Evolution of terrestrial herbivory: nutrient stoichiometry, body size, and dietary diversity
- Department of Earth and Planetary Sciences, Stanford University, Stanford, CA, United States
Direct fossil preservation of leaf damage, arthropod mouthparts, and vertebrate teeth has understandably led to a focus on oral processing of plant material when considering the evolution of herbivory in deep time. Here, nutrient stoichiometry is advocated as an important alternative constraint on the evolution of herbivory. Most life possesses C:N ratios of approximately 7:1, but uniquely among land plants, that ratio can be skewed to 1,000:1 in some tissues due to the abundance of cell wall structural polymers that lack nitrogen entirely. Since the superabundance of carbon is unevenly distributed between and within plant organs and tissues, avoidance is a viable strategy for herbivores, but availability is dependent on herbivore body size. Sub-millimeter herbivores can attack plants cell-by-cell, avoiding cell wall consumption entirely in favor of cell contents, thereby presenting little stoichiometric distinction between herbivory and consumption of animals, fungi, or unicellular life. Insect-sized herbivory at least allows avoidance of the most carbon-rich/nutrient-poor tissues within a plant organ. However, vertebrate sizes prevent such selectivity. The increasing challenges of nutrient stoichiometry with increasing herbivore body size are recapitulated through time in the fossil record. The first herbivores were microherbivores that can avoid cell wall consumption—present already with their first opportunity for fossil preservation in the Early Devonian. Tissue-specific consumption by insect herbivores followed in the Carboniferous. One hundred fifty million years would pass after the first microherbivory record before vertebrate herbivory would reach its modern prevalence.
1 Introduction
1.1 Paleontological record of herbivory
For vertebrate and insect fossils alike, discerning diet involves a focus on the mouthparts that engage in the mechanics of feeding as filtered through consideration of which materials have the greatest opportunity for fossil preservation. For vertebrates, that means teeth: shape, low-crowned versus high-crowned, and whether wear patterns suggest browsing, grazing, or specialization on fruits and seeds (Strömberg, 2011; Christensen, 2014; Mihlbachler et al., 2016). For insects, an early focus was on mouthpart function, such as chewing mandibles versus piercing stylets (Labandeira and Sepkoski, 1993; Labandeira, 1997).
Direct preservation of interaction between identifiable herbivore and plant host is rare. Coprolites can provide detail regarding what was consumed but the degradation inherent in digestion tends to limit plant identification to higher taxonomic levels (Prasad et al., 2005; Khosla et al., 2015). As for the herbivore, even if the dung is so large as to require a sauropod or so small as to require a mite, that does not specify which sauropod or which mite (Edwards et al., 1995; Habgood et al., 2003). In situ gut contents can provide better constraint (Krassilov and Rasnitsyn, 1996; Sues and Reisz, 1998; Labandeira, 2000; Huang et al., 2016; Zheng et al., 2018; O’Connor, 2019), but preservation is uncommon. Fossil insects dusted with pollen grains can provide reliable clues, but only applies to a narrow class of interactions (Labandeira et al., 2007a; Peñalver et al., 2012; Peñalver et al., 2015; Wappler et al., 2015; Labandeira et al., 2016; Khramov et al., 2023).
Another side of the interaction is the traces of damage left in plant organs. Although not available after wholesale consumption by vertebrate herbivores, fossil leaf damage has been transformative as a record of insect herbivory; many paleoentomologists now work almost exclusively from fossil localities where any direct insect preservation is incidental. Feeding traces, oviposition scars, and galls can all be preserved (Labandeira et al., 2007b). The widespread availability and abundance of plant fossils invites comparative work, for example, of how damage diversity and frequency change with mass extinction or other perturbations (Wilf et al., 2006; Currano et al., 2008).
Because damage is the direct fossil preservation of an ecological interaction, an impulse has been to apply modern ecological analyses that investigate species networks. However, these interactions are ones where only one partner is known with certainty, the plant. Without more detail that might allow further discrimination, a leaf miner trail could have been produced by sawfly, moth, fly, or beetle. This one-sidedness means that a damage type deemed generalist as seen across several plant species may have represented an unrelated series of specialist herbivores producing similar damage, each on a different plant. Even when a damage type is deemed diagnostic for a particular insect lineage, that can still mean thousands of species given the prodigiousness of insects (Wilf et al., 2000) and dozens of insect species may be capable of making similar damage even at individual localities (Carvalho et al., 2014). Furthermore, modern ecological analyses have been worked out assuming the sampling to completion of the entire fauna and flora as available to modern ecology, but complete sampling is never available from the fossil record (Schachat et al., 2023). Acknowledging these limitations, metrics have been advocated for comparing damage richness across fossil localities without requiring full species-level resolution and complete sampling (Schachat et al., 2022).
1.2 What is missing?
This history of paleontological study documents finer and finer dissection of detail from the available fossils to illuminate herbivory through time. That approach has guided progress, but also suggests blind spots. Not all aspects of the system have been preserved; regardless of how deep one digs into the fossils, the fossil record of the evolution of herbivory is not the same thing as the evolution of herbivory.
First, paleontological emphasis is unavoidably on the fossils: the gazelles and grasshoppers. However, nematodes can be herbivores (Blaxter, 2011; Kiontke and Fitch, 2013; Potapov et al., 2022); so, too, can tardigrades, symphylan myriapods, and collembolan hexapods (Eltoum and Berry, 1985; Endlweber et al., 2009; Schill et al., 2011; Potapov et al., 2022). Herbivorous mites do receive consideration in fossil leaf damage studies as possible culprits for some galls (Labandeira et al., 2007b), but mites can also feed on plant tissues in more varied ways (Labandeira et al., 2014; Potapov et al., 2022). Indeed, the fossil damage record itself is biased in that these less-considered herbivores typically feed on roots and root hairs—underrepresented as fossils versus plant organs shed above ground. Although arriving on land much later, decapod crustaceans and gastropod mollusks can be herbivores as well; snails and slugs can damage leaves but only rarely are considered explicitly in fossil studies (Carvalho et al., 2014; Labandeira et al., 2014).
Second, the nature of fossil preservation and how herbivory is discerned requires emphasis on the physical act of feeding: the shredding of fibrous tissue or cracking of nuts in the case of vertebrates, and the sipping of nectar or the excavating of leaves in the case of insects. As an example of this pervasive emphasis, frass-filled galleries preserved in Early Devonian specimens of Prototaxites (Hueber, 2001) have been compared to wood-feeding, 15–20 million years (Myr) prior to the evolution of wood (Labandeira, 2007). From the perspective of feeding mechanics, inclusion with examples of wood consumption is reasonable: Prototaxites could produce large trunks composed of cell wall material durable enough to survive fluvial transport as logs. An animal capable of burrowing into Prototaxites probably would have been physically capable of wood boring if wood had existed at the time. However, metabolism is biochemistry, not biomechanics. Prototaxites was a putative fungus and unquestionably was not a vascular plant (Hueber, 2001; Boyce et al., 2007; Nelsen and Boyce, 2022). Thus, cell wall composition in Prototaxites was potentially chitin and definitely not the lignocellulose of wood.
When chemical properties have been considered for the evolution of herbivory, the context is typically that of organic biochemistry. For insects, emphasis tends to be on plant resins and secondary defensive compounds that either interfere with herbivore metabolism, such as flavonoids or tannins, or gum up mouth parts, such as latex—with this latter category bringing the topic back to the physical act of feeding (Krings et al., 2003; Martìnez-Delclòs et al., 2004; Labandeira et al., 2014; McCoy et al., 2022). For vertebrate lineages, emphasis has centered on acquisition of microbial symbionts for cellulose digestion (Sues and Reisz, 1998; Reisz and Fröbisch, 2014). Expectation of similar symbiont necessities for insects has been muddled by documentation of native enzymatic capacity for cellulose breakdown in some—roaches and termites, grasshoppers, beetles—but not all (Watanabe and Tokuda, 2001; Watanabe and Tokuda, 2010).
Focus on cellulose breakdown obscures that no organism can be constructed entirely of sugar. Capacity for cellulose digestion can be a useful part of catabolic metabolism to fuel behavior but is less relevant to the anabolic production of new cells and tissues, including for reproduction. Other nutrients are essential, including nitrogen and phosphorus that are widely recognized as potentially limiting in modern environments. This foundation, as established for decades in fields like soil biology or physiological ecology (Sterner and Elser, 2003; Bardgett, 2005; Karasov and del Rio, 2020), is rarely considered in the context of the deep-time evolution of herbivory. Nutrient stoichiometry may not be subject to quantification from fossils but is advocated here as an important constraint on dietary evolution during the early assembly of terrestrial ecosystems.
2 Nutrient stoichiometry and the terrestrial biota
Nucleic acids, membrane phospholipids, and proteins all contain nitrogen and/or phosphorus (Figure 1A), resulting in relatively stable ratios of carbon, nitrogen, and phosphorus in living cells, i.e., the classic Redfield Ratio of 106:16:1 (Redfield, 1958). Precipitation of biominerals such as calcium carbonate is a frequent structural addition to marine phytoplankton and algae, but rapid diffusive gas exchange in a subaerial context on land makes photosynthetic carbon fixation the more readily available source of structural materials. The Redfield Ratio, based on marine plankton, is roughly followed by animal life across all environments but is greatly skewed by land plants due to an excess of organic carbon (Elser et al., 2000).
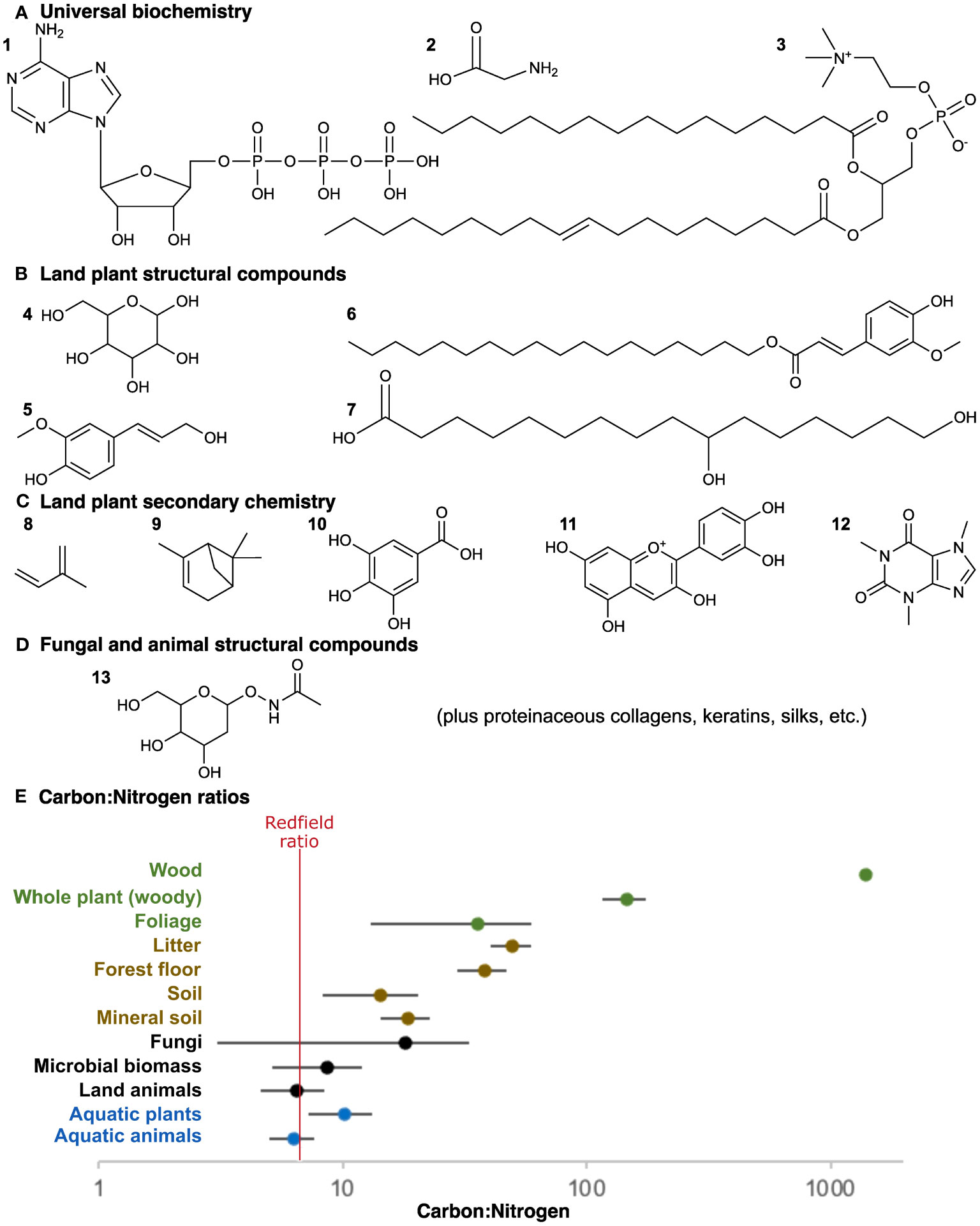
Figure 1 Nutrient stoichiometry. (A) Many universal biochemical constituents contain nitrogen and/or phosphorus: (1) nucleic acids, (2) amino acids, and (3) phospholipids. (B) Land plant structural polymers are exclusively carbon-based with no nitrogen or phosphorus: (4) glucose monomer of cellulose, and example monomers of (5) lignin, (6) suberin, and (7) cutin. Not shown, sporopollenin is also a mixture of aliphatic and aromatic moieties like suberin. Other than cellulose, these polymers can be complexly heterogeneous both in their bonding and in their monomeric content; the monomers shown are exemplars of a wider diversity. (C) Land plant secondary chemistry, in most cases, is also exclusively carbon-based: (8) isoprene base of the terpenoids common in latex and resins, (9) a terpene, (10) a tannin, (11) an anthocyanin derivative of flavonoids, and (12) an alkaloid. Alkaloids are distinct in that they do involve nitrogen. (D) Animal and fungal structural polymers do contain nitrogen: (13) N-acetyl glucosamine used as a monomer in chitin, as in fungi and some animals, and also employed in a different polymeric context with some bacterial cell walls. Other structural polymers of animals are proteins and, thus, contain nitrogen. (E) C:N ratios across different ecosystem components as compared to the Redfield ratio (red), including freshwater aquatic animals and plants (blue), non-plant constituents of the terrestrial biota (black), litter and soil organic matter ultimately derived from land plant material (brown), and land plants (green). Values collected from the literature as discussed further in the text (Redfield, 1958; Elser et al., 2000; Cleveland and Liptzin, 2007; Yang and Luo, 2011; Smyth et al., 2016; Zhang and Elser, 2017).
Land plant structural compounds include cellulose and other polysaccharides, lignin, cutin and cuticular waxes, suberin, and sporopollenin (Hatcher and Clifford, 1997; Bernards, 2002; Fich et al., 2016; Li et al., 2019). As with cellulose, all these compounds are at least somewhat resistant to enzymatic attack. Their recalcitrance has been a focus because these plant structural compounds have been primary contributions to fossil organic matter (Van Bergen et al., 1995; Hatcher and Clifford, 1997; Boyce et al., 2002; Gupta et al., 2007; Boyce et al., 2010). However, all these plant structural compounds—making up most of terrestrial biomass—can also be recognized as an abundance of carbon coupled with a complete lack of nitrogen and phosphorus (Figure 1B). Much of plant secondary chemistry is also exclusively carbon-based (Figure 1C). In contrast, heterotrophs like animals and fungi have structural compounds with nitrogen in abundances consistent with the organism overall, such as chitin, collagen, and keratin (Figure 1D). With proteins, nucleic acids, and phospholipids being universal requirements, the interior biochemistry of plant cells will still have similar nutritional content as other life for a potential consumer. However, no organism could ever be constructed exclusively from herbivorous consumption of the extracellular polymers found in plant cell walls and cuticles, regardless of how much was consumed. Indeed, herbivore cellulase activity might be viewed as maceration as much as digestion, speeding access to nutrient-rich cell contents.
Although not digesting cellulose as a sugar source, phloem-feeding Hemiptera provide a concrete illustration of the limitations of a sugar-based diet: most is excreted to concentrate small quantities of essential nutrients that are also transported in phloem (Mittler, 1958). Ants and others may collect this excreted honeydew, but as a fraction of a more nutrient-rich diet. Any structural use of the flood of sugar hemipterans endure would be in their creativity with waxes as protective shells and camouflaging flourishes—like the sugar, those waxes represent an abundance of carbon with no nitrogen or phosphorus. A cow trying to subsist solely off the breakdown of cellulose and its glucose monomers would be unable to grow, reproduce, or lactate.
Herbivory is a difficult stoichiometric proposition and is so specifically on land (Figure 1E). Through billions of years of evolution, life may not have strayed far from C:N ratios of approximately 7:1 (Elser et al., 2000; Cleveland and Liptzin, 2007; Neveu et al., 2016), but uniquely among land plants that ratio can be skewed to extremes due to the abundance of cell wall structural polymers that lack nitrogen entirely. Woody trees average approximately 150:1 and wood itself averages more than 1,000:1 (Yang and Luo, 2011; Smyth et al., 2016). The skewed nutrient ratios accompanying land plant evolution has been recognized as an important shift in the geological carbon cycle (Lenton et al., 2016), but this skew also matters for the evolution of ecological interactions between the organisms themselves. Land plants represent a challenge to heterotrophic consumption unique in Earth history.
3 Body size-based opportunities for herbivory
Unless specializing on the concentrated nutrition of seeds, tetrapod herbivores tend to be large and possess voluminous guts to filter an adequate quantity of limiting nutrients from a large quantity of nutrient-poor food (Karasov and del Rio, 2020). Mammals smaller than 1 kg require reingestion of feces to double the effective length of their digestive tract and other modifications to their behavior and digestion in order to accommodate herbivory despite their small size (Hirakawa, 2001). Yet, the largest extant insect herbivore weighs just 70 g; herbivorous mites and nematodes are orders of magnitude smaller.
Fundamental biological differences are important for meeting the challenge of terrestrial herbivory despite the small body size. Nitrogen fixation by microbial symbionts is found in the gut or other tissues of at least some insect herbivores, and most terrestrial arthropods have Malpighian tubules that empty nitrogenous waste into the midgut with the opportunity for reabsorption after microbial processing (Bar-Shmuel et al., 2020; Ren et al., 2022). Such avenues for nitrogen fixation and recycling appear insignificant in vertebrates (Leng and Nolan, 1984). Additionally, metamorphosis in winged insects allows for extreme dietary change over ontogeny. An adult diet even just of nectar can be viable if the nutrient stores needed for egg production were acquired as a leaf-feeding caterpillar or host-consuming parasitoid (Coll and Guershon, 2002; O’Brien et al., 2002)—although this particular opportunity applies to no potential herbivores other than winged insects.
Biological differences aside, small body size itself can help solve the stoichiometric problems of herbivory: since the carbon superabundance of plants is unevenly distributed, avoidance is a possibility but is only so for the small. The smallest herbivores with millimeter-scale or smaller body widths can attack plants cell-by-cell, avoiding cell wall consumption entirely in favor of cell contents and thereby encountering little stoichiometric distinction between herbivory versus consumption of animals, fungi, or unicellular life. Microherbivores, such as nematodes and tardigrades, have mouthparts consisting of stylets to puncture cell walls and access the interiors of cells to the exclusion of nutritionally challenging wall material (Kiontke and Fitch, 2013; Potapov et al., 2022). Flaps and stringers of disrupted wall material are left behind with the cell-by-cell feeding on cell contents seen in early liverwort fossils, as would be consistent with herbivory by mites or other microfauna (Labandeira et al., 2014).
At the centimeter scale, insect herbivory at least allows avoidance of the most carbon-rich/nutrient-poor tissues within a plant organ (Labandeira et al., 2007b). Mandibulate margin-feeding insects will typically avoid the larger leaf veins, where cell wall material is abundant and nutritious cell contents are lost during xylem differentiation. Hole-feeders will often leave either the upper or lower cuticle intact. Leaf miners avoid both upper and lower cuticles along with the larger veins, focusing on the less nutrient-poor photosynthetic mesophyll. These feeding strategies can impose size constraints even among insects. For example, the prevalence of small size and leaf mining among basal moths (Mitter et al., 2017) are not independent traits. Adult size reflects the largest juvenile; if the juvenile must live within the thinness of a leaf, then the adult insect can never be big. Larger sizes within adult Macroheterocera necessarily would have required the transition to external feeding by the caterpillars.
4 Alternatives to herbivory
Predation presents the simplest and most readily available diet, free from challenges of nitrogen and phosphorus availability since prey and predator will have a similar composition. The preservation of coprolites from predatory tetrapods by authigenic phosphate mineral precipitation highlights the potential for actual nutrient excess, particularly if bone is consumed (Chin et al., 1998).
Detritivory is also a favorable alternative to herbivory—perhaps surprising since organic detritus was either passed over by herbivores when alive or eaten and passed through as feces. However, nitrogen content in litter accumulates over time via prokaryotic nitrogen fixation (Crocker and Major, 1955; Yang and Luo, 2011; Smyth et al., 2016). Furthermore, detritivores may ingest detritus but typically digest only the microbial life engaged in the actual chemical reduction of detrital organic matter, primarily bacteria and fungi (Bardgett, 2005). Thus, detritivores are like predators in the consumption of food that is more stoichiometrically balanced than would be the bulk consumption of plant material (Cleveland and Liptzin, 2007; Zhang and Elser, 2017).
Detritivory can scale up to animals larger than the sub-millimeter body sizes of nematodes and mites. Terrestrial annelid detritivores include earthworms not just enchytraeids; myriapods have millipedes not just pauropods. As with herbivory, however, larger sizes involve tolerance of a lower nutritional content of the material being ingested. Whereas nematodes can even be selective over which bacteria are consumed, larger detritivores require volume feeding and greater gut capacity (Potapov et al., 2022). Alternatively, detritivores can maintain selectivity despite larger body sizes via the sophistication of active fungal farming as with leafcutter ants, Macrotermitinae termites, and ambrosia beetles, or at least fungal inoculation of the substrate in the case of wood wasps; these cultivations can also involve nitrogen-fixing prokaryotes (Bar-Shmuel et al., 2020; Ren et al., 2022). As fungus feeders, these insects all are more like detritivores than herbivores, regardless of whether some may play an active role in harvesting vegetation.
5 Discussion—the evolutionary history of dietary ecology
The early assembly of terrestrial ecosystems shows remarkable correspondence between the sequential addition of different dietary strategies and the extent to which those strategies avoid the stoichiometric challenges unique to terrestrial life (Figure 2). The first Silurian fossils of a land fauna are all either predators (scorpion and trigontarbid arachnids as well as centipede myriapods) or detritivores (millipede myriapods) (Jeram et al., 1990; Shear and Edgecombe, 2010; Dunlop and Selden, 2013). Predators added in the Devonian record include harvestmen and pseudoscorpion arachnids (Dunlop and Garwood, 2017; Harms and Dunlop, 2017). Other lineages preserved for the first time in the Devonian—nematodes, mites, collembolans, and apterygote insects—include detritivory prominently among their modern ecologies (Shear et al., 1984; Poinar et al., 2008; Dunlop and Garwood, 2017; Potapov et al., 2022).
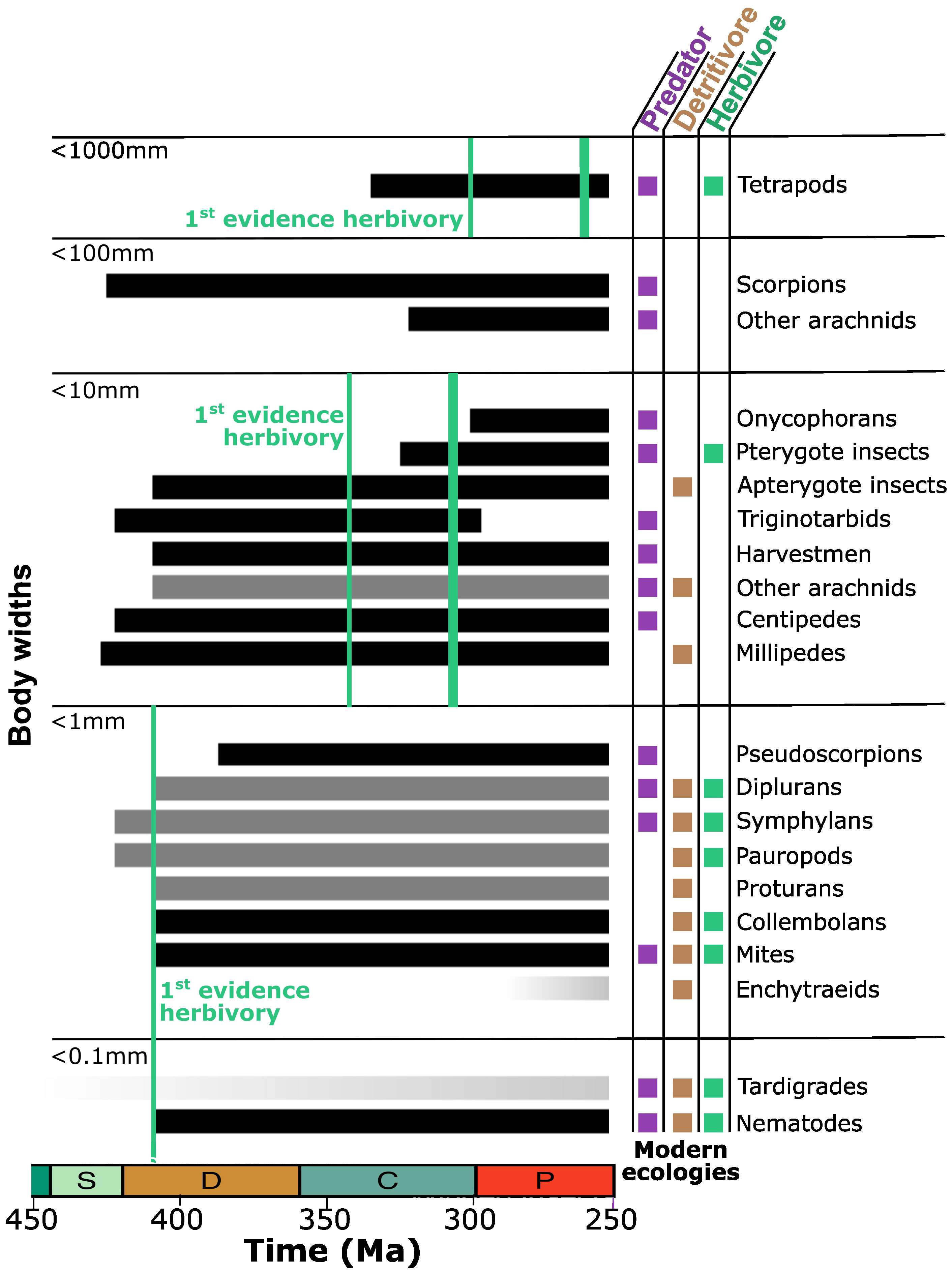
Figure 2 Early accumulation of the terrestrial fauna in the Paleozoic. Lineages are binned by typical body sizes, although outliers often exist (e.g., fossil arthropleuran myriapods and palaeodictyopteran insects, living heterojapygid diplurans). Modern ecologies are indicated. Temporal ranges of lineages depicted in black are based on fossil occurrences. Lineages depicted in gray have no direct fossil record but can be recognized to have been distinct based on close terrestrial relatives that do have a fossil record. The two lineages depicted with a darkening gradient through time—eutardigrades and enchytraeid annelids—have neither fossil record nor close terrestrial relatives but are included based on time-calibrated phylogenies. By size class, initial fossil evidence of herbivory is indicated with the first green line. For insects and vertebrates, the second green line indicates when herbivory first reached its modern prominence. Time depicted from 450 to 250 Ma spans a fraction of the Ordovician through Silurian, Devonian, Carboniferous, and Permian. Information presented was collected from the literature as discussed further in the text (Shear et al., 1984; Jeram et al., 1990; Bishop et al., 2015; Garwood et al., 2016; Schachat et al., 2018; Erséus et al., 2020; Magalhaes et al., 2020; Brookfield et al., 2021; Howard et al., 2022; Potapov et al., 2022).
Herbivory is recorded in the Devonian but as microherbivory. The Devonian nematode fossils are directly preserved inside plant hosts, and the cell-by-cell damage recorded in a liverwort fossil also represents microherbivory, whether caused by mite, collembolan, or some other lineage from the microfauna that has so far gone unpreserved (Poinar et al., 2008; Labandeira et al., 2014). The few other instances of Devonian plant damage are also consistent with microherbivores and or cell puncture feeding (Kevan et al., 1975; Banks, 1981). The fossil record is incomplete, particularly for the small, but phylogenetic relationships (Shear and Edgecombe, 2010; Regier et al., 2013; Misof et al., 2014; Howard et al., 2020) can at least be used to establish what ghost lineages must have been present in the Devonian based on the fossil preservation of a sibling lineage. Only predators, detritivores, and microherbivores are to be found among the modern representation of those lineages: pauropod and symphylan myriapods, dipluran and proturan hexapods, and various arachnids (Potapov et al., 2022). This sibling approach to lineage dating is not available for tardigrades, but molecular clock studies are broadly consistent with terrestriality by the mid-Paleozoic (Marchioro et al., 2012; Rota-Stabelli et al., 2013; Howard et al., 2022). Similarly, there is little cause to expect that the first preservation of terrestrial nematodes and mites represents their time of true origin, but any unrecorded prehistory for these lineages can still be expected to have involved predators, detritivores, and/or microherbivores.
Lags have been noted between the evolution of different plant organs and their first exploitation by herbivores (Labandeira, 2007). For the first microherbivores attacking early plants cell-by-cell, however, the living tissues of stem, leaf, and root should be largely equivalent other than their proximity to the soil. Alternatively, assessment of evolutionary lags might best be focused on the animals themselves and the appearance of herbivory in different size classes. Interpreting the record of the smallest terrestrial animal life is complicated by how infrequently it is preserved, and we cannot say how early nematodes were first on land. However, we can specify that the first time that nematodes and other microfauna are preserved, microherbivory was already in place; no known lag exists.
Lags do exist in the evolution of herbivory among larger animals. Sixty million years passed between the first cm-scale insect preserved in the Lower Devonian Rhynie Chert and the first leaf damage seen in the Early Carboniferous (Iannuzzi and Labandeira, 2008; Gorman, 2013); another 20 Myr would pass before the explosive diversification of winged insects (Schachat et al., 2018) followed by insect herbivory becoming systemically pervasive (Labandeira, 1997; Labandeira, 1998). Thirty million years passed between the first tetrapod vertebrates showing the capacity for life on land as adults (Milner and Sequeira, 1993; Smithson et al., 1993; Bishop et al., 2015) and the first to have dentition consistent with herbivory in the latest Carboniferous (Reisz and Fröbisch, 2014); another 40 Myr would pass before vertebrate herbivores reached their modern prevalence in the Late Permian (Sues and Reisz, 1998).
At some point since the Ordovician appearance of land plants, the terrestrial fauna transitioned from exclusively predators and detritivores to predators, detritivores, and microherbivores. Those systems were in place for at least 100 Myr, until the explosive diversification of winged insects and their centimeter- to decimeter-scale herbivores. Even then, 100 Myr more would pass before vertebrate herbivores would reach their modern prominence. The challenges of terrestrial herbivory may even have strengthened over time. Given a lack of lignin and an incomplete cuticle, modern bryophytic land plants are intermediate in their stoichiometry between algae and vascular plant leaves (Fernández-Martínez et al., 2021); the earliest pre-vascular land plants can be expected to have been similarly intermediate. With the extremity of the carbon overabundance in vascular plants likely to have fluctuated somewhat over time along with atmospheric CO2 (Reich et al., 2006; Sardans and Peñuelas, 2012), the plants themselves may have presented moving targets to potential herbivores, although this CO2 dependence may have been largely confined to post-Cretaceous flowering plants (Boyce and Zwieniecki, 2012). The environmental balance between nitrogen and phosphorus limitation may even have shifted over the 460-Myr history of land plants (Boyce et al., 2023).
The transitions with the evolution of larger herbivore size classes cannot be viewed as a progressive evolutionary sequence since spanning lineages that are only distantly related and have no terrestrial common ancestry. While the smallest tetrapods did scarcely overlap in size with the largest insects—and continue to do so now, albeit at different sizes—the first vertebrate herbivores were meter-scale, and no vertebrate has ever been small enough to graze bacteria or feed on plants cell-by-cell along with the mites and nematodes. Yet, introduction of herbivory among insects and tetrapods represented increases in body size over an original trophic floor of primary consumers no larger than detritivores and microherbivores. Introduction of larger body sizes in primary consumers will also influence the potential body sizes of secondary consumers (Knoll and Follows, 2016). After the first evidence of insect herbivory, terrestrially adapted tetrapods are first seen, initially all predators. After vertebrate herbivory became abundant, the largest vertebrate body sizes began to increase dramatically in the Triassic. Surmounting the stoichiometric challenges of herbivory can be seen as a key constraint on the tempo of ecosystem assembly.
Data availability statement
The original contributions presented in the study are included in the article. Further inquiries can be directed to the corresponding author.
Author contributions
CB: Conceptualization, Writing – original draft.
Funding
The author(s) declare that no financial support was received for the research, authorship, and/or publication of this article.
Acknowledgments
The author thanks the editors for suggesting a submission to this volume. S. Schachat, C. Labandeira, S. Callaghan, and T. Frank have provided useful discussion.
Conflict of interest
The author declares that the research was conducted in the absence of any commercial or financial relationships that could be construed as a potential conflict of interest.
Publisher’s note
All claims expressed in this article are solely those of the authors and do not necessarily represent those of their affiliated organizations, or those of the publisher, the editors and the reviewers. Any product that may be evaluated in this article, or claim that may be made by its manufacturer, is not guaranteed or endorsed by the publisher.
References
Banks H. P. (1981). Peridermal activity (wound repair) in an Early Devonian (Emsian) trimerophyte from the Gaspé Peninsula, Canada. Palaeobotanist 28, 20–25. doi: 10.54991/jop.1981.1395
Bardgett R. (2005). The biology of soil: a community and ecosystem approach (Oxford: Oxford University Press).
Bar-Shmuel N., Behar A., Segoli M. (2020). What do we know about biological nitrogen fixation in insects? Evidence and implications for the insect and the ecosystem. Insect Sci. 27, 392–403. doi: 10.1111/1744-7917.12697
Bishop P. J., Walmsley C. W., Phillips M. J., Quayle M. R., Boisvert C. A., McHenry C. R. (2015). Oldest pathology in a tetrapod bone illuminates the origin of terrestrial vertebrates. PloS One 10, e0125723. doi: 10.1371/journal.pone.0125723
Blaxter M. (2011). Nematodes: the worm and its relatives. PloS Biol. 9, e1001050. doi: 10.1371/journal.pbio.1001050
Boyce C. K., Cody G. D., Fesser M., Jacobsen C., Knoll A. H., Wirick S. (2002). Organic chemical differentiation within fossil plant cell walls detected with X-ray spectromicroscopy. Geology 30, 1039–1042. doi: 10.1130/0091-7613(2002)030<1039:OCDWFP>2.0.CO;2
Boyce C. K., HoJon C. L., Fogel M. L., Cody G. D., Hazen R. M., Knoll A. H., et al. (2007). Devonian landscape heterogeneity recorded by a giant fungus. Geology 35, 399–402. doi: 10.1130/G23384A.1
Boyce C. K., Abrecht M., Zhou D., Gilbert P. U. P. A. (2010). X-ray photoelectron emission spectromicroscopic analysis of arborescent lycopsid cell wall composition and Carboniferous coal ball preservation. Int. J. Coal Geol. 83, 146–153. doi: 10.1016/j.coal.2009.10.008
Boyce C. K., Ibarra D. E., Nelsen M. P., D’Antonio M. P. (2023). Nitrogen-based symbioses, phosphorus availability, and accounting for a modern world more productive than the Paleozoic. Geobiology 21, 86–101. doi: 10.1111/gbi.12519
Boyce C. K., Zwieniecki M. A. (2012). Leaf fossil record suggests limited influence of atmospheric CO2 on terrestrial productivity prior to angiosperm evolution. Proc. Natl. Acad. Sci. 109, 10403–10408. doi: 10.1073/pnas.1203769109
Brookfield M. E., Catlos E. J., Suarez S. E. (2021). Myriapod divergence times differ between molecular clock and fossil evidence: U/Pb zircon ages of the earliest fossil millipede-bearing sediments and their significance. Historical Biol. 33, 2014–2018. doi: 10.1080/08912963.2020.1762593
Carvalho M. R., Wilf P., Barrios H., Windsor D. M., Currano E. D., Labandeira C. C., et al. (2014). Insect leaf-chewing damage tracks herbivore richness in modern and ancient forests. PloS One 9, e94950. doi: 10.1371/journal.pone.0094950
Chin K., Tokaryk T. T., Erickson G. M., Calk L. C. (1998). A king-sized theropod coprolite. Nature 393, 680–682. doi: 10.1038/31461
Christensen H. B. (2014). Similar associations of tooth microwear and morphology indicate similar diet across marsupial and placental mammals. PloS One 9, e102789. doi: 10.1371/journal.pone.0102789
Cleveland C. C., Liptzin D. (2007). C: N: P stoichiometry in soil: is there a “Redfield ratio” for the microbial biomass? Biogeochemistry 85, 235–252. doi: 10.1007/s10533-007-9132-0
Coll M., Guershon M. (2002). Omnivory in terrestrial arthropods: mixing plant and prey diets. Annu. Rev. Entomol. 47, 267–297. doi: 10.1146/annurev.ento.47.091201.145209
Crocker R. L., Major J. (1955). Soil development in relation to vegetation and surface age at Glacier Bay, Alaska. J. Ecol. 43, 427–448. doi: 10.2307/2257005
Currano E. D., Wilf P., Wing S. L., Labandeira C. C., Lovelock E. C., Royer D. L. (2008). Sharply increased insect herbivory during the Paleocene–Eocene Thermal Maximum. Proc. Natl. Acad. Sci. U.S.A. 105, 1960–1964. doi: 10.1073/pnas.0708646105
Dunlop J. A., Garwood R. J. (2017). Terrestrial invertebrates in the Rhynie chert ecosystem. Philos. Trans. R. Soc. B: Biol. Sci. 373, 20160493. doi: 10.1098/rstb.2016.0493
Dunlop J. A., Selden P. A. (2013). Scorpion fragments from the silurian of powys, wales. Arachnology 16, 27–33. doi: 10.13156/arac.2013.16.1.27
Edwards D., Selden P. A., Richardson J. B., Axe L. (1995). Coprolites as evidence for plant-animal interaction in Siluro-Devonian terrestrial ecosystems. Nature 377, 329–331. doi: 10.1038/377329a0
Elser J. J., Fagan W. F., Denno R. F., Dobberfuhl D. R., Folarin A., Huberty A., et al. (2000). Nutritional constraints in terrestrial and freshwater food webs. Nature 408, 578–580. doi: 10.1038/35046058
Eltoum E., Berry R. (1985). Influence of garden symphylan (Symphyla: Scutigerellidae) root injury on physiological processes in snap beans. Environ. Entomol. 14, 408–412. doi: 10.1093/ee/14.4.408
Endlweber K., Ruess L., Scheu S. (2009). Collembola switch diet in presence of plant roots thereby functioning as herbivores. Soil Biol. Biochem. 41, 1151–1154. doi: 10.1016/j.soilbio.2009.02.022
Erséus C., Williams B. W., Horn K. M., Halanych K. M., Santos S. R., James S. W., et al. (2020). Phylogenomic analyses reveal a Palaeozoic radiation and support a freshwater origin for clitellate annelids. Zool. Scripta 49, 614–640. doi: 10.1111/zsc.12426
Fernández-Martínez M., Preece C., Corbera J., Cano O., Garcia-Porta J., Sardans J., et al. (2021). Bryophyte C: N: P stoichiometry, biogeochemical niches and elementome plasticity driven by environment and coexistence. Ecol. Lett. 24, 1375–1386. doi: 10.1111/ele.13752
Fich E. A., Segerson N. A., Rose J. K. (2016). The plant polyester cutin: biosynthesis, structure, and biological roles. Annu. Rev. Plant Biol. 67, 207–233. doi: 10.1146/annurev-arplant-043015-111929
Garwood R. J., Edgecombe G. D., Charbonnier S., Chabard D., SoJy D., Giribet G. (2016). Carboniferous Onychophora from Montceau-les-Mines, France, and onychophoran terrestrialization. Invertebr. Biol. 135, 179–190. doi: 10.1111/ivb.12130
Gorman M. A. (2013). The earliest record of insect herbivory presents a diversity of damage types (M.S. Thesis) (Geophysical Sciences, University of Chicago).
Gupta N. S., Briggs D. E.G., Collinson M. E., Evershed R. P., Michels R., Jack K. S., et al. (2007). Evidence for the in situ polymerisation of labile aliphatic organic compounds during the preservation of fossil leaves: Implications for organic matter preservation. Organ. Geochem. 38, 499–522. doi: 10.1016/j.orggeochem.2006.06.011
Habgood K. S., Hass H., Kerp H. (2003). Evidence for an early terrestrial food web: coprolites from the Early Devonian Rhynie chert. Earth Environ. Sci. Trans. R. Soc. Edinburgh 94, 371–389. doi: 10.1017/S0263593300000754
Harms D., Dunlop J. A. (2017). The fossil history of pseudoscorpions (Arachnida: Pseudoscorpiones). Mitteilungen aus dem Museum für Naturkunde in Berlin. Fossil Rec. 20, 215. doi: 10.5194/fr-20-215-2017
Hatcher P. G., Clifford D. J. (1997). The organic geochemistry of coal: from plant materials to coal. Organ. Geochem. 27, 251–274. doi: 10.1016/S0146-6380(97)00051-X
Hirakawa H. (2001). Coprophagy in leporids and other mammalian herbivores. Mammal Rev. 31, 61–80. doi: 10.1046/j.1365-2907.2001.00079.x
Howard R. J., Puttick M. N., Edgecombe G. D., Lozano-Fernandez J. (2020). Arachnid monophyly: Morphological, palaeontological and molecular support for a single terrestrialization within Chelicerata. Arthropod Struct. Dev. 59, 100997. doi: 10.1016/j.asd.2020.100997
Howard R. J., Giacomelli M., Lozano-Fernandez J., Edgecombe G. D., Fleming J. F., Kristensen R. M., et al. (2022). The Ediacaran origin of Ecdysozoa: integrating fossil and phylogenomic data. J. Geol. Soc. 179, jgs2021–107. doi: 10.1144/jgs2021-107
Huang D.-Y., Bechly G., Nel P., Engel M. S., Prokop J., Azar D., et al. (2016). New fossil insect order Permopsocida elucidates major radiation and evolution of suction feeding in hemimetabolous insects (Hexapoda: Acercaria). Sci. Rep. 6, 1–9. doi: 10.1038/srep23004
Hueber F. M. (2001). Rotted wood-alga-fungus: the history and life of Prototaxites Dawson 1859. Rev. Palaeobotany Palynol. 116, 123–158. doi: 10.1016/S0034-6667(01)00058-6
Iannuzzi R., Labandeira C. (2008). The oldest record of external foliage feeding and the expansion of insect folivory on land. Ann. Entomol. Soc. America 101, 79–94. doi: 10.1603/0013-8746(2008)101[79:TOROEF]2.0.CO;2
Jeram A. J., Selden P. A., Edwards D. (1990). Land animals in the Silurian: arachnids and myriapods from Shropshire, England. Science 250, 658–661. doi: 10.1126/science.250.4981.658
Kevan P. G., Chaloner W. G., Savile D. B. O. (1975). Interrelationships of early terrestrial arthropods and plants. Palaeontology 18, 391–417.
Khosla A., Chin K., Alimohammadin H., DuJa D. (2015). Ostracods, plant tissues, and other inclusions in coprolites from the Late Cretaceous Lameta Formation at Pisdura, India: Taphonomical and palaeoecological implications. Palaeogeogr. Palaeoclimatol. Palaeoecol. 418, 90–100. doi: 10.1016/j.palaeo.2014.11.003
Khramov A. V., Foraponova T., Węgierek P. (2023). The earliest pollen-loaded insects from the Lower Permian of Russia. Biol. Lett. 19, 20220523. doi: 10.1098/rsbl.2022.0523
Knoll A. H., Follows M. J. (2016). A bottom-up perspective on ecosystem change in Mesozoic oceans. Proc. R. Soc. B: Biol. Sci. 283, 20161755. doi: 10.1098/rspb.2016.1755
Krassilov V., Rasnitsyn A. P. (1996). Pollen in the guts of Permian insects: first evidence of pollinivory and its evolutionary significance. Lethaia 29, 369–372. doi: 10.1111/j.1502-3931.1996.tb01672.x
Krings M., Kellogg D. W., Kerp H., Taylor T. N. (2003). Trichomes of the seed fern Blanzyopteris praedentata: implications for plant–insect interactions in the Late Carboniferous. Bot. J. Linn. Soc. 141, 133–149. doi: 10.1046/j.1095-8339.2003.00135.x
Labandeira C. C. (1997). Insect mouthparts: ascertaining the paleobiology of insect feeding strategies. Annu. Rev. Ecol. System. 28, 153–193. doi: 10.1146/annurev.ecolsys.28.1.153
Labandeira C. C. (2000). The paleobiology of pollination and its precursors. Paleontol. Soc. Pap. 6, 233–270. doi: 10.1017/S1089332600000784
Labandeira C. (2007). The origin of herbivory on land: initial patterns of plant tissue consumption by arthropods. Insect Sci. 14, 259–275. doi: 10.1111/j.1744-7917.2007.00141.x-i1
Labandeira C. C., Kvaček J., Mostovski M. B. (2007a). Pollination drops, pollen, and insect pollination of Mesozoic gymnosperms. Taxon 56, 663–695. doi: 10.2307/25065852
Labandeira C. C., Wilf P., Johnson K. R., Marsh F. (2007b). Guide to insect (and other) damage types on compressed plant fossils. Version 3.0 (Washington, D. C.: Smithsonian Institution).
Labandeira C. C., Tremblay S. L., Bartowski K. E., VanAller Hernick L. (2014). Middle Devonian liverwort herbivory and antiherbivore defence. New Phytol. 202, 247–258. doi: 10.1111/nph.12643
Labandeira C. C., Yang Q., Sanfago-Blay J. A., HoJon C. L., Monteiro A., Wang Y.-J., et al. (2016). The evolutionary convergence of mid-Mesozoic lacewings and Cenozoic butterflies. Proc. R. Soc. B: Biol. Sci. 283, 20152893. doi: 10.1098/rspb.2015.2893
Labandeira C. C., Sepkoski J. J. Jr. (1993). Insect diversity in the fossil record. Science 261, 310–315. doi: 10.1126/science.11536548
Leng R., Nolan J. (1984). Nitrogen metabolism in the rumen. J. Dairy Sci. 67, 1072–1089. doi: 10.3168/jds.S0022-0302(84)81409-5
Lenton T. M., Dahl T. W., Daines S. J., Mills B. J., Ozaki K., Saltzman M. R., et al. (2016). Earliest land plants created modern levels of atmospheric oxygen. Proc. Natl. Acad. Sci. 113, 9704–9709. doi: 10.1073/pnas.1604787113
Li F.-S., Phyo P., Jacobowitz J., Hong M., Weng J.-K. (2019). The molecular structure of plant sporopollenin. Nat. Plants 5, 41–46. doi: 10.1038/s41477-018-0330-7
Magalhaes I. L., Azevedo G. H., Michalik P., Ramírez M. J. (2020). The fossil record of spiders revisited: implications for calibrating trees and evidence for a major faunal turnover since the Mesozoic. Biol. Rev. 95, 184–217. doi: 10.1111/brv.12559
Marchioro T., Rota Stabelli O., Rebecchi L., Guidetti R., Pisani D. (2012). “Dating tardigrade evolution and early terrestrialization events,” in 12th International Symposium on Tardigrada, Aguas e Parco biologico de Gaia, EEM/Facultade de Ciencias da Universidade. 80–80.
Martìnez-Delclòs X., Briggs D. E., Peñalver E. (2004). Taphonomy of insects in carbonates and amber. Palaeogeogr. Palaeoclimatol. Palaeoecol. 203, 19–64. doi: 10.1016/S0031-0182(03)00643-6
McCoy V. E., Gee C. T., Michalski J. M., Wings O. (2022). Oldest fossil evidence of latex sabotaging behavior by herbivorous insects. Rev. Palaeobotany Palynol. 300, 104631. doi: 10.1016/j.revpalbo.2022.104631
Mihlbachler M. C., Campbell D., Ayoub M., Chen C., Ghani I. (2016). Comparative dental microwear of ruminant and perissodactyl molars: Implications for paleodietary analysis of rare and extinct ungulate clades. Paleobiology 42, 98–116. doi: 10.1017/pab.2015.33
Milner A., Sequeira S. (1993). The temnospondyl amphibians from the Viséan of east Kirkton, West Lothian, Scotland. Earth Environ. Sci. Trans. R. Soc. Edinburgh 84, 331–361. doi: 10.1017/S0263593300006155
Misof B., Liu S., Meusemann K., Peters R. S., Donath A., Mayer C., et al. (2014). Phylogenomics resolves the timing and pattern of insect evolution. Science 346, 763–767. doi: 10.1126/science.1257570
Mitter C., Davis D. R., Cummings M. P. (2017). Phylogeny and evolution of lepidoptera. Annu. Rev. Entomol. 62, 265–283. doi: 10.1146/annurev-ento-031616-035125
Mittler T. (1958). Studies on the feeding and nutrition of Tuberolachnus salignus (Gmelin)(Homoptera, Aphididae) II. The nitrogen and sugar composition of ingested phloem sap and excreted honeydew. J. Exp. Biol. 35, 74–84. doi: 10.1242/jeb.35.1.74
Nelsen M. P., Boyce C. K. (2022). What to do with prototaxites? Int. J. Plant Sci. 183, 556–565. doi: 10.1086/720688
Neveu M., Poret-Peterson A. T., Anbar A. D., Elser J. J. (2016). Ordinary stoichiometry of extraordinary microorganisms. Geobiology 14, 33–53. doi: 10.1111/gbi.12153
O’Brien D. M., Fogel M. L., Boggs C. L. (2002). Renewable and nonrenewable resources: amino acid turnover and allocation to reproduction in Lepidoptera. Proc. Natl. Acad. Sci. 99, 4413–4418. doi: 10.1073/pnas.072346699
O’Connor J. K. (2019). The trophic habits of early birds. Palaeogeogr. palaeoclimatol. Palaeoecol. 513, 178–195. doi: 10.1016/j.palaeo.2018.03.006
Peñalver E., Labandeira C. C., Barrón E., Delclòs X., Nel P., Nel A., et al. (2012). Thrips pollination of Mesozoic gymnosperms. Proc. Natl. Acad. Sci. 109, 8623–8628. doi: 10.1073/pnas.1120499109
Peñalver E., Arillo A., Perez-de la Fuente R., Riccio M. L., Delclos X., Barron E., et al. (2015). Long-proboscid flies as pollinators of Cretaceous gymnosperms. Curr. Biol. 25, 1917–1923. doi: 10.1016/j.cub.2015.05.062
Poinar G. Jr., Kerp H., Hass H. (2008). Palaeonema phyticum gen. n., sp. n.(Nematoda: Palaeonematidae fam. n.), a Devonian nematode associated with early land plants. Nematology 10, 9–14. doi: 10.1163/156854108783360159
Potapov A. M., Beaulieu F., Birkhofer K., Bluhm S. L., Degtyarev M. I., DeveJer M., et al. (2022). Feeding habits and multifunctional classification of soil-associated consumers from protists to vertebrates. Biol. Rev. 97, 1057–1117. doi: 10.1111/brv.12832
Prasad V., Strömberg C. A. E., Alimohammadian H., Sahni A. (2005). Dinosaur coprolites and the early evolution of grasses and grazers. Science 310, 1177–1180. doi: 10.1126/science.1118806
Redfield A. C. (1958). The biological control of chemical factors in the environment. Am. Scientist 46, 205–221.
Regier J. C., MiJer C., Zwick A., Bazinet A. L., Cummings M. P., Kawahara A. Y., et al. (2013). A large-scale, higher-level, molecular phylogenetic study of the insect order Lepidoptera (moths and butterflies). PloS One 8, (3). doi: 10.1371/journal.pone.0058568
Reich P. B., Hungate B. A., Luo Y. (2006). Carbon-nitrogen interactions in terrestrial ecosystems in response to rising atmospheric carbon dioxide. Annu. Rev. Ecol. Evol. Syst. 37, 611–636. doi: 10.1146/annurev.ecolsys.37.091305.110039
Reisz R. R., Fröbisch J. (2014). The oldest caseid synapsid from the Late Pennsylvanian of Kansas, and the evolution of herbivory in terrestrial vertebrates. PloS One 9, e94518. doi: 10.1371/journal.pone.0094518
Ren X., Guo R., Akami M., Niu C. (2022). Nitrogen acquisition strategies mediated by insect symbionts: a review of their mechanisms, methodologies, and case studies. Insects 13, 84. doi: 10.3390/insects13010084
Rota-Stabelli O., Daley A. C., Pisani D. (2013). Molecular timetrees reveal a Cambrian colonization of land and a new scenario for ecdysozoan evolution. Curr. Biol. 23, 392–398. doi: 10.1016/j.cub.2013.01.026
Sardans J., Peñuelas J. (2012). The role of plants in the effects of global change on nutrient availability and stoichiometry in the plant-soil system. Plant Physiol. 160, 1741–1761. doi: 10.1104/pp.112.208785
Schachat S. R., Labandeira C. C., Saltzman M. R., Cramer B. D., Payne J. L., Boyce C. K. (2018). Phanerozoic pO2 and the early evolution of terrestrial animals. Proc. R. Soc. B: Biol. Sci. 285, 20172631. doi: 10.1098/rspb.2017.2631
Schachat S. R., Payne J. L., Boyce C. K., Labandeira C. C. (2022). Generating and testing hypotheses about the fossil record of insect herbivory with a theoretical ecospace. Rev. Palaeobotany Palynol. 297, 104564. doi: 10.1016/j.revpalbo.2021.104564
Schachat S. R., Payne J. L., Boyce C. K. (2023). Linking host plants to damage types in the fossil record of insect herbivory. Paleobiology 49, 232–258. doi: 10.1017/pab.2022.35
Schill R. O., Jönsson K. I., Pfannkuchen M., Brümmer F. (2011). Food of tardigrades: a case study to understand food choice, intake and digestion. J. Zool. System. Evolutionary Res. 49, 66–70. doi: 10.1111/j.1439-0469.2010.00601.x
Shear W. A., Edgecombe G. D. (2010). The geological record and phylogeny of the Myriapoda. Arthropod Struct. Dev. 39, 174–190. doi: 10.1016/j.asd.2009.11.002
Shear W. A., Bonamo P. M., Grierson J. D., Rolfe W. I., Smith E. L., Norton R. A. (1984). Early land animals in North America: evidence from Devonian age arthropods from Gilboa, New York. Science 224, 492–494. doi: 10.1126/science.224.4648.492
Smithson T., Carroll R., Panchen A., Andrews S. (1993). Westlothiana lizziae from the Viséan of East Kirkton, West Lothian, Scotland, and the amniote stem. Earth Environ. Sci. Trans. R. Soc. Edinburgh 84, 383–412. doi: 10.1017/S0263593300006192
Smyth C., Titus B., Trofymow J., Moore T., Preston C., Prescott C., et al. (2016). Patterns of carbon, nitrogen and phosphorus dynamics in decomposing wood blocks in Canadian forests. Plant Soil 409, 459–477. doi: 10.1007/s11104-016-2972-4
Sterner R. W., Elser J. J. (2003). Ecological stoichiometry: the biology of elements from molecules to the biosphere (Princeton: Princeton University Press).
Strömberg C. A. E. (2011). Evolution of grasses and grassland ecosystems. Annu. Rev. Earth Planetary Sci. 39, 517–544. doi: 10.1146/annurev-earth-040809-152402
Sues H.-D., Reisz R. R. (1998). Origins and early evolution of herbivory in tetrapods. Trends Ecol. Evol. 13, 141–145. doi: 10.1016/S0169-5347(97)01257-3
Van Bergen P. F., Collinson M. E., Briggs D. E.G., De Leeuw J. W., Scott A. C., Evershed R. P., et al. (1995). Resistant biomacromolecules in the fossil record. Acta Botanica Neerlandica 44, 319–342. doi: 10.1111/j.1438-8677.1995.tb00791.x
Wappler T., Labandeira C. C., Engel M. S., ZeJer R., Grímsson F. (2015). Specialized and generalized pollen-collection strategies in an ancient bee lineage. Curr. Biol. 25, 3092–3098. doi: 10.1016/j.cub.2015.09.021
Watanabe H., Tokuda G. (2001). Animal cellulases. Cell. Mol. Life Sci. CMLS 58, 1167–1178. doi: 10.1007/PL00000931
Watanabe H., Tokuda G. (2010). Cellulolytic systems in insects. Annu. Rev. Entomol. 55, 609–632. doi: 10.1146/annurev-ento-112408-085319
Wilf P., Labandeira C. C., Kress W. J., Staines C. L., Windsor D. M., Allen A. L., et al. (2000). Timing the radiations of leaf beetles: Hispines on gingers from the latest Cretaceous to recent. Science 289, 291–294. doi: 10.1126/science.289.5477.291
Wilf P., Labandeira C. C., Johnson K. R., Ellis B. (2006). Decoupled plant and insect diversity after the end-Cretaceous extinction. Science 313, 1112–1115. doi: 10.1126/science.1129569
Yang Y., Luo Y. (2011). Carbon: nitrogen stoichiometry in forest ecosystems during stand development. Global Ecol. Biogeography 20, 354–361. doi: 10.1111/j.1466-8238.2010.00602.x
Zhang J., Elser J. J. (2017). Carbon: nitrogen: phosphorus stoichiometry in fungi: a meta-analysis. Front. Microbiol. 8, 1281. doi: 10.3389/fmicb.2017.01281
Keywords: herbivory, insect, microfauna, nitrogen, Paleozoic, soil fauna, stoichiometry, vertebrate
Citation: Boyce CK (2023) Evolution of terrestrial herbivory: nutrient stoichiometry, body size, and dietary diversity. Front. Ecol. Evol. 11:1304831. doi: 10.3389/fevo.2023.1304831
Received: 30 September 2023; Accepted: 23 October 2023;
Published: 14 November 2023.
Edited by:
Juan Manuel Robledo, Centro de Ecología Aplicada del Litoral (CONICET), ArgentinaReviewed by:
Barbara Cariglino, National Scientific and Technical Research Council (CONICET), ArgentinaCopyright © 2023 Boyce. This is an open-access article distributed under the terms of the Creative Commons Attribution License (CC BY). The use, distribution or reproduction in other forums is permitted, provided the original author(s) and the copyright owner(s) are credited and that the original publication in this journal is cited, in accordance with accepted academic practice. No use, distribution or reproduction is permitted which does not comply with these terms.
*Correspondence: C. Kevin Boyce, ckboyce@stanford.edu