Analyses of intra-annual density fluctuation signals in Himalayan cedar trees from Himachal Pradesh, western Himalaya, India, and its relationship with apple production
- 1Birbal Sahni Institute of Palaeosciences, Lucknow, India
- 2Academy of Scientific and Innovative Research, Ghaziabad, Uttar Pradesh, India
- 3Department of Botany, Institute of Science, Banaras Hindu University, Varanasi, India
- 4Department of Geology, University of Lucknow, Lucknow, India
Intra-annual density fluctuation (IADF) refers to anatomical changes in the tree ring caused by a sudden change in wood density triggered by a combination of climate variations and various biotic and abiotic influences. To reveal the occurrence of IADFs, we analyze the growth rings of Himalayan cedar (Cedrus deodara) growing over the Kullu region, Himachal Pradesh, western Himalaya. Using 30 increment cores, we precisely dated and developed a 214-year-long tree-ring chronology extending back to AD 1808. The tree–growth–climate relationship using ring-width chronology and observed climate data revealed that cool and moist condition provides favorable condition for Himalayan cedar tree growth. Delving deeper into wood anatomy of growth rings, we revealed the frequent occurrences of IADFs in both earlywood (IADFe) and latewood (IADFl). The formation of IADFs in earlywood (IADFe) is related to the reduced precipitation from April to July, causing moisture stress in the soil and surrounding climate. However, wetter conditions in the late growing season, mainly August–October, activated the formation of IADFs in latewood (IADFl). The study revealed several IADF years in earlywood and latewood, such as 1901, 1902, 1903, 1914, 1915, 1919, 1920, 1923, 1925, 1943, 1958, 1959 and 1937, 1955, 1956, 1988, respectively. These IADF years corresponded with unusual climatic fluctuations that severely affected apple production, the major cash crop in the region. The analyses demonstrated that the IADF chronology of Himalayan cedar would be a valuable proxy to understand abrupt and unusual climatic fluctuations from a long-term perspective for the data-scarce western Himalayan region.
1 Introduction
In the late 1400s, Leonardo da Vinci noticed that the number of rings in the tree’s branches designates their age (translation of Leonardo da Vinci’s Treatise on Painting) and tree-ring width is associated with the climate conditions during the year of their formation (Stallings, 1937; Sarton, 1954; Corona, 1986). Furthermore, cross-dating was initially employed by French naturalists Duhamel and Buffon to pinpoint the 1709 frost ring in the samples collected in 1737 (Dean, 1978; Webb, 1986). In 1827, Twining played a crucial role in bringing the process to light (Dean, 1978), while Babbage notably elaborated on it in 1838 (Babbage, 1838), consistently championing its significance. Later, Douglass made significant advancements by developing the primary skeleton plotting method, and refining the cross-dating technique in 1904, which is supported by extensive testing and documentation (Webb, 1986; Nash, 1999). However, since Douglass’s (1914) dendrochronological investigations, relationships between annual tree-growth changes and climatic conditions have been recognized. Tree rings are expressed as “well-defined increments surrounding the entire tree” and are generated in response to climatic and genetic factors. Each tree ring is subsequently dated to one calendar year, the same year of tree-ring formation, and is a fundamental concept of dendrochronology. However, IADFs are characterized by the appearance of latewood-like cells in earlywood or earlywood-like cells in latewood (Fritts, 2001). These IADFs are identifiable as a layer of cells within a tree ring recognized by distinct characteristics, such as shape, size, and wall thickness (Kaennel and Schweingruber, 1995). Mostly, IADFs are also accepted as false rings (Schulman, 1938), multiple rings (Kozlowski, 1971), or intra-annual growth bands (Fritts, 1976), and they are formed in annual rings in accordance with short-term climatic variability throughout the growing season. The formation of IADFs is not limited to a specific group of trees but extends to several species, including both hardwood trees like Himalayan birch (Betula utilis) and oak (Quercus spp.) and softwood trees like Himalayan cedar (Cedrus deodara), strawberry tree (Arbutus unedo), and Aleppo pine (Pinus halepensis) in different climates globally (Cherubini et al., 2003; De Micco et al., 2016). Therefore, IADFs comprise an essential tool to examine and evaluate intra-annual changes in climatic factors, giving enormous seasonal level clues worldwide (Copenheaver et al., 2006; De Luis et al., 2007; Battipaglia et al., 2010; Edmondson, 2010; Olivar et al., 2012; Gao et al., 2021; Tucker et al., 2022). The formation of IADF reflects tree’s ability to modify wood anatomical characters to temporary fluctuation in climatic conditions. This adaptation helps them balance hydraulic efficiency and protection instead of embolism throughout wet and dry spells, respectively (De Micco and Aronne, 2009; Wilkinson et al., 2015). IADFs have been occasionally linked to disruptions or reductions in photosynthesis due to environmental limitations, including severe aridity, frost events, and defoliation resulting from fire or pathogen attacks (De Micco et al., 2007; Schweingruber, 2007). However, precipitation and temperature are the most commonly examined factors when studying the occurrence of IADF, as they play a crucial role in interpreting the ecological significance of IADF formation (De Micco et al., 2016). Besides the climate variables, IADF can offer valuable insights into climate modeling, such as analyzing climate variations at a seasonal temporal scale and comparing modeled climate data with observed IADF patterns, which can assess the accuracy of model outputs and make necessary adjustments. Using IADFs in climate modeling contributes to our understanding of short-term climate variability, enhance our capacity to predict and adapt to changing environmental conditions.
Globally, the occurrence of IADF is known to exhibit variability based on several tree-related factors, such as tree’s age, sex, size, and width of tree ring (Rigling et al., 2001; Wimmer, 2002; Campelo et al., 2007, 2013; De Luis et al., 2009; Vieira et al., 2009; Olano et al., 2015). According to Vogel et al. (2001), the formation of false rings is also more likely to occur in younger trees and trees that grow faster. The increased likelihood of IADFs in juvenile tree rings can be attributed to the early reactivation of the cambium resulting in an extended growing season, along with a rapid physiological and morphological response to environmental alterations (Villalba and Veblen, 1996; Vieira et al., 2009). However, younger trees with shallower root systems may also be more vulnerable to environmental changes, which can lead to the production of the IADF in response to changes in water availability (Ehleringer and Dawson, 1992; Battipaglia et al., 2014). Pacheco et al. (2016) discovered the link between a higher IADF frequency in tree rings and a shallower root system, indicating that the shallow-rooted Spanish juniper (Juniperus thurifera) in north-eastern Spain is more susceptible to summer and autumn rains than the deeper-rooted Aleppo pine (Pinus halepensis). According to the Carvalho et al. (2015) hypothesis, higher spring cell production rates contribute latewood to developing IADFs, successively increasing the number of cells undergoing enlargement following the summer drought and forming wider rings. A range of variations in the occurrence of IADF across species dispersion is often mentioned (Novak et al., 2013; Nabais et al., 2014). From the Mediterranean climate, a higher frequency of IADF in cluster pine (Pinus pinaster) was reported in younger trees than older ones (Bogino and Bravo, 2009; Vieira et al., 2009). A homogeneous age trend has been analyzed in Aleppo pine growing over the Iberian Peninsula. Specifically, the relative age and size of IADF frequency in both Aleppo pine and cluster pine species have been observed, revealing that the highest frequency of IADF is seen in trees of age ~27 years old, in the juvenile stage, and characterized by wider than narrower tree rings (Novak et al., 2013). In Spain, the occurrences of IADF in cluster pine have been conversely correlated with secondary growth rates (Bogino and Bravo, 2009). Notably, no significant relationship between IADF frequency with age and tree-ring width has been observed in young trees with age < 35 years from the wetter north-western region of Spain. In the case of Scots pine (Pinus sylvestris) growing in dry sites within the central Alps, a distinct relationship between IADF frequencies and tree age has been identified (Rigling et al., 2001, 2002). An extensive investigation of a database of cluster pine, aleppo pine, and stone pine (Pinus pinea) trees within the Mediterranean region revealed that IADFs appeared more frequently in juvenile trees with wide rings (Zalloni et al., 2016). However, a higher frequency of IADF was reported in tree rings of black spruce (Picea mariana) and jack pine (Pinus banksiana) from eastern Manitoba (Hoffer and Tardif, 2009), which showed no remarkable relationship between IADF occurrence and tree-ring width.
Moreover, the IADF-based studies carried out to understand the impact of sudden climatic changes in tree’s radial growth focusing on intra-seasonal and seasonal resolution (Priya and Bhat, 1998; Braüning, 1999; Speer et al., 2004; Copenheaver et al., 2010; Gonda-King et al., 2012; Palakit et al., 2012; De Micco et al., 2014; Campelo et al., 2015; Ren et al., 2015). The western Himalayan region has not yet received much attention on IADF research, primarily focusing on the high-latitude temperate and Mediterranean regions. In India, tree-ring studies have predominantly confined to the application of ring-width chronologies in climatic reconstructions (Singh et al., 2009; Yadav et al., 2014, 2015, 2017; Misra et al., 2015, 2021; Yadava et al., 2016, 2021; Shekhar et al., 2017; Shah et al., 2019; Singh et al., 2021, 2022). However, few studies have been carried out on wood density (Hughes and Davies, 1987; Hughes, 1992, 2001; Borgaonkar et al., 2001), stable isotopes (Ramesh et al., 1985, 1986; Managave et al., 2011, 2020; Sano et al., 2017), and IADFs from northeast India (Singh et al., 2016; Thomte et al., 2022). Considering the significance of IADFs, efforts have been made to analyze the short-term intra-annual climatic changes from the western Himalaya.
2 Materials and methods
2.1 Study area and tree-ring data
Himalayan cedar (Cedrus deodara) is a long-lived conifer generally growing over steep rocky slopes with well-drained soil cover and moderate-to-heavy winter snowfall at an elevation varying from 1,200 to 3,300 masl (Raizada and Sahni, 1960; Champion and Seth, 1968). To comprehend the effect of climate on Himalayan cedar, we selected the Jari (31° 59′ 43″ N, 77° 14′ 16″ E) restricted forest area located in Parvati Valley, Kullu, Himachal Pradesh (Figure 1). The lithology around Jari village comprises carbonaceous phyllites, quartz schist with recrystallized limestone bands (overlying thrust sheet) that form a semi-klippen structure over the Manikaran Quartzite with a thrust contact (Choubey et al., 1997).
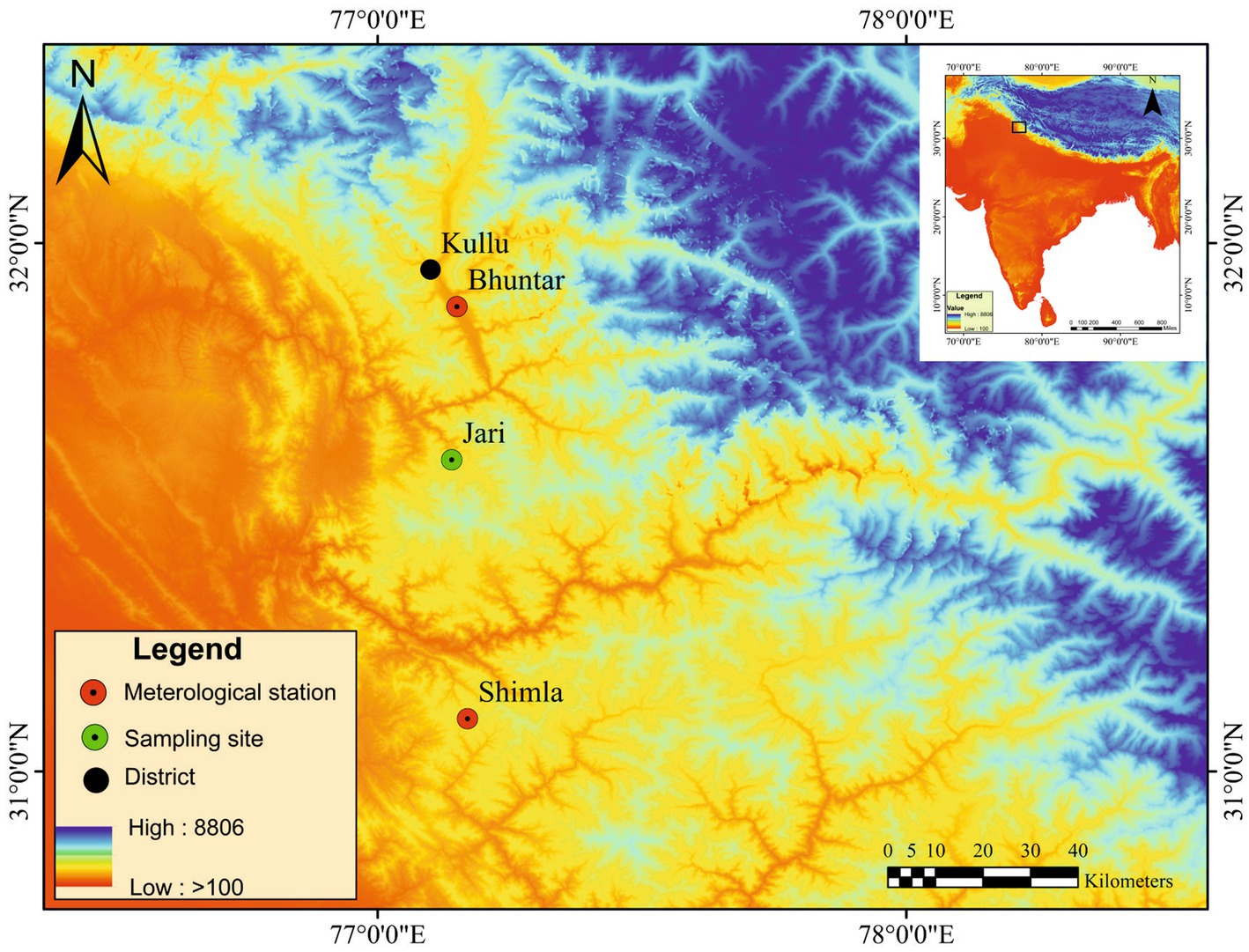
Figure 1. Location of the study area, tree-ring sampling site, and meteorological station used in the present study.
For the tree-ring sampling, healthy undisturbed trees free from any visible mark of injury were chosen and cored at breast height (~1.4 m) perpendicular to the slope. For this study, 30 core samples from 25 trees were collected during May 2022. Subsequently, the transverse surface of increment core samples was mounted on wooden frames and polished using abrasives of varying grits (220 and 400 grit) until the cellular features were discernible under the stereozoom binocular microscope. The established dendrochronological procedures were adopted for cross-dating of each series (Fritts, 1976).
Using LINTAB (Rinn, 2003) measuring machine connected to a personal computer, the ring widths of accurately dated increment core samples were measured with a resolution of 0.01 mm. The dating quality control program COFECHA demonstrated robust coherence in the growth patterns of the trees. It showed a high correlation among the radii, n = 30 (mean r = 0.84), indicating common climate factors affecting tree growth over the site (Holmes, 1983). The statistical program ARSTAN (Cook, 1985) was used to develop tree-ring chronology and removed the juvenile growth trend and other noises in the samples by curve fitting. A 67% cubic smoothing spline with a 50% frequency response cutoff was applied to detrend the ring-width data series. To stabilize the variation in each series, the tree-ring-width series were power transformed before being detrended (Cook and Peters, 1997). Detrending was accomplished by biweight robust mean computed (Cook, 1985), and mean chronology was developed by merging all standardized series. The resulting 214-year-long ring-width chronology (AD 1808–2021) of Himalayan cedar was developed from Kullu, Himachal Pradesh (Figure 2). A sufficient number of increment cores were used with a threshold value of expressed population signal (EPS) > 0.85 from AD 1855, which reflects the validation of chronology for analyses (Figure 2). The chronology statistics, such as mean sensitivity, standard deviation, and first-order autocorrelation, showed strong signals of interannual climatic variations (Table 1).
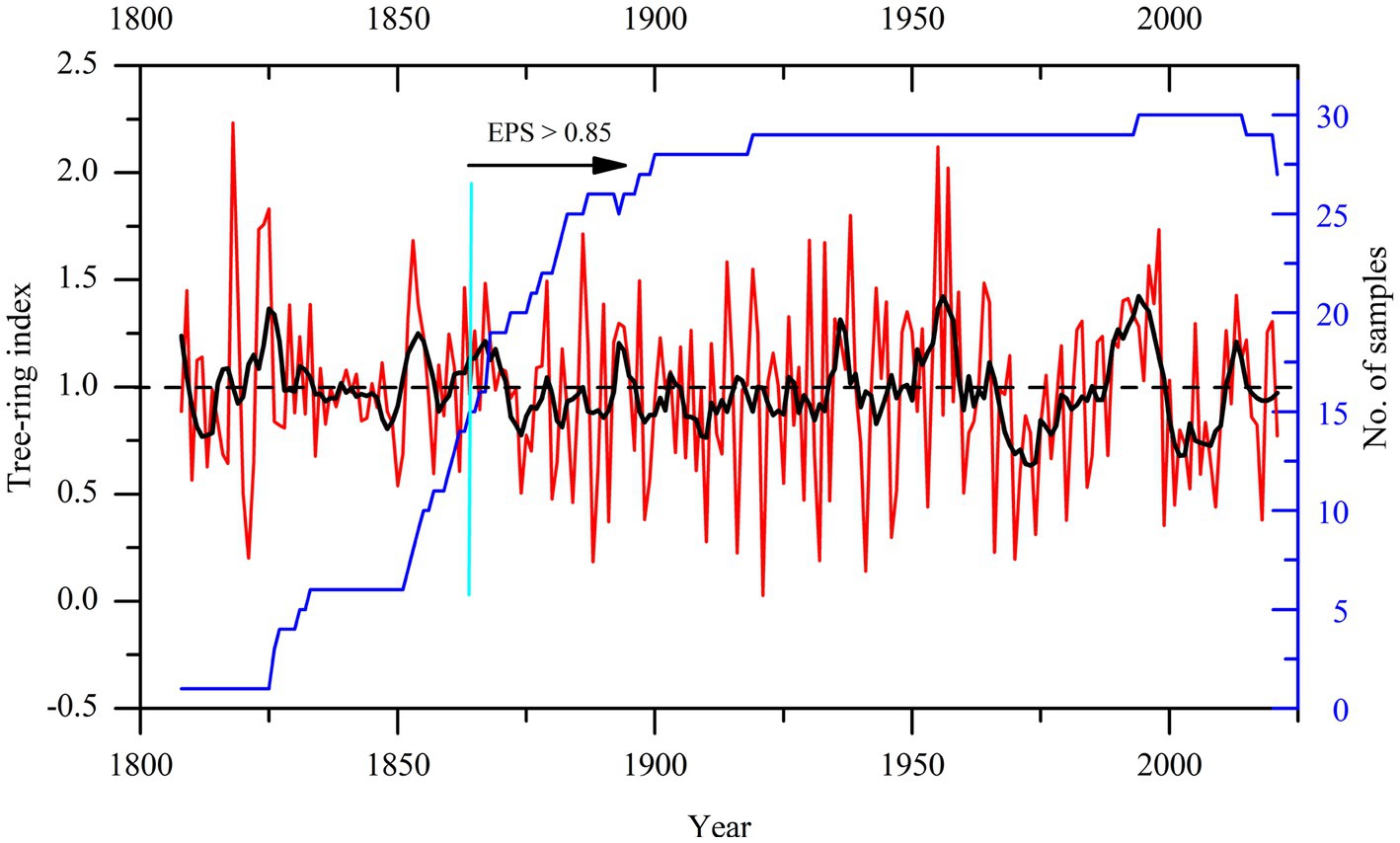
Figure 2. Himalayan cedar tree-ring chronology developed from Kullu, Himachal Pradesh (AD 1808–2021), with the number of samples used in the development of chronology.
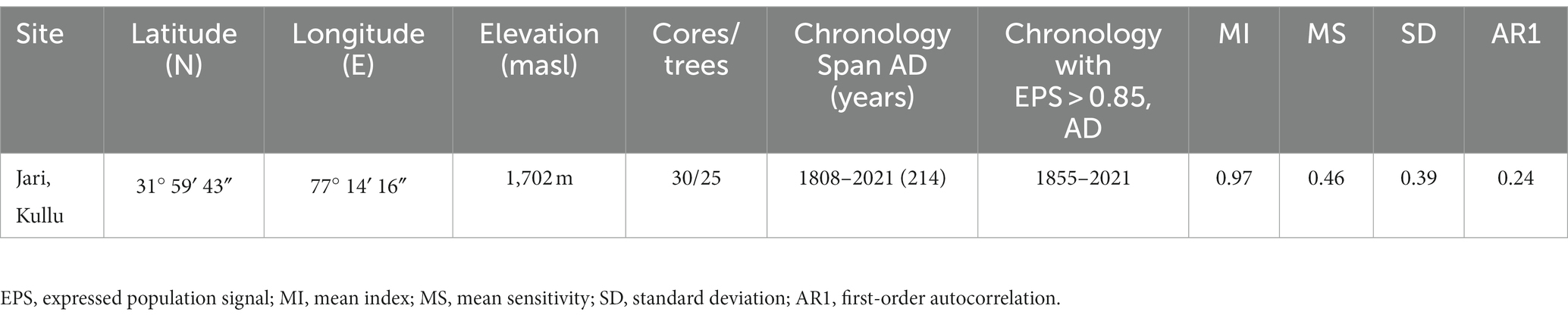
Table 1. Himalayan cedar tree-ring chronology statistics developed from Jari, Kullu, Himachal Pradesh, India (1808–2021).
2.2 Climate signal in ring-width chronology
The climate over the orography-dominated Himalayan region varies from valley to valley or within a very short distance in the valley. Meteorological data for the western Himalayan region are geographically erratic and restricted to short duration (Singh et al., 2021, 2022). For the present study, monthly precipitation data (1901–2010) from Bhuntar and mean monthly temperature data (1876–1998) from Shimla were selected for the analyses (Figure 3). Bootstrap correlation analysis was performed using the program DENDROCLIM2002 (Biondi and Waikul, 2004) for the tree–growth–climate relationship (Figure 4). This analysis using residual chronology specified that the radial growth of the Himalayan cedar has a direct correlation with precipitation throughout the year except for June and August.
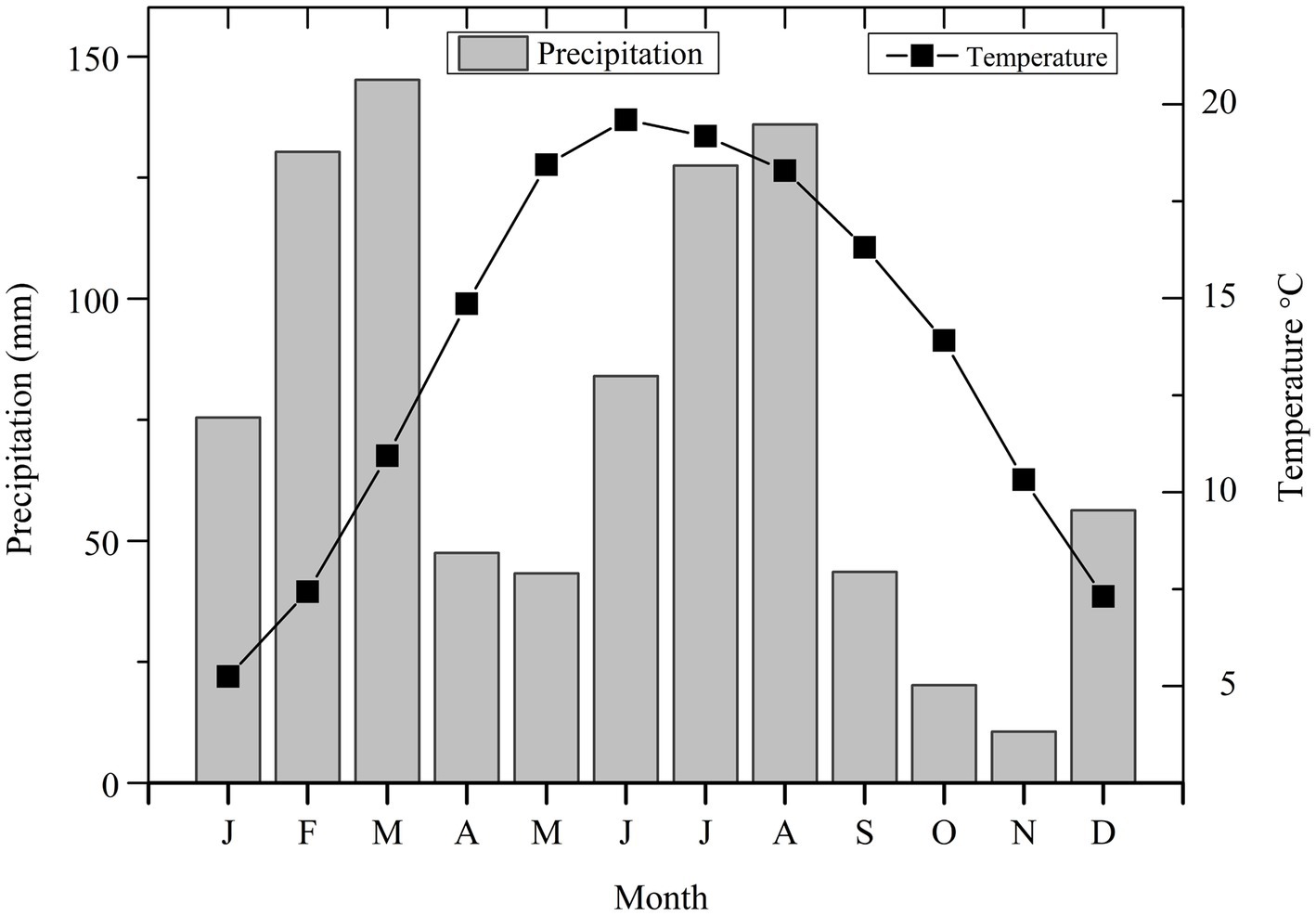
Figure 3. Recorded monthly variation in precipitation from Bhuntar and mean temperature from Shimla of Himachal Pradesh.
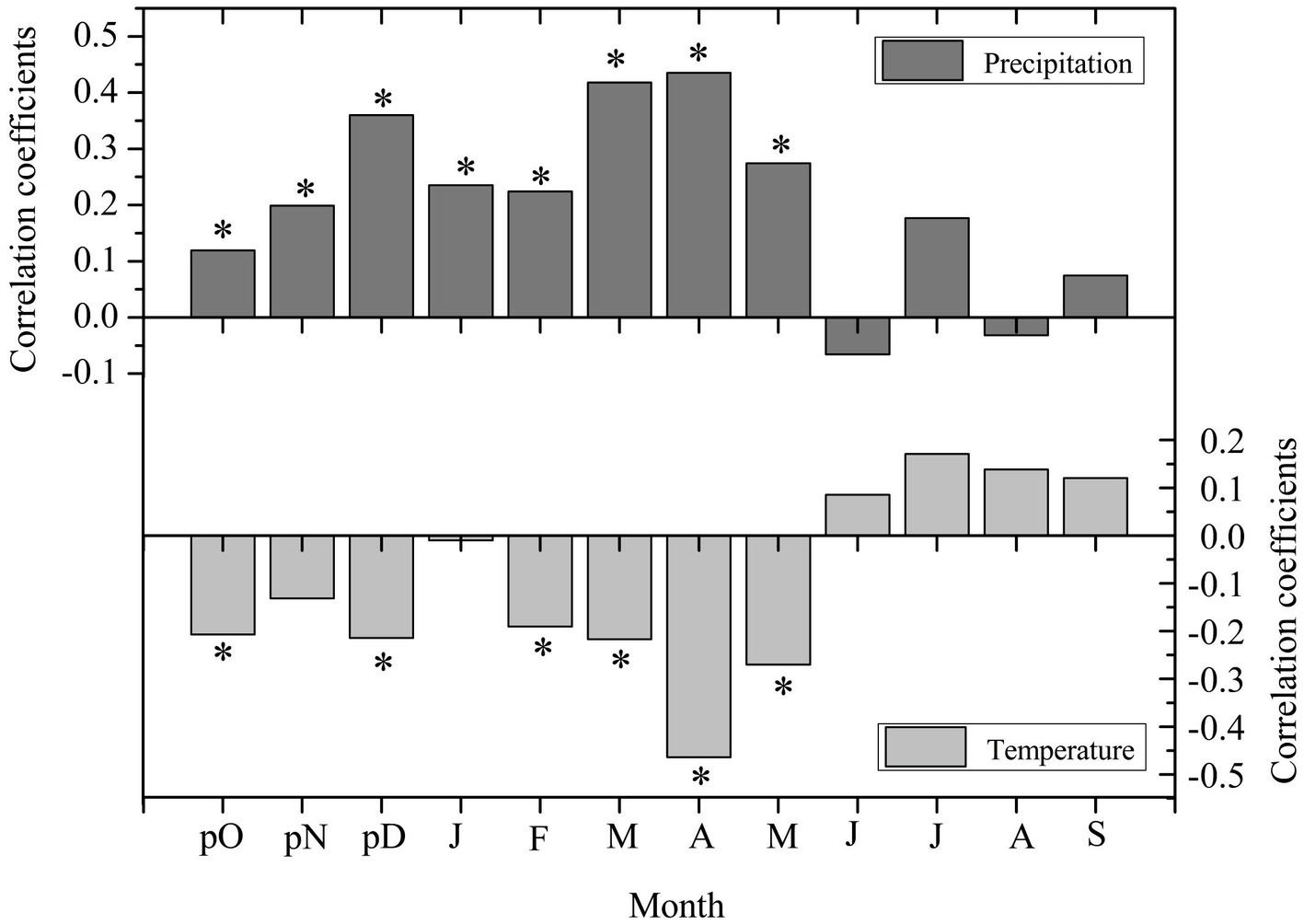
Figure 4. Bootstrap correlation between ring-width chronology and climate variables (Precipitation and temperature). *Represents correlation values significant at a 95% confidence level.
From the previous year November to the current year May month’s precipitation plays a significant positive role in the Himalayan cedar growth. Analyses with temperature data showed an inverse relationship with tree growth during the whole year except for the monsoon months (July–September). Current-year February–May are the significant months for tree growth. However, an increase in the February–May temperature initiates high evapotranspiration during these months, resulting slowdown in the tree growth. Overall correlation analyses showed that cool and wet conditions favor the Himalayan cedar tree growth over the study site.
2.3 Cambium activity and growth ring in Himalayan cedar
The Himalayan cedar is a conifer tree species native to Himalaya, thrives across a range extending from Afghanistan to Garhwal Himalaya, and is also found in patches within the Kumaun Himalaya. Sub-tropical to temperate climate in a monsoon and monsoon shadow region of western Himalaya provides favorable conditions for its growth. Most trees and woody plants possess a thin tissue layer known as the cambium, located between the xylem and phloem that develops new cells and is responsible for secondary growth (increase in radial growth; Wang et al., 2021). In temperate climates, cambium exhibits a seasonal rhythm of activity responsible for the xylem formation throughout a prolonged growing period from March to November. In the winter, cambium activity goes dormant from December to February. During the growing season, cells are larger and lighter in color; on the other hand, in autumn, cells become smaller and darker in color.
2.4 Intra-annual density fluctuations
This study involved precisely dated growth ring sequences with Intra-annual density fluctuation (IADF) in cores under a stereozoom binocular microscope with magnification capabilities of up to 50×. IADFs are observed as thin bands of tracheids with thick walls (resembling latewood) within the earlywood portion, which is close to both sides by tracheids with thinner walls and larger diameters (Singh et al., 2016). On the other hand, the IADFs in the latewood portion are thin-walled tracheids (earlywood-like) neighboring on both sides by thicker walled, smaller diameter latewood tracheids (Singh et al., 2016). Their precise location within the ring may serve as a robust proxy of the climatic variables on the resolution of the intra-seasonal level, as the formation of IADFs takes place by climatic variation during the growing season. In the present study, IADFs were recorded in trees when both cores from a tree contained an IADF in an annual ring. However, when only one core from a tree was present, the IADFs in the associated rings were considered, with the single core representing one tree. With precisely dated increment core samples, the percentage (F) and occurrence of IADFs in growth rings of samples for respective years were calculated as ratios: F = 100* n/N where n is the number of trees that developed IADF in a particular year in early/latewood along with N is the total number of trees in that specific year.
3 Results
3.1 Correlation between seasonalized precipitation and tree-ring index
Tree growth and climate relationship using residual chronology specified that the radial growth of Himalayan cedar has a significant positive correlation with the monthly precipitation, especially March–April–May (MAM). Specifically, the correlation coefficient (r) was calculated at 0.62, spanning the years from 1901 to 2010. This correlation coefficient value indicates a relatively strong and consistent relationship between MAM precipitation and tree-ring growth (Figure 5). The observed positive correlation between the ring-width chronology and monthly precipitation can likely be attributed to the increased soil moisture content resulting from snow melting, which accumulates during winter. When the snow begins to melt, it effectively acts as a slow-release fertilizer, gradually providing essential nitrogen to the trees. This nitrogen is thought to be attached to snowflakes as they fall through the atmosphere, and when the snow accumulates on the ground and then melts, it releases this nitrogen into the soil. As a result, the trees experience improved growth conditions due to the enhanced soil moisture and the gradual nitrogen supply.
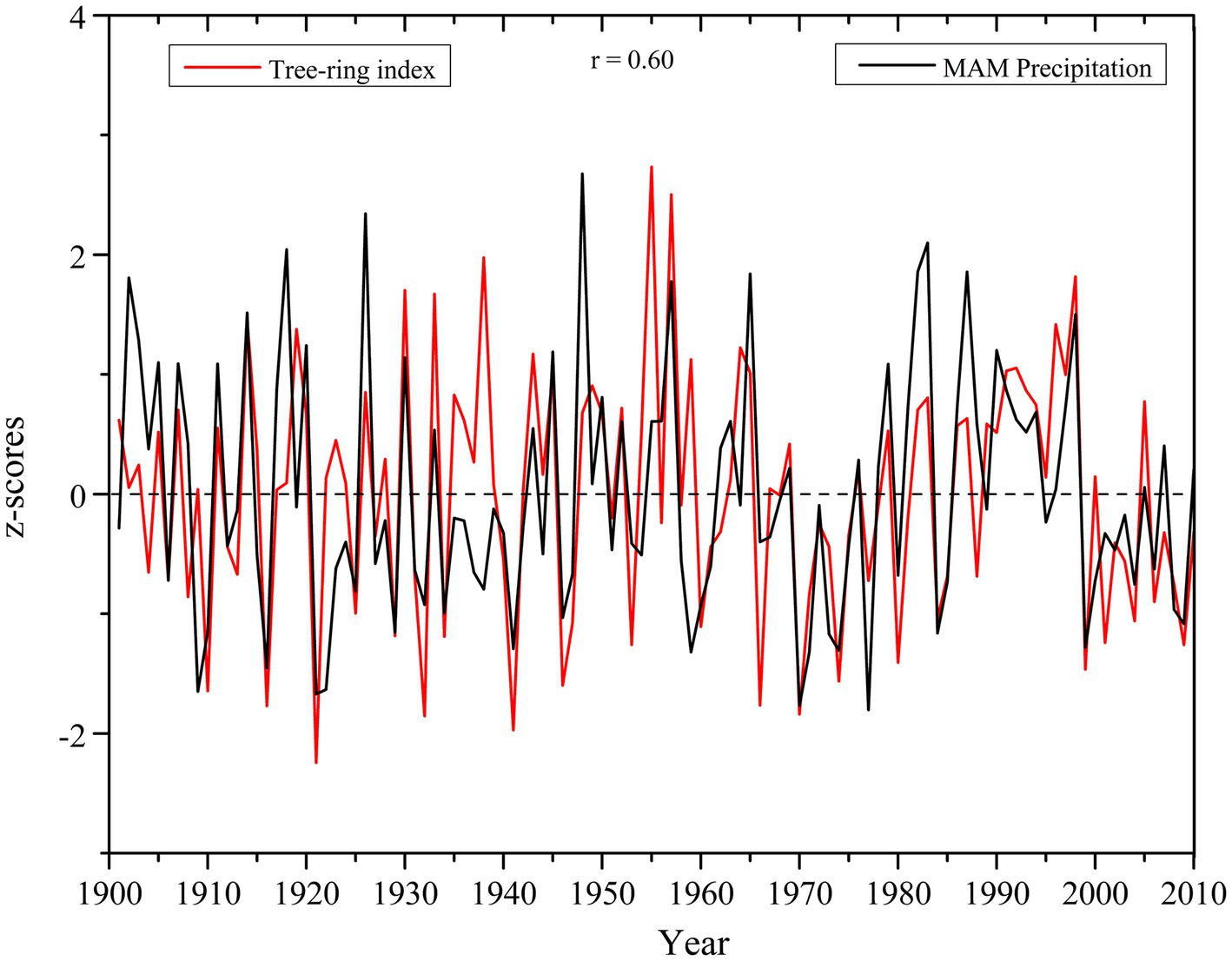
Figure 5. Ring-width chronology and seasonalized March–April–May (MAM) precipitation plotted together to show the relationship. The two series showed Pearson correlation of 0.62 (1901–2010, two-tailed p = 0.01).
3.2 Relationship between IADF frequency and tree age
A variation in the timing and length of xylem development may account for the age-dependent IADF frequency. In our study, when we examined the relationship between the frequency of IADF and the age of Himalayan cedar trees, we observed that there was a higher incidence of IADF during the juvenile stage of the trees, which typically falls within the age range of ~50–150 years (Figure 6). Interestingly, we also noted that the highest occurrences of IADF were predominantly found within the wider growth rings of the trees, as depicted in Figure 7. This suggests that IADF is more prevalent and pronounced in the earlier years of the tree’s life, particularly in the broader annual growth rings.
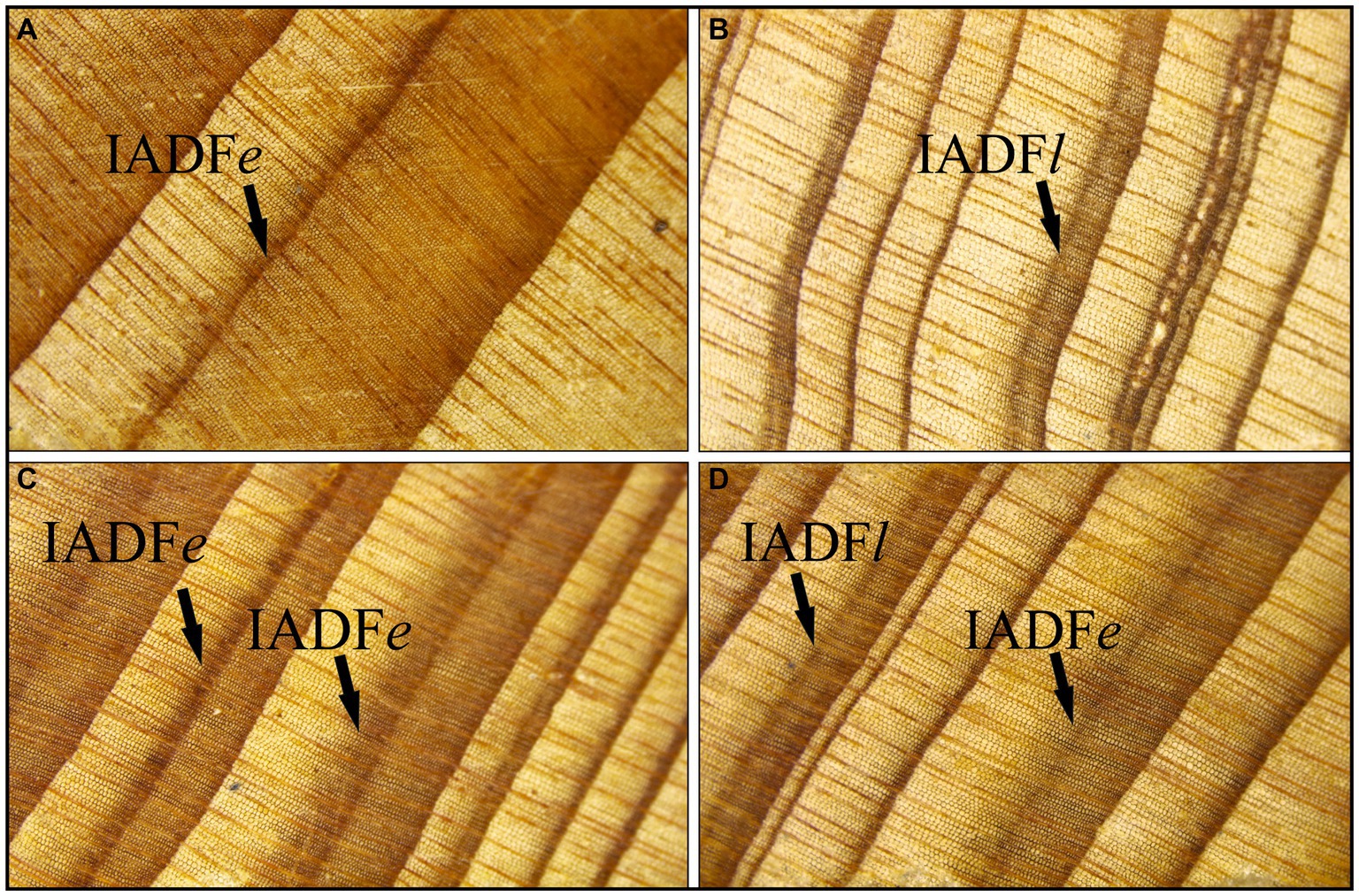
Figure 7. Images showing the intra-annual density fluctuations (IADFs); (A) IADF in earlywood, (B) two successive IADFs in earlywood, (C) IADFs in latewood, (D) IADFs in both early and latewood.
3.3 Climatic implications of IADFs
In investigation, we observed IADFs within the annual growth rings of Himalayan cedar. It is intriguing that these IADFs were not confined to a specific portion of the growth ring but rather appeared consistently in both the earlywood and latewood. This uniform presence suggests a similarity in moisture fluctuation patterns throughout the growing season, as visually represented in Figure 7. To shed light on the factors responsible for forming these IADFs, we conducted a thorough analysis of climate data for the years that exhibited a high incidence of IADFs. This approach aimed to uncover any climatic patterns or anomalies that might offer insight into the underlying causes of these density fluctuations within the annual growth rings. By examining the climatic conditions during these specific years, we sought to establish potential links between climate variations and the occurrence of IADFs, providing a deeper understanding of the ecological and environmental drivers of this phenomenon.
4 Discussion
4.1 Climatic interpretation of IADFs in earlywood
IADFs in earlywood (IADFe) are characterized by thin-lumen, thick-walled tracheids that are bordered on both sides by large-diameter, thin-walled tracheids typical of earlywood.
These IADFe tracheids are due to lack of soil moisture throughout the early growing period (Kuo and McGinnes, 1973). Our analysis showed that the IADFs in earlywood were more frequent than in the latewood of Himalayan cedar tree rings growing in Kullu, Himachal Pradesh (Figure 8). Due to inequality in the length of climate data, a common period for precipitation and temperature from 1901 to 1998 was taken, and IADF investigation restricted between them. IADF chronology showed major occurrences of IADFe in growth rings of a threshold value of 40% has been taken. The 40% to above IADFe years are 1901, 1902, 1903, 1914, 1915, 1919, 1920, 1923, 1925, 1943, 1958, and 1959 (Figure 9).
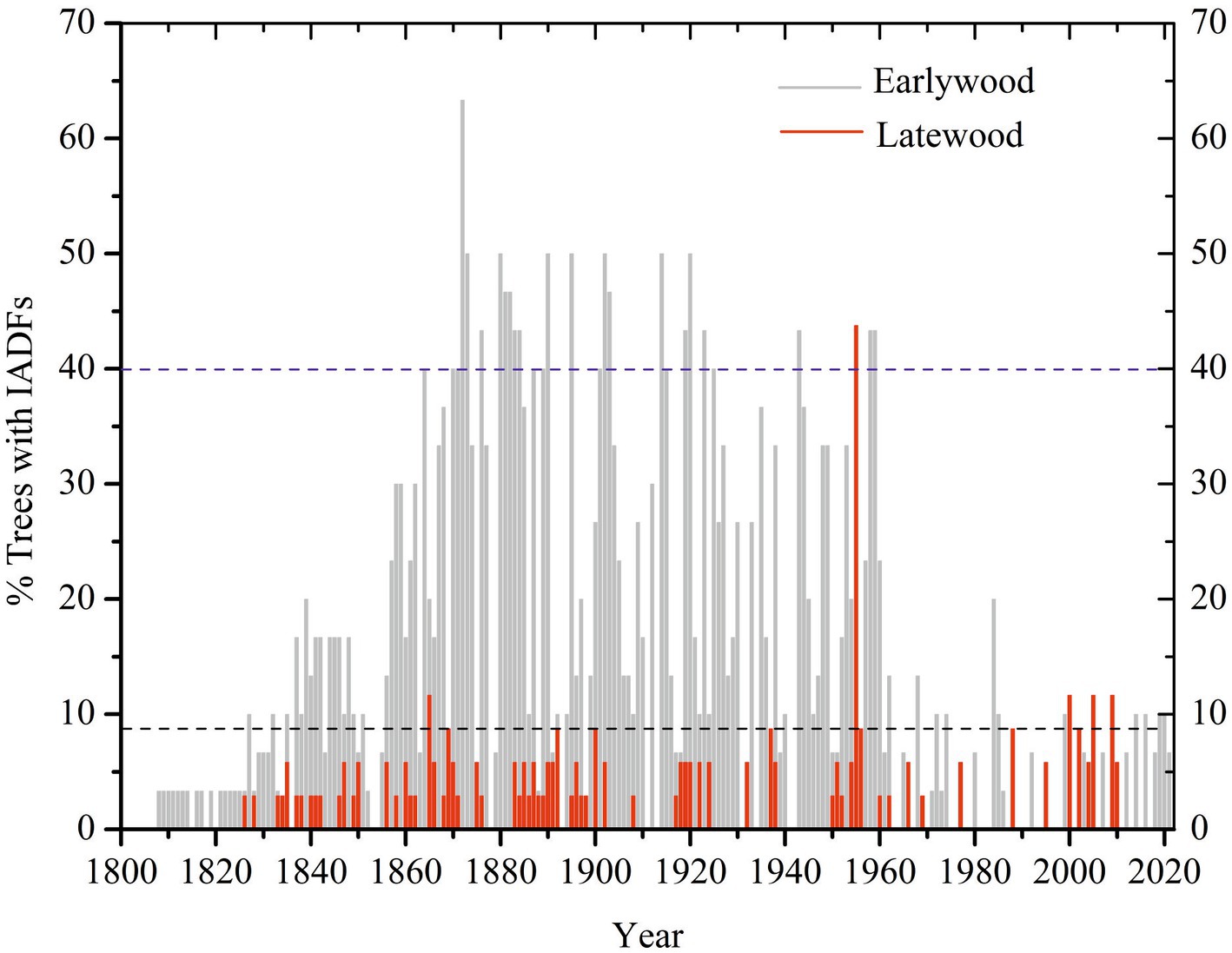
Figure 8. Earlywood and latewood IADF chronology of Himalayan cedar trees from Kullu, Himachal Pradesh.
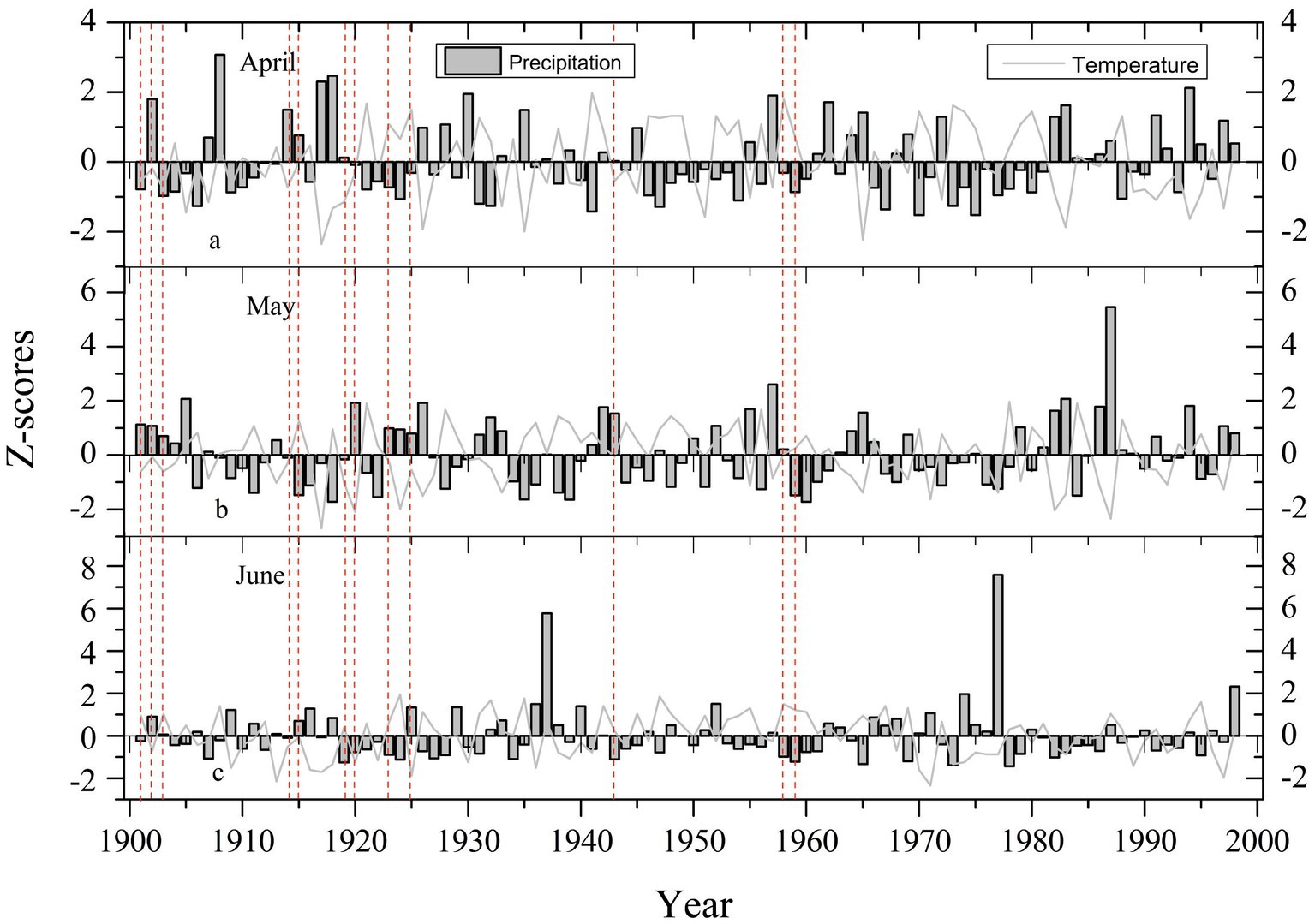
Figure 9. Z-scores of climatic variables (precipitation and temperature) for the period of 1901–1998. The red vertical dotted lines represent the years of intense IADF development in earlywood.
Monthly precipitation data from Bhuntar indicated that during the year 1901, precipitation depicted in the months of April was only 39.1 mm, which was very low as compared with the 1901–1998 mean of 80 mm for April month, causing the moisture scarcity and formation of IADFe. Successively, in the year 1902, winter precipitation during January (15.2 mm) and February (28.7 mm) was meager as compared with the 1901–1998 mean of January 106 mm and February 114 mm, causing soil moisture deficiency in the growing period, and formation of IADFs takes place. However, high temperature in January (7.5°C) and February (8°C) compared with the 1901–1998 mean of January (5.3°C) and February (6.68°C) triggered snow melting before the vegetation period and moisture scarcity in upcoming months. Like April 1901, precipitation depicted in April at only 29.2 mm significantly deviated from the 1901–1998 mean of 80 mm for April month, causing the moisture scarcity in 1903 to form IDAFe. Only 26.4 mm of precipitation in January 1914 and 8.1 mm in May 1915 were the influential factors for IADFe in 1914 and 1915, respectively. In 1915, May temperature (20.6°C) was much higher compared with the 1901–1998 mean of 18.51°C, which increased evapotranspiration and formation of IADFs in earlywood. Notably, 1915 was marked by a moderate annual drought in Una, Keylong, and Kilba as reported by Chandel and Brar (2013). Premonsoon precipitation failure in June 1919, 1920, and 1923, only 6.9, 25.9, and 21.1 mm, respectively, that was very low compared to the 1901–1998 mean of 53 mm for June caused the formation of IADFe in the respective years. Increased June temperatures for 1919 (20.2°C) and 1923 (20.5) were higher than the 1901–1998 mean 19.7°C of June. Moderate annual drought years were recorded in the year 1915 from Una, Keylong, and Kilba; in the year 1919, drought conditions were reported from Kilba and in the year 1920 from Una and Dehra Gopipur of Himachal Pradesh (Chandel and Brar, 2013). For the year 1919, on average, 43.66 mm of June month precipitation was below the average of 90.26 mm (for the period 1901–2002) in the whole of Himachal Pradesh state (Randhawa et al., 2015). Yadav et al. (2015) also reported a 5-year mean SPI of May (SPI7-May) analysis, which revealed drought conditions in 1920–1924 from the Kumaun Himalaya.
Precipitation during January–April (182 mm) was 2.4 times less than the 1901–1998 mean of January–April (436 mm), causing the moisture deficiency in the growing period in the year 1925. However, the increased April temperature (17.2°C) was much higher than the 1901–1998 mean of April (14.7°C), triggering the formation of IADFe. According to Randhawa et al. (2015), in the year 1925, January–May precipitation in the whole state was 69.08 mm compared to the previous year’s January–May month precipitation of 157.5 mm. In 1925, a La Nina phase was observed in winter and spring, which triggered the scarcity of rainfall in this region NOAA Physical Sciences Laboratory (n.d.). Precipitation during June months in the years 1943 (12 mm) and 1958 (17 mm) was meager as compared to the 1901–1998 mean of 53 mm; and in 1959, May month precipitation of 7.8 mm was also very low as compared to 1901–1998 mean for May 56.6 mm. For the years 1958 and 1959, June month temperature was too high as compared to the average of 19.7°C for 1901–1998, causing increased evapotranspiration and moisture deficiency in early monsoon month and annual high mean temperature anomaly recorded in the year 1958–1959 in comparison with the previous year 1957 (Anonymous, 2022). According to the NOAA Physical Science Laboratory (PSL), the years 1958 and 1959 were hit by a strong El Niño Southern Oscillation (ENSO) incident that caused the failure of monsoonal rainfall.
4.2 Climatic interpretation of IADFs in latewood
The IADFs in latewood (IADFl) are characterized as large thin-wall tracheids in latewood due to the sudden increase in soil moisture throughout the late growing season (Uggla et al., 2001). To investigate, the triggering climatic factor for IADFl was analyzed and a threshold value of up to 8% or more was observed in the chronology (Figure 10). The years 1937, 1955, 1956, and 1988 were the years in which IADFl was formed. Precipitation in September 1937 was 2.6 times higher than the 1901–1998 mean of September (73 mm), causing sudden moisture availability during the closing phase of the tree ring and formation of IADFl. Similarly, heavy precipitation during the month of October 1955 (346.7 mm) and 1956 (151.6 mm) was very high as compared to the 1901–1998 mean of October (30.1 mm). Increased moisture content in the month of October for two consecutive years (1955 and 1956) lowered the temperature to 13.6°C than the average range 1901–1998 for October (14.58°C). The year 1955 was reported as a year of surplus rainfall in the Chamba, Hamirpur, Mandi, and Una districts (Anonymous, 2017). On 4–6 October 1955, a massive flood destroyed the flood embankments of the Bambanwala–Ravi–Bedian–Dipalpur link canal in the Ravi basin (Groot et al., 2018). Again, in September 1988, heavy precipitation (260.4 mm) compared to August (130.2 mm) triggered the formation of IADFl. In September 1988, a cloud burst created flash flood conditions, causing massive landslide incidents along the eastern slope of Soldan Khad. The 20-m bridge on Soldan Khad washed away, and a 2-km-long road leading to Ponda was destroyed. The sudden flash flood destroyed agricultural land, houses, and apple trees and killed 32 people. Furthermore, the landslide-induced blockage temporarily halted the Satluj River flow, creating a temporary lake dimension roughly 6 km long (Anonymous, 2017). However, in the same year, excessive rainfall was reported in the Sirmour, Chamba, Hamirpur, Mandi, and Una districts of Himachal Pradesh (Anonymous, 2017).
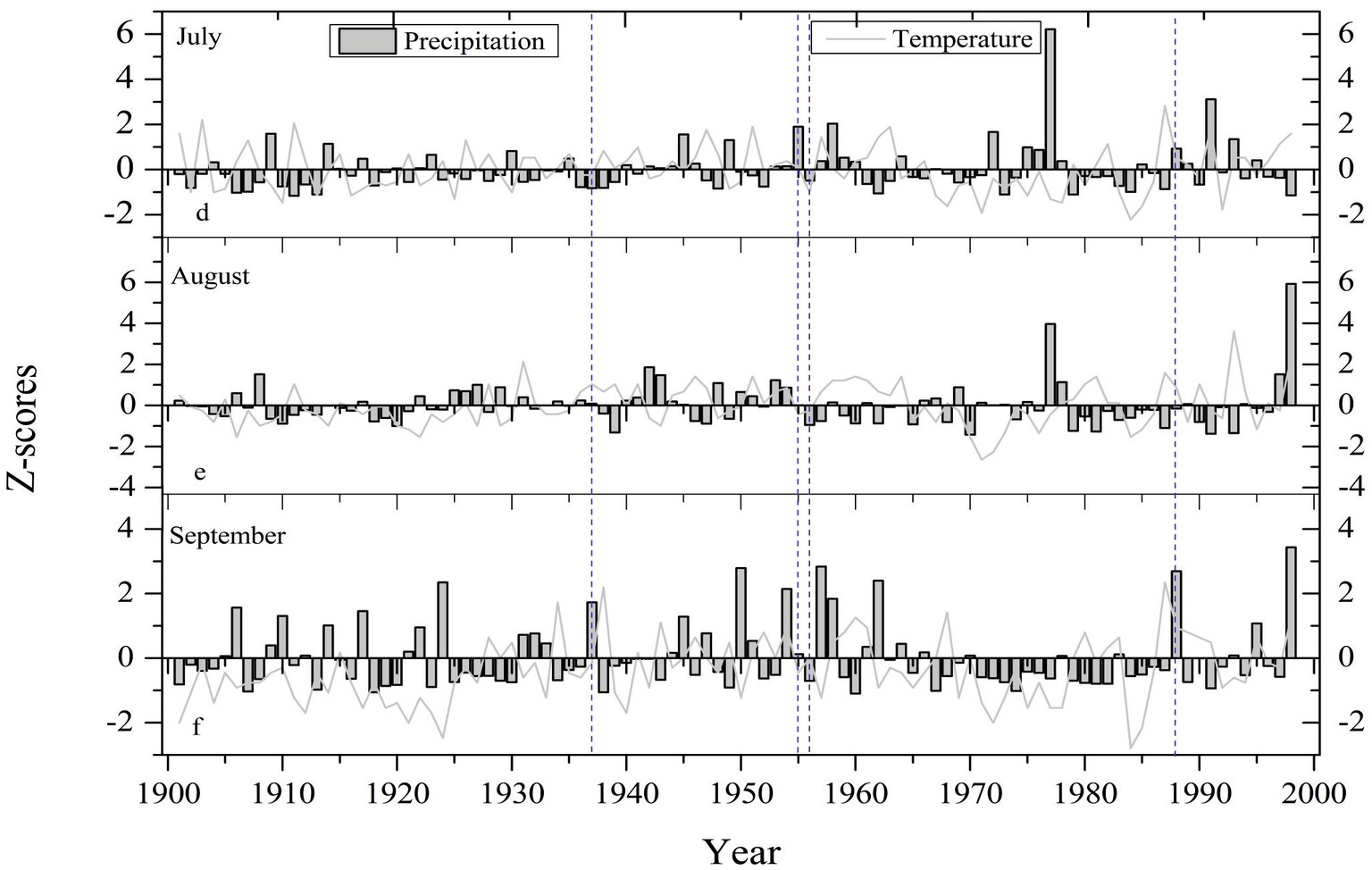
Figure 10. Z-scores of climatic variables (precipitation and temperature) data for the period of 1901–1998. The blue vertical dotted lines are the years of the intense formation of IADFs in latewood.
4.3 Socioeconomic relevance of IADF chronology
Apple is the state’s main fruit crop, which has recently overtaken other fruit harvests as the most valuable cash crop. After Jammu and Kashmir, Himachal Pradesh is India’s second-largest apple-producing state (Wani and Songara, 2018). Apple tree belongs to the “Rosaceae” family and the “Malus” genus. Apple can be cultivated at 1,500 to 2,700 masl (National Horticulture Board, 2012). The climate plays a crucial role in influencing apple fruit growth, and a typical apple orchard needs between 21°C and 24°C temperature during the growing season and 100–130 cm of uniform annual rainfall. However, extremely cold temperatures could also harm the apple crop (National Horticulture Board, 2012).
To analyze the socioeconomic relevance of IADFs chronology of Himalayan cedar growing over Kullu, Himachal Pradesh, we studied the variability in apple production data available from 1973 to 2015 (Singh et al., 2012; Randhawa et al., n.d.). In Himachal Pradesh, the apple crop set up approximately 49% of the total area, giving lucrative employment to millions of people. The apple production data of Kullu (1973–2015) showed that apple crop production was deficient at 1.43 tons/ha in the year 1974. The year 1974 featured the presence of IADFe in Himalayan cedar trees in the study area. This year, the precipitation is below the mean average for all the months. However, precipitation of February was extremely reduced to only 0.2 mm compared to the 1901–1998 average. In 1984, a deficient apple production of 2.41 tons/ha was recorded due to only 7.3 mm precipitation in May, that is, very low compared to the 1901–1998 average of 56.6 mm and failure of upcoming monsoon precipitation. Low precipitation induced dry conditions in the year 1984 was reported and about 1,497 forest fire cases identified, causing, burnt a large area of 50,364 ha in the state (Parikh et al., 2015). The year 1984 drought also triggers severe crop damage in Shimla and Kangra districts. In only Kangra district, approximately 3,100 villages were affected, and crops worth 24 crore rupees were damaged (Chandel and Brar, 2013). Low apple productivity of 3.10 tons/ha in the year 1988 was due to three times higher precipitation in September month average that caused heavy damage to apple crops due to unusually heavy and prolonged spell of rainfall from 22 to 27 September over the north-western part of India (Chandel and Brar, 2013; Punjab, Himachal Pradesh, and Jammu and Kashmir) with an exceptionally heavy rain of 30–50 cm/day. This surplus spell initiated a catastrophic flood in Punjab (Prasad, 1992). Surplus precipitation in July 2000 was more than two times higher than the average, causing very low apple crop productivity in the year 2000, i.e., 0.38 tons/ha. Excess precipitation in July 2000 triggered flash flood conditions and washed away a large cultivated area (1887.50 bighas) in Kinnaur, Kullu, and Shimla, resulting in a loss of 31.92 crores (Kumar, 2022). Meager precipitation, only 6.8 mm in April 2007, caused moisture scarcity during the early flowering season of the apple crop, causing heavy loss in apple production, i.e., 2.0 tons/ha, and the year 2007 coincided with the presence of IADF in earlywood. Early in 2007, the Himachal Pradesh region experienced a drought-like condition that severely affected the apple crop in the higher areas while also causing damage to the potato, wheat, and oil seed crops in the lower regions (Chandel and Brar, 2013). Prevailed dry conditions initiated 550 forest fire incidents and brunt approximately 8,393-ha area in the state (Parikh et al., 2015). These findings elevate the importance of IADF chronologies to understand short-term climate incidents from a socioeconomic perspective.
5 Conclusion
For the present study, a 214-year-long Himalayan cedar tree-ring-width chronology extending back to AD 1808 was developed from the Kullu, Himachal Pradesh. The Himalayan cedar trees growing over poorly porous crystalline rock surfaces provide favorable conditions to record moisture signals during precipitation events, encouraging us for the IADF study. The tree–growth–climate relationship revealed that cool and moist condition favors tree growth, and increased evapotranspiration during the growing season hampers tree growth. IADFs, which reflect short-term fluctuations in the precipitation during the growing and autumn season, offer fascinating, unusual, but varying climatic impressions from associated ring-width series. Analyses revealed sometimes successively multiyear IADF years in earlywood and latewood such as 1901, 1902, 1903, 1914, 1915, 1919, 1920, 1923, 1925, 1943, 1958, 1959 and 1937, 1955, 1956, 1988, respectively. Occurrences of IADFe during the growing season positively correlate to apple crop failure due to moisture scarcity during the flowering and fruiting period. However, the IADFl formation in autumn revealed that surplus rainfall causes heavy loss of apple crop production at the ripening time. The several low apple crop production years were 1974, 1984, 1988, 2000, and 2007 associated with IADFe/l. In 1974, only 1.3848 tons/ha of apple production occurred due to precipitation being below the mean average for all the months. However, in the year 2000, surplus precipitation in the month of July badly affected apple production up to 0.38 tons/ha. The present IADF analyses of Himalayan cedar from western Himalaya, performed for the first time, have enormous potential to study short-term climate fluctuations. Furthermore, adding an extensive network of IADF chronologies will be helpful socioeconomically in understanding climatic fluctuations more precisely over the western Himalayan region.
Data availability statement
The raw data supporting the conclusions of this article will be made available by the authors, without undue reservation.
Author contributions
RSM and KGM drafted the manuscript. RSM, KGM, and SV collected, processed, and cross-dated the tree-ring samples. RSM, KGM, SV, VS, SM, and AY evaluated the results and provided comments to improve the manuscript. All authors contributed to the article and approved the submitted version.
Acknowledgments
The authors sincerely acknowledge to Director, Birbal Sahni Institute of Palaeosciences, Lucknow, for providing the necessary facilities, support, and permission (BSIP/RDCC/05/2023-24) to publish this study. We also thank the Forest Department, Himachal Pradesh, for providing all essential assistance and logistical support during the collection of tree-ring samples. RSM was thankful to the Department of Science and Technology, New Delhi, for INSPIRE Fellowship (IF 180864, IVR No. 201800028174) and KGM to SERB-DST, New Delhi (SCP/2022/000706). We sincerely thank both the reviewers for their valuable suggestions, which greatly improved the manuscript.
Conflict of interest
The authors declare that the research was conducted in the absence of any commercial or financial relationships that could be construed as a potential conflict of interest.
Publisher’s note
All claims expressed in this article are solely those of the authors and do not necessarily represent those of their affiliated organizations, or those of the publisher, the editors and the reviewers. Any product that may be evaluated in this article, or claim that may be made by its manufacturer, is not guaranteed or endorsed by the publisher.
References
Anonymous (2017). Himachal Pradesh State Disaster Management Plan. Himachal Pradesh State Disaster Management Authority. 1–262.
Anonymous (2022). Statement on climate for the state of Himachal Pradesh: 2022. Indian Meteorological Department, Pune, 1–16.
Babbage, C. (1838). “On the age of strata, as inferred from the rings of trees embedded in them” in The ninth Bridgewater treatise, a fragment. 2nd ed (John Murray, London), 256–264.
Battipaglia, G., De Micco, V., Brand, W. A., Linke, P., Aronne, G., Saurer, M., et al. (2010). Variations of vessel diameter and δ13C in false rings of Arbutus unedo L. reflect different environmental conditions. New Phytol. 188, 1099–1112. doi: 10.1111/j.1469-8137.2010.03443.x
Battipaglia, G., De Micco, V., Brand, W. A., Saurer, M., Aronne, G., Linke, P., et al. (2014). Drought impact on water use efficiency and intra-annual density fluctuations in Erica arborea on Elba (Italy). Plant Cell Environ. 37, 382–391. doi: 10.1111/pce.12160
Biondi, F., and Waikul, K. (2004). DENDROCLIM2002: a C++ program for statistical calibration of climate signals in tree-ring chronologies. Comput. Geosci. 30, 303–311. doi: 10.1016/j.cageo.2003.11.004
Bogino, S., and Bravo, F. (2009). Climate and intraannual density fluctuations in Pinus pinaster subsp. mesogeensis in Spanish woodlands. Can. J. For. Res. 39, 1557–1565. doi: 10.1139/X09-074
Borgaonkar, H. P., Rupa Kumar, K., Pant, G. B., Okada, N., Fujiwara, T., and Yamashita, K. (2001). Climatic implications of tree-ring density variations in Himalayan conifers. Palaeobotanist 50, 27–34. doi: 10.54991/jop.2001.1801
Braüning, A. (1999). Dendroclimatological potential of drought-sensitive tree stands in southern Tibet for the reconstruction of monsoonal activity. IAWA J. 20, 325–338. doi: 10.1163/22941932-90000695
Campelo, F., Nabais, C., Freitas, H., Gutierrez, E., and Cristina, N. (2007). Climatic significance of tree-ring width and intra-annual density fluctuations in Pinus pine a from a dry Mediterranean area in Portugal. Ann. For. Sci. 64, 229–238. doi: 10.1051/forest:2006107
Campelo, F., Vieira, J., Battipaglia, G., de Luis, M., Nabais, C., Freitas, H., et al. (2015). Which matters most for the formation of intra-annual density fluctuations in Pinus pinaster: age or size? Trees 29, 237–245. doi: 10.1007/s00468-014-1108-9
Campelo, F., Vieira, J., and Nabais, C. (2013). Tree-ring growth and intraannual density fluctuations of Pinus pinaster responses to climate: does size matter? Trees 27, 763–772. doi: 10.1007/s00468-012-0831-3
Carvalho, A., Nabais, C., Vieira, J., Rossi, S., and Campelo, F. (2015). Plastic response of tracheids in Pinus pinaster in a water-limited environment: adjusting lumen size instead of wall thickness. PloS One 10:136305. doi: 10.1371/journal.pone.0136305
Champion, H. G., and Seth, S. K. (1968). The revised survey of the Forest types of India, Manager of Publications, New Delhi.
Cherubini, P., Gartner, B. L., Tognetti, R., Bräker, O. U., Schoch, W., and Innes, J. L. (2003). Identification, measurement, and interpretation of tree rings in woody species from Mediterranean climates. Biol. Rev. 78, 119–148. doi: 10.1017/S1464793102006000
Choubey, V. M., Sharma, K. K., and Ramola, R. C. (1997). Geology of radon occurrence around Jari in Parvati valley, Himachal Pradesh, India. J. Environ. Radioact. 34, 139–147. doi: 10.1016/0265-931X(96)00024-0
Cook, E. R. (1985). A time series analysis approach to tree-ring standardization. Ph.D. thesis. Tucson, Arizona, USA: University of Arizona.
Cook, E. R., and Peters, K. (1997). Calculating unbiased tree-ring indices for the study of climatic and environmental change. Holocene 7, 361–370. doi: 10.1177/095968369700700314
Copenheaver, C. A., Gartner, H., Schafer, I., Vaccari, F. P., and Cherubini, P. (2010). Drought-triggered false ring formation in a Mediterranean shrub. Botany 88, 545–555. doi: 10.1139/B10-029
Copenheaver, C. A., Pokorski, E. A., Currie, J. E., and Abrams, M. D. (2006). Causation of false ring formation in Pinus banksiana: a comparison of age, canopy class, climate, and growth rate. For. Ecol. Manage. 236, 348–355. doi: 10.1016/j.foreco.2006.09.020
Corona, E. (1986). Dendrocronologia: principi e applicazioni. In: Dendrocronologia: Principi e Applicazioni. Atti del Seminario a Verona nei giorni 14–15 November 1984. Istituto Italiano di Dendrocronologia, Verona, Italy, 7–32.
Chandel, V. B. S., and Brar, K. K. (2013). Drought in Himachal Pradesh, India: A Historical-Geographical Perspective, 1901–2009. Trans. Inst. Indian Geogr. 35, 259–273. doi: 10.17501/icfow.2018.1102
De Luis, M., Gricar, J., Cufar, K., and Raventos, J. (2007). Seasonal dynamics of wood formation in Pinus halepensis from dry and semi-arid ecosystems in Spain. IAWA J. 28, 389–404. doi: 10.1163/22941932-90001651
De Luis, M., Novak, K., Cufar, K., and Raventós, J. (2009). Size mediated climate-growth relationships in Pinus halepensis and Pinus pinea. Trees 23, 1065–1073. doi: 10.1007/s00468-009-0349-5
De Micco, V., and Aronne, G. (2009). Seasonal dimorphism in wood anatomy of the Mediterranean Cistus incanus L. subsp. incanus. Trees 23, 981–989. doi: 10.1007/s00468-009-0340-1
De Micco, V., Battipaglia, G., Cherubini, P., and Aronne, G. (2014). Comparing methods to analyse anatomical features of tree rings with and without intra-annual density fluctuations (IADFs). Dendrochronologia 32, 1–6. doi: 10.1016/j.dendro.2013.06.001
De Micco, V., Campelo, F., de Luis, M., Braüning, A., Grabner, M., Battipaglia, G., et al. (2016). Formation of intra-annual-density-fluctuations in tree rings:how, when, where and why? IAWA J. 37, 232–259. doi: 10.1163/22941932-20160132
De Micco, V., Saurer, M., Aronne, G., Tognetti, R., and Cherubini, P. (2007). Variations of wood anatomy and δ13C within tree rings of coastal Pinus pinaster Ait. Showing intra-annual density fluctuations. IAWA J. 28, 61–74. doi: 10.1163/22941932-90001619
Dean, J. (1978). Tree-ring dating in archaeology. in University of Utah Anthropological Papers: Misc. Paper, edited by J. S. Jennings University of Utah Press, Salt Lake City. pp. 24 129–163.
Douglass, A. E. (1914). A method of estimating rainfall by the growth of trees. Geol. Soc. Am. Bull. 46, 321–335. doi: 10.2307/201814
Edmondson, J. R. (2010). The meteorological significance of false rings in eastern red cedar (Juniperus virginiana L) from the southern great plains USA. Tree-Ring Res. 66, 19–33. doi: 10.3959/2008-13.1
Ehleringer, J. R., and Dawson, T. E. (1992). Water-uptake by plants – perspectives from stable isotope composition. Plant Cell Environ. 15, 1073–1082. doi: 10.1111/j.1365-3040.1992.tb01657.x
Gao, J., Rossi, S., and Yang, B. (2021). Origin of intra-annual density fluctuations in a semi-arid area of northwestern China. Front. Plant Sci. 12:777753. doi: 10.3389/fpls.2021.777753
Gonda-King, L., Radville, L., and Preisser, E. L. (2012). False ring formation in eastern hemlock branches: impacts of hemlock woolly Adelaid and elongate hemlock scale. Environ. Entomol. 41, 523–531. doi: 10.1603/EN11227
Groot, A., Singh, T., Pandey, A., Gioli, G., Ahmed, B., Ishaq, S., et al. (2018). Literature review of critical climate-stress moments in the Hindu Kush Himalaya: A resource kit. HI-AWARE Resource Kit, Kathmandu.
Hoffer, M., and Tardif, J. C. (2009). False rings in jack pine and black spruce trees from eastern Manitoba as indicators of dry summers. Can. J. For. Res. 39, 1722–1736. doi: 10.1139/X09-088
Holmes, R. L. (1983). Computer-assisted quality control in tree-ring dating and measurement. Tree-Ring Res. 43, 69–78. doi: 10.4324/9781315748689-14
Hughes, M. K. (1992). “Dendroclimatic evidence from the western Himalaya” in Climate since 1500 AD. eds. R. S. Bradley and P. D. Jones (London: Routledge), 415–431.
Hughes, M. K. (2001). An improved reconstruction of summer temperature at Srinagar, Kashmir since 1660 a D, based on tree ring width and maximum latewood density of Abies pindrow (Royle) Spach. Palaeobotanist 50, 13–19.
Hughes, M. K., and Davies, A. C. (1987). “Dendrochronology in Kashmir using ring widths and densities in sub-alpine conifers” in Methods of dendrochronology: East/west approaches. eds. L. Kairiukstis, Z. Bednarz, and E. Feliksik (Laxenburg: IIASA/Polish Academy of Sciences), 163–176.
Kaennel, M., and Schweingruber, F. H. (1995). Multilingual glossary of dendrochronology. Terms and definitions in English, Spanish, Italian, Portuguese, and Russian. Swiss Federal Research Institute WSL, Birmensdorf/Haupt, Bern.
Kuo, M., and McGinnes, E. A. (1973). Variation of anatomical structure of false rings in eastern red cedar. Wood Sci. 5, 205–210.
Kumar, V. (2022). Floods and flash floods in Himachal Pradesh. J. Geogr. Nat. Disasters 12:1000252. doi: 10.35841/2167-0587.22.12.252
Managave, S. R., Sheshshayee, M. S., Ramesh, R., Borgaonkar, H. P., Shah, S. K., and Bhattacharyya, A. (2011). Response of cellulose oxygen isotope values of teak trees in differing monsoon environments to monsoon rainfall. Dendrochronologia 29, 89–97. doi: 10.1016/j.dendro.2010.05.002
Managave, S. R., Shimla, P., Yadav, R. R., Ramesh, R., and Balakrishnan, S. (2020). Contrasting centennial-scale climate variability in High Mountain Asia revealed by a tree-ring oxygen isotope record from Lahaul-Spiti. Geophys. Res. Lett. 47:e2019GL086170. doi: 10.1029/2019GL086170
Misra, K. G., Singh, V., Yadava, A. K., Misra, S., Maurya, R. S., and Vishwakarma, S. (2021). Himalayan blue pine deduced precipitation records from cold arid Lahaul-Spiti, Himachal Pradesh, India. Front. Earth Sci. 9:645959. doi: 10.3389/feart.2021.645959
Misra, K. G., Yadav, R. R., and Misra, S. (2015). Satluj river flow variations since AD 1660 based on tree-ring network of Himalayan cedar from western Himalaya, India. Quat. Int. 371, 135–143. doi: 10.1016/j.quaint.2015.01.015
Nabais, C., Campelo, F., Viera, J., and Cherubini, P. (2014). Climatic signals of tree-ring width and intra-annual density fluctuations in Pinus pinaster and Pinus pinea along a latitudinal gradient in Portugal. Forestry 87, 598–605. doi: 10.1093/forestry/cpu021
Nash, S. E. (1999). Time, trees, and prehistory: Tree-ring dating and the development of north American archaeology, 1914–1950. The University of Utah Press: Salt Lake City.
National Horticulture Board. (2012). Available at: https://nhb.gov.in/report_files/apple/APPLE.htm
Novak, K., Saz-Sánchez, M. A., Čufar, K., Raventós, J., and De Luis, M. (2013). Age, climate and intraannual density fluctuations in Pinus halepensis in Spain. IAWA J. 34, 459–474. doi: 10.1163/22941932-00000037
NOAA Physical Sciences Laboratory (n.d.). El Niño Southern Oscillation (ENSO). Available at: https://psl.noaa.gov/enso/climaterisks/years/top24enso.html
Olano, J. M., García-Cervigón, A. I., Arzac, A., and Rozas, V. (2015). Intraannual wood density fluctuations and tree-ring width patterns are sex and site-dependent in the dioecious conifer Juniperus thurifera L. Trees 29, 1341–1353. doi: 10.1007/s00468-015-1212-5
Olivar, J., Bogino, S., Spiecker, H., and Bravo, F. (2012). Climate impact of growth dynamic and intra-annual density fluctuations in Aleppo pine (Pinus halepensis) trees of different crown classes. Dendrochronologia 30, 35–47. doi: 10.1016/j.dendro.2011.06.001
Pacheco, A., Camarero, J. J., and Carrer, M. (2016). Linking wood anatomy and xylogenesis allows pinpointing of climate and drought influences on growth of coexisting conifers in continental Mediterranean climate. Tree Physiol. 36, 502–512. doi: 10.1093/treephys/tpv125
Palakit, K., Siripattanadilok, S., and Duangsathaporn, K. (2012). False ring occurrences and their identification in teak (Tectona grandis) in North-Eastern Thailand. J. Trop For Sci. 24, 387–398.
Parikh, J., Sharma, A., Singh, C., Gupta, M. K., Kaushik, A., and Dhingra, M. (2015). Final technical report: Socio economic vulnerability of Himachal Pradesh to climate change, 1–94.
Prasad, K. (1992). A diagnostic study of flood producing rainstorm of September, over Northwest India with the aid of a fine mesh numerical analysis system. Mausam 43, 71–76. doi: 10.54302/mausam.v43i1.3320
Priya, P. B., and Bhat, K. M. (1998). False ring formation in teak (Tectona grandis L f) and the influence of environmental factors. For. Ecol. Manage. 108, 215–222. doi: 10.1016/S0378-1127(98)00227-8
Raizada, M. B., and Sahni, K. C. (1960). Living Indian gymnosperms, part 1 (Cycades, Ginkgoales and Coniferales). Indian For Rec. 5, 1–150.
Ramesh, R., Bhattacharya, S. K., and Gopalan, K. (1985). Dendrochronological implications of isotope coherence in trees from Kashmir, India. Nature 317, 802–804. doi: 10.1038/317802a0
Ramesh, R., Bhattacharya, S. K., and Gopalan, K. (1986). Climatic correlations in the stable isotope records of silver fir (Abies pindrow) trees from Kashmir, India. Earth Planet. Sci. Lett. 79, 66–74. doi: 10.1016/0012-821X(86)90041-5
Randhawa, S. S., Randhawa, S., and Rai, I. (2015). Himachal Pradesh state Centre on climate change. Seasonal, Monthly and Annual Rainfall Trends in Himachal Pradesh during 1901-2002, 1–13.
Randhawa, S. S., Sharma, Y. P., Vaidya, P., Chakrabarty, N., Randhawa, S., Lata, K., et al. (n.d.). Status report: Impact of climate change assessment on horticulture sector in district Kullu Himachal Pradesh, 1–77.
Ren, P., Rossi, S., Gricar, J., Liang, E., and Cufar, K. (2015). Is precipitation a trigger for the onset of xylogenesis in Juniperus przewalskii on the north-eastern Tibetan plateau? Ann. Bot. 115, 629–639. doi: 10.1093/aob/mcu259
Rigling, A., Bräker, O., Schneiter, G., and Schweingruber, F. (2002). Intra-annual tree-ring parameters indicating differences in drought stress of Pinus sylvestris forests within the Erico-pinion in the Valais (Switzerland). Plant Ecol. 163, 105–121. doi: 10.1023/A:1020355407821
Rigling, A., Waldner, P. O., Forster, T., Bräker, O. U., and Pouttu, A. (2001). Ecological interpretation of tree-ring width and interannual density fluctuations in Pinus sylvestris on dry sites in the Central Alps and Siberia. Can. J. For. Res. 31, 18–31. doi: 10.1139/x00-126
Rinn, F. (2003). TSAP-win time series analysis and presentation for dendrochronology and related applications, version 0.53 for Microsoft windows. Rinn Tech, Heidelberg, Germany.
Sano, M., Dimri, A. P., Ramesh, R., Xu, C., Li, Z., and Nakatsuka, T. (2017). Moisture source signals preserved in a 242-year tree-ring δ18O chronology in the western Himalaya. Global Planet. Change 157, 73–82. doi: 10.1016/j.gloplacha.2017.08.009
Shah, S. K., Pandey, U., Mehrotra, N., Wiles, G. C., and Chandra, R. (2019). A winter temperature reconstruction for the Lidder Valley, Kashmir, northwest Himalaya based on tree rings of Pinus wallichiana. Climate Dynam. 53, 4059–4075. doi: 10.1007/s00382-019-04773-6
Shekhar, M., Bhardwaj, A., Singh, S., Ranhotra, P. S., Bhattacharyya, A., Pal, A. K., et al. (2017). Himalayan glaciers experienced significant mass loss during later phases of little ice age. Sci. Rep. 7, 1–14. doi: 10.1038/s41598-017-09212-2
Singh, V., Misra, K. G., Singh, A. D., Yadav, R. R., and Yadava, A. K. (2021). Little ice age revealed in tree-ring-based precipitation record from the northwest Himalaya, India. Geophys. Res. Lett. 48:e2020GL091298. doi: 10.1029/2020GL091298
Singh, V., Misra, K. G., Yadav, R. R., Yadava, A. K., Vishwakarma, S., and Maurya, R. S. (2022). High-elevation tree-ring record of 263-year summer temperature for a cold-arid region in the Western Himalaya, India. Dendrochronologia 73:125956. doi: 10.1016/j.dendro.2022.125956
Singh, R., Vaidya, C. S., Saraswat, S. P., Singh, I., and Pawan Verma, M. (2012). Impact of high-density apple plantation under horticulture in Himachal Pradesh, 1–126.
Singh, N. D., Yadav, R. R., Venugopal, N., Singh, V., Yadava, A. K., Misra, K. G., et al. (2016). Climate control on ring width and intra-annual density fluctuations in Pinus kesiya growing in a sub-tropical forest of Manipur, Northeast India. Trees 30, 1711–1721. doi: 10.1007/s00468-016-1402-9
Singh, J., Yadav, R. R., and Wilmking, M. (2009). A 694-year tree-ring based rainfall reconstruction from Himachal Pradesh, India. Climate Dynam. 33, 1149–1158. doi: 10.1007/s00382-009-0528-5
Speer, J. H., Orvis, K. H., Grissino-Mayer, H. D., Kennedy, L. M., and Horn, S. P. (2004). Assessing the dendrochronological potential of Pinus occidentalis Swartz in the cordillera central of the Dominican Republic. Holocene 14, 563–569. doi: 10.1191/0959683604hl732rp
Tucker, C. S., Pearl, J. K., Elliott, E. A., Bregy, J. C., Friedman, J. M., and Therrell, M. D. (2022). Baldcypress false ring formation linked to summer hydroclimatic extremes in the South-Eastern United States. Environ. Res. Lett. 17:114030. doi: 10.1088/1748-9326/ac9745
Thomte, L., Shah, S. K., Mehrotra, N., Bhagabati, A. K., and Saikia, A. (2022). Influence of climate on multiple tree-ring parameters of Pinus kesia from Manipur, Northeast India. Dendrochronologia 71:125906. doi: 10.1016/j.dendro.2021.125906
Uggla, C., Magel, E., Moritz, T., and Sundberg, B. (2001). Function and dynamics of auxin and carbohydrates during earlywood/latewood transition in scots pine. Plant Physiol. 125, 2029–2039. doi: 10.1104/pp.125.4.2029
Vieira, J., Campelo, F., and Nabais, C. (2009). Age-dependent responses of tree-ring growth and intra-annual density fluctuations of Pinus pinaster to Mediterranean climate. Trees 23, 257–265. doi: 10.1007/s00468-008-0273-0
Villalba, R., and Veblen, T. T. (1996). A tree-ring record of dry spring wet summer events in the forest-steppe ecotone northern Patagonia, Argentina. Tree Rings Environ Human, 107–116.
Vogel, J. C., Fuls, A., and Visser, E. (2001). Radiocarbon adjustments to the dendrochronology of a yellowwood tree. South Afr. J. Sci. 97, 164–166.
Wang, D., Chen, Y., Li, W., Li, Q., Lu, M., Zhou, G., et al. (2021). Vascular cambium: the source of wood formation. Front. Plant Sci. 12:700928. doi: 10.3389/fpls.2021.700928
Wani, F. A., and Songara, A. (2018). Status and position of apple crop in area, production, and productivity in Himachal Pradesh. Int. J. Multidiscip. Res. Dev. 5, 106–111.
Webb, G. E. (1986). Solar physics and the origins of dendrochronology. Isis 77, 291–301. doi: 10.1086/354133
Wilkinson, S., Ogee, J., Domec, J. C., Rayment, M., and Wingate, L. (2015). Biophysical modelling of intra-ring variations in tracheid features and wood density of Pinus pinaster trees exposed to seasonal droughts. Tree Physiol. 35, 305–318. doi: 10.1093/treephys/tpv010
Wimmer, R. (2002). Wood anatomical features in tree-rings as indicators of environmental change. Dendrochronologia 20, 21–36. doi: 10.1078/1125-7865-00005
Yadav, R. R., Gupta, A. K., Kotlia, B. S., Singh, V., Misra, K. G., Yadava, A. K., et al. (2017). Recent wetting and glacier expansion in the northwest Himalaya and Karakoram. Sci. Rep. 7:6139. doi: 10.1038/s41598-017-06388-5
Yadav, R. R., Misra, K. G., Kotlia, B. S., and Upreti, N. (2014). Premonsoon precipitation variability in Kumaon Himalaya, India over a perspective of ~300 years. Quat. Int. 325, 213–219. doi: 10.1016/j.quaint.2013.09.005
Yadav, R. R., Misra, K. G., Yadava, A. K., Kotlia, B. S., and Misra, S. (2015). Tree-ring footprints of drought variability in last ∼300 years over Kumaun Himalaya, India and its relationship with crop productivity. Quat. Sci. Rev. 117, 113–123. doi: 10.1016/j.quascirev.2015.04.003
Yadava, A. K., Braüning, A., Singh, J., and Yadav, R. R. (2016). Boreal spring precipitation variability in the cold arid western Himalaya during the last millennium, regional linkages, and socio-economic implications. Q. Sci. Rev. 144, 28–43. doi: 10.1016/j.quascirev.2016.05.008
Yadava, A. K., Misra, K. G., Singh, V., Misra, S., Sharma, Y. K., and Kotlia, B. S. (2021). 244 YEAR long tree-ring based drought records from Uttarakhand, western Himalaya, India. Quat. Int. 599-600, 128–137. doi: 10.1016/j.quaint.2020.12.038
Keywords: intra-annual density fluctuation, earlywood, latewood, Himalayan cedar, cambium, western Himalaya
Citation: Maurya RS, Misra KG, Vishwakarma S, Singh V, Misra S and Yadava AK (2023) Analyses of intra-annual density fluctuation signals in Himalayan cedar trees from Himachal Pradesh, western Himalaya, India, and its relationship with apple production. Front. For. Glob. Change. 6:1243352. doi: 10.3389/ffgc.2023.1243352
Edited by:
Hülya Torun, Duzce University Duzce, TürkiyeReviewed by:
Yadav Ankit, Indian Institute of Science Education and Research Mohali, IndiaKevin Smith, Forest Service (USDA), United States
Copyright © 2023 Maurya, Misra, Vishwakarma, Singh, Misra and Yadava. This is an open-access article distributed under the terms of the Creative Commons Attribution License (CC BY). The use, distribution or reproduction in other forums is permitted, provided the original author(s) and the copyright owner(s) are credited and that the original publication in this journal is cited, in accordance with accepted academic practice. No use, distribution or reproduction is permitted which does not comply with these terms.
*Correspondence: Ravi S. Maurya, ravishankarmaurya94@gmail.com; Krishna G. Misra, kgmisrabsip@gmail.com