Differential Responses of Small Mammals to Woody Encroachment in a Semi-Arid Grassland
- 1Ashoka Trust for Research in Ecology and the Environment (ATREE), Bengaluru, India
- 2Manipal Academy of Higher Education, Manipal, India
- 3Guru Gobind Singh Indraprastha University, New Delhi, India
- 4School of Life Sciences, University of KwaZulu-Natal, Westville, South Africa
- 5DBT/Wellcome Trust India Alliance Program, Hyderabad, India
Encroachment by woody invasive plants is a major threat to grasslands and savannah ecosystems worldwide. Rodents, being primary consumers, are likely to be the first to respond to changes in the structure and composition of native vegetation. We examined the effect of an invasive shrub Prosopis juliflora (hereafter Prosopis) on the native rodent community of an arid grassland system of Western India. Our sampling plots were divided into five categories representing different stages of Prosopis invasion and other land cover types. These consisted of restored native grassland, agriculture fallow, open brushland, sparse-Prosopis plots, and Prosopis-dominated plots. We also examined the impact of woody invasion on the response of native rodents toward moonlight and temperature. As hypothesized, we found a significantly higher abundance of rodent species in the native grassland habitat compared to sparse-Prosopis habitats. However, there was no significant difference in rodent abundance and diversity between the grassland and Prosopis-dominated habitats. Thus, species richness and abundance of rodents were the highest in the restored grasslands and dense Prosopis thickets, and the lowest in the sparse Prosopis, potentially showing a “U” shaped response to Prosopis invasion. We observed a species-specific effect of Prosopis on the activity of Tatera indica, Bandicota bengalensis, and Millardia meltada. Habitat type mediated the effect of different environmental factors (moonlight and temperature) on the activity of the most commonly ocurring species T. indica while activity of M. meltada showed a weak association with environmental factors. B. bengalensis was the most generalist species showing similar activity across all habitat types. Thus, the impact of Prosopis invasion on the rodent community was uneven, and depended on species as well as on local environmental characteristics.
Introduction
Dry grasslands and savannah ecosystems across the world have been negatively affected due to the rapid expansion of woody invasive species (White et al., 2000; Ratajczak et al., 2012; Murphy et al., 2014; Stevens et al., 2016). An increase in woody cover in these landscapes has been attributed to a range of environmental and anthropogenic factors such as fire suppression, change in herbivore community, and an increase in atmospheric temperature and CO2. Deliberate introduction of non-native species by humans remains a leading cause of the increase in woody cover in many parts of the world (Archer et al., 2001; Popp et al., 2007; Auken, 2009; Ratajczak et al., 2012). Introduced species often spread out of control and can have large-scale impacts on native ecological communities. Once established in a new ecosystem, such species alter the composition of the native vegetation community either by outcompeting native plants in resource acquisition (Dyderski and Jagodziński, 2019) or by secreting toxic metabolites in the soil which inhibit the growth of native vegetation under their cover (Kaur et al., 2012). Examples of such successful invasion and establishment include Rhododendron ponticum in northern Europe (Tyler et al., 2006), Prosopis glandulosa in South Africa (Wild, 2018), and Lantana camara and P. juliflora in India (Kaur et al., 2012; Sundaram and Hiremath, 2012).
Invasion driven changes in species composition and the structure of native vegetation communities can affect native fauna through direct or indirect pathways (Stanton et al., 2018). While changes in plant species composition can alter food availability, the structural complexity of vegetation defines the perceived predation risk in the landscape (Prugh and Golden, 2014). For example, non-palatable invasive species can alter the availability of food resources for herbivores and other primary consumers (Mogashoa et al., 2020; Nerlekar et al., 2021). Bush encroachment can also either increase predation risk by providing enhanced cover for predators (Chen et al., 2021), or, decrease predation risk by providing escape cover for prey, particularly small mammals (Ceradini and Chalfoun, 2017; Jayadevan et al., 2018). As primary consumers, small mammals such as rodents are likely to be the first to respond to these changes in vegetation composition and structure. Open grassland habitats may be rich in food due to the high availability of palatable grasses and seeds but also riskier due to higher visibility for predators, compared to habitats with thick shrub cover. Therefore, rodents have to balance their use of open grasslands to take advantage of high food availability by adjusting their activity time to minimize their detection by predators (Guiden and Orrock, 2019), or select habitats with protective cover (Malo et al., 2013; Loggins et al., 2019a,b).
These impacts of bush encroachment do not apply to all species evenly and can vary with species within a guild (Kluever et al., 2019). For example, structural changes in sagebrush steppe due to invasive cheatgrass Bromus tectorum reduced the abundance and foraging efficiency of small mammals due to increased vegetation cover (Ostoja and Schupp, 2009; Bachen et al., 2018). On the other hand, invasive Rhododendron ponticum in southern England reduced predation risk for wood mice Apodemus sylvaticus thus increasing their abundance under its protective cover (Malo et al., 2013). Similarly, the abundance of deer mice Peromyscus maniculatus in the Great Basin Desert was negatively correlated with the intensity of cheatgrass cover. But in the same community, the abundance of Ord’s kangaroo rat Dipodomys ordii initially increased with rising cheatgrass cover up to a threshold before it started to decline (Kluever et al., 2019). Thus, woody invasion can alter resource abundance for some species, but can also reduce predation risk for others, and this differential response can be seen even within the same ecosystem, depending on the species of rodents.
The presence of invasive woody species can also alter how small mammals respond to abiotic factors such as moonlight and temperature. In open habitats, rodent activity tends to be lower, since bright moonlight increases risk due to better visibility for predators (Kotler et al., 2010; Jayadevan et al., 2018). However, encroachment of invasive woody species in open habitats mediates the effect of moonlight on the activity pattern of nocturnal rodents by reducing light penetration and consequently lowering the risk of predation (Guiden and Orrock, 2019). Similarly, woody vegetation can also mediate the effect of temperature on the activity of rodents by altering the micro climatic condition under its cover. Woody encroached areas can provide a micro-climate up to 2°C warmer at night compared to open grassland habitats (He et al., 2010). The warmer climate has been associated with significantly higher activity of desert rodent community (O’Farrell, 1974) as lower night-time temperature imposes a higher thermoregulatory cost to desert rodent communities (Ghosh et al., 1979).
Finally, structural changes induced by bush encroachment can also mediate interspecies interactions between two competitive species and alter their activity and habitat use in a landscape. For example, increased woody canopy cover in North and Central Florida favors gray squirrels Sciurus carolinensis resulting in reduced fox squirrel S. niger occupancy in these areas due to interspecific competition (Sovie et al., 2020). The dominant species can replace sub-ordinate species from habitats with high resource availability thus changing their habitat selection (Brown and Munger, 1985). For example, the rodent Dipodomys spectabilis was found to dominate patches with high food availability, resulting in a reduction in the abundance of the smaller species D. merriami and D. ordii. The experimental removal of D. spectabilis from the patch released the smaller species from competition, thus increasing their abundance (Brown and Munger, 1985).
In this study, we aimed to understand the response of a rodent community to a change in vegetation structure due to the encroachment of a woody invasive species in a semi-arid grassland ecosystem. Introduction of the South American P. juliflora (hereafter Prosopis) has been a major driver of change in vegetation structure of the Banni landscape in western India, along with the recent expansion of area under agriculture. Previous studies have shown reduced growth and productivity of native grasses and other vegetation under Prosopis cover (Kaur et al., 2012; Nerlekar et al., 2021). A recent study in the landscape showed a high abundance of generalist rodents under dense Prosopis cover compared to sparse Prosopis cover (Jayadevan et al., 2018). However, the response of the native rodent community toward other natural and agricultural habitats as well as restored native grasslands in relation to Prosopis, as well as how Prosopis invasion can mediate the effect of moonlight and temperature on rodent activity, remain unknown.
These restored grasslands plots support significantly higher cover of palatable grasses compared to Prosopis patches (Nerlekar et al., 2021). These grass species are a major food source for the generalist rodent community in the landscape (Prakash and Singh, 2005; Jayadevan et al., 2018). Therefore, we hypothesized that rodent species diversity and abundance would be the highest in these restored patches of grasslands compared to Prosopis encroached areas. We expected that agricultural fields would also support high rodent abundance due to increased food availability in the form of agricultural produce but lower species richness as shown in previous studies (Fraschina et al., 2014; Mamba et al., 2019). We hypothesized that native brushland would support more specialist rodent species due to the unique nature of the arid and highly saline soil with halophytic vegetation. We assessed the activity of rodent species under these different habitat types, and their interaction with abiotic factors such as temperature, and moonlight. Finally, we hypothesized that in areas where multiple species co-occur, potential intraguild interaction could also affect the activity of these species.
Materials and Methods
Study Area
The Banni grassland ecosystem is situated at the northern border of Kutch district in Gujarat, India (23° 19′ to 23° 52′ N latitude and 68° 56′ to 70° 32′ E longitude) encompassing an area of ∼ 2500 sq km. Climatically the region falls under the hot arid region of Western India with an annual average rainfall of ∼300 mm. The short rainy season lasts between June to September, resulting in seasonal flooding in low-lying areas.
The native vegetation of Banni is typically grass-dominated along with halophilic vegetation in high saline areas. Physiognomically this area is classified as a Dichanthium-Cenchrus-Lasiurus type of grassland (Dabadghao and Shankarnarayan, 1973). Complex social-ecological interactions have shaped the land cover type of the landscape. Prosopis was first introduced during the time of the erstwhile rulers of Kutch, the Maharao, in colonial India, but large-scale plantation occurred only in the early 1960s following the first national forest policy of independent India (Ramya Ravi unpublished). The introduction was aimed at reducing soil salinity and providing fuelwood and alternate livelihoods to local pastoralists. Owing to its fast growth and drought tolerance, the invasive shrub has converted large parts of the once open grasslands into dense woodland. Over time, the local communities have begun harvesting Prosopis to make charcoal and have also cleared patches of it for the cultivation of seasonal crops such as castor, cluster beans, and yellow mustard. Other natural vegetation consists of patches of saline open brushland of Suaeda fruticosa and salt-tolerant grasses such as Aeluropus lagopoides and Urochondra sp.
Indian gerbil (Tatera indica), soft furred field rat (Millardia meltada), sandy-colored metad (M. gleadowi), and Indian field mouse (Mus booduga) are some of the generalist rodent species reported from the landscape (Jayadevan et al., 2018). The major predators of rodents include several species of large owls, snakes, the Bengal monitor lizard (Varanus bengalensis), and mammalian mesopredators such as the golden jackal (Canis aureus), desert fox (Vulpes vulpes pusilla), Indian fox (V. bengalensis), jungle cat (Felis chaus), and desert cat (Felis silvestris ornata).
Sampling Design
We defined our sampling plots into five categories, each representative of different stages of Prosopis invasion and land cover types (Figure 1). These categories are (i) Grassland plots- native grassland plots maintained free of Prosopis for grazing and fodder after its mechanical removal by the local communities. Vegetation in these plots is mainly dominated by palatable grasses such as Dichanthium annulatum and Eleusine compressa. Many of these plots are around 40-years old, range in size from 0.54 hectares to 42 hectares and may be representative of native grassland vegetation before the Prosopis invasion. (ii) Prosopis-dominated plots – dense thickets of Prosopis (51–100% Prosopis cover) representing a high invasion of the shrub. (iii) Sparse Prosopis plots- areas with less than 50% cover mixed with native vegetation. These habitats are relatively more open compared to Prosopis dominated habitats. (iv) Agriculture fallow – these are open fallow agricultural land left after harvesting of crops such as castor, cluster beans, and yellow mustard and (v) Saline open brush – these open brushlands are dominated by Suaeda fruticosa and salt-tolerant grasses such as Aeluropus lagopoides, Urochondra spp. in saline areas. Percentage Prosopis cover for each 50 × 50 m plot was calculated from a classified sentinel-2 image with a spatial resolution of 10 m for both sampling years (2017–2018 and 2019–2020). The land cover classification was based on spectral properties of sentinel-2 bands using “SmileCart” classifier in Google Earth Engine. We overlaid our 50 × 50 m sampling grids on the classified image counted the number of Prosopis pixels to obtain the percentage Prosopis cover in each sampling site. The difference in mean Prosopis cover between Prosopis dominated and sparse Prosopis plots are shown in Figure 1D.
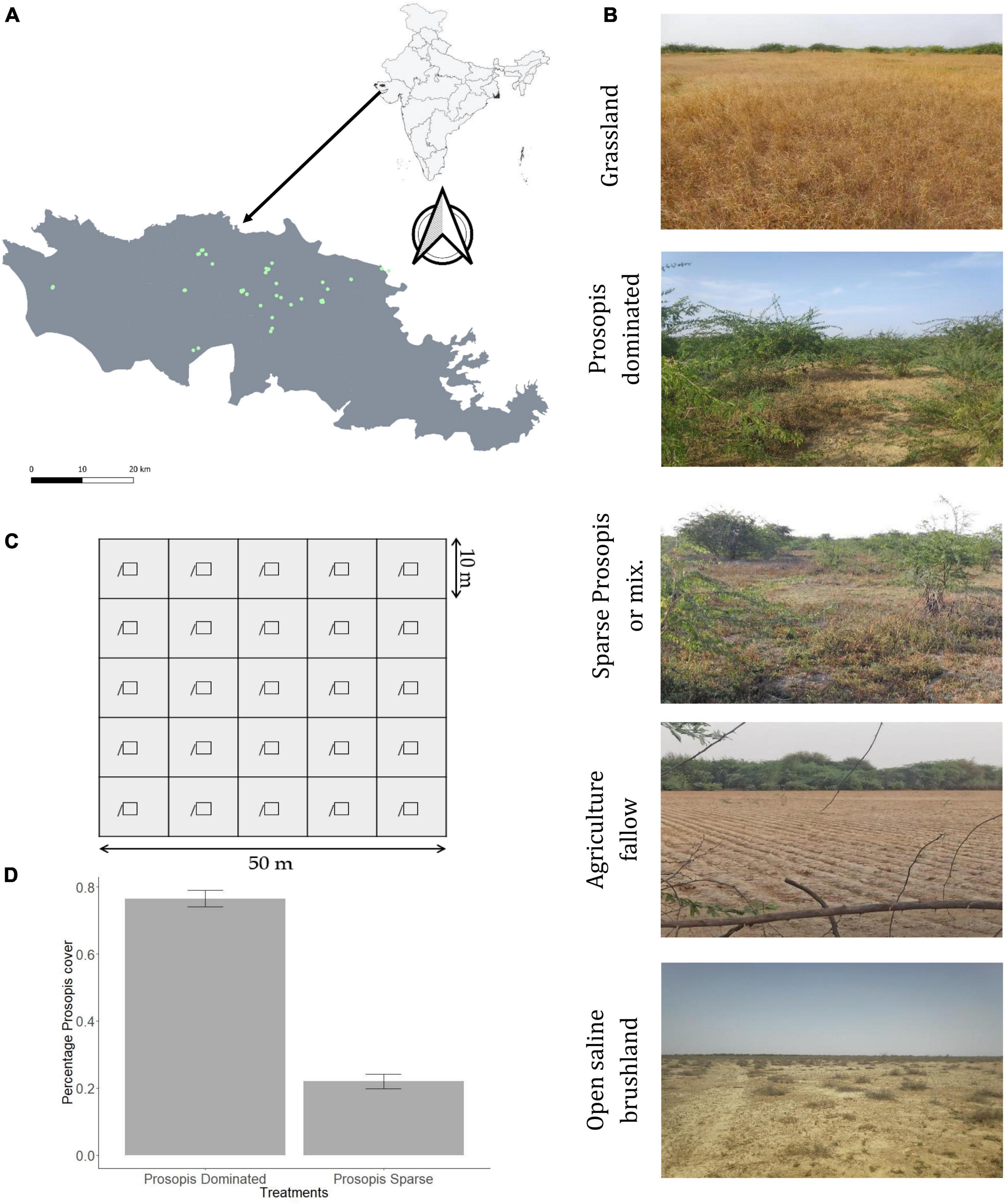
Figure 1. Sampling schema. (A) Map of the study area (The Banni grassland) showing all the sampling sites as green points. (B) Representative photographs showing the physical structure of vegetation of each type of habitat. (C) Diagram depicting trap arrangements in each 50 × 50 m plot. (D) Difference in mean percentage Prosopis cover between Prosopis sparse (mean = 0.22, S.E. = 0.021) and Prosopis dominated (mean = 0.77, S.E. = 0.025) sampling plots.
Our sampling schema consisted of a matched pair of a Prosopis thicket closest to a selected grassland enclosure (<250 m to 1 km), to control for changes in climatic, soil condition, elevation, and other anthropogenic factors (in 2017–2018 and 2019–2020). The minimum distance between the closest plots was well above the daily distance traveled (60.38 ± 09.3 m) by the most commonly found species of the rodent community; T. indica (Prakash and Singh, 2005). The sparse Prosopis plots were sampled in areas that are either recently invaded or where Prosopis is regenerating after a recent lopping cycle (in 2017–2018 and 2019–2020). Agriculture plots were sampled only in 2019–2020 in recently cultivated fields after crops were harvested. Open brush plots represent the area occupied by the sparse cover of Suaeda brush and bare soil with short ephemeral vegetation. Thus, we sampled a total of 43 sites; 9 in the sampling year 2017–2018 (3 grasslands, 3 Prosopis dominated, and 3 sparse Prosopis) and 34 in the sampling year 2019–2020 (8 grasslands, 7 Prosopis dominated, 6 sparse Prosopis, 7 agriculture fallow, and 6 open brushlands). The selection of these plots was based on spatial representation, as well as the willingness of local pastoralists to allow us access for sampling (Table 1).
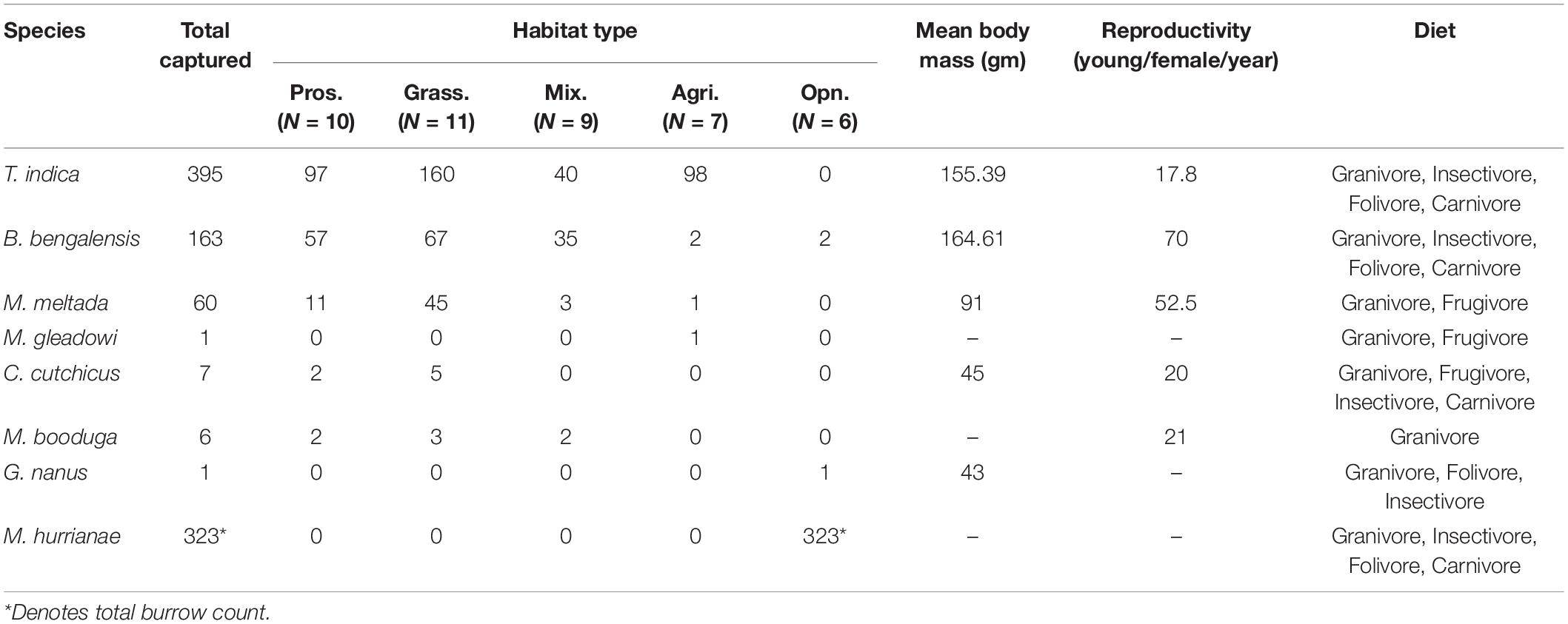
Table 1. Summary of the total number of unique individuals of each species captured in the different habitat types. Also shown are the associated mean body mass, level of reproductivity, and diet type for each species (from Prakash and Singh, 2005).
Data Collection
We sampled rodent abundance and diversity in a 50 × 50 m plot at each sampling site. We used high-density Sherman live trap grids (10 m spacing) and moderate sampling duration (4 nights) following (Smith et al., 2017) to determine the rodent species composition at each sampling plot. We divided each 50 × 50 m plot into 25 sub-cells of 10 × 10 m and one Sherman trap was placed in the center of each sub-cell. We also counted the total number of burrows of the diurnal rodent species Meriones hurrianae as an index of abundance within the sampling grid because we were not able to trap during the daytime due to the risk of theft of our traps. The burrow counts in a colony have been found to correlate very well (r2 = 0.96) with the abundance of M. hurrianae (Ramesh et al., 2013). Sampling was done for four consecutive nights at each site for each habitat type.
Traps were baited with a mix of peanut butter and pearl millet, and a piece of cotton was provided as bedding. Traps were set between 1700 to 1800 h and checked the next morning. Trapped individuals were captured and marked with numbered ear tags or non-toxic permanent markers. Information on sex, weight, body length, tail length, and length of hindlimb and forelimb were recorded for each trapped individual. Capture protocol was approved by ATREE’s Animal Ethics Committee (No: AAEC/109/2019).
Analysis
The data analyses consisted of three steps. First, we estimated the rodent population for each sampled site using a suitable estimator as described in the “population estimation” section. Then, these population estimates were taken as response variable to run a linear mixed effect model to examine the relationship between rodent population and habitat category. Further, daily capture rates of three dominant species T. indica, B. bengalensis, and M. meltada were taken as an index of rodent activity and generalized linear models (GLM) were run to examine the effect of different habitat type, moonlight, and temperature on the activity of different rodent species. A GLM was also run to examine the relationship between species richness at each sampling occasion and habitat types. The results of the statistical analyses are shown in Tables 2–5.
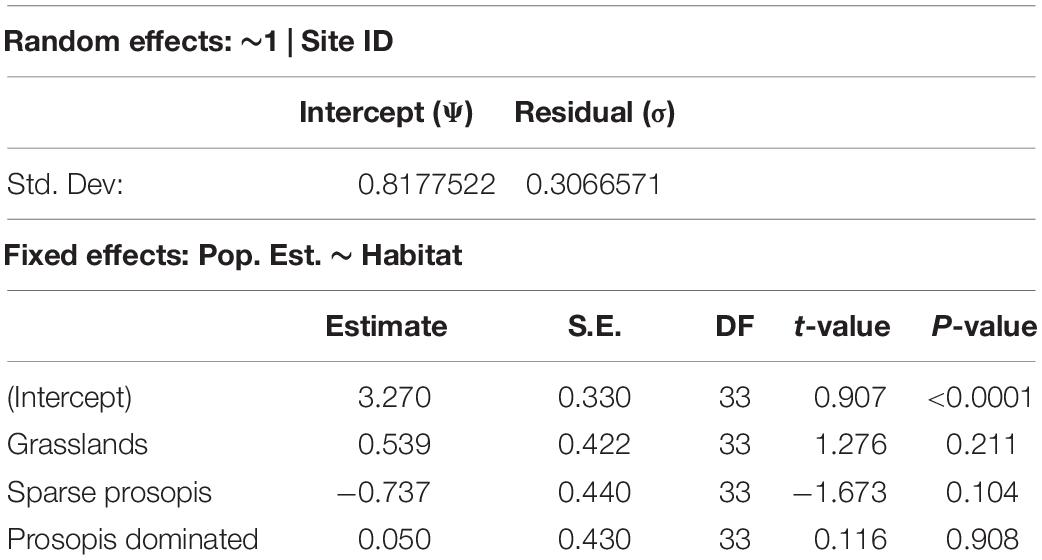
Table 2. Result of a linear mixed-effect model comparing variation in log-transformed estimated rodent abundance across different habitat types. Shown are associated β estimates, standard error (S.E.), degrees of freedom (DF), t-statistics, and p-values. The habitat type “agricultural fallow” was the reference term (intercept) and individual site ID was included as a random effect.
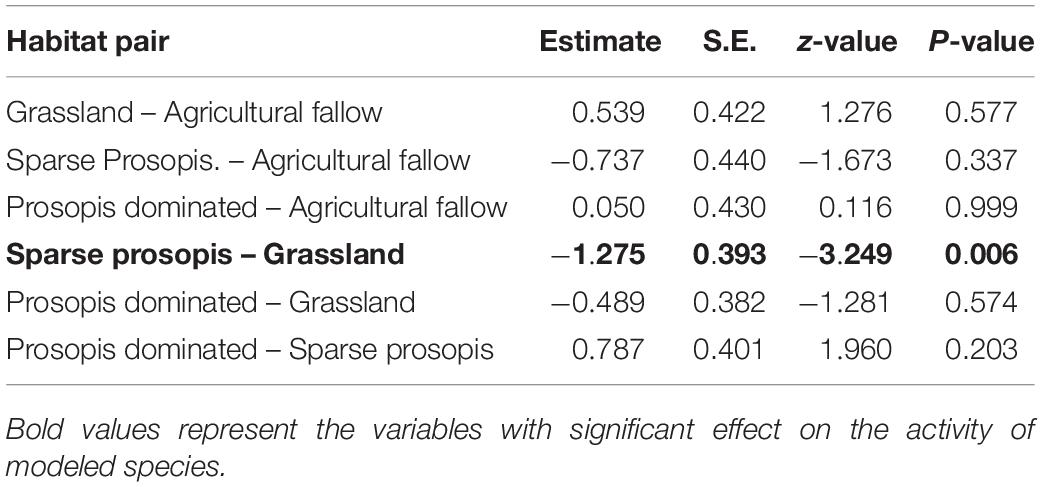
Table 3. Results of Tukey- pairwise comparisons for variation in log-transformed rodent abundance. The estimates are differences between means of two groups along with associated standard error (S.E.), Z statistic, and p-value.
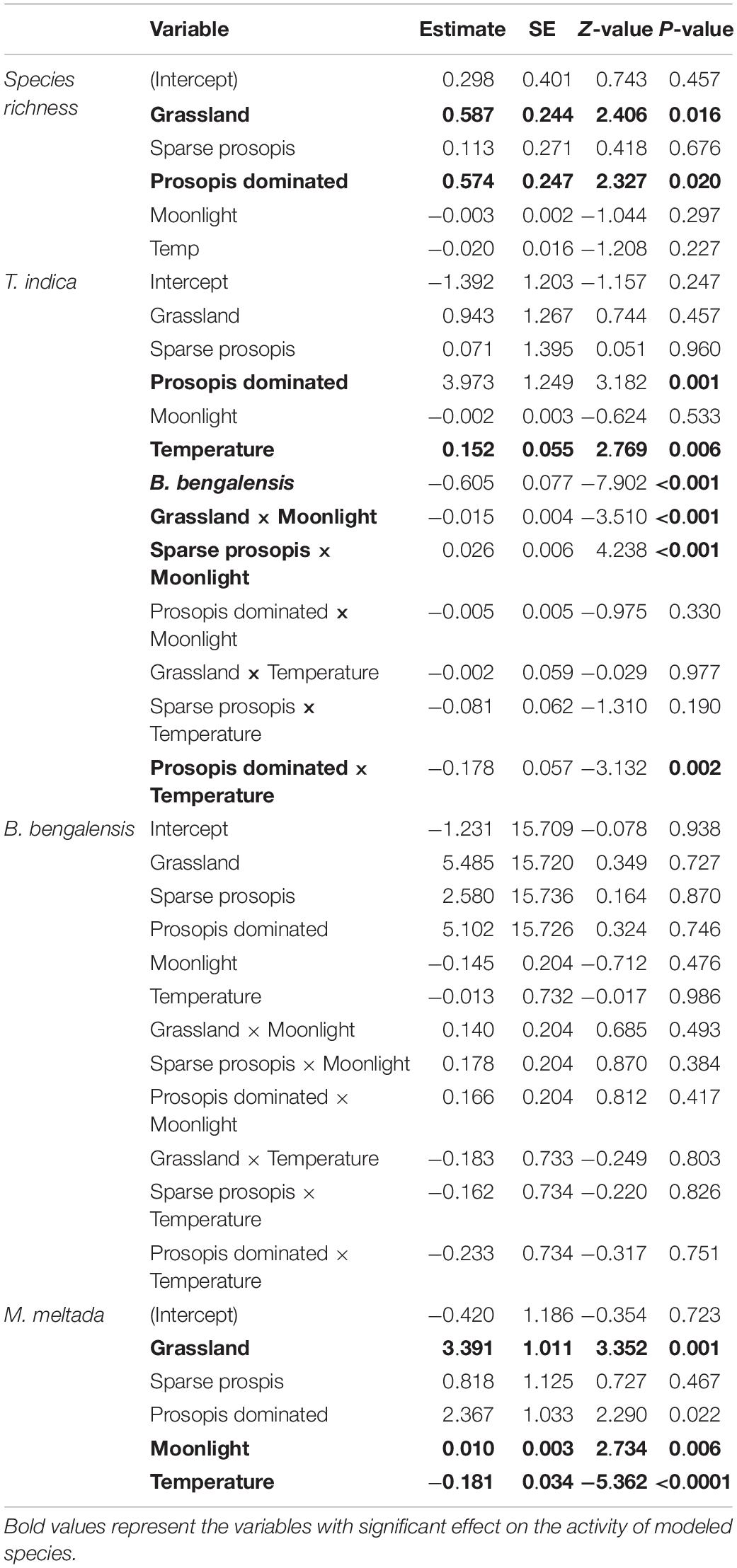
Table 5. Results of generalized linear models (GLM) for species richness, measured as the total number of species captured on each sampling occasion, as well as the activity (total individuals captured at each sampling occasion) of T. indica, B. bengalensis, and M. meltada. Predictors for the Environment models included habitat type (agricultural fallow was taken as the reference level), moonlight conditions, and minimum nighttime temperature.
Population Estimation
We used three different approaches to estimate rodent abundance at different sites to account for variation in capture and recapture rates. First we used Huggins’ closed capture model with likelihood conditioned on the number of individuals detected or captured (Huggins, 1991). These models used “p” (probability of capture) and “c” (probability of individual getting recaptured after first capture occasion) to derive abundance parameter N for each sampling site in program Mark (Cooch and White, 2019). The best model was selected based on AIC values along with site-specific observations during field data collection. We observed trap happy behavior of T. indica during sampling as the species was frequently captured within movements of trap placements, and recaptures were high. Based on these observations the effect of trap happy behavior on capture probability for the species cannot be rejected (Singh et al., 2010) therefore we selected model Mb to get abundance estimation at the sites where T. indica was the dominant species (N = 4, Supplementary Table 1).
Due to insufficient recaptures at a few sites (n = 5), Huggins’ models failed to converge and provide estimates. Therefore, for these sites we used a second method in the package “Rcapture” to estimate the population (Baillargeon and Rivest, 2007). The package uses log-linear models to estimate closed population from capture history data. We compared models of abundance M0 (null model), Mh (heterogeneity model), Mb (behavior model), and Mt (time varying model) based on AIC values. The top selected model based on AIC was run through a bias correction described by Rivest and Lévesque (2001) using the function “closedp.bc” to get a robust estimate. It uses a correction factor for missed units along with total number of units caught at least once.
Finally, two sample sites (agricultural fallow) had an extremely low number (one individual captured only) of captures; thus, we couldn’t estimate population size with any of the other methods. These low numbers of captures are also of biological significance, and therefore should not be truncated. We therefore used a canonical estimator to get the upper bound limit of rodent abundance for these sites. We calculated p* (capture probability) using the formula 1-[(1-p)4], where p is taken as the lowest value of capture probability at any occasion across all sampling sites and 4 is the number of sampling occasions. This novel approach of using three different methods to derive population estimates enables use of the most robust method where possible, thus reducing bias in estimates, but also allowed us to use the full dataset where captures or recaptures were low as this may reflect actual biological processes at these sites. The caveat here is that each method was not equally applied for each habitat type, and therefore may be a potential source of bias in the estimation of populations across habitats. However, we found a high correlation between the population abundance estimates from the Huggin’s model and the Log-linear model (r2 = 0.91, p < 0.001; Supplementary Figure 1). Furthermore, the canonical estimator was used only for two sites of agricultural fallow. Therefore, we are confident that the unequal application of various methods for estimating abundance is unlikely to have introduced any substantial bias in explaining the relationship between rodent abundance and habitat types. The estimated population of all sampling sites has been provided in Supplementary Table 1.
Linear Mixed Effect Models
Linear mixed-effect model was used to understand the relation between estimated rodent abundance and habitat types. We excluded saline open brushland from any further analysis as it was dominated by a single diurnal rodent species, the Indian desert jird M. hurrianae, as opposed to a nocturnal rodent-dominated community in all other habitats. As our sampling sites were distributed widely across the landscape, we used individual site ID as a random effect variable and habitat types as fixed effect variables. We used the “lme” function from package “nlme” in the R analysis environment (R Development Core Team, 2020) to run mixed effect models (Bates et al., 2015). We checked for data normality using the “descdist” function from the package “fitdistrplus”. Our response variable (abundance) was log-transformed to meet the assumption of normality. We also performed post hoc Tukey tests using the package “multcomp” to identify significant pairwise interactions between the fixed effects of habitat type (Hothorn et al., 2008). To determine possible inter-annual variation in rodent densities between the sampling years, we ran a student t-test to compare the estimated rodent population in grasslands, Prosopis dominated habitat, and sparse Prosopis habitat. We did not find any significant difference in estimated rodent population between sampling years in grassland (t = 0.002, p = 0.998), Prosopis dominated habitat (t = 0.191, p = 0.852), and sparse Prosopis habitat (t = 1.125, p = 0.298) and therefore pooled the data across years for further analysis.
Generalized Linear Models
Daily capture rates of three dominant species T. indica, B. bengalensis, and M. meltada were taken as an index of rodent activity and were modeled with predictor variables using generalized linear models (glm). We determined the influence of different habitat types, percentage moon luminosity, minimum nighttime temperature (in °C), and co-occurring species on the activity of these rodent species. Data on percentage moon luminosity for each sampling occasion were collected from the lunar calendar from moongiant.com (accessed on 01/6/2020). Temperature data were collected from the weather station installed at BRC (Banni Research Center), which measures temperature at five-minute intervals. All our plots (except 1) were within 20 kms of this station, and given the flat topography of the landscape, we assumed that this station captured general weather patterns across the landscape. We used the minimum night time temperature for each sampling night as a predictor variable. Our response variable was count data with a non-normal distribution, hence we used Poisson distribution with log-link function during modeling (Bolker et al., 2009).
We first ran a model for the most commonly captured species, T. indica, with activity as a response variable and habitat type, environmental factors (temperature and moonlight), and the per night capture of co-occurring B. bengalensis as predictors. We modeled an interaction term between habitat type and each of the environment factors to determine the effect of moonlight and temperature under different habitat cover. We did not have sufficient sample size to model more complex interaction terms between B. bengalensis activity and habitat type. A similar model was run for B. bengalesis to understand the effect of habitat type, environment factors, and their interaction on their activity. We then constructed a series of sub-models based on the hypotheses tested (Table 4). We ran similar models for the activity of M. meltada but did not add co-occurring species as a predictor because we did not assume any potential competition for this species.
The best fit model was selected based on the AICc value, normal Q-Q plot were visualized and the effect size of each predictor was compared for the best fit model. All analyses were done in the program R v. 4.0.2 (R Development Core Team, 2020).
Results
Abundance and Diversity
A total of 43 trapping sites were sampled during the winter of December 2017 to March 2018 (N = 9) and December 2019 to March 2020 (N = 34) (Table 1). We captured 633 unique individuals of 7 species in 4300 trap nights across sampling sites in five different habitat types. The relative abundances of species captured were T. indica (62.4%), B. bengalensis (25.75%), M. meltada (9.48%), Cremnomys cutchicus (1.11%), M. booduga (0.95%), M. gleadowi (0.16%), and Gerbillus nanus (0.16%). The diurnal species M. hurrianae was found only in open saline brushland (mean burrow count = 107.67, S.E. = 10.71). Generalized linear model predicted the highest per night species richness in both grasslands (β = 0.59, S.E. = 0.24, p = 0.02) and Prosopis dominated plots (β = 0.57, S.E. = 0.25, p = 0.02) compared to all other habitat types, though this result had limited ecological relevance with the difference in richness being <1 species. The species composition for both habitat types were similar and composed of four species; T. indica, B. bengalensis, M. meltada, C. cutchicus, and M. booduga (Figure 2). Agriculture habitats were dominated by T. indica, and sparse Prosopis sites had only three species; T. indica, B. bengalensis, M. meltada. The native brushlands had only open habitat specialist G. nanus and M. hurrianae.
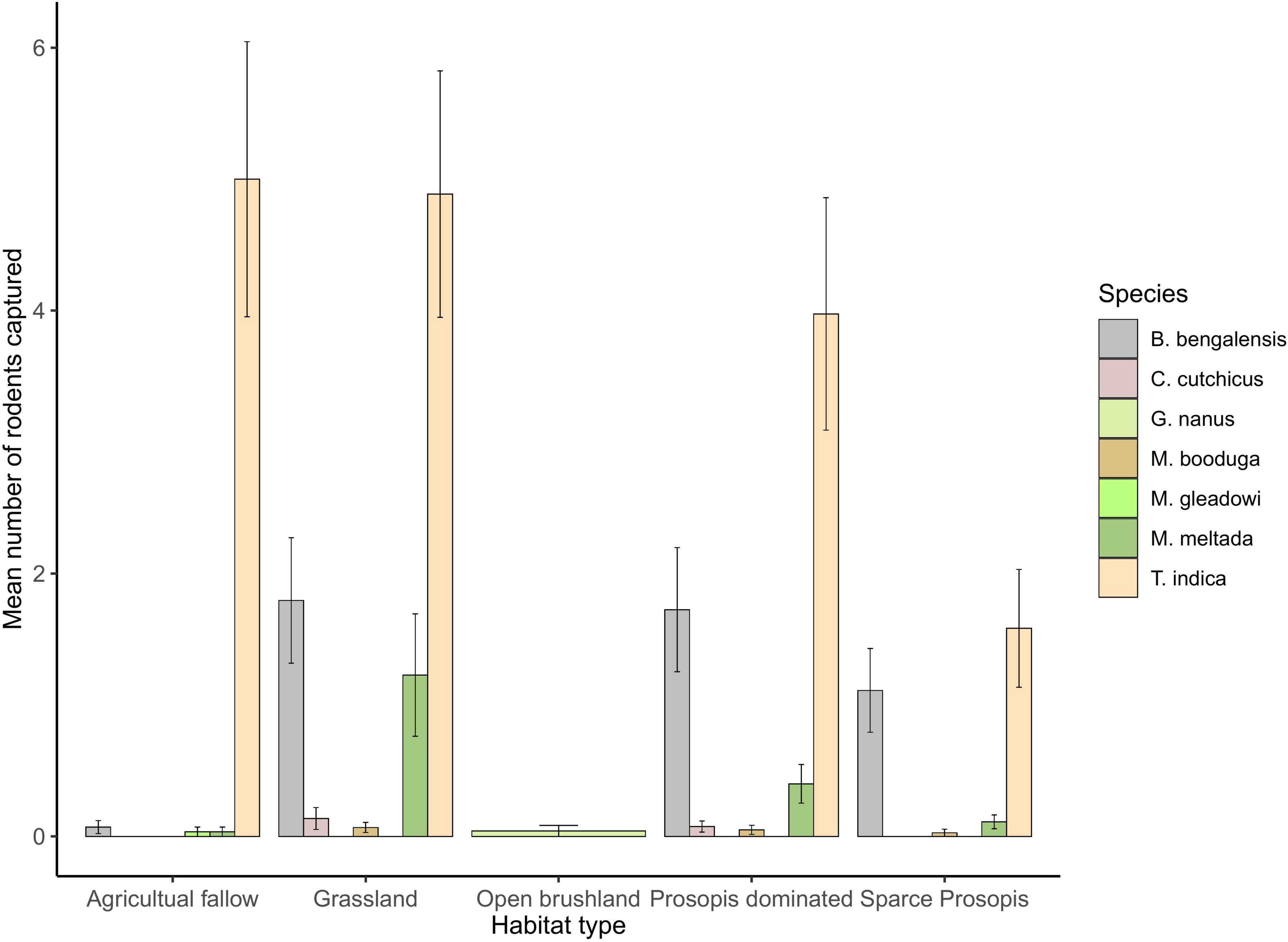
Figure 2. Species composition of rodents in different habitat types based on per night captures. Note that grassland and Prosopis-dominated habitats show similar species composition.
Linear mixed effect models (Table 2) showed that 87% of residual variation (Ψ = 0.82, σ = 0.31) was explained by the random intercept term. Tukey’s pairwise post hoc analysis (Table 3) shows rodent abundance in grasslands is significantly higher compared to sparse Prosopis plots (β = −1.28, SE = 0.39, p = 0.006). Total rodent abundance did not differ between grassland and Prosopis dominated habitat as well as between grassland and agricultural fallow significantly.
Rodent Activity
The models with interaction terms performed better than the main effects models for both T. indica and B. bengalensis (Table 4). Activity of T. indica was found to be higher under Prosopis dominated habitat (β = 3.973, S.E. = 1.249, p = 0.001), and no other habitats were significant predictors of activity. As moon phase progressed from new moon to full moon, the activity of T. indica in sparse Prosopis habitat increased from 1 to ∼13 individuals captured per night (β = 0.026, S.E. = 0.006, p < 0.0001), whereas it decreased in grassland habitat from ∼12 to ∼3 individuals captured per night (β = −0.15, S.E. = 0.004, p < 0.0001; Figure 3).
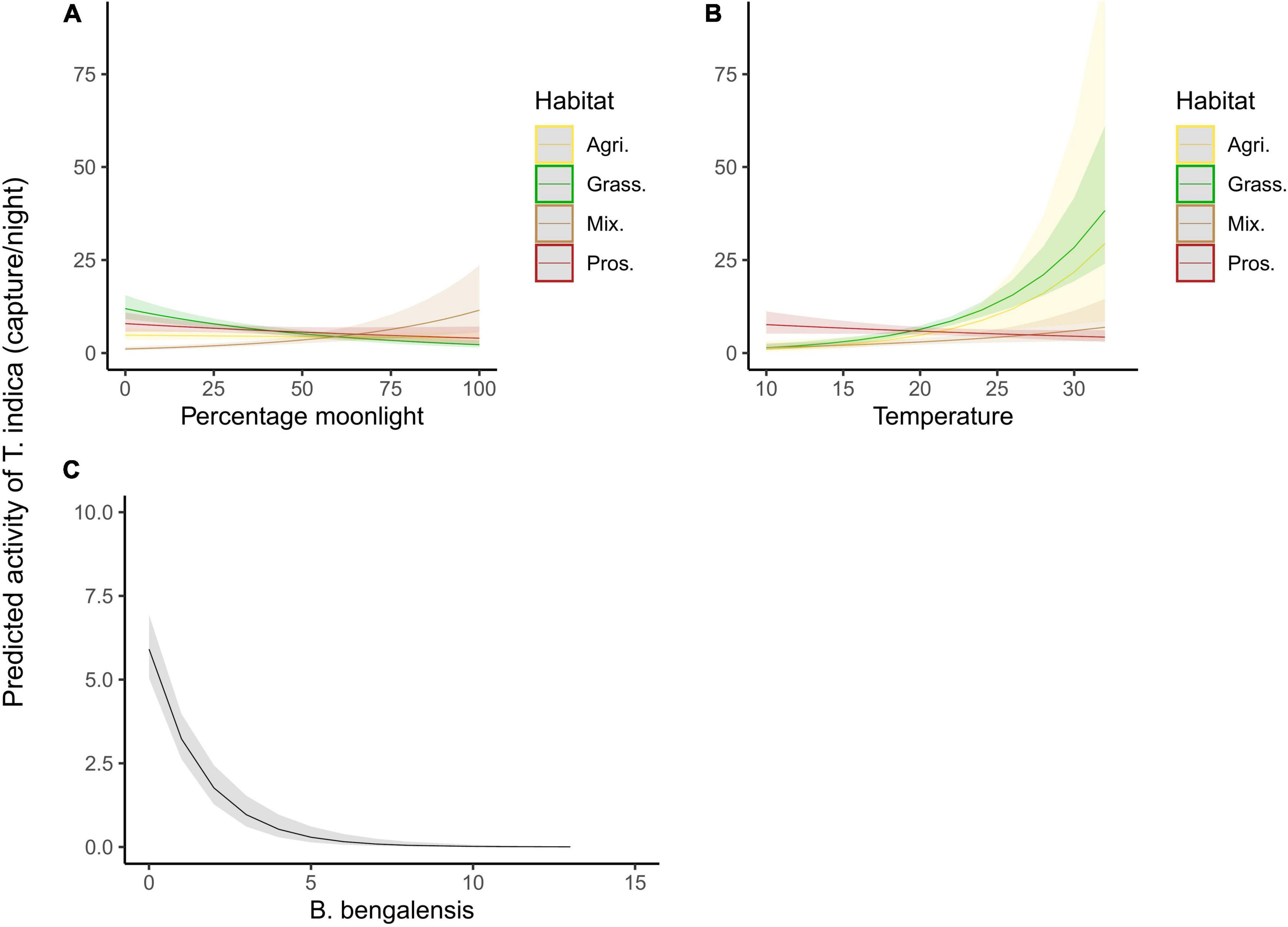
Figure 3. Predicted response of the activity of T. indica to different predictor variables based on top model. (A) Effect of percentage moon luminosity on activity under different habitat cover. (B) Effect of minimum nighttime temperature on activity under different habitat cover. (C) Effect of co-occurring B. bengalensis on the activity of T. indica.
Overall minimum night temperature was positively correlated with the activity of T. indica (β = 0.015, S.E. = 0.06, p = 0.006). However, this pattern reversed in Prosopis dominated habitats (β = −0.178, S.E. = 0.06, p = 0.002); as the ambient temperature increased from 10°C to 30°C the activity of T. indica decreased from ∼9 to ∼5 individual captured per night.
The activity of T. indica was negatively associated with the presence of B. bengalensis (β = −0.605, S.E. = 0.08, p < 0.001), decreasing from ∼6 individuals captured per night to 0 individuals for every ∼5 unit increase in the activity of co-occurring B. bengalensis.
The activity of B. bengalensis did not show any significant relationship with any habitat type, moonlight or temperature. Models with habitat type, moonlight and temperature performed well for Millardia meltada. We found higher activity of M. meltada (β = 3.39, S.E. = 1.01, p = 0.001) in grassland and Prosopis dominated habitat (β = 2.37, S.E. = 1.03, p = 0.02). No other habitat type was found to be a significant predictor of activity. As moon phase progressed from new moon to full moon, the activity of M. meltada showed no ecologically meaningful change (∼0.6 to ∼1.4 individuals captured per night, β = 0.01, S.E. = 0.003, p = 0.006; Figure 4). As the minimum night time temperature rises from 10°C to 30°C the activity reduces from ∼4.8 individual to ∼0 individual (β = −0.18, S.E. = 0.03, p < 0.0001).
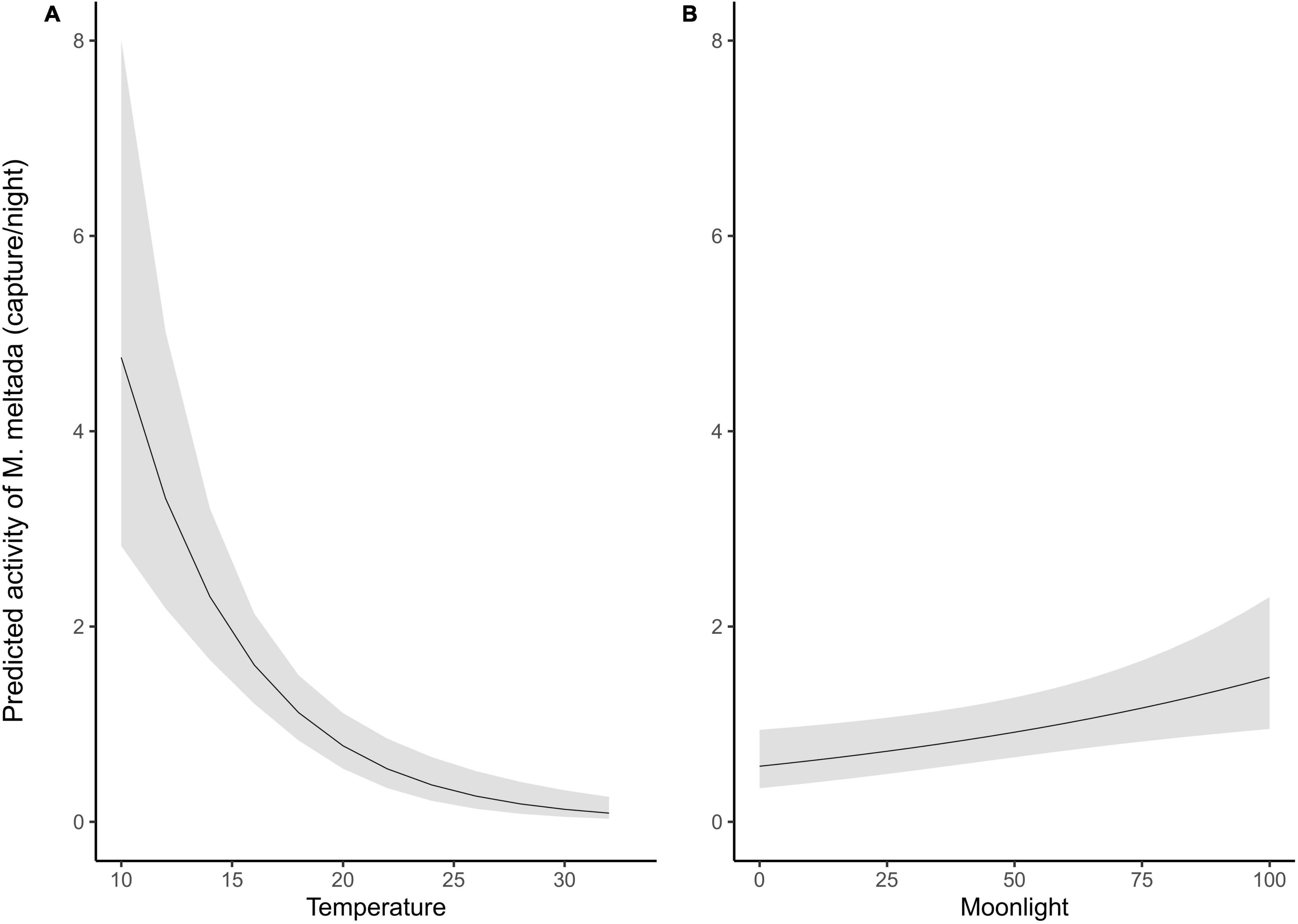
Figure 4. Predicted response of the activity of M. meltada to different predictor variables based on top model; (A) effect of temperature (B) effect of moonlight.
Discussion
Bush encroachment has been known to have un-even effects on native vertebrate communities across biomes and continents (Stanton et al., 2018), and is mainly species and environment specific (Eldridge and Soliveres, 2014). In our study, as expected, we found highest abundance and diversity of rodents in native grassland habitats and lowest in the sparse Prosopis habitats compared to all other habitats. However, rodent abundance and diversity was not significantly different in invasive Prosopis-dominated habitats compared to grasslands. We found that habitat type mediated the effect of moonlight and temperature on the activity of the most common rodent species.
Bush Encroachment and Rodent Abundance and Diversity
Our results showed a high diversity and abundance of rodents in native grassland plots, potentially attributable to the high availability of food in the form of palatable grass and seeds (Nerlekar et al., 2021). Notably, these grassland plots in the Banni landscape are a result of habitat restoration, and that outside of the fenced restored grasslands, there are no large patches of native grasslands in Banni. The patch size of different habitats can influence rodent species richness and abundance across the landscape. Given how rapidly patches of Prosopis change (due to harvesting), we were unable determine patch size for these habitats. Previous studies have, however, shown that close to 50% of the landscape is now occupied by either dense or sparse cover of Prosopis (Vaibhav et al., 2012). We found similar rodent richness and abundance in plots with high Prosopis cover as we did in the grassland habitats. A previous study in the same landscape has shown that dense Prosopis cover provides shelter from predators and supports a higher abundance of generalist rodent species compared to sparse Prosopis cover (Jayadevan et al., 2018). Our findings are consistent with this observation, and that the level of Prosopis cover may be altering species distribution at the landscape level.
Some species, such as M. hurrianae are highly adapted to xeric conditions and are restricted to those areas where Prosopis invasion is unlikely due to the saline nature of the soil. M. hurrianae along with co-existing nocturnal species G. nanus or pigmy gerbil were recorded only in the saline open brushland. Thus, rodents may show a non-linear “U” response to Prosopis invasion, occurring at their highest diversity and abundance in either native grasslands or in dense Prosopis, and at their lowest in sparse Prosopis. This could likely be linked to the relatively high productivity of these habitat types. A recent global meta-analysis shows that the negative effect of shrub-encroachment reduces in areas with higher net primary productivity (Stanton et al., 2018). Our Prosopis dominated plots were sampled close to the grassland dominated plots, and are likely to have similar levels of primary productivity and soil conditions. A similar response of the rodent community was also observed in Namibia where rodent species composition and abundance were similar in both bush-encroached and non-bush encroached habitats (Karuaera, 2011).
Our sampling sites were also widely distributed across the ∼2500 sq km landscape (Figure 1), and not surprisingly, random variation between individual sites explained a substantial amount of variation in rodent abundance. Expansion of agricultural land has become a major driver of land-use change beyond the Prosopis eradication efforts in recent years. Although agriculture here remains monsoon-dependent, it is expected to provide additional resource subsidies to generalist rodent species (Fraschina et al., 2014). For example, sugarcane monocultures in the African savannah support a high density of generalist species but have a lower diversity of species (Mamba et al., 2019). In the Banni we found a similar pattern as total rodent abundance did not significantly differ from restored grassland but was dominated by a single species T. indica. Given that agriculture here is often a single crop of non-grain produce, it is unlikely that these fields have sufficient resources to sustain multiple species of rodents beyond the harvest season.
Bush Encroachment and Rodent Activity
The most common species, T. indica was more active in Prosopis dominated habitat compared to any other habitats. The structural complexity of habitats can alter perceived predation risk (Ceradini and Chalfoun, 2017), and alter activity patterns of small vulnerable prey, especially on moonlit nights. As expected, in open grasslands, the activity of T. indica reduced with increased moon luminosity, whereas it increased under the cover of sparse Prosopis with higher moon luminosity, as observed in other systems (Kotler et al., 1993; Guiden and Orrock, 2019).
For small-bodied nocturnal rodents, the minimum nighttime temperature is an important predictor of activity (Kotler et al., 1993). Due to a lower surface-to-body mass ratio and reduced thermal conductance, the cost of foraging on colder nights is high and can result in rapid heat loss from the body (Ghosh et al., 1979). As the temperature increased, the activity of T. indica also increased across most habitat types, except under dense Prosopis. This could likely be due to rodents using more open areas adjoining Prosopis thickets on warmer nights. This provides additional evidence that vegetation structure mediates the effect of temperature on the activity of small mammals (Milling et al., 2017).
We found that M. meltada was active in both open grassland and Prosopis dominated habitat, but its activity showed an opposite response toward moonlight and temperature compared to those shown by T. indica. This response of M. meltada might also be driven by other variables such as habitat type or competition, but due to low number of captures we were not able to examine more complex models for this species.
The activity of T. indica was also negatively correlated with the presence of B. bengalensis. Both species are generalists with similar dietary habits (Prakash and Singh, 2005) and are comparable in size (body mass ratio < 1.5). However, B. bengalensis, due to its aggressive nature (Sridhara and Krishnamoorthy, 1983; Meehan, 1984) and higher reproductive capacity (70 young/female/annum) can have a competitive advantage over the more docile, and slower breeding (17.8 young/female/annum) T. indica (Prakash and Singh, 2005). It is likely that T. indica shows some form of spatial/temporal separation to potentially avoid interference competition from B. bengalensis. This response is likely independent of other factors such as year of sampling, since our plots were sampled under relatively similar conditions in consecutive years, and we found no difference in overall rodent abundance between years (t = 1.125, p = 0.298; Figure 5). We were not able to model the interactive effect of habitat and B. bengalensis occurrence on activity due to sample size. Furthermore, B. bengalensis was absent in the first year of sampling, but was remarkably common across all habitat types in the second year. We speculate that this could be due to the increased rainfall in that year. Spatially, B. bengalensis was also found in areas that were inundated for longer periods, and thus had higher soil moisture (Sridhara and Tripathi, 2005). We also observed high consumption of Prosopis bark by B. bengalensis in all Prosopis habitats where the species was found, showing their potential dietary competitive advantage.
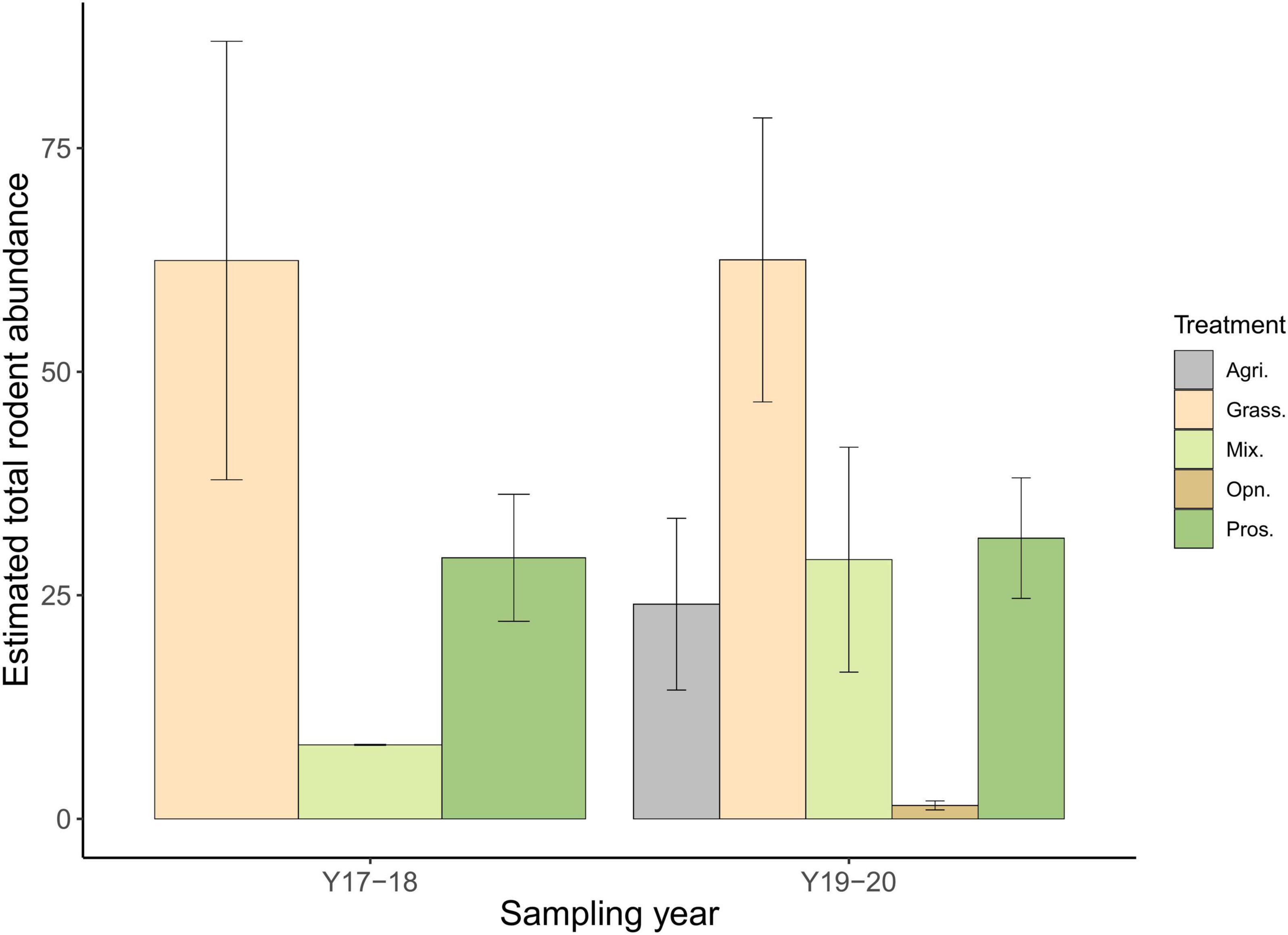
Figure 5. Difference in estimated total rodent abundance between different habitat types and year. There was no significant difference observed (t = 1.125, p = 0.298) in estimated total rodent abundance between sampling years in grassland (N2017–2018 = 3, N2018–2019 = 8) (t = 0.002, p = 0.998), Prosopis dominated habitat (N2017–2018 = 3, N2018–2019 = 7) (t = 0.191, p = 0.852), and sparse Prosopis habitat (N2017–2018 = 3, N2018–2019 = 6).
Overall, the effect of bush encroachment appeared to be highly species specific. Prosopis dominated habitats favor the abundance and activity of generalist rodent species T. indica and B. bengalensis while habitat specialists such as M. hurrianae and G. nanus were absent from bush encroached areas. These patterns of avoiding woody encroached habitat are also reflected in how other species, especially mammalian mesocarnivores such as desert fox Vulpes vulpes pusilla that are dependent on rodents, respond to changes in the habitat (Misher and Vanak, 2021). Though the Prosopis dominated habitat may represent similar diversity and abundance of rodents as do grasslands in Banni, their further expansion in native open brushlands can drastically change species composition at landscape level. These brushlands occupy a substantial proportion of the landscape and have escaped Prosopis encroachment due to annual water logging and high soil salinity, thus supporting highly specialized rodent community that is absent in Prosopis encroached areas.
The landscape has seen a higher mean annual rainfall consecutively for last 2 years. Such pulses of high precipitation can further influence the rodent community in the landscape. Although, we did not find any significant effect of sampling years on total rodent abundance, the species composition during our second sampling year was different due to the presence of B. bengalensis. Jayadevan et al. (2018) did not report presence of B. bengalensis during their sampling in 2016–2017, and we did not detect it either in our first sampling year. Long-term research is required to understand the effect of changing rainfall patterns on the small mammal community of the landscape. It is likely that a long dry spell of more than 2 years may again reduce the population of B. bengalensis, as the species is typically associated with more moist climatic conditions.
Although native, species such T. indica and B. bengalensis are also major agricultural pests in the dry-lands of India (Tripathi, 2014). They have been subjected to systematic long-term eradication programs due to the high economic cost they inflict by damaging crops (Sridhara and Tripathi, 2005). Further expansion of Prosopis along with high rainfall can also facilitate the expansion of generalist pests such as T. indica and B. bengalensis in these landscapes. Finally, our study provides a much-needed example of the impacts of shrub encroachment on vertebrate communities from the Asian continent (Stanton et al., 2018), and a starting point for further studies.
Data Availability Statement
The raw data supporting the conclusions of this article will be made available by the authors on request, without undue reservation.
Ethics Statement
The animal capturing protocol was reviewed and approved by ATREE’s Animal Ethics Committee (Application No: AAEC/109/2019).
Author Contributions
CM and AV conceived and designed the study. CM collected data, performed analyses, and wrote the manuscript. GV collected data. AV provided overall supervision. All authors edited and approved the final manuscript version.
Funding
This study was funded by the Partnerships for Enhanced Engagement in Research (PEER) program supported by the United States Agency for International Development (USAID) under cooperative agreement AID-OAA-A-11-00012 and a National Research Foundation, South Africa grant to AV No. 103659. Article processing charges were funded by the University of KwaZulu-Natal Research Office.
Conflict of Interest
The authors declare that the research was conducted in the absence of any commercial or financial relationships that could be construed as a potential conflict of interest.
Publisher’s Note
All claims expressed in this article are solely those of the authors and do not necessarily represent those of their affiliated organizations, or those of the publisher, the editors and the reviewers. Any product that may be evaluated in this article, or claim that may be made by its manufacturer, is not guaranteed or endorsed by the publisher.
Acknowledgments
We would like to thank Shomen Mukherjee for various inputs during the design phase of the study, and Maria Thaker and Ankila Hiremath for inputs on an earlier draft of the manuscript. We would also like to thank Anjan Katna, Ramya Ravi, and Anusha Chaudhary for their help with different stages of data collection, analysis, and writing. We would also further like to thank Sanjay Molur and Pratap Singh for help with species identification. We are grateful to Pankaj Joshi, Sahjeevan, and RAMBLE (Research and Monitoring in the Banni landscapE) field station director late Nirav Mehta and field assistants Sher Khan, Gagan, Habbu Marwada, Rasul Jat, Kheraj Kharet, and Kabul Halipotra. We thank the pastoralists of the Banni landscape for their warm hospitality.
Supplementary Material
The Supplementary Material for this article can be found online at: https://www.frontiersin.org/articles/10.3389/fevo.2022.755903/full#supplementary-material
References
Archer, S., Boutton, T. W., and Hibbard, K. A. (2001). “Trees in grasslands: biogeochemical consequences of woody plant expansion,” in Global Biogeochemical Cycles in the Climate System, eds E. D. Schulze, S. P. Harrison, M. Heimann, E. A. Holland, J. Lloyd, I. C. Prentice, et al. (San Diego: Academic Press).
Auken, O. W. V. (2009). Causes and consequences of woody plant encroachment into western North American grasslands. J. Environ. Manage 90, 2931–2942. doi: 10.1016/j.jenvman.2009.04.023
Bachen, D. A., Litt, A. R., and Gower, C. N. (2018). Simulating cheatgrass (Bromus tectorum) invasion decreases access to food resources for small mammals in sagebrush steppe. Biol. Invasions 20, 2301–2311. doi: 10.1007/s10530-018-1701-8
Baillargeon, S., and Rivest, L. P. (2007). Rcapture: loglinear models for capture-recapture in R. J. Stat. Softw. 19, 1–31.
Bates, D. M. M., Bolker, B., and Walker, S. (2015). Fitting linear mixed-effects models using lme4. J. Stat. Softw. 67, 1–48.
Bolker, B. M., Brooks, M. E., Clark, C. J., Geange, S. W., Poulsen, J. R., Stevens, M. H. H., et al. (2009). Generalized linear mixed models: a practical guide for ecology and evolution. Trends Ecol. Evol. 24, 127–135. doi: 10.1016/j.tree.2008.10.008
Brown, J. H., and Munger, J. C. (1985). Experimental manipulation of a desert rodent community: food addition and species removal. Ecology 66, 1545–1563.
Ceradini, J. P., and Chalfoun, A. D. (2017). When perception reflects reality: non-native grass invasion alters small mammal risk landscapes and survival. Ecol. Evol. 7, 1823–1835. doi: 10.1002/ece3.2785
Chen, A., Reperant, L., Fischhoff, I. R., and Rubenstein, D. I. (2021). Increased vigilance of plains zebras (Equus quagga) in response to more bush coverage in a Kenyan savanna. Clim. Chang. Ecol. 1:100001.
Cooch, E. G., and White, G. C. (2019). Program MARK: A Gentle Introduction. http://www.phidot.org/software/mark/docs/book/ (accessed May 27, 2021).
Dabadghao, P. M., and Shankarnarayan, K. A. (1973). The Grass Cover of India. New Delhi: The Grass Cover India.
Dyderski, M. K., and Jagodziński, A. M. (2019). Functional traits of acquisitive invasive woody species differ from conservative invasive and native species. NeoBiota 41, 91–113. doi: 10.3897/neobiota.41.31908
Eldridge, D. J., and Soliveres, S. (2014). Are shrubs really a sign of declining ecosystem function? Disentangling the myths and truths of woody encroachment in Australia. Aust. J. Bot. 62, 594–608. doi: 10.1071/bt14137
Fraschina, J., Andrea, L. V., and Maria, B. (2014). Role of landscape scale in the distribution of rodents in an agroecosystem of Argentina. J. Agric. Sci. 6, 22–35.
Ghosh, P. K., Goyal, S. P., and Prakash, I. (1979). Metabolism and ecophysiology of Rajasthan desert rodents. Thermoregulation at a moderately low temperature (21 °C) during winter. J. Arid Environ. 2, 77–83. doi: 10.1016/S0140-1963(18)31706-3
Guiden, P. W., and Orrock, J. L. (2019). Invasive shrubs modify rodent activity timing, revealing a consistent behavioral rule governing diel activity. Behav. Ecol. 30, 1069–1075. doi: 10.1093/beheco/arz050
He, Y., D’Odorico, P., De Wekker, S. F., Fuentes, J. D., and Litvak, M. (2010). On the impact of shrub encroachment on microclimate conditions in the northern Chihuahuan desert. J. Geophys. Res. Atmos. 115:D21120. doi: 10.1029/2009JD013529
Hothorn, T., Bretx, F., and Westfall, P. (2008). Simultaneous inference in general parametric models. Biom. J. 50, 346–363. doi: 10.1002/bimj.200810425
Huggins, R. M. (1991). Some practical aspects of a conditional likelihood approach to capture experiments. Biometrics 47, 725–732. doi: 10.2307/2532158
Jayadevan, A., Mukherjee, S., and Vanak, A. T. (2018). Bush encroachment influences nocturnal rodent community and behaviour in a semi-arid grassland in Gujarat, India. J. Arid Environ. 153, 32–38. doi: 10.1016/j.jaridenv.2017.12.009
Karuaera, N. A. G. (2011). Assessing the Effects of Bush Encroachment on Species Abundance, Composition and Diversity of Small Mammals at the Neudamm Agricultural Farm, Khomas Region, Namibia. Dissertation/Master’s thesis. Namibia: University of Namibia.
Kaur, R., Gonzales, W. L., Llambi, L. D., Soriano, P. J., Callaway, R. M., Rout, M. E., et al. (2012). Community impacts of Prosopis juliflora invasion: biogeographic and congeneric comparisons. PLoS One 7:e44966. doi: 10.1371/journal.pone.0044966
Kluever, B. M., Smith, T. N., and Gese, E. M. (2019). Group effects of a non-native plant invasion on rodent abundance. Ecosphere 10:e02544. doi: 10.1002/ecs2.2544
Kotler, B. P., Brown, J., Mukherjee, S., Berger-Tal, O., and Bouskila, A. (2010). Moonlight avoidance in gerbils reveals a sophisticated interplay among time allocation, vigilance and state-dependent foraging. Proc. R Soc. B Biol. Sci. 277, 1469–1474. doi: 10.1098/rspb.2009.2036
Kotler, B. P., Brown, J. S., and Mitchell, W. A. (1993). Environmental factors affecting patch use in two species of gerbilline rodents. J. Mammal. 74, 614–620. doi: 10.2307/1382281
Loggins, A. A., Monadjem, A., Kruger, L. M., Reichert, B. E., and McCleery, R. A. (2019a). Vegetation structure shapes small mammal communities in African savannas. J. Mammal. 100, 1243–1252. doi: 10.1093/jmammal/gyz100
Loggins, A. A., Shrader, A. M., Monadjem, A., and McCleery, R. A. (2019b). Shrub cover homogenizes small mammals’ activity and perceived predation risk. Sci. Rep. 9:16857. doi: 10.1038/s41598-019-53071-y
Malo, A. F., Godsall, B., Prebble, C., Grange, Z., McCandless, S., Taylor, A., et al. (2013). Positive effects of an invasive shrub on aggregation and abundance of a native small rodent. Behav. Ecol. 24, 759–767. doi: 10.1093/beheco/ars202
Mamba, M., Fasel, N. J., Mahlaba, T. A. M., Austin, J. D., McCleery, R. A., and Monadjem, A. (2019). Influence of sugarcane plantations on the population dynamics and community structure of small mammals in a savanna-agricultural landscape. Glob. Ecol. Conserv. 20:e00752. doi: 10.1016/j.gecco.2019.e00752
Milling, C. R., Rachlow, J., Johnson, T., Forbey, J., and Shipley, L. (2017). Seasonal variation in behavioral thermoregulation and predator avoidance in a small mammal. Behav. Ecol. 28, 1236–1247. doi: 10.1093/beheco/arx084
Misher, C., and Vanak, A. T. (2021). Occupancy and diet of the Indian desert fox Vulpes vulpes pusilla in a Prosopis juliflora invaded semi-arid grassland. Wildlife Biol. 2021:wlb.00781. doi: 10.2981/wlb.00781
Mogashoa, R., Dlamini, P., and Gxasheka, M. (2020). Grass species richness decreases along a woody plant encroachment gradient in a semi-arid savanna grassland, South Africa. Landsc. Ecol. 36, 617–636. doi: 10.1007/s10980-020-01150-1
Murphy, B. P., Lehmann, C. E. R., Smith, J. R., and Lawes, M. J. (2014). Fire regimes and woody biomass dynamics in Australian savannas. J. Biogeogr. 41, 133–144. doi: 10.1111/jbi.12204
Nerlekar, A. N., Mehta, N., Bhagwat, M., Misher, C., Joshi, P., and Hiremath, A. J. (2021). Removal or utilisation? Testing alternative approaches to the management of an invasive woody legume in an arid Indian grassland. Restor. Ecol. 30:e13477. doi: 10.1111/rec.13477
O’Farrell, M. J. (1974). Seasonal activity patterns of rodents in a sagebrush community. J. Mammal. 55, 809–823. doi: 10.2307/1379409
Ostoja, S. M., and Schupp, E. W. (2009). Conversion of sagebrush shrublands to exotic annual grasslands negatively impacts small mammal communities. Divers. Distrib. 15, 863–870. doi: 10.1111/j.1472-4642.2009.00593.x
Popp, A., Schwager, M., Blaum, N., and Jeltsch, F. (2007). Simulating the impacts of vegetation structure on the occurrence of a small mammalian carnivore in semi-arid savanna rangelands. Ecol. Modell. 209, 136–148. doi: 10.1016/j.ecolmodel.2007.06.017
Prakash, I. P., and Singh, P. (2005). Ecology of Small Mammals of Desert and Montane Ecosystems. Jodhpur: Scientific publishers (India).
Prugh, L. R., and Golden, C. D. (2014). Does moonlight increase predation risk? Meta-analysis reveals divergent responses of nocturnal mammals to lunar cycles. J. Anim. Ecol. 83, 504–514. doi: 10.1111/1365-2656.12148
R Development Core Team (2020). R: a Language and Environment for Statistical Computing. Version 4.0.2.
Ramesh, D., Home, C., Jhala, Y. V., and Qureshi, Q. (2013). Calibration of a burrow count index for the Indian desert jird, Meriones hurrianae. Popul. Ecol. 55, 241–245. doi: 10.1007/s10144-012-0340-7
Ratajczak, Z., Nippert, J. B., and Collins, S. L. (2012). Woody encroachment decreases diversity across North American grasslands and savannas. Rep. Ecol. 93, 697–703. doi: 10.1890/11-1199.1
Rivest, L.-P., and Lévesque, T. (2001). Improved log-linear model estimators of abundance in capture-recapture experiments. Can. J. Stat. 29, 555–572. doi: 10.2307/3316007
Singh, P., Gopalaswamy, A. M., and Karanth, K. U. (2010). Factors influencing densities of striped hyenas (Hyaena hyaena) in arid regions of India. J. Mammal. 91, 1152–1159. doi: 10.1644/09-MAMM-A-159.1
Smith, T. N., Gese, E., and Kluever, B. M. (2017). Evaluating the impact of an exotic plant invasion on rodent community richness and abundance. West. N. Am. Nat. 77, 515–525.
Sovie, A. R., Greene, D. U., and McCleery, R. A. (2020). Woody cover mediates fox and gray squirrel interactions. Front. Ecol. Evol. 8:239. doi: 10.3389/fevo.2020.00239
Sridhara, S., and Krishnamoorthy, R. V. (1983). Aggressive behaviour of captiveBandicota bengalensis. Proc. Anim. Sci. 92, 185–191. doi: 10.1007/BF03186185
Sridhara, S., and Tripathi, R. S. (2005). Distribution of Rodent in Indian Agriculture. New Delhi: Network Coordinator for Indian Council of Agricultural Research (ICAR).
Stanton, R. A., Boone, W. W., Soto-Shoender, J., Fletcher, R. J., Blaum, N., and McCleery, R. A. (2018). Shrub encroachment and vertebrate diversity: a global meta-analysis. Glob. Ecol. Biogeogr. 27, 368–379. doi: 10.1111/geb.12675
Stevens, N., Erasmus, B. F. N., Archibald, S., and Bond, W. J. (2016). Woody encroachment over 70 years in South African savannahs: overgrazing, global change or extinction aftershock? Phil. Trans. R. Soc. B Biol. Sci. 371:20150437. doi: 10.1098/rstb.2015.0437
Sundaram, B., and Hiremath, A. J. (2012). Lantana camara invasion in a heterogeneous landscape: Patterns of spread and correlation with changes in native vegetation. Biol. Invasions 14, 1127–1141. doi: 10.1007/s10530-011-0144-2
Tripathi, R. S. (2014). “Integrated management of rodent pests,” in Integrated Pest Management: Current Concepts and Ecological Perspective, eds D. P. Abrol (New York, NY: Elsevier Inc). doi: 10.1016/B978-0-12-398529-3.00022-1
Tyler, C., Pullin, A. S., and Stewart, G. B. (2006). Effectiveness of management interventions to control invasion by Rhododendron ponticum. Environ. Manage. 37, 513–522. doi: 10.1007/s00267-005-0127-0
Vaibhav, V., Inamdar, A. B., and Bajaj, D. N. (2012). “Above ground biomass and carbon stock estimation from Prosopis juliflora in Banni grassland using satellite and ancillary data,” in Proceedings of the 33rd Asian Conference on Remote Sensing 2012, ACRS-2012 (Tokyo: Asian Association on Remote Sensing), 1286–1291.
White, R., Murray, S., and Rohweder, M. (2000). Pilot Analysis of Global Ecosystems: Grassland Ecosystems. Washington DC: World Resource Inst.
Keywords: woody encroachment, dry grassland, savanna ecosystem, rodents, Prosopis juliflora
Citation: Misher C, Vats G and Vanak AT (2022) Differential Responses of Small Mammals to Woody Encroachment in a Semi-Arid Grassland. Front. Ecol. Evol. 10:755903. doi: 10.3389/fevo.2022.755903
Received: 09 August 2021; Accepted: 01 March 2022;
Published: 24 March 2022.
Edited by:
Christopher Richard Dickman, The University of Sydney, AustraliaReviewed by:
Rachel Vera Blakey, University of California, Los Angeles, United StatesYu Liu, Northwest A&F University, China
Copyright © 2022 Misher, Vats and Vanak. This is an open-access article distributed under the terms of the Creative Commons Attribution License (CC BY). The use, distribution or reproduction in other forums is permitted, provided the original author(s) and the copyright owner(s) are credited and that the original publication in this journal is cited, in accordance with accepted academic practice. No use, distribution or reproduction is permitted which does not comply with these terms.
*Correspondence: Chetan Misher, chetan.misher@atree.org