Iron Availability Influences the Tolerance of Southern Ocean Phytoplankton to Warming and Elevated Irradiance
- 1Research School of Earth Sciences, Australian National University, Canberra, ACT, Australia
- 2Antarctic Climate and Ecosystems Cooperative Research Centre, University of Tasmania, Hobart, TAS, Australia
- 3Institute for Marine and Antarctic Studies, University of Tasmania, Hobart, TAS, Australia
The Southern Ocean is responsible for approximately 40% of oceanic carbon uptake through biological and physical processes. In the Southern Ocean, phytoplankton growth is limited by low iron (Fe) and light supply. Climate model projections for the Southern Ocean indicate that temperature, underwater irradiance and Fe supply are likely to change simultaneously in the future due to increasing anthropogenic carbon dioxide emissions. The individual effects of these environmental properties on phytoplankton physiology have been extensively researched, and culturing studies using Southern Ocean phytoplankton have shown that temperature and Fe will play a key role on setting growth under future conditions. To explore the potential responses of Southern Ocean phytoplankton to these environmental changes, we cultured the haptophyte Phaeocystis antarctica and the diatoms Chaetoceros flexuosus, Proboscia inermis, and Thalassiosira antarctica under two light and iron combinations and over a range of temperatures. Our study revealed that the thermal response curves of key Southern Ocean phytoplankton are diverse, with the highest growth rates measured at 5°C (the annual temperature range at the isolation sites is currently 1–4°C). Warming had species-specific effects on the photochemical efficiency of photosystem II (PSII; Fv/Fm), the functional absorption cross-section of PSII (σPSII), carbon:nitrogen ratio and cellular Chlorophyll a concentrations. Iron availability increased species’ ability to tolerate warmer conditions by increasing the upper limit for growth and subsequently increasing the thermal niche that each species inhabit.
Introduction
Phytoplankton productivity in the Southern Ocean plays an important role in the transfer of carbon from the atmosphere to the ocean’s interior, in a process called the biological carbon pump. The strength of the biological carbon pump is important in regulating global climate. Southern Ocean productivity, in turn, is regulated by the availability of iron (Fe), light, and temperature, which influence the efficiency of the carbon pump (Sunda and Huntsman, 1997; Boyd et al., 2010). The effect of temperature on model diatom species has been well documented (Sunda and Huntsman, 2011); however, the interaction between temperature, light, and Fe on phytoplankton growth rate has only recently been explored in Southern Ocean species (Zhu et al., 2017; Boyd, 2019). Studies of subantarctic phytoplankton have identified Fe and temperature as key controls on phytoplankton growth with light, macronutrients and CO2 playing a lesser role (Boyd et al., 2016). Future warming of the Southern Ocean is expected to shift thermal niches poleward resulting in an associated shift in the biogeographical range of species, as they accommodate environmental changes (Thomas et al., 2012). Limited information is available on the thermal tolerance of specialized Southern Ocean phytoplankton, especially when temperature varies concurrently with other environmental variables (Boyd et al., 2013; Coello-Camba and Agustí, 2017).
Phytoplankton generally have an optimum growth temperature above the average mid-summer water temperature in which they grow, thus protecting them against short-term temperature fluctuations (Thomas et al., 2012). The growth rate of phytoplankton generally increases with temperature, until an optimum temperature is reached (Eppley, 1972). Once this temperature optimum has been exceeded, growth rate decreases and eventual mortality occurs (Kudo et al., 2000; Boyd et al., 2013; Zhu et al., 2017). Unlike tropical phytoplankton, which are already at or near at their thermal capacity, cold-adapted phytoplankton display optimum growth temperatures higher than the temperature of their current environment (Thomas et al., 2012). Thus, Southern Ocean phytoplankton may have a thermal safety net that will allow them to withstand the expected global warming associated with increasing CO2 concentrations.
In addition to temperature, Fe supply plays a key role in controlling productivity in the Southern Ocean (Boyd and Law, 2001; Blain et al., 2007). The biochemical importance of Fe in photosynthesis has been demonstrated by laboratory experiments (Greene et al., 1991, 1992; Sunda and Huntsman, 1997; Strzepek et al., 2011) and extensively explored through theoretical calculations by Raven (1990). The greatest metabolic requirement for Fe in phytoplankton is photosynthetic electron transport (Strzepek and Harrison, 2004). Furthermore, Rose et al. (2009) showed that while warming increased phytoplankton productivity, there is also evidence for an increased Fe demand and an earlier onset of nutrient and Fe limitation when compared to a lower temperature control group.
Future changes in net productivity under climate change scenario RCP 8.5 were explored by Laufkötter et al. (2015), who found large discrepancies in primary productivity between simulations. Consequently, these large uncertainties suggest that a dedicated and sustained effort should be undertaken to provide greater certainty in models by providing quality datasets that underpin model development. Defining the thermal tolerances of marine biota from a range of latitudes is central to improved biogeochemical modeling as most species have important roles in the cycling of nutrients (nitrate, silicic acid, phosphate), trace element cycling (e.g., zinc, copper, and Fe), and CO2 sequestration and export to the oceans’ interior. Additionally, temperature-induced floristic changes will have wide-scale impacts on global food webs. For example, Southern Ocean diatoms support most krill-based food webs (Feng et al., 2010); thus a shift in the community composition away from diatoms could have negative consequences for higher trophic levels.
The goal of this work is to identify the thermal thresholds of phytoplankton isolated from the Southern Ocean and provide basic physiological data on how Southern Ocean phytoplankton may respond to predicted ocean warming. We report the physiological responses of three Southern Ocean diatoms (Chaetoceros flexuosus, Proboscia inermis, and Thalassiosira antarctica), as well as the Southern Ocean haptophyte Phaeocystis antarctica, grown in low and high Fe and light treatments, and over a range of temperatures (3–14°C). Because the diatoms used in this study were isolated from the same locale, we hypothesize that they will share similar responses to light, Fe and temperature due to the unique photosynthetic specializations needed to grow in this area (Strzepek et al., 2019).
Materials and Methods
Culturing Conditions and Temperature Manipulation
The haptophyte P. antarctica (Clone SX9) was isolated from water collected in the Australasian sector of the Southern Ocean (62°08.72′S and 174°08.94′E) in December 2004 (Strzepek et al., 2012). The Southern Ocean diatoms grown for this research were C. flexuosus, P. inermis, and T. antarctica; all of which were isolated from seawater collected in November 2001 from 57°51.10′S and 139°50.70′E at a temperature of 3°C (Figure 1; Strzepek et al., 2011).
The artificial seawater medium Aquil (Price et al., 1989) was used to culture Southern Ocean phytoplankton. The Aquil medium was microwave-sterilized and enriched with filter-sterilized (0.2 μm, Gelman Acrodisc PF) trace metals and vitamins, and chelexed macronutrients (nitrate = 300 μmol L–1, silicate = 100 μmol L–1, phosphate = 10 μmol L–1). Previously reported experiments were used to select high Fe (Fe-replete) versus low Fe (Fe-limiting) conditions (Strzepek et al., 2011) and are listed in Supplementary Table 1. For the high Fe treatments, the synthetic ligand Ethylenediaminetetraacetic acid (EDTA) was used to buffer Fe and the other trace metals (e.g., Zn, Cu, Ni, etc.) in solution. The final EDTA concentration within the media was 10 μmol L–1. Iron was added to the media in a 1:1 complex with EDTA to prevent Fe precipitation. The final Fe concentration of the high Fe treatments was either 58 nmol L–1 or 4.4 nmol L–1. Values for [Fe′] were calculated using Visual MINTEQ (version 3.0; default thermodynamic database) and the calculations of Sunda and Huntsman (2003) as described in Strzepek et al. (2012).
For the low Fe treatments, the siderophore desferrioxamine B mesylate (DFB) was added to culture media because of its ability to strongly complex to Fe. Low Fe media were prepared using a premixed solution containing 3.5 nmol L–1 FeCl3 complexed with 4, 40, or 400 nmol L–1 of the siderophore DFB and added to Aquil medium containing 10 μmol L–1 of EDTA to buffer the other trace metals as described in Strzepek et al. (2011). The low Fe media were designed to reduce phytoplankton growth rates by ∼50% at growth saturating irradiance; therefore, the Fe:DFB ratios (nmol L–1: nmol L–1) of the Aquil media differed between species: P. antarctica – Fe:DFB 3.5:400; C. flexuosus and P. inermis – Fe:DFB 3.5:40; and T. antarctica – Fe:DFB 3.5:4.
Growth Rate Measurements
Cultures were maintained in exponential growth with a 1:100 dilution by transfer into fresh media as required. Growth rate data were collected from cultures acclimated to experimental temperature, irradiance or Fe treatments for a minimum of 15 generations. Each transfer was grown for at least 10 generations. In vivo chlorophyll a fluorescence of acclimated cultures was measured using a Turner Designs model 10-AU (Brand et al., 1981) and specific growth rates (μ) were determined from least-squares regressions of ln in vivo fluorescence versus time during the exponential phase of growth.
Seawater Temperature Conditions
Current surface seawater temperatures for the Southern Ocean were obtained from satellite data1 and match the temperature at which each species was isolated (Figure 1). To estimate future surface sweater temperatures in the Southern Ocean, we increased temperature by 2°C, in line with simulated predictions by Boyd et al. (2015) and Ito et al. (2015) and predicted by the RCP8.5 climate change scenario estimated in the Fifth Assessment Report of the Intergovernmental Panel on Climate Change (IPCC; Stocker et al., 2013).
In order to mimic the warming that polar phytoplankton might encounter over the coming century, Southern Ocean cultures were exposed to higher temperatures in a stepwise fashion over 2–4 months. After acclimation to each temperature, a subset of cultures was moved to a higher temperature, allowing at least ten generations of growth at each temperature. The cultures were subsequently acclimated to experimental conditions for a further 4 weeks before the experiment was initiated, and growth responses recorded. The higher temperature experimental cultures in this study were grown at 8, 10, 12, and 14°C in 3 L water baths under a mean continuous irradiance of 17 ± 5 μmol photons m–2 s–1 or 90 ± 10 μmol photons m–2 s–1 using LED lights. The temperature of each of the water baths was regulated using a PID temperature controller with a k type thermocouple thermometer. The lower temperature experimental cultures were grown at 3 and 5°C in an incubator under a continuous irradiance of 20 ± 5 μmol photons m–2 s–1 (low light treatment), 90 ± 5 μmol photons m–2 s–1 (saturating light treatment), or 200 ± 10 μmol photons m–2 s–1 (high light treatment); using Philips Alto II fluorescent tubes (Supplementary Table 1). The spectra for both light sources were measured using an Ocean Optic USB4000 spectrometer. Both light sources span the same wavelength range with a similar intensity. Growth saturating irradiances were determined in previous studies (Strzepek et al., 2012; Boyd et al., 2013; Boyd, 2019).
Cellular Physiology
Cell dimensions and culture density for C. flexuosus, P. inermis, and T. antarctica were determined by microscopy and cell volumes were calculated assuming a cylindrical geometry. Triplicate samples (1 mL) for cell diameter and culture density for P. antarctica were determined by Coulter Counter® (Model MS4) and cell volumes were calculated assuming a spherical geometry. This information was obtained from mid-exponential phase cultures at the same time as cells were collected for in vitro Chlorophyll a, cellular carbon and nitrogen, and Fast Repetition Rate fluorometry (FRRf) analyses.
Particulate Organic Carbon/Particulate Organic Nitrogen
Samples for cellular carbon (POC) and nitrogen (PON) were collected by filtering 25 ml of cells on to pre-combusted 13 mm GF/F filters (Merck Millipore). All filter holders and funnels were washed with 10% HCl, rinsed with deionized (Milli-Q®) water and then dried before use. The filters were then placed in sterile plastic wells and left to dry in an oven at 50°C for 2 weeks. The filters were then wrapped and stored at −80°C. A Sercon-Callisto CF-IRMS stable isotope analysis system was used for total organic carbon and organic nitrogen determination.
Chlorophyll a Analysis
Cells were collected for Chlorophyll a (Chl a) analysis from mid-exponential phase of growth. Cells (25–50 mL) were collected under a low vacuum (<100 mm Hg) onto 25 mm glass fiber filters (Whatman GF/F), rinsed three times with synthetic ocean water to ensure all cells were collected onto the filter and then stored frozen at −20°C in 15 mL centrifuge tubes. Pigments were extracted in 10 mL of 90% acetone and Chl a concentrations determined by in vitro fluorometry (Wright et al., 2005).
Fast Repetition Rate Fluorometry
Photochemical measurements of PSII were determined using a LIFT-FRR Fluorometer (Soliense, United States). After low light (∼15 μmol photons m–2 s–1) acclimation for ∼30 min, samples were exposed to 140 flashes of 470 nm light every 2.5 μs (saturation sequence) in order to saturate photosystem II (PSII) and the first stable electron acceptor, QA. The time interval between flashes was then increased exponentially (relaxation sequence) for 90 flashes to relax PSII and determine the rate of electron transport between the first stable electron acceptor, QA, through to Photosystem I (PSI). Fv/Fm and σPSII were determined from the mean of 200 iterations of the fluorescence induction and relaxation protocol.
Statistical Analyses
Statistical analysis was completed with R version 3.4. A three-way ANOVA was used to analyze statistical differences. Factors were Fe with two levels (Fe+ and Fe–), light with three levels (200 μmol photons m–2 s–1, 90 μmol photons m–2 s–1 and 20 μmol photons m–2 s–1) and temperature with six levels (3, 5, 8, 10, 12, and 14°C; Supplementary Tables 4, 5). To obtain specific information for each temperature, light and Fe condition a pairwise comparison of variables was undertaken using the Tukey post hoc test. All testing was done at the 95% confidence level.
Results
This study outlines the responses of four Southern Ocean species to multiple stressors or drivers, namely Fe, light, and temperature. Due to the complex interaction between experimental treatments, the temperature responses of each species will be separated into two sections. The first section will examine the thermal tolerance curves of the haptophyte P. antarctica, and the two diatoms C. flexuosus and T. antarctica grown under sub-saturating and saturating irradiances from 3 to 14°C in both high Fe and low Fe media (Supplementary Table 2). The second section compares the responses of four species grown at present-day (isolation temperature = 3°C) and future (projected 1.5–2°C increase in the Southern Ocean = 5°C) temperature conditions combined with variations in Fe and light availability. This section will also focus on the impact of temperature on growth rates and changes in cell physiology coupled with measurements of photochemical physiology (Supplementary Table 3).
Temperature Response Curves Under Combined Fe and Light Limitation
Consistent with our hypothesis that all species will respond in a similar way to warming, P. antarctica, C. flexuosus, and T. antarctica all responded positively to temperature increases up to 5°C, after which growth rates declined with subsequent temperature increases. This optimal growth temperature was observed at 5°C under both sub-saturating and saturating light (Figure 2). Despite having a temperature optimum comparable to the other species, C. flexuosus exhibited a different response to low Fe treatments when temperature was increased under saturating light. While P. antarctica and T. antarctica growth ceased at temperatures above about 10°C, and a greater tolerance to increased temperature was observed in high Fe cultures, C. flexuosus was able to withstand warming up to 12°C regardless of Fe supply.
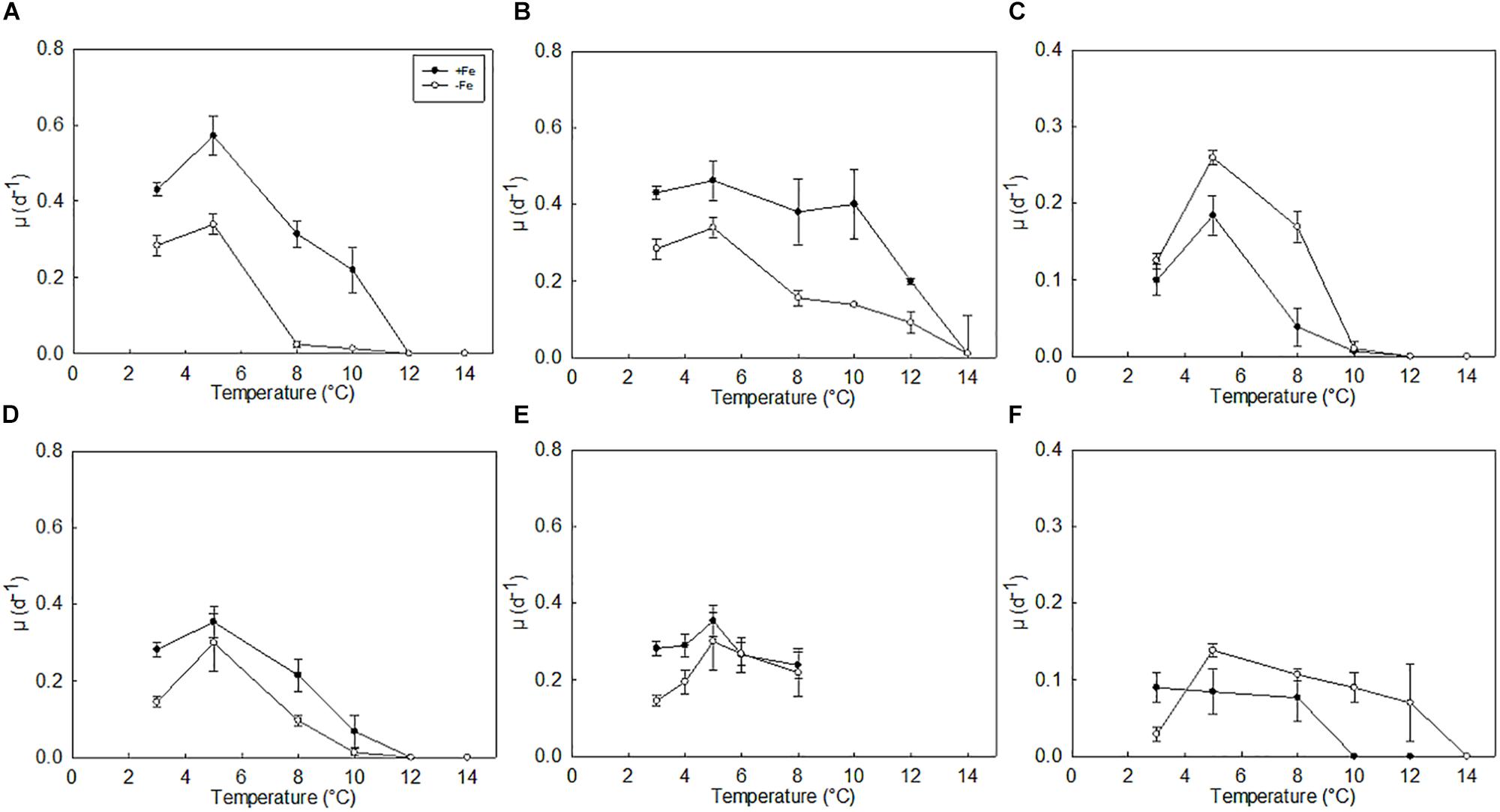
Figure 2. Thermal tolerance curves of P. antarctica (A,D); C. flexuosus (B,E); and T. antarctica (C,F); grown under low and high Fe concentrations at (A–C) growth saturating light (90 μmol photons m–2 s–1) or (D–F) growth limiting light levels (20 μmol photons m–2 s–1). Errors are standard deviation, n = 3.
Phaeocystis antarctica cultures grew more rapidly under saturating irradiance under high Fe conditions, with growth approximately 2-fold higher than when grown under low light conditions (p < 0.05; Figures 2A,D). Under low Fe conditions the growth rate for P. antarctica declined sharply under high light conditions beyond a temperature of 5°C. Under low light conditions at comparable iron and temperature conditions growth declined but not as dramatically, at 8°C growth rates were ∼0.1 ± 0.01 d–1 under low light and low Fe conditions, compared to the high light and low Fe treatment at 8°C where growth rates were reduced to ∼ 0.02 ± 0.01 d–1.
Chaetoceros flexuosus was unable to grow at 14°C when grown under high irradiance in both high Fe and low Fe conditions (Figures 2B,E). Under sub-saturating irradiance, C. flexuosus was only grown across a temperature range of 3–8°C. At temperatures above 5°C, Fe limitation was alleviated as growth rates for the low Fe and high Fe treatments converged (Figure 2E). C. flexuosus cultures grew more rapidly under saturating irradiance and under high Fe conditions, with growth approximately twofold higher at 5°C (0.46 ± 0.05 d–1) compared to the low light treatment (0.29 ± 0.03 d–1, p < 0.05; Figures 2B,E).
In contrast to the two other species, T. antarctica displayed an inconsistent growth response to the decrease in Fe concentration. Instead of the expected reduction of growth rate with the decrease of inorganic Fe concentration (Fe′), T. antarctica tended to have a higher growth rate when the Fe′ was reduced from 100–500 pmol L–1 (FeEDTA medium) to 3.7 pmol L–1 (FeDFB medium). This positive growth response to the reduction in Fe′ upon DFB addition to media in T. antarctica was observed under high and low irradiances (p < 0.05; Figures 2C,F). T. antarctica cultures were unable to grow at 12°C under high light, however, mortality of low Fe (FeDFB media) T. antarctica cultures was not realized until 14°C when grown under low light.
Contrary to our hypothesis that species will share the same response to warming, trends in the thermal performance of PSII (Fv/Fm and σPSII) differed between species. The photochemical efficiency (Fv/Fm) measurements of P. antarctica revealed different trends in treatments grown under high and low light, however, these differences due to changes in light are not significant (p > 0.05; Figure 3). Generally, in P. antarctica, Fv/Fm was unchanged between 3 and 5°C; above this temperature, Fv/Fm declined with increasing temperature (p < 0.05; Figure 3D). In contrast, Fv/Fm values for C. flexuosus did not change with increasing temperature when grown under high light (p > 0.05). Fv/Fm values for C. flexuosus grown under low light showed a subtle increase with increasing temperature (Figures 3B,E). In contrast to these two species, the Fv/Fm of T. antarctica cultures was highest under low light conditions and responded significantly to the individual and combined effects of light, Fe and temperature (p < 0.05; Figure 3F).
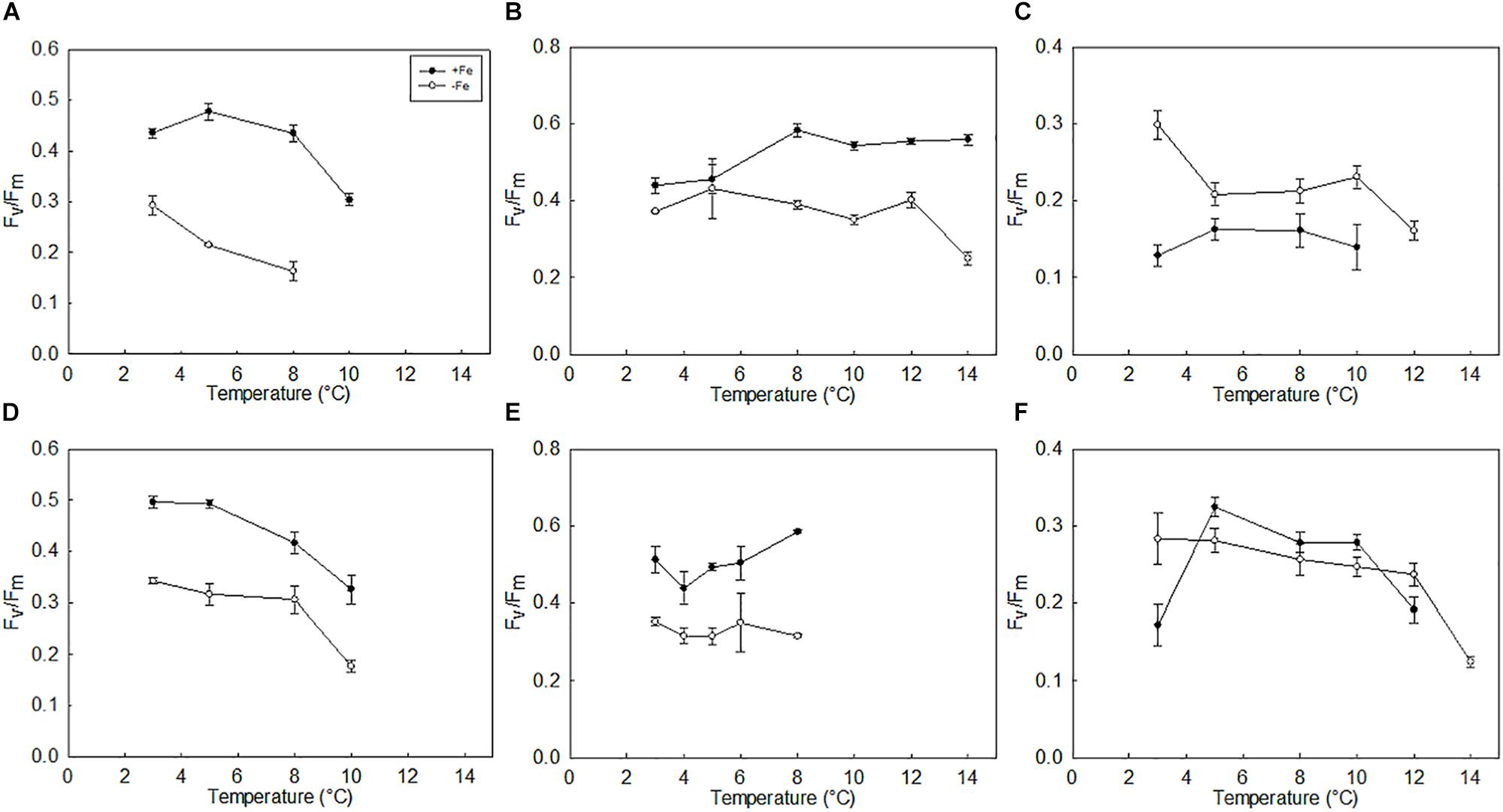
Figure 3. Photochemical efficiency (Fv/Fm) P. antarctica (A,D); C. flexuosus (B,E); and T. antarctica (C,F); grown under Fe limited and Fe replete conditions at (A–C) growth saturating light (90 μmol photons m–2 s–1) or (D–F) growth limiting light levels (20 μmol photons m–2 s–1). Errors are standard deviation, n = 3.
The functional absorption cross-section of PSII (σPSII) also varied between species in response to temperature light and Fe (Figure 4). High Fe concentrations decreased σPSII in all species except P. antarctica, as did increasing light intensity (p < 0.05). For cultures of P. antarctica, σPSII increased with increasing temperature (p < 0.05; Figures 4A,D). In contrast, σPSII for C. flexuosus remained constant under high light when temperature increased in both low and high Fe cultures (p < 0.05), but increased ∼2-fold in low light, low iron cultures in response to temperature (Figure 4E). In all treatments, σPSII for T. antarctica decreased with increasing temperature (p < 0.05; Figures 4C,F). Regardless of experimental conditions, σPSII was large, as previously observed for Southern Ocean species (Strzepek et al., 2012, 2019).
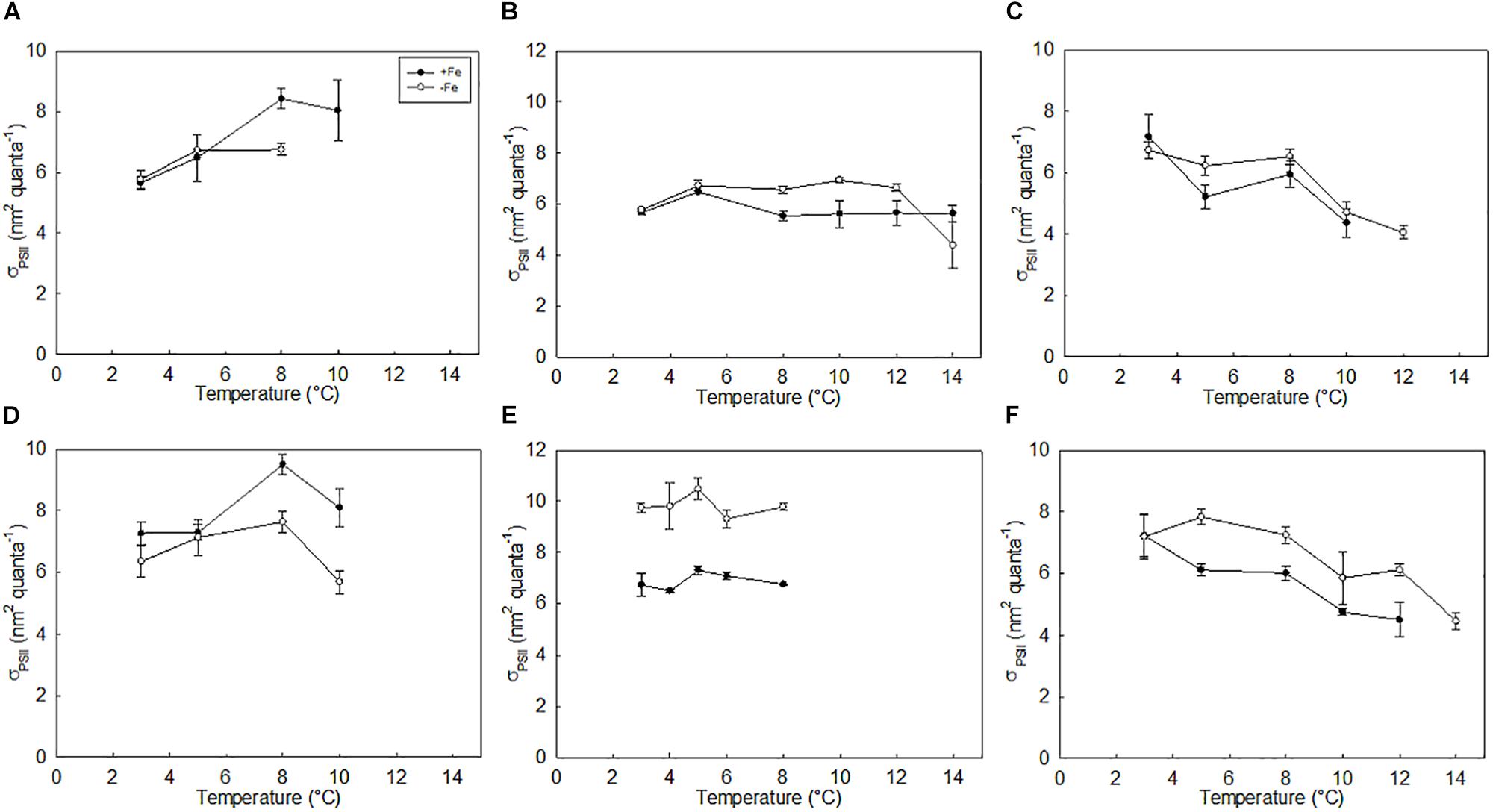
Figure 4. Functional absorption cross-section of PSII (σPSII) of P. antarctica (A,D); C. flexuosus (B,E); and T. antarctica (C,F); grown under Fe limited and Fe replete conditions at (A–C) growth saturating light (90 μmol photons m–2 s–1) or (D–F) growth limiting light levels (20 μmol photons m–2 s–1). Errors are standard deviation, n = 3.
Interactive Effects of Fe, Light, and Temperature on Southern Ocean Phytoplankton at 3 and 5°C
In vivo measurements of Chl a indicated uniform growth rates for all species over the 24 months the work was undertaken. These rates are largely comparable to those previously reported for treatments with each species grown under similar conditions (Strzepek et al., 2011, 2012; Boyd et al., 2013), except for T. antarctica which unexpectedly responded by increasing their growth rate in Fe deficient media (Figure 5). In this section, the growth responses of P. antarctica, T. antarctica, and C. flexuosus will be directly compared to each other and to P. inermis at ecologically relevant temperatures: isolation temperature of 3°C and a future warming scenario of 5°C, at high (200 μmol photons m–2 s–1) and low (20 μmol photons m–2 s–1) light.
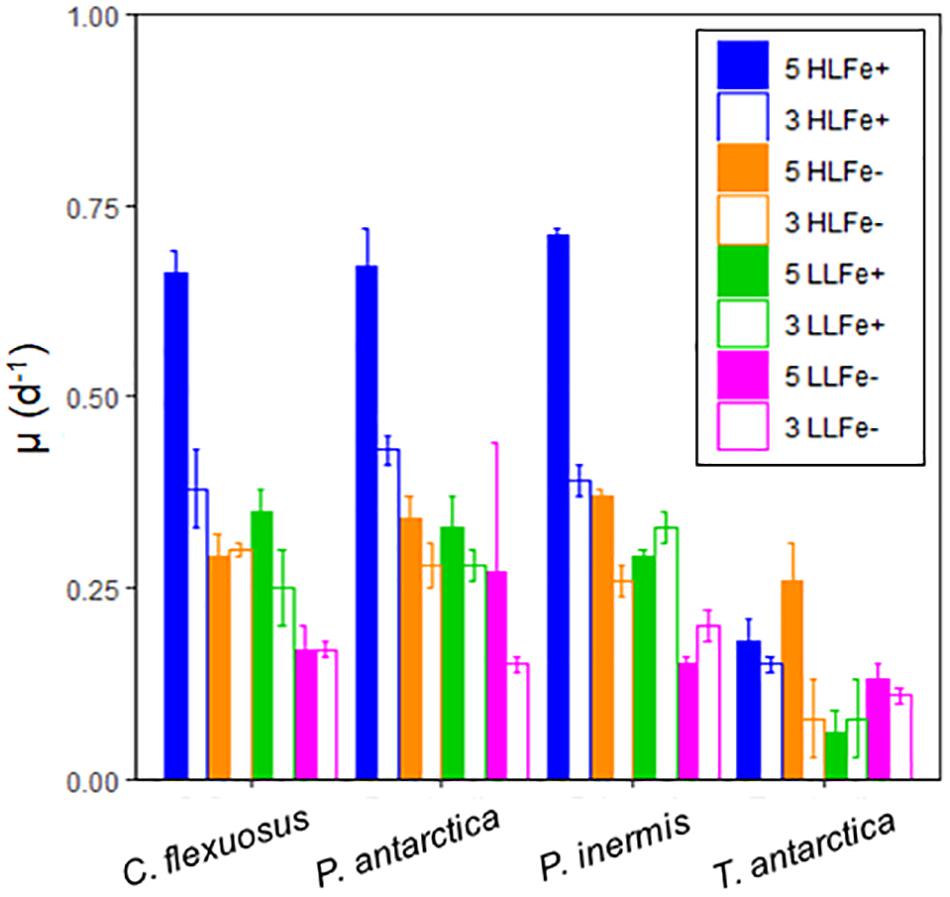
Figure 5. Specific growth rate (μ, d–1) responses of Southern Ocean phytoplankton species under high (5°C) and low (3°C) high temperature, high (200 μmol photons m–2 s–1) and low (20 μmol photons m–2 s–1) light, grown in high and low Fe media. Errors are standard deviation, n = 5.
The highest growth rates for P. antarctica, P. inermis, and C. flexuosus were observed in cultures grown at 5°C under high light and Fe replete conditions (0.67 ± 0.05, 0.71 ± 0.02, and 0.66 ± 0.03 d–1, respectively; Figure 5). In comparison, at 3°C growth rates for these species were significantly lower (p < 0.05) with values: 0.43 ± 0.02, 0.39 ± 0.02, and 0.38 ± 0.05 d–1 for the same Fe and light conditions. P. inermis was the only species observed to have growth negatively affected by increased temperature in some treatments (Figure 5). Under low light conditions at 5°C, the growth rate for P. inermis decreased in both the high Fe and low Fe treatments in comparison to the low temperature high Fe and low Fe treatments. The optimum growth rates for T. antarctica were observed in cultures grown in low Fe media (p < 0.05). This unexpected growth rate under low Fe was tested across multiple batches of FeEDTA (high Fe) media and FeDFB (low Fe) media at 3°C over a 24-month period with consistent results across experiments.
Increasing temperature from 3 to 5°C generally increased C:N ratio across all treatments in P. antarctica, P. inermis, and C. flexuosus but not T. antarctica (p < 0.05; Figure 6A). In contrast, C:N mostly decreased or remained the same when temperature increased in T. antarctica. Warming elevated C:N in T. antarctica when grown under low light and high Fe treatment, however, this relationship was not significant (p > 0.05). C:N ratio was also found to be significantly correlated with Fv/Fm and Chl a in cultures of C. flexuosus (p < 0.05).
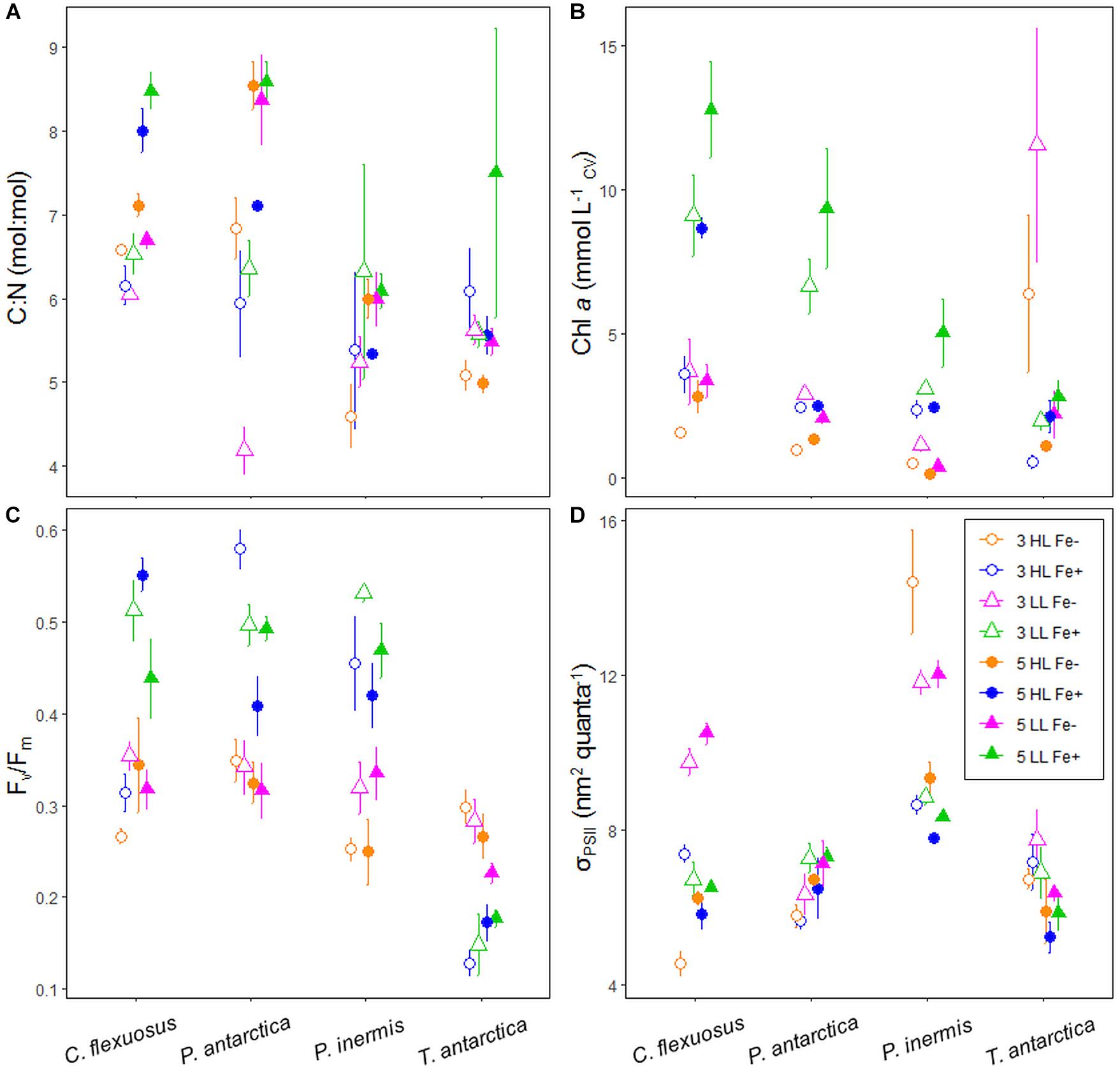
Figure 6. (A) Elemental stoichiometry (C:N), (B) Chlorophyll a concentration normalized to cell volume (mmol L–1CV), (C) photochemical efficiency of PSII (Fv/Fm), and (D) functional absorption cross-section of PSII (σPSII) of Southern Ocean phytoplankton exposed to changes in light, temperature and Fe. Errors are standard deviation, n = 3.
The three fastest growing species in this study, P. antarctica, P. inermis, and C. flexuosus displayed similar relationship in their Chl a concentration per cell volume. The highest Chl a concentrations were observed for the 5°C low light high Fe treatments (p < 0.05; Figure 6B). The lowest Chl a concentrations were generally observed for the high light and low Fe treatments. Increasing temperature generally increased Chl a concentrations regardless of light or Fe supply, for P. antarctica, P. inermis, C. flexuosus, and T. antarctica with a couple of exceptions (Figure 6B). Chl a concentrations of T. antarctica followed a similar pattern to the other study species despite the high growth rate observed in low Fe medium. Increasing temperature under low light and low Fe resulted in a decrease in Chl a concentration for P. antarctica and P. inermis (p < 0.05).
Fv/Fm values for P. antarctica, P. inermis, and C. flexuosus were generally elevated in high light and high Fe treatments (at 3°C, 0.57 ± 0.01, 0.44 ± 0.01, and 0.31 ± 0.01 d–1). In contrast, the highest Fv/Fm values were measured for T. antarctica in the low Fe treatments (at 3°C: 0.30 ± 0.02, high light; 0.28 ± 0.03, low light). Light had a significant effect on Fv/Fm in all species except P. antarctica (p > 0.05; Figure 6C). Warming also had a significant effect on Fv/Fm in all species except T. antarctica (p < 0.05). A significant positive relationship was observed between Chl a and Fv/Fm but only in C. flexuosus and P. inermis (p < 0.05).
σPSII values for P. antarctica, P. inermis and C. flexuosus generally increased with higher temperature with some important exceptions (p < 0.05; Figure 6D). At high light and high Fe σPSII decreased due to temperature in cultures of P. inermis, C. flexuosus, and T. antarctica. σPSII was consistently larger in P. inermis than other study species when experimental treatments were compared, with the largest σPSII measured in cultures of all species grown at 5°C under low light and low Fe conditions. A significant negative relationship was observed between growth and σPSII but only in P. inermis (p < 0.05).
Discussion
The Southern Ocean possesses distinct flora due to its unique environmental conditions and geographical isolation. The principal goal of this research is to predict how such isolated populations will fare in a rapidly changing ocean by first understanding how these primary producers respond to the three major environmental factors that limit primary productivity in the Southern Ocean – low iron supply, low temperatures, and low underwater light levels. Previous research has revealed systematic differences occur between “model” diatom species (typically coastal, temperate isolates) and Southern Ocean diatoms, pointing to adaptations in Southern Ocean species that allow them to overcome the increase in cellular Fe requirements that generally occurs with decreasing light (Strzepek et al., 2012, 2019). Similar to Boyd (2019), we found that low Fe conditions resulted in mortality at a lower temperature than high Fe cultures while the optimal growth temperature in our study agrees with the calculated growth temperature optimum of 5.2°C for Southern Ocean phytoplankton (Coello-Camba and Agustí, 2017).
The temporal succession of Southern Ocean phytoplankton is not fully resolved, with multiple suggestions as to what triggers the change from one dominant group over another (Petrou et al., 2016). It is suggested that over winter, the deep mixed layer regenerates the surface nutrients and Fe supply, while in spring as the sea surface temperatures increases and winds decrease, the mixed layer shallows – therefore increasing the total photosynthetic active radiation (PAR) allowing early spring blooming cells to bloom (Boyd et al., 2010). Following this, and as Fe supply declines due to Fe uptake by larger cells, smaller cells with lower Fe requirements would tend to dominate (Tagliabue et al., 2014). While temperature is projected to increase in the future, irradiance is also predicted to increase due to a decrease in mixed layer depth (Boyd et al., 2015). However, there are still uncertainties surrounding future Fe supply, which hinders our understanding of primary productivity in the Southern Ocean (Laufkötter and Gruber, 2018).
The Peculiarities of T. antarctica With Respect to Fe Supply
The optimal growth rate for T. antarctica is less clear due to its difficulty to be cultured at elevated Fe concentrations, i.e., Fe:EDTA media (Figure 5). The growth rates of T. antarctica grown in high Fe media and 90 μmol photons m–2 s–1 at 3°C in our study are similar to those reported by Strzepek et al. (2011). However, previously reported growth rates of T. antarctica grown in low Fe media are significantly lower than our results even though the experimental conditions in our study are comparable to those used in past studies (Strzepek et al., 2011).
Surprisingly, Fv/Fm values for T. antarctica did not change appreciably in cultures grown under high light conditions and at 10°C in high Fe medium, or at 12°C in low Fe medium, even though growth rates were severely reduced in these treatments (p < 0.05; Figures 3C,F). This finding suggests that at these higher temperatures, T. antarctica cells appear photosynthetically competent but for some unknown reason are unable to divide. We cannot exclude the possibility that cells were undergoing sexual reproduction and hence stopped dividing asexually.
It has been shown that T. antarctica lack the gene ferritin (FTN) (Moreno et al., 2018). This ability to store Fe via FTN may mean that Fe may be “toxic” at higher concentrations. The higher growth rates observed for T. antarctica under Fe deficient conditions may also be due to the presence of DFB in the media. We propose that this key difference in chelator, i.e., EDTA vs. DFB, may cause Fe to be held in solution in a more desirable form for uptake by the cell. We suggest that growth experiments should be considered to understand the response of T. antarctica cultures grown in different media containing an assortment of ligands to test our hypothesis regarding preference for Fe uptake.
Thermal Response Curves of Southern Ocean Phytoplankton
To understand changes in the ability to sequester and export carbon due to climate-induced alterations in net primary productivity it is crucial to understand the underlying mechanisms that phytoplankton use to cope with environmental change (Padfield et al., 2016). Photophysiological measurements are used routinely to quantify the quantum yield of photochemistry in PSII (Fv/Fm) and are derived from the three possible paths for solar energy absorbed by photosynthesizing organisms (Falkowski and Raven, 2007). Absorbed photons can be (a) used for charge separation events leading to carbon (organic) synthesis, (b) be dissipated as heat, or (c) emitted back to the environment as fluorescence (Butler and Strasser, 1977). Such measurements have been used to suggest that compared to temperate coastal diatoms, Southern Ocean phytoplankton compensate for low Fe conditions by modifying their photosynthetic machinery to capture light when irradiance is limiting by modifying the size rather than the number of photosynthetic units (Strzepek et al., 2019).
Typically, σPSII (PSII absorption cross-section area) increases under low Fe conditions, either due to an increase in the number of PSII antennae complexes relative to reaction center complexes or an apparent increase in absorption cross-section area due to the decoupling of light harvesting antennae from PSII reaction center complexes (Greene et al., 1991; Behrenfeld et al., 2008; Strzepek et al., 2012). While σPSII was observed to increase for C. flexuosus and T. antarctica under low Fe conditions, σPSII increased under high conditions for P. antarctica. The coupled increase in photochemical efficiency (Fv/Fm) and antennae size (σPSII) for P. antarctica (Figures 3, 4) cultured under high Fe conditions at all temperatures are inconsistent with published literature and unexpected due to the decreased probability of excitation energy transfer to the reaction centers associated with larger antennae size (Kolber et al., 1994; Strzepek et al., 2012). The combination of increased Chl a and σPSII size may provide insight into these conflicting results, as the increase of photosynthetic pigments energetically coupled to PSII reaction centers in high Fe cells may exceed the degree of decoupling of the PSII reaction center from light harvesting antennae (reflected as an increase in σPSII) typically observed under Fe limitation. By reducing the amount of photons that are absorbed by the PSII reaction center, this unexpected increase in σPSII and Fv/Fm may suggest that P. antarctica can withstand higher light intensities without compromising the photosynthetic efficiency of PSII.
While our study shows that biological activity increases with temperature up to an optimal temperature of 5°C, most photochemical reactions are thought to be temperature independent (Somero and Hochachka, 1976). That said, thermal stress has been shown to damage the photosynthetic apparatus (Schreiber and Berry, 1977) and cause the PSII reaction centers and light-harvesting complex of PSII to functionally and physically dissociate (Armond et al., 1980). Thermal damage to PSII was most obviously observed in P. antarctica, shown as a decrease in Fv/Fm and an increase in σPSII with warming (Figures 3, 4). This was observed under both experimental irradiances. The photochemical physiology of T. antarctica was less affected by temperature, with a slight negative relationship between Fv/Fm and σPSII with warming. In contrast, warming had little effect on Fv/Fm and σPSII of C. flexuosus, expressed as a flat response curve, suggesting the photosynthetic apparatus of C. flexuosus and T. antarctica are thermally stable (Baker et al., 2016).
Southern Ocean Phytoplankton Responses to Climate Change
In addition to predicted changes in light and Fe availability in the future (Boyd et al., 2015), the results from this study suggest that the 2°C temperature increase estimated for the Southern Ocean at the end of this century (Ito et al., 2015), will result in significant ecological and biochemical changes to the Southern Ocean. P. antarctica plays a major role in the export of carbon in the Southern Ocean (Arrigo et al., 1999), while P. inermis has been shown to dominate austral spring/summer blooms, often outlasting other species into late summer (Annett et al., 2010; Lin et al., 2017). As the ocean warms, this will stimulate growth under high Fe conditions and high light (Figure 6). However, each species responds differently to other combinations of temperature, Fe and light supply – suggesting extensions or decreases in temporal bloom formation (Figures 1, 6). It is also apparent that populations of P. inermis would diminish as they do not grow as well at higher temperatures (Figure 7).
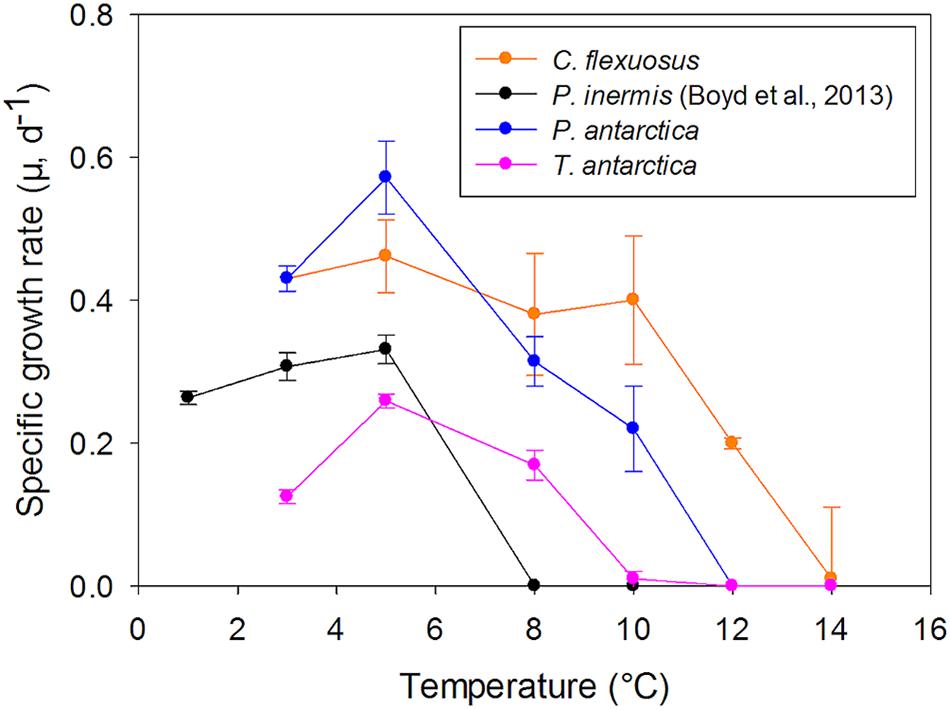
Figure 7. Comparison of thermal tolerance curves of Southern Ocean phytoplankton grown under nutrient replete conditions at 90 μmol photons m–2 s–1). Errors are standard error, n = 3–6. Growth data for P. inermis from Boyd et al. (2013).
The Southern Ocean has a distinct diatom community due to ocean circulation barriers, e.g., the Antarctic Circumpolar Current (ACC) (Malviya et al., 2016). But recently it has been shown that large temperate macroalgae (southern bull kelp: Durvillaea antarctica) can frequently disperse across the ACC and it is the extreme conditions of the Southern Ocean that prevent the establishment of temperate-adapted taxa (Fraser et al., 2018). Thus as the Southern Ocean warms, it may become more habitable for species with a higher thermal tolerance while becoming less hospitable for species with a lower thermal tolerance (Griffiths et al., 2017). If the Ross Sea warms above 8°C, the distribution and abundance of phytoplankton would change to favor species that retain the ability to photosynthesize optimally at elevated temperatures. The generalist Chaetoceros genus is one of the most abundant globally (Suto, 2006), thus it is likely that this genus can inhabit empty niches much faster than specialist species (Sriswasdi et al., 2017). This is due to the high speciation rates measured in generalist species and their ability to tolerate environmental change (Birand et al., 2011; Vamosi et al., 2014). It is likely that our study species C. flexuosus recently evolved from a temperate ancestor and has adapted to tolerate the low light and Fe environment of the Southern Ocean. Thus, it is likely that its heat resistant cellular functions will give it a competitive advantage over polar diatoms as anthropogenic CO2 emissions and sea surface temperatures increase.
Like temperature, the light climate that phytoplankton will be exposed to in the future will vary. As the oceans warm and stratify, phytoplankton will be exposed to increased irradiances and damaging radiation (Doney, 2006). This could reduce rates of photosynthesis, growth and survival in phytoplankton species that are unable to successfully tune their photosynthetic repair and protection strategies to regulate excitation pressure. In turn, high temperatures can increase enzymatic turnover rates (therefore productivity) through increasing activity of thermally sensitive enzymes or accelerating protein synthesis (Boyd et al., 2016; Wagner et al., 2016). Indigenous Southern Ocean phytoplankton have specific molecular and physiological adaptations to this low Fe and low light polar region (Strzepek et al., 2019), however, their narrow temperature tolerances (e.g., 0–8°C) will limit their ability to adapt to increasing sea surface temperatures (Libralato et al., 2015).
Studies on the intraspecies variation in resident Antarctic phytoplankton species show there is some phenotypic plasticity to temperature (Reusch and Boyd, 2013) and light and Fe availability (Luxem et al., 2017), thus phytoplankton may not require physiological or molecular adaptations to photosynthesize optimally in the cold, low light and low Fe environment (Boyd, 2019). We propose that invading species with generalist lineages and high temperature tolerances may dominate blooms under future Southern Ocean conditions instead of species that have more specialized adaptations. However, due to the lack of evolutionary studies on Antarctic phytoplankton, the implications of physiological plasticity of polar species to environmental conditions are limited, therefore, it is difficult to extend predictions to the ecosystem level.
Conclusion
In this study, we demonstrate the importance of investigating the response of non-model phytoplankton species isolated from the same region to assess their thermal optima. Phytoplankton species were cultured using a matrix of environmental conditions to determine any synergistic or antagonistic effects of light, temperature and iron limitation on phytoplankton physiology. Our results clearly show that Fe and light availability affect not only growth rate, but also the temperature range over which growth can occur. Iron stressed cells generally reached mortality at lower temperatures than high Fe cells.
The unique temperature reaction norms observed in this study show that polar phytoplankton utilize different specializations that allow them to coexist in the Southern Ocean, which is probably indicative of their different evolutionary history. Furthermore, Fe deficiency decreases the upper thermal limit for growth, which agrees with recent studies on temperate and polar phytoplankton (Thomas et al., 2017; Boyd, 2019). The high light and low Fe conditions expected in the austral spring/summer conditions in 2100 will limit the productivity of some species while enhancing the growth of others.
The question about the variation in thermal tolerance raised in this study suggests that understanding a species evolutionary history will play a key role in predicting the success of future Antarctica phytoplankton populations. More information on genetic differences between phytoplankton displaying stark differences in their thermal tolerances would help to establish more accuracy in understanding how species will respond to future climate changes.
Data Availability Statement
All datasets generated for this study are included in the article/Supplementary Material.
Author Contributions
SA, RS, HM, and ME designed the research. SA and HM performed the research. SA wrote the manuscript with contributions from ME, RS, and PB.
Funding
This work was supported by an Australian Government Research Training Program Stipend Scholarship (SA) and Australian Research Council’s Discovery Program (DP170102108 and DP130100679).
Conflict of Interest
The authors declare that the research was conducted in the absence of any commercial or financial relationships that could be construed as a potential conflict of interest.
Supplementary Material
The Supplementary Material for this article can be found online at: https://www.frontiersin.org/articles/10.3389/fmars.2019.00681/full#supplementary-material
Footnotes
References
Annett, A. L., Carson, D. S., Crosta, X., Clarke, A., and Ganeshram, R. S. (2010). Seasonal progression of diatom assemblages in surface waters of ryder bay, Antarctica. Polar Biol. 33, 13–29.
Armond, P. A., Björkman, O., and Staehelin, L. A. (1980). Dissociation of supramolecular complexes in chloroplast membranes a manifestation of heat damage to the photosynthetic apparatus. Biochim. Biophy. Acta 601, 433–442.
Arrigo, K. R., Robinson, D. H., Worthen, D. L., Dunbar, R. B., Ditullio, G. R., Vanwoert, M., et al. (1999). Phytoplankton community structure and the drawdown of nutrients and CO2 in the southern ocean. Science 283, 365–367. doi: 10.1126/science.283.5400.365
Baker, K. G., Robinson, C. M., Radford, D. T., Mcinnes, A. S., Evenhuis, C., and Doblin, M. A. (2016). Thermal performance curves of functional traits aid understanding of thermally induced changes in diatom-mediated biogeochemical fluxes. Front. Mar. Sci. 3:44. doi: 10.3389/fmars.2016.00044
Behrenfeld, M. J., Halsey, K. H., and Milligan, A. J. (2008). Evolved physiological responses of phytoplankton to their integrated growth environment. Philos. Trans.R. Soc. B Biol. Sci. 363, 2687–2703. doi: 10.1098/rstb.2008.0019
Birand, A., Vose, A., and Gavrilets, S. (2011). Patterns of species ranges, speciation, and extinction. Am. Nat. 179, 1–21. doi: 10.1086/663202
Blain, S., Quéguiner, B., Armand, L., Belviso, S., Bombled, B., Bopp, L., et al. (2007). Effect of natural iron fertilization on carbon sequestration in the southern ocean. Nature 446, 1070–1074.
Boyd, P., and Law, C. (2001). The southern ocean iron release experiment (SOIREE)—introduction and summary. Deep Sea Res. Part II Oceanogr. Res. Pap. 48, 2425–2438. doi: 10.1016/s0967-0645(01)00002-9
Boyd, P. W. (2019). Physiology and iron modulate diverse responses of diatoms to a warming southern ocean. Nat. Clim. Change 9, 148–152. doi: 10.1038/s41558-018-0389-1
Boyd, P. W., Dillingham, P. W., Mcgraw, C. M., Armstrong, E. A., Cornwall, C. E., Feng, Y. Y., et al. (2016). Physiological responses of a southern ocean diatom to complex future ocean conditions. Nat. Clim Change 6, 207–213. doi: 10.1038/nclimate2811
Boyd, P. W., Lennartz, S. T., Glover, D. M., and Doney, S. C. (2015). Biological ramifications of climate-change-mediated oceanic multi-stressors. Nat. Clim. Change 5, 71–79. doi: 10.1038/nclimate2441
Boyd, P. W., Rynearson, T. A., Armstrong, E. A., Fu, F. X., Hayashi, K., Hu, Z. X., et al. (2013). Marine phytoplankton temperature versus growth responses from polar to tropical waters - outcome of a scientific community-wide study. PLoS One 8:e63091. doi: 10.1371/journal.pone.0063091
Boyd, P. W., Strzepek, R., Fu, F., and Hutchins, D. A. (2010). Environmental control of open-ocean phytoplankton groups: now and in the future. Limnol. Oceanogr. 55:1353. doi: 10.4319/lo.2010.55.3.1353
Brand, L. E., Guillard, R. R., and Murphy, L. S. (1981). A method for the rapid and precise determination of acclimated phytoplankton reproduction rates. J. Plankton Res. 3, 193–201. doi: 10.1093/plankt/3.2.193
Butler, W. L., and Strasser, R. J. (1977). Tripartite model for the photochemical apparatus of green plant photosynthesis. Proc. Natl. Acad. Sci. U.S.A. 74, 3382–3385. doi: 10.1073/pnas.74.8.3382
Coello-Camba, A., and Agustí, S. (2017). Thermal thresholds of phytoplankton growth in polar waters and their consequences for a warming polar ocean. Front. Mar. Sci. 4:168. doi: 10.3389/fmars.2017.00168
Doney, S. C. (2006). Oceanography: plankton in a warmer world. Nature 444, 695–696. doi: 10.1038/444695a
Falkowski, P. G., and Raven, J. A. (2007). Aquatic Photosynthesis, 2nd Edn. Princeton, NJ: Princeton University Press.
Feng, Y., Hare, C., Rose, J., Handy, S., Ditullio, G., Lee, P., et al. (2010). Interactive effects of iron, irradiance and CO2 on ross sea phytoplankton. Deep Sea Res. Part I Oceanogr. Res. Pap. 57, 368–383. doi: 10.1016/j.dsr.2009.10.013
Fraser, C. I., Morrison, A. K., Hogg, A. M., Macaya, E. C., Van Sebille, E., Ryan, P. G., et al. (2018). Antarctica’s ecological isolation will be broken by storm-driven dispersal and warming. Nat. Clim. Change 8, 704–708. doi: 10.1038/s41558-018-0209-7
Greene, R. M., Geider, R. J., and Falkowski, P. G. (1991). Effect of iron limitation on photosynthesis in a marine diatom. Limnol. Oceanogr. 36, 1772–1782. doi: 10.1111/jpy.12607
Greene, R. M., Geider, R. J., Kolber, Z., and Falkowski, P. G. (1992). Iron-induced changes in light harvesting and photochemical energy conversion processes in eukaryotic marine algae. Plant Physiol. 100, 565–575. doi: 10.1104/pp.100.2.565
Griffiths, H. J., Meijers, A. J., and Bracegirdle, T. J. (2017). More losers than winners in a century of future Southern Ocean seafloor warming. Nat. Clim. Chang. 7, 749–754. doi: 10.1038/nclimate3377
Ito, T., Bracco, A., and Deutsch, C. (2015). The future of the southern ocean carbon storage in CMIP5 models. US CLIVAR 13, 24–28.
Kolber, Z. S., Barber, R. T., Coale, K. H., Fitzwateri, S. E., Greene, R. M., Johnson, K. S., et al. (1994). Iron limitation of phytoplankton photosynthesis in the equatorial pacific ocean. Nature 371, 145–149. doi: 10.1371/journal.pone.0004601
Kudo, I., Miyamoto, M., Noiri, Y., and Maita, Y. (2000). Combined effects of temperature and iron on the growth and physiology of the marine diatom Phaeodactylum tricornutum (Bacillariophyceae). J. Phycol. 36, 1096–1102. doi: 10.1046/j.1529-8817.2000.99042.x
Laufkötter, C., and Gruber, N. (2018). Will marine productivity wane? Science 359, 1103–1104. doi: 10.1126/science.aat0795
Laufkötter, C., Vogt, M., Gruber, N., Aita-Noguchi, M., Aumont, O., Bopp, L., et al. (2015). Drivers and uncertainties of future global marine primary production in marine ecosystem models. Biogeosciences 12, 6955–6984. doi: 10.5194/bg-12-6955-2015
Libralato, S., Caccin, A., and Pranovi, F. (2015). Modeling species invasions using thermal and trophic niche dynamics under climate change. Front. Mar. Sci. 2:29. doi: 10.3389/fmars.2015.00029
Lin, Y., Cassar, N., Marchetti, A., Moreno, C., Ducklow, H., and Li, Z. (2017). Specific eukaryotic plankton are good predictors of net community production in the western antarctic peninsula. Sci. Rep. 7:14845. doi: 10.1038/s41598-017-14109-1
Luxem, K. E., Ellwood, M. J., and Strzepek, R. F. (2017). Intraspecific variability in Phaeocystis antarctica’s response to iron and light stress. PLoS One 12:e0179751. doi: 10.1371/journal.pone.0179751
Malviya, S., Scalco, E., Audic, S., Vincent, F., Veluchamy, A., Poulain, J., et al. (2016). Insights into global diatom distribution and diversity in the world’s ocean. Proc. Natl. Acad. Sci. U.S.A. 113, E1516–E1525. doi: 10.1073/pnas.1509523113
Moreno, C. M., Lin, Y., Davies, S., Monbureau, E., Cassar, N., and Marchetti, A. (2018). Examination of gene repertoires and physiological responses to iron and light limitation in southern ocean diatoms. Polar. Biol. 41, 679–696. doi: 10.1007/s00300-017-2228-7
Padfield, D., Yvon-Durocher, G., Buckling, A., Jennings, S., and Yvon-Durocher, G. (2016). Rapid evolution of metabolic traits explains thermal adaptation in phytoplankton. Ecol. Lett. 19, 133–142. doi: 10.1111/ele.12545
Petrou, K., Kranz, S. A., Trimborn, S., Hassler, C. S., Ameijeiras, S. B., Sackett, O., et al. (2016). Southern ocean phytoplankton physiology in a changing climate. J. Plant Physiol. 203, 135–150. doi: 10.1016/j.jplph.2016.05.004
Price, N. M., Harrison, G. I., Hering, J. G., Hudson, R. J., Nirel, P. M., Palenik, B., et al. (1989). Preparation and chemistry of the artificial algal culture medium Aquil. Biol. Oceanogr. 6, 443–461.
Raven, J. A. (1990). Predictions of Mn and Fe use efficiencies of phototrophic growth as a function of light availability for growth and of C assimilation pathway. New Phytol. 116, 1–18. doi: 10.1111/j.1469-8137.1990.tb00505.x
Reusch, T. B., and Boyd, P. W. (2013). Experimental evolution meets marine phytoplankton. Evolution 67, 1849–1859. doi: 10.1111/evo.12035
Rose, J. M., Feng, Y., DiTullio, G. R., Dunbar, R. B., Hare, C. E., Lee, P. A., et al. (2009). Synergistic effects of iron and temperature on Antarctic phytoplankton and microzooplankton assemblages. Biogeosciences 6, 3131–3147. doi: 10.5194/bg-6-3131-2009
Schreiber, U., and Berry, J. A. (1977). Heat-induced changes of chlorophyll fluorescence in intact leaves correlated with damage of the photosynthetic apparatus. Planta 136, 233–238. doi: 10.1007/BF00385990
Somero, G., and Hochachka, P. (1976). ”Biochemical Adaptations to Temperature,” in Adaptation to Environment: Essays on the Physiology of Marine Animals. London: Butterworths, 125–190.
Sriswasdi, S., Yang, C.-C., and Iwasaki, W. (2017). Generalist species drive microbial dispersion and evolution. Nat. Commun. 8:1162. doi: 10.1038/s41467-017-01265-1
Stocker, T. F., Qin, D., Plattner, G.-K., Tignor, M., Allen, S. K., Boschung, J., et al. (2013). Climate Change 2013. The Physical Science Basis. Working Group I Contribution to the Fifth Assessment Report of the Intergovernmental Panel on Climate Change-Abstract for Decisionmakers (Geneva: IPCC).
Strzepek, R. F., Boyd, P. W., and Sunda, W. G. (2019). Photosynthetic adaptation to low iron, light, and temperature in southern ocean phytoplankton. Proc.Natl. Acad. Sci. U.A.S. 116, 4388–4393. doi: 10.1073/pnas.1810886116
Strzepek, R. F., and Harrison, P. J. (2004). Photosynthetic architecture differs in coastal and oceanic diatoms. Nature 431, 689–692. doi: 10.1038/nature02954
Strzepek, R. F., Hunter, K. A., Frew, R. D., Harrison, P. J., and Boyd, P. W. (2012). Iron-light interactions differ in southern ocean phytoplankton. Limnol. Oceanogr. 57, 1182–1200. doi: 10.4319/lo.2012.57.4.1182
Strzepek, R. F., Maldonado, M. T., Hunter, K. A., Frew, R. D., and Boyd, P. W. (2011). Adaptive strategies by southern ocean phytoplankton to lessen iron limitation: uptake of organically complexed iron and reduced cellular iron requirements. Limnol. Oceanogr. 56:1983. doi: 10.4319/lo.2011.56.6.1983
Sunda, W. G., and Huntsman, S. A. (1997). Interrelated influence of iron, light and cell size on marine phytoplankton growth. Nature 390, 389–392. doi: 10.1038/37093
Sunda, W. G., and Huntsman, S. A. (2003). Effect of pH, light, and temperature on Fe-EDTA chelation and Fe hydrolysis in seawater. Mar. Chem. 84, 35–47. doi: 10.1016/s0304-4203(03)00101-4
Sunda, W. G., and Huntsman, S. A. (2011). Interactive effects of light and temperature on iron limitation in a marine diatom: implications for marine productivity and carbon cycling. Limnol. Oceanogr. 56, 1475–1488. doi: 10.4319/lo.2011.56.4.1475
Suto, I. (2006). The explosive diversification of the diatom genus Chaetoceros across the Eocene/Oligocene and Oligocene/Miocene boundaries in the Norwegian Sea. Mar. Micropaleontol. 58, 259–269. doi: 10.1016/j.marmicro.2005.11.004
Tagliabue, A., Sallée, J.-B., Bowie, A. R., Lévy, M., Swart, S., and Boyd, P. W. (2014). Surface-water iron supplies in the southern ocean sustained by deep winter mixing. Nat. Geosci. 7, 314–320. doi: 10.1038/ngeo2101
Thomas, M. K., Aranguren-Gassis, M., Kremer, C. T., Gould, M. R., Anderson, K., Klausmeier, C. A., et al. (2017). Temperature–nutrient interactions exacerbate sensitivity to warming in phytoplankton. Glob. Change Biol. 23, 3269–3280. doi: 10.1111/gcb.13641
Thomas, M. K., Kremer, C. T., Klausmeier, C. A., and Litchman, E. (2012). A global pattern of thermal adaptation in marine phytoplankton. Science 338, 1085–1088. doi: 10.1126/science.1224836
Vamosi, J. C., Armbruster, W. S., and Renner, S. S. (2014). Evolutionary ecology of specialization: insights from phylogenetic analysis. Proc Biol Sci. 281:20142004. doi: 10.1098/rspb.2014.2004
Wagner, H., Jakob, T., Lavaud, J., and Wilhelm, C. (2016). Photosystem II cycle activity and alternative electron transport in the diatom Phaeodactylum tricornutum under dynamic light conditions and nitrogen limitation. Photosynth. Res. 128, 151–161. doi: 10.1007/s11120-015-0209-7
Wright, S., Jeffrey, S., and Mantoura, R. (2005). Phytoplankton Pigments in Oceanography: Guidelines to Modern Methods. California, CA: UNESCO Pub.
Keywords: temperature, climate change, photosynthesis, evolution, multiple stressors, carbon
Citation: Andrew SM, Morell HT, Strzepek RF, Boyd PW and Ellwood MJ (2019) Iron Availability Influences the Tolerance of Southern Ocean Phytoplankton to Warming and Elevated Irradiance. Front. Mar. Sci. 6:681. doi: 10.3389/fmars.2019.00681
Received: 04 April 2019; Accepted: 21 October 2019;
Published: 01 November 2019.
Edited by:
Elizabeth A. Fulton, Commonwealth Scientific and Industrial Research Organisation (CSIRO), AustraliaReviewed by:
Matthew McGinness Mills, Stanford University, United StatesKatherina Petrou, University of Technology Sydney, Australia
Copyright © 2019 Andrew, Morell, Strzepek, Boyd and Ellwood. This is an open-access article distributed under the terms of the Creative Commons Attribution License (CC BY). The use, distribution or reproduction in other forums is permitted, provided the original author(s) and the copyright owner(s) are credited and that the original publication in this journal is cited, in accordance with accepted academic practice. No use, distribution or reproduction is permitted which does not comply with these terms.
*Correspondence: Sarah M. Andrew, sarah.andrew@unc.edu