Decline of a North American rocky intertidal foundation species linked to extreme dry, downslope Santa Ana winds
- 1Bren School of Environmental Science and Management, University of California, Santa Barbara, Santa Barbara, CA, United States
- 2Channel Islands National Park, U.S. National Park Service, Ventura, CA, United States
- 3Marine Science Institute, University of California, Santa Barbara, Santa Barbara, CA, United States
- 4Ecology and Evolutionary Biology Department, University of California, Santa Cruz, Santa Cruz, CA, United States
- 5Biological Sciences, California State Polytechnic University, Pomona, CA, United States
Foundation species are essential to ecosystem function, but their role as habitat providers is predicated on their spatial dominance. Worldwide, kelps, seagrasses, corals, and other marine foundation species have declined. This is true also for rockweeds, the canopy-forming analog of subtidal kelp forests in temperate rocky intertidal ecosystems. On the west coast of North America, dense beds of the rockweed Silvetia compressa occur across large biogeographic regions, benefitting numerous species by ameliorating physical stress caused by sun exposure, desiccation, heat, and wave disturbance. Like many rockweed species, Silvetia is long-lived, slow-growing, and short-dispersing – characteristics that reduce its resilience to disturbance. Using a generalized additive mixed-effects model with explicit spatial effects, we analyzed canopy cover data from 30 sites spanning 18 years, and we tested the hypothesis that Silvetia population trends are tightly linked to atmospheric climate conditions, particularly Santa Ana wind events (SAWs): strong, hot, and dry downslope winds that originate inland and move offshore. We found that the rockweed had declined markedly, particularly at sites south of the major biogeographic break, Point Conception (PC), including the California Channel Islands and southern California mainland, and a highly significant negative effect of dewpoint depression, a measure of moisture content in the atmosphere, on Silvetia cover across all three regions in this study. Our results suggest that any increases in the frequency or intensity of SAWs are likely to lead to large declines and possible extirpation of Silvetia, as well as the important ecological services the species provides.
1 Introduction
Foundation species define much of the structure of the community they occupy by modulating fundamental ecosystem processes and creating locally stable conditions for other species (Dayton, 1985; Ellison et al., 2005). Examples include mangroves (Duke et al., 2007), corals (Hughes et al., 2003), seagrasses (Short et al., 2006), kelps (Steneck et al., 2002), oysters (Lenihan et al., 2001), cordgrass (Zedler et al., 2001), and many tree species including redwood, hemlock, and birch (Ellison et al., 2005). These species share commonness and high local abundances, hence the tendency to name ecosystems after them – seagrass beds, kelp forests, oyster beds, and hemlock forests. Maintaining their foundational role in ecosystems, and the resulting effects on biodiversity, community composition, and ecosystem function, requires high local abundances, not simply species survival. However, many foundation species are declining due to coastal development (Zedler et al., 2001; Duke et al., 2007), pollution (Duke et al., 2007), invasive species (Steneck et al., 2002), introduced pathogens (Ellison et al., 2005), over-harvesting (Jackson et al., 2001; Steneck et al., 2002; Ellison et al., 2005), and climate change (Sagarin et al., 1999; Hughes et al., 2003), likely resulting in cascading impacts on associated communities (Sarà et al., 2021; Smale et al., 2022; Wernberg et al., 2023 and references therein).
In temperate rocky intertidal ecosystems, perennial fucoid macroalgae, commonly referred to as rockweeds, are often important foundation species (Chapman, 1995; Schiel and Foster, 2006). At low tide, rockweed canopies protect the substratum and communities beneath the canopy from sun exposure, desiccation, and heat stress (Bertness et al., 1999; Sapper and Murray, 2003; Råberg and Kautsky, 2007; Marzinelli et al., 2014) and thereby enhance biodiversity (Råberg and Kautsky, 2007; Marzinelli et al., 2014). Via this protection, as well as hydrodynamic effects, rockweed canopies also facilitate the recruitment of numerous intertidal species, including the rockweeds themselves (Bertness et al., 1999; Viejo et al., 1999), a positive feedback loop that likely contributes to their long-term population stability (Bertness et al., 1999). In addition to these ecosystem engineering effects, rockweeds are highly productive (Golléty et al., 2008; Tait and Schiel, 2010; Tait et al., 2014; Bordeyne et al., 2015), providing an important source of food for intertidal herbivores (Moore, 1977; Lubchenco, 1983; Steinberg, 1985; Bertness and Leonard, 1997; Jenkins et al., 2004; Hawkins et al., 2008) and detrital consumers (Bishop et al., 2010; Golléty et al., 2010; Renaud et al., 2015), and contributing to nutrient cycling (Schmidt et al., 2011).
Silvetia compressa (Agardh, 1824) (Phaeophyceae, hereafter Silvetia) is the dominant rockweed species in the northeast Pacific from Monterey County, California to Punta Baja, Baja California, Mexico (Abbott and Hollenberg, 1992; Silva et al., 2004; Skamarock and Klemp, 2008). Silvetia can live at least eight years (Gunnill, 1980) and is slow to recover from population declines due to the short-range dispersal of its gametes (Hays, 2006). Attaining frond lengths of 90 cm with up to 20 orders of branching (Silva et al., 2004), Silvetia can form large and dense beds (Figure 1) that harbor a diverse understory community. For example, Sapper and Murray (2003) documented 47 species of algae, 20 sessile and 44 mobile invertebrate species under the canopy formed by Silvetia at a rocky intertidal site in southern California.
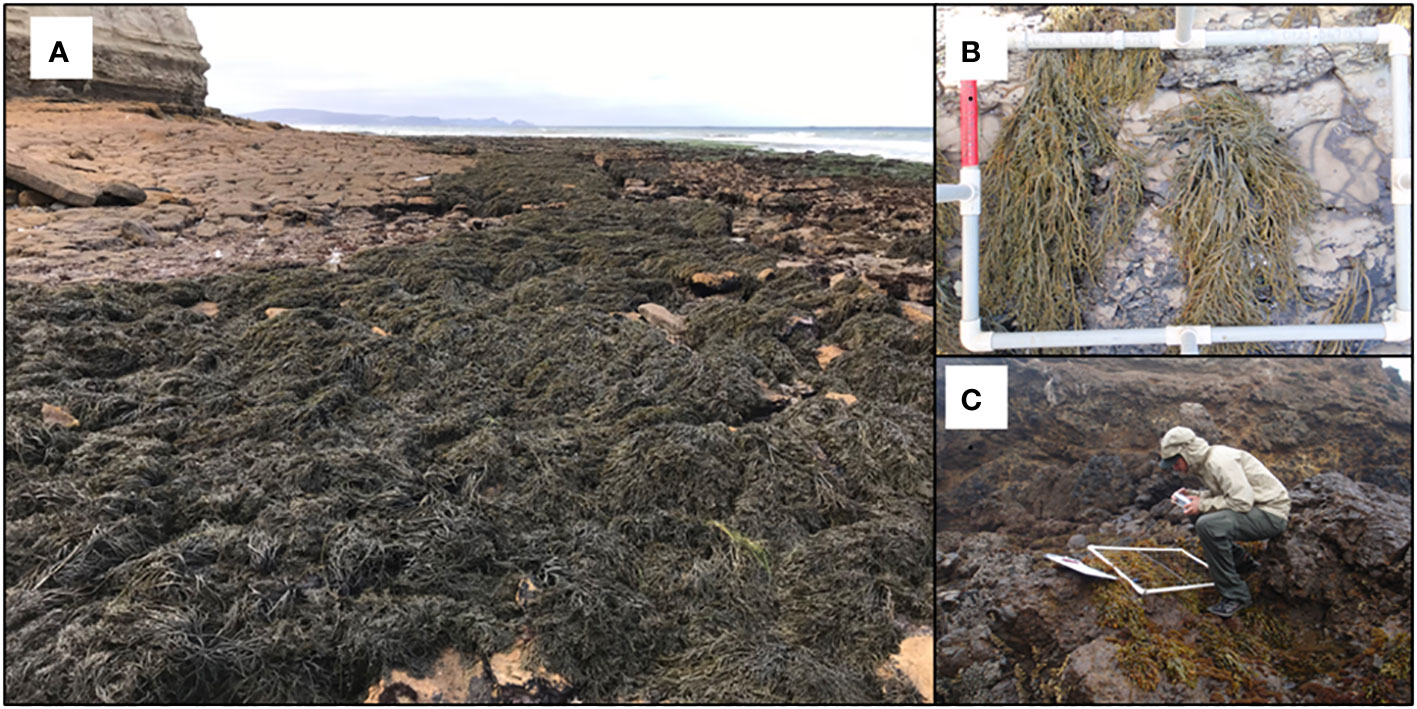
Figure 1 Rockweed, Silvetia compressa, forming large beds in the middle intertidal zone on the northwest side of Santa Rosa Island (A); fixed plot established to document the percent cover of the rockweed, Silvetia compressa (B) (photos, S. Whitaker); lead author S. Whitaker scoring a fixed plot for rockweed (C) (photo, K. Chan).
Upper and middle intertidal rockweeds, including Silvetia, are regularly subjected to prolonged periods of aerial exposure (i.e., emersion) during low tides. Rapidly fluctuating atmospheric variables, such as temperature, irradiance, wind speed, and relative humidity, impose increasingly greater stress on intertidal biota as a function of shore height (e.g., Hawkins and Hartnoll, 1985). Physiological stress generally results in increased rates of mortality (Graham et al., 2000) and reduced physiological performance (Schonbeck and Norton, 1980), and individuals living higher on the shore often exhibit morphological differences in response, such as thickened and stunted body forms (Sideman and Mathieson, 1985; Davison and Pearson, 1996). As a result, stress from emersion, and particularly desiccation, is considered among the most important drivers of species performance and abundance in the rocky intertidal zone (Brinkhuis et al., 1976; Brawley and Johnson, 1993; Davison and Pearson, 1996; Stengel and Dring, 1998; Helmuth and Hofmann, 2001 and references therein).
The coast from central California to northern Baja California including southern California and the Channel Islands in the Southern California Bight is periodically exposed to strong, dry, and often warm downslope winds, commonly referred to as Santa Ana winds (SAWs) (Abatzoglou et al., 2021; Guirguis et al., 2023). These harsh winds result from sharp gradients between high-pressure systems across the interior of the western United States and low pressure at the coast. As the air masses move from the dry Great Basin to the west over the coastal mountain ranges, they are compressed down the mountains and through canyons where they accelerate, heat, and dry in the process termed downsloping. SAWs can reach sustained speeds of 7-13 m s-1 (and gusts of >25 m s-1) and are characterized by low humidity (<15%) and warm air (>21°C) (Rolinski et al., 2019). SAWs can occur any time of the year, but they peak in late fall through early spring.
Recent modeling studies have revealed marked interannual variation in the frequency, intensity, and spatial coverage of SAWs (Jones et al., 2010; Abatzoglou et al., 2013; Guzman‐Morales et al., 2016; Dye et al., 2020), and these temporal trends have been linked to variation in environmental indices, including the El Niño-Southern Oscillation (Raphael, 2003), Pacific Decadal Oscillation, and the Atlantic Multi-decadal Oscillation (Li et al., 2016). SAWs are well studied due to their impact on vegetation and catastrophic wildfire threats (e.g., Moritz et al., 2010; Dye et al., 2020), but research on their impacts on marine life and oceanographic patterns is scarce. Intertidal organisms in southern California are severely stressed in late fall and winter when SAWs frequently coincide with extreme low tides (Seapy and Hoppe, 1973; Gunnill, 1980; Littler, 1980), and die-backs of Silvetia and other species have been attributed to prolonged aerial exposure (Seapy and Littler, 1982).
According to the Santa Ana Wildfire Index, a model derived from the climatological data used in this study to generate time series representing the trend for SAWs from 1981-2016, the annual frequency gradually increased beginning in 2000 (Li et al., 2016; see Figure 17 in Rolinski et al., 2019). By 2006, the mean number of SAW days had increased 54% from 46 days per year (1981-2005) to 71; a trend that persisted through the remainder of the climatology (Rolinski et al., 2019). This trend appears inversely proportionate to changes in Silvetia cover observed at numerous long-term monitoring study sites in the region affected by SAWs, with the most precipitous losses in cover occurring after 2005.
Here, we test the hypothesis that SAW events are driving declines in Silvetia populations in southern California. To perform our test, we used climatological time series data along with long-term monitoring data for Silvetia measured throughout much of its geographical range, extending across ca. 900 km of shoreline from Los Angeles, California to near the California/Mexico border, including several of the offshore Channel Islands in southern California.
2 Materials and methods
2.1 Study sites
Thirty long-term monitoring sites (Figure 2; Supplementary Table S1) were established by the Multi-Agency Rocky Intertidal Network (MARINe; pacificrockyintertidal.org), a consortium of government agencies, academic institutions, and nonprofit groups, from 1981 to 1999 across Silvetia’s range. Sites were established on bedrock benches with Silvetia beds. Refer to Engle et al. (2022) for detailed descriptions of methods.
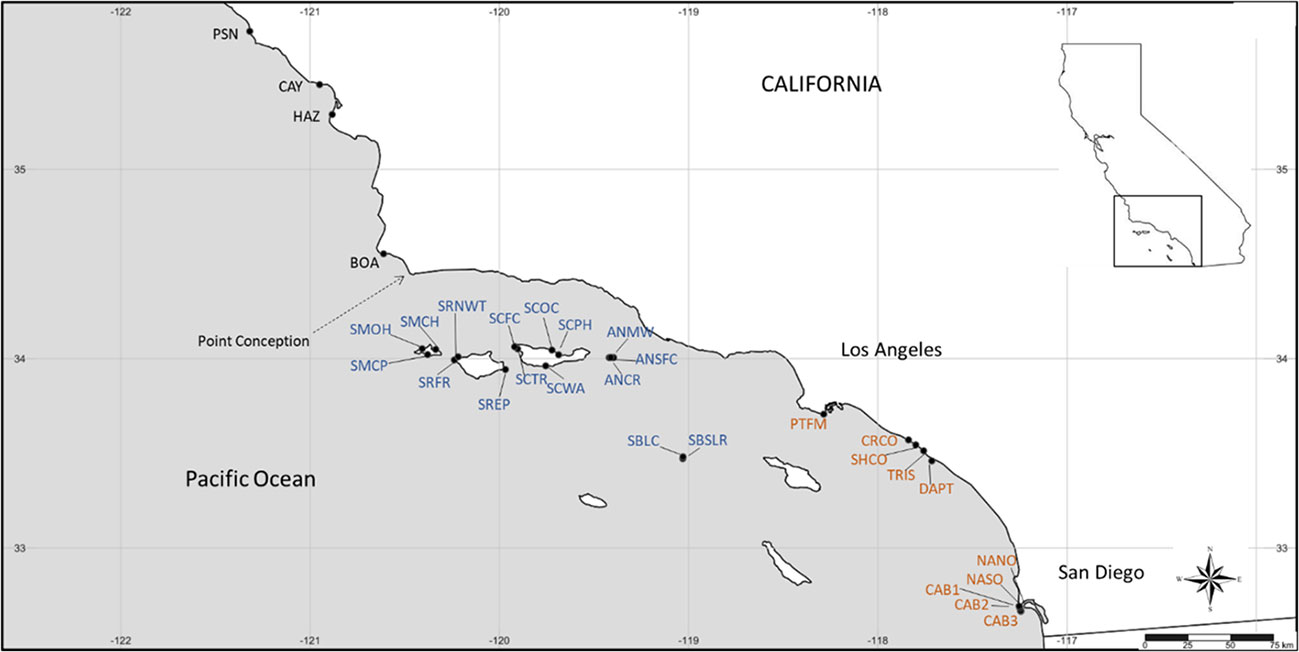
Figure 2 Map of long-term monitoring sites for Silvetia compressa used in this study. See Supplementary Table S1 for full site names and positions. Site abbreviations are color-coded by region (black = central California, blue = Channel Islands, orange = southern California).
Sites were grouped consistent with biogeographic regions described in Blanchette et al. (2008): central California (CEN, n = 4), defined as sites north of Point Conception (PC), a major biogeographic barrier separating the southern California sites (SOU, n = 10). Although located in the Southern California Bight, the offshore Channel Islands are exposed to a latitudinal gradient in environmental and oceanographic conditions that differs from the mainland (Dailey et al., 1993; Harms and Winant, 1998; Kapsenberg and Hofmann, 2016). Therefore, we grouped the Channel Islands (CHA, n = 16) sites separately from the southern California mainland sites. The sites were primarily limited to the northern Channel Islands which are subjected to colder water temperatures than the southern Channel Islands.
2.2 Survey methods
At each of the sites, five fixed rockweed plots (50 x 75 cm) were established mostly in the mid-1980s to 1990s and were originally chosen haphazardly at each site in areas of high canopy cover of Silvetia (Engle et al., 2022) (Figure 1). Stainless steel hex bolts were installed in three corners of each rectangular plot so they could be relocated. Percent cover of Silvetia, as well as other species or bare substrate when Silvetia was absent, was scored in each plot using a point contact method with a grid of 100 points, either in the field or using a digital photo of the plot (Engle et al., 2022). Until 2015, fixed plots were photographed and sampled biannually in the spring (March–May) and fall (October – January) during daytime low tides. Beginning in 2015, plots were sampled annually to reduce survey effort since seasonal differences in Silvetia cover were not significant (Raimondi et al., 2018), and most annual monitoring was conducted during the fall period. The long-term monitoring, fixed-plot approach used by MARINe was established to support a reasonable sampling effort while providing effective statistical power to detect changes over space and time. To maximize spatial and temporal coverage, we included all data on Silvetia cover from 2002-2020. The initial year was chosen based on the period when protocols and the resolution of taxonomic identifications were standardized across monitoring sites.
2.3 Climatology
For each Silvetia monitoring site, daily mean and max statistics (3-km horizontal resolution) for dewpoint depression (Dd) (°C) and wind velocity (Ws) (km h-1) were generated using a numerical weather prediction and atmospheric simulation system, the Weather Research and Forecasting (WRF) model (Rolinski et al., 2016). Daily values for Dd and Ws for each site were averaged between sampling events to provide a synopsis of the environmental conditions prior to measuring the percent cover of Silvetia. Dewpoint depression, the difference between air temperature and dewpoint temperature, together with near-surface wind gust time series, represents the drying process characteristic of synoptically driven offshore Santa Ana winds. Refer to Rolinski et al. (2016) and Skamarock et al. (2008) for detailed descriptions of how the atmospheric data used in this study were generated.
2.4 Statistical analysis
Data exploration of SAW patterns was carried out following the protocol described by Zuur et al. (2010). Dewpoint depression (Dd) and Ws climatological data were visually inspected using QQ plots, histograms, and a pair plot. Collinearity between Dd and Ws was assessed using scatterplots. Data transformations were deemed unnecessary, since the time series appeared approximately normally distributed, and various transformations including square root, cubic root, and logarithmic calculations did not significantly improve the linearity of the time series (Akaike, 1974). Data examination revealed nonlinear temporal and covariate effects.
To test for relationships between Silvetia cover and SAW time series data, trends in Silvetia populations were modeled as a function of the covariates using generalized additive mixed-effects models (GAMM) via restricted maximum likelihood (REML) in the mgcv package (Wood, 2011) using R Ver. 4.2.2 (R Core Team, 2022). Fixed covariates included Season_number (ordered integer with 36 levels), Region [categorical with three levels, central California (CEN), southern California (SOU), and the Channel Islands (CHA)], and a summary statistic (mean or maximum) for SAW time series data (Dd and Ws). Site (30 levels) was used as a random intercept to incorporate dependency among observations from the same site. A smoother for the Site spatial coordinates (Xkm, Ykm) was included to account for spatial dependency among sites.
We used interactions to test for regional differences in SAW time series data (e.g., meanDd Region) and time (i.e., Season_number Region) resulting in four full models: 1) interactions of Region with maxW2, maxDd, and Season_number, 2) interactions of Region with meanWs, meanDd, and Season_number, 3) interactions of Region with maxWs, meanDd, and Season_number, and 4) interactions of Region with meanWs, maxDd, and Season_number. We compared multiple reduced models based on the full models to test whether to include the interactions and both covariates (Ws and Dd) (Supplementary Table S2). The Akaike Information Criterion (AIC) and Bayesian Information Criterion (BIC) were used to identify the preferred model (i.e., lowest AIC and BIC) (Akaike, 1974; Schwarz, 1978). A beta distribution with a logistic link was used to ensure that the fitted values ranged from 0 to 1 for the response variable, mean percent cover of Silvetia [Equation (1)]. To account for the extremes 0 and 1, response variable data were transformed using (y · (n − 1) + 0.5)/n where n is the sample size (Smithson and Verkuilen, 2006).
Using GAMM, we analyzed the following equation:
where Coverij is the jth observation in site i, and i = 1, …, 30, is an unknown parameter controlling the variance, and Siteiis the random intercept, which is assumed to be normally distributed with mean 0 and variance .
To account for temporal dependency observed in the time-series data, we incorporated an autoregressive component [AR(1) correlation structure] into the model in which the random intercept w_it at time t and location i is similar to w_i, t-1 at time t-1 at location i. The AR(1) generates a latent variable that is spatially correlated, slowly changes over time, and captures any spatial and temporal patterns that are not modeled by the covariates. This latent variable ensures that the model residuals are independent and imposes a dependency structure on the response variable, Silvetia cover.
Underlying model assumptions including independence and absence of residual patterns were verified by plotting residuals versus fitted values and each covariate in the model. Temporal autocorrelation was assessed via partial autocorrelation function (PACF) plots of the model residuals. We then simulated 10,000 datasets from the preferred GAMM and calculated a frequency table for each simulated dataset. An average frequency table was generated from the simulated data and compared with the frequency table of the observed data.
Nonlinear trends and linear fits for Silvetia cover were generated at the region and site levels along with the regional relationship between Silvetia cover and maxDd using JMP Ver. 14.2.0 (SAS Institute Inc., 2018).
3 Results
3.1 Trends
Cover of the rockweed Silvetia compressa was highly variable in space and time across California. At the regional level, central California (CEN) Silvetia cover was relatively stable over time with slight declines until around 2009 and after 2015 (Figure 3). Channel Islands (CHA) Silvetia cover declined precipitously until around 2012 followed by a period of stabilization. Similarly, southern California (SOU) Silvetia cover followed a relatively steep negative trajectory but failed to recover.
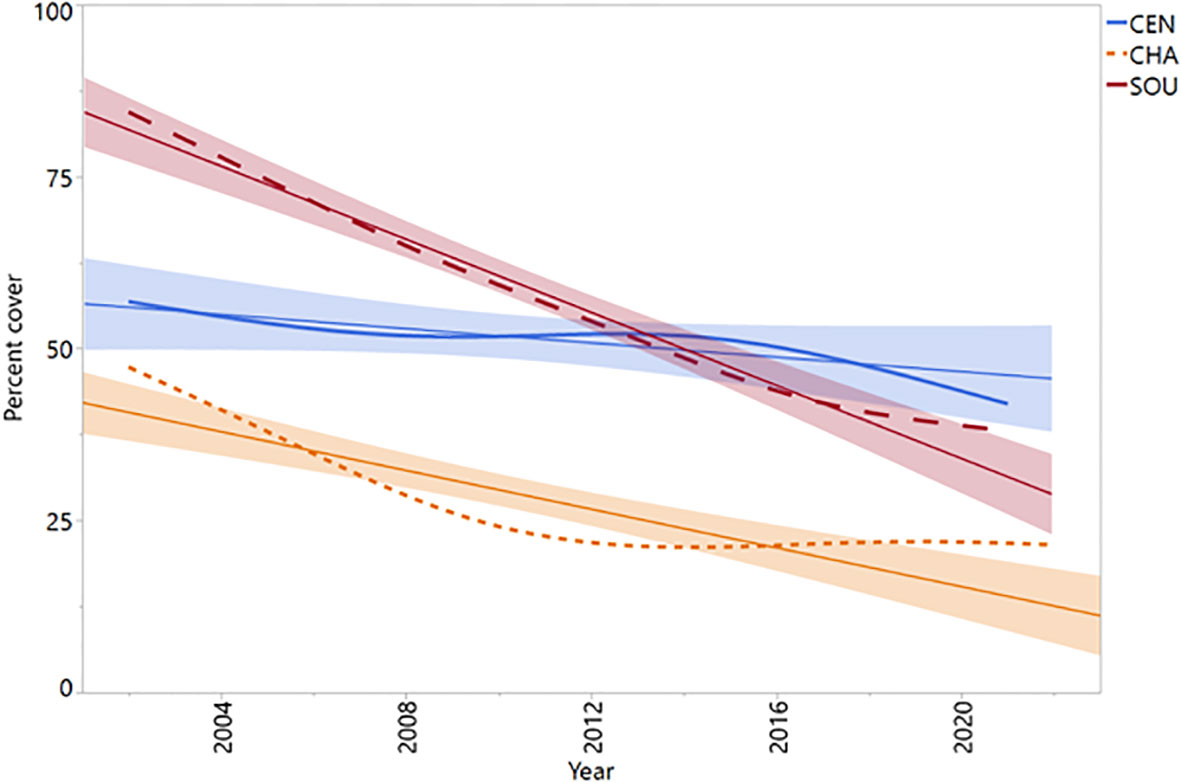
Figure 3 Mean annual percent cover data for Silvetia by region. Shade lines represent approximate linear fit. CEN, Central California region (P = 0.0982); CHA, Channel Islands region (P < 0.0001); SOU, Southern California region (P < 0.0001).
Most sites (22 of 30) exhibited significant declines in rockweed cover over the study period. Declines were most prevalent south of Point Conception on the Channel Islands and the southern California mainland (Figure 4). The SOU sites exhibited high incidence (90% of sites) of decline, and nearly all declines exceeded 50% cover followed by minimal or no recovery. Within the CHA region, Silvetia cover decreased at all but five sites (ANSFC, SBLC, SBSLR, SRFR, SRNWT) with similar trajectories. CEN rockweed populations appeared most resilient, with one site (CAY) increasing in cover significantly during the study period and two sites (BOA, PSN) declining. The remaining sites across the three regions had relatively stable populations of rockweed over time (CEN 25%, CHA 25%, SOU 10%).
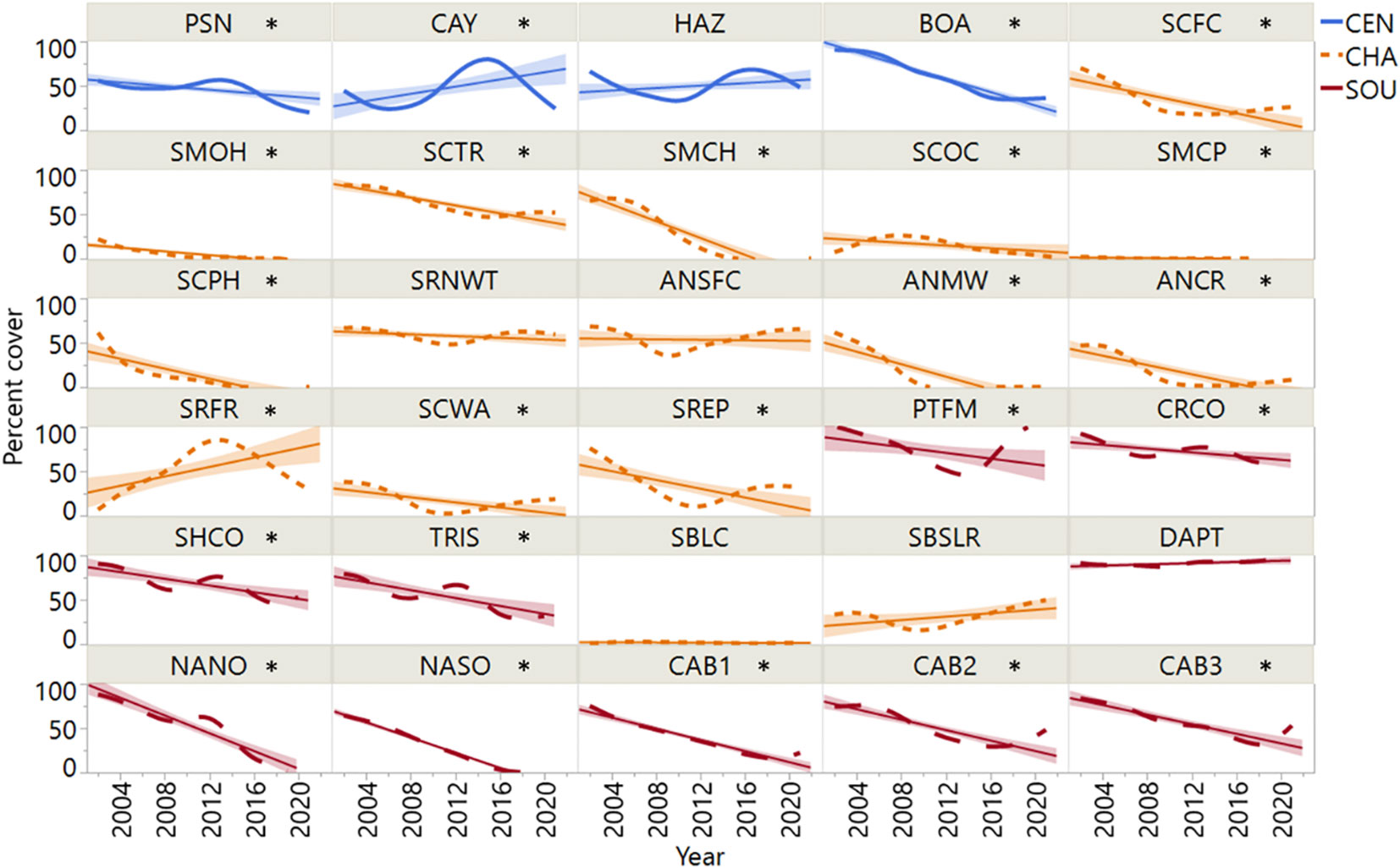
Figure 4 Mean annual percent cover data for Silvetia at each of the thirty sites arranged by latitude. Site codes are defined in Table 1. Asterisk denotes significance of the linear regression slope (P = <0.05). CEN, Central California region; CHA, Channel Islands region; SOU, Southern California region.
Most Silvetia declines occurred steadily after 2005 until approximately 2015 when Silvetia cover either slightly increased or continued declining for the remainder of the study period (Figures 3, 4). Sites that had stable or increasing Silvetia populations generally peaked in cover near the beginning of the study period and/or after 2015. Sites that precipitously declined more than 30% failed to recover.
3.2 Model results
The model (M17) with the lowest AIC and BIC included a smoother for time (i.e., Season_number) and an interaction with Region and a smoother for the covariate maxDd (Table 1; Supplementary Table S2). Based on , M9, M11 and M13 were considered comparable to M17 since AIC values for each were< 5 points apart. However, M9, M11 and M13 each were more complex than M17. Therefore, we identified M17 as the preferred model. Based on , only one other model (M18) was comparable to the preferred model (M17).
Residuals from M17 met regression assumptions including normality and homogeneity of variance, and no clear patterns were seen when the residuals were plotted against covariates included and not included in the model. The AR(1) correlation structure markedly reduced the autocorrelation of the model residuals for M17 (Supplementary Figure S1). Residuals for the spatial coordinates represented variation in Silvetia cover not captured by covariates modeled with the GAMM (Supplementary Figure S2). Slightly higher residuals were observed in the middle latitudes corresponding with the CHA region. Lower residuals occurred in the southern latitudes near the lower portion of the SOU region. Low variability in the residuals was observed in the remaining study areas.
3.3 Dewpoint depression
Maximum dewpoint depression (maxDd) was negatively correlated with Silvetia cover in all three regions (Table 1; Figures 5, 6). The relationship between Silvetia cover and maxDd appeared nonlinear for the three regions (Figures 5, 6). Model selection indicated that an interaction between maxDd and Region was not necessary since AIC for the full models was not substantially lower compared with the models excluding the interaction.
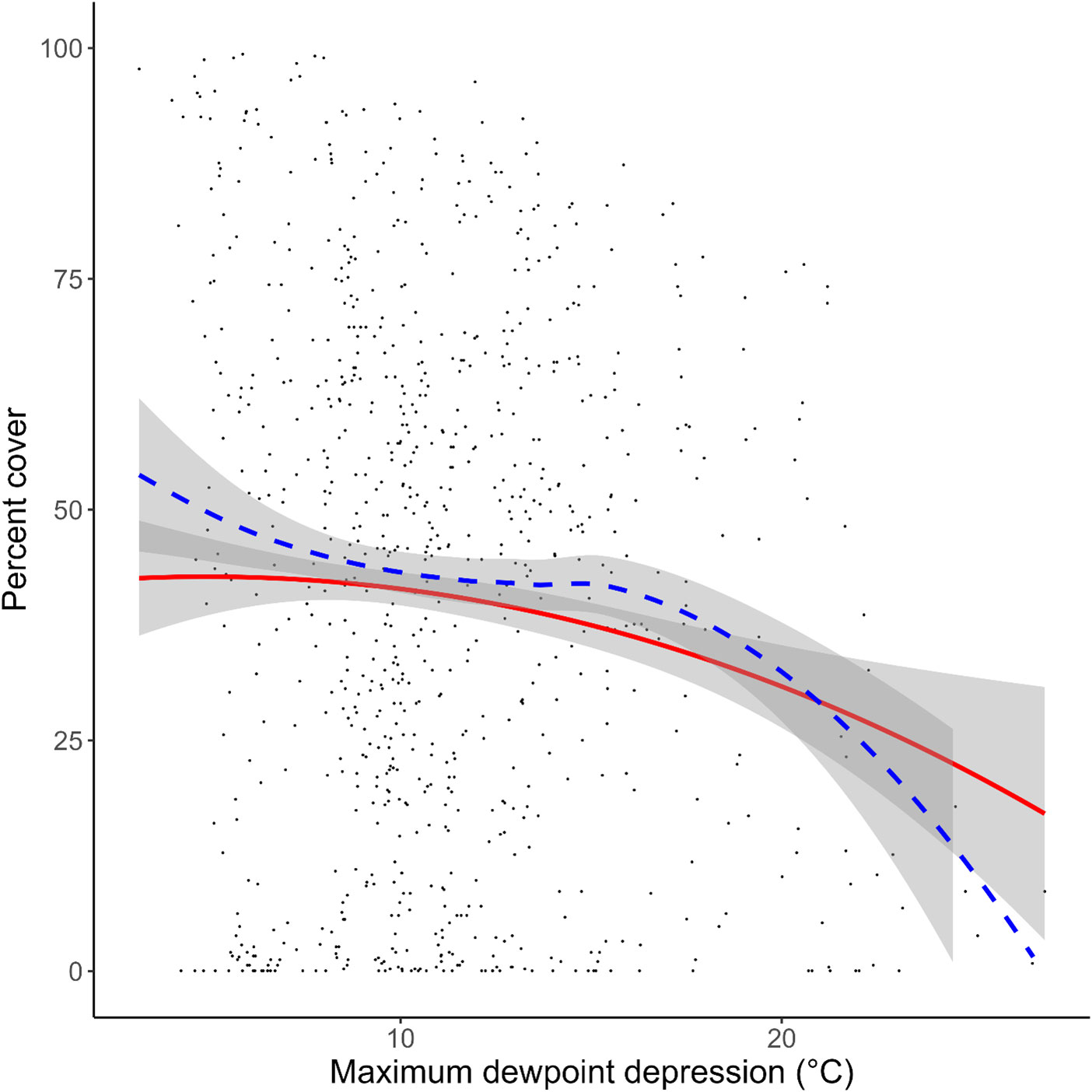
Figure 5 Effect of max dewpoint depression (maxDd) on Silvetia cover at an average site and year plotted on the original scale. The blue dashed line represents a smoothed fit using the actual data. The red line represents a smoothed fit using the model (predicted data). The grey areas indicate the 95% confidence interval for the actual data and the modeled fit. Points denote measured data.
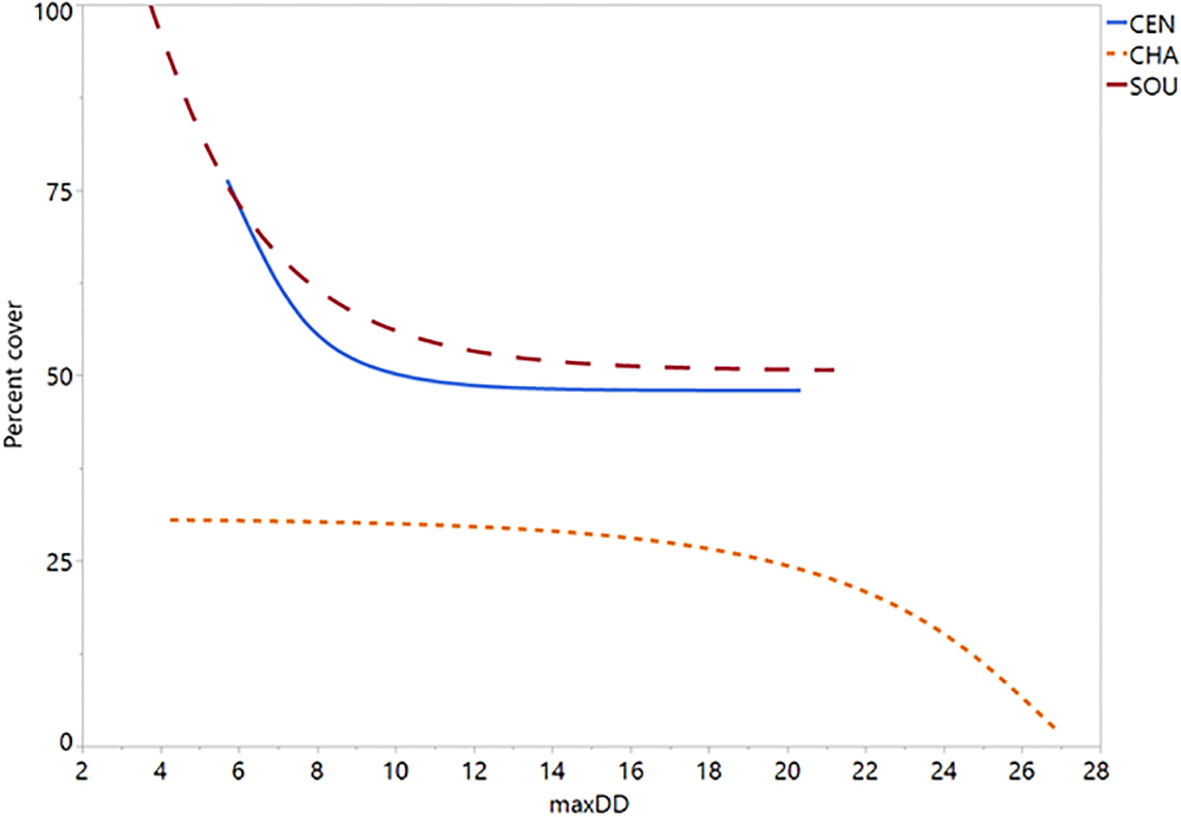
Figure 6 Silvetia percent cover plotted on scale of maximum dewpoint depression (°C). Regression slopes are all significant (P = 0). CEN, Central California; CHA, Channel Islands; SOU, Southern California.
Simulating the preferred model (M17) 10,000 times, we found strong correspondence between the actual data and our modeled data (Figure 5). Silvetia cover across all three regions declined steadily as maxDdincreased according to the actual data and the modeled fit. At the regional level, Silvetia cover in CEN and SOU responded similarly to increasing maxDdby decreasing precipitously until approximately 8°C then stabilizing (Figure 6). At the CHA region, Silvetia cover remained relatively stable until around 20°C before declining as maxDdincreased (Figure 6).
3.4 Wind
Mean near-surface wind gust (meanWs) and max near-surface wind gust (maxWs) were not significantly correlated with Silvetia cover in the three regions (Supplementary Table S2). Collinearity between Dd and Ws was low (Supplementary Figure S3). Model selection indicated that neither meanWs nor maxWs should be included in the preferred model based on AIC and BIC (Supplementary Table S2).
4 Discussion
We found large declines of the intertidal foundation species, Silvetia putatively driven by harsh, desiccating wind events across most of its geographical range, particularly the California Channel Islands and southern California mainland (Figures 3, 4). This pattern mirrors worldwide declines and range shifts in rockweeds and other fucoids (Bokn and Lein, 1978; Kautsky et al., 1986; Vogt and Schramm, 1991; Munda, 1993; Sagarin et al., 1999; Thompson et al., 2002; Lotze and Milewski, 2004; Keser et al., 2005; Torn et al., 2006; Airoldi and Beck, 2007; Ugarte et al., 2009; Lamela-Silvarrey et al., 2012; Martínez et al., 2012; Nicastro et al., 2013; Riera et al., 2015; Buonomo et al., 2018; Whitaker et al., 2023 and references therein). Spatio-temporal modeling revealed a pervasive trend of declining abundance for Silvetia populations at the Channel Islands and the California mainland south of Point Conception (PC), an important biogeographic break. Sites north of PC were characterized by a heterogeneous combination of trends, most of which indicated that Silvetia was relatively stable or increasing in cover during the study period.
Although declining rockweed populations were most prevalent in the southern California region, trends varied by site, and all three regions had examples of declining and stable sites (Figure 4). The possible causes of this complexity may, like the between-region patterns, reflect variability in meteorological and oceanographic climate, but on different spatial scales. The central California region is influenced primarily by the cool California current, while the California Current and the opposing, warmer Southern California Countercurrent (Hickey, 1979) combine to form a more complex seascape in the SCB (the region below PC including the islands). As a result, SST along the southern California mainland and the southern islands is consistently warmer, and onshore winds and fog are generally lighter relative to central California and the northwestern islands, San Miguel, and Santa Rosa Islands (Dailey et al., 1993). The islands in between occupy a transitional zone where these currents mix and SST can be highly variable (Dailey et al., 1993).
At the site level, variations in substratum aspect, slope (Harley, 2008) and extent (Gedan et al., 2011) interact with atmospheric and oceanographic variables to affect the conditions experienced by intertidal organisms including aerial temperature (Helmuth and Hofmann, 2001), wave energy (Harley and Helmuth, 2003), solar radiation (Schoch and Dethier, 1996), wind velocity, relative humidity and fog (Helmuth and Hofmann, 2001; Helmuth et al., 2006). A high degree of spatial heterogeneity exists across the network of sites in this study given its broad spatial scale, which likely corresponds to significant site-level variation in abiotic conditions (Choi et al., 2019). As a result, the mosaic of trends for Silvetia likely reflects environmental heterogeneity at both the local and regional levels, thereby influencing the population dynamics of Silvetia.
During the study period, sea surface temperatures (SST) rose more rapidly in southern California than in most other regions of the world (Hobday and Pecl, 2014). Anomalously high SSTs occurred in the northeastern Pacific Ocean during El Niño events in 2009-10 and 2014-16. The latter event, one of the strongest El Niño’s in recent history (Huang et al., 2016), resulted in exceptionally high SST anomalies in part due to the concurrence of a widespread marine heat wave in the North Pacific (Jacox et al., 2016). In addition, the average annual air temperature in southern California steadily increased from 1950 to 2010 (Gonzalez, 2020). Macroalgae including Silvetia can be stressed by oceanographic parameters including seawater temperature (Breeman, 1988; Wernberg et al., 2011; Hurd et al., 2014; Graham et al., 2018) and wave energy (Vadas et al., 1990), as well as by atmospheric conditions such as high temperatures (Bell, 1995) and high irradiance (Henley, 1992). These changes in regional atmospheric and oceanographic conditions are consistent with the general latitudinal pattern of trends we identified in Silvetia cover and suggest that declines in southern California may be linked to overall warming conditions both in the coastal ocean and on land.
Downsloping, offshore wind is a natural occurrence in western North America due to gradients between high-pressure systems in the inland Great Basin and low pressure over the Pacific Ocean. As low-pressure systems offshore of California pull air masses from inland desert areas, winds more than 25 m s−1 (56 mi h−1) (Keeley et al., 2004; Cao and Fovell, 2013) are generated through canyons and mountain passes compressing, drying, and heating the air in the process. Below PC, these conditions are called Santa Ana winds (SAWs), and recent studies suggest that downslope wind systems in central and northern California coincide with SAWs (Gershunov et al., 2021; Guirguis et al., 2023). SAWs occur annually between September and June (Cao and Fovell, 2016). However, SAW frequency and intensity are greatest during the winter months of December and January, respectively, (Guzman-Morales et al., 2016) a time of year coincident with periods of day-time aerial exposure for intertidal ecosystems in southern California. More than any other environmental variable, the seasonal pattern of diurnal emersion has been attributed to changes in the abundances of intertidal algae in the Southern California Bight (SCB) (Emerson and Zedler, 1978; Gunnill, 1980; Seapy and Littler, 1982; Littler et al., 1991).
The Santa Ana Wildfire Index (SAWTI), a model generated from the climatological data used in this study, indicates that the monthly and seasonal SAW day counts were variable but mostly remained near average or below average from 1981 to the early 2000s (see Figure 17 in Rolinski et al., 2019). After 2006, the number of SAW days per season was significantly elevated for the remainder of the study period through 2016 (Rolinski et al., 2019). This trend corresponds with negative changes in Silvetia cover observed at many study sites in the two regions, Channel Islands (CHA) and southern California mainland (SOU) which exhibited gradual declines from the beginning of the study period through around 2015 with the most precipitous losses in percent cover occurring after 2005 (Figures 3, 4).
Despite rockweeds being very desiccation-resistant for a large frondose alga (Schonbeck and Norton, 1978), extreme conditions during warm and dry periods, especially with strong, dry winds, can be detrimental. During SAW events occurring during low tide periods, Silvetia is often observed exhibiting severe drying out with fronds turning crispy. Desiccation stress is known to affect various physiological processes and conditions in rockweeds and other fucoids, including oxidative damage (Martins et al., 2021), reduced net photosynthesis, survival, and growth (Dethier et al., 2005), increased susceptibility to loss from wave action (Haring et al., 2002), and limited recruitment success (Dudgeon and Petraitis, 2001). Over time, these effects are likely to cause population declines, as observed at our monitoring sites.
SAW activity has been linked with environmental cycles including the Atlantic multi-decadal oscillation (AMO), the PDO, and the ENSO, with elevated periods of SAW activity typically coinciding with cool phases of the PDO (Rolinski et al., 2019) and ENSO (Raphael, 2003; Rolinski et al., 2019), and the warm phase of the AMO (Li et al., 2016). Although Guzman-Morales et al. (2016) found seasonal SAW intensity to increase during the warm phases of the PDO and ENSO and vice versa. This information is critical for projecting SAW activity. However, it remains unclear how SAW events will change under anthropogenic climate warming conditions. Projections of SAW activity due to climate change remain mixed due, in part, to differences in approaches used to distinguish and downscale the events (e.g., Miller and Schlegel, 2006; Hughes et al., 2011; Abatzoglou et al., 2013; Guzman-Morales et al., 2016). Some studies indicate a reduction of SAW events as anthropogenic warming increases due to a weakening in the temperature gradient between the ocean and the Great Basin (Hughes et al., 2009; Hughes et al., 2011; Guzman Morales, 2018). Others suggest that anthropogenic warming will increase the frequency of strong SAWs in late fall (Yue et al., 2014), or that more SAW days may occur at the beginning (September) and end (June) of the SAW season (Rolinski et al., 2019). Clearly, more research is needed to increase the accuracy of projecting SAW variability as climate warms. Based on patterns discussed here, however, any increases in the frequency or intensity of SAWs are likely to lead to large declines and possible extirpation of Silvetia, as well as the important ecological services the species provides.
Our results demonstrate that Silvetia, like many other fucoids throughout the world (Sagarin et al., 1999; Lamela-Silvarrey et al., 2012; Martínez et al., 2012; Nicastro et al., 2013; Riera et al., 2015), has declined significantly in southern California and the Channel Islands. This trend corresponds with elevated dewpoint depression, an indicator of SAW activity, which, in turn, may represent the phases of environmental cycles such as PDO, ENSO, and AMO that the data in this study were collected or anthropogenic forcing on SAW activity. Spatial and temporal patterns in rockweed populations, however, are complex, varying between sites as well as across regions. The network of long-term monitoring sites established by the Multi Agency Rocky Intertidal Monitoring Network (MARINe) that provided the data for this study is an ideal milieu for a detailed comparative and experimental program to elucidate the causes of rockweed declines. This information, along with more accurate future projections of SAW activity under climate change, are critical for informing conservation, and potentially restoration, of this important foundation species and the rocky intertidal biodiversity it supports.
5 Implications
Population dynamics are influenced by local- to regional-scale variability in environmental conditions across a broad range of temporal scales (Oro, 2013; Dallas and Kramer, 2022). Variations in environmental conditions arise from extensive habitat heterogeneity common in ecosystems (Tilman and Kareiva, 1997), including the rocky intertidal, where climatic variables range widely across regions to individual plots (Helmuth and Hofmann, 2001). Extreme variability in atmospheric factors such as air temperature, humidity, and wind, results from both continental scale differences in topographical landscapes composed of mountains, valleys, and plains (Keeley et al., 2004; Cao and Fovell, 2013), and microtopographical differences in substratum orientation, aspect, hue, and rugosity (Helmuth and Hofmann, 2001; Helmuth et al., 2006; Harley, 2008; Choi et al., 2019). Similarly, variance in oceanographic conditions such as temperature, pH, and wave energy, occurs across the large spatial scales of regional ocean currents and prominent land features such as headlands, to local offshore reefs and kelp forests (Harley and Helmuth, 2003). Temporally, rocky intertidal ecosystems vary as atmospheric and oceanographic conditions change on short- and long-term timescales due to tides, local weather patterns, season, and interannual and decadal climatological cycles such as the El Niño Southern Oscillation and the Pacific Decadal Oscillation (Wootton et al., 1996; Thompson et al., 2002; Menge et al., 2008). This variability presents complex challenges for ecologists testing broad-scale ecological hypotheses and forecasting population dynamics.
To account for high levels of spatial and temporal variability in environmental conditions in our study, we used long-term observational data at multiple spatial scales to test the simple hypothesis that canopy cover of the foundational rockweed Silvetia is reduced by dry air events associated with offshore Santa Ana winds. We found extensive spatial and temporal variability in Silvetia cover driven by a combination of large- and small-scale processes. Rockweed cover in all three regions of our study was significantly and often dramatically reduced by dry air events associated with Santa Ana winds, but the response of rockweed varied markedly by region. Rockweed in the southern and central study regions responded very differently depending on site, whereas the rockweed in the northern region was more stable and homogeneous across sites. Including ‘site’ as a random effect increased the deviance explained by approximately 10%, suggesting that local processes influence the dynamics of Silvetia cover, reducing or overriding the effects of regional scale factors including Santa Anas. Our results underscore the need for continued long-term monitoring of ecosystems across regions to capture both local and large-scale variation and effectively inform the conservation and restoration of important foundation species.
Data availability statement
The raw data supporting the conclusions of this article will be made available by the authors, without undue reservation.
Author contributions
SW: Conceptualization, Data curation, Formal analysis, Funding acquisition, Investigation, Methodology, Project administration, Resources, Software, Supervision, Validation, Visualization, Writing – original draft, Writing – review & editing. PR: Formal analysis, Writing – review & editing. JS: Writing – review & editing. HL: Writing – review & editing. SG: Writing – review & editing. RM: Funding acquisition, Supervision, Writing – review & editing.
Funding
The author(s) declare financial support was received for the research, authorship, and/or publication of this article. Funding for this work was provided by the California Ocean Protection Council (agreement C0302106), the NASA Biodiversity and Ecological Forecasting Program (grant no. NNX14AR62A), the Bureau of Ocean Energy Management, Environmental Studies Program (BOEM Agreement MC15AC00006), the National Oceanic and Atmospheric Administration in support of the Santa Barbara Channel Marine Biodiversity Observation Network, and the National Park Service.
Acknowledgments
We are grateful to S. Capps, Principal of Atmospheric Data Solutions, for providing the climatological time series data used in this study. The biological data we analyzed were provided by the Multi-Agency Rocky Intertidal Network (MARINe). Funding for MARINe was contributed by Bureau of Ocean Energy Management, National Park Service, Partnership of Interdisciplinary Studies of Coastal Oceans and California Ocean Protection Council. We thank the National Park Service, the University of California Natural Reserve System and The Nature Conservancy for site access and logistical support. The authors also thank A. Zuur and L. Pandori for statistical advice, L. Kui for statistical assistance, and L. Lee, R. Gaddam and M. Miner for database assistance.
Conflict of interest
The authors declare that the research was conducted in the absence of any commercial or financial relationships that could be construed as a potential conflict of interest.
Publisher’s note
All claims expressed in this article are solely those of the authors and do not necessarily represent those of their affiliated organizations, or those of the publisher, the editors and the reviewers. Any product that may be evaluated in this article, or claim that may be made by its manufacturer, is not guaranteed or endorsed by the publisher.
Supplementary material
The Supplementary Material for this article can be found online at: https://www.frontiersin.org/articles/10.3389/fevo.2024.1291310/full#supplementary-material
References
Abatzoglou J. T., Barbero R., Nauslar N. J. (2013). Diagnosing Santa Ana winds in southern California with synoptic-scale analysis. Weather Forecasting 28 (3), 704–710. doi: 10.1175/WAF-D-13-00002.1
Abatzoglou J. T., Hatchett B. J., Fox-Hughes P., Gershunov A., Nauslar N. J. (2021). Global climatology of synoptically-forced downslope winds. Int. J. Climatology 41 (1), 31–50. doi: 10.1002/joc.6607
Abbott I. A., Hollenberg G. J. (1992). Marine Algae of California (Stanford, California: Stanford University Press).
Airoldi L., Beck M. (2007). Loss, status and trends for coastal marine habitats of Europe. Oceanography Mar. Biol. 45, 345–405. doi: 10.1201/9781420050943.ch7
Akaike H. (1974). A new look at the statistical model identification. IEEE Trans. Automatic Control 19, 716–723. doi: 10.1109/TAC.1974.1100705
Bell E. C. (1995). Environmental and morphological influences on thallus temperature and desiccation of the intertidal alga Mastocarpus papillatus Kützing. J. Exp. Mar. Biol. Ecol. 191, 29–55. doi: 10.1016/0022-0981(95)00037-R
Bertness M. D., Leonard G. H. (1997). The role of positive interactions in communities: lessons from intertidal habitats. Ecology 78, 1976–1989. doi: 10.1890/0012-9658(1997)078[1976:TROPII]2.0.CO;2
Bertness M. D., Leonard G. H., Levine J. M., Schmidt P. R., Ingraham A. O. (1999). Testing the relative contribution of positive and negative interactions in rocky intertidal communities. Ecology 80, 2711–2726. doi: 10.1890/0012-9658(1999)080[2711:TTRCOP]2.0.CO;2
Bishop M. J., Coleman M. A., Kelaher B. P. (2010). Cross-habitat impacts of species decline: Response of estuarine sediment communities to changing detrital resources. Oecologia 163, 517–525. doi: 10.1007/s00442-009-1555-y
Blanchette C. A., Melissa Miner C., Raimondi P. T., Lohse D., Heady K. E., Broitman B. R. (2008). Biogeographical patterns of rocky intertidal communities along the Pacific coast of North America. J. Biogeography 35 (9), 1593–1607. doi: 10.1111/j.1365-2699.2008.01913.x
Bokn T. L., Lein T. E. (1978). Long-term changes in fucoid association of the inner Oslofjord, Norway. Norway J. Bot. 25 (1), 9–14.
Bordeyne F., Migné A., Davoult D. (2015). Metabolic activity of intertidal Fucus spp. communities: Evidence for high aerial carbon fluxes displaying seasonal variability. Mar. Biol. 162, 2119–2129. doi: 10.1007/s00227-015-2741-6
Brawley S. H., Johnson L. E. (1993). Predicting desiccation stress in microscopic organisms the use of agarose beads to determine evaporation within and between intertidal microhabitats. J. Phycology 29, 528–535. doi: 10.1111/j.1529-8817.1993.tb00154.x
Breeman A. M. (1988). Relative importance of temperature and other factors in determining geographic boundaries of seaweeds: Experimental and phenological evidence. Helgoländer Meeresunters 42, 199–241. doi: 10.1007/BF02366043
Brinkhuis B. H., Tempel N. R., Jones R. F. (1976). Photosynthesis and respiration of exposed salt-marsh fucoids. Mar. Biol. 34, 349–359. doi: 10.1007/BF00398128
Buonomo R., Chefaoui R. M., Lacida R. B., Engelen A. H., Serrão E. A., Airoldi L. (2018). Predicted extinction of unique genetic diversity in marine forests of Cystoseira spp. Mar. Environ. Res. 138, 119–128. doi: 10.1016/j.marenvres.2018.04.013
Cao Y., Fovell R. (2013). “Predictability and sensitivity of downslope windstorms in San Diego County,” Proc. 15th Conf. on Mesoscale Processes. (Portland, OR: American Meteor Society), 7.6. Available at: https://ams.confex.com/ams/15MESO/webprogram/Paper228055.html.
Cao Y., Fovell R. G. (2016). Downslope windstorms of San Diego County. Part I: A case study. Monthly Weather Rev. 144 (2), 529–552. doi: 10.1175/MWR-D-15-0147.1
Chapman A. R. O. (1995). Functional ecology of fucoid algae: Twenty-three years of progress. Phycologia 34, 1–32. doi: 10.2216/i0031-8884-34-1-1.1
Choi F., Gouhier T., Lima F., Rilov G., Seabra R., Helmuth B. (2019). Mapping physiology: Biophysical mechanisms define scales of climate change impacts. Conserv. Physiol. 7 (1), coz028. doi: 10.1093/conphys/coz028
Dailey M. D., Reish D. J., Anderson J. W. (1993). Ecology of the Southern California Bight: A Synthesis and Interpretation (Berkeley and Los Angeles, California: University of California Press).
Dallas T. A., Kramer A. M. (2022). Temporal variability in population and community dynamics. Ecology 103 (2), e03577. doi: 10.1002/ecy.3577
Davison I. R., Pearson G. A. (1996). Stress tolerance in intertidal seaweeds. J. Phycology 32, 197–211. doi: 10.1111/j.0022-3646.1996.00197.x
Dayton P. K. (1985). Ecology of kelp communities. Annu. Rev. Ecology Evol. Systematics 16 (1), 215–245. doi: 10.1146/annurev.es.16.110185.001243
Dethier M. N., Williams S. L., Freeman A. (2005). Seaweeds under stress: manipulated stress and herbivory affect critical life-history functions. Ecol. Monogr. 75 (3), 403–418. doi: 10.1890/03-4108
Dudgeon S., Petraitis P. S. (2001). Scale-dependent recruitment and divergence of intertidal communities. Ecology 82 (4), 991–1006. doi: 10.1890/0012-9658(2001)082[0991:SDRADO]2.0.CO;2
Duke N. C., Meynecke J. O., Dittmann S., Ellison A. M., Anger K., Berger U., et al. (2007). A world without mangroves? Science 317, 41–42. doi: 10.1126/science.317.5834.41b
Dye A. W., Kim J. B., Riley K. L. (2020). Spatial heterogeneity of winds during Santa Ana and non-Santa Ana wildfires in Southern California with implications for fire risk modeling. Heliyon 6, e04159. doi: 10.1016/j.heliyon.2020.e04159
Ellison A. M., Bank M. S., Clinton B. D., Colburn E. A., Elliott K., Ford C. R., et al. (2005). Loss of foundation species: consequences for the structure and dynamics of forested ecosystems. Front. Ecol. Environ. 3, 479–486. doi: 10.1890/1540-9295(2005)003[0479:LOFSCF]2.0.CO;2
Emerson S. E., Zedler J. B. (1978). Recolonization of intertidal algae: an experimental study. Mar. Biol. 44, 315–324. doi: 10.1007/BF00390895
Engle J. M., Anderson L., Burnaford J. L., Douglas M., Lohse D. P., Parsons-Field A. (2022). Unified Monitoring Protocols for the Multi-Agency Rocky Intertidal Network (Camarillo, California: BOEM Cooperative Agreement No. M19AC00023). Department of the Interior. Bureau of Ocean Energy Management. Pacific OCS Region. doi: 10.6085/AA/marine_ltm.15.3
Gedan K. B., Bernhardt J., Bertness M. D., Leslie H. M. (2011). Substrate size mediates thermal stress in the rocky intertidal. Ecology 92, 576–582. doi: 10.1890/10-0717.1
Gershunov A., Guzman Morales J., Hatchett B., Guirguis K., Aguilera R., Shulgina T., et al. (2021). Hot and cold flavors of southern California’s Santa Ana winds: Their causes, trends, and links with wildfire. Climate dynamics 57 (7-8), 2233–2248. doi: 10.1007/s00382-021-05802-z
Golléty C., Migné A., Davoult D. (2008). Benthic metabolism on a sheltered rocky shore: Role of the canopy in the carbon budget. J. Phycology 44, 1146–1153. doi: 10.1111/j.1529-8817.2008.00569.x
Golléty C., Riera P., Davoult D. (2010). Complexity of the food web structure of the Ascophyllum nodosum zone evidenced by a δ13C and δ15N study. J. Sea Res. 64 (3), 304–312. doi: 10.1016/j.seares.2010.04.003
Gonzalez P. (2020). Human-caused climate change in United States national parks and solutions for the future. Parks Steward. Forum 36, 1–64. doi: 10.5070/P536248262
Graham L. E., Graham J. M., Wilcox L. W., Cook M. E. (2000). Algae, 2nd Edition (Upper Saddle River: Prentice Hall).
Graham S., Hong B., Mutschler S., Saunders B., Bredvik J. (2018). Changes in abundance of Silvetia compressa at San Clemente Island before and during the 2015–2016 El Niño. Western North Am. Nat. 78 (4), 605–616. doi: 10.3398/064.078.0405
Guirguis K., Gershunov A., Hatchett B. J., DeFlorio M. J., Subramanian A. C., Clemesha R., et al. (2023). Subseasonal prediction of impactful california winter weather in a hybrid dynamical‐statistical framework. Geophys. Res. Lett. 50 (23), 1729. doi: 10.1007/s00382-022-06361-7
Gunnill F. C. (1980). Demography of the intertidal brown alga Pelvetia fastigiata in southern California, USA. Mar. Biol. 59, 169–179. doi: 10.1007/BF00396865
Guzman Morales J. (2018). Santa Ana winds of southern California: historical variability and future climate projections [PhD thesis]. (San Diego: University of California), 68. Available at: https://escholarship.org/uc/item/6hm499nj.
Guzman-Morales J., Gershunov A., Theiss J., Li H., Cayan D. (2016). Santa Ana winds of southern California: Their climatology, extremes, and behavior spanning six and a half decades. Geophysical Res. Lett. 43, 2827–2834. doi: 10.1002/2016GL067887
Haring R. N., Dethier M. N., Williams S. L. (2002). Desiccation facilitates wave-induced mortality of the intertidal alga Fucus gardneri. Mar. Ecol. Prog. Ser. 232, 75–82. doi: 10.3354/meps232075
Harley C. (2008). Tidal dynamics, topographic orientation, and temperature-mediated mass mortalities on rocky shores. Mar. Ecol. Prog. Ser. 371, 37–46. doi: 10.3354/meps07711
Harley C. D. G., Helmuth B. S. T. (2003). Local- and regional-scale effects of wave exposure, thermal stress, and absolute versus effective shore level on patterns of intertidal zonation. Limnology Oceanography 48, 1498–1508. doi: 10.4319/lo.2003.48.4.1498
Harms S., Winant C. D. (1998). Characteristic patterns of the circulation in the Santa Barbara Channel. J. Geophysical Research: Oceans 103, 3041–3065. doi: 10.1029/97JC02393
Hawkins S., Hartnoll R. (1985). Factors determining the upper limits of intertidal canopy-forming algae. Mar. Ecol. Prog. Ser. 20, 265–271. doi: 10.3354/meps020265
Hawkins S. J., Moore P. J., Burrows M. T., Poloczanska E., Mieszkowska N., Herbert R. J. H., et al. (2008). Complex interactions in a rapidly changing world: Responses of rocky shore communities to recent climate change. Climate Res. 37, 123–133. doi: 10.3354/cr00768
Hays C. (2006). Ecological consequences of dispersal and gene flow in an intertidal alga - ProQuest (Santa Cruz, Santa Cruz, CA: University of California).
Helmuth B., Broitman B. R., Blanchette C. A., Gilman S., Halpin P., Harley C. D. G., et al. (2006). Mosaic patterns of thermal stress in the rocky intertidal zone: Implications for climate change. Ecol. Monogr. 76, 461–479. doi: 10.1890/0012-9615(2006)076[0461:MPOTSI]2.0.CO;2
Helmuth B. S. T., Hofmann G. E. (2001). Microhabitats, thermal heterogeneity, and patterns of physiological stress in the rocky intertidal zone. Biol. Bull. 201, 374–384. doi: 10.2307/1543615
Henley W. J. (1992). Growth and photosynthesis of Ulva rotundata (chlorophyta) as a function of temperature and square wave irradiance in indoor culture. J. Phycology 28, 625–634. doi: 10.1111/j.0022-3646.1992.00625.x
Hickey B. M. (1979). The California current system-hypotheses and facts. Prog. Oceanography 8, 191–279. doi: 10.1016/0079-6611(79)90002-8
Hobday A. J., Pecl G. T. (2014). Identification of global marine hotspots: Sentinels for change and vanguards for adaptation action. Rev. Fish Biol. Fisheries 24, 415–425. doi: 10.1007/s11160-013-9326-6
Huang B., L'Heureux M., Hu Z. Z., Zhang H. M. (2016). Ranking the strongest ENSO events while incorporating SST uncertainty. Geophysical Res. Lett. 43 (17), 9165–9172. doi: 10.1002/2016GL070888
Hughes T. P., Baird A. H., Bellwood D. R., Card M., Connolly S. R., Folke C., et al. (2003). Climate change, human impacts, and the resilience of coral reefs. Science 301, 929–933. doi: 10.1126/science.1085046
Hughes M., Hall A., Kim J. (2009). Anthropogenic reduction of Santa Ana winds. (Sacramento CA: California Energy Commission), 19. Available at: https://www.energy.ca.gov/2009publications/CEC-500-2009-015/CEC-500-2009-015-D.pdf.
Hughes M., Hall A., Kim J. (2011). Human-induced changes in wind, temperature and relative humidity during Santa Ana events. Climatic Change 109, 119–132. doi: 10.1007/s10584-011-0300-9
Hurd C. L., Harrison P. J., Bischof K., Lobban C. S. (2014). Seaweed Ecology and Physiology (Cambridge, UK: Cambridge University Press).
Jackson J. B. C., Kirby M. X., Berger W. H., Bjorndal K. A., Botsford L. W., Bourque B. J., et al. (2001). Historical overfishing and the collapse of marine ecosystems. Science 293, 629–638. doi: 10.1126/science.1059199
Jacox M. G., Hazen E. L., Zaba K. D., Rudnick D. L., Edwards C. A., Moore A. M., et al. (2016). Impacts of the 2015–2016 El Niño on the California Current System: Early assessment and comparison to past events. Geophysical Res. Lett. 43 (13), 7072–7080. doi: 10.1002/2016GL069716
Jenkins S. R., Norton T. A., Hawkins S. J. (2004). Long term effects of Ascophyllum nodosum canopy removal on mid shore community structure. J. Mar. Biol. Assoc. United Kingdom 84, 327–329. doi: 10.1017/S0025315404009221h
Jones C., Fujioka F., Carvalho L. M. V. (2010). Forecast skill of synoptic conditions associated with Santa Ana Winds in Southern California. Monthly Weather Rev. 138, 4528–4541. doi: 10.1175/2010MWR3406.1
Kapsenberg L., Hofmann G. E. (2016). Ocean pH time-series and drivers of variability along the northern Channel Islands, California, USA. Limnolology Oceanography 61, 953–968. doi: 10.1002/lno.10264
Kautsky N., Kautsky H., Kautsky U., Waern M. (1986). Decreased depth penetration of Fucus vesiculosus (L.) since the 1940’s indicates eutrophication of the Baltic Sea. Mar. Ecol. Prog. Ser. 28 (1/2), 1–8.
Keeley J. E., Fotheringham C. J., Moritz M. A. (2004). Lessons from the october 2003. Wildfires in Southern California. J. Forestry 102, 26–31. doi: 10.1093/jof/102.7.26
Keser M., Swenarton J. T., Foertch J. F. (2005). Effects of thermal input and climate change on growth of Ascophyllum nodosum (Fucales, Phaeophyceae) in eastern Long Island Sound (USA). J. Sea Res. 54, 211–220. doi: 10.1016/j.seares.2005.05.001
Lamela-Silvarrey C., Fernández C., Anadón R., Arrontes J. (2012). Fucoid assemblages on the north coast of Spain: Past and preseny, (1977- 2007). Botanica Marina 55, 199–207. doi: 10.1515/bot-2011-0081
Lenihan H. S., Peterson C. H., Byers J. E., Grabowski J. H., Thayer G. W., Colby D. R. (2001). Cascading of habitat degradation: oyster reefs invaded by refugee fishes escaping stress. Ecol. Appl. 11, 748–764. doi: 10.1890/1051-0761(2001)011[0764:COHDOR]2.0.CO;2
Li A., Paek H., Yu J. Y. (2016). The changing influences of the AMO and PDO on the decadal variation of the Santa Ana winds - IOPscience. Environ. Res. Lett. 11, 1–8. doi: 10.1088/1748-9326/11/6/064019
Littler M. M. (1980). “Overview of the rocky intertidal systems of Southern California,” in The California Islands: Proceedings of a Multidisciplinary Symposium, ed. Power D.M. (Santa Barbara: Santa Barbara Museum of Natural History), 265e306.
Littler M. M., Littler D. S., Murray S. N., Seapy R. R. (1991). “Southern California rocky intertidal ecosystems,” in Ecosystems of the world. Volume 24. Intertidal and littoral ecosystems, Eds. Mathieson A.C., Nienhuis P.H. (Amsterdam: Elsevier), 273–296.
Lotze H. K., Milewski I. (2004). Two centuries of multiple human impacts and successive changes in a North Atlantic food web. Ecol. Appl. 14, 1428–1447. doi: 10.1890/03-5027
Lubchenco J. (1983). Littornia and Fucus: Effects of Herbivores, Substratum heterogeneity, and plant escapes during succession. Ecology 64, 1116–1123. doi: 10.2307/1937822
Martínez B., Arenas F., Rubal M., Burgués S., Esteban R., García-Plazaola I., et al. (2012). Physical factors driving intertidal macroalgae distribution: physiological stress of a dominant fucoid at its southern limit. Oecologia 170, 341–353. doi: 10.1007/s00442-012-2324-x
Martins M., Soares C., Figueiredo I., Sousa B., Torres A. C., Sousa-Pinto I., et al. (2021). Fucoid macroalgae have distinct physiological mechanisms to face emersion and submersion periods in their southern limit of distribution. Plants 10, 1892. doi: 10.3390/Plants10091892
Marzinelli E. M., Campbell A. H., Vergés A., Coleman M. A., Kelaher B. P., Steinberg P. D. (2014). Restoring seaweeds: does the declining fucoid Phyllospora comosa support different biodiversity than other habitats? J. Appl. Phycology 26, 1089–1096. doi: 10.1007/s10811-013-0158-5
Menge B. A., Chan F., Lubchenco J. (2008). Response of a rocky intertidal ecosystem engineer and community dominant to climate change. Ecol. Lett. 11, 151–162. doi: 10.1111/j.1461-0248.2007.01135.x
Miller N. L., Schlegel N. J. (2006). Climate change projected fire weather sensitivity: California Santa Ana wind occurrence. Geophysical Res. Lett. 33 (15), 1–5. doi: 10.1029/2006GL025808
Moore P. G. (1977). “Organization in simple communities: observations on the natural history of Hyale nilssoni (Amphipoda) in high littoral seaweeds,” in Biology of Benthic Organisms. Presented at the 11th European Symposium on Marine Biology, Galway. Eds. Keegan B. F., Ceidigh P. O., Boaden P. J. S. (Oxford: Pergamon Press), 443–451. doi: 10.1016/B978-0-08-021378-1.50050-6
Moritz M. A., Moody T. J., Krawchuk M. A., Hughes M., Hall A. (2010). Spatial variation in extreme winds predicts large wildfire locations in chaparral ecosystems. Geophys. Res. Lett. 37 (4), 1–5. doi: 10.1029/2009GL041735
Munda I. M. (1993). Changes and degradation of seaweed stands in the Northern Adriatic. Hydrobiologia 260/261, 239–253. doi: 10.1007/BF00049025
Nicastro K. R., Zardi G. I., Teixeira S., Neiva J., Serrão E. A., Pearson G. A. (2013). Shift happens: trailing edge contraction associated with recent warming trends threatens a distinct genetic lineage in the marine macroalga Fucus vesiculosus. BMC Biol. 11, 6. doi: 10.1186/1741-7007-11-6
Oro D. (2013). Grand challenges in population dynamics. Front. Ecol. Evol. 1. doi: 10.3389/fevo.2013.00002
Råberg S., Kautsky L. (2007). A comparative biodiversity study of the associated fauna of perennial fucoids and filamentous algae. Estuarine Coast. Shelf Sci. 73, 249–258. doi: 10.1016/J.ECSS.2007.01.005
Raimondi P., Ammann K., Gilbane L., Whitaker S., Ostermann-Kelm S. (2018). Power analysis of the intertidal monitoring programs at Channel Islands National Park and the Bureau of Ocean Energy Management (Fort Collins, Colorado: Natural Resource Report). NPS/MEDN/NRR—2018/1661. National Park Service.
Raphael M. N. (2003). The Santa Ana winds of California. Earth Interact. 7, 1. doi: 10.1175/1087-3562(2003)007<0001:TSAWOC>2.0.CO;2
R Core Team. (2022). R: A language and environment for statistical computing (Vienna, Austria: R Foundation for Statistical Computing). Available at: http://www.R-project.org/.
Renaud P. E., Løkken T. S., Jørgensen L. L., Berge J., Johnson B. J. (2015). Macroalgal detritus and food-web subsidies along an Arctic fjord depth-gradient. Front. Mar. Sci. 2. doi: 10.3389/fmars.2015.00031
Riera R., Sangil C., Sanson M. (2015). Long-term herbarium data reveal the decline of a temperate-water algae at its southern range. Estuarine Coast. Shelf Sci. 165, 159–165. doi: 10.1016/j.ecss.2015.05.008
Rolinski T., Capps S. B., Fovell R. G., Cao Y., D’Agostino B. J., Vanderburg S. (2016). The Santa Ana Wildfire Threat Index: Methodology and operational implementation. Weather Forecast 31, 1881–1897. doi: 10.1175/WAF-D-15-0141.1
Rolinski T., Capps S. B., Zhuang W. (2019). Santa Ana Winds: a descriptive climatology. Weather Forecast 34, 257–275. doi: 10.1175/WAF-D-18-0160.1
Sagarin R. D., Barry J. P., Gilman S. E., Baxter C. H. (1999). Climate-related change in an intertidal community over short and long time scales. Ecol. Monogr. 69, 465–490. doi: 10.1890/0012-9615(1999)069[0465:CRCIAI]2.0.CO;2
Sapper S. A., Murray S. N. (2003). Variation in structure of the subcanopy assemblage associated with southern California populations of the intertidal rockweed Silvetia compressa (Fucales). Pacific Sci. 57, 433–462. doi: 10.1353/psc.2003.0035
Sarà G., Giommi C., Giacoletti A., Conti E., Mulder C., Mangano M. C. (2021). Multiple climate-driven cascading ecosystem effects after the loss of a foundation species. Sci. total Environ. 770, 144749. doi: 10.1016/j.scitotenv.2020.144749
SAS Institute Inc. (2018). SAS Software, Copyright, SAS Institute Inc. SAS and all other SAS Institute Inc. product or service names are registered trademarks or trademarks of SAS Institute Inc. (Cary, NC, USA).
Schiel D. R., Foster M. S. (2006). The population biology of large brown seaweeds: ecological consequences of multiphase life histories in dynamic coastal environments. Annu. Rev. Ecology Evolution Systematics 37, 343–372. doi: 10.1146/annurev.ecolsys.37.091305.110251
Schmidt A. L., Coll M., Romanuk T., Lotze H. K. (2011). Ecosystem structure and services in eelgrass Zostera marina and rockweed Ascophyllum nodosum habitats. Mar. Ecol. Prog. Ser. 437, 51–68. doi: 10.3354/meps09276
Schoch G. C., Dethier M. N. (1996). Scaling up: The statistical linkage between organismal abundance and geomorphology on rocky intertidal shorelines. J. Exp. Mar. Biol. Ecol. 201, 37–72. doi: 10.1016/0022-0981(95)00167-0
Schonbeck M., Norton T. A. (1978). Factors controlling the upper limits of fucoid algae on the shore. J. Exp. Mar. Biol. Ecol. 31 (3), 303–313. doi: 10.1016/0022-0981(78)90065-5
Schonbeck M. W., Norton T. A. (1980). Factors controlling the lower limits of fucoid algae on the shore. J. Exp. Mar. Biol. Ecol. 43, 131–150. doi: 10.1016/0022-0981(80)90021-0
Seapy R. R., Hoppe W. J. (1973). Morphological and behavioral adaptations to desiccation in the intertidal limpet Acmaea (Collisella) strigatella. Veliger 16 (2), 181–188.
Seapy R. R., Littler M. M. (1982). Population and species diversity fluctuations in a rocky intertidal community relative to severe aerial exposure and sediment burial. Mar. Biol. 71, 87–96. doi: 10.1007/BF00396995
Short F. T., Koch E. W., Creed J. C., Magalhães K. M., Fernandez E., Gaeckle J. L. (2006). SeagrassNet monitoring across the Americas: case studies of seagrass decline. Mar. Ecol. 27, 277–289. doi: 10.1111/j.1439-0485.2006.00095.x
Sideman E. J., Mathieson A. C. (1985). Morphological variation within and between natural populations of non-tide pool Fucus distichus (Phaeophyta) in New England. J. Phycology 21, 250–257. doi: 10.1111/j.0022-3646.1985.00250.x
Silva P. C., Pedroche F. F., Chacana M. E., Aguilar-Rosas R., Aguilar-Rosas L. E., Raum J. (2004). Geographic correlation of morphological and molecular variation in Silvetia compressa (Fucaceae, Fucales, Phaeophyceae). Phycologia 43, 204–214. doi: 10.2216/i0031-8884-43-2-204.1
Skamarock W. C., Klemp J. B. (2008). A time-split nonhydrostatic atmospheric model for weather research and forecasting applications. J. Comput. Phys. 227 (7), 3465–3485. doi: 10.1016/j.jcp.2007.01.037
Skamarock W. C., Klemp J. B., Dudhia J., Gill D. O., Barker D., Duda M. G., et al. (2008). A description of the advanced research WRF version 3. NCAR Tech. Note NCAR/TN-475+STR (University Corporation for Atmospheric Research), 113. doi: 10.5065/D68S4MVH
Smale D. A., Teagle H., Hawkins S. J., Jenkins H. L., Frontier N., Wilding C., et al. (2022). Climate-driven substitution of foundation species causes breakdown of a facilitation cascade with potential implications for higher trophic levels. J. Ecol. 110 (9), 2132–2144. doi: 10.1111/1365-2745.13936
Smithson M., Verkuilen J. (2006). A better lemon squeezer? Maximum-likelihood regression with beta-distributed dependent variables. psychol. Methods 11 (1), 54. doi: 10.1037/1082-989X.11.1.54
Steinberg P. D. (1985). Feeding preferences of Tegula funebralis and chemical defenses of marine brown algae. Ecol. Monogr. 55, 333–349. doi: 10.2307/1942581
Steneck R. S., Graham M. H., Bourque B. J., Corbett D., Erlandson J. M., Estes J. A., et al. (2002). Kelp forest ecosystems: biodiversity, stability, resilience and future. Environ. Conserv. 29, 436–459. doi: 10.1017/S0376892902000322
Stengel D. B., Dring M. J. (1998). Seasonal variation in the pigment content and photosynthesis of different thallus regions of Ascophyllum nodosum (Fucales, Phaeophyta) in relation to position in the canopy. Phycologia 37, 259–268. doi: 10.2216/i0031-8884-37-4-259.1
Tait L. W., Hawes I., Schiel D. R. (2014). Shining light on benthic macroalgae: Mechanisms of complementarity in layered macroalgal assemblages. PloS One 9 (12), e114146. doi: 10.1371/journal.pone.0114146
Tait L. W., Schiel D. R. (2010). Primary productivity of intertidal macroalgal assemblages: Comparison of laboratory and in situ photorespirometry. Mar. Ecol. Prog. Ser. 416, 115–125. doi: 10.3354/meps08781
Thompson R. C., Crowe T. P., Hawkins S. J. (2002). Rocky intertidal communities: Past environmental changes, present status and predictions for the next 25 years. Environ. Conserv. 29, 168–191. doi: 10.1017/S0376892902000115
Tilman D., Kareiva P. (Eds.) (1997). Spatial Ecology: The role of space in population dynamics and interspecific interactions (MPB-30) (Princeton, New Jersey: Princeton University Press). Available at: http://www.jstor.org/stable/j.ctv36zpzm.
Torn K., Krause-Jensen D., Martin G. (2006). Present and past depth distribution of bladderwrack (Fucus vesiculosus) in the Baltic Sea. Aquat. Bot. 84, 53–62. doi: 10.1016/j.aquabot.2005.07.011
Ugarte R. A., Critchley A., Serdynska A. R., Deveau J. P. (2009). Changes in composition of rockweed (Ascophyllum nodosum) beds due to possible recent increase in sea temperature in eastern Canada. J. Appl. Phycology 21, 591–598. doi: 10.1007/s10811-008-9397-2
Vadas R. L., Wright W. A., Miller S. L. (1990). Recruitment of Ascophyllum nodosum: wave action as a source of mortality. Mar. Ecol. Prog. Ser. 61, 263–272. doi: 10.3354/meps061263
Viejo R. M., Cervin G., Lindegarth M. (1999). The interactive effects of adult canopy, germling density and grazing on germling survival of the rockweed Ascophyllum nodosum. Mar. Ecol. Prog. Ser. 187, 113–120.
Vogt H., Schramm W. (1991). Conspicuous decline of Fucus in Kiel Bay (western Baltic): What are the causes? Mar. Ecol. Prog. Ser. 69, 189–194. doi: 10.3354/meps069189
Wernberg T., Russell B. D., Thomsen M. S., Gurgel C. F. D., Bradshaw C. J. A., Poloczanska E. S., et al. (2011). Seaweed communities in retreat from ocean warming. Curr. Biol. 21, 1828–1832. doi: 10.1016/j.cub.2011.09.028
Wernberg T., Thomsen M. S., Baum J. K., Bishop M. J., Bruno J. F., Coleman M. A., et al. (2023). Impacts of climate change on marine foundation species. Annu. Rev. Mar. Sci. 16, 247–282. doi: 10.1146/annurev-marine-042023-093037
Whitaker S. G., Ambrose R. F., Anderson L. M., Fales R. J., Smith J. R., Sutton S., et al. (2023). Ecological restoration using intertidal foundation species: Considerations and potential for rockweed restoration. Ecosphere 14 (3), e4411. doi: 10.1002/ecs2.4411
Wood S. N. (2011). Fast stable restricted maximum likelihood and marginal likelihood estimation of semiparametric Generalized Linear Models. J. R. Stat. Soc. Ser. B Stat. Method. 73 (1), 3–36. doi: 10.1111/j.1467-9868.2010.00749.x
Wootton J. T., Power M. E., Paine R. T., Pfister C. A. (1996). Effects of productivity, consumers, competitors, and El Niño events on food chain patterns in a rocky intertidal community. Proc. Natl. Acad. Sci. U. S. A. 93 (24), 13855–13858. doi: 10.1073/pnas.93.24.13855
Yue X., Mickley L. J., Logan J. A. (2014). Projection of wildfire activity in southern California in the mid-twenty-first century. Climate dynamics 43, 1973–1991. doi: 10.1007/s00382-013-2022-3
Zedler J. B., Callaway J. C., Sullivan G. (2001). Declining biodiversity: Why species matter and how their functions might be restored in Californian tidal marshes: Biodiversity was declining before our eyes, but it took regional censuses to recognize the problem, long-term monitoring to identify the causes, and experimental plantings to show why the loss of species matters and which restoration strategies might reestablish species. BioScience 51, 1005–1017. doi: 10.1641/0006-3568(2001)051[1005:DBWSMA]2.0.CO;2
Keywords: ecosystem engineer, desiccation, rockweed, fucoid, offshore winds, prolonged desiccation events
Citation: Whitaker SG, Raimondi PT, Smith JR, Lenihan HS, Gaines SD and Miller RJ (2024) Decline of a North American rocky intertidal foundation species linked to extreme dry, downslope Santa Ana winds. Front. Ecol. Evol. 12:1291310. doi: 10.3389/fevo.2024.1291310
Received: 08 September 2023; Accepted: 19 January 2024;
Published: 15 February 2024.
Edited by:
Robert Klinger, United States Department of the Interior, United StatesReviewed by:
Kathryn Schoenrock, University of Galway, IrelandAline Migné, Sorbonne Université(CNRS), France
Fabio Abel Labra, Universidad Santo Tomás, Chile
Dominique Davoult, Sorbonne Universités, France
Copyright © 2024 Whitaker, Raimondi, Smith, Lenihan, Gaines and Miller. This is an open-access article distributed under the terms of the Creative Commons Attribution License (CC BY). The use, distribution or reproduction in other forums is permitted, provided the original author(s) and the copyright owner(s) are credited and that the original publication in this journal is cited, in accordance with accepted academic practice. No use, distribution or reproduction is permitted which does not comply with these terms.
*Correspondence: Stephen G. Whitaker, Stephen_whitaker@nps.gov