Comparing Acoustic Tag Attachments Designed for Mobile Tracking of Hatchling Sea Turtles
- 1Chesapeake Biological Laboratory, University of Maryland Center for Environmental Science, Solomons, MD, United States
- 2The Leatherback Trust, Monterey, CA, United States
- 3The Lost Years – Pelagic Life History Fund, The Ocean Foundation, Washington, DC, United States
- 4Indiana University-Purdue University Fort Wayne, Fort Wayne, IN, United States
The poorly understood movements of sea turtles during the “lost years” of their early life history have been characterized as a “passive drifter” stage. Biologging technology allows us to study patterns of dispersal, but the small body size of young life stages requires particular consideration that such tagging does not significantly impede animal movements. We tested the effect of instrument attachment methods for mobile acoustic tracking of hatchling sea turtles, including a design that would be suitable for leatherback turtles (Dermochelys coriacea). We obtained 8-week-old hatchery-reared green sea turtles (Chelonia mydas) (n = 12 individuals) and examined the effect of attaching Vemco V5 acoustic tags. Each animal's swim speed, swimming depth, and stroke frequency were determined under three scenarios: control, direct Velcro® attachment to the carapace, and harness attachment, to determine if there was a significant difference amongst treatments. Turtle swimming speed was significantly slower during the middle period of the trial for the harness attachment compared with the control. No significant change in swim speed was observed when the tag was attached directly with Velcro®, and no significant change in dive depth was observed for either treatment compared to the control. Stroke frequency was significantly greater compared to the control at the end of the trial for the Velcro® attachment only, although there was no corresponding increase in swimming speed. This information can be used to design effective approaches for actively tracking free-ranging hatchling sea turtles to understand dispersal and survival of these vulnerable marine species.
Introduction
Effective conservation efforts for marine species are hindered by a lack of knowledge regarding movements and habitat utilization (Bowen and Karl, 2007). Highly migratory marine species, such as sea turtles, pose additional complexities for management as they traverse large distances and cross international boundaries throughout their life history (Hays and Scott, 2013). Young life stages, notoriously difficult to track, can even undergo these long migrations (Bolten, 2003; Hazen et al., 2012; Shillinger G. et al., 2012). The “lost years” of sea turtles are an enigmatic period of unknown distribution and developmental habitat after hatchlings leave natal beaches. This oceanic period of sea turtle life history has been increasingly studied in recent years as sea turtle conservation efforts expand beyond terrestrial zones. These investigations of at-sea movements during early life stages have been made possible by advances in biologging technology (e.g., Mansfield et al., 2014; Scott et al., 2014; Thums et al., 2016). Threatened and endangered species with unknown life history patterns, including highly vulnerable sea turtles (Lascelles et al., 2014), have been a research focus to advance conservation and management strategies (Hamann et al., 2010).
Information about sea turtle dispersal and behaviour during the “lost years” has been gained through modelling approaches (Hays et al., 2010; Shillinger G. L. et al., 2012; Putman et al., 2013; Casale and Mariani, 2014), telemetry (Nagelkerken et al., 2003; Witherington et al., 2012; Mansfield et al., 2014; Scott et al., 2014), and other emerging technologies, such as stable isotopes (Bowen and Karl, 2007; Reich et al., 2007; Snover et al., 2010; López-Castro et al., 2014). Due to a lack of information on active dispersal capacity, modelling efforts rely heavily on classifying young turtles as “passive drifters,” with little influence on their environment (Hays et al., 2010; Gaspar et al., 2012; Shillinger G. L. et al., 2012; Putman and Mansfield, 2015). Biophysical models can be strengthened and verified by incorporating behavioural data, such as swim speed and orientation (Putman et al., 2012, 2013; Kobayashi et al., 2014; Briscoe et al., 2016), as both swim behaviour and ocean currents control young sea turtles' directionality and influence dispersal outcomes (Gaspar et al., 2012; Putman and Mansfield, 2015; Briscoe et al., 2016). Behavioural data can be collected by deploying instruments to track turtle movements (Putman et al., 2012; Thums et al., 2013; Mansfield et al., 2014; Scott et al., 2014).
Reduction or elimination of tag effects when examining sea turtle early life stages is of high importance in order to maximize field data integrity and minimize negative impacts on tagged individuals (Jones et al., 2013). Acoustic tags are lighter and smaller than satellite tags, but appropriate methods of attaching these tags to turtle hatchlings are still under development and lacking for many species (Hazen et al., 2012; Shillinger G. et al., 2012). Small turtles experience a higher drag ratio compared to larger, more frequently-tracked adult turtles, resulting in higher transport costs of attachments. Impacts on turtle movements and behaviour are presumed to be negligible when below the colloquial 3% tag-to-body-weight threshold. Hatchling sea turtles tagged with miniature acoustic tags generally meet this requirement (Thums et al., 2013, 2016; Scott et al., 2014), but the influence of tag attachments on animal behaviour should still be carefully considered prior to field studies on threatened and endangered species (Vandenabeele et al., 2012). An examination of movement metrics, such as speed and diving depth, should be undertaken prior to deploying transmitters on wild turtles to ensure that the tracking process is unlikely to decrease fitness or survival, whilst providing biologically representative information (Casper, 2009; Mansfield et al., 2012; Jones et al., 2013). A direct attachment method on the plastron has been developed for flatback (Natator depressus), green (Chelonia mydas), and loggerhead (Caretta caretta) hatchlings (Thums et al., 2013, 2016; Scott et al., 2014). However, we sought a design that would be suitable for leatherback turtles (Dermochelys coriacea), which have a unique oily skin, and that would detach easily during recovery to ensure the tags were guaranteed to be recovered and removed from a critically endangered sea turtle population in field studies. The direct attachment method would also make it difficult to maintain visual contact with the small, dark bodies of hatchling turtles during mobile active tracking in the open ocean. The existing direct plastron attachment method utilized in other studies (Thums et al., 2013, 2016; Scott et al., 2014) was therefore not suitable and an alternative attachment design was required.
The objectives of this study were to (1) develop a protocol for attaching miniature acoustic transmitters to hatchling sea turtles suitable for mobile tracking of hatchling leatherbacks in the open ocean and (2) quantify the effects of tag and attachment materials on young sea turtle swim speed and dive behaviour. Analysis of the stroke frequency further provided insight into swimming performance. We tested the assumption that attaching Vemco V5 acoustic tags would not affect post-hatchling turtle swim speed, dive depth, and stroke frequency. We sought an attachment design to allow for multiple means of observation to increase the likelihood of maintaining contact in field studies using mobile tracking and detach easily during recovery from these at-risk species. To evaluate potential effects, this study was undertaken with hatchery-reared post-hatchling stage green sea turtles in Grand Cayman serving as a conservative proxy for leatherbacks. Due to a low drag coefficient, combined with their frontal area and carapace length, young green sea turtles may encounter greater drag costs than other sea turtle species (Jones et al., 2013). Therefore, this study will provide a reasonable conservative baseline understanding of potential effects of tag attachment methods for mobile tracking across sea turtle species.
Materials and Methods
Tag Attachment Protocol
We conducted experiments with twelve 8-week-old hatchery-reared green sea turtles (Chelonia mydas) at the Cayman Turtle Farm on Grand Cayman in December 2014. The sample size (n = 12) reflects the number of post-hatchlings available from the hatchery at the time of the study. The turtles remained out of public view prior to experiments. The mean weight of the turtles was 59.9 g (range = 38.3–74.3 g), and the mean straight carapace length notch-to-tip was 73.5 mm (range = 64.0–78.5 mm). All weights were recorded in-air.
We tested two alternative methods for attaching Vemco V5-180 kHz acoustic transmitters (0.65 g) (Vemco Ltd, Halifax, Canada) to hatchling turtles. For both turtle attachment methods, tags were affixed to a tether in a similar arrangement to Gearheart et al. (2011). Attachments had braided monofilament line (1.75 m) suspending two painted floats (4.4 cm by 1.9 cm) behind the turtles (Figure 1). The monofilament line was doubled onto itself to mimic the weight of an anticipated longer fieldwork attachment due to restrictions encountered by the size of the tank. The acoustic tag was suspended 0.25 m from the second float, and the combined weight of the line, floats, and tag was 7.5 g. There were two attachment mechanisms tested in this experiment. The line-float-transmitter assembly was affixed to the turtles' carapace by one of two methods. For the Velcro® treatment, a 1 cm2 Velcro® square (1.71 g) was directly bonded to the carapace with several drops of Vetbond™ (Jones et al., 2000; Salmon et al., 2004; Thums et al., 2013; Scott et al., 2014) and linked to a sister piece of Velcro® on the line-float-transmitter assembly. Initial testing of Vetbond™ used for the Velcro® treatment was conducted with naturally deceased hatchlings to ensure the bonding agent would dissolve and separate from hatchlings. The Velcro® attachment could be removed easily with a slight pull within a few days, suggesting the attachment material would be shed easily under natural conditions. For the harness treatment, the line-float-transmitter assembly was linked to a harness (0.47 g) made from 3M™ Coban™, a self-sticking latex/spandex/polyethylene compound. The harness attachment consisted of the same braided monofilament line and float setup, slipped over the head, and wrapped around the widest part of the turtle (Figure 2). Trials with the Velcro® treatment, harness treatment, and a control treatment with no attachment were conducted with each turtle in a randomized fashion.
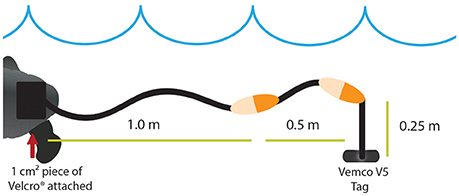
Figure 1. Acoustic transmitter Velcro® attachment method modified from Gearheart et al. (2011). Symbols are courtesy of the Integration and Application Network, University of Maryland Center for Environmental Science (ian.umces.edu/symbols/).
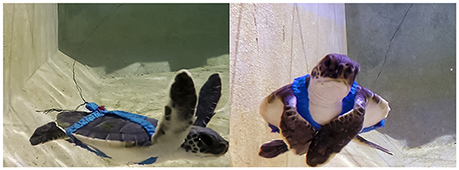
Figure 2. Images of the harness design and application on Chelonia mydas hatchlings. Footage from an underwater GoPro camera.
We conducted trials to monitor for behavioural responses of turtles to each treatment in a 12.25 m2 hexagonal tank filled with seawater to 0.6 m depth with a flow rate of 60 litres per minute (Figure 3). A 25 cm by 25 cm grid was placed over the tank to track distance travelled by each turtle for speed calculations. Vertical distance was labelled by a pole with centimetre intervals in the middle of the tank. Every turtle was observed individually for 25 min under each of three treatment scenarios: control, Velcro®, and harness. Turtles were randomly selected for each treatment and given a minimum period of 2 days between treatments over the 2 week study period. Movements were recorded using two GoPro HERO 4 cameras (GoPro, Inc., San Mateo, CA), one placed underwater near a corner of the tank and one hoisted 5.1 m centred overhead.
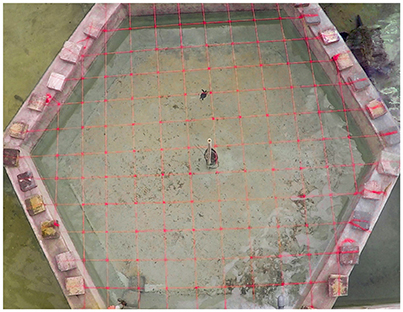
Figure 3. Scale of the tank experiment at the Cayman Turtle Farm. The GoPro camera hoisted 5.1 m above the hexagonal tank filmed each turtle for 25 min per treatment. Each square is 25 cm by 25 cm to serve as a distance reference.
Our aim was to choose the least intrusive methods of attachment to address potential concerns for animal welfare. There were no evident injuries from the Velcro® or harness attachments. Permission for all procedures was obtained prior to the experiment from the University of Maryland Center for Environmental Science's Institutional Animal Care and Use Committee (Research Protocol No. S-CBL-14-14). The research was conducted under approval of scientific study from the Cayman Islands' Department of Environment.
Horizontal Movement Analysis
Video was compiled with Adobe Creative Premiere Pro CC (Adobe Systems, Inc., San Jose, CA), and turtle movements were analysed using the Tracker Video Analysis and Modeling Tool program, an Open Source Physics Java framework (Brown, 2014). Horizontal swim speed was calculated within the program as a function of movement in the x- and y-directions. Speed was estimated every second and averaged at 10 s intervals for each 25 min trial. This 10 s interval provided a fine-scale measure of the variability in speed without oversampling. Time was then split into 5 min blocks, producing five time periods over each 25 min trial to allow us to investigate changes in the response across a time scale more appropriate to field conditions. Analyses were run in the R statistical software environment (R Core Team, 2016).
A within-subjects repeated measures ANOVA with a block on each post-hatchling was conducted to test differences in speed using the R package “nlme” (Pinheiro et al., 2016). The response variable of speed was square-root transformed based on results of a Box-Cox transformation to meet model assumptions (package “MASS”) (Venables and Ripley, 2002). Variation in turtles' speed was investigated using explanatory categorical factors of treatment (control, harness, and Velcro®), time period (5 min blocks), and the interaction of these variables. The best error structure fit with restricted maximum likelihood was a lag 1 autoregressive structure combined with a nested random effects structure of random intercepts among treatments for individual turtles. The autoregressive process of order 1 error structure suggests there is a dependency in the errors between the current value and the previous value, adjusting for correlations among repeated measures. The appropriate fixed effects structure was determined to be the interaction of treatment with time using maximum likelihood. The final model was refit using restricted maximum likelihood. The appropriate ANOVA model was chosen by the Akaike information criterion (AIC) at each step (e.g., “drop1” in R software). The Tukey's honest significant difference test from the package “multcomp” was used in post-hoc analysis (Hothorn et al., 2008).
Vertical Movement Analysis
To determine if diving behaviour was affected by transmitter attachments, an underwater camera captured each turtle's movement over time for each treatment. The camera was physically moved side to side as turtles moved throughout the tank to ensure all turtle movements were captured. A depth threshold of 15 cm was set to delineate time spent at the surface vs. time spent diving. For these trials, this resulted in the surface classified as the upper quarter of the water column where swimming was underneath the air-water interface in contrast to definitive diving behaviour. Diving behaviour was measured this way because a true dive depth could not be measured within the available tank. Only a field experiment with a depth recorder could provide this level of estimation without potential tank interference. This classification was a compromise to generically categorize whether having an attachment altered vertical movements through the water column. Water column depth was estimated every second, and these counts of being at the surface or below were compiled every 10 s. This provided a proportion interval similar to the horizontal analysis. Time spent below 15 cm vs. time spent at the surface could then be compared amongst treatments. In a similar manner to speed, data were separated into 5 min blocks across the 25 min recording time. A generalized linear mixed model with a binomial error distribution and logit link function was applied to the response variable of the proportion of time below 15 cm within each 10 s period (package “lme4”) (Bates et al., 2015). The categorical explanatory variables were treatment, time in the form of 5 min periods, and their interaction. Using AIC, the best random effects structure was initially found to be a random intercept varying among turtles and among treatments for each turtle (Zuur et al., 2009). To account for model overdispersion, an unstructured random effects term of record number was included in the model. The interaction of treatment and time was significant for the best fixed effects structure. Therefore, the final model was the interaction of treatment and time with 3 random effects: among turtles, among treatments for individuals, and an unstructured error. Model contrasts against the control treatment were completed for each time period to provide a post-hoc test for appropriate significance values across these levels and treatments of the linear model.
Stroke Frequency Analysis
The stroke frequency of swimming animals is useful to understanding behavioural changes and swimming performance (e.g., Burgess et al., 2006; Booth, 2014; Sim et al., 2015). Swimming speed is not correlated with stroke frequency in green sea turtles (Booth, 2014), thus making it an independent measurement. We calculated each stroke as the combination of both a down-stroke and up-stroke (Davenport et al., 1984). Strokes were counted during 10 s intervals, and counts were divided by the time spent actively stroking to obtain stroke frequency (Hz) (Ischer et al., 2009). Time spent gliding, dog paddling (Salmon and Wyneken, 1987), resting, or outside the view of the camera was not included in the analysis. As in the previous analyses, time was divided into 5 min blocks, resulting in 5 blocks over the 25 min recording period.
A within-subjects repeated measures ANOVA was run in the same manner as that of speed. A Box Cox transformation indicated the response variable of stroke frequency required a log transformation. Explanatory variables of time (5 min blocks), treatment (control, harness, and Velcro®), mass (g), and the interaction of time and treatment were examined to determine changes in stroke frequency. The best covariance structure allowed for variance to change per treatment combination with the same nested random effects structure used in the prior ANOVA. The best model was chosen at each step through AIC and confirmed with both Bayesian Information Criterion and AICc, which corrects for small sample sizes. Post-hoc analysis was completed with Tukey's honest significant difference test.
Results
Horizontal Movement Analysis
There was a statistically significant interaction between treatment and time on turtle speed (Table 1; Figure 4A). Swim speed was not significantly different for the Velcro® treatment compared to the control for any time periods (Tukey's; α = 0.05). Swim speed was significantly reduced with the harness attachment compared to the control during the middle 5-20 min of the trial, time-steps 2–4 (Tukey's; p < 0.01; p = 0.026; p < 0.01, respectively).
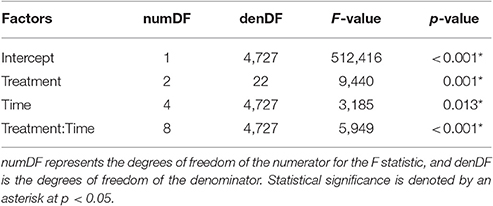
Table 1. Repeated measures ANOVA results examining square-root transformed speed (cm/s) as a function of the interaction of treatment and time as 5 min periods.
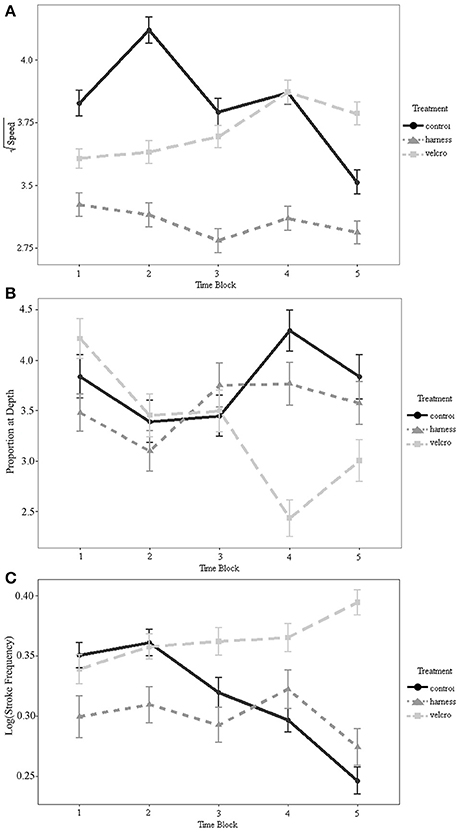
Figure 4. (A) Interaction plot of the square-root transformed speed (cm/s) as a function of time for each treatment. (B) Interaction plot of the proportion of time spent below 15 cm as a function of time for each treatment. (C) Interaction plot of the log transformed stroke frequency (Hz) as a function of time for each treatment. Minutes comprising the time periods are 1 = (0–5 min), 2 = (5–10 min), 3 = (10–15 min), 4 = (15–20 min), 5 = (20–25 min). Error bars represent standard errors.
Vertical Movement Analysis
Turtles spent 36% of trial time below the surface 15 cm of the tank (Figures 4B, 5). The generalized linear mixed model did not find significant differences in the proportion of time at the surface amongst treatments at each of the 5 min time periods (α = 0.05; Table 2). There is no evidence to suggest diving behaviour was different between treatments.
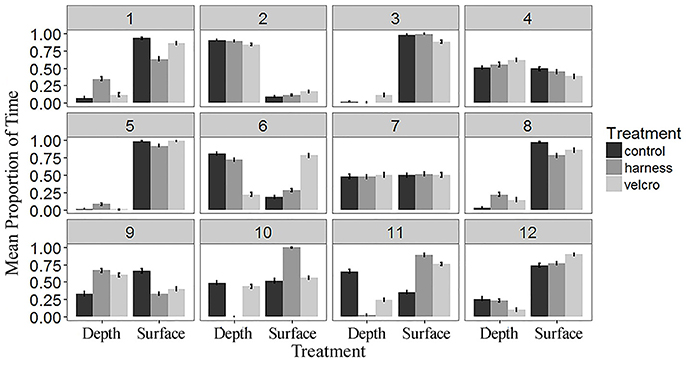
Figure 5. The mean proportion of time spent below 15 cm (“Depth”) and at the surface (“Surface”) by subject and treatment over the study period. Water column depth was measured every second over the 25 min study period. Each subject is indicated by the number in the grey box. Error bars represent standard errors.
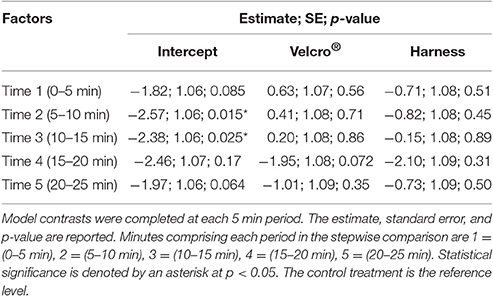
Table 2. The generalized linear mixed model with a binomial error and logit link function results for the proportion of time spent below 15 cm in relation to the interaction of treatment and time.
Stroke Frequency Analysis
Mass was not statistically significant in the repeated measures ANOVA (p = 0.941), but the interaction between treatment and time was significant (α = 0.05; Table 3; Figure 4C). Across all time periods, stroke frequency was not significantly different for the harness treatment compared to the control (Tukey's; α = 0.05), and stroke frequency was significantly greater for the Velcro® treatment at the final time-step (at 20–25 min during the trial) compared to the control (Tukey's; p = 0.024).
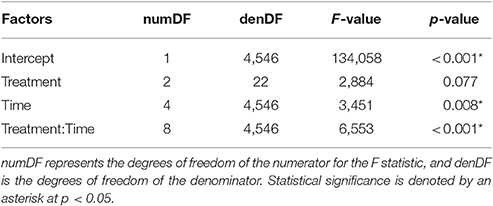
Table 3. Results of the repeated measures ANOVA examining stroke frequency (Hz) with the interaction of treatment and time (5 min blocks).
Discussion
We tested miniature acoustic transmitter attachment protocols for efficient tagging of leatherback turtles, using green turtles as a proxy, to minimize impediment of swimming and diving of small sea turtles, while still providing a means of visual contact with diving turtles. Our study suggests outfitting young sea turtles with Vemco V5 acoustic tags will not significantly alter their swim speed or dive behaviour with a Velcro® attachment configuration to the carapace, at least in controlled lab conditions. The Velcro® attachment approach did not result in a significant change in the swim speed or dive behaviour of the turtles at any point during the trials. However, this attachment did result in a faster stroke frequency during the last 5 min block of the trial. This suggests a possible change in the swimming performance or swimming effort at the end of this treatment, which may have resulted in an increase in energetic expenditure. However, the increase in stroke frequency did not correspond with an increase in swimming speed (Figures 4A,C). There may have been a compensatory reduction in front flipper amplitude during that time period (Davenport et al., 1984; Booth, 2014). A significant decrease in stroke rate that would be indicative of decreased swimming ability (Burgess et al., 2006) was not observed for either attachment method.
The Velcro® attachment was ultimately determined to be more suitable than the harness attachment, which significantly decreased swim speeds during the middle 15 min of the trial. Our visual observations suggest that the harness disrupted turtle behaviour compared to the control, possibly from constriction of the shoulder girdle, thus reducing swimming speed. We observed that turtles with harness attachments initially spent time at the surface attempting to remove the harness, then conducted a series of rapid dives, whereas the control treatments generally had smooth transitions between the surface and depth separations within the water column. Irritation caused by the harness attachments make this approach less desirable for field experiments and could increase the risk of predation at sea. It could also alter interpretations of past studies that utilized harness methodologies on young sea turtles over short time frames. Based on our results, an experiment utilizing harness attachments should allow for an acclimation period of at least 20 min, while the Velcro® attachment method does not require acclimation. This study can help inform tagging procedures for field studies examining movement of free-ranging hatchling sea turtles.
There are limitations to studies such as this because gaining access to endangered species is difficult. The inability to access at-risk sea turtles resulted in a low sample size. Mansfield et al. (2012) utilized an ANOVA framework with smaller sample sizes on sea turtles, and our sample size is within the generally accepted size for this statistical test. While a larger sample size could theoretically increase statistical robustness, this was not feasible given available turtles at the time, and data corrections were applied to meet all model assumptions. Speed is highly variable and individualistic, inconsequential of sample size. Therefore, the sample size may be low, but a larger sample size would not guarantee a more robust statistical test given the high variability inherent in the measured parameter.
Our approach of using a line-float-transmitter attachment was chosen over a direct tag attachment to the plastron at the cost of increased drag because it allows for visual tracking in the water during mobile tracking and should prevent signal dampening or distortion during future field experiments (Thums et al., 2013). This will also help field studies better interpret sources of signal loss at a given location, from events such as predation, tag malfunction, wave interference, or departure from the study site (Thums et al., 2013). Mobile acoustic tracking is very difficult when trying to obtain fine-scale movements through an area when the detection range may extend to 0.25 km. Visual contact with the tracked organism provides the means of fully tracking an organism during a given time period. Although the tag to body weight ratio increases with the Velcro® attachment, there were no significant differences from the control in the swimming speed and diving metrics we measured. Overall, both attachments allow for a safe, full removal from actively tracked turtles, reducing experimental exposure time for wild turtles. Any object placed on an organism adapted to live in its environment may affect its natural behaviours and increase its energetic costs. Consequently, an objective of this methodology was to provide appropriate consideration to the development of tag attachments for leatherback turtles that minimize these negative effects and extend beyond controlled tank environments (Mansfield et al., 2012; Jones et al., 2013). Given that the oily, rubbery skin of leatherbacks could reduce adherence, we wanted to test two attachment techniques in case there were conditions in the field that made a particular method less effective. A vertically attached tag to the plastron, as used by Thums et al. (2016), would not allow for maintained visual contact with deep-diving leatherback hatchlings, as well as provide a very small attachment site on oily skin that has the potential to react differently to Vetbond™. Therefore, methods that would allow for both visual and acoustic contact to be maintained were considered most effective for actively tracking critically endangered leatherback turtles.
Although there was no significant difference between the control and harness for the proportion of time spent below the surface, this may have resulted from individuals generally spending greater amounts of time at the surface during the control because behavioural reactions to the attachment generally occurred within the surface layer. Any tag attached to an organism should theoretically increase drag, and it is possible the turtles increased power output (e.g., swam harder) to overcome this additional drag, something a longer temporal study might determine (Jones et al., 2013). Stroke frequency was not significantly related to body mass within the limited range of sizes in our study, although such relationships have been found in adult seabirds, pinnipeds, and cetaceans (Sato et al., 2007). Cornick et al. (2006) found harnessed sea lions significantly increased stroke frequency and decreased swimming speed, resulting in overall lower swimming efficiency with reductions in dive durations and foraging times. Further, adult leatherbacks with satellite tags attached to their carapace had faster swimming speeds and longer dive times than harnessed adults, suggesting harnesses were more likely to alter swimming and diving abilities (Fossette et al., 2008). Constraints on the experiment prevented other fine-scale measurements of swimming performance, such as front flipper amplitude, front flipper angle, and proportion of time spent powerstroking, which can aid in understanding the drag costs incurred by individuals (e.g., Davenport et al., 1984; Booth, 2014).
Limitations in both vertical and horizontal movements may have resulted from the experimental tank design. However, visual observation indicated the turtles moved vertically throughout the tank in a similar manner across all treatments, which was supported by the results of the GLM. Edge effects of the tank could alter turtle behaviour through more frequent changes in direction or by seeking shelter, for example, and the depth of the tank may have changed diving patterns. Although the tank was shallower than the length of the attachment, time spent at depth was usually sustained swimming around the circumference of the tank. Therefore, it adequately provides information on whether the attachment changed their vertical movements. We did not provide direct estimates of swimming speed as we recognize that the tank will potentially limit the speed capacity of the turtles, and it would be an inappropriate comparison to other studies of this species. The repeated measures ANOVA appropriately examined changes in speed within individuals, which was the goal of the analysis. Given these turtles generally swam in continuous circles during the study period, we believe any changes in drag which turtles experienced as they moved throughout the tank (e.g., if the line went slack upon changing course) was properly accounted for in our models. A few turtles became entangled in the gear, and untangled themselves. This was an artefact of multiple factors: the size and shape of the tank, as well as the age and behaviour of the turtles. In the open ocean, for which this method was developed, this is not an anticipated concern if turtles are in a frenzied state where swimming will be directed and continuous (Wyneken and Salmon, 1992). Further, the short duration and controlled design did not consider wind drift effects, which have the potential to impact movement during longer studies utilizing these methods (Jones et al., 2013).
Sea turtle early life histories are poorly understood, and lack of knowledge regarding movements and developmental habitat may impede conservation efforts. Advancement of appropriate management strategies requires an understanding of movement and dispersal beyond the adult stage. The “lost years” paradigm begins upon denatant dispersal of hatchlings in a neritic-to-oceanic migration to unknown or unclearly defined nursery habitats. Combining miniaturized tag technology and physical modelling efforts enables much-needed characterization of movement, habitat utilization, behaviour, and life strategies of young sea turtles throughout these cryptic years (Briscoe et al., 2016). As habitats are drastically changed by anthropogenic forces, migrations of many species may shrink or shift (Brower and Malcolm, 1991; Wilcove and Wikelski, 2008). Understanding the mechanisms underlying these movements will improve our ability to describe sea turtle environmental utilization, predict population dynamics, and manage species internationally under changing conditions (Nathan et al., 2008; Bauer et al., 2009). The challenge thus remains to decipher movements among ontogenetic habitats within and across species and understand how to manage these highly migratory species throughout multiple life stages.
Author Contributions
GS and HB provided support and assistance with experimental design, data analysis, permits, and manuscript revisions. JS contributed to data collection and experimental design. AH designed, ran, and analysed the experiment, followed by manuscript composition.
Conflict of Interest Statement
The authors declare that the research was conducted in the absence of any commercial or financial relationships that could be construed as a potential conflict of interest.
Acknowledgments
Financial support and field assistance were provided by The Leatherback Trust, the Chesapeake Biological Laboratory's Graduate Education Committee Fellowship, and Indiana University-Purdue University Fort Wayne. Funding was also provided by the Cinco Hermanos Fund, The Resource Legacy Group, and The Lost Years - Pelagic Life History Fund of The Ocean Foundation. The authors are grateful to Dr. Walter Mustin of the Cayman Turtle Farm for providing access to the turtles, equipment, and many hours of invaluable help, insight, and support of the experiment. We thank Dr. Martha Keller for advice with egg-rearing and hatchling development, and all of the Cayman Turtle Farm and its employees for their assistance with the research. The Leatherback Trust provided equipment, including the GoPro cameras, Vemco tags, and attachment supplies. The authors thank Lauren Cruz for much-needed assistance in data recording and public outreach, Amber Fandel for helping with the video analysis, and Dr. Frank Paladino for project support.
References
Bates, D., Maechler, M., Bolker, B., and Walker, S. (2015). Fitting linear mixed-effects models using lme4. J. Stat. Softw. 67, 1–48. doi: 10.18637/jss.v067.i01
Bauer, S., Barta, Z., Ens, B. J., Hays, G. C., McNamara, J. M., and Klaassen, M. (2009). Animal migration: linking models and data beyond taxonomic limits. Biol. Lett. 5, 433–435. doi: 10.1098/rsbl.2009.0324
Bolten, A. B. (2003). “Active swimmers – passive drifters: the oceanic juvenile stage of loggerheads in the Atlantic system,” in Loggerhead Sea Turtles, eds A. B. Bolten and B. E. Witherington (Washington, DC: Smithsonian Institution Press), 63–78.
Booth, D. T. (2014). Kinematics of swimming and thrust production during powerstroking bouts of the swim frenzy in green turtle hatchlings. Biol. Open 3, 887–894. doi: 10.1242/bio.20149480
Bowen, B. W., and Karl, S. A. (2007). Population genetics and phylogeography of sea turtles. Mol. Ecol. 16, 4886–4907. doi: 10.1111/j.1365-294X.2007.03542.x
Briscoe, D. K., Parker, D. M., Balazs, G. H., Kurita, M., Saito, T., Okamoto, H., et al. (2016). Active dispersal in loggerhead sea turtles (Caretta caretta) during the “lost years.” Proc. R. Soc. B Biol. Sci. 283:20160690. doi: 10.1098/rspb.2016.0690
Brower, L. P., and Malcolm, S. B. (1991). Animal migrations: endangered phenomena. Am. Zool. 31, 265–276.
Brown, D. (2014). Tracker Video Analysis and Modeling Tool. Available online at: http://physlets.org/tracker/
Burgess, E. A., Booth, D. T., and Lanyon, J. M. (2006). Swimming performance of hatchling green turtles is affected by incubation temperature. Coral Reefs 25, 341–349. doi: 10.1007/s00338-006-0116-7
Casale, P., and Mariani, P. (2014). The first “lost year” of Mediterranean sea turtles: dispersal patterns indicate subregional management units for conservation. Mar. Ecol. Prog. Ser. 498, 263–274. doi: 10.3354/meps10640
Casper, R. M. (2009). Guidelines for the instrumentation of wild birds and mammals. Anim. Behav. 78, 1477–1483. doi: 10.1016/j.anbehav.2009.09.023
Cornick, L. A., Inglis, S. D., Willis, K., and Horning, M. (2006). Effects of increased swimming costs on foraging behavior and efficiency of captive Steller sea lions: evidence for behavioral plasticity in the recovery phase of dives. J. Exp. Mar. Biol. Ecol. 333, 306–314. doi: 10.1016/j.jembe.2006.01.010
Davenport, J., Munks, S. A., and Oxford, P. J. (1984). A comparison of the swimming of marine and freshwater turtles. Proc. R. Soc. B Biol. Sci. 220, 447–475. doi: 10.1098/rspb.1984.0013
Fossette, S., Corbel, H., Gaspar, P., Le Maho, Y., and Georges, J. (2008). An alternative technique for the long-term satellite tracking of leatherback turtles. Endanger Species Res. 4, 33–41. doi: 10.3354/esr00039
Gaspar, P., Benson, S., Dutton, P., Réveillère, A., Jacob, G., Meetoo, C., et al. (2012). Oceanic dispersal of juvenile leatherback turtles: going beyond passive drift modeling. Mar. Ecol. Prog. Ser. 457, 265–284. doi: 10.3354/meps09689
Gearheart, G., Maturbongs, A., Dutton, P. H., Sprintall, J., Kooyman, G. L., Tapilatu, R. F., et al. (2011). Tracking leatherback (Dermochelys coriacea) hatchlings at sea using radio and acoustic tags. Mar. Turt. Newsl. 130, 2–6.
Hamann, M., Godfrey, M. H., Seminoff, J. A., Arthur, K., Barata, P. C. R., Bjorndal, K. A., et al. (2010). Global research priorities for sea turtles: informing management and conservation in the 21st century. Endanger Species Res. 11, 245–269. doi: 10.3354/esr00279
Hays, G. C., Fossette, S., Katselidis, K. A., Mariani, P., and Schofield, G. (2010). Ontogenetic development of migration: lagrangian drift trajectories suggest a new paradigm for sea turtles. J. R. Soc. Interface 7, 1319–1327. doi: 10.1098/rsif.2010.0009
Hays, G. C., and Scott, R. (2013). Global patterns for upper ceilings on migration distance in sea turtles and comparisons with fish, birds and mammals. Funct. Ecol. 27, 748–756. doi: 10.1111/1365-2435.12073
Hazen, E., Maxwell, S., Bailey, H., Bograd, S., Hamann, M., Gaspar, P., et al. (2012). Ontogeny in marine tagging and tracking science: technologies and data gaps. Mar. Ecol. Prog. Ser. 457, 221–240. doi: 10.3354/meps09857
Hothorn, T., Bretz, F., and Westfall, P. (2008). Simultaneous inference in general parametric models. Biometrical 50, 346–363. doi: 10.1002/bimj.200810425
Ischer, T., Ireland, K., and Booth, D. T. (2009). Locomotion performance of green turtle hatchlings from the Heron Island rookery, great barrier reef. Mar. Biol. 156, 1399–1409. doi: 10.1007/s00227-009-1180-7
Jones, T. T., Salmon, M., Wyneken, J., and Johnson, C. (2000). Rearing leatherback hatchlings: protocols, growth and survival. Mar. Turt. Newsl. 90, 3–6.
Jones, T. T., Van Houtan, K. S., Bostrom, B. L., Ostafichuk, P., Mikkelsen, J., Tezcan, E., et al. (2013). Calculating the ecological impacts of animal-borne instruments on aquatic organisms. Methods Ecol. Evol. 4, 1178–1186. doi: 10.1111/2041-210X.12109
Kobayashi, D. R., Farman, R., Polovina, J. J., Parker, D. M., Rice, M., and Balazs, G. H. (2014). “Going with the flow” or not: evidence of positive rheotaxis in oceanic juvenile loggerhead turtles (Caretta caretta) in the South pacific ocean using satellite tags and ocean circulation data. PLoS ONE 9:e103701. doi: 10.1371/journal.pone.0103701
Lascelles, B., Di Sciara, G. N., Agardy, T., Cuttelod, A., Eckert, S., Glowka, L., et al. (2014). Migratory marine species: their status, threats and conservation management needs. Aquat. Conserv. Mar. Freshw. Ecosyst. 24, 111–127. doi: 10.1002/aqc.2512
López-Castro, M. C., Bjorndal, K. A., Kamenov, G. D., and Bolten, A. B. (2014). Identifying oceanic foraging grounds of sea turtles in the Atlantic using lead isotopes. Mar. Biol. 161, 2269–2278. doi: 10.1007/s00227-014-2504-9
Mansfield, K. L., Wyneken, J., Porter, W. P., and Luo, J. (2014). First satellite tracks of neonate sea turtles redefine the “lost years” oceanic niche. Proc. R. Soc. B Biol. Sci. 281:20133039. doi: 10.1098/rspb.2013.3039
Mansfield, K. L., Wyneken, J., Rittschof, D., Walsh, M., Lim, C. W., and Richards, P. M. (2012). Satellite tag attachment methods for tracking neonate sea turtles. Mar. Ecol. Prog. Ser. 457, 181–192. doi: 10.3354/meps09485
Nagelkerken, I., Pors, L. P. J. J., and Hoetjes, P. (2003). Swimming behaviour and dispersal patterns of headstarted loggerhead turtles Caretta caretta. Aquat. Ecol. 37, 183–190. doi: 10.1023/A:1023924631480
Nathan, R., Getz, W. M., Revilla, E., Holyoak, M., Kadmon, R., Saltz, D., et al. (2008). A movement ecology paradigm for unifying organismal movement research. Proc. Natl. Acad. Sci. U.S.A. 105, 19052–19059. doi: 10.1073/pnas.0800375105
Pinheiro, J., Bates, D., DebRoy, S., Sarkar, D., and Team, R. C. (2016). Nlme: Linear and Nonlinear Mixed Effects Models. Available online at: http://cran.r-project.org/package=nlme
Putman, N. F., and Mansfield, K. L. (2015). Direct evidence of swimming demonstrates active dispersal in the sea turtle “lost years.” Curr. Biol. 25, 1221–1227. doi: 10.1016/j.cub.2015.03.014
Putman, N. F., Mansfield, K. L., He, R., Shaver, D. J., and Verley, P. (2013). Predicting the distribution of oceanic-stage Kemp's ridley sea turtles. Biol. Lett. 9:20130345. doi: 10.1098/rsbl.2013.0345
Putman, N. F., Scott, R., Verley, P., Marsh, R., and Hays, G. C. (2012). Natal site and offshore swimming influence fitness and long-distance ocean transport in young sea turtles. Mar. Biol. 159, 2117–2126. doi: 10.1007/s00227-012-1995-5
R Core Team (2016). R: A Language and Environment for Statistical Computing. R Foundation for Statistical Computing. Available online at: http://www.r-project.org/
Reich, K. J., Bjorndal, K. A., and Bolten, A. B. (2007). The “lost years” of green turtles: using stable isotopes to study cryptic lifestages. Biol. Lett. 3, 712–714. doi: 10.1098/rsbl.2007.0394
Salmon, M., Jones, T. T., and Horch, K. W. (2004). Ontogeny of diving and feeding behavior in juvenile seaturtles : leatherback seaturtles (Dermochelys coriacea) and green seaturtles (Chelonia mydas) in the Florida Current. J. Herpetol. 38, 36–43. doi: 10.1670/228-01A
Salmon, M., and Wyneken, J. (1987). Orientation and swimming behavior of hatchling loggerhead turtles Caretta caretta during their offshore migration. J. Exp. Mar. Biol. Ecol. 109, 137–153. doi: 10.1016/0022-0981(87)90012-8
Sato, K., Watanuki, Y., Takahashi, A., Miller, P. J., Tanaka, H., Kawabe, R., et al. (2007). Stroke frequency, but not swimming speed, is related to body size in free-ranging seabirds, pinnipeds and cetaceans. Proc. R. Soc. B Biol. Sci. 274, 471–477. doi: 10.1098/rspb.2006.0005
Scott, R., Biastoch, A., Roder, C., Stiebens, V. A., and Eizaguirre, C. (2014). Nano-tags for neonates and ocean-mediated swimming behaviours linked to rapid dispersal of hatchling sea turtles. Proc. R. Soc. B Biol. Sci. 281:20141209. doi: 10.1098/rspb.2014.1209
Shillinger, G., Bailey, H., Bograd, S., Hazen, E., Hamann, M., Gaspar, P., et al. (2012). Tagging through the stages: technical and ecological challenges in observing life histories through biologging. Mar. Ecol. Prog. Ser. 457, 165–170. doi: 10.3354/meps09816
Shillinger, G. L., Di Lorenzo, E., Luo, H., Bograd, S. J., Hazen, E. L., Bailey, H., et al. (2012). On the dispersal of leatherback turtle hatchlings from Mesoamerican nesting beaches. Proc. R Soc. B Biol. Sci. 279, 2391–2395. doi: 10.1098/rspb.2011.2348
Sim, E. L., Booth, D. T., and Limpus, C. J. (2015). Incubation temperature, morphology and performance in loggerhead (Caretta caretta) turtle hatchlings from Mon Repos, Queensland, Australia. Biol. Open 4, 685–692. doi: 10.1242/bio.20148995
Snover, M. L., Hohn, A. A., Crowder, L. B., and Macko, S. A. (2010). Combining stable isotopes and skeletal growth marks to detect habitat shifts in juvenile loggerhead sea turtles Caretta caretta. Endanger Species Res. 13, 25–31. doi: 10.3354/esr00311
Thums, M., Whiting, S. D., Reisser, J., Pendoley, K. L., Pattiaratchi, C. B., Hetzel, Y., et al. (2016). Artificial light on water attracts turtle hatchlings during their near shore transit. R. Soc. Open Sci. 3:160142. doi: 10.1098/rsos.160142
Thums, M., Whiting, S. D., Reisser, J. W., Pendoley, K. L., Pattiaratchi, C. B., Harcourt, R. G., et al. (2013). Tracking sea turtle hatchlings - a pilot study using acoustic telemetry. J. Exp. Mar. Bio. Ecol. 440, 156–163. doi: 10.1016/j.jembe.2012.12.006
Vandenabeele, S. P., Shepard, E. L., Grogan, A., and Wilson, R. P. (2012). When three per cent may not be three per cent; device-equipped seabirds experience variable flight constraints. Mar. Biol. 159, 1–14. doi: 10.1007/s00227-011-1784-6
Venables, W. N., and Ripley, B. D. (2002). Modern Applied Statistics with S. New York, NY: Springer-Verlag. doi: 10.1007/978-0-387-21706-2
Wilcove, D. S., and Wikelski, M. (2008). Going, going, gone: is animal migration disappearing. PLoS Biol. 6:e188. doi: 10.1371/journal.pbio.0060188
Witherington, B., Hirama, S., and Hardy, R. (2012). Young sea turtles of the pelagic Sargassum-dominated drift community: habitat use, population density, and threats. Mar. Ecol. Prog. Ser. 463, 1–22. doi: 10.3354/meps09970
Keywords: telemetry, sea turtle, behaviour, movement, migration, tracking, dispersal, “lost years”
Citation: Hoover AL, Shillinger GL, Swiggs J and Bailey H (2017) Comparing Acoustic Tag Attachments Designed for Mobile Tracking of Hatchling Sea Turtles. Front. Mar. Sci. 4:225. doi: 10.3389/fmars.2017.00225
Received: 07 April 2017; Accepted: 06 July 2017;
Published: 20 July 2017.
Edited by:
Sara M. Maxwell, Old Dominion University, United StatesReviewed by:
Patricia Briones-Fourzan, National Autonomous University of Mexico, MexicoLeslie Cornick, Eastern Washington University, United States
Copyright © 2017 Hoover, Shillinger, Swiggs and Bailey. This is an open-access article distributed under the terms of the Creative Commons Attribution License (CC BY). The use, distribution or reproduction in other forums is permitted, provided the original author(s) or licensor are credited and that the original publication in this journal is cited, in accordance with accepted academic practice. No use, distribution or reproduction is permitted which does not comply with these terms.
*Correspondence: George L. Shillinger, george_shillinger@yahoo.com