Larval development of Holothuria tubulosa, a new tractable system for evo-devo
- 1Marine Biological Laboratory, The Eugene Bell Center for Regenerative Biology and Tissue Engineering, Woods Hole, MA, United States
- 2Stazione Zoologica Anton Dohrn, Department of Biology and Evolution of Marine Organisms, Naples, Italy
To explore animal diversity, new experimentally tractable organisms must be established. Echinoderms include five groups of marine animals that have been used as developmental models for over a century thanks to their low costs, high fecundity, optically clear larvae and genetic tractability. An additional advantage of echinoderms is that their larval forms display diverse morphologies. This rich diversity enables comparative studies to investigate the evolutionary relationships among cell types, tissues, and organs. However, reproducible protocols to obtain gametes, detailed information on embryogenesis, and genomic tools have been optimized only for selected species of sea urchins and sea stars. To address this gap, we established the abundant Mediterranean sea cucumber Holothuria tubulosa as a new experimental system. Here we describe a method to reliably obtain gametes and make embryonic cultures multiple times from the same animal and characterize unique larval tissues combining immunohistochemistry and high-resolution microscopy. This work represents a step forward in our understanding of holothurian development and establishes H. tubulosa as an emerging experimental system for evo-devo and other biological disciplines.
Introduction
Echinoderms are a diverse group of organisms that includes five classes (echinoids, asteroids, crinoids, ophiuroids and holothuroids) and together with Hemichordates belong to Ambulacraria, sister group to Chordates. Studies on echinoderm embryos and larvae have made fundamental contributions to our knowledge of embryonic patterning, organ development and cell-type evolution (reviewed in Hart, 2002; Arnone et al., 2015, Arnone et al., 2016). Although belonging to the same phylum, each echinoderm larva evolved distinct cell types, such as the skeletogenic cells that support long arms in sea urchin and brittle star pluteus larvae, or the large, neuron-rich oral hoods present in the auricularia and brachiolaria larvae of sea cucumbers and sea stars. This diversity represents a valuable resource for evolutionary studies and should be thoroughly explored. However, most research so far has focused on sea urchins and sea stars, while investigations on other echinoderm classes are still limited, partially due to the lack of established protocols for embryonic cultures and genetic manipulations.
An echinoderm that has been insufficiently studied in developmental biology is the sea cucumber. As deposit-feeders, sea cucumbers are an important component of the benthic community acting as seafloor bioturbators (Purcell et al., 2016). In addition, sea cucumbers are exploited for human consumption as luxury food in Asia, and source of bioactive molecules by industries (Bordbar et al., 2011; Purcell et al., 2016; Aminur Rahman et al., 2022), resulting in uncontrolled illegal fishing. Adult sea cucumbers are also studied for their incredible regenerative capacities (García-Arrarás et al., 1999; Mashanov and García-Arrarás, 2011). While there is an increasing number of studies exploring the biology and aquaculture use of adult sea cucumbers (Perillo et al., 2024), the molecular basis of their embryonic and larval development are poorly investigated beyond two Pacific species, Apostichopus japonicus and Apostichopus parvimensis (Perillo et al., 2024). With the goal of identifying an experimentally tractable sea cucumber model in Europe, we established Holothuria tubulosa (Gmelin, 1788) for cell and developmental biology. This species is abundant in the Mediterranean Sea and the Eastern Atlantic Ocean (Tortonese et al., 1965; Koukouras et al., 2007), it accelerates the degradation of seagrass detritus in Posidonia oceanica meadows (Gustato et al., 1982; Francour, 1990; Coulon and Jangoux, 1993; Costa et al., 2014) and it is now severely endangered by the local fish market (Rakaj et al., 2018, Rakaj et al., 2021).
The Mediterranean H. tubulosa has an annual reproduction cycle with the breeding season between June and September (Despalatović et al., 2004; Dereli et al., 2016; Tahri et al., 2019; Pasquini et al., 2022). Natural spawning events have been reported in the literature (Valls, 2004; Ocana et al., 2005) and reproduced in laboratory conditions through a combination of thermal stimulation and thermal shock (Rakaj et al., 2018; Léonet et al., 2019). These methods require freshly caught animals that are separated into males and females only by gamete observation, hence after the beginning of the spawning process, and leave the animals with empty gonads. One alternative to obtain gametes is whole body dissection (Léonet et al., 2019), but it has the disadvantage of leading to dead animals. As a consequence, these techniques make it difficult for researchers to consistently obtain embryonic cultures from the same individual and require the use of many animals, stressing the need of developing non-invasive methods for gonad extraction.
In sea cucumber ovaries, the oocytes are arrested in prophase I of meiosis. Therefore, biopsied oocytes, in contrast to those naturally spawned, must be matured for in vitro fertilization. This was achieved for H. tubulosa and Holothuria scabra using Thioredoxin-2 (Trx), a protein isolated from sea urchin spawns (Léonet et al., 2019; Delroisse et al., 2021; Léonet et al., 2019) and only on H. scabra using the synthetic six amino acid catalytic site of Trx (Léonet et al., 2019).
In this work, we present a reproducible protocol to obtain gametes through microsurgery and to mature oocytes and culture H. tubulosa embryos up to the juvenile stage. Combining immunohistochemistry and high-resolution microscopy, we describe the development of this species focusing on unique structures of sea cucumber larvae. Finally, using serotonin immunostaining, we provide an example of what echinoderm larvae (including H. tubulosa) can teach us about the diversification of anatomical structures in closely related organisms.
Materials and methods
Animal collection and embryonic cultures
Two groups of twenty H. tubulosa adult individuals were collected by scuba-diving at 5–10 meters depth from a rocky site of the Gulf of Naples (40°47’50’’N and 14°12’04’’E) along the southern Tyrrhenian coast in the summer 2023. The animals were collected by the IRM core facility and transferred to the Marine Resources for Research Facility at SZN, where they were acclimated in 150L tanks for one week (Figure 1A) and then transferred to 500L tanks for long term maintenance. The tanks are connected to an open system of running seawater directly pumped from the sea (9 meters depth, 250 meters from the coast), therefore water follows seasonal temperature variation (14 ± 1°C to 25 ± 1°C). By keeping adult H. tubulosa in these conditions we could obtain gametes from June to January.
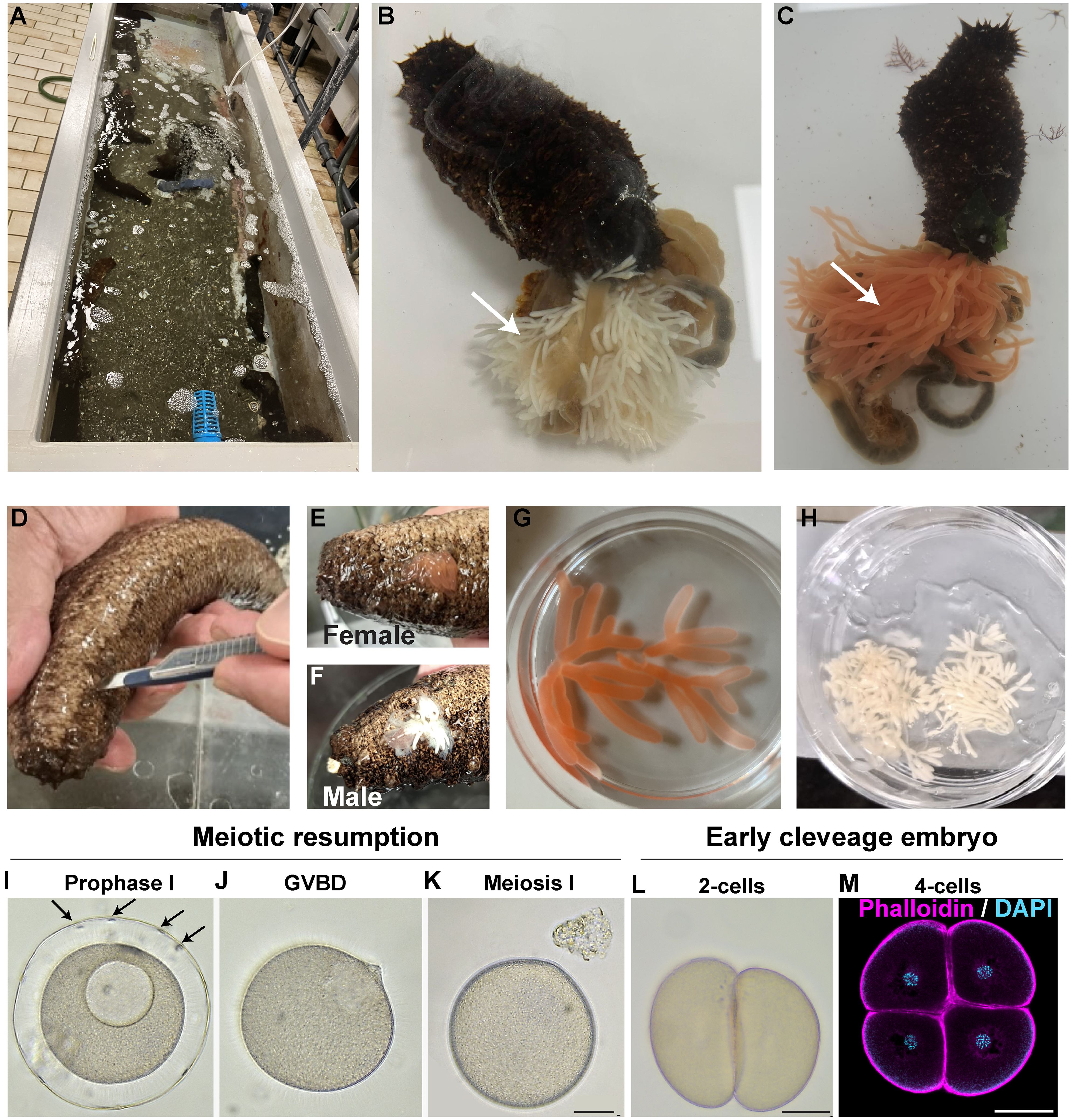
Figure 1 New methods for gonad extraction and oocyte maturation in H. tubulosa. (A) H. tubulosa adults are collected in the Gulf of Naples and housed in circulated sea water tanks at the Marine Resources for Research Facility, Stazione Zoologica Anton Dohrn. (B, C) Evisceration of (B) male and (C) female H. tubulosa. Arrows indicate male (white) and female (orange) gonads. (D) Gonads can be extracted by making a cut on the ventral-left side of the animal. (E, F) Female (E) or male (F) gonads are collected by squeezing around the cut site. (G) Ready to use female ovaries and (H) male testis in a dish. (I–K) Oocyte meiotic resumption using the Trx 6-aa. Arrows in I point at follicle cells around the prophase I arrested oocyte. (L, M) First embryonic divisions generate equal blastomeres. Scale bars 50um.
Oocyte maturation was achieved using the Trx peptide (Biomatik) 1mg/mL with a maturation index of 82.88% (on 247 total oocytes counted) assessed counting the number of oocytes that lost follicle cells and presented broken down nuclei. Mature eggs were washed with FSW once, 1 μl of dry sperm was diluted in 10 mL of FSW and some drops were added to the eggs concentrated in 10 mL. After 5 minutes, fertilized eggs were washed several times with FSW. Embryos were reared in 500 mL glass beakers at 24°C under a 12h light/dark cycle. Larval cultures were maintained up to the juvenile stage by exchanging half of the FSW with fresh FSW and by feeding them with a mix of Isochrysis galbana and Rhodomonas salina algae 2–3 times per week.
Immunohistochemistry
Specimens were collected and fixed overnight at 4°C in 4% paraformaldehyde (PFA) in FSW, washed x3 in PBS and stored in 70% EtOH for anti-MHC (Andrikou et al., 2013) and anti-acetylated tubulin (Sigma-Aldrich, St Louis, MO, USA) immunohistochemistry. Specimens were fixed in 4% PFA in FSW 3 hours at room temperature (RT) and stored in FSW with 0.01M sodium azide for anti-serotonin (#S5545; Sigma-Aldrich) and anti synaptotagmin (1E11,Nakajima et al., 2004) immunohistochemistry. Samples were then washed in 0.3% triton X-100 in PBS for 15 min at RT, followed by 3–5 washes in 0.1% Tween-20/PBS and finally incubated in blocking solution (3% bovine serum albumin/PBST) for at least 1 hour at RT before adding primary antibodies (anti-MHC 1:100, anti-serotonin 1:500, anti-acetylated tubulin 1:200) and phalloidin 1:300 O/N at 4°C. The appropriate Alexa Fluor secondary antibody was used 1:1000. DAPI was added at a final concentration of 0.01 mg/mL to stain nuclei.
Image acquisition and analysis
Specimens were imaged using a LEICA DMi8 Inverted Microscope operating in bright field and polarized light mode. For the immunostaining, embryos and larvae were mounted for imaging with a Zeiss (Jena, Germany) LSM 700 confocal microscope and pictures were analyzed using ImageJ (v1.53v) (Schindelin et al., 2012). Statistical analysis was performed using GraphPad Prism Version 10.2.1 (339).
Results
New methods to obtain gonads and induce oocyte maturation in H. tubulosa
A challenge working with sea cucumbers is the lack of reproducible and sustainable protocols to obtain gametes. Because with natural spawning (Rakaj et al., 2018) it is not possible to control fertilization in vitro, or to obtain gametes from the same animal multiple times, we tested two alternative methods.
First, we tried evisceration, an ejection of organs from the body cavity that represents the sea cucumber’s natural response to stresses such as predation. We induced evisceration by injecting 1mL of potassium chloride (1M) in the body cavity, followed by a few seconds of animal shaking. After less than a minute the animals eviscerated, releasing gonads and other internal organs into the sea water (Figures 1B, C). Although highly reproducible, this method has the disadvantage of obtaining a huge amount of gonads that most likely are not needed for developmental studies. Moreover, evisceration leads to empty animals that need months to regenerate their gonads. As a second method, we tested gonad extraction by microsurgery. We made a 1.5 cm cut along the ventral-left side of the animal with a clean scalpel (Figure 1D). By gently squeezing the animal we were able to obtain a small amount of gonads (Figures 1E–H) that is enough to make thousands of embryos. The advantage of this method we developed is that the wound closes in a few hours and heals in a few days and the same animals can be reused multiple times.
Because these methods do not follow the natural spawning cycle, the oocytes obtained from the female ovaries through evisceration and microsurgery are arrested in prophase I of meiosis. A hormonal stimulus is therefore required to induce oocyte maturation for subsequent fertilization (Delroisse et al., 2021). The lack of a universal hormone suitable for every sea cucumber species has been a limiting step for their use in developmental biology. Recently, a 6-aa peptide isolated from the hormone thioredoxin has been successfully used to induce oocyte maturation in Holothuria polii (Léonet et al., 2019). We synthetized this peptide and tested its activity on H. tubulosa oocytes at a range of concentrations from 0.1 mg to 1 mg in sea water. H. tubulosa oocytes are usually wrapped in a layer of follicle cells (Figure 1I, arrows) that detach during maturation. The oocytes treated with 1 mg/mL peptide all resumed meiotic maturation within two hours, as seen by germinal vesicle breakdown and release of follicle cells (Figures 1J, K). No sign of maturation was observed at lower concentrations, even with longer incubation times. Activated oocytes were successfully fertilized (as confirmed by the first cell cleavage observed in most oocytes about two hours after the addition of the sperm (Figure 1L)) but we did not observe formation of a clear, thick fertilization membrane as previously reported by natural spawning methods (Rakaj et al., 2018). Altogether, we defined a reliable method to obtain and mature oocytes in H. tubulosa that allows researchers to work with the same animal multiple times and without depending on natural spawning events.
Morphogenesis of H. tubulosa larvae
We next characterized embryonic and larval development using light and confocal microscopy. As previously reported, the first embryonic divisions give rise to 2 equal blastomeres 2 hours after fertilization (hpf) and 4 blastomeres 3 hpf (Rakaj et al., 2018; Perillo et al., 2024) (Figures 1L, M). Blastulae (12 hpf) are round and F-actin staining showed polarization of ectodermal cells, with apical polarity opposite to the blastocoel (Figure 2A). At the gastrula stage (29 hpf) the embryo elongates along its antero-posterior axis and the archenteron invagination begins at the vegetal pole (Figure 2B). Cells in the archenteron (the future gut) at the early and mid- gastrula stages appear bigger than the ectodermal cells and with a round shape (Figure 2C, insert). By the end of gastrulation, a straight gut is formed and larvae start feeding (50 hpf, Figures 2D, E).
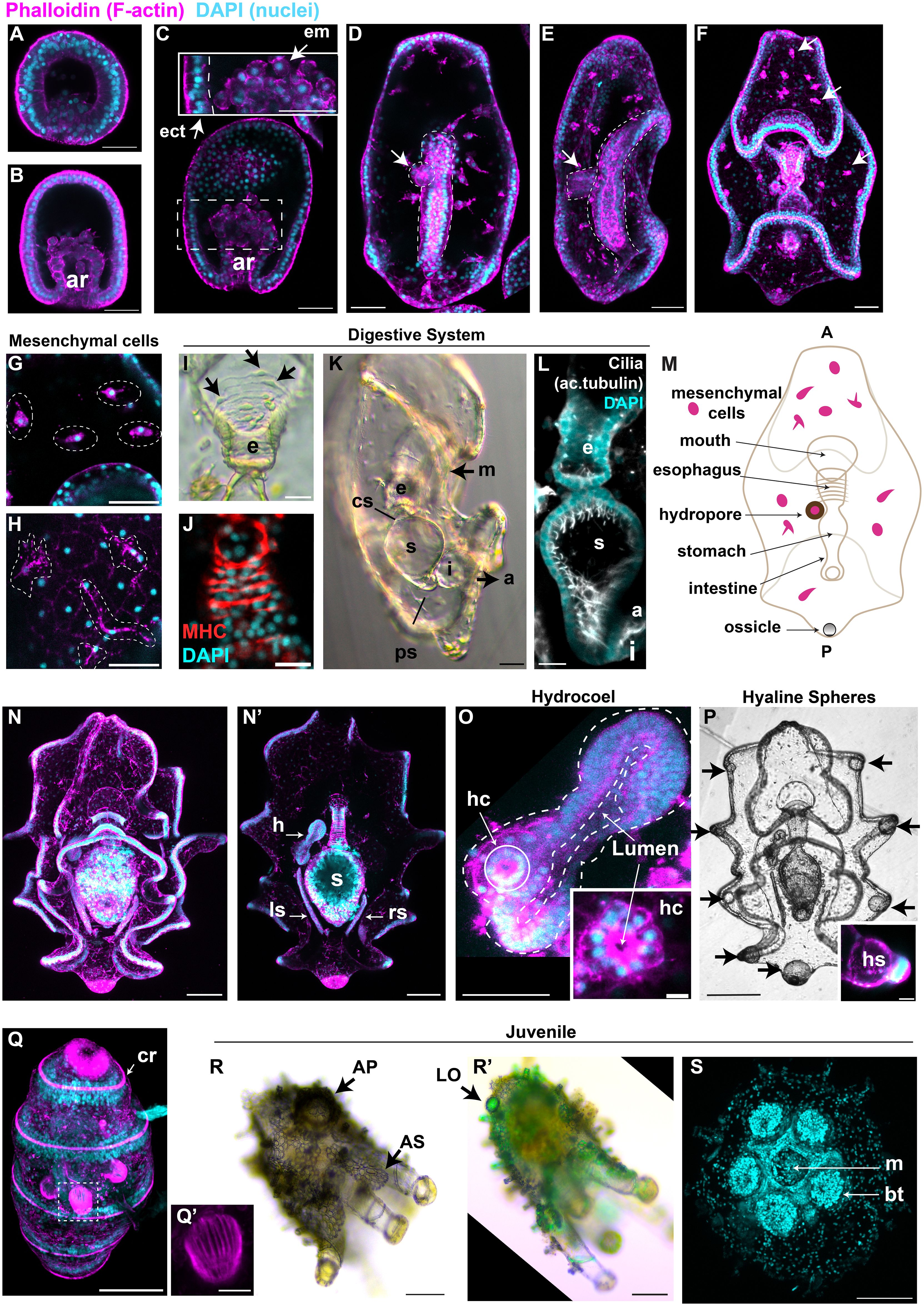
Figure 2 Detailed characterization of H. tubulosa larval development highlights distinct structures. For all images, nuclei are labeled with DAPI in cyan and F-actin is labeled with phalloidin in magenta. (A) Swimming blastula and (B) early gastrula (ar, archenteron). (C) Gastrula, insert shows the endomesodermal round cells from the tip of the growing archenteron (ect, ectoderm; em, endomesoderm). (D) Dorsal view of an early auricularia larva formed at the end of gastrulation. (E) Lateral view of early auricularia. Dotted lines highlight the straight gut, arrow points at the hydropore. (F) Auricularia larva, arrows point at mesenchymal cells rich in actin. (G, H) Mesenchymal cells in auricularia (G) and late auricularia (H). (I) Bright field image of the esophagus (e) of an auricularia larva shows single esophageal muscle fibers. Arrows indicate muscle cell body. (J) Muscles are also stained with the anti-myosin heavy chain (MHC) antibody. (K) Auricularia lateral view (m, mouth; cs, cardiac sphincter; s, stomach; i, intestine; ps, pyloric sphincter; a, anus). (L) Lateral view shows that the auricularia digestive system is highly ciliated. (M) cartoon shows the main tissues of the auricularia (A, anterior; P, posterior). (N) Ventral view of a late auricularia, focus on the ectodermal folding. (N’) Same larva but with a focus on the internal tissues (h, hydrocoel, lf, left somatocoel; rl, right somatocoel). (O) Focus on the hydrocoel (dotted lines). The hydropore canal (hc) is the dorsal-most region of the hydrocoel and it is highlighted by a solid white line. (P) Black and white image of a late auricularia, arrows point at the hyaline spheres (hs). Insert shows the thick actin layer around the hyaline sphere, scale bar 20μm. (Q) Late doliolaria has five ciliary rings (cr) rich in actin. (Q’) Tentacles are formed by parallel muscle fibers. (R) Bright field image of an early juvenile. Arrow indicates one of the many adult skeletal elements (AS). AP: ambulacral podium (R’) Same early juvenile under the polarized light to visualize the skeleton. Arrow points at the larval ossicle that formed during embryogenesis (LO). (P, Q) are ventral-lateral views. (S) Ventral view of an early juvenile shows the mouth (m) and buccal tentacle (bt). Fluorescent images are z-projections from inverted confocal microscopy. Scale bars are 20μm in: (I–L, Q’); 50μm in: (A–H, O); 100μm in: (N, N’, P–R, R’, S).
By this stage the hydropore is formed, a short tube that connects the blastocoel to the outside environment (Figures 2D, E, arrow). The early auricularia (3 days) is characterized by new folds in the ectoderm, the formation of the ossicle (described in Figure 3) and by the appearance of mesenchymal cells that are rich in actin and are localized around the blastocoel (Figures 2F arrows, G). These mesenchymal cells are still rich in actin in the later auricularia stage, with long filamentous projections (Figure 2H). At this stage, the tripartite gut is composed of an esophagus covered by parallel circumesophageal muscle fibers that are mononucleated (Figures 2I) and are positive to an anti-myosin heavy chain antibody (Figure 2J), a round stomach and a tubular intestine (Figure 2K). The esophagus and the stomach are separated by the cardiac sphincter constriction, and a pyloric sphincter is present between stomach and intestine, differently from the sea star larval gut (Annunziata et al., 2013) (Figure 2K). The lumen of the digestive system is heavily ciliated as shown by acetylated tubulin staining (Figure 2L). Summary of these structures are shown in Figure 2M.
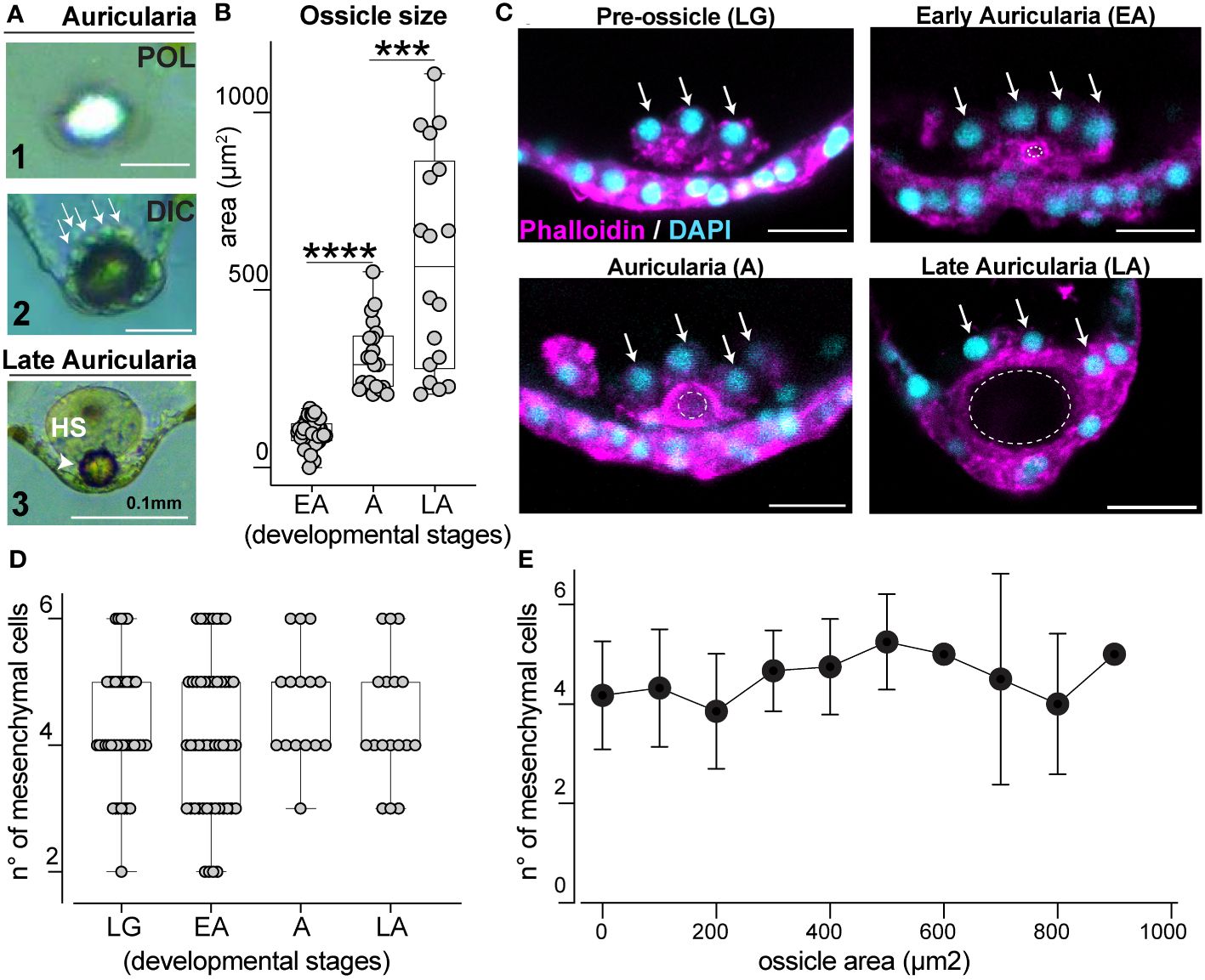
Figure 3 Ossicle grows in close association with a defined pool of mesenchymal cells. (A) Ossicle seen under polarized (POL, panel 1) and differential interference contrast (DIC, panel 2 and 3) lights. Arrows indicate mesenchymal cells on the anterior-end of the ossicle. Panel 3 shows a hyaline sphere on top of an ossicle in a late larva; HS, hyaline sphere. (B) Ossicle size increases over time. EA, early auricularia; A, auricularia; LA, late auricularia; n= individual larvae; for EA n=48, mean= 98.90μm2; for A n=21, mean= 310.7μm2; for LA n=18 mean= 579.7μm2. (C) Mesenchymal cells (arrows) at the posterior end of the larva are associated with the ossicle (dotted circles). Scale bar 20μm. (D) Number of mesenchymal cells near the ossicle site during development does not significantly change overtime. LG, late gastrula; EA, early auricularia; A, auricularia; LA, late auricularia. n= individual larvae; LG n= 42, mean n°= 4.286; EA n= 50, mean n°= 4.140; A n= 15, mean n°= 4.667; LA n= 17, mean n°= 4.412. (E) Correlation between ossicle size (area) and number of mesenchymal cells associated with it. Values are the same from graphs (B, D). In (B, D), bars represent mean with standard error of the mean. For graphs in (B, D) the Two-sided Student’s t-test was run, ****p<0.0001; ***p<0.001. Experiments were repeated for three biological replicates.
As larvae increase in size, the number of ectodermal folds increase (Figure 2N). At this stage, the hydropore becomes part of the hydrocoel (the future water vascular canal (Udagawa et al., 2022)), a tissue with an actin-rich apical side towards the central lumen (Figures 2N’, O and insert). Two additional thin tubes, the left and right somatocoels, form laterally to the stomach (Figure 2N’). The function of these additional tissues is unknown, but similar tubular tissues are shared with sea star larvae and are thought to be involved with buoyancy (Potts, 2003; Perillo et al., 2023). At this stage, the hyaline spheres appear (Figure 2P), spherical structures that serve as energy storage to be used during metamorphosis (Peters-Didier and Sewell, 2019). Hyaline spheres are located at the tip of the larval arms where numerous nuclei are clustered (Figure 2P insert).
During metamorphosis there is a dramatic rearrangement of larval structures to transform into a doliolaria and then a pentactula, stages characterized by five ciliary band rings strongly enriched for actin (Figure 2Q, late doliolaria). Actin staining shows that the tentacles are formed by many parallel muscle fibers (Figure 2Q’). The juvenile is characterized by skeletal elements that are visible under bright field and polarized light, such as adult skeletal structures that wrap around the tentacles and on the body walls, and the larval ossicle that is located posteriorly (Figures 2R, R’). A ventral oral view of the juvenile shows the central mouth surrounded by the five buccal tentacles (Figure 2S).
Overall, this detailed analysis of larval morphology with immunostainings, bright field and polarized light allowed us to define the different tissues of H. tubulosa larval stages.
Ossicle morphogenesis is accompanied by a defined pool of mesenchymal cells
Ossicles, or skeletal rods, are unique structures of sea cucumber larvae that are thought to be involved with orientation of the larval body (Pennington and Strathmann, 1990; Young et al., 2003). In the Holothuria genus, larvae only have one ossicle that is localized near the posterior ectoderm (Young et al., 2003). In H. tubulosa, this single, spherical ossicle can be visualized with polarized light (Figure 3A1) and is surrounded by mesenchymal cells (Figure 3A2, arrows). In the late auricularia, a hyaline sphere (HS) is visible on the anterior-end of the ossicle (Figure 3A3). To explore ossicle morphogenesis, we characterized how this structure forms over developmental time. We first measured the area of the central section of the ossicle from when it first appears (early auricularia, EA) until the late auricularia stage and found that ossicle size consistently increases over time (Figure 3B).
In other echinoderm larvae, specialized mesenchymal cells contribute to skeletal element formation (Vidavsky et al., 2014). In H. tubulosa, we observed clusters of mesenchymal cells gathering at the larval posterior end from before the ossicle appears and throughout its growth (Figure 3C, arrows). We next tested whether ossicle growth correlates with the number of mesenchymal cells clustering around it over time and found that this number is constant, averaging between 2 and 6 from before ossicle formation until later stages (Figure 3D, see figure legend for statistics). To filter out the effect of the developmental stages and to further define the relationship between ossicle size and number of mesenchymal cells, we correlated ossicle size (from the smallest to the biggest area) with the number of cells around it. This analysis showed that increased ossicle size does not correlate with increased number of mesenchymal cells (Figure 3E). Altogether our data show that ossicle growth does not rely on an increased number of cells around it, but it is instead associated with a defined pool of mesenchymal cells that cluster around the posterior end of the larva prior to the formation of the ossicle.
H. tubulosa serotonergic neurons: comparisons with other echinoderms
An open question in evo-devo is how functionally related structures such as organs evolved different organizations and shapes. An example is the arrangement of serotonergic neurons, that are among the first type of neurons to differentiate in echinoderm larvae and are involved in the regulation of their swimming behavior (Bisgrove and Burke, 1987; D’Aniello et al., 2020; Obukhova et al., 2024). To explore the development of this neuronal type in H. tubulosa larvae, we performed serotonin immunostaining on larval stages (early auricularia, auricularia and late auricularia) to analyze the distribution of serotonin neurons. The first serotonergic neurons appeared at the end of gastrulation in the early auricularia and are localized in the anterior hood and dorsal anterior ectoderm (Figures 4A-A’’). At the auricularia stage these neurons extend long projections in both territories, and the neurons of the anterior hood are localized in the ciliary band and ventral ectoderm (Figures 4B-B’’). By the late auricularia stage serotonergic neurons are restricted to the anterior ventral and dorsal ciliary band only (Figures 4C-C’’). Using the pan neuronal marker synaptotagmin (1E11 antibody), we confirmed that serotonergic neurons are specifically localized within the anterior ciliary band, including the oral hood (Figures 4D-D’’).
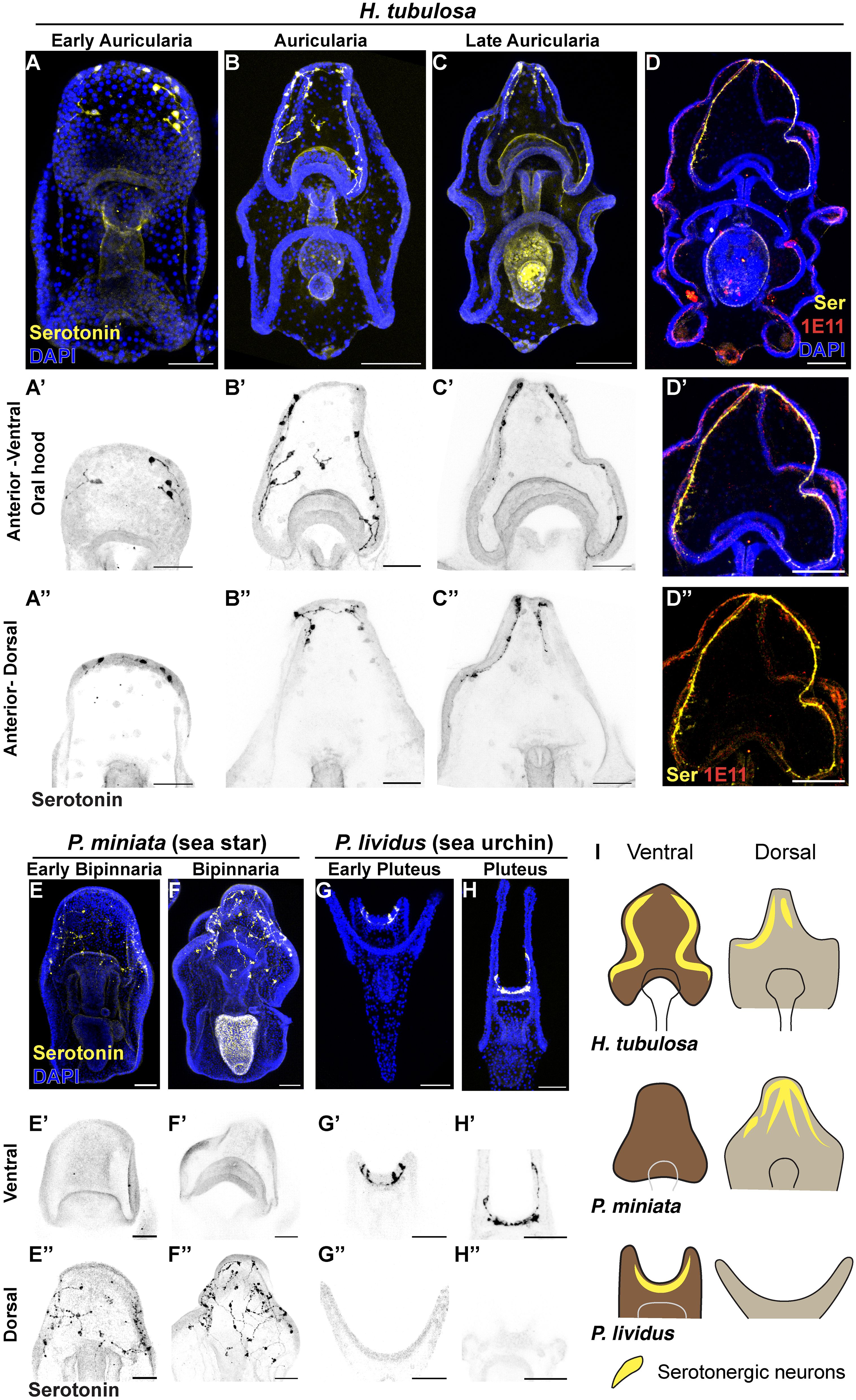
Figure 4 The localization of serotonergic neurons in H. tubulosa larvae differs from sea star and sea urchin patterns. (A–C’’) Immunostaining of serotonin at three auricularia stages (3 days early auricularia; 1 week auricularia; 2 weeks late auricularia). (D–D’’) Serotonin immunostaining overlaps with the pan-neuronal marker 1E11 in a late auricularia larva. (E–H’’) Serotonin immunostaining in the sea star Patiria miniata 4 days (E) and 1 week (F) bipinnariae and the sea urchin Paracentrotus lividus 48 hours (G) and 1 week (H) plutei. Signal in the stomach of larvae in C and F is not specific as it is autofluorescence from algae. (I) Cartoons summarizing the localization of serotonergic neurons in three echinoderm larvae. Dark brown: anterior-ventral; Light brown: anterior-dorsal. Yellow lines indicate serotonergic neurons. Scale bar 100μm in: (A–D, D’, D’’); 50μm in: A’-A’’, B’-B’’, C’-C’’, E-H’’.
To provide an example of how valuable the use of echinoderm larvae is to explore the evolution of the nervous system, we performed serotonin immunohistochemistry on larvae of the sea star Patiria miniata and of the sea urchin Paracentrotus lividus. Although sea star larvae have the anterior ectoderm organized in a large oral hood like sea cucumbers, serotonergic neurons are exclusively localized in the anterior dorsal ectoderm and are absent from the oral hood, as reported also for other stages (Cheatle Jarvela et al., 2016; Pagowski, 2024) (Figures 4E-E’’; 4F-F’’). Conversely, serotonergic neurons in the sea urchin P. lividus are localized in the apical organ of the anterior hood but absent from the dorsal ectoderm (Figures 4G-G’’; 4H-H’’), similarly to what was previously reported in other species (Beer et al., 2001; Burke et al., 2006; Angerer et al., 2011; Perillo et al., 2018; Wood et al., 2018; Cocurullo et al., 2024). Moreover, in the late pluteus larva we found that these serotonergic neurons extend towards the arms (Figures 4H-H’).
Together, these results show that while serotonergic neurons are distributed in both the ventral and dorsal anterior ectoderm in H. tubulosa, the same neurons are exclusively expressed in only one domain, being dorsal in the sea star and ventral in sea urchin (Figure 4I).
Discussion
The field of evo-devo relies on developmental comparisons across phylogenetically related species. For instance, several species of sea urchins contributed to scientific advance by leveraging on their diverse biology and geographic locations (i.e. Strongylocentrotus purpuratus, Lytechinus variegatus, Lytechinus pictus, P. lividus, Hemicentrotus pulcherrimus) (McClay, 2011).
In a recent review paper (Perillo et al., 2024), we have thoroughly discussed how sea cucumber embryos and larvae can be valuable systems to address the open questions in evo-devo, including understanding the origins of bilaterian structures. Among sea cucumbers, developmental and evo-devo studies have relied almost exclusively on A. parvimensis from the US Pacific Coast and A. japonicus from Asia (Pacific Ocean), both species belonging to the same genus Apostichopus (Clark, 1913; Yang et al., 2015).
In this work we aimed to expand the breadth of sea cucumber species used as experimental models and introduce H. tubulosa as the first species of the Holothuria genus that has been established to explore animal development and that will synergize with the other echinoderms.
Advantages of this species are that adult H. tubulosa are locally available in the Tyrrhenian Sea, right offshore SZN where animals can be kept in tanks (avoiding the spawning risks associated with shipping and ensuring animals stay gravid from June to January), plus embryos and larvae are optically clear. These features make H. tubulosa the first sea cucumber species that can be reliably used in Europe for evo-devo.
A bottleneck that prevented the use of these animals in the laboratory has been lack of methods to obtain embryonic cultures without depending on natural spawning (Perillo et al., 2024) and difficulty of having a species locally available. We addressed this challenge with our microsurgery method that enables the extraction of small pieces of gonads from H. tubulosa by making a cut along the anterior ventral-left side of the animals. Distinct from the other echinoderms, sea cucumbers possess a single gonad located anteriorly (Ruppert et al., 2004) that in Holothuridae is positioned to the left of the mediodorsal mesentery (Smirnov, 2012). Our method is inspired by the methodology used for decades to extract gonads from sea stars (Meijer and Guerrier, 1984) and has proved to be efficient and sustainable, allowing the reuse of the same animals several times.
The extraction method, coupled with the oocytes maturation by the 6aa-Trx peptide, enables fertilization of H. tubulosa oocytes resulting in abundant and synchronous embryonic cultures that reach the metamorphosis stage in about four weeks. In the past, H. tubulosa embryonic development has been described with drawings (Selenka, 1867) and light microscopy (Rakaj et al., 2018) only. In our study we use high resolution light and confocal microscopy coupled to immunohistochemistry to examine specific details of the embryos and larvae and of juveniles. This detailed description provides the foundations for further investigations aiming to functionally dissect H. tubulosa development. We also describe the dynamics of ossicle formation during development, from the late gastrula stage to the late auricularia stage, showing that while the ossicle grows in size, the number of mesenchyme cells surrounding it is stable over time. The sea cucumber ossicle does not elongate into skeletal rods like in the sea urchin larvae, however similarly to what observed in sea urchins (Zuch and Bradham, 2019; Gildor et al., 2021), there is a defined number of mesenchymal cells that contributes to spicule formation. The only report available on the ossicle formation of another sea cucumber species, A. parvimensis, shows that the gene Alx1 plays an important role for ossicle specification (McCauley et al., 2012) as it happens in sea urchins. Moreover, the enriched F-actin staining around the ossicle (Figure 3C) resembles what observed around the spicules of sea urchin larvae (Winter et al., 2021; Hijaze et al., 2024). This advocates for a similar role of cytoskeleton in biomineral formation, suggesting that these two structures might be evolutionary related. In the future, it will be critical to develop molecular tools to investigate the contribution of mesenchyme cells to ossicle morphogenesis and to understand its contribution to larval behaviors.
Finally, we present the first serotonin immunostaining in H. tubulosa from early to late auricularia and highlight pattern commonalities and differences compared to other described echinoderm species. In accordance with the description in other sea cucumber species (Burke et al., 1986; Lacalli and Kelly, 2002; Byrne et al., 2006; Nakano et al., 2006; Bishop and Burke, 2007; Zheng et al., 2022) and differently from sea urchin and sea stars, serotonin neurons are absent in the oral region of the larvae. When we compared serotonin staining in H. tubulosa auriculariae with sea urchin and sea star larvae (Figure 4I), we found that whereas serotonergic neurons are exclusively located in the dorsal ectoderm in sea star and in the ventral ectoderm in sea urchin, in H. tubulosa they are distributed in both sides, a trait that could be specific to sea cucumber larvae or to the Holothuridae family. How does this distribution of serotonergic neurons relate to the way larvae perceive and react to the environment? This is an intriguing question to be addressed in a comparative framework within echinoderms.
In the future, it will be important to overcome the reproductive seasonality of H. tubulosa by breeding animals in laboratory-controlled conditions. Also, the development of genomic and transcriptomic information (that are currently lacking except for a draft genome with low coverage (Kyritsi et al., 2023)), the setup of functional approaches such as morpholino antisense oligonucleotide injection and CRISPR-Cas9 and the development of scRNAseq data from different larval stages, will be the next steps to characterize this new emerging experimental system in evo-devo. The outcome of increased detailed characterization of H. tubulosa larvae will likely result in novel research questions.
Data availability statement
The original contributions presented in the study are included in the article/supplementary material. Further inquiries can be directed to the corresponding authors.
Ethics statement
Ethical approval was not required for the study involving animals in accordance with the local legislation and institutional requirements because The species used in the work (Holothuria tubulosa) is not included in any taxa listed in Directive 2010/63/EU that regulates the use of live animals for experimental purposes. Therefore, the experiments and animal sampling do not require authorizations.
Author contributions
MP: Conceptualization, Investigation, Methodology, Resources, Supervision, Writing – original draft, Writing – review & editing. TA: Investigation, Methodology, Writing – review & editing. AT: Methodology, Writing – review & editing. RA: Conceptualization, Investigation, Methodology, Resources, Supervision, Writing – original draft, Writing – review & editing.
Funding
The author(s) declare financial support was received for the research, authorship, and/or publication of this article. MP is supported by the Eugene Bell Center Endowment Fund. RA and AT are supported by SZN institutional funds. TA is supported by SZN-Open University PhD fellowship.
Acknowledgments
We thank Maria Ina Arnone for kindly providing the MHC antibody, Davide Caramiello for his assistance with animal husbandry and Zak Swartz for useful comments on the manuscript.
Conflict of interest
The authors declare that the research was conducted in the absence of any commercial or financial relationships that could be construed as a potential conflict of interest.
Publisher’s note
All claims expressed in this article are solely those of the authors and do not necessarily represent those of their affiliated organizations, or those of the publisher, the editors and the reviewers. Any product that may be evaluated in this article, or claim that may be made by its manufacturer, is not guaranteed or endorsed by the publisher.
References
Aminur Rahman M., Ismail M., Shamim Parvez M., Asadujjaman M., Ashik A., Habibur Rahman Molla M. (2022). Echinoderm fisheries: their culture, conservation, bioactive compounds and therapeutic applications. J. Biol. Stud. 5(3), 413–443. doi: 10.62400/jbs.v5i3.7053
Andrikou C., Iovene E., Rizzo F., Oliveri P., Arnone M. I. (2013). Myogenesis in the sea urchin embryo: the molecular fingerprint of the myoblast precursors. Evodevo 4, 33–33. doi: 10.1186/2041-9139-4-33
Angerer L., Yaguchi S., Angerer R., Burke R. (2011). The evolution of nervous system patterning: Insights from sea urchin development. Dev. (Cambridge England) 138, 3613–3623. doi: 10.1242/dev.058172
Annunziata R., Martinez P., Arnone M. I. (2013). Intact cluster and chordate-like expression of ParaHox genes in a sea star. BMC Biol. 11, 68. doi: 10.1186/1741-7007-11-68
Arnone M. I., Andrikou C., Annunziata R. (2016). Echinoderm systems for gene regulatory studies in evolution and development. Curr. Opin. Genet. Dev. 39, 129–137. doi: 10.1016/j.gde.2016.05.027
Arnone M. I., Byrne M., Martinez P. (2015). “Echinodermata,” in Evolutionary developmental biology of invertebrates 6: Deuterostomia, Vienna: Springer. 1–58.
Beer A.-J., Moss C., Thorndyke M. (2001). Development of serotonin-like and SALMFamide-like immunoreactivity in the nervous system of the sea urchin psammechinus miliaris. Biol. Bull. 200, 268–280. doi: 10.2307/1543509
Bisgrove B. W., Burke R. D. (1987). Development of the nervous system of the pluteus larva of Strongylocentrotus droebachiensis. Cell Tissue Res. 248, 335–343. doi: 10.1007/BF00218200
Bishop C. D., Burke R. D. (2007). Ontogeny of the holothurian larval nervous system: evolution of larval forms. Dev. Genes Evol. 217, 585–592. doi: 10.1007/s00427-007-0169-9
Bordbar S., Anwar F., Saari N. (2011). High-value components and bioactives from sea cucumbers for functional foods–a review. Mar. Drugs 9, 1761–1805. doi: 10.3390/md9101761
Burke R. D., Angerer L. M., Elphick M. R., Humphrey G. W., Yaguchi S., Kiyama T., et al. (2006). A genomic view of the sea urchin nervous system. Dev. Biol. 300, 434–460. doi: 10.1016/j.ydbio.2006.08.007
Burke R. D., Brand D. G., Bisgrove B. W. (1986). STRUCTURE OF THE NERVOUS SYSTEM OF THE AURICULARIA LARVA OF PARASTICOPUS CALIFORNICUS. Biol. Bull. 170, 450–460. doi: 10.2307/1541854
Byrne M., Sewell M. A., Selvakumaraswamy P., Prowse T. A. (2006). The larval apical organ in the holothuroid Chiridota gigas (Apodida): inferences on evolution of the Ambulacrarian larval nervous system. Biol. Bull. 211, 95–100. doi: 10.2307/4134584
Cheatle Jarvela A. M., Yankura K. A., Hinman V. F. (2016). A gene regulatory network for apical organ neurogenesis and its spatial control in sea star embryos. Development 143, 4214–4223. doi: 10.1242/dev.134999
Clark H. L. (1913). Echinoderms from Lower California, with descriptions of new species (New York: Bulletin of the American Museum of Natural History). Available at: https://www.biodiversitylibrary.org/page/26890747.
Cocurullo M., Paganos P., Benvenuto G., Arnone M. I. (2024). Characterization of thyrotropin-releasing hormone producing neurons in sea urchin, from larva to juvenile. Front. Neurosci. 18. doi: 10.3389/fnins.2024.1378520
Costa V., Mazzola A., Vizzini S. (2014). Holothuria tubulosa Gmelin 1791 (Holothuroidea, Echinodermata) enhances organic matter recycling in Posidonia oceanica meadows. J. Exp. Mar. Biol. Ecol. 461, 226–232. doi: 10.1016/j.jembe.2014.08.008
Coulon P., Jangoux M. (1993). Feeding rate and sediment reworking by the holothuroid Holothuria tubulosa (Echinodermata) in a Mediterranean seagrass bed off Ischia Island, Italy. 201–204. doi: 10.3354/meps092201
D’Aniello E., Paganos P., Anishchenko E., D’Aniello S., Arnone M. I. (2020). Comparative neurobiology of biogenic amines in animal models in deuterostomes. Front. Ecol. Evol. 8. doi: 10.3389/fevo.2020.587036
Delroisse J., Léonet A., Alexandre H., Eeckhaut I. (2021). Intracellular pathways of holothuroid oocyte maturation induced by the thioredoxin Trx-REES. Antioxidants 10, 1201. doi: 10.3390/antiox10081201
Dereli H., ÇULHA S. T., Çulha M., Özalp B., Tekinay A. (2016). Reproduction and population structure of the sea cucumber Holothuria tubulosa in the Dardanelles Strait, Turkey. Mediterr. Mar. Sci. 17, 47–55. doi: 10.12681/mms.1360
Despalatović M., Grubelić I., Antolić B., Žuljević A. (2004). Reproductive biology of the holothurian Holothuria tubulosa (Echinodermata) in the Adriatic Sea. J. Mar. Biol. Assoc. United Kingdom 84, 409–414. doi: 10.1017/S0025315404009361h
Francour P. (1990). Dynamique de l’écosystème à Posidonia oceanica dans le parc national de Port-Cros: analyse des compartiments matte, litière, faune vagile, échinodermes et poissons Paris 6. University P. M. Curie, Paris
García-Arrarás J. E., Díaz-Miranda L., Torres I. I., File S., Jiménez L. B., Rivera-Bermudez K., et al. (1999). Regeneration of the enteric nervous system in the sea cucumber Holothuria glaberrima. J. Comp. Neurol. 406, 461–475. doi: 10.1002/(SICI)1096–9861(19990419)406:4<461::AID-CNE4>3.0.CO;2–0
Gildor T., Winter M. R., Layous M., Hijaze E., Ben-Tabou de-Leon S. (2021). The biological regulation of sea urchin larval skeletogenesis – From genes to biomineralized tissue. J. Struct. Biol. 213, 107797. doi: 10.1016/j.jsb.2021.107797
Gustato G., Villari A., Del Gaudio S., Pedata P. (1982). Ulteriori dati sulla distribuzione di Holothuria tubulosa, Holothuria polii, e Holothuria stellati nel golfo di Napoli. Bollettino della Società dei naturalisti Napoli 91, 75–88.
Hart M. W. (2002). Life history evolution and comparative developmental biology of echinoderms. Evol. Dev. 4, 62–71. doi: 10.1046/j.1525-142x.2002.01052.x
Hijaze E., Gildor T., Seidel R., Layous M., Winter M., Bertinetti L., et al. (2024). ROCK and the actomyosin network control biomineral growth and morphology during sea urchin skeletogenesis. eLife 12. doi: 10.7554/eLife.89080.4
Koukouras A., Sinis A. I., Bobori D., Kazantzidis S., Kitsos M.-S. (2007). The echinoderm (Deuterostomia) fauna of the Aegean Sea, and comparison with those of the neighbouring seas. J. Biol. Res. 7, 67–92.
Kyritsi M., Tsiolas G., Michailidou S., Koukaras K., Argiriou A. (2023). Genomic and 16S metabarcoding data of Holothuria tubulosa Gmeli. Data Brief 48, 109171. doi: 10.1016/j.dib.2023.109171
Lacalli T. C., Kelly S. J. (2002). Anterior neural centres in echinoderm bipinnaria and auricularia larvae: cell types and organization. Acta Zoologica 83, 99–110. doi: 10.1046/j.1463-6395.2002.00103.x
Léonet A., Delroisse J., Schuddinck C., Wattiez R., Jangoux M., Eeckhaut I. (2019). Thioredoxins induce oocyte maturation in holothuroids (Echinodermata). Aquaculture 510, 293–301. doi: 10.1016/j.aquaculture.2018.12.090
Mashanov V. S., García-Arrarás J. E. (2011). Gut regeneration in holothurians: A snapshot of recent developments. Biol. Bull. 221, 93–109. doi: 10.1086/BBLv221n1p93
McCauley B. S., Wright E. P., Exner C., Kitazawa C., Hinman V. F. (2012). Development of an embryonic skeletogenic mesenchyme lineage in a sea cucumber reveals the trajectory of change for the evolution of novel structures in echinoderms. EvoDevo 3, 17. doi: 10.1186/2041-9139-3-17
McClay D. R. (2011). Evolutionary crossroads in developmental biology: sea urchins. Development 138, 2639–2648. doi: 10.1242/dev.048967
Meijer L., Guerrier P. (1984). Maturation and fertilization in starfish oocytes. Int. Rev. Cytol 86, 129–196. doi: 10.1016/S0074-7696(08)60179-5
Nakajima Y., Kaneko H., Murray G., Burke R. D. (2004). Divergent patterns of neural development in larval echinoids and asteroids. Evol. Dev. 6, 95–104. doi: 10.1111/j.1525-142X.2004.04011.x
Nakano H., Murabe N., Amemiya S., Nakajima Y. (2006). Nervous system development of the sea cucumber Stichopus japonicus. Dev. Biol. 292, 205–212. doi: 10.1016/j.ydbio.2005.12.038
Obukhova A. L., Khabarova M. Y., Semenova M. N., Starunov V. V., Voronezhskaya E. E., Ivashkin E. G. (2024). Spontaneous intersibling polymorphism in the development of dopaminergic neuroendocrine cells in sea urchin larvae: impacts on the expansion of marine benthic species. Front Neurosci. 18, 1348999 doi: 10.3389/fnins.2024.1348999
Ocana A., Tocino L. S., San Martín G. (2005). Description of egg masses of three species of sedentary polychaetes of the Mediterranean Sea. Zool. baetica 16, 165–169.
Pagowski V. (2024). A description of the bat star nervous system throughout larval ontogeny. Evol. Dev. 26, e12468. doi: 10.1111/ede.12468
Pasquini V., Porcu C., Marongiu M. F., Follesa M. C., Giglioli A. A., Addis P. (2022). New insights upon the reproductive biology of the sea cucumber Holothuria tubulosa (Echinodermata, holothuroidea) in the Mediterranean: Implications for management and domestication. Front. Mar. Sci. 9, 1029147. doi: 10.3389/fmars.2022.1029147
Pennington J. T., Strathmann R. R. (1990). Consequences of the calcite skeletons of planktonic echinoderm larvae for orientation, swimming, and shape. Biol. Bull. 179, 121–133. doi: 10.2307/1541746
Perillo M., Paganos P., Mattiello T., Cocurullo M., Oliveri P., Arnone M. I. (2018). New neuronal subtypes with a “Pre-pancreatic” Signature in the sea urchin stongylocentrotus purpuratus. Front. Endocrinol. 9. doi: 10.3389/fendo.2018.00650
Perillo M., Sepe R. M., Paganos P., Toscano A., Annunziata R. (2024). Sea cucumbers: an emerging system in evo-devo. EvoDevo 15, 3. doi: 10.1186/s13227-023-00220-0
Perillo M., Swartz S. Z., Pieplow C., Wessel G. M. (2023). Molecular mechanisms of tubulogenesis revealed in the sea star hydro-vascular organ. Nat. Commun. 14, 2402. doi: 10.1038/s41467-023-37947-2
Peters-Didier J., Sewell M. A. (2019). The role of the hyaline spheres in sea cucumber metamorphosis: lipid storage via transport cells in the blastocoel. EvoDevo 10, 8. doi: 10.1186/s13227-019-0119-4
Potts W. T. (2003). The physiological function of the coelom in starfish larvae and its evolutionary implications. Physiol. Biochem. Zool 76, 771–775. doi: 10.1086/381463
Purcell S., Conand C., Uthicke S., Byrne M. (2016). Ecological roles of exploited sea cucumbers. Oceanography Mar. Biol. 54, 367–386. doi: 10.1201/9781315368597–8
Rakaj A., Fianchini A., Boncagni P., Lovatelli A., Scardi M., Cataudella S. (2018). Spawning and rearing of Holothuria tubulosa: A new candidate for aquaculture in the Mediterranean region. Aquaculture Res. 49, 557–568. doi: 10.1111/are.2018.49.issue-1
Rakaj A., Morroni L., Grosso L., Fianchini A., Pensa D., Pellegrini D., et al. (2021). Towards sea cucumbers as a new model in embryo-larval bioassays: Holothuria tubulosa as test species for the assessment of marine pollution. Sci. Total Environ. 787, 147593. doi: 10.1016/j.scitotenv.2021.147593
Ruppert E. E., Fox R. S., Barnes R. D. (2004). Invertebrate zoology: a functional evolutionary approach. 7th ed (Belmont, CA: Thomson-Brooks/Cole). Available at: http://catalog.hathitrust.org/api/volumes/oclc/53021401.html.
Schindelin J., Arganda-Carreras I., Frise E., Kaynig V., Longair M., Pietzsch T., et al. (2012). Fiji: an open-source platform for biological-image analysis. Nat. Methods 9, 676–682. doi: 10.1038/nmeth.2019
Selenka E. (1867). Beiträge zur Anatomie und Systematik der Holothurien. Z. für wissenschaftliche Zoologie 17, 291–374.
Smirnov A. (2012). System of the class holothuroidea. Paleontological J. 46. doi: 10.1134/S0031030112080126
Tahri Y., Dermeche S., Chahrour F., Bouderbala M. (2019). Thin 1791 (Echinodermata holothuroidea holothuriidae) in oran coast. Algeria. Biodivers. J. 10, 159–172. doi: 10.31396/Biodiv.Journ.
Tortonese E., Accademia nazionale italiana di, e, Unione zoologica, i (1965). Fauna d’Italia/6, Echinodermata/a cura di Enrico Tortonese (Bologna: Calderini).
Udagawa S., Ikeda T., Oguchi K., Kohtsuka H., Miura T. (2022). hydrocoel morphogenesis forming the pentaradial body plan in a sea cucumber, Apostichopus japonicus. Sci. Rep. 12, 6025. doi: 10.1038/s41598–022-09691-y
Valls A. (2004). Natural spawning observation of Holothuria tubulosa. SPC Beche-de-mer Inf. Bull. 19, 40.
Vidavsky N., Addadi S., Mahamid J., Shimoni E., Ben-Ezra D., Shpigel M., et al. (2014). Initial stages of calcium uptake and mineral deposition in sea urchin embryos. Proc. Natl. Acad. Sci. 111, 39–44. doi: 10.1073/pnas.1312833110
Winter M. R., Morgulis M., Gildor T., Cohen A. R., Ben-Tabou de-Leon S. (2021). Calcium-vesicles perform active diffusion in the sea urchin embryo during larval biomineralization. PloS Comput. Biol. 17, e1008780. doi: 10.1371/journal.pcbi.1008780
Wood N. J., Mattiello T., Rowe M. L., Ward L., Perillo M., Arnone M. I., et al. (2018). Neuropeptidergic systems in pluteus larvae of the sea urchin Strongylocentrotus purpuratus: neurochemical complexity in a “simple” nervous system. Front. Endocrinol. 9, 415674. doi: 10.3389/fendo.2018.00628
Yang H., Hamel J.-F., Mercier A., Mercier A. (2015). The Sea Cucumber Apostichopus Japonicus: History, Biology and Aquaculture (Academic Press, Cambridge: Elsevier Science & Technology). Available at: http://ebookcentral.proquest.com/lib/brown/detail.action?docID=1913804.
Young C. M., Sewell M. A., Rice A. P. (2003). Atlas of marine invertebrate larvae. Aquaculture Res. 34, 437–437. doi: 10.1046/j.1365-2109.2003.00819.x
Zheng Y., Cong X., Liu H., Wang Y., Storey K. B., Chen M. (2022). Nervous system development and neuropeptides characterization in embryo and larva: insights from a non-chordate deuterostome, the sea cucumber apostichopus japonicus. Biology 11, 1538. doi: 10.3390/biology11101538
Keywords: ossicle, nervous system, echinoderms, serotonin, gamete collection
Citation: Perillo M, Alessandro T, Toscano A and Annunziata R (2024) Larval development of Holothuria tubulosa, a new tractable system for evo-devo. Front. Ecol. Evol. 12:1409174. doi: 10.3389/fevo.2024.1409174
Received: 29 March 2024; Accepted: 06 May 2024;
Published: 24 May 2024.
Edited by:
Smadar Ben-Tabou De-Leon, University of Haifa, IsraelReviewed by:
Pedro Martinez, University of Barcelona, SpainRoman P. Kostyuchenko, Saint Petersburg State University, Russia
Copyright © 2024 Perillo, Alessandro, Toscano and Annunziata. This is an open-access article distributed under the terms of the Creative Commons Attribution License (CC BY). The use, distribution or reproduction in other forums is permitted, provided the original author(s) and the copyright owner(s) are credited and that the original publication in this journal is cited, in accordance with accepted academic practice. No use, distribution or reproduction is permitted which does not comply with these terms.
*Correspondence: Rossella Annunziata, rossella.annunziata@szn.it; Margherita Perillo, mperillo@mbl.edu