education, Training, and Labor), Canada Summer Jobs Program (Employment and Social Development Canada), 2011 TD Canada Trust Community Leadership Scholarship (to Cody R. Alderson), and University of New Brunswick.
Acknowledgments
We thank Cody R. Alderson, Tyler D. Trask, and Sarah G. Neima for data collection and reporting in 2011, 2014, and 2016, respectively; and research assistants and MITACS ACCELERATE interns for field work. We also thank James Watmough, Nic R. McLellan, Jeff Ollerhead, Wade Lewis, Brigitte Leblon, and Armand LaRocque for support, discussion, and feedback.
Conflict of interest
The authors declare that the research was conducted in the absence of any commercial or financial relationships that could be construed as a potential conflict of interest.
Publisher’s note
All claims expressed in this article are solely those of the authors and do not necessarily represent those of their affiliated organizations, or those of the publisher, the editors and the reviewers. Any product that may be evaluated in this article, or claim that may be made by its manufacturer, is not guaranteed or endorsed by the publisher.
Supplementary material
The Supplementary material for this article can be found online at: https://www.frontiersin.org/articles/10.3389/fevo.2022.1000075/full#supplementary-material
References
Anastasiou, C. J., and Brooks, J. R. (2003). Effects of soil pH, redox potential, and elevation on survival of Spartina patens planted at a west Central Florida salt marsh restoration site. Wetlands 23, 845–859. doi: 10.1672/0277-5212(2003)023[0845:EOSPRP]2.0.CO;2
Anderson, M., Gorley, R. N., and Clarke, K. R. (2008). PERMANOVA+ for PRIMER: Guide to software and statistical methods. Plymouth, United Kingdom: Primer-E Limited.
Anderson, C. M., and Treshow, M. (1980). A review of environmental and genetic factors that affect height in Spartina alterniflora Liosel. (salt marsh cord grass). Estuaries 3, 168–176. doi: 10.2307/1352066
Ayres, D. R., Smith, D. L., Zaremba, K., Klohr, S., and Strong, D. R. (2004). Spread of exotic cordgrass and hybrids (Spartina sp.) in the tidal marshes of San Francisco Bay, California, USA. Biol. Invasions 6, 211–231. doi: 10.1023/B:BINV.0000022140.07404.b7
Baerlocher, F., and Moulton, V. D. (1999). Spartina alterniflora in two New Brunswick salt marshes. I. Growth and decomposition. Bull. Mar. Sci. 64, 299–305.
Barrett, N. E., and Niering, W. A. (1993). Tidal marsh restoration: trends in vegetation change using a geographical information system (GIS). Restor. Ecol. 1, 18–28. doi: 10.1111/j.1526-100X.1993.tb00005.x
Bergen, A., Alderson, C., Bergfors, R., Aquila, C., and Matsil, M. A. (2000). Restoration of a Spartina alterniflora salt marsh following a fuel oil spill, New York City, NY. Wetl. Ecol. Manag. 8, 185–195. doi: 10.1023/A:1008496519697
Bertness, M. D. (1991). Zonation of Spartina patens and Spartina alterniflora in a New England salt marsh. Ecology 72, 138–148. doi: 10.2307/1938909
Betzen, B. M., Smart, C. M., Maricle, K. L., and Maricle, B. R. (2019). Effects of increasing salinity on photosynthesis and plant water potential in Kansas salt marsh species. Trans. Kans. Acad. Sci. 122, 49–58. doi: 10.1660/062.122.0105
Boone, L. K., Ollerhead, J., Barbeau, M. A., Beck, A. D., Sanderson, B. G., and McLellan, N. R. (2017). “Chapter 21. Returning the tide to dikelands in a macrotidal and ice-influenced environment: challenges and lessons learned,” in Coastal Wetlands: Alteration and Remediation. eds. C. W. Finkl and C. Makowski (Cham, Switzerland: Springer International Publishing), 705–749.
Bortolus, A., Adam, P., Adams, J. B., Ainouche, M. L., Ayres, D., Bertness, M. D., et al. (2019). Supporting Spartina: interdisciplinary perspective shows Spartina as a distinct solid genus. Ecology 100:e02863. doi: 10.1002/ecy.2863
Bowron, T. M., Neatt, N., van Proosdij, D., and Lundholm, J. (2012). “Salt marsh tidal restoration in Canada’s maritime provinces,” in Tidal Marsh Restoration: A Synthesis of Science and Management. eds. C. T. Roman and D. M. Budrick (Washington DC: Island Press), 191–209.
Briske, D. D., Fuhlendorf, S. D., and Smeins, F. E. (2005). State-and-transition models, thresholds, and rangeland health: a synthesis of ecological concepts and perspectives. Rangel. Ecol. Manag. 58, 1–10. doi: 10.2111/1551-5028(2005)58<1:SMTARH>2.0.CO;2
Broome, S. W., Seneca, E. D., and Woodhouse, W. W. Jr. (1988). Tidal salt marsh restoration. Aquat. Bot. 32, 1–22. doi: 10.1016/0304-3770(88)90085-X
Burdick, D. M. I., Dionne, M., Boumans, R. M., and Short, F. T. (1996). Ecological responses to tidal restorations of two northern New England salt marshes. Wetl. Ecol. Manag. 4, 129–144. doi: 10.1007/BF01876233
Callaway, J. C., and Josselyn, M. N. (1992). The introduction and spread of smooth cordgrass (Spartina alterniflora) in South San Francisco Bay. Estuaries 15, 218–226. doi: 10.2307/1352695
Costanza, R., Perez-Maqueo, O., Martinez, M. L., Sutton, P., Sharolyn, J., and Mulder, K. (2008). The value of coastal wetlands for hurricane protection. Ambio 37, 241–248. doi: 10.1579/0044-7447(2008)37[241:TVOCWF]2.0.CO;2
Craine, J. M., and Dybzinski, R. (2013). Mechanisms of plant competition for nutrients, water, and light. Funct. Ecol. 27, 833–840. doi: 10.1111/1365-2435.12081
Desplanque, C., and Mossman, D. (2004). Tides and their seminal impact on the geology, geography, history, and socio-economics of the Bay of Fundy, eastern Canada. Atlantic Geol. 40, 1–130.
Fearnley, S. (2008). The soil physical and chemical properties of restored and natural back-barrier salt marsh on isles Dernieres, Louisiana. J. Coast. Res. 24, 84–94. doi: 10.2112/05-0620.1
Fisheries and Oceans Canada (2022a). Tides, currents, and water levels [online], Available at: https://www.tides.gc.ca/eng/find/zone/30 (Accessed June 27, 2022).
Fisheries and Oceans Canada (2022b). Tides, currents, and water levels [online], Available at: https://www.tides.gc.ca/en/stations/190 (Accessed September 14, 2022).
Fraser, A., and Kindscher, K. (2005). Spatial distribution of Spartina pectinata transplants to restore wet prairie. Restor. Ecol. 13, 114–151. doi: 10.1111/j.1526-100X.2005.00017.x
French, C. E., French, J. R., Clifford, N. J., and Watson, C. J. (2000). Sedimentation-erosion dynamics of abandoned reclamations: the role of waves and tides. Cont. Shelf Res. 20, 1711–1733. doi: 10.1016/S0278-4343(00)00044-3
Frenkel, R. E., and Boss, T. R. (1988). Introduction, establishment and spread of Spartina patens on Cox Island, Siuslaw Estuary, Oregon. Wetlands 8, 33–49. doi: 10.1007/BF03160807
Garbutt, R. A., Reading, C. J., Wolters, M., Gray, A. J., and Rothery, P. (2006). Monitoring the development of intertidal habitats on former agricultural land after the managed realignment of coastal defences at Tollesbury, Essex, UK. Mar. Pollut. Bull. 53, 155–164. doi: 10.1016/j.marpolbul.2005.09.015
Gedan, K. B., Silliman, B. R., and Bertness, M. D. (2009). Centuries of human-driven change in salt marsh ecosystems. Annu. Rev. Mar. Sci. 1, 117–141. doi: 10.1146/annurev.marine.010908.163930
Gleason, M. L., and Zieman, J. C. (1981). Influence of tidal inundation on internal oxygen of Spartina alterniflora and Spartina patens. Estuar. Coast. Shelf Sci. 13, 47–57. doi: 10.1016/S0302-3524(81)80104-1
Hester, M. W., Mendelssohn, I. A., and McKee, K. L. (1996). Intraspecific variation in salt tolerance and morphology in the coastal grass Spartina patens (Poaceae). Am. J. Bot. 83, 1521–1527. doi: 10.1002/j.1537-2197.1996.tb12811.x
Hupp, C. R. (1992). Riparian vegetation recovery patterns following stream channelization: a geomorphic perspective. Ecology 73, 1209–1226. doi: 10.2307/1940670
Keizer, P. D., Hargrave, B. T., and Gordon, D. C. Jr. (1989). Sediment-water exchange of dissolved nutrients at an intertidal site in the upper reaches of the bay of Fundy. Estuaries 12, 1–12. doi: 10.2307/1351444
Langley, A. J., Mozdzer, T. J., Shepard, K. A., Hagerty, S. B., and Patrick, M. J. (2013). Tidal marsh plant responses to elevated CO2, nitrogen fertilization, and sea level rise. Glob. Chang. Biol. 19, 1495–1503. doi: 10.1111/gcb.12147
Levin, S. A. (1992). The problem of pattern and scale in ecology: the Robert H MacArthur award lecture. Ecology 73, 1943–1967.
Liu, Q. X., Rietkerk, M., Herman, P. M. J., Piersma, T., Fryxell, J. M., and van de Koppel, J. (2016). Plant separation driven by density-dependent movement: a novel mechanism for ecological patterns. Phys Life Rev 19, 107–121. doi: 10.1016/j.plrev.2016.07.009
Lundholm, J., Rabinowitz, T. M., Greene, L., Glogowski, A. D., Bowron, T., and van Proosdij, D (2021). Hitchhiking halophytes in wrack and sediment-laden ice blocks contribute to tidal marsh development in the upper bay of Fundy. Research Square [Preprint]. doi: 10.21203/rs.3.rs-1021544/v1
Macfarlane, C. B. A., Drolet, D., Barbeau, M. A., Hamilton, D. J., and Ollerhead, J. (2013). Dispersal of marine benthic invertebrates through ice rafting. Ecology 94, 250–256. doi: 10.1890/12-1049.1
Mcleod, E., Chmura, G. L., Bouillon, S., Salm, R., Bjork, M., Duarte, C. M., et al. (2011). A blueprint for blue carbon: toward an improved understanding of the role of vegetated coastal habitats in sequestering CO2. Front. Ecol. Environ. 9, 552–560. doi: 10.1890/110004
Mendelssohn, I. A., and Seneca, E. D. (1980). The influence of soil drainage on the growth of salt marsh cordgrass Spartina alterniflora in North Carolina. Estuar. Coast. Mar. Sci. 11, 27–40. doi: 10.1016/S0302-3524(80)80027-2
Merino, J. H., Huval, D., and Nyman, A. J. (2010). Implication of nutrient and salinity interaction on the productivity of Spartina patens. Wetl. Ecol. Manag. 18, 111–117. doi: 10.1007/s11273-008-9124-4
Metcalfe, W. S., Ellison, A. M., and Bertness, M. D. (1986). Survivorship and spatial development of Spartina alterniflora Loisel. (Gramineae) seedlings in a New England salt marsh. Ann. Bot. 58, 249–258. doi: 10.1093/oxfordjournals.aob.a087202
Millard, K., Redden, A. M., Webster, T., and Steward, H. (2013). Use of GIS and high resolution LiDAR in salt marsh restoration site suitability assessments in the upper bay of Fundy, Canada. Wetl. Ecol. Manag. 21, 243–262. doi: 10.1007/s11273-013-9303-9
Minello, T. J., Able, K. W., Weinstein, M. P., and Hays, C. G. (2003). Salt marshes as nurseries for nekton: testing hypotheses on density, growth and survival through meta-analysis. Mar. Ecol. Prog. Ser. 246, 39–59. doi: 10.3354/meps246039
Minitab 18 Statistical Software (2015). [Computer software]. Minitab Inc. Pennsylvania State University, PA, USA (www.minitab.com).
Morgan, V. H., and Sytsma, M. D. (2013). Potential ocean dispersal of cordgrass (Spartina spp.) from core infestations. Invas. Plant Sci. Manag. 6, 250–259. doi: 10.1614/IPSM-D-12-00042.1
Mossman, H. L., Brown, M. J. H., Davy, A. J., and Grant, A. (2012). Constraints of salt marsh development following managed coastal realignment: dispersal limitation or environmental tolerance? Restor. Ecol. 21, 65–75. doi: 10.1111/j.1526-100X.2010.00745.x
Neumeier, U., and Ciavola, P. (2004). Flow resistance and associated sedimentary processes in a Spartina maritima salt-marsh. J. Coast. Res. 20, 435–447. doi: 10.2112/1551-5036(2004)020[0435:FRAASP]2.0.CO;2
Norris, G. S., Leblon, B., LaRocque, A., Barbeau, M. A., and Hanson, A. R. (2022). “Effect of textural features for landcover classification of UAV multispectral imagery of a salt marsh restoration site.” The International Archives of the Photogrammetry, Remote Sensing and Spatial Information Sciences. International Society for Photogrammetry and Remote Sensing XXIV ISPRS congress, Nice, France, 951–958.
Norris, G. S., Meed, N. R., Barbeau, M. A., Ollerhead, J., and McLellan, N. R. (2020). ‘Restoration of salt marsh in a former freshwater impoundment in Musquash, NB: the first year after breaching the dike’, Department of Biology, University of new Brunswick, Fredericton, NB. Prepared for: Ducks Unlimited Canada, NB Department of Transportation and Infrastructure, Fisheries and Oceans Canada, and Nature Conservancy of Canada for 2019, 0–48.
Novy, A., Smouse, P. E., Hartman, J. M., Struwe, L., Honig, J., Miller, C., et al. (2010). Genetic variation of Spartina alterniflora in the New York metropolitan area and its relevance for marsh restoration. Wetlands 30, 603–608. doi: 10.1007/s13157-010-0046-6
Peng, S., Zhou, T., Liang, L., and Ren, W. (2012). Landscape pattern dynamics and mechanisms during vegetation restoration: a multiscale, hierarchical patch dynamics approach. Restor. Ecol. 20, 95–102. doi: 10.1111/j.1526-100X.2010.00741.x
Pennings, S. C., Grant, M. B., and Bertness, M. D. (2005). Plant zonation in low-altitude salt marshes: disentangling the roles of flooding, salinity and competition. J. Ecol. 93, 159–167. doi: 10.1111/j.1365-2745.2004.00959.x
Peterson, P. M., Romaschenko, K., Arrieta, Y. H., and Saarela, J. M. (2014). A molecular phylogeny and new subgeneric classification of Sporobolus (Poaceae: Chloridoideae: Sporobolinae). Taxon 63, 1212–1243. doi: 10.12705%2F636.19"10.12705/636.19
Proffitt, C. E., Chiasson, R. L., Owens, A. B., Edwards, K. R., and Travis, S. E. (2005). Spartina alterniflora genotype influences facilitation and suppression of high marsh species colonizing an early successional salt marsh. J. Ecol. 93, 404–416. doi: 10.1111/j.0022-0477.2005.00983.x
Proffitt, C. E., Travis, S. E., and Edwards, K. R. (2003). Genotype and elevation influence Spartina alterniflora colonization and growth in a created salt marsh. Ecol. Appl. 13, 180–192. doi: 10.1890/1051-0761(2003)013[0180:GAEISA]2.0.CO;2
Rabinowitz, T. R., Lundholm, J. T., Graham, J. M., Bowron, T. M., and van Proosdij, D. (2022). Planting techniques and abiotic variation at two salt marsh restoration sites in the bay of Fundy. Restor. Ecol. e137070. doi: 10.1111/rec.13707
Radeloff, V. C., Mladenoff, D. J., and Boyce, M. S. (2001). A historical perspective and future outlook on landscape scale restoration in the Northwest Wisconsin pine barrens. Restor. Ecol. 8, 119–126. doi: 10.1046/j.1526-100x.2000.80018.x
Redfield, A. C. (1972). Development of a New England salt marsh. Ecol. Monogr. 42, 201–237. doi: 10.2307/1942263
Roderick, M. L., and Barnes, B. (2004). Self-thinning of plant populations from a dynamic viewpoint. Funct. Ecol. 18, 197–203. doi: 10.1111/j.0269-8463.2004.00832.x
Roman, C. T., and Burdick, D. M. (2012). Tidal Marsh Restoration: A Synthesis of Science and Management. Washington, DC, USA: Island Press.
Roman, C. T., Niering, W. A., and Warren, R. S. (1984). Salt marsh vegetation change in response to tidal restriction. Environ. Manag. 8, 141–149. doi: 10.1007/BF01866935
Seliskar, D., Gallagher, J., Burdick, D., and Mutz, L. A. (2002). The regulation of ecosystem functions by ecotypic variation in the dominant plant: a Spartina alterniflora salt-marsh case study. J. Ecol. 90, 1–11. doi: 10.1046/j.0022-0477.2001.00632.x
Seneca, E. D. (1974). Germination and seedling response of Atlantic and gulf coasts populations of Spartina alterniflora. Am. J. Bot. 61, 947–956. doi: 10.1002/j.1537-2197.1974.tb14034.x
Silvertown, J. (2008). The evolutionary maintenance of sexual reproduction: evidence from the ecological distribution of asexual reproduction in clonal plants. Int. J. Plant Sci. 169, 157–168. doi: 10.1086/523357
Sinicrope, T. L., Hine, P. G., Warren, R. S., and Niering, W. A. (1990). Restoration of an impounded salt marsh in New England. Estuaries 13, 25–30. doi: 10.2307/1351429
Smith, S. M., and Warren, R. S. (2012). “Vegetation responses to tidal restoration,” in Tidal Marsh Restoration. eds. C. T. Roman and D. M. Budrick (Washington DC: Island Press), 59–80.
Somers, G. F., and Grant, D. (1981). Influence of seed source upon phenology of flowering of Spartina alterniflora Loisel. And the likelihood of cross pollination. Am. J. Bot. 68, 6–9. doi: 10.1002/j.1537-2197.1981.tb06349.x
Taylor, C. M., and Hastings, A. (2004). Finding optimal control strategies for invasive species: a density-structured model for Spartina alterniflora. J. Appl. Ecol. 41, 1049–1057. doi: 10.1111/j.0021-8901.2004.00979.x
Tilman, D., and Kareiva, P. (1997). Spatial Ecology: The Role of Space in Population Dynamics and Interspecific Interactions. Princeton, New Jersey, USA: Princeton University Press
Travis, S. E., Proffitt, C. E., Lowenfeld, R. C., and Mitchell, T. W. (2002). A comparative assessment of genetic diversity among differently aged populations of Spartina alterniflora on restored versus natural wetlands. Restor. Ecol. 10, 37–42. doi: 10.1046/j.1526-100X.2002.10104.x
Travis, S. E., Proffitt, C. E., and Ritland, K. (2004). Population structure and inbreeding vary with successional stage in created Spartina alterniflora marshes. Ecol. Appl. 14, 1189–1202. doi: 10.1890/03-5135
Trilla, G. G., Kandus, P., Negrin, V., Vicari, R., and Marcovecchio, J. (2009). Tiller dynamic and production on a SW Atlantic Spartina alterniflora marsh. Estuar. Coast. Shelf Sci. 85, 126–133. doi: 10.1016/j.ecss.2009.07.034
Underwood, A. J. (1997). Experiments in Ecology: Their Logical Design and Interpretation Using Analysis of Variance. Cambridge, UK: Cambridge University Press
van Proosdij, D., Lundholm, J., Neatt, N., Bowron, T., and Graham, J. (2010). Ecological reengineering of a freshwater impoundment for salt marsh restoration in a hypertidal system. Ecol. Eng. 36, 1314–1332. doi: 10.1016/j.ecoleng.2010.06.008
van Proosdij, D., Ollerhead, J., and Davidson-Arnott, R. G. D. (2006). Seasonal and annual variations in the volumetric sediment balance of a macro-tidal salt marsh. Mar. Geol. 225, 103–127. doi: 10.1016/j.margeo.2005.07.009
Vasquez, E. A., Glenn, E. P., Guntenspergen, G. R., Brown, J. J., and Nelson, S. G. (2006). Salt tolerance and osmotic adjustment of Spartina alterniflora (Poaceae) and the invasive M halotype of Phragmites australis (Poaceae) along a salinity gradient. Am. J. Bot. 93, 1784–1790. doi: 10.3732/ajb.93.12.1784
Virgin, S. D. S., Beck, A. D., Boone, L. K., Dykstra, A. K., Ollerhead, J., Barbeau, M. A., et al. (2020). A managed realignment in the upper bay of Fundy: community dynamics during salt marsh restoration over 8 years in a megatidal, ice-influenced environment. Ecol. Eng. 149:105713. doi: 10.1016/j.ecoleng.2020.105713
Wang, X. Y., Shen, D. W., Jiao, J., Xu, N. N., Yu, S., Zhou, X. F., et al. (2012). Genotypic diversity enhances invasive ability of Spartina alterniflora. Mol. Ecol. 21, 2542–2551. doi: 10.1111/j.1365-294X.2012.05531.x
Wang, L. W., Showalter, A. M., and Ungar, I. A. (2005). Effects of intraspecific competition on growth and photosynthesis of Atriplex prostrata. Aquat. Bot. 83, 187–192. doi: 10.1016/j.aquabot.2005.06.005
Warren, R. S., Baird, L. M., and Thompson, A. K. (1985). Salt tolerance in cultured cells of Spartina pectinata. Plant Cell Rep. 4, 84–87. doi: 10.1007/BF00269213
Warren, R. S., Fell, P. E., Rozsa, R., Brawley, A. H., Orsted, A. C., Olson, E. T., et al. (2002). Salt marsh restoration in Connecticut: 20 years of science and management. Restor. Ecol. 10, 497–513. doi: 10.1046/j.1526-100X.2002.01031.x
Webb, J. W. (1983). Soil water salinity variations and their effects on Spartina alterniflora. Contrib. Mar. Sci. 26, 1–15.
Weinstein, M. P., Hazen, R., and Litvin, S. Y. (2019). Response of nekton to tidal salt marsh restoration, a meta-analysis of restoration trajectories. Wetlands 39, 575–585. doi: 10.1007/s13157-018-1106-6
Wu, J., and Loucks, O. L. (1995). From balance of nature to hierarchical patch dynamics: a paradigm shift in ecology. Q. Rev. Biol. 70, 439–466. doi: 10.1086/419172
Zerebecki, R. A., Crutsinger, G. M., and Highes, A. R. (2017). Spartina alterniflora genotypic identity affects plant and consumer responses in an experimental marsh community. J. Ecol. 105, 661–673. doi: 10.1111/1365-2745.12703
Zerebecki, R. A., Sotka, E. E., Hanley, T. C., Bell, K. L., Gehring, C., Nice, C. C., et al. (2021). Repeated genetic and adaptive phenotypic divergence across tidal elevation in a foundation plant species. Am. Nat. 198, 152–169. doi: 10.1086/716512
Zhang, D., Hu, Y., Liu, M., Chang, Y., Yan, X., Bu, R., et al. (2017). Introduction and spread of an exotic plant, Spartina alterniflora, along coastal marshes of China. Wetlands 37, 1181–1193. doi: 10.1007/s13157-017-0950-0
Keywords: pattern-building, process quantification, spread rate, density, canopy height, Bay of Fundy, Spartina, Sporobolus
Citation: Norris GS, Virgin SDS, Schneider DW, McCoy EM, Wilson JM, Morrill KL, Hayter L, Hicks ME and Barbeau MA (2022) Patch-level processes of vegetation underlying site-level restoration patterns in a megatidal salt marsh. Front. Ecol. Evol. 10:1000075. doi: 10.3389/fevo.2022.1000075
Edited by:
Arnaldo Marín, University of Murcia, SpainReviewed by:
Cathleen Wigand, United States Environmental Protection Agency (EPA), United StatesJosefa Velasco, University of Murcia, Spain
Copyright © 2022 Norris, Virgin, Schneider, McCoy, Wilson, Morrill, Hayter, Hicks and Barbeau. This is an open-access article distributed under the terms of the Creative Commons Attribution License (CC BY). The use, distribution or reproduction in other forums is permitted, provided the original author(s) and the copyright owner(s) are credited and that the original publication in this journal is cited, in accordance with accepted academic practice. No use, distribution or reproduction is permitted which does not comply with these terms.
*Correspondence: Gregory S. Norris, gnorris1@unb.ca