Vacuolar proteases and autophagy in phytopathogenic fungi: A review
- Laboratorio de Biología Molecular de Bacterias y Levaduras, Departamento de Microbiología, Instituto Politécnico Nacional, Escuela Nacional de Ciencias Biológicas, Mexico City, Mexico
Autophagy (macroautophagy) is a survival and virulence mechanism of different eukaryotic pathogens. Autophagosomes sequester cytosolic material and organelles, then fuse with or enter into the vacuole or lysosome (the lytic compartment of most fungal/plant cells and many animal cells, respectively). Subsequent degradation of cargoes delivered to the vacuole via autophagy and endocytosis maintains cellular homeostasis and survival in conditions of stress, cellular differentiation, and development. PrA and PrB are vacuolar aspartyl and serine endoproteases, respectively, that participate in the autophagy of fungi and contribute to the pathogenicity of phytopathogens. Whereas the levels of vacuolar proteases are regulated by the expression of the genes encoding them (e.g., PEP4 for PrA and PRB1 for PrB), their activity is governed by endogenous inhibitors. The aim of the current contribution is to review the main characteristics, regulation, and role of vacuolar soluble endoproteases and Atg proteins in the process of autophagy and the pathogenesis of three fungal phytopathogens: Ustilago maydis, Magnaporthe oryzae, and Alternaria alternata. Aspartyl and serine proteases are known to participate in autophagy in these fungi by degrading autophagic bodies. However, the gene responsible for encoding the vacuolar serine protease of U. maydis has yet to be identified. Based on in silico analysis, this U. maydis gene is proposed to be orthologous to the Saccharomyces cerevisiae genes PRB1 and PBI2, known to encode the principal protease involved in the degradation of autophagic bodies and its inhibitor, respectively. In fungi that interact with plants, whether phytopathogenic or mycorrhizal, autophagy is a conserved cellular degradation process regulated through the TOR, PKA, and SNF1 pathways by ATG proteins and vacuolar proteases. Autophagy plays a preponderant role in the recycling of cell components as well as in the fungus-plant interaction.
Introduction
Since fungal pathogens sense their nutrient supply and the changes in their environment, they respond with survival mechanisms aimed at establishing and promoting growth on their hosts. The course of invasion involves the remodeling of the fungal cell wall, adhesion, filamentation, appressorium formation, and sporulation. When first arriving to the surface of a plant, fungal pathogens face a nutrient-scarce environment and must constantly seek the appropriate mechanisms for survival. Particularly important are the mechanisms related to the release, transport, and metabolism of nutrients found during the different stages of infection (Johns et al., 2021).
Macroautophagy, hereafter called autophagy, is a highly conserved non-selective catabolic mechanism in eukaryotic cells consisting of the sequestering of cytosolic material (e.g., proteins and organelles) by autophagosomes and their delivery as autophagic bodies to the interior of the vacuole (for most fungal and plant cells) or their fusion with the lysosome (for many animal cells). A key element of autophagy is the delivery of hydrolytic enzymes into the vacuole (Fernandez et al., 2014; Zhu et al., 2019). After the membranes of autophagic bodies are degraded and the contents broken down by the vacuolar hydrolytic enzymes, degradation products are effluxed to the cytoplasm and reutilized in anabolic pathways (Parzych and Klionsky, 2019) (Figure 1A).
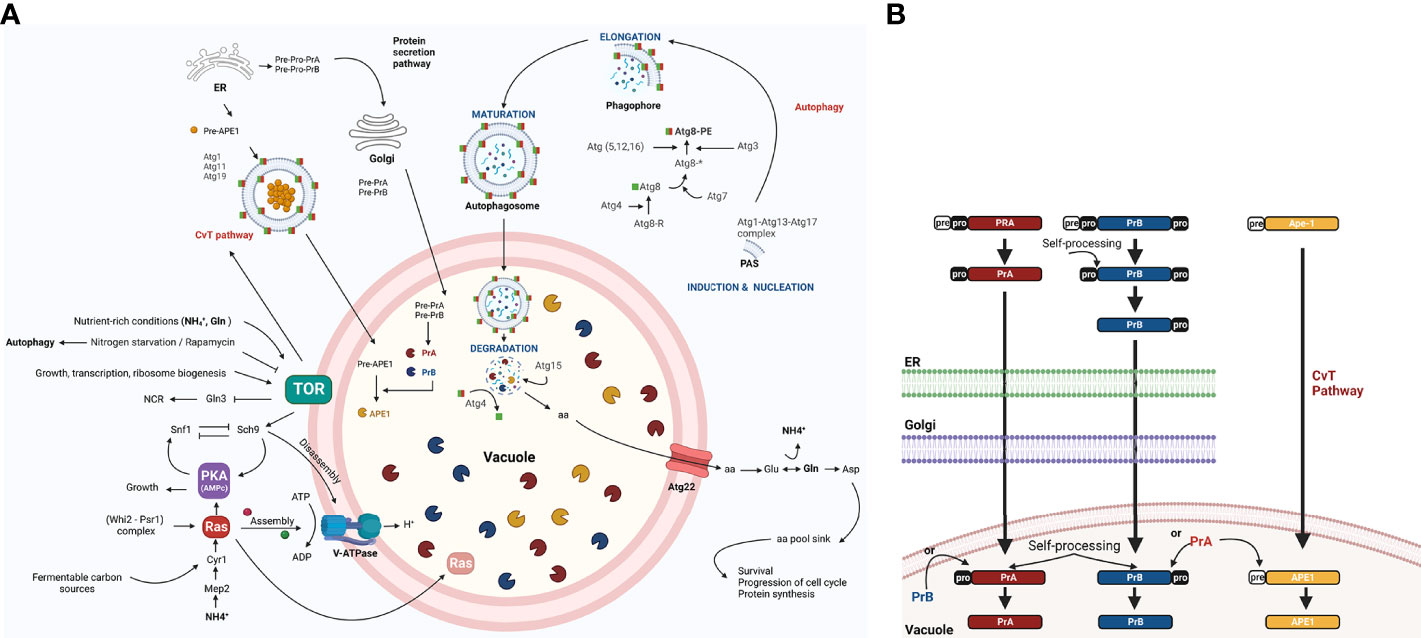
Figure 1 The role of autophagy, including the regulation of vacuolar proteases, in the yeast S. cerevisiae. (A) The TOR and RAS/cAMP kinases negatively regulate autophagy when the yeast is growing in nutrient rich conditions (glucose, NH+4, and Gln), thus avoiding G0 arrest. Both kinases inactivate Atg1, Atg13, and Atg8 proteins, which are involved in the first step of development of autophagosomes (Cebollero and Reggiori, 2009) and in the inactivation of transcription factor Gln3. The latter activates NCR genes and the Snf1 kinase. Under conditions of nutrient scarcity, Snf1 kinase is located in the vicinity of the vacuole and is released from the inhibition exerted by TOR and PKA, allowing it to inactivate TOR and PKA kinases. This inactivation takes place in part due to the targeting of Ras2 to the vacuole for proteolysis, mediated by the complex cell cycle regulator Whi2-phosphatase Psr1 (Leadsham et al., 2009). In addition to degrading specific proteins (e.g., Ras2), proteases and lipases break down autophagic bodies and their cargos, releasing amino acids and other biosynthetic units. These are either reused directly or induce Glu and Asp synthesis to replenish the amino acid sinks and therefore assure cell survival (Liu et al., 2021). In response to carbon availability, PKA and TOR regulate assembly and disassembly of V-ATPase through their effector Sch9 (Wilms et al., 2017). Vacuolar pH is regulated to maintain intracellular pH homeostasis and allow for the auto maturation carried out by protease PrA (Kane, 2006). (B) PrA matures to some extent in the endoplasmic reticulum (ER) before arriving to the vacuole and completing the process through automaturation. Subsequently, it stimulates the maturation of other vacuolar proteases, which are also synthesized as zymogens. Figure created by BioRender.com (accessed in April 2022).
By degrading damaged molecules and organelles, autophagy facilitates the cellular homeostasis and at the same time their health and longer life span (Aman et al., 2021; Tyler and Johnson, 2018). During vegetative budding, DNA damage can induce cell cycle arrest and autophagy. Furthermore, the selective autophagy-related cytoplasm-to-vacuole targeting pathway (CvT) is also crucial for cellular homeostasis and operates during nutrient rich conditions (Levine and Klionsky, 2004). When facing a sufficiency of nutrients nutrient uptake, carried out by plasma membrane transporters of the cell, that participate in nutrient acquisition to sustain cellular growth and much of them also as receptor, are degraded via endocytosis to avoid an excess of nutrient uptake (Busto and Wedlich-Söldner, 2019).
Autophagy is induced under certain stress conditions, including nutrient deprivation, oxidative damage to organelles, cell cycle dysregulation, and critical levels of aging-derived molecules. It is suggested that the size of the insult determines whether autophagy favors cell survival or cell death. The latter option avoids the propagation of damaged or mutated cells (Azzopardi et al., 2017). Hence, the proper balance of autophagy seems to be vital. Whereas a dysfunction in autophagy leads to an accumulation of toxic subcellular components, resulting in aging and disease (Azzopardi et al., 2017; Aman et al., 2021), an excess of autophagy generates detrimental effects, as exemplified by its capacity to promote tumor cell survival and proliferation (Azzopardi et al., 2017).
Unlike in other organisms, autophagy is induced in yeasts, mainly by the signal of nutrient scarcity. The process is initiated by inhibiting its master negative regulator, the TOR kinase (target of rapamycin) (Noda and Ohsumi, 1998). Indeed, it is implicated in yeast survival during nutritional stress (Liu et al., 2021). The autophagic process is initiated by the inhibition of its master negative regulator, the TOR kinase (target of rapamycin) (Noda and Ohsumi, 1998). The RAS/cAMP pathway negatively regulates autophagy as well, while Snf11 stimulates this macromolecular turnover system (Cebollero and Reggiori, 2009; Shashkova et al., 2015).
Protein degradation and the subsequent efflux of amino acids by the transporter Atg22 allow the cell to directly reuse free amino acids to synthesize glutamate and aspartate through a series of deamination and transamination reactions (Liu et al., 2021). Poor nutrient conditions not only cause bulk degradation of proteins in the vacuole, but also degradation of specific proteins (Figure 1A). As a consequence of the specific degradation of active Ras2, for instance, the absence of this protein in the mitochondria prevents the activation of autophagic apoptosis (Leadsham et al., 2009).
The vacuolar proteolytic system of the yeast Saccharomyces cerevisiae encompasses at least nine different unspecific proteases. Endoproteases PrA and PrB (an aspartic and serine protease, respectively) (Takeshige et al., 1992) participate in the activation of various vacuolar hydrolases and thus play a well-documented role in autophagy. PrA and PrB are first synthesized as preproenzymes and then mature over a series of post translational modifications, such as proteolysis, that take place in the endoplasmic reticulum and the Golgi apparatus. After the arrival of PrA to a vacuole, the last step of processing occurs via pH-dependent automaturation. Subsequently, PrA matures PrB and other canonical vacuolar proteases. One example is aminopeptidase Ape1, which is targeted to the vacuole through the TOR-induced CvT pathway (Parzych and Klionsky, 2019) (Figure 1B). Although vacuolar proteases PrA and PrB were first described in S. cerevisiae, they have since been found in phytopathogens and other fungal models and, they take part in autophagic bodies and protein degradation, that increase from 40% to 85% under conditions of starvation compared to conditions of growth. This capacity is sharply reduced when impaired V-ATPase function alters vacuolar acidification (Kane, 2006).
Autophagy has been extensively studied in yeasts such as S. cerevisiae and other ascomycetes, both pathogenic and non-pathogenic. However, there is less information available on filamentous fungi, and much less so regarding basidiomycete plant-interacting fungi. The current review aims to summarize the existing knowledge on autophagy, including its molecular basis of regulation via the TOR, PKA, and Snf1 kinases and by means of vacuolar proteases and transporters during the vacuolar efflux process. Furthermore, the biological function of vacuolar proteases in autophagy-dependent process such as morphogenesis and pathogenesis are explored. Regulation of proteolytic activity at different levels is also discussed, underlining endogenous protease inhibitors. These questions are discussed in relation to three phytopathogenic fungi: the hemibiotrophic M. oryzae and necrotrophic A. alternata of the Ascomycota phylum as well as the more distant biotrophic basidiomycete U. maydis (Figure 2). The conservation of autophagy is suggested to be beneficial for mycorrhizal fungi-plant symbiosis (Nehls and Plassard, 2018; Zhou et al., 2021), however they scape to the scope of this review.
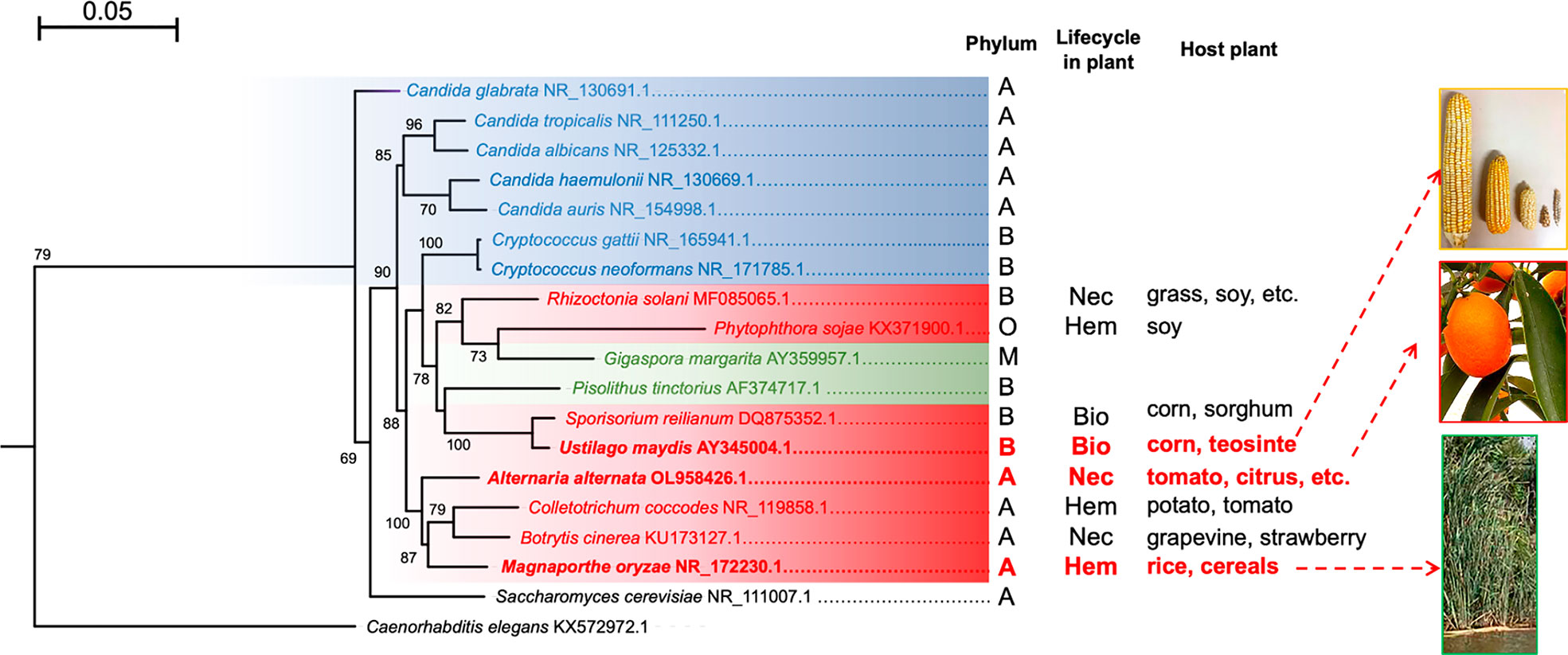
Figure 2 Maximum likelihood phylogenetic tree constructed with the sequences of the ITS regions of different fungi. Human pathogenic fungi are portrayed by blue branches, phytopathogenic fungi by red branches, and mycorrhizal fungi by green branches. S. cerevisiae and C. elegans are illustrated with black branches because the former is rarely isolated as a pathogen (its interest being purely biotechnological) and the latter served as an outgroup. The tree was generated with the MEGA11 program based on sequence alignment by using the MUSCLE algorithm, the substitution model HKY+G calculated by JModelTest, and 1,000 bootstrap replicates, as explained in the supplementary material. It was edited with the FigTree program. The phyla: A = Ascomycota, B = Basidiomycota, M = Mucoromycota, and O = Oomycota. The types of phytopathogen: Nec =necrotrophic, Bio = biotrophic, and Hem = hemibiotrophic. The host plant for each species is shown. The tree was generated with the MEGA11 program based on sequence alignment by using the MUSCLE algorithm, the substitution model HKY+G calculated by JModelTest, and 1,000 bootstrap replicates, as indicated in the supplementary material. It was edited with the FigTree program.
Autophagy in Ustilago maydis
Research on Ustilago maydis (the corn smut fungus) has provided insights into the ATG genes, the vacuolar proteases UmPrA and UmPrB, and the participation of such proteins in autophagy (Nadal and Gold, 2010; Soberanes-Gutiérrez et al., 2019), a well-conserved process in eukaryotes. To gain insights into the process of autophagy in U. maydis, it is necessary to first understand the context of the life cycle and pathogenesis of this fungus. Unlike other phytopathogenic fungi that are capable of infecting a wide variety of plants, U. maydis is a highly specific dimorphic phytopathogenic fungus only able to infect corn (Zea mays) and teosinte (Zea mays subsp. parviglumis). These two plants are closely related phylogenetically, likely sharing a common ancestor (Bruggeman et al., 2020).
U. maydis is a dimorphic fungus with a saprophytic and biotrophic phase during its life cycle. Its cycle begins with the germination of diploid teliospores followed by meiosis. Afterwards, haploid basidiospores are formed and present apical budding, allowing the sexually complementary yeasts to merge and establish a dikaryon, a stage in which the fungus can penetrate the maize plant tissue. The dikaryotic mycelium proliferates in the biotrophic phase of the fungus, and the fragmentation of the mycelium gives rise to dikaryotic teliospores that subsequently undergo karyogamy to generate mature melanized diploid teliospores. Damage to corn takes the form of galls or tumors (with a charcoal appearance) on the cob and other symptoms on the stem and leaves (chlorosis, increased anthocyanins, etc.) (Matei and Doehlemann, 2016) (Figure 3A).
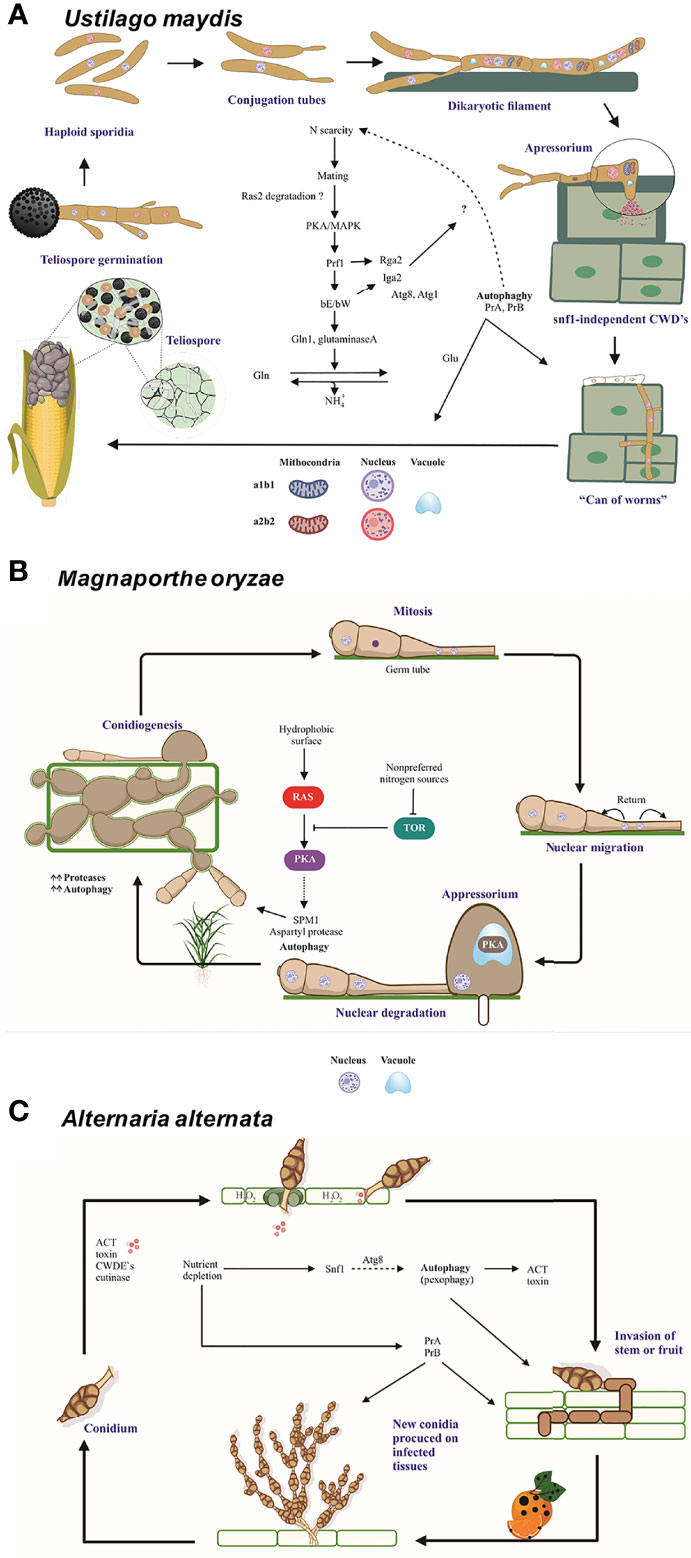
Figure 3 The role of autophagy and vacuolar proteases PrA and PrB in the pathosystem of U. maydis, M. oryzae, and A alternata. (A) In the course of an infection by U. maydis, nitrogen scarcity promotes filamentation of haploid sporidia mediated by the bE and bW genes (in an independent and nonredundant manner) and the Ump2 transporter, probably preparing the cells for mating (Wallen et al., 2021). After the mating of two compatible basidiospores, Rbf1 and bE/bW induce cell cycle arrest and dikaryotic filaments extend apically over the host tissue. Vacuolated areas separated from empty sections by septa are generated during filament extension. Subsequently, non-melanized appressoria develop and penetrate the plant cells by means of CWDE’s (Nadal et al., 2010). During this early stage, autophagy-related genes and vacuolar proteases PRB1 and PEP4 are overexpressed. Then the cell cycle restarts, fungal effectors are produced, and plant tissue is colonized. At the time of tumor formation, carbon, nitrogen and oligopeptide transporters, as well as autophagy-related proteins and proteases, all of them are overexpressed. Furthermore, the transcription factors nit2 and snf1 are upregulated (Lanver et al., 2018). In this late stage, there is a metabolic change in plant tissue that favors the growth of reproductive over vegetative tissue. Thus, carbon transporters Hxt1 and Suc1 are indispensable for fungal virulence (Schmitz et al., 2018). During the biotrophic phase, U. maydis faces conditions of nutrient stress and deploys many strategies to establish an effective infection system. (B) When three-celled conidia of M. oryzae arrive to the plant surface, the germ tube emerges, mitosis takes place, and the nuclei travel. As the appressoria mature, the conidia undergo autophagic programmed cell death to sustain appressorium function. The formation of appressoria is positively and negatively regulated by the Ras/cAMP and TOR pathways, respectively (Marroquin-Guzman et al., 2017), which in turn are regulated by the Whis2-Psr1 complex. The latter maintains the appropriate levels of cAMP and perhaps targets the Ras22 protein to the vacuole (Shi et al., 2021). (C) When the multicellular conidia of A alternata arrive to the plant tissue, they germinate and enter the plant cells through stomates or breaches by using an appressorium-like structure and CWDE’s. The plant cell membrane is immediately disrupted by ACT, which causes plant cell necrosis. Then the fungus proliferates and conidia are formed and released. The generation of H2O2 by the plant cell as a defense mechanism gives rise to pexophagy. The good functioning of peroxisomes is vital for the production of ACT toxin (Wu et al., 2021). Additionally, different aspects of autophagy are important for the pathogenicity of A alternata. The Δatg8 strain is unable of either to form aerial hyphae and provoke necrotic lesions, similarly to the Δprb and a pep4-silenced strain. Interestingly PrB participating in the synthesis of secreted proteases (Fu et al., 2020). Nutrient scarcity leads to the expression of the entire autophagic machinery as well as the induction of Snf1, an essential protein for carbon utilization, vegetative growth, conidiation, and cell wall functions (Tang et al., 2020). Schemes were drawn whit BioRender.com (accessed in April 2022) and Corel Drawn v. 19.
Orthologous genes of ATG1 and ATG8 in U. maydis
Carbon stress conditions in U. maydis favor the accumulation of autophagic bodies in the cell vacuoles as well as the overexpression of the genes atg1 and atg8 (Nadal and Gold, 2010). The autophagy genes ATG1 and ATG8 play an essential role in the induction of autophagy and the assembly of autophagosomes, respectively, in the nonpathogenic S. cerevisiae (Figure 1A). Strains of U. maydis lacking such genes have been evaluated (Nadal and Gold, 2010). According to the findings, the atg8 gene of U. maydis is related to the process of autophagy in a manner similar to its orthologous gene ATG8 of S. cerevisiae.
The deletion of atg8 was observed in both the saprobic and biotrophic phases. The ∆atg8 mutants lacked the accumulation of autophagosomes in the vacuole under conditions of carbon starvation, leading to reduced survival. There was an alteration of the characteristic apical budding and cell separation caused by the deletion of atg8, as shown by the structure of “lateral buds” of the yeasts in the exponential and stationary growth phase. The infection of maize plants with sexually complementary atg8 mutant strains, versus wild-type (WT) strains, led to limited colonization of plants by the fungus, a decline in the number of plants that presented galls, and a diminished virulence index. Additionally, a relatively low density of invading hyphae was found in plant tissues as well as a decrease in the formation of teliospores.
The Atg8 protein of S. cerevisiae undergoes post-translational processing at the C-terminus by the cysteine protease Atg4 to generate Atg8G116. The latter binds covalently to phosphatidylethanolamine (PE) in a ubiquitin-like reaction catalyzed by Atg3 and Atg7 (Ichimura et al., 2000; Kirisako et al., 2000). The analysis of the amino acid sequence of the protein deduced from the U. maydis atg8 gene and its phylogenetic relationship with the sequences of other organisms (Figure 4) supports its characterization as the ATG8 ortholog of S. cerevisiae, since the protein sequence of this basidiomycete contains the G116 residue in a conserved amino acid context (Nadal and Gold, 2010).
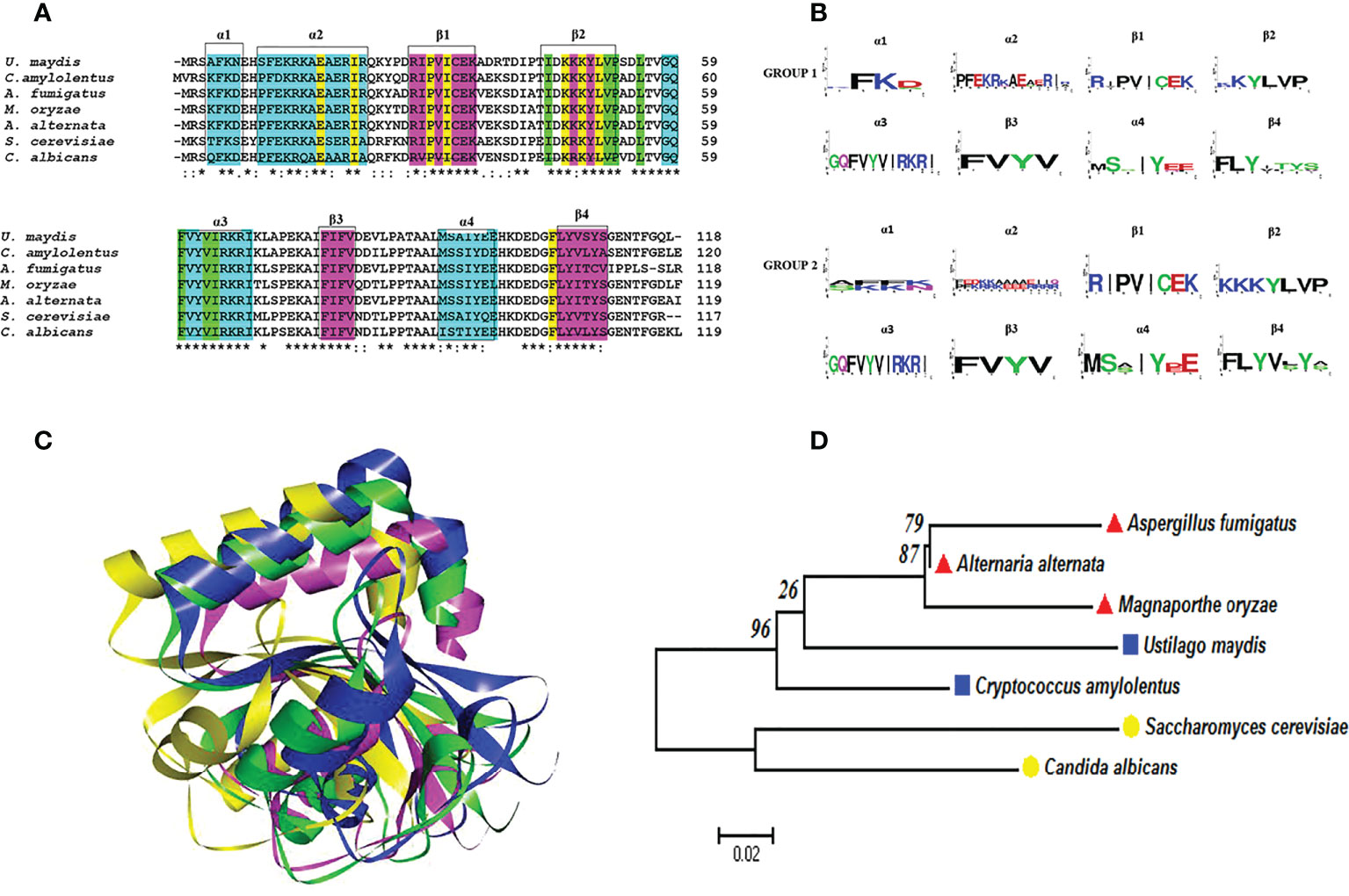
Figure 4 Atg8 proteins are conserved in pathogenic and nonpathogenic fungi. (A) Multiple alignments were performed with Clustal Omega. In the alignment, the amino acids associated with alpha-helical (cyan) or beta-strand (fuchsia) secondary structures are highlighted with boxes. Likewise, the important amino acid residues for the two deep hydrophobic pockets HP1 and HP2 function are highlighted in yellow and green, respectively. An asterisk (*) denotes a completely conserved residue; a colon (:) represents the conservation of the properties of the residual side chain; a dot (.) indicates residues with side chains of weakly similar properties; and a hyphen (-) designates a gap. (B) Consensus logos were generated with WebLogo. Group 1 (S. cerevisiae, C albicans, A fumigatus, M. oryzae, and A alternata) and group 2 (C. amylolentus and U. maydis). (C) 3D model of the Atg8 proteins. The overlap of S. cerevisiae (green) with the fungal phytopathogens is represented by utilizing the best model of the Atg8 of each fungus: U. maydis (blue), M. oryzae (fuchsia), and A alternata (yellow). The model was generated by homology modeler by using the Modeller 9.23 program, as explained in the supplementary material. (D) Phylogenetic analysis of the Atg8 of different organisms, carried out in the MEGA6 program with the maximum likelihood method, the WAG+G model, and 100 bootstrap replicates. The phylogenetic tree is drawn to scale, with the length of the branches depicting the corresponding evolutionary distances. Fungi that are grouped together in the same clade are portrayed with red, blue, and yellow symbols.
On the other hand, the deletion of the orthologous atg1 gene of U. maydis resulted in phenotypes similar to the ∆atg8 mutants during the saprobic phase. The ∆atg1 mutants were slightly less pathogenic for maize plants than the WT strains, although teliospore production was not affected. However, the phenotype of a double mutation at ∆atg8 and ∆atg1 was additive, evidenced by the even greater decline in pathogenicity as well as reduced teliospore production compared to the single-mutant strains. The mating process and the growth of filaments were not affected by the deletion of the atg8 and atg1 genes.
The importance of nutrient stress in triggering autophagy along with cellular adaptation and reprogramming in various organisms has been recognized for about two decades (Levine and Klionsky, 2004). For U. maydis and other fungi, carbon stress conditions existing at distinct stages of the fungal life cycle are able to cause cellular reprogramming, which can be provoked by a number of conditions. For example, the mating process involving the conjugation and fusion of two complementary sporidia of U. maydis is preferably carried out under conditions of starvation or a low nitrogen concentration (Wallen et al., 2021). Apart from its participation in the fungal response to stress conditions, autophagy might play a relevant role in the uniparental inheritance of a2 mitochondria in U. maydis. This is mediated by the small mitochondrial proteins Rga2 and Lga2, the encoding genes of which are located exclusively in the a2 locus (Mendoza et al., 2020).
Vacuolar protease A and autophagy in U. maydis
The pep4 gene of U. maydis encodes a vacuolar aspartyl proteinase denominated UmPrA (also known as proteinase A or UmPep4). Its proteolytic activity, detected in the soluble fraction of cell extracts from U. maydis, is greater when the fungus is in the mycelial versus yeast phase. The presence of pepstatin, a specific inhibitor of aspartyl proteases, impedes the dimorphic transition from yeast to mycelium (Mercado-Flores et al., 2003).
According to proteomics studies, the protein encoded by the pep4 gene in U. maydis is overexpressed during the yeast-mycelium transition, which is induced by the master regulator bE/bW. In addition to UmPep4, proteins orthologous to S. cerevisiae Gln1 and glutaminase A are overexpressed (Böhmer et al., 2007). These three proteins, involved in the later steps of autophagy in S. cerevisiae, carry out amino acid and glutamine synthesis under conditions of nutrient depletion (Liu et al., 2021). A similar strategy may exist for U. maydis to replenish N pools (Figures 1A and 3A).
Mutants of U. maydis were generated in the pep4 gene, creating a deficiency in protease A that diminished the dimorphic transition triggered by acid pH or by the use of fatty acids as the sole carbon source. As a consequence, the pathogenicity of the fungus towards the corn plant was affected. The ∆pep4 mutants caused a lower number of plants to be infected, a decrease in the signs of infection (e.g., chlorosis and an increase in anthocyanins), and less galls on the ears of corn (Soberanes-Guitiérrez et al., 2015). The construction of chimeric PrA proteins with green and red fluorescent proteins (PrA-GPF and PrA-RPF) confirmed the vacuolar location of the protease (Soberanes-Guitiérrez et al., 2015).
According to ultrastructural analysis of fungal cells, mutants of U. maydis deficient in PrA accumulate autophagosomes in the vacuole when incubated under conditions of carbon starvation stress (Soberanes-Gutiérrez et al., 2019). This is probably due to the inability of the yeasts to degrade autophagosomes. In the S. cerevisiae model, a similar phenomenon of autophagosome accumulation in vacuoles is observed in the absence of protease B or protease A (Takeshige et al., 1992).
Carbon or nitrogen starvation produces stress during the growth of S. cerevisiae, making a recycling system necessary. Autophagy is the primary transport pathway for bulk protein and organelle degradation (Teter and Klionsky, 2000), which occurs in the vacuole. The structure of the vacuole varies according to environmental conditions (Pratt et al., 2007). Autophagosomes collect material and enter the vacuole, where the enzymes of this organelle degrade their membrane, allowing for the recycling of the cytoplasmic-derived content (Abeliovich and Klionsky, 2001). In the mutants of U. maydis deficient in the acid proteinase UmPep4p (the OR1 and OR2 strains), the accumulation of autophagosomes was evident after nutrient shortage. CMAC staining revealed that the size and number of fusion events of vacuoles increased in complete medium for both WT and mutant strains as time progressed (Soberanes-Gutiérrez et al., 2019).
The U. maydis pep4 gene has been cloned and heterologously expressed in order to biochemically characterize the recombinant protein. Based on its inhibition by pepstatin, the protein was found to be an aspartyl protease. Additionally, the tertiary structure of UmPrA turned out to be highly homologous to human cathepsin D, indicating that Umpep4 could be the ortholog of the gene encoding human cathepsin D (Juárez-Montiel et al., 2018).
Interestingly, human cathepsin D, encoded by the CTSD gene, is active in the lysosome (the compartment equivalent to the fungal vacuole), and mutations in the gene result in protein accumulation in the organelle as well as nerve cell death (CLN10 disease). Genetic variants of the CTSD gene have been linked to neurodegenerative diseases such as Parkinson’s and Alzheimer’s disease (Bunk et al., 2021). Apart from degrading proteins, human cathepsin D can activate other proteins and regulate apoptosis (Di et al., 2021). The reduction in cathepsin D activity in human fibroblasts affects autophagic degradation, a change associated with insufficient lysosomal function (Tatti et al., 2012).
When detected in mammary secretions, human cathepsin D has been proposed as a cancer marker because its overexpression is related to tumor invasion. The overexpression of PEP4 in S. cerevisiae has led to the presence of the enzyme in the nucleus of the yeast. This aspartyl protease may contribute to the degradation of nucleoporins during H2O2 stress (Kerstens and Van Dijck, 2018).
Taking all the prior evidence into account, the Pep4 protein from U. maydis is probably orthologous to human cathepsin D and Pep4 from S. cerevisiae (Juárez-Montiel et al., 2018). Furthermore, these vacuolar/lysosomal aspartyl proteases not only show a conserved tertiary structure with those of Magnaporthe and Alternaria but also a similar function (Supplementary Figure 1), reinforcing the idea of their evolutive relationship.
Vacuolar protease B and autophagy in U. maydis
The activity of U. maydis protease B (UmPrBp), a serine protease, is inhibited by phenyl-methylsulfonyl fluoride (PMSF). The latter enzyme was detected in U. maydis for the first time in 2003, using Hide–Remazol Brilliant Blue R (Hide powder azure) substrate at pH 7 and in the presence of an endogenous inhibitor activated and released at acidic pH (Mercado-Flores et al., 2003). Considering that the inhibition of UmPrB with PMSF led to the accumulation of autophagosomes, this serine protease (like UmPrA) likely participates in the degradation of autophagic bodies (Soberanes-Gutiérrez et al., 2019).
The absence of protease B during the process of autophagy in S. cerevisiae causes an accumulation of autophagosomes in the vacuoles (Takeshige et al., 1992). After autophagosome formation and the transportation of the cellular cargoes to vacuoles, autophagic membranes are degraded by vacuolar enzymes, allowing the cellular components inside to meet the nutritional requirements of S. cerevisiae under conditions of starvation (Takeshige et al., 1992; Abeliovich and Klionsky, 2001).
In S. cerevisiae cells under conditions of carbon or nitrogen starvation, and in mutants deficient in protease A and protease B, ribosomes and mitochondria are observed inside of autophagosomes. Hence, these vacuolar proteases and the process of autophagy in general seem to degrade and recycle intracellular components (Takeshige et al., 1992). In WT U. maydis, autophagic bodies did not accumulate in the cell vacuoles until the serine protease inhibitor PMSF was added. In contrast, such accumulation occurred in sporidia with a mutation in the acid proteinase Pep4p even in the absence of PMSF. Overall, the findings provide evidence of a key role for PrB in the degradation of autophagic bodies in U. maydis. In addition, PMSF-treated WT FB2 cells were shown to accumulate autophagic bodies, suggesting that vacuolar proteinase B is involved in the degradation of the autophagic bodies in other systems as well. In the same sense, autophagic bodies have been reported to disappear if cells are transferred to a culture medium without PMSF (Takeshige et al., 1992; Baba et al., 1994).
As already mentioned, the vacuolar proteases PrA and PrB of S. cerevisiae undergo distinct proteolytic processes from the beginning of their synthesis to their passage through the secretion route via the endoplasmic reticulum-Golgi apparatus-vacuole (Parzych and Klionsky, 2019). Whereas PrB from S. cerevisiae needs 4 post-translational processing sites to become a mature enzyme (Figures 1B, 5), the proteolytic processing of the putative proteinase B of U. maydis, encoded by the gene with access number Um4400, is herein proposed to encompass only three such sites (Figure 5). Given its carboxyl terminal portion, UmPrB may not mature until it reaches the vacuole. At this time, automaturation likely takes place rather than a process directly involving UmPrA. Moreover, the predicted protein presents the characteristic domain of a subtilisin-like serine protease of the S8 family. Even though Magnaporthe and Alternaria are ascomycete fungi, processing sites similar to those of UmPrB are found in their respective PrB proteins, as are similar domains and tertiary structures. Furthermore, the phylogenetic relationship of UmPrB with the PrB of other related fungi indicates the probable orthology between them (Figure 5).
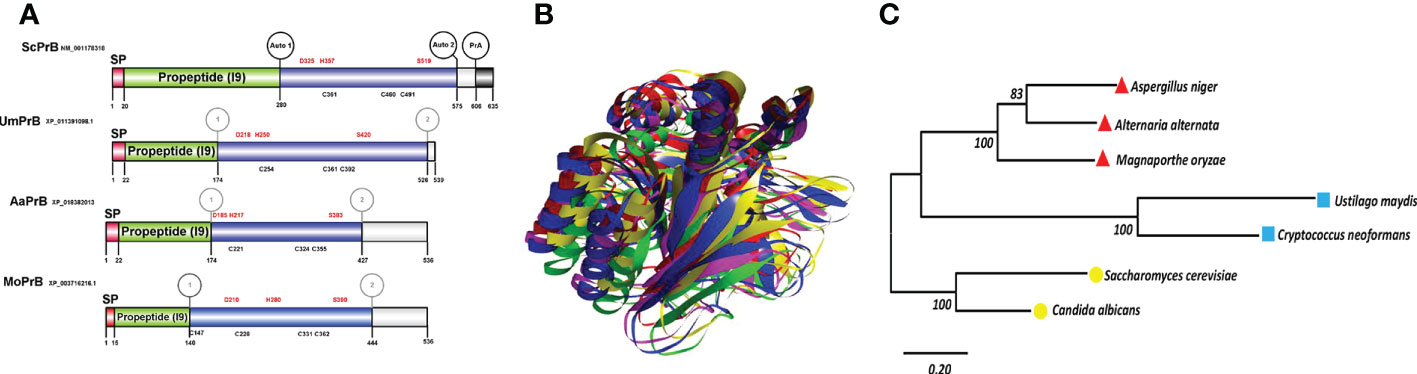
Figure 5 Serine vacuolar endoproteases PrBs are conserved in pathogenic and nonpathogenic fungi. (A) The characteristic domains of vacuolar proteases of the S8 family are illustrated, including the DHS catalytic triad of serine proteases, the probable maturation sites, and the I9 inhibitor domain of the propeptide (predicted in the Expasy server). Proteases such as ScPrB are apparently synthesized as zymogens, which are inactive until propeptides are removed in the vacuole. The cysteines in the protein are also shown. The circles in the S. cerevisiae protease designate the regions where autocatalysis and PrA processing are conducted. In the rest of the proteases, the circles denote regions of theoretical maturation. The alignment of sequences was performed with Clustal X and the drawing with BioRender.com (accessed in April 2022). (B) Tertiary structure of fungal PrBs. The overlap is represented by utilizing the best model of PrB of S. cerevisiae (green) and of the three fungal phytopathogens herein studied: U. maydis (blue), M. oryzae (fuchsia), and A alternata (yellow). (C) Phylogenetic analysis of the PrB of different organisms. Carried out in the MEGA6 program with the maximum likelihood method, the WAG+G model, and 100 bootstrap replicates. The phylogenetic tree is drawn to scale, with the length of the branches depicting the corresponding evolutionary distances. Fungi that are grouped together in the same clade are portrayed with red, blue, and yellow symbols.
Both PrA and PrB propeptides inhibit their cognate proteases until the latter are removed upon reaching the vacuole. The endogenous cytoplasmic inhibitors for these proteases are known, AI3 and PBI2 (I2B, YIB2) for PrA and PrB, respectively (Dunaevsky et al., 2014). The inhibitory activity of PrB propeptide and the PBI2 inhibitor depends on their C-terminal region. It has been determined that the C-terminal region of the propeptide of S. cerevisiae PrB and the inhibitor PBI2 share conserved regions with the subtilisin BPN´ propeptide, which inhibits its cognate protein. All these inhibitors belong to the I9 family, which lacks disulfide bonds, an unusual characteristic of serine protease inhibitors (Kojima et al., 1999). Apart from its inhibitory function, PBI2 participates in vacuole inheritance (together with thioredoxin) by regulating vacuole coalescence. Thus, inhibitor-protease binding may be a regulating mechanism of the latter process (Slusarewicz et al., 1997).
An AI3 homolog has not been identified in U. maydis, though a probable PBI2 ortholog was detected (with access number Um10059, JGI portal Um02129). Whereas the predicted protein contains a unique region not present in PrB propeptides or PBI2, its C-terminal region is conserved (Supplementary Figure 2). The docking of this peptide with PrB of Saccharomyces, Ustilago, and Alternaria evidenced its interaction with similar amino acid residues in each case, including the ones that make up the catalytic triad (Asp, His, and Ser) (Figure 6). The PBI2 of S. cerevisiae interacts with most of the same catalytic residues of these PrBs (Supplementary Figure 3). Similar interactions have been seen for the subtilisin of B. amyloliquefaciens (Gallagher et al., 1995). The protein Um10059 might be responsible for the inhibition of the UmPrB activity reported by Mercado-Flores et al. (2003).
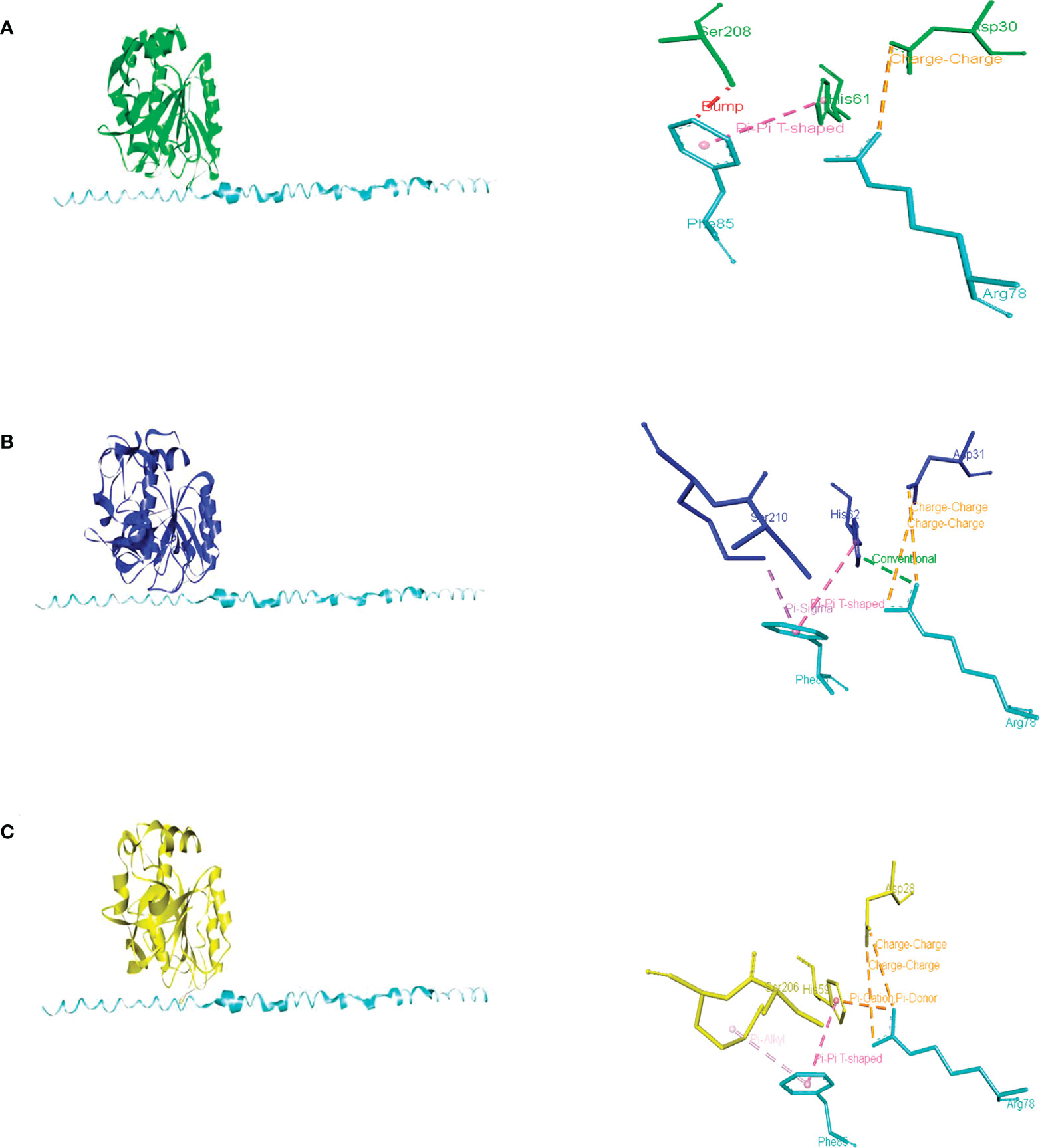
Figure 6 Intermolecular interactions of inhibitory peptide Um10059 (Um2129) (cyan) with PrBSc (A), PrBUm (B), and PrBAa (C). Dotted lines indicate the type of interactions. For the purpose of clarity, the interactions between the amino acid residues of the PrB catalytic triad (Asp, His, and Ser) and the inhibitory Um10059 are shown. The interactions with M. oryzae were not shown since none of the residues of the catalytic triad interact with the inhibitor of U. maydis.
According to the docking analysis, there are distinct characteristics of specificity of the propeptides, and such differences are related to the phylum of the fungi. While the PrB propeptide of S. cerevisiae was able to interact with the catalytic residues of PrB of Saccharomyces, Magnaporthe, and Alternaria, the PrB propeptide of Ustilago seems to be different because it could only interact with PrB residues distinct from the catalytic ones (Supplementary Figure 4 and Supplementary Table 2).
In addition to the biological implication of these inhibitors, interesting biotechnological applications have been suggested in medicine (e.g., to treat cancer and other diseases) and agriculture (e.g., to target phytopathogens) (Dunaevsky et al., 2014. Applications of inhibitors are discussed later in this review.
Atg8 and Atg1 proteins and autophagy in Magnaporthe oryzae
Magnaporthe oryzae (anamorph: Pyricularia oryzae) is a hemibiotrophic ascomycete and the causal agent of rice blast disease. It develops a highly melanized dome-shape appressorium from a three-celled conidium (spore) (Wilson and Talbot, 2009). After germination of M. oryzae conidia, mitosis and nuclear migration occur. Then the nuclei of the conidia are degraded via autophagy to sustain the successful formation of infectious hyphae (Figure 3B).
Under nutrient-poor conditions, Δatg8 mutants did not exhibit autophagic activity. The accumulation of autophagic bodies was not seen inside vacuoles in mycelium cells (using mono-dansyl cadaverine stain), even when PMSF (an inhibitor of serine proteases) was added. The structure of the M. oryzae Atg8 protein is similar to the one existing in S. cerevisiae Atg8 (Figure 4), and there was no nuclear breakdown in the Δatg8 mutant that was capable of affecting pathogenesis without affecting its capacity to produce appressoria (Veneault-Fourrey et al., 2006; Veneault-Fourrey and Talbot, 2007).
A mechanical force derived from the high osmotic pressure inside the appressorium facilitates the disruption of the plant cuticle (Figure 3B). Since this pressure is favored by glycerol accumulation and melanization, it causes cytoskeleton reorientation and polarization, leading to the formation of the penetration peg (Osés-Ruiz and Talbot, 2017). In M. oryzae, deletion of the ATG14 genes altered autophagy, glycogen mobilization, the number of lipid droplets, and turgor pressure, thus affecting infection and conidiation (Liu et al., 2017). Bulk autophagy of the material of the conidium plays a major role in the turgor pressure, melanization, and repolarization of appressoria. However, glycogen mobilization is not essential in the generation of turgor pressure. Rather, the degradation of lipid droplets seems to be the main source of glycerol and melanin (Foster et al., 2017).
Vacuolar protease A and autophagy in M. oryzae
During appressorium formation induced on a hydrophobic GelBond surface, two aspartyl proteases genes, MGG_09351.5 and MGG_00981.5, were overexpressed. Similar results were obtained when the development of appressoria was triggered by the addition of cAMP. In either case, the most upregulated genes were generally related to protein and amino acid degradation as well as carbohydrate and lipid metabolism, while genes linked to protein synthesis tended to be downregulated (Oh et al., 2008). On the other hand, the most probable vacuolar aspartyl protease shows a conserved 3D structure in relation to the PrA from other yeasts and filamentous fungi and to human cathepsin D (Supplementary Figure 1). Hence, all of them may be homologous and implicated in the last step of autophagy. Overall, this information highlights the crucial function of aspartyl proteases in eukaryotic organisms.
Vacuolar protease B and autophagy in M. oryzae
SPM1 (MGG_03670.5) in M. oryzae encodes the endoprotease Spm1, related to PrB in S. cerevisiae (Figure 5), which is targeted to the vacuole in the course of an infection. Spm1 is upregulated at the time of appressorium formation. Although mutants produced melanized appressoria in the presence of cAMP and under conditions of nitrogen starvation in synthetic media, a decrease was found in virulence, aerial hyphae, and conidiation (Oh et al., 2008). Spm1 is involved in multiple aspects of the infection process such as conidia germination, invasion, and endocytosis. As evidence of its role in endocytosis, spm1 mutants were not capable of sustaining the vacuolar accumulation of FM4-64. In agreement with the canonical function of the vacuolar serin endoproteases, deletion of the SPM1 gene leads to the accumulation of granular particles inside the vacuoles of appressoria and conidia (Saitoh et al., 2009).
Atg8 and Atg1 proteins and autophagy in Alternaria alternata
Alternaria alternata (an ascomycete) is able to cause disease in a plethora of hosts by diverse pathotypes, each of which secretes a host-specific toxin (HST) crucial for successful infection. For instance, the tangerine pathotype produces adenylate cyclase toxin (ACT), an HST for Citrus reticulata, Citrus sinensis, and Citrus paradisi. ACT is responsible for the necrotic lesions on the leaves and fruit, typical symptoms of citrus brown spot (Kohmoto et al., 1993). Unlike biotrophic and hemibiotrophic fungi, necrotrophic pathogens such as the tangerine pathotype of A. alternata kill their host before colonization. This is done by generating ACT and cell wall degrading enzymes (CWDE’s) and occurs immediately after penetrating the plant through stomata on the abaxial side of the leaves as well by a small non-melanized appressorium on the adaxial side (Figure 3C).
As a defensive response to fungal infection, a plant is to produce a great quantity of H2O2 (the hypersensitive response). However, necrotrophic pathogens have developed mechanisms to cope with H2O2 stress (Lin et al., 2011). For example, the tangerine pathotype of A. alternata activates Yap1 and Tfb5 transcription factors, regulator Skn7, HSK and Hog1 kinases, catalase, and superoxide dismutase (SOD). Fe+, the SOD cofactor, is taken from the environment through siderophores and stored in vacuoles. Whereas the secretion of siderophores is indispensable for the pathogenicity of A. alternata, it is dispensable for the pathogenicity of U. maydis (Chung, 2012). On the other hand, the regulation of Fe+ via vacuolar compartmentalization is unknown in the latter biotrophic fungus.
Peroxisomes are usually involved in the elimination of H2O2. Nevertheless, subsequent to H2O2 treatment of Alternaria, the level of peroxisomes declines and they are found colocalized with vacuoles, suggesting that pexophagy is taking place. The alteration of the function of peroxisomes in Alternaria Δpex6 mutant affects pathogenicity and autophagy, partially restored adding purified ACT toxin. In Δpex6 mutants, there was reduced expression of ATG8, a gene responsible for encoding the Atg8 protein, which accumulates in vacuoles and participates in autophagosome formation when A. alternata grows in the absence of nitrogen (Fu et al., 2020; Wu et al., 2021). Moreover, this Atg8 has a highly conserved tertiary structure in relation to the homologous proteins of the other fungi described herein (Figure 4). Overall, the evidence supports the notion that the function of Atg8 could be similar in fungi, as has been proposed in previous reports (Ivory et al., 2021).
In A. alternata, as in diverse yeasts and filamentous fungi, macroautophagy appears to be important for maintaining the homeostasis of cells under conditions of stress. The pathway might be similar, involving factors such as Atg proteins, TOR, Snf1, PKA, and MAPK kinases.
Vacuolar protease A and autophagy in Alternaria alternata
The crucial role of the aspartyl protease in the course of plant infection by the A. alternata tangerine pathotype was demonstrated in a pep4 gene silencing assay. The downregulation of pep4 gave rise to the incapacity of A. alternata to produce conidia in PDA medium, and the resulting small necrotic plant lesions indicated reduced virulence. In the silenced pep4 strain, autophagic bodies accumulated inside the vacuole, revealing the essential participation of the corresponding protein in autophagy (Fu et al., 2020). This information together with the similarity between the predicted structure of PrA of A. alternata with the structure of the same protein in other fungi (Supplementary Figure 1) suggests that all the vacuolar aspartyl proteases discussed herein are orthologous proteins.
Vacuolar protease B and autophagy in A. alternata
In addition to the canonical vacuolar aspartyl protease in A. alternata, a subtilisin-like serine protease of the S8 family has also been detected. Based on the domains identified and the physical interaction with PrA, demonstrated by using a yeast two-hybrid assay (Fu et al., 2020), as well as the similar maturation and probable self-regulation (by the I9 domain of the propeptide of PrB of S. cerevisiae) these two proteins are probably orthologous. During incubation in minimal medium, growth was slower for A. alternata Δprb1 strains than the WT strain. Normal growth was restored by adding citrus leaves, yeast extract, and urea as a nitrogen source, but the mutant was not capable of normal conidiation even in PDA medium. Although A. alternata Δprb exhibited decreased pathogenicity compared to the WT strain (evidenced by the small necrotic lesions it induced), its production of ACT was not affected. Similar to PrA in A. alternata, its PrB is essential for breaking down vacuolar autophagic bodies in conditions of nutrient scarcity (Fu et al., 2020). It is similar in structure to other PrBs and is phylogenetically related to them (Figure 5). Furthermore, it is inhibited by the predicted U. maydis Um00159 protein and the PBI2 inhibitor (Figure 6C and Supplementary Figure 3D).
Microbial virulence, a measure of the capacity of a given microbe to cause damage in a susceptible host, can be enhanced, lost, and restored. While the virulence of phytopathogenic fungi depends on secreted proteins such as effectors and toxins, cellular metabolism, morphogenesis, and sporulation are also involved (Johns et al., 2021). The relationship between the process of autophagy in phytopathogenic fungi and their virulence has been established. The phytopathogenic fungi U. maydis, M. oryzae, and A. alternata lacking the vacuolar proteases PrA and PrB and the subtilisin-like protease Spm1 were deficient in terms of autophagy, morphological transitions related to the infection processes, and probably the secretome (Saitoh et al., 2009; Soberanes-Gutiérrez et al., 2015) (Figure 3).
Possible applications derived from the study of autophagy
As already mentioned, the process of autophagy has been investigated in humans and other mammals, and its role in the pathogenesis of neurodegenerative diseases and cancer has been demonstrated. Inhibitors and activators of autophagy could serve to better understand the regulation of this process in human diseases. The testing of autophagy-targeted drugs has also been proposed (Yang et al., 2013). Given that the process of autophagy is highly regulated, a balance must exist between its induction and inhibition (Meijer and Codogno, 2009). According to several authors, activators of autophagy may have therapeutic benefits for various diseases (Yang et al., 2013). Among such activators are endoplasmic reticulum stress inducers (Ciechomska et al., 2013), rapamycin and its derivatives (Ravikumar et al., 2004), trehalose (Sarkar et al., 2007), and inositol monophosphatase (IMPase) inhibitors (e.g., lithium chloride) (Sarkar et al., 2005). On the other hand, autophagy can be suppressed at any of its stages. One of the problems is the lack of specificity of many autophagy-inhibiting compounds. The following compounds have been posed as autophagy inhibitors: PI3K inhibitors (Castino et al., 2010); cycloheximide (Oliva et al., 1992), vacuolar-type H(+) ATPase inhibitors (Wu et al., 2009), lysosomal lumen alkalizers (Harhaji-Trajkovic et al., 2012), and acid protease inhibitors (e.g., leupeptin and pepstatin A) (Kominami et al., 1983; Tanida et al., 2005). In addition to contemplating pharmacological modulation of autophagy based on the type and stage of certain diseases, gene-targeting approaches have been postulated as a new therapeutic option for human diseases associated with the deregulation of the process of autophagy (Yang et al., 2013).
The inhibition of autophagy in human pathogenic fungi is an approach worthy of investigation since it represents a mechanism distinct from conventional antifungals and thus could plausibly help to combat the multi-resistance problem of yeasts of the genus Candida, which has become a public health problem in recent years (Madrigal-Aguilar et al., 2022). The participation of the ATG1 and ATG11 genes in virulence and autophagy has been assessed in Candida glabrata and Candida albicans (Cui et al., 2019; Shimamura et al., 2019), as has the role of vacuolar proteases in conditions of nutritional stress and their possible association with the process of autophagy (Sepúlveda-González et al., 2016; Cortez-Sánchez et al., 2018). Future research is needed into the application of inhibitors or activators of autophagy in phytopathogens and fungi pathogenic to humans in order to reduce or avoid the effects on the corresponding hosts.
Conclusions
The current review focuses on the role of vacuolar proteases PrA and PrB, the Atg8 and other molecular players in the process of autophagy of three phytopathogenic fungi (belonging to two phyla) with different plant-interaction lifestyles. The insights provided may be useful for the regulation of the process of autophagy in both the host and pathogenic fungi. Included in the study were a biotrophic basidiomycete (U. maydis), a hemibiotrophic ascomycete (M. oryzae), and a necrotrophic ascomycete (A. alternata). The plausible orthology between the vacuolar proteases PrA and PrB in such fungi and in S. cerevisiae is suggested by both molecular phylogeny and their respective functions, as they all participate in the degradation of autophagic bodies in the vacuole during the process of autophagy. Moreover, the catalytic domains are conserved in the primary and tertiary structures of the proteins from the three species of phytopathogenic fungi herein examined. According to the molecular modeling analysis, the endogenous inhibitor of PrB, denominated PBI2, exists not only in S. cerevisiae but probably also in U. maydis. This inhibitor regulates the proteolytic activity of PrB. PBI2 and Um10059 each bind to the catalytic residues of their corresponding protease and those of the Alternaria protease. Likewise, the Atg8 protein is conserved in these phytopathogenic species. Based on the analyses of the amino acid sequences of the corresponding proteins of U. maydis, M. oryzae, and A. alternata, they are probably orthologs of S. cerevisiae Atg8. Overall, the process of autophagy seems to be conserved in phytopathogenic fungi, in non-pathogenic fungi such as mycorrhizae, and in other eukaryotic organisms (e.g., mammals). The fact that, unlike the propeptide of PrB, the putative PrB of U. maydis does not recognize the catalytic residues of its respective serine protease or those of the serine proteases of other fungi is likely due to adaptation and the evolutive distance between the organisms (ascomycetes and basidiomycetes). The function of vacuolar proteases PrA and PrB and of autophagy in general is part of the cell response to nutritional stress in order to replenish critical constituents needed for survival. Under basal conditions, autophagy removes long-lived, damaged, and redundant proteins or organelles to allow for cellular longevity. It serves as a type of programmed cell death in the course of cell development and differentiation. In the process of infection, fungal phytopathogens sense nutritional scarcity and other environmental conditions through the cAMP/PKA and MAPK signaling pathways. These pathways regulate the nutrient sensor TOR as well as the Atg proteins that initiate autophagy and participate in the distinct stages of fungal development of the three phytopathogenic fungi presently examined. During the stages of spore germination, mating, penetration of the host plant, and sporogenesis, it is crucial for the fungus to maintain its virulence and pathogenicity. Hence, autophagy plays a major role in cell biology from the first to the last steps of the process.
Author contributions
LV-T and MJ-M conceived of the project and wrote the manuscript. DC-F, PTM, and EC performed the phylogenetic analysis of the fungi and the vacuolar proteases. DA-P, JH-G, and CH-R carried out docking and the bioinformatic analysis of proteins as well as the evolutive analysis. All authors contributed to the article and approved the submitted version.
Funding
This work was supported by SIP-IPN grants (20220742, 20220795, and 20221723).
Acknowledgments
DC-F, PT-M and EC appreciate the graduate scholarship awarded by CONACyT as well as the scholarship complements furnished by the SIP-IPN (BEIFI). The authors would like to thank BLfor proofreading the manuscript. MJ-M, CH-R, and LV-T are fellows of the Estímulos al Desempeño de los Investigadores (EDI-IPN) and Comisión de Operación y Fomento de Actividades Académicas (COFAA-IPN) programs.
Conflict of interest
The authors declare that the research was conducted in the absence of any commercial or financial relationships that could be construed as a potential conflict of interest.
Publisher’s note
All claims expressed in this article are solely those of the authors and do not necessarily represent those of their affiliated organizations, or those of the publisher, the editors and the reviewers. Any product that may be evaluated in this article, or claim that may be made by its manufacturer, is not guaranteed or endorsed by the publisher.
Supplementary material
The Supplementary Material for this article can be found online at: https://www.frontiersin.org/articles/10.3389/ffunb.2022.948477/full#supplementary-material
References
Abeliovich H., Klionsky D. J. (2001). Autophagy in yeast: Mechanistic insights and physiological function. microbiol. Mol. Biol. Rev. 65 (3), 463–479. doi: 10.1128/MMBR.65.3.463-479.2001
Aman Y., Schmauck-Medina T., Hansen M., Morimoto R. I., Simon A. K., Bjedov I., et al. (2021). Autophagy in healthy aging and disease. Nat. Aging 1 (8), 634–650. doi: 10.1038/s43587-021-00098-4
Azzopardi M., Farrugia G., Balzan R. (2017). Cell-cycle involvement in autophagy and apoptosis in yeast. Mech. Ageing Dev. 161, 211–224. doi: 10.1016/j.mad.2016.07.006
Baba M., Takeshige K., Baba N., Ohsumi Y. (1994). Ultrastructural analysis of the autophagic process in yeast: Detection of autophagosomes and their characterization. J. Cell Biol. 124 (6), 903–913. doi: 10.1083/jcb.124.6.903
Böhmer M., Colby T., Böhmer C., Bräutigam A., Schmidt J., Bölker M. (2007). Proteomic analysis of dimorphic transition in the phytopathogenic fungus ustilago maydis. Proteomics 7 (5), 675–685. doi: 10.1002/pmic.200600900
Bruggeman S. A., Horvath D. P., Fennell A. Y., Gonzalez-Hernandez J. L., Clay S. A. (2020). Teosinte (Zea mays ssp parviglumis) growth and transcriptomic response to weed stress identifies similarities and differences between varieties and with modern maize varieties. PloS One 15 (8), e0237715. doi: 10.1371/journal.pone.0237715
Bunk J., Prieto Huarcaya S., Drobny A., Dobert J. P., Walther L., Rose-John S., et al. (2021). Cathepsin d variants associated with neurodegenerative diseases show dysregulated functionality and modified α-synuclein degradation properties. Front. Cell Dev. Biol. 9. doi: 10.3389/fcell.2021.581805
Busto J. V., Wedlich-Söldner R. (2019). Integration through separation – the role of lateral membrane segregation in nutrient uptake. Front. Cell Dev. Biol. 7. doi: 10.3389/fcell.2019.00097
Castino R., Bellio N., Follo C., Murphy D., Isidoro C. (2010). Inhibition of PI3k class III-dependent autophagy prevents apoptosis and necrosis by oxidative stress in dopaminergic neuroblastoma cells. J. Toxicol. Sci. 117 (1), 152–162. doi: 10.1093/toxsci/kfq170
Cebollero E., Reggiori F. (2009). Regulation of autophagy in yeast saccharomyces cerevisiae. Biochim. Biophys. Acta 1793 (9), 1413–1421. doi: 10.1016/j.bbamcr.2009.01.008
Chung K. R. (2012). Stress response and pathogenicity of the necrotrophic fungal pathogen alternaria alternata. Scientifica, 2012, 635431. doi: 10.6064/2012/635431
Ciechomska I. A., Gabrusiewicz K., Szczepankiewicz A. A., Kaminska B. (2013). Endoplasmic reticulum stress triggers autophagy in malignant glioma cells undergoing cyclosporine a-induced cell death. Oncogene 32 (12), 1518–1529. doi: 10.1038/onc.2012.174
Cortez-Sánchez J. L., Cortés-Acosta E., Cueto-Hernández V. M., Reyes-Maldonado E., Hernández-Rodríguez C., Villa-Tanaca L., et al. (2018). Activity and expression of candida glabrata vacuolar proteases in autophagy-like conditions. FEMS Yeast Res. 18 (2), foy006. doi: 10.1093/femsyr/foy006
Cui L., Zhao H., Yin Y., Liang C., Mao X., Liu Y., et al. (2019). Function of Atg11 in non-selective autophagy and selective autophagy of candida albicans. Biochem. Bioph. Res. Commun. 516 (4), 1152–1158. doi: 10.1016/j.bbrc.2019.06.148
Di Y. Q., Han X. L., Kang X. L., Wang D., Chen C. H., Wang J. X., et al. (2021). Autophagy triggers CTSD (cathepsin d) maturation and localization inside cells to promote apoptosis. Autophagy 17 (5), 1170–1192. doi: 10.1080/15548627.2020.1752497
Dunaevsky Y. E., Popova V. V., Semenova T. A., Beliakova G. A., Belozersky M. A. (2014). Fungal inhibitors of proteolytic enzymes: Classification, properties, possible biological roles, and perspectives for practical use. Biochimie 101, 10–20. doi: 10.1016/j.biochi.2013.12.007
Fernandez J., Marroquin-Guzman M., Wilson R. A. (2014). Mechanisms of nutrient acquisition and utilization during fungal infections of leaves. Annu. Rev. Phytopathol. 52, 155–174. doi: 10.1146/annurev-phyto-102313-050135
Foster A. J., Ryder L. S., Kershaw M. J., Talbot N. J. (2017). The role of glycerol in the pathogenic lifestyle of the rice blast fungus magnaporthe oryzae. Environ. Microbiol. 19 (3), 1008–1016. doi: 10.1111/1462-2920.13688
Fu H., Chung K. R., Liu X., Li H. (2020). Aaprb1, a subtilsin-like protease, required for autophagy and virulence of the tangerine pathotype of alternaria alternata. Microbiol. Res. 240, 126537. doi: 10.1016/j.micres.2020.126537
Gallagher T., Gilliland G., Wang L., Bryan P. (1995). The prosegment-subtilisin BPN' complex: Crystal structure of a specific 'foldase'. Curr. Biol. 3 (9), 907–914. doi: 10.1016/S0969-2126(01)00225-8
Harhaji-Trajkovic L., Arsikin K., Kravic-Stevovic T., Petricevic S., Tovilovic G., Pantovic A., et al. (2012). Chloroquine-mediated lysosomal dysfunction enhances the anticancer effect of nutrient deprivation. Pharm. Res. 29 (8), 2249–2263. doi: 10.1007/s11095-012-0753-1
Ichimura Y., Kirisako T., Takao T., Satomi Y., Shimonishi Y., Ishihara N., et al. (2000). A ubiquitin-like system mediates protein lipidation. Nature 408 (6811), 488–492. doi: 10.1038/35044114
Ivory B. J., Smith H. M., Cabrera E., Robinson M. R., Sparks J. T., Solem A., et al. (2021). ATG8 is conserved between saccharomyces cerevisiae and psychrophilic, polar-collected fungi. MicroPubl. Biol. 2021 doi: 10.17912/micropub.biology.000446
Johns L. E., Goldman G. H., Ries L. N., Brown N. A. (2021). Nutrient sensing and acquisition in fungi: mechanisms promoting pathogenesis in plant and human hosts. Fungal Biol. Rev. 36, 1–14. doi: 10.1016/j.fbr.2021.01.002
John E., Singh K. B., Oliver R. P., Kan-Chun T. (2022). Transcription factor control of virulence in phytopathogenic fungi. Mol. Plant Pathol. 22 (7), 858–881. doi: 10.1111/mpp.13056
Juárez-Montiel M., Tesillo-Moreno P., Cruz-Angeles A., Soberanes-Gutiérrez V., Chávez-Camarillo G., Ibarra J. A., et al. (2018). Heterologous expression and characterization of the aspartic endoprotease Pep4um from ustilago maydis, a homolog of the human cathepsin d, an important breast cancer therapeutic target. Mol. Biol. Rep. 45 (5), 1155–1163. doi: 10.1007/s11033-018-4267-8
Kane P. M. (2006). The where, when, and how of organelle acidification by the yeast vacuolar h+-ATPase. Microbiol. Mol. Biol. Rev. 70 (1), 177–191. doi: 10.1128/MMBR.70.1.177-191.2006
Kerstens W., Van Dijck P. (2018). A Cinderella story: how the vacuolar proteases Pep4 and Prb1 do more than cleaning up the cell's mass degradation processes. Microb. Cell 5 (10), 438–443. doi: 10.15698/mic2018.10.650
Kirisako T., Ichimura Y., Okada H., Kabeya Y., Mizushima N., Yoshimori T., et al. (2000). The reversible modification regulates the membrane-binding state of Apg8/Aut7 essential for autophagy and the cytoplasm to vacuole targeting pathway. J. Cell Biol. 151 (2), 263–276. doi: 10.1083/jcb.151.2.263
Kohmoto K., Itoh Y., Shimomura N., Kondoh Y., Otani H., Kodama M., et al. (1993). Isolation and biological activities of two host-specific toxins from the tangerine pathotype of alternaria alternata. Phytopathology 83, 495–502.
Kojima S., Deguchi M., Miura K. I. (1999). Involvement of the c-terminal region of yeast proteinase b inhibitor 2 in its inhibitory action. J. Mol. Biol. 286 (3), 775–785. doi: 10.1006/jmbi.1998.2498
Kominami E., Hashida S., Khairallah E. A., Katunuma N. (1983). Sequestration of cytoplasmic enzymes in an autophagic vacuole-lysosomal system induced by injection of leupeptin. J. Biol. Chem. 258 (10), 6093–6100. doi: 10.1016/S0021-9258(18)32377-9
Lanver D., Müller A. N., Happel P., Schweizer G., Haas F. B., Franitza M., et al. (2018). The biotrophic development of ustilago maydis studied by RNA-seq analysis. Plant Cell 30 (2), 300–323. doi: 10.1105/tpc.17.00764
Leadsham J. E., Miller K., Ayscough K. R., Colombo S., Martegani E., Sudbery P., et al. (2009). Whi2p links nutritional sensing to actin-dependent ras-cAMP-PKA regulation and apoptosis in yeast. J. Cell Sci. 122 (Pt 5), 706–715. doi: 10.1242/jcs.042424
Levine B., Klionsky D. J. (2004). Development by self-digestion: molecular mechanisms and biological functions of autophagy. Dev. Cell 6 (4), 463–477. doi: 10.1016/s1534-5807(04)00099-1
Lin C. H., Yang S. L., Chung K. R. (2011). Cellular responses required for oxidative stress tolerance, colonization, and lesion formation by the necrotrophic fungus alternaria alternata in citrus. Curr. Microbiol. 62 (3), 807–815. doi: 10.1007/s00284-010-9795-y
Liu K., Sutter B. M., Tu B. P. (2021). Autophagy sustains glutamate and aspartate synthesis in saccharomyces cerevisiae during nitrogen starvation. Nat. Commun. 12 (1), 57. doi: 10.1038/s41467-020-20253-6
Liu X. H., Zhao Y. H., Zhu X. M., Zeng X. Q., Huang L. Y., Dong B., et al. (2017). Autophagy-related protein MoAtg14 is involved in differentiation, development and pathogenicity in the rice blast fungus magnaporthe oryzae. Sci. Rep. 7, 40018. doi: 10.1038/srep40018
Madrigal-Aguilar D. A., Gonzalez-Silva A., Rosales-Acosta B., Bautista-Crescencio C., Ortiz-Álvarez J., Escalante C. H., et al. (2022). Antifungal activity of fibrate-based compounds and substituted pyrroles that inhibit the enzyme 3-hydroxy-methyl-glutaryl-coA reductase of candida glabrata (CgHMGR), thus decreasing yeast viability and ergosterol synthesis. Microbiol. Spectr. 10 (2), e0164221. doi: 10.1128/spectrum.01642-21
Marroquin-Guzman M., Sun G., Wilson R. A. (2017). Glucose-ABL1-TOR signaling modulates cell cycle tuning to control terminal appressorial cell differentiation. PloS Genet. 13 (1), e1006557. doi: 10.1371/journal.pgen.1006557
Matei A., Doehlemann G. (2016). Cell biology of corn smut disease-ustilago maydis as a model for biotrophic interactions. Curr. Opin. Microbiol. 34, 60–66. doi: 10.1016/j.mib.2016.07.020
Meijer A. J., Codogno P. (2009). Autophagy: Regulation and role in disease. Crit. Rev. Clin. Lab. Sci. 46 (4), 210–240. doi: 10.1080/10408360903044068
Mendoza H., Perlin M. H., Schirawski J. (2020). Mitochondrial inheritance in phytopathogenicfungi—everything is known, or is it?. Int J Mol Sci. 21 (11), 3883. doi: 10.3390/ijms21113883
Mercado-Flores Y., Hernández-Rodríguez C., Ruiz-Herrera J., Villa-Tanaca L. (2003). Proteinases and exopeptidases from the phytopathogenic fungus ustilago maydis. Mycologia 95 (2), 327–339. doi: 10.2307/3762044
Nadal M., Garcia-Pedrajas M. D., Gold S. E. (2010). The snf1 gene of ustilago maydis acts as a dual regulator of cell wall degrading enzymes. Phytopathology 100 (12), 1364–1372. doi: 10.1094/PHYTO-01-10-0011
Nadal M., Gold S. E. (2010). The autophagy genes atg8 and atg1 affect morphogenesis and pathogenicity in ustilago maydis. Mol. Plant Pathol. 11 (4), 463–478. doi: 10.1111/j.1364-3703.2010.00620.x
Nehls U., Plassard C. (2018). Nitrogen and phosphate metabolism in ectomycorrhizas. N. Phytol. 220 (4), 1047–1058. doi: 10.1111/nph.15257
Noda T., Ohsumi Y. (1998). Tor, a phosphatidylinositol kinase homologue, controls autophagy in yeast. J. Biol. Chem. 273 (7), 3963–3966. doi: 10.1074/jbc.273.7.3963
Oh Y., Donofrio N., Pan H., Coughlan S., Brown D. E., Meng S., et al. (2008). Transcriptome analysis reveals new insight into appressorium formation and function in the rice blast fungus magnaporthe oryzae. Genome Biol. 9 (5), R85. doi: 10.1186/gb-2008-9-5-r85
Oliva O., Réz G., Pálfia Z., Fellinger E. (1992). Dynamics of vinblastine-induced autophagocytosis in murine pancreatic acinar cells: Influence of cycloheximide post-treatments. Exp. Mol. Path. 56 (1), 76–86. doi: 10.1016/0014-4800(92)90025-7
Osés-Ruiz M., Talbot N. J. (2017). Cell cycle-dependent regulation of plant infection by the rice blast fungus magnaporthe oryzae. Commun. Integr. Biol. 10 (5-6), e1372067. doi: 10.1080/19420889.2017.1372067
Parzych K. R., Klionsky D. J. (2019). Vacuolar hydrolysis and efflux: current knowledge and unanswered questions. Autophagy 15 (2), 212–227. doi: 10.1080/15548627.2018.1545821
Pratt P. L., Bryce J. H., Stewart G. G. (2007). The yeast vacuole - a scanning electron microscopy during high gravity wort fermentations. J. Inst. Brew 113, 55–60. doi: 10.1002/j.2050-0416.2007.tb00256.x
Ravikumar B., Vacher C., Berger Z., Davies J. E., Luo S., Oroz L. G., et al. (2004). Inhibition of mTOR induces autophagy and reduces toxicity of polyglutamine expansions in fly and mouse models of huntington disease. Nat. Genet. 36 (6), 585–595. doi: 10.1038/ng1362
Saitoh H., Fujisawa S., Ito A., Mitsuoka C., Berberich T., Tosa Y., et al. (2009). SPM1 encoding a vacuole-localized protease is required for infection-related autophagy of the rice blast fungus magnaporthe oryzae. FEMS Microbiol. Lett. 300 (1), 115–121. doi: 10.1111/j.1574-6968.2009.01769.x
Sarkar S., Davies J. E., Huang Z., Tunnacliffe A., Rubinsztein D. C. (2007). Trehalose, a novel mTOR-independent autophagy enhancer, accelerates the clearance of mutant huntingtin and alpha-synuclein. J.Biol. Chem. 282 (8), 5641–5652. doi: 10.1074/jbc.M609532200
Sarkar S., Floto R. A., Berger Z., Imarisio S., Cordenier A., Pasco M., et al. (2005). Lithium induces autophagy by inhibiting inositol monophosphatase. J. Cell Biol. 170 (7), 1101–1111. doi: 10.1083/jcb.200504035
Schmitz L., McCotter S., Kretschmer M., Kronstad J. W., Heimel K. (2018). Transcripts and tumors: Regulatory and metabolic programming during biotrophic phytopathogenesis. F1000Research. 7, F1000 Faculty Rev-1812 doi: 10.12688/f1000research.16404.1
Sepúlveda-González M. E., Parra-Ortega B., Betancourt-Cervantes Y., Hernández-Rodríguez C., Xicohtencatl-Cortes J., Villa-Tanaca L. (2016). Vacuolar proteases from candida glabrata: Acid aspartic protease PrA, neutral serine protease PrB and serine carboxypeptidase CpY. The nitrogen source influences their level of expression. Rev. Iberoam. Micol. 33 (1), 26–33. doi: 10.1016/j.riam.2014.10.005
Shashkova S., Welkenhuysen N., Hohmann S. (2015). Molecular communication: Crosstalk between the Snf1 and other signaling pathways. FEMS Yeast Res. 15 (4), fov026. doi: 10.1093/femsyr/fov026
Shimamura S., Miyazaki T., Tashiro M., Takazono T., Saijo T., Yamamoto K., et al. (2019). Autophagy-inducing factor Atg1 is required for virulence in the pathogenic fungus candida glabrata. Front. Microbiol. 10. doi: 10.3389/fmicb.2019.00027
Shi H., Meng S., Qiu J., Wang C., Shu Y., Luo C., et al. (2021). MoWhi2 regulates appressorium formation and pathogenicity via the MoTor signalling pathway in magnaporthe oryzae. Mol. Plant Pathol. 22 (8), 969–983. doi: 10.1111/mpp.13074
Slusarewicz P., Xu Z., Seefeld K., Haas A., Wacker W. T. (1997). I2B is a small cytosolic protein that participates in vacuole fusion. Proc. Natl. Acad. Sci. U.S.A. 94 (11), 5582–5587. doi: 10.1073/pnas.94.11.5582
Soberanes-Gutiérrez V., Juárez Montiel M., Olguín Rodríguez O., Hernández Rodríguez C., Ruiz Herrera J., Villa Tanaca L. (2015). The pep4 gene encoding proteinase a is involved in dimorphism and pathogenesis of ustilago maydis. Mol. Plant Pathol. 16 (8), 837–846. doi: 10.1111/mpp.12240
Soberanes-Gutiérrez C. V., Vázquez-Carrada M., López-Villegas E. O., Vega-Arreguín J. C., Villa-Tanaca L., Ruiz-Herrera J. (2019). Autophagosomes accumulation in the vacuoles of the fungus ustilago maydis and the role of proteases in their digestion. FEMS Microbiol. Lett. 366 (10), fnz108. doi: 10.1093/femsle/fnz108
Takeshige K., Baba M., Tsuboi S., Noda T., Ohsumi Y. (1992). Autophagy in yeast demonstrated with proteinase-deficient mutants and conditions for its induction. J. Cell Biol. 119 (2), 301–311. doi: 10.1083/jcb.119.2.301
Tang K., Lv W., Zhang Q., Zhou C. (2020). Coding the α-subunit of SNF1 kinase, Snf1 is required for the conidiogenesis and pathogenicity of the alternaria alternata tangerine pathotype. Fungal Biol. 124 (6), 562–570. doi: 10.1016/j.funbio.2020.02.008
Tanida I., Minematsu-Ikeguchi N., Ueno T., Kominami E. (2005). Lysosomal turnover, but not a cellular level, of endogenous LC3 is a marker for autophagy. Autophagy 1 (2), 84–91. doi: 10.4161/auto.1.2.1697
Tatti M., Motta M., Di Bartolomeo S., Scarpa S., Cianfanelli V., Cecconi F., et al. (2012). Reduced cathepsins b and d cause impaired autophagic degradation that can be almost completely restored by overexpression of these two proteases in sap c-deficient fibroblasts. Hum. Mol. Gen. 21 (23), 5159–5173. doi: 10.1093/hmg/dds367
Teter S. A., Klionsky D. J. (2000). Transport of proteins to the yeast vacuole: autophagy, cytoplasm-to-vacuole targeting, and role of the vacuole in degradation. Semin. Cell Dev. Biol. 11 (3), 173–179. doi: 10.1006/scdb.2000.0163
Tyler J. K., Johnson J. E. (2018). The role of autophagy in the regulation of yeast life span. Ann. N. Y. Acad. Sci. 1418 (1), 31–43. doi: 10.1111/nyas.13549
Veneault-Fourrey C., Barooah M., Egan M., Wakley G., Talbot N. J. (2006). Autophagic fungal cell death is necessary for infection by the rice blast fungus. Science 312 (5773), 580–583. doi: 10.1126/science.1124550
Veneault-Fourrey C., Talbot N. J. (2007). Autophagic cell death and its importance for fungal developmental biology and pathogenesis. Autophagy 3 (2), 126–127. doi: 10.4161/auto.3529
Wallen R. M., Richardson K., Furnish M., Mendoza H., Dentinger A., Khanal S., et al. (2021). Hungry for sex: Differential roles for ustilago maydis b locus components in haploid cells vis à vis nutritional availability. J. Fungi (Basel Switzerland) 7 (2), 135. doi: 10.3390/jof7020135
Wilms T., Swinnen E., Eskes E., Dolz-Edo L., Uwineza A., Van Essche R., et al. (2017). The yeast protein kinase Sch9 adjusts V-ATPase assembly/disassembly to control pH homeostasis and longevity in response to glucose availability. PloS Genet. 13 (6), e1006835. doi: 10.1371/journal.pgen.1006835
Wilson R., Talbot N. (2009). Under pressure: investigating the biology of plant infection by magnaporthe oryzae. Nat. Rev. Microbiol. 7, 185–195. doi: 10.1038/nrmicro2032
Wu P. C., Chen Y. K., Yago J. I., Chung K. R. (2021). Peroxisomes implicated in the biosynthesis of siderophores and biotin, ell all integrity, autophagy, and response to hydrogen peroxide in the citrus pathogenic fungus alternaria alternata. Front. Microbiol. 12. doi: 10.3389/fmicb.2021.645792
Wu Y. C., Wu W. K., Li Y., Yu L., Li Z. J., Wong C. C., et al. (2009). Inhibition of macroautophagy by bafilomycin A1 lowers proliferation and induces apoptosis in colon cancer cells. Biochem. Bioph. Res. Commun. 382 (2), 451–456. doi: 10.1016/j.bbrc.2009.03.051
Yang Y. P., Hu L. F., Zheng H. F., Mao C. J., Hu W. D., Xiong K. P., et al. (2013). Application and interpretation of current autophagy inhibitors and activators. Acta Pharm. Sin. 34 (5), 625–635. doi: 10.1038/aps.2013.5
Zhou X., Li J., Tang N., Xie H., Fan X., Chen H., et al. (2021). Genome-wide analysis of nutrient signaling pathways conserved in arbuscular mycorrhizal fungi. Microorganisms 9, 1557. doi: 10.3390/microorganisms9081557
Keywords: phytopathogenic fungus, autophagy, ATG8 and TOR, vacuolar proteases PrA and PrB, autophagic body degradation
Citation: Juárez-Montiel M, Clark-Flores D, Tesillo-Moreno P, de la Vega-Camarillo E, Andrade-Pavón D, Hernández-García JA, Hernández-Rodríguez C and Villa-Tanaca L (2022) Vacuolar proteases and autophagy in phytopathogenic fungi: A review. Front. Fungal Biol. 3:948477. doi: 10.3389/ffunb.2022.948477
Received: 19 May 2022; Accepted: 11 October 2022;
Published: 26 October 2022.
Edited by:
Antonio Moretti, National Research Council (CNR), ItalyReviewed by:
Patricia Xander, Federal University of São Paulo, BrazilMarcelo Afonso Vallim, Federal University of São Paulo, Brazil
Copyright © 2022 Juárez-Montiel, Clark-Flores, Tesillo-Moreno, de la Vega-Camarillo, Andrade-Pavón, Hernández-García, Hernández-Rodríguez and Villa-Tanaca. This is an open-access article distributed under the terms of the Creative Commons Attribution License (CC BY). The use, distribution or reproduction in other forums is permitted, provided the original author(s) and the copyright owner(s) are credited and that the original publication in this journal is cited, in accordance with accepted academic practice. No use, distribution or reproduction is permitted which does not comply with these terms.
*Correspondence: Lourdes Villa-Tanaca, mvillat@ipn.mx; lourdesvillatanaka@gmail.com