In-planta Gene Targeting in Barley Using Cas9 With and Without Geminiviral Replicons
- John Innes Centre, Norwich Research Park, Norwich, United Kingdom
Advances in the use of RNA-guided Cas9-based genome editing in plants have been rapid over the last few years. A desirable application of genome editing is gene targeting (GT), as it allows a wide range of precise modifications; however, this remains inefficient especially in key crop species. Here, we describe successful, heritable gene targeting in barley at the target site of Cas9 using an in-planta strategy but fail to achieve the same using a wheat dwarf virus replicon to increase the copy number of the repair template. Without the replicon, we were able to delete 150 bp of the coding sequence of our target gene whilst simultaneously fusing in-frame mCherry in its place. Starting from 14 original transgenic plants, two plants appeared to have the required gene targeting event. From one of these T0 plants, three independent gene targeting events were identified, two of which were heritable. When the replicon was included, 39 T0 plants were produced and shown to have high copy numbers of the repair template. However, none of the 17 lines screened in T1 gave rise to significant or heritable gene targeting events despite screening twice the number of plants in T1 compared with the non-replicon strategy. Investigation indicated that high copy numbers of repair template created by the replicon approach cause false-positive PCR results which are indistinguishable at the sequence level to true GT events in junction PCR screens widely used in GT studies. In the successful non-replicon approach, heritable gene targeting events were obtained in T1, and subsequently, the T-DNA was found to be linked to the targeted locus. Thus, physical proximity of target and donor sites may be a factor in successful gene targeting.
Introduction
Genome editing has exploded in recent years due to advances in programmable nucleases which allow a double-stranded DNA break to be created at a predefined locus. First on the scene were Zinc-finger nucleases (Kim et al., 1996) followed by transcription activator-like effector nucleases (TALENS) (Christian et al., 2010) and, more recently, clustered regularly interspaced short palindromic repeats (CRISPR) systems, especially the SpCas9 (Jinek et al., 2012) which was the first CRISPR nuclease reported to function in plants (Feng et al., 2013; Li et al., 2013; Nekrasov et al., 2013; Shan et al., 2013; Xie and Yang, 2013). Although insertion of exogenously supplied DNA into plant genomes has been possible for many years via Agrobacterium-mediated transformation or physical delivery, location was impossible to control precisely. Some success is reported inserting DNA in a precise manner by homologous recombination in rice without creating a double-strand break (DSB) at the target site, although it was necessary to use a positive/negative selection system (Terada et al., 2002) which was later shown to produce no successful modifications in barley (Horvath et al., 2017). The value of creating a DSB at the target site to initiate DNA repair and facilitate insertion by homologous recombination was shown early on in plants with the non-programmable I-SceI meganuclease (Fauser et al., 2012), so it was a natural progression to repurpose Cas9 for precise insertional modifications. Many DSBs are repaired by non-homologous end-joining (NHEJ) mechanisms which are error prone but shown to be capable of inserting an exogenously supplied DNA template at the break point in plants (Salomon and Puchta, 1998; Chilton and Que, 2003; Tzfira et al., 2003; Lee et al., 2019) although precision is likely to be compromised by indels created at the spliced junctions as well as issues controlling orientation, truncation and concatenation.
Targeted DSBs can be introduced very efficiently and with great precision into plant genomes using RNA-guided Cas9, and this has made it facile over recent years to produce gene knockouts by the introduction of indels and larger deletions due to the error-prone nature of NHEJ. Many reports now exist describing single and multiple gene knockouts at efficiencies often approaching 100% although the precise nature of the edit is often not possible to predict. Lesions typically lead to a shift in reading frame and a premature stop codon. Base and prime editing technologies (Komor et al., 2016; Gaudelli et al., 2017; Anzalone et al., 2019) have partially addressed the precision issue allowing defined single base changes as well as short insertions and deletions, although larger precise changes such as adding an in-frame reporter fusion are unlikely to be possible in this way.
Gene targeting (GT) can be defined as the introduction of a precise predefined modification into a plant genome, either an insertion, deletion or replacement via the introduction of a supplied repair template using homology-dependent recombination (HDR) and usually a DSB at the target site. By making available a repair template containing the required modification flanked by sequence homologous to each side of the DSB, a precise change can be introduced into the genome. This change can be either small, for example, a single amino acid conversion (Budhagatapalli et al., 2015; Sun et al., 2016; Svitashev et al., 2016; Wolter et al., 2018; Danilo et al., 2019; Wolter and Puchta, 2019), or large such as the in-frame insertion of a reporter gene (Zhao et al., 2016; Wang et al., 2017; Miki et al., 2018).
Whilst GT is able to address both large and small precise modifications, it is usually much harder to achieve than knockout, and so researchers have sought ways in which rare events can be screened for easily and means to boost the frequency at which they occur. Early GT efforts in crops have focussed on creating a precise change resulting in resistance to an herbicide or antibiotic which can then be used to select for resistant plants containing the desired GT event. ALS (acetolactate synthase) is a plant gene essential in the production of branched chain amino acids that is a target for inhibitors used as herbicides which has been extensively used in plants for GT experiments (Svitashev et al., 2015, 2016; Sun et al., 2016; Wolter et al., 2018; Danilo et al., 2019). Sometimes, a visual marker has been used in the screen such as insertion of a 35s promoter upstream of ANT1 leading to a purple phenotype (Cermak et al., 2015), or restoration of gl1 leading to trichome production in Arabidopsis (Hahn et al., 2018). This approach, however, means that modification is restricted to genes which allow such a selectable or visible phenotype, which many editing projects will not.
Many crop plants may only be transformed at efficiencies of a few percent or less, which, when combined with the low efficiency of GT, makes regeneration of T0 gene targeted plants hugely labour intensive or just inconceivable. One way around this is to adopt an in-planta strategy whereby just a few primary transgenics containing the editing reagents are created, but the numbers required to retrieve the rare GT events are generated by the plants themselves through the normal process of flowering and seed production (Fauser et al., 2012; Schiml et al., 2014, 2017). Each progeny plant may give rise to successful GT events, perhaps just as somatic sectors, but these can enter the germline and prove to be heritable in subsequent generations. In this approach, all the editing reagents can be included on a single T-DNA with a selection cassette to allow transgenic production, a nuclease programmed to create a DSB at the target site and a repair template containing the desired modification with flanking sequence homologous to each side of the target site DSB. Recognition sequences for the nuclease can also be added to the ends of the repair template to allow cutting and its transfer to the target site (Schiml et al., 2014; Zhao et al., 2016). Here, the screen can be based on the genotype rather than the phenotype, with plants containing the required edits being detected by PCR for example. A widely adopted approach is to PCR screen using one primer within the modified region of the repair template and the second primer outside of the repair template in the sequence flanking the target site. In this way, the PCR must cross the junction where the repair template stops, and the flanking genomic sequence begins.
It has been suggested that one major constraint on successful GT is the availability of repair template sequence at the correct time and in sufficient quantity for it to be incorporated as intended. In order to address this, Geminivirus replicons have been utilised (Baltes et al., 2014; Butler et al., 2016; Gil-Humanes et al., 2017; Wang et al., 2017; Dahan-Meir et al., 2018; Hahn et al., 2018; Vu et al., 2020), simultaneously pushing plant cells into more of an S phase-like state where HDR repair occurs more frequently and by replicating to high copy numbers providing many copies of the repair template to the target site. Here, the coat and movement protein section of the viral genome can be replaced by the repair template and then supplied in linear form to the plant on a T-DNA for Agrobacterium-mediated delivery. Once within the plant cell, the viral REP proteins are expressed leading to rolling circle replication and many copies of the repair template. Cas9 can also be delivered on the same T-DNA allowing simultaneous DSB at the target site and production of large quantities of the repair template. This approach has been most successful in tomato (Cermak et al., 2015; Dahan-Meir et al., 2018; Vu et al., 2020) but has also been described in wheat (Gil-Humanes et al., 2017), rice (Wang et al., 2017) and potato (Butler et al., 2016) although Arabidopsis appeared to be recalcitrant to any GT benefits (Hahn et al., 2018).
To date, there have been two reports of GT in barley: one a transient single amino acid conversion of a GFP transgene to YFP (Budhagatapalli et al., 2015) and the second a stable modification of a non-functional hptII transgene to a functional form (Watanabe et al., 2016). The former was identified in epidermal cells and the latter was one-sided GT events—one side of the repair was by HDR and the other by NHEJ. Our aim was to achieve heritable Cas9 GT in barley which would modify a locus of interest that was not a transgene and could be selected genotypically; thus, we chose to create a partial deletion of a native barley gene of interest, simultaneously fusing an in-frame reporter to the remaining part. To keep the number of transgenics required to a minimum and to potentially make the approach suitable for genotypes more recalcitrant to transformation, we used an in-planta strategy and attempted to increase efficiency by incorporating the repair template within a Geminivirus replicon. We present efficiencies using strategies with and without inclusion of the replicon.
Materials and Methods
PCR and Sanger Sequencing of HORVU4Hr1G061310 Target Locus for Indel Detection
PCR was done using 30 ng of genomic DNA as template, 400 nM of primers F4 and R5 (Supplementary Table 6), Qiagen 2× PCR Master Mix and a total reaction volume of 25 μl being completed by water. After initial denaturation at 94°C for 3 min, 40 cycles of 94°C for 30 s/58°C for 1 min/72°C for 45 s were performed. A final extension of 72°C for 5 min was given. One microliter of the cleaned product (see Sanger sequencing PCR products) was then used in separate Sanger reactions with both F4 and R5 primers. ABI chromatograms were compared with known WT chromatograms using the web-based ICE CRISPR analysis tool (www.synthego.com/products/bioinformatics/crispr-analysis) to identify lines carrying indels at target sites A and B.
Sanger Sequencing of PCR Products
PCR reactions were prepared for Sanger sequencing by adding 10 units of exonuclease 1 and 1 unit of shrimp alkaline phosphatase to 10 μl of PCR reaction before incubation at 37°C for 30 min followed by 80°C for 10 min to inactivate the enzymes. One microliter of the cleaned product was used as sequencing template where the amplicon was 1 kb or less in size and 2 μl when over 1 kb. Sequencing reactions were in 10 μl volumes with 100 nM primer, 1.5 μl BigDye buffer and 1 μl BigDye 3.1, made up to 10 μl with water. After a denaturation step of 96°C for 2 min, 35 cycles of 96°C for 10 s/52°C for 15 s/60°C for 3 min were performed. Finally, reactions were held at 72°C for 1 min, sent for commercial data extraction and returned in the form of ABI files.
qPCR Assay for T-DNA (HptII) and Repair Template (mCherry) Copy Number Determination
Hydrolysis probe-based quantitative real-time PCR (qPCR) was used to determine copy number of the T-DNA (HptII) and repair template (mCherry) in transgenic barley lines. The reaction compared the Cq values of an HptII amplicon to a single-copy barley gene CO2 (Constans-like, AF490469) amplicon and the Cq values of an mCherry amplicon to a single-copy barley gene CO2 (Constans-like, AF490469) amplicon within FAM/VIC duplexed assays (see Supplementary Table 6). The reactions used Thermo ABGene Absolute qPCR Rox Mix (Cat. number AB1139) with the probes and primers at final concentrations of 200 nM (HptII and mCherry) and 100 nM (CO2). The assay contained 5 μl DNA solution and was optimised for DNA concentrations of 1–10 ng/μl (5–50 ng DNA in the assay). PCRs were carried out as 25 μl reactions in a Bio-Rad CFX96 machine (C1000 Touch, Bio-Rad, Hercules, CA, USA). The detectors used were FAM-TAMRA and VIC-TAMRA. The PCR cycling conditions were 95°C for 15 min (enzyme activation), 40 cycles of 95°C for 15 s, and 60°C for 60 s. Cq values were determined using CFX96 software (version 3.1), with Cq determination set to regression mode. Values obtained were used to calculate copy number according to published methods (Weng et al., 2004).
PCR Screening for GT
F1/R1 (left junction) and F2/R2 (right junction) primer sequences are given in Supplementary Table 6. Each left and right junction PCR reaction contained 30 ng genomic DNA template, 2.5 μl 10× buffer 1, 200 μM dNTPs, 200 nM primers, 0.625 units AmpliTaq Gold (Thermo Fisher, Waltham, MA, USA) and water to 25 μl. Reactions were cycled as follows: 95°C 10 min (enzyme activation), then 40 cycles of 95°C for 30 s/58°C for 30 s/72°C for 1 min. The final extension was at 72°C for 5 min. Amplicons were sequenced with the following primers (Supplementary Table 6): F1/R1 amplicon: Seq1, Seq2, Seq3 and Seq10; F2/R2 amplicon: Seq6, Seq7, Seq8, Seq9 and Seq1. Primers for the less sensitive but fully diagnostic F1/R3 PCR are given in Supplementary Table 6. Each reaction contained 30 ng genomic DNA template, 10 μl 5× GoTaq buffer, 1.5 mm MgCl2, 200 nM primers, 200 μM dNTPs, 5 units GoTaq DNA polymerase (Promega, Madison, WI, USA) and water to 50 μl. Reactions were cycled at 94°C for 3 min then 40 cycles of 94°C for 30 s/58°C for 30 s/72°C for 2 min and 30 s, before final extension at 72°C for 5 min. F1/R3 amplicons were sequenced with the following primers (Supplementary Table 6): Seq1, Seq2, Seq3, Seq4, Seq5, Seq6, Seq7, Seq8, and Seq10.
gDNA Prep and Quantification by Qubit
Genomic DNA was prepared from the leaves according to a published protocol (Edwards et al., 1991). Preps were quantified using the Qubit dsDNA HS Assay Kit (Thermo Fisher) according to the manufacturer's instructions and diluted to a concentration of 30 ng/μl.
Barley Transformation
Barley (cv. “Golden Promise”) was transformed by Agrobacterium tumefaciens-mediated transformation of immature embryos as described by Hinchliffe and Harwood (2019).
Construct Assembly
Constructs were assembled using previously described parts and methods (Lawrenson et al., 2015), except for the protospacers, repair template, and wheat dwarf virus (WDV) components. Protospacers, repair template, extended repair template, and replicon sequences are given in Supplementary Table 6. Protospacer, repair template, extended repair template, and replicon sequences were commercially synthesised as modules compatible with the parts and cloning methods previously described (Lawrenson et al., 2015). Sequence-confirmed constructs A, B, C, and D were transformed into Agrobacterium strain AGL1 (Lazo et al., 1991).
Crossing
Barley was crossed according to a published protocol (Thomas et al., 2019).
Chromosome Walking
A published PCR-based protocol (Wang et al., 2007) was used to determine sequences flanking the T-DNA borders. Primer sequences used are shown in Supplementary Table 6. SP2 products were cloned into pGEMT-Easy (Promega) and sequenced with M13 and M13R universal primers (Eurofins, Louisville, KY, USA). pGEMT-Easy, left and right T-DNA border sequences were identified in the reads showing that the remaining barley sequence represented the sequence flanking the T-DNA in the barley genome.
Results
GT Construct Design
In our design strategy, high-efficiency introduction of DSBs was considered important as the benefits of DSBs to GT have been reported (Fauser et al., 2012). As part of a gene knockout project, HORVU4Hr1G061310 was identified as being efficiently targeted by protospacers A and B when provided to barley plants simultaneously in the same DNA construct. Here, 18 T0 barley lines were created containing a construct with architecture according to a published work (Lawrenson et al., 2015) and screened by Sanger sequencing amplicons which spanned target sites A and B. Chromatograms from the 18 lines were compared with wild-type controls using the web-based ICE CRISPR analysis tool. Protospacer A was able to create indels in 9/18 (50%) of independent transgenic lines and protospacer B 16/18 (89%) of the same lines (Supplementary Table 7). Therefore, for our selected native barley target (HORVU4Hr1G061310), these two protospacers were used and allowed for a strategy to delete around 150 bp of the coding sequence of this single exon gene whilst simultaneously fusing in-frame mCherry in its place (Figures 1A–C). To maximise the chance of success, we decided to incorporate both guides into our design as two DSBs at the target site might be better than one. In the repair template, homology to the target site was maximised by continuing the right and left homology arm sequences fully up to the Cas9 cuts sites, i.e., 3 bp from the native PAM. This allowed omission of the PAM on the left arm and the protospacer on the right arm of the repair template, preventing the Cas9 from cutting within it, both before and after GT (Figures 1A,C). Target sequences (full protospacer and PAM) were included in the flanks of the repair template (Figure 2) to allow cutting and facilitate its incorporation into the target site. The repair template was added to the construct containing Cas9 and the guide A and B cassettes to arrive at construct A (Figure 2A) which was similar in architecture to a previous example shown to enable GT in Arabidopsis (Schiml et al., 2014). The predicted GT event is shown in Figure 1C.
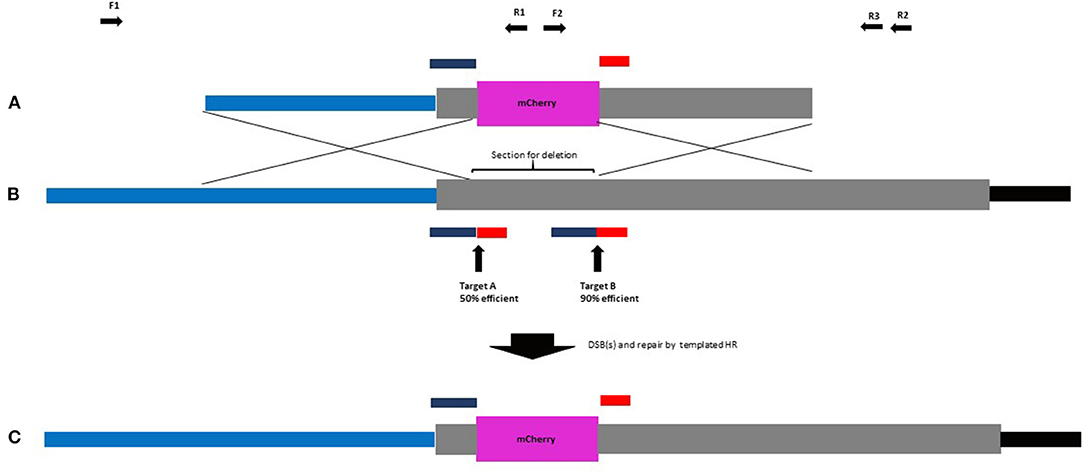
Figure 1. (A) Repair template, (B) target locus and (C) successful GT into the target locus. Promoter region of HORVU4Hr1G061310 in light blue, mCherry CDS in purple, HORVU4Hr1G061310 CDS in grey, and 3′ UTR in black. Protospacer sequences (A,B) in dark blue. Associated PAMs in red. Guide efficiency is shown as % of lines in which indels are detected. Complete protospacer/PAM sequences are absent from (A,C) to prevent cleavage by Cas9. A successful event leads to a partial deletion of the HORVU4Hr1G06131 CDS with the remainder being fused in-frame to mCherry. Forward (F) and reverse (R) screening primers are indicated as black horizontal arrows.
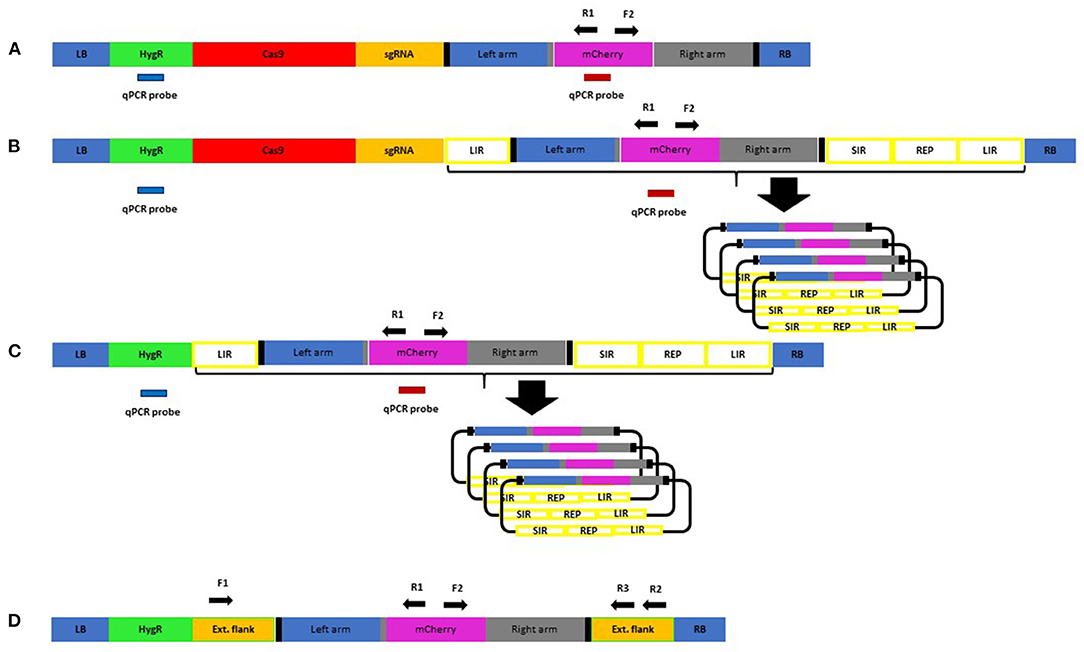
Figure 2. Constructs for plant transformation used in this study: RB, right border; LB, left border; HygR, hygromycin resistance cassette; Cas9 Hs, codon-optimised SpCas9 driven by Zm Ubiquitin promoter; sgRNA, guide RNA expression cassette for two guides (A,B); Left arm, left homology arm; mCherry, mCherry reporter CDS; Right arm, right homology arm; LIR, long intergenic region; SIR, short intergenic region; REP, replicase proteins; qPCR probe used for copy number determination. Thick black vertical bars indicate target sites for the Cas9/guides. Construct (A) is the basic GT version, and construct (B) is the same except the repair template is contained between replicon sequences. Construct (C) is the same as (B) but lacks the Cas9/gRNA and ability to introduce DSBs. Construct (D) has no Cas9/gRNA or replicon but has the repair template with extended homology arms. It was transformed into barley and used to establish a sensitive PCR assay. F1, R1, F2, R2, and R3 are PCR primers used for screening.
WDV is part of the Geminivirus family, whose members have been used in both dicotyledonous and monocotyledonous plants previously as replicons to deliver genome editing reagents and, in particular, the repair template for GT (Baltes et al., 2014; Butler et al., 2016; Gil-Humanes et al., 2017; Wang et al., 2017; Dahan-Meir et al., 2018; Hahn et al., 2018; Vu et al., 2020). The coat and movement protein coding sequence can be completely removed and replaced by a fragment of no maximally determined size whilst still retaining the ability to replicate within its host to high copy numbers after introduction by Agrobacterium or physical means. We used the basic template (LIR-SIR-REP-LIR) previously used with success (Gil-Humanes et al., 2017; Wang et al., 2017) but supplemented for our purposes by using the genome sequence from a strain of WDV isolated from barley (WDV-Bar [Hu]) (Ramsell et al., 2009). The WDV-Bar[Hu] version of LIR-SIR-REP-LIR was included in construct B (Figure 2B) such that it would allow rolling circle replication of the repair template already present in construct A. We chose not to include the Cas9 within the replicon such that it would replicate to a high copy number as no benefit was previously seen in GT experiments when this was done with other sequence-specific nucleases (Baltes et al., 2014). Only when the repair template was inserted into the replicon was GT boosted, suggesting that it was largely an increase in copy number of the donor and not a replicon-induced increase in DSB formation that was beneficial to GT. Previously, such replicons have often been shown functional in terms of replicative ability by using PCR to detect the circular replicating form of the linearly supplied unit. We chose to develop a qPCR copy number assay using amplicon/probe combinations in the repair template, hygromycin selection cassette (Figure 2) and a single-copy barley gene to enable quantification of replication in stable transgenic lines.
Construct C (Figure 2C) was identical to B other than lacking the Cas9 and sgRNA cassettes, so it was able to amplify the repair template but unable to induce the site-specific DSBs. This was to test the importance of targeted DSBs in GT which have been shown to be beneficial (Fauser et al., 2012) although not always essential (Terada et al., 2002).
Design of Assay for Detection of GT Events
Construct D (Figure 2D) was produced as a means of optimising the PCR screening strategy for GT detection, as high sensitivity and specificity would be vital due to the rarity of GT events and the expectation that we could be searching for somatic sectors which might represent a small proportion of the cells within leaf samples taken for analysis (Schiml et al., 2017). Somatic sectors can be inherited through the germline and also indicate active lines where further events are likely to occur. Construct D contains the repair template as found in constructs A, B, and C; however, the homology arms have been extended for a few 100 nucleotides with the native HORVU4Hr1G061310 genomic sequence to include the binding sites for the F1, R2, and R3 primers (Figure 2D; Supplementary Table 6). By creating a single-copy transgenic line with construct D, as determined by qPCR assay, a more realistic scenario to derive template for optimisation was possible than by using plasmid alone. In order to allow distinction from true GT events, polymorphisms at the junctions of the extended flanks and the homology arms were introduced which would not be present in the predicted true GT events (Supplementary Table 6). Various PCR conditions were tried and the best (see Methods) were found to work well with primer combinations F1/R1, F2/R2, and F1/R3. The most sensitive were found to be junction PCRs F1/R1 and F2/R2 which would identify GT events at either the left or right junction, respectively. By serially diluting 30 ng of construct D genomic DNA, considering the 5.3-Gbp haploid barley genome and the average weight of 650 Da per base pair, it was possible to calculate the number of template copies in each PCR reaction and thus determine the threshold sensitivity. This was found to be in the region of 40 copies for the F1/R1 primer pair (Supplementary Figure 1), so theoretically capable of identifying a somatic sector containing the same number of cells with a GT event. PCR with primers F1/R3, although covering the entire GT event over both left and right junctions, was less sensitive, presumably due to the greater amplicon size and the competitive tendency of the smaller WT allele to amplify and dominate the products (see Figure 3). The limit of detection for the F1/R3 amplicon was in the region of 1,000 template copies (data not shown). For this reason, it was decided to use the more sensitive F1/R1 and F2/R2 junction combinations for screening primary transgenics where small somatic GT sectors were likely.
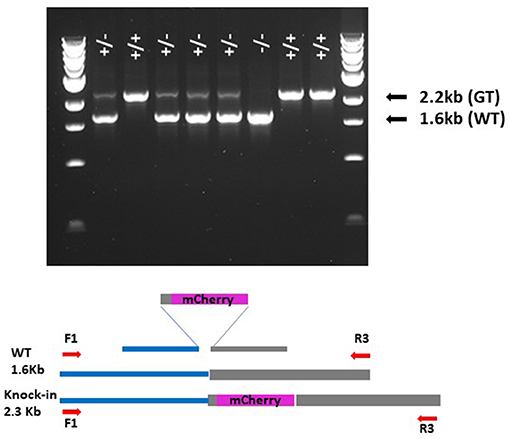
Figure 3. Gel showing segregation of GT event in 1826-8-1_A T2. Bands are the products of F1/R3 primers. + indicates GT allele; – indicates WT allele.
Production and Analysis of the T0 Generation
Barley cv. “Golden Promise” was transformed with constructs A, B, C, and D using Agrobacterium delivery, and selection of transgenic plants was done on hygromycin-containing media (Hinchliffe and Harwood, 2019). Two hundred immature embryos were inoculated each for constructs A, B, and C. One hundred embryos were inoculated for construct D. Construct A yielded 14 T0 lines (1826 prefix), construct B (2158 prefix) 39 T0 lines, construct C (2291 prefix) 17 lines, and construct D 8 lines. Transformation efficiencies for constructs A–D, respectively, were 7, 19.5, 8.8, and 8%. As the purpose of construct D lines was to optimise PCR conditions for screening, they were destructively harvested for genomic DNA once rooted in tissue culture. After qPCR copy number determinations for construct D lines, suitable genomic DNA template containing a single-copy T-DNA insertion was identified for PCR screen optimisation as described in the previous section. The 1826, 2158, and 2291 T0 lines were screened and scored using the F1/R1 and F2/R2 primer pairs as well as being assayed by qPCR for their HptII (T-DNA) and mCherry (repair template) copy numbers. These data are given in Supplementary Table 1 which show that in the case of construct A (1826) lines, the copy numbers of HptII and mCherry correspond as expected for two single-copy elements on a T-DNA. The 39 construct B (2158) lines, however, show an average of 7,575 copies of mCherry, whilst the HptII copy number remains largely one or two. This indicates that in many of the 2158 lines, rolling circle replication is occurring giving rise to huge numbers of repair template copies. Supplementary Table 1 also shows the presence or absence of F1/R1 and F2/R2 PCR products of the correct size, and for 1826 lines, 2/14 (14%) scored positive for both left and right junction PCRs, whilst for 2158 lines, 22/39 (56%) scored positive for the same two PCRs.
To check the identity and fidelity of these PCR products, F1/R1 and F2/R2 products were purified and Sanger sequenced for the lines 2158-9-1, 2158-14-1, 1826-5-2, and 1826-8-1 and found to be identical and, as expected, perfect for GT events (Supplementary Table 2). As expected, construct C lines (2291 prefix) also generated many copies of repair template, but unexpectedly, also produced correctly sized PCR products with primers F1/R1 and F2/R2 which are shown in Supplementary Table 1. In fact, 8/16 (50%) of the 2291 lines gave both left and right junction PCR products of the size indicative of a GT event and, furthermore, when purified and sequenced, gave exactly the same sequence as seen with the 1826 and 2158 lines. Looking at the relation between mCherry copy number and the presence/absence of F1/R1 and F2/R2 PCR products (Supplementary Table 1), it was apparent that high numbers of repair template and PCR success were linked. Whilst this could mean that increasing the number of repair template copies was causing GT, it could also indicate that false PCR positives were being triggered by the high number of repair templates produced by the replicon.
To test this latter idea, plasmid DNA containing the repair template was mixed with wild-type Golden Promise DNA (where GT could not have occurred) and F2/R2 PCR was performed. Initially, 30 ng of barley DNA (as used in all other screening PCRs described) was mixed with around 7.72 × 109 copies of repair template, and this resulted in the production of the 1,047-bp F2/R2 band. This plasmid was then titrated against the 30-ng wild-type barley DNA (representing 5,240 target site copies) to determine the minimum number of repair template copies per target site necessary to trigger the false positive when 30 ng of barley DNA was used as template. This is shown in Supplementary Figure 2 and was found to be in the region of 700 copies per target site, based on the 5.3-Gbp genome size and the average weight of a single base pair to be 650 Da. This result can be related to the qPCR copy number determinations for mCherry (repair template) in the replicon lines where the numbers in Supplementary Table 1 relate to copies per haploid genome or in other words per target site (there is one copy of HORVU4Hr1G061310 per haploid genome). Looking at Supplementary Table 1, it is evident that F1/R1 and F2/R2 products begin to appear in 2158 and 2291 lines at around 600 or 700 copies of mCherry per genome/target site, meaning it is likely that many of the PCR bands produced in replicon lines are false positives. This was further confirmed by sequencing a band from the plasmid titration test (Supplementary Figure 2) in the lane labelled 736641 which proved identical in sequence to the F2/R2 bands obtained for the 2158, 2291, and 1826 lines. Presumably, by increasing the number of repair template copies with the replicon, we had inadvertently also increased the likelihood of partial primer extension from within the repair template. For example, R1 could in one cycle of PCR be partially extended from within mCherry to somewhere in the left homology arm. After denaturation, the partially extended product would be free to anneal at its 5′ end with the homologous site in the target region (template switching) where it could then be extended beyond the position of the F1 primer binding site. F1 could then prime against this site and extend to produce double-stranded DNA of sequence identical to the predicted GT event and allow exponential amplification and production of the false positive.
The 1826 lines all had relatively low copy numbers of repair template (highest was 2), way below 600 per target site, and so our testing indicated that the lines 1826-8-1 and 1826-5-2 would be true positives. These were the only two 1826 lines with both F1/R1 and F2/R2 bands, indicating HR events at the left and right junctions, suggestive of a perfect GT event. Other lines such as 1826-3-1 showed an F2/R2 band but not F1/R1, which could be indicative of imperfect, one-sided GT events, for example, homologous recombination at the right junction but NHEJ at the left junction. Of course, false positives in the replicon lines could be masking true positives in the background, so the 39 individual 2158 lines were subject to F1/R3 PCR which was expected to be more specific due to the requirement for two template switches for false amplification to occur. The 14 individual 1826 lines also underwent the F1/R3 PCR; however, no T0 lines produced a band although this was unsurprising due to the low sensitivity of this large amplicon PCR. Accordingly, lines 1826-8-1 and 1826-5-2 were sown out for T1 screening due to being likely true positive GT lines, whilst 17 F1/R1 and F2/R2 positive 2158 lines were selected for T1 screening based on the assumption that some true positive GT events may be masked by false positives created via replicon amplification.
Analysis of the T1 Generation and Beyond
Because of the false-positive PCR issue, and to detect GT events and somatic sectors of significant size likely to become heritable, it was decided to screen T1 plants with the less sensitive F1/R3 primer pair. For each of the 17 selected T0 2158 lines, ~70 siblings were sown out, giving a total of around 1,200 from which no F1/R3 positives were identified. T0 line 1826-5-2 produced 228 seeds and all were sown and screened producing no F1/R3 positive band. T0 line 1826-8-1 was, however, more productive and yielded 467 seeds, and from these, 3 T1 plants produced a band of 2.2 kb indicative of the sought-after GT event as well as a second band of 1.6 kb corresponding to the wild-type allele. These 3 T1 siblings were designated 1826-8-1_A, 1826-8-1_B and 1826-8-1_C. The 2.2-kb band was purified for all three siblings and sequenced from end to end, showing that all were identical to the predicted GT event (Supplementary Table 2). The three sibling T1 plants were grown to maturity and harvested before sowing out seeds for T2 screening. Ninety-four individual 1826-8-1_A T2 siblings were screened and 75 gave the GT PCR product and 19 gave no band, corresponding to a 3:1 ratio (Supplementary Table 3), which is expected if the event was heterozygous in the T1 parent. Eight of these T2 siblings are shown in Figure 3 after F1/R3 PCR, where homozygous, heterozygous and wild-type plants can all be clearly seen. This strongly indicates that the GT event occurred either in the T0 generation or very early in T1, i.e., just after fertilisation. All 94 of the 1826-8-1_A T2 siblings contained two copies of the T-DNA (homozygous) as determined by qPCR, so homozygous GT plants (Figure 3) were selected for crossing to wild-type Golden Promise in order to segregate away the T-DNA from the GT event in F2.
T1 line 1826-8-1_B was also sown out for T2 screening, but out of 94 siblings, none screened positive for GT. This indicates that the event which was detected in T1 with F1/R3 primers and sequencing would, according to the sensitivity of the assay, represent a somatic sector of at least 1,000 cells, which was unable to pass through the germline into T2 plants and was therefore lost. All 94 of the 1826-8-1_B T2 siblings contained two copies of the T-DNA (homozygous).
T1 line 1826-8-1_C was sown out for T2 screening, and this time, 11/94 screened positive for GT. All 94 of the 1826-8-1_C T2 siblings contained two copies of the T-DNA (homozygous). Three of the GT-positive T2 siblings were designated 1826-8-1_C1, 1826-8-1_C2, and 1826-8-1_C3 and sown out again for T3 screening; 17/24 1826-8-1_C1 siblings (3:1), 16/24 1826-8-1_C2 siblings (3:1) and 24/24 1826-8-1_C3 siblings were positive for GT indicating that T2 parents were likely GT heterozygotes (C1, C2) or GT homozygotes (C3) (see Supplementary Table 3). This is consistent with a T1 parent which was a cellular mosaic of the GT event(s) which passed through the germline into T2 progeny at a subsequently lower fraction than the 75% expected from a heterozygous parent. Alternatively, GT could have occurred independently in the T2 lines 1826-8-1_C1, 1826-8-1_C2, and 1826-8-1_C3 to give the same T3 GT zygosity.
As with line 1826-8-1_A, all T2 siblings of 1826-8-1_C were homozygous for the T-DNA insertion, so it was not possible to lose the transgene without backcrossing to Golden Promise. As all 1826-8-1 lines share the same T-DNA insertion and the crossing was already underway for 1826-8-1_A, this was not done for 1826-8-1_C.
Linkage of the T-DNA and GT Event
All 19 F1 lines produced for the 1826-8-1_A × Golden Promise cross were heterozygous for the T-DNA and GT as determined by qPCR and F1/R3 PCR. In F2, 74/96 (3:1) siblings screened positive for GT as expected. All 96 were also tested for the presence of the transgene by qPCR which showed that all siblings containing the GT also contained the T-DNA and all GT free plants were also free of the T-DNA (Supplementary Table 3); in other words, the T-DNA and GT locus are linked. To see how close the GT and T-DNA were to each other, a chromosome walking technique was used to determine the flanking sequences of the T-DNA. BLAST search using the sequence obtained as query against the barley genome revealed the T-DNA to be located 4.23 Mb from the GT locus on chromosome 4 in line 1826-8-1 (Supplementary Table 4). The same chromosome walking was also done for line 1826-5-2 which was found to harbour the T-DNA on chromosome 7 (Supplementary Table 4).
Discussion
Figure 4 summarises the key findings described above for all plants analysed. Heritable GT was confined to line 1826-8-1 with the event in 1826-8-1_A occurring either in T0 or very early T1 and the 1826-8-1_C events occurring in T1 or T2. Additionally, a significant event leading to detection with the low sensitivity primer pair F1/R3 was recovered in 1826-8-1_B but lost by T2 so must have occurred in T1. This shows that the 1826-8-1 family tree had diverged before the origin of these independent GT events, and so for some reason, the line 1826-8-1 was relatively prolific in terms of GT. A comparable line 1826-5-2 showed somatic GT in T0 but did not go on to result in subsequent heritable GT. This may be related to the T-DNA containing the repair template being linked to the target site in 1826-8-1 but not in 1826-5-2. It was previously reported that if the repair template and target site were present on the same chromosome, then GT was around twice as frequent as when they were on different chromosomes (Fauser et al., 2012). Successful GT in line 1826-8-1 also makes sense in light of evidence that DNA repair by HDR using a sister chromatid template is common in barley (Vu et al., 2014). Being on the same chromosome is likely to impact on the physical proximity of target and donor site. It was recently reported in rice that using a Cas9-VirD2 fusion to direct the repair template to the target site had a beneficial effect on GT (Ali et al., 2020). It is also reported that the zygosity of the repair template has a similar impact (Puchta et al., 1995), where a homozygous transgene was 50% more likely to lead to intrachromosomal HR-based gene repair than if hemizygous. In line with this, all three 1826-8-1 T1 siblings of interest were homozygous for the T-DNA, whilst the overall T1 T-DNA inheritance in this line showed 3:1 segregation.
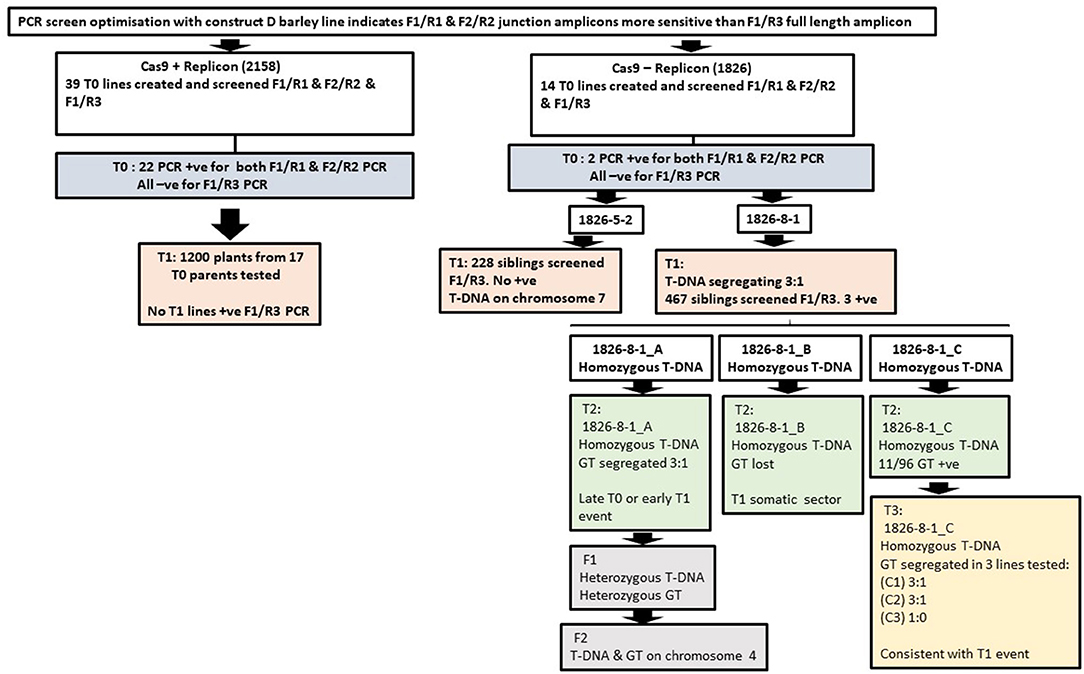
Figure 4. Key findings comparing in-planta GT with and without geminiviral replicon; 2158 are replicon lines (construct B) and 1826 lines are non-replicon lines (construct A).
A limitation of our study is the establishment of a causal role for Cas9 in the GT observed. Although we have no results from a control containing the repair template in the absence of Cas9 and guides, it has previously been reported (Horvath et al., 2017) that GT in barley did not occur from an estimated 6,838 independent transformation events where DSBs were not induced. In this GT report, successful targeting would have led to herbicide resistance allowing whole plant regeneration in tissue culture. Comparison of these 6,838 events to the 14 transformed T0 plants we created with construct A (1826 lines) does not necessarily indicate the benefit of induced DSBs as the number of chances for GT to occur in a multicellular regenerated plant containing the editing reagents is much greater than in a single transformation event that does not proceed beyond the single-cell stage in tissue culture. However, the benefits to GT of creating such targeted DSBs in plants are now extensively shown (Puchta et al., 1996; de Pater et al., 2009, 2013; Shukla et al., 2009; Townsend et al., 2009; Zhang et al., 2013; Endo et al., 2016).
We did not carry out any microscopy study to see if the in-frame mCherry HORVU4Hr1G061310 fusion created was functional as screening all 19 1826-8-1_A F1 plants produced showed that they still contained the T-DNA-based repair template (data not shown). Similarly, six GT-positive T3 plants from each of 1826-8-1_C1, 1826-8-1_C2, and 1826-8-1_C3 all contained the T-DNA-based repair template (data not shown). This repair template contains the promoter region, mCherry and much of the HORVU4Hr1G061310 CDS so may well have given a fluorescent signal despite not being integrated at the target locus by GT. This would not allow distinction of a signal arising from GT at the target locus and a signal from the repair template still located in the T-DNA.
We did not find F1/R3 detectable GT events in 2158 lines despite screening twice the number of plants in T1 compared with the 1826 lines; 2158 lines had very high copy numbers of repair template in T0 and in T1 where its amplification co-segregated with the T-DNA (Supplementary Table 5). Although the replicationally functional linear replicon form is thus able to pass through the germline successfully, we do not have any data to support whether donor amplification was occurring in cells giving rise to sex cells, and so a failure to achieve heritable replicon GT events could be a result of cell-specific type variation in replicon activity. With this in mind, it is still possible that the replicon had a positive effect on GT in leaf cells where rolling circle replication was detectable. However, titration of repair template plasmid against wild-type Golden Promise DNA in vitro indicated that the GT activity detected in T0 2158 lines was potentially a PCR artefact as junction PCR bands begin to appear at around 700 copies of repair template per target site, which is very close to the ratio seen in-planta with the replicon where the junction PCR began yielding product.
Future GT experiments utilising high copy numbers of repair template should be aware that such a junction PCR approach is liable to produce false-positive results and would benefit from strategies to prevent them. One way to do this may be to reduce the length of homology arms to a minimum, thus reducing the size of the region in which partial primer extension may occur before template switching during PCR. Whilst reducing the length of homology arms may result in a decrease in overall GT efficiency, relatively short homology arms of 196 and 74 bp have been shown to function in rice (Li et al., 2020). Another way to reduce false-positive junction PCR may be to simply increase the size of the amplicon by moving the primer in the flanking non-repair template region further out, which may in turn reduce the chances of a partially extended product being fully extended after template switching. However, larger amplicons are likely to reduce sensitivity, which could affect the detection of small somatic sectors, that may go on to be usefully heritable. A third way to reduce or remove false junction PCRs could be to do one round of full-length PCR with primers outside the repair template—F1/R3 in this case, and then to use the product as template for nested junction PCR—F1/R1 or F2/R2 in this case. The requirement for two template switching events to occur with F1/R3 in the production of a false positive may be sufficient to produce only true full-length GT products even with small somatic sectors which could then be amplified to detection point in a second round of nested junction PCR after template dilution. On the other hand, it is also possible that the increased sensitivity of nested junction PCR would also lead to false positives due to counteracting the reduced but not completely removed potential of the full-length F1/R3 PCR to switch template. Future GT experiments may benefit from trialling PCR screening methods thoroughly before implementation. It could be that junction PCR is a suitable method of screening where repair template copy number is low such as in our non-replicon approach. In our setup, plasmid/genomic DNA titration suggested that false positives were only triggered when the molar ratio of repair template:target site exceeded 600. This is currently mainly an issue with replicon and particle bombardment approaches.
Although we screened a greater number of T1 progeny (1,200 > 695) from a greater number of T0 parents (17 > 2) for the replicon (2158) compared with the non-replicon (1826) lines, we cannot be sure that this is a valid replicon/non-replicon comparison as indel formation at target sites may have been unequal for some reason. Using Cas9 has the potential disadvantage that indel formation is likely to mutate the “seed region” of the target site such that further DSBs are not possible as the relevant guide no longer matches the site. We know that both guides A and B were able to induce indels in 50 and 90% of T0 lines tested, respectively, often representing half of the alleles detected which may well indicate that many target sites would no longer be available for GT. The target sites in T0, T1, T2, etc. lines created could be sequenced to gain more insight into the remaining availability of WT target sites. As we only had one non-replicon (1826) T0 transgenic that yielded heritable GT events, a larger number of true GT events would need to be investigated in order to make a replicon/non-replicon comparison.
Recently, it has been shown in Arabidopsis that timing the occurrence of DSBs to the egg cell greatly increases GT efficiency (Miki et al., 2018; Wolter et al., 2018). Similarly, by using Cas12a instead of Cas9, GT efficiency was increased (Wolter and Puchta, 2019). Two features here address the potential lack in availability of WT target sites that may be shutting down DSB formation in our experiment. Firstly, restricting DSBs to egg cells would mean each female gamete has the potential for DSBs to occur and in turn undergo GT, rather than a reduced or non-existent fraction resulting from indels formed earlier during development under ubiquitous Cas9 expression. Secondly, Cas12a cuts outside of its seed region and would be expected to resist a certain amount of indel formation and may therefore keep creating DSBs for an increased length of time compared with Cas9, giving more potential for GT to occur. It will be interesting to see if the benefits to GT of egg cell-specific Cas12a can be translated to crops. It has been shown in tomato that the frequency of GT using Cas9 increased in line with temperature when it was carried out between 18 and 31°C, from around 1% at the lower temperature to around 5% at the higher temperature (Vu et al., 2020). Such a temperature regime may have been beneficial in our experiments although care would be needed to avoid a detrimental effect on fertility as high temperatures are known to have a negative impact in the latter stages of the barley life cycle (Jacott and Boden, 2020).
A previous report of in-planta GT in Arabidopsis (Hahn et al., 2018) found no beneficial effect from including the repair template within a replicon, whilst a single-copy repair template (similar to our construct A) gave rise to inheritable GT. However, this study investigated the progeny of just three primary transformant lines per DNA construct and may also suffer from indels shutting down target sites. In tomato, bean yellow dwarf virus-based replicons have been shown to result in heritable GT events (Cermak et al., 2015; Dahan-Meir et al., 2018; Vu et al., 2020). In one tomato study utilising an in-planta approach, it increased the percentage of inheritable T0 events from 8% without a replicon to 25% with a replicon (Dahan-Meir et al., 2018). Rice (Wang et al., 2017), wheat (Gil-Humanes et al., 2017) and potato (Butler et al., 2016) replicon/GT reports describe junction PCR/sequencing assays similar to our false-positive-prone F1/R1 and F2/R2 and no GT heritability. It could be that the benefits of replicons to heritable GT are restricted to certain plant species, which according to existing literature would include only tomato.
Our work in barley has extended what has previously been shown in this species as we created the first heritable true GT events at a native locus. However, we were unable to segregate away the editing reagents on the T-DNA, possibly due to an inadvertent selection for linkage. Whilst it may be possible to separate the two loci by searching for meiotic recombinants, this probably represents an unreasonable amount of work. Increasing the number of heritable GT events detected will probably allow the isolation of unlinked versions which would in turn be easier if GT efficiency was boosted in other ways, such as egg cell Cas12a expression. Additionally, a pooling strategy may enable more plants to be screened which should increase the numbers of GT events recovered.
Data Availability Statement
The raw data supporting the conclusions of this article will be made available by the authors, without undue reservation.
Author Contributions
TL designed the experiments, conducted the molecular work and analysis, and prepared the manuscript. AH carried out barley transformations. MC carried out the crossing programme. YM assisted with molecular analysis and crossing. WH contributed to the study design and manuscript preparation. All authors read and approved the final manuscript.
Funding
We acknowledge the support from the project Engineering Nitrogen Symbiosis for Africa (ENSA) currently supported through a grant to the University of Cambridge by the Bill and Melinda Gates Foundation and the Foreign, Commonwealth and Development Office (FCDO). The work was also supported by the UK Biotechnology and Biological Sciences Research Council (BBSRC) through grant BB/P013511/1.
Conflict of Interest
The authors declare that the research was conducted in the absence of any commercial or financial relationships that could be construed as a potential conflict of interest.
Acknowledgments
We thank JIC Horticultural Services for plant care.
Supplementary Material
The Supplementary Material for this article can be found online at: https://www.frontiersin.org/articles/10.3389/fgeed.2021.663380/full#supplementary-material
Supplementary Figure 1. Gel showing sensitivity obtained in F1/R1 PCR screen setup. Construct D was transformed into barley and DNA extracted from a regenerated plant (transgene copy number 1) and quantified by Qubit fluorescence. Serial dilutions were made of this DNA for subsequent PCR. The copy number of transgene D are shown for each lane. The limit of detection is around 40 copies of the target.
Supplementary Figure 2. Copies of repair template plasmid per target site. Thirty ng of wild type barley DNA was mixed with serial dilutions of plasmid DNA containing the repair template. The lane numbers represent the copies of repair template: target site ratio. Positive control was a construct D line. The 1047 bp band in lane 736641 was excised, purified and sequenced.
Supplementary Table 1. qPCR determined copy numbers of HptII (TDNA), mCherry (repair template), and presence/absence of junction PCR products (left: F1/R1; right: F2/R2) for T0 lines.
Supplementary Table 2. Sequences of GT events for lines 2158-9-1, 2158-14-1, 1826-5-2, and 1826-8-1 showing F1/R1 (T0), F2/R2 (T0), and F1/R3 (T1) products.
Supplementary Table 3. Table showing zygosity of T-DNA and GT events in interesting descendants of 1826-8-1 throughout the generations analysed in this study.
Supplementary Table 4. Flanking sequences of the T-DNA in lines 1826-8-1 and 1826-5-2.
Supplementary Table 5. T1 inheritance of the T-DNA and associated replicon activity.
Supplementary Table 6. Primer and probe sequences used in the study and sequences of construct components.
Supplementary Table 7. Analysis of T0 lines for indels at target sites A and B in HORVU4Hr1G061310.
References
Ali, Z., Shami, A., Sedeek, K., Kamel, R., Alhabsi, A., Tehseen, M., et al. (2020). Fusion of the Cas9 endonuclease and the VirD2 relaxase facilitates homology-directed repair for precise genome engineering in rice. Commun. Biol. 3:44. doi: 10.1038/s42003-020-0768-9
Anzalone, A. V., Randolph, P. B., Davis, J. R., Sousa, A. A., Koblan, L. W., Levy, J. M., et al. (2019). Search-and-replace genome editing without double-strand breaks or donor DNA. Nature 576, 149–157. doi: 10.1038/s41586-019-1711-4
Baltes, N. J., Gil-Humanes, J., Cermak, T., Atkins, P. A., and Voytas, D. F. (2014). DNA replicons for plant genome engineering. Plant Cell 26, 151–163. doi: 10.1105/tpc.113.119792
Budhagatapalli, N., Rutten, T., Gurushidze, M., Kumlehn, J., and Hensel, G. (2015). Targeted modification of gene function exploiting homology-directed repair of TALEN-mediated double-strand breaks in barley. Genes Genomes Genet. 5, 1857–1863. doi: 10.1534/g3.115.018762
Butler, N. M., Baltes, N. J., Voytas, D. F., and Douches, D. S. (2016). Geminivirus-mediated genome editing in potato (Solanum tuberosum L.) using sequence-specific nucleases. Front. Plant Sci. 7:1045. doi: 10.3389/fpls.2016.01045
Cermak, T., Baltes, N. J., Cegan, R., Zhang, Y., and Voytas, D. F. (2015). High-frequency, precise modification of the tomato genome. Genome Biol. 16:232. doi: 10.1186/s13059-015-0796-9
Chilton, M. D. M., and Que, Q. D. (2003). Targeted integration of T-DNA into the tobacco genome at double-stranded breaks: new insights on the mechanism of T-DNA integration. Plant Physiol. 133, 956–965. doi: 10.1104/pp.103.026104
Christian, M., Cermak, T., Doyle, E. L., Schmidt, C., Zhang, F., Hummel, A., et al. (2010). Targeting DNA double-strand breaks with TAL effector nucleases. Genetics 186, 757–761. doi: 10.1534/genetics.110.120717
Dahan-Meir, T., Filler-Hayut, S., Melamed-Bessudo, C., Bocobza, S., Czosnek, H., Aharoni, A., et al. (2018). Efficient in planta gene targeting in tomato using geminiviral replicons and the CRISPR/Cas9 system. Plant J. 95, 5–16. doi: 10.1111/tpj.13932
Danilo, B., Perrot, L., Mara, K., Botton, E., Nogue, F., and Mazier, M. (2019). Efficient and transgene-free gene targeting using Agrobacterium-mediated delivery of the CRISPR/Cas9 system in tomato. Plant Cell Rep. 38, 459–462. doi: 10.1007/s00299-019-02373-6
de Pater, S., Neuteboom, L. W., Pinas, J. E., Hooykaas, P. J. J., and van der Zaal, B. J. (2009). UN-induced mutagenesis and gene-targeting in Arabidopsis through Agrobacterium-mediated floral dip transformation. Plant Biotechnol. J. 7, 821–835. doi: 10.1111/j.1467-7652.2009.00446.x
de Pater, S., Pinas, J. E., Hooykaas, P. J. J., and van der Zaal, B. J. (2013). ZFN-mediated gene targeting of the Arabidopsis protoporphyrinogen oxidase gene through Agrobacterium-mediated floral dip transformation. Plant Biotechnol. J. 11, 510–515. doi: 10.1111/pbi.12040
Edwards, K., Johnstone, C., and Thompson, C. (1991). A simple and rapid method for the preparation of plant genomic dna for pcr analysis. Nucleic Acids Res. 19, 1349–1349. doi: 10.1093/nar/19.6.1349
Endo, M., Mikami, M., and Toki, S. (2016). Biallelic gene targeting in rice. Plant Physiol. 170, 667–677. doi: 10.1104/pp.15.01663
Fauser, F., Roth, N., Pacher, M., Ilg, G., Sanchez-Fernandez, R., Biesgen, C., et al. (2012). In planta gene targeting. Proc. Natl. Acad. Sci. U.S.A. 109, 7535–7540. doi: 10.1073/pnas.1202191109
Feng, Z., Zhang, B., Ding, W., Liu, X., Yang, D.-L., Wei, P., et al. (2013). Efficient genome editing in plants using a CRISPR/Cas system. Cell Res. 23, 1229–1232. doi: 10.1038/cr.2013.114
Gaudelli, N. M., Komor, A. C., Rees, H. A., Packer, M. S., Badran, A. H., Bryson, D. I., et al. (2017). Programmable base editing of A.T to G.C in genomic DNA without DNA cleavage. Nature 551, 464–471. doi: 10.1038/nature24644
Gil-Humanes, J., Wang, Y., Liang, Z., Shan, Q., Ozuna, C. V., Sanchez-Leon, S., et al. (2017). High-efficiency gene targeting in hexaploid wheat using DNA replicons and CRISPR/Cas9. Plant J. 89, 1251–1262. doi: 10.1111/tpj.13446
Hahn, F., Eisenhut, M., Mantegazza, O., and Weber, A. P. M. (2018). Homology-directed repair of a defective glabrous gene in arabidopsis with Cas9-based gene targeting. Front. Plant Sci. 9:424. doi: 10.3389/fpls.2018.00424
Hinchliffe, A., and Harwood, W. A. (2019). Agrobacterium-mediated transformation of barley immature embryos. Methods Mol. Biol. 1900, 115–126. doi: 10.1007/978-1-4939-8944-7_8
Horvath, M., Steinbiss, H. H., and Reiss, B. (2017). Gene targeting without DSB induction is inefficient in barley. Front. Plant Sci. 7:1973. doi: 10.3389/fpls.2016.01973
Jacott, C. N., and Boden, S. A. (2020). Feeling the heat: developmental and molecular responses of wheat and barley to high ambient temperatures. J. Exp. Bot. 71, 5740–5751. doi: 10.1093/jxb/eraa326
Jinek, M., Chylinski, K., Fonfara, I., Hauer, M., Doudna, J. A., and Charpentier, E. (2012). A programmable dual-RNA-guided DNA endonuclease in adaptive bacterial immunity. Science 337, 816–821. doi: 10.1126/science.1225829
Kim, Y. G., Cha, J., and Chandrasegaran, S. (1996). Hybrid restriction enzymes: zinc finger fusions to Fok I cleavage domain. Proc. Natl. Acad. Sci. U.S.A. 93, 1156–1160. doi: 10.1073/pnas.93.3.1156
Komor, A. C., Kim, Y. B., Packer, M. S., Zuris, J. A., and Liu, D. R. (2016). Programmable editing of a target base in genomic DNA without double-stranded DNA cleavage. Nature 533, 420–424. doi: 10.1038/nature17946
Lawrenson, T., Shorinola, O., Stacey, N., Li, C., Ostergaard, L., Patron, N., et al. (2015). Induction of targeted, heritable mutations in barley and Brassica oleracea using RNA-guided Cas9 nuclease. Genome Biol. 16:258. doi: 10.1186/s13059-015-0826-7
Lazo, G. R., Stein, P. A., and Ludwig, R. A. (1991). A DNA transformation-competent arabidopsis genomic library in agrobacterium. Bio-Technology 9, 963–967. doi: 10.1038/nbt1091-963
Lee, K., Eggenberger, A. L., Banakar, R., McCaw, M. E., Zhu, H., Main, M., et al. (2019). CRISPR/Cas9-mediated targeted T-DNA integration in rice. Plant Mol. Biol. 99, 317–328. doi: 10.1007/s11103-018-00819-1
Li, J.-F., Norville, J. E., Aach, J., McCormack, M., Zhang, D., Bush, J., et al. (2013). Multiplex and homologous recombination-mediated genome editing in Arabidopsis and Nicotiana benthamiana using guide RNA and Cas9. Nat. Biotechnol. 31, 688–691. doi: 10.1038/nbt.2654
Li, S. Y., Zhang, Y. X., Xia, L. Q., and Qi, Y. P. (2020). CRISPR-Cas12a enables efficient biallelic gene targeting in rice. Plant Biotechnol. J. 18, 1351–1353. doi: 10.1111/pbi.13295
Miki, D., Zhang, W., Zeng, W., Feng, Z., and Zhu, J.-K. (2018). CRISPR/Cas9-mediated gene targeting in Arabidopsis using sequential transformation. Nat. Commun. 9:1967. doi: 10.1038/s41467-018-04416-0
Nekrasov, V., Staskawicz, B., Weigel, D., Jones, J. D. G., and Kamoun, S. (2013). Targeted mutagenesis in the model plant Nicotiana benthamiana using Cas9 RNA-guided endonuclease. Nat. Biotechnol. 31, 691–693. doi: 10.1038/nbt.2655
Puchta, H., Dujon, B., and Hohn, B. (1996). Two different but related mechanisms are used in plants for the repair of genomic double-strand breaks by homologous recombination. Proc. Natl. Acad. Sci. U.S.A. 93, 5055–5060. doi: 10.1073/pnas.93.10.5055
Puchta, H., Swoboda, P., Gal, S., Blot, M., and Hohn, B. (1995). Somatic intrachromosomal homologous recombination events in populations of plant siblings. Plant Mol. Biol. 28, 281–292. doi: 10.1007/BF00020247
Ramsell, J. N. E., Boulton, M. I., Martin, D. P., Valkonen, J. P. T., and Kvarnheden, A. (2009). Studies on the host range of the barley strain of Wheat dwarf virus using an agroinfectious viral clone. Plant Pathol. 58, 1161–1169. doi: 10.1111/j.1365-3059.2009.02146.x
Salomon, S., and Puchta, H. (1998). Capture of genomic and T-DNA sequences during double-strand break repair in somatic plant cells. EMBO J. 17, 6086–6095. doi: 10.1093/emboj/17.20.6086
Schiml, S., Fauser, F., and Puchta, H. (2014). The CRISPR/Cas system can be used as nuclease for in planta gene targeting and as paired nickases for directed mutagenesis in Arabidopsis resulting in heritable progeny. Plant J. 80, 1139–1150. doi: 10.1111/tpj.12704
Schiml, S., Fauser, F., and Puchta, H. (2017). CRISPR/Cas-mediated in planta gene targeting. Methods Mol. Biol. 1610, 3–11. doi: 10.1007/978-1-4939-7003-2_1
Shan, Q., Wang, Y., Li, J., Zhang, Y., Chen, K., Liang, Z., et al. (2013). Targeted genome modification of crop plants using a CRISPR-Cas system. Nat. Biotechnol. 31, 686–688. doi: 10.1038/nbt.2650
Shukla, V. K., Doyon, Y., Miller, J. C., DeKelver, R. C., Moehle, E. A., Worden, S. E., et al. (2009). Precise genome modification in the crop species Zea mays using zinc-finger nucleases. Nature 459, 437–441. doi: 10.1038/nature07992
Sun, Y., Zhang, X., Wu, C., He, Y., Ma, Y., Hou, H., et al. (2016). Engineering herbicide-resistant rice plants through CRISPR/Cas9-mediated homologous recombination of acetolactate synthase. Mol. Plant 9, 628–631. doi: 10.1016/j.molp.2016.01.001
Svitashev, S., Schwartz, C., Lenderts, B., Young, J. K., and Cigan, A. M. (2016). Genome editing in maize directed by CRISPR-Cas9 ribonucleoprotein complexes. Nat. Commun. 7:13274. doi: 10.1038/ncomms13274
Svitashev, S., Young, J. K., Schwartz, C., Gao, H. R., Falco, S. C., and Cigan, A. M. (2015). Targeted mutagenesis, precise gene editing, and site-specific gene insertion in maize using Cas9 and guide RNA. Plant Physiol. 169, 931–945. doi: 10.1104/pp.15.00793
Terada, R., Urawa, H., Inagaki, Y., Tsugane, K., and Iida, S. (2002). Efficient gene targeting by homologous recombination in rice. Nat. Biotechnol. 20, 1030–1034. doi: 10.1038/nbt737
Thomas, W. T. B., Bull, H., Booth, A., Hamilton, R., Forster, B. P., and Franckowiak, J. D. (2019). A practical guide to barley crossing. Methods Mol. Biol. 1900, 21–36. doi: 10.1007/978-1-4939-8944-7_3
Townsend, J. A., Wright, D. A., Winfrey, R. J., Fu, F., Maeder, M. L., Joung, J. K., et al. (2009). High-frequency modification of plant genes using engineered zinc-finger nucleases. Nature 459, 442–445. doi: 10.1038/nature07845
Tzfira, T., Frankman, L. R., Vaidya, M., and Citovsky, V. (2003). Site-specific integration of Agrobacterium tumefaciens T-DNA via double-stranded intermediates. Plant Physiol. 133, 1011–1023. doi: 10.1104/pp.103.032128
Vu, G. T. H., Cao, H. X., Watanabe, K., Hensel, G., Blattner, F. R., Kumlehn, J., et al. (2014). Repair of site-specific DNA double-strand breaks in barley occurs via diverse pathways primarily involving the sister chromatid. Plant Cell 26, 2156–2167. doi: 10.1105/tpc.114.126607
Vu, T. V., Sivankalyani, V., Kim, E.-J., Doan, D. T. H., Tran, M. T., Kim, J., et al. (2020). Highly efficient homology-directed repair using CRISPR/Cpf1-geminiviral replicon in tomato. Plant Biotechnol. J. 18, 2133–2143. doi: 10.1111/pbi.13373
Wang, M. G., Lu, Y. M., Botella, J. R., Mao, Y. F., Hua, K., and Zhu, J. K. (2017). Gene targeting by homology-directed repair in rice using a geminivirus-based CRISPR/Cas9 system. Mol. Plant 10, 1007–1010. doi: 10.1016/j.molp.2017.03.002
Wang, S., He, J., Cui, Z., and Li, S. (2007). Self-formed adaptor PCR: a simple and efficient method for chromosome walking. Appl. Environ. Microbiol. 73, 5048–5051. doi: 10.1128/AEM.02973-06
Watanabe, K., Breier, U., Hensel, G., Kumlehn, J., Schubert, I., and Reiss, B. (2016). Stable gene replacement in barley by targeted double-strand break induction. J. Exp. Bot. 67, 1433–1445. doi: 10.1093/jxb/erv537
Weng, H., Pan, A. H., Yang, L. T., Zhang, C. M., Liu, Z. L., and Zhang, D. B. (2004). Estimating number of transgene copies in transgenic rapeseed by real-time PCR assay with HMG I/Y as an endogenous reference gene. Plant Mol. Biol. Rep. 22, 289–300. doi: 10.1007/BF02773139
Wolter, F., Klemm, J., and Puchta, H. (2018). Efficient in planta gene targeting in Arabidopsis using egg cell-specific expression of the Cas9 nuclease of Staphylococcus aureus. Plant J. 94, 735–746. doi: 10.1111/tpj.13893
Wolter, F., and Puchta, H. (2019). In planta gene targeting can be enhanced by the use of CRISPR/Cas12a. Plant J. 100, 1083–1094. doi: 10.1111/tpj.14488
Xie, K., and Yang, Y. (2013). RNA-guided genome editing in plants using a CRISPRCas system. Mol. Plant 6, 1975–1983. doi: 10.1093/mp/sst119
Zhang, Y., Zhang, F., Li, X., Baller, J. A., Qi, Y., Starker, C. G., et al. (2013). Transcription activator-like effector nucleases enable efficient plant genome engineering. Plant Physiol. 161, 20–27. doi: 10.1104/pp.112.205179
Keywords: wheat dwarf virus, homology-dependent recombination, knock-in, precise insertion, repair template
Citation: Lawrenson T, Hinchliffe A, Clarke M, Morgan Y and Harwood W (2021) In-planta Gene Targeting in Barley Using Cas9 With and Without Geminiviral Replicons. Front. Genome Ed. 3:663380. doi: 10.3389/fgeed.2021.663380
Received: 02 February 2021; Accepted: 29 April 2021;
Published: 15 June 2021.
Edited by:
Yiping Qi, University of Maryland, College Park, United StatesReviewed by:
Goetz Hensel, Heinrich Heine University Düsseldorf, GermanyFiliz Gürel, University of Maryland, College Park, United States
Copyright © 2021 Lawrenson, Hinchliffe, Clarke, Morgan and Harwood. This is an open-access article distributed under the terms of the Creative Commons Attribution License (CC BY). The use, distribution or reproduction in other forums is permitted, provided the original author(s) and the copyright owner(s) are credited and that the original publication in this journal is cited, in accordance with accepted academic practice. No use, distribution or reproduction is permitted which does not comply with these terms.
*Correspondence: Wendy Harwood, wendy.harwood@jic.ac.uk