Trophic Dynamics of Zooplankton Before and After Polar Night in the Kongsfjorden (Svalbard): Evidence of Trophic Position Estimated by δ15N Analysis of Amino Acids
- 1Department of Marine Sciences and Convergent Technology, Hanyang University, Ansan, South Korea
- 2Division of Polar Marine Environment, Korea Polar Research Institute, Incheon, South Korea
- 3Biodiversity Research Institute, Marine Act Co., Seoul, South Korea
- 4School of Biological Sciences, Seoul National University, Seoul, South Korea
In polar ecology, zooplankton diets and survival rates vary according to the seasonality of solar radiation and oceanographic conditions. Each zooplankton species has evolved feeding strategies to survive in the diet-limited conditions of the “polar night.” Many zooplankton studies have reported seasonal adaptations in feeding activity during polar night based on their trophic niches. Nitrogen isotope analysis of amino acids has provided improved accuracy in estimates of trophic position (TP) in various marine species. In this study, field work was conducted in Kongsfjorden before (October 2017) and after polar night (April 2018). As representative zooplankton, an amphipod (Themisto abyssorum), euphausids (Meganycitiphanes norvegica and Thysanoessa sp.), a chaetognath (Parasagitta elegans), and copepods (Calanus spp. and Oithona similis) were collected. trophic position values of each taxon were estimated using the nitrogen isotope ratio of glutamic acid (δ15NGlu) and phenylalanine (δ15NPhe). Results showed that TP values of P. elegans were relatively constant, averaging 3.2 in both seasons, likely due to continuous feeding activity during polar night. Trophic position values were also constant for Calanus spp., ranging 2.5–3.0 in both seasons, due to their ability to utilize stored high-energy wax. In contrast, average TP values for O. similis, an omnivorous zooplankton, were 2.9 in October and 2.3 the following April. Trophic position values for O. similis before polar night can be attributed to the relatively high availability of algae during longer periods of daylight. We found that TP variation in zooplankton before and after polar night differed according to feeding activities in diet-restricted circumstances.
Introduction
The Arctic Ocean is an extreme environment, with seasonal cycles of solar radiation. This seasonality creates an extended period of darkness known as the polar night, which typically begins in late October and lasts until March. During polar night, primary production is low due to insufficient irradiation, and the diet available to consumers is limited. In the polar marine ecosystem, the relationship between diet and survival among zooplankton in diet-limited conditions has been the subject of continuous study (Søreide et al., 2008; Varpe, 2012; Kraft et al., 2013). Zooplankton species have evolved over-wintering strategies that vary by their trophic niche or metabolism. Herbivorous zooplankton, such as some calanoid copepods, reportedly survive periods of food shortages by consuming accumulated storage lipids (Conover, 1988). Chaetognaths, which are carnivorous zooplankton, usually feed on calanoid copepods, surviving on a reduced diet during polar night (Grigor et al., 2014). For omnivorous zooplankton, seasonal feeding strategies can lead to changes in dietary composition, which in turn can alter a species’ trophic position (TP).
Feeding strategies of zooplankton in polar and subpolar regions have been reported using feeding experiments (Atkinson, 1996), gut-content analysis (Kruse et al., 2010), and stable isotope analysis of bulk tissue (Søreide et al., 2008; Grigor et al., 2015). Recently, compound-specific isotope analysis of amino acids (CSIA-AAs) has been used to estimate the TP of consumers (Bowes and Thorp, 2015; McMahon and McCarthy, 2016). Nitrogen isotope (δ15N) analysis of individual amino acids (AAs) can provide useful information on the trophic structure of the food web by separating the trophic elevation and isotopic baseline of ecosystems. The trophic elevation can be explained as trophic AAs, which show large δ15N enrichment (3–8‰), and the isotopic baseline is represented by source AAs that exhibit smaller δ15N enrichment (by 0–1‰). The TP can be estimated by the δ15N values of glutamic acid and phenylalanine as representative of trophic and source AAs, respectively (Chikaraishi et al., 2009). Because CSIA-AAs is independent of the baseline of the δ15N ratio of the food web and is based on internal tissue measurements of the consumer itself, no basal food source sampling is required to estimate TP (Bowes and Thorp, 2015). In addition, a TP determined by CSIA-AAs could be accurately estimated even with variation of nitrogen sources at the base of the food web (Hannides et al., 2009).
The TP can supply important information about food webs. Temporal or spatial differentiation of the TP of consumers usually reflects changes in dietary utilization (Vander Zanden et al., 1999; Pauly and Watson, 2005; Branch et al., 2010). Because the polar night results in a food-limited environment, most zooplankton can be expected to either reduce the amount of food they consume (Grigor et al., 2014) or rest until better conditions return. On the other hand, opportunistic zooplankton can easily switch from algae to protists (Iversen and Seuthe, 2011). Their TP would therefore change seasonally (Seuthe et al., 2011).
Kongsfjorden, which is a fjord on the island of Svalbard, is one of the most studied coastal areas in the entire Arctic (Hop et al., 2002; Svendsen et al., 2002). A large number of zooplankton studies have been conducted through the international scientific institutes and laboratories in Ny-Ålesund for several decades (Bämstedt, 1976; Scott et al., 2000; Berge et al., 2015a). In this study, we hypothesized that the TPs of zooplankton species can serve as an indicator of feeding activity before and after polar night. Temporal and spatial variation of representative zooplankton species abundance and their relative contributions in the inner and outer regions of Kongsfjorden and their TP values were precisely compared through CSIA-AAs before and after polar night.
Materials and Methods
Study Site
Kongsfjorden lies on western Spitsbergen, Svalbard, in the European Arctic. Different environmental conditions in Kongsfjorden accompany seasonal and spatial changes in the zooplankton community (Walkusz et al., 2009) because the oceanographic conditions in Kongsfjorden are strongly influenced by sea-ice dynamics and currents flowing around the Svalbard Archipelago (Svendsen et al., 2002). Warm and saline Atlantic water, relatively cold Arctic water, and freshwater run-off from the glaciers make seasonally variable contributions to marine conditions in Kongsfjorden. According to Walkusz et al. (2009), the inflow of Atlantic water to Kongsfjorden is inhibited and a distinct frontal zone is dominated by winter-cooled water that forms in the spring. In contrast, most of the fjord is filled with Atlantic water inflow during the summer and autumn. Because each water mass contains indigenous zooplankton communities, Kongsfjorden experiences large seasonal and spatial variability in the zooplankton community (Varpe, 2012).
Sample Collection and Processing
Zooplankton samples were collected in Kongsfjorden (Figure 1) during the autumn before polar night (October 18 and 19, 2017) and the spring after polar night (April, 12–14 2018). Nine stations, except for St. 03 and St. 06, were selected from the inner to the outer fjord. Locations and depths of sampling sites are provided in Table 1. Vertical profiles of temperature and salinity were measured with conductivity, temperature, and depth (CTD) profiler. We used an SD204 CTD (SAIV A/S, rented from Marine Laboratory in Ny-Ålesund) in October and Concerto 3 loggers (RBR) in April. Zooplankton samples were collected at each station with a plankton net (mesh size of 200 μm, diameter in 50 cm, and 2.5 m in length) at a vertical velocity of 60 m/min. Detailed depths for zooplankton netting are provided in Table 1. In the harbor, additional zooplankton sampling was conducted by hand using a plankton net with the same dimensions. After transportation to the laboratory, zooplankton samples were sorted by microscope (LEICA DFC495), and dominant species were identified. Their abundances were counted and biomasses weighed. The calanoid copepod Calanus was defined as Calanus spp., which includes Calanus finmarchicus, Calanus glacialis, and copepodites of those two species (stage CIV–CV). The chaetognath Parasagitta elegans was separated into those with body lengths >20 mm and ≤20 mm according to a size-cohort grouping method described by Grigor et al. (2014). The amphipod Themisto abyssorum and euphausid Meganycitiphanes norvegica were sorted in October. The euphausid Thysanoessa sp. was sorted in April. After subsampling of the zooplankton, samples were freeze-dried for 24 h, homogenized, and stored at −20°C before further processing and analysis.
Oithona similis samples were sorted after chemical preservation because the species’ body size was too small (<1 mm) to isolate sufficient numbers of individuals in the available time. We preserved O. similis using formalin and Dess-Martin solultion in October and April, respectively. Preserved O. similis samples were soaked for 24 h in distilled water, and oven-dried at 60°C for 24 h.
Preservation Effect on Samples
Formalin and ethanol fixation on samples reportedly does not affect δ15N values of AAs in samples of fish and zooplankton (Bämstedt, 1976; Hannides et al., 2009; Ogawa et al., 2013; Berge et al., 2015a). As an alternative preservative for formalin or ethanol, Dess-Martin preservation was used for morphological and polymerase chain reaction analysis (Yoder et al., 2006). However, we could find no reports on the isotopic effects of AAs under Dess-Martin preservation. To identify isotopic effects on samples preserved in formalin and Dess-Martin solution, we compared the δ15N of AAs in frozen (control) and preserved samples using lab-cultured shrimp (Paratya spp.). The Dess-Martin solution was prepared by mixing 20% dimethyl sulfoxide (DMSO) and 0.25 M disodium EDTA saturated with NaCl at pH 8.0 as reported by Seutin et al. (1991). Control samples were frozen at −20°C in a refrigerator and freeze-dried for 24 h. We preserved each Paratya spp. specimen in formalin, 70% ethanol, and Dess-Martin solutions for periods of 1 week and 1 month. All preserved samples were soaked for 24 h in distilled water and dried at 60°C in an oven for 24 h.
Amino Acid Nitrogen Isotope Analysis
For nitrogen isotope analysis of AAs, we collected 5 or 6 individuals of Calanus spp., 2 or 3 Parasagitta elegans individuals, a single individual of the euphausid, 2 or 3 amphipod individuals, and approximately 200 O. similis individuals. Nitrogen isotopes of AAs for all zooplankton samples were analyzed without any replication at each sampling site because of the need for collecting a large number of individuals. However, in this study, a total of 2 (O. similis) or 3 (Calanus spp. and P. elegans) replicates of representative zooplankton species were compared to discuss their trophic dynamics before and after polar night. Samples were hydrolyzed for 20 h with 12 M HCl at 110°C. After removing the hydrophobic contents with 3:2 n-hexane/dichloromethane (v/v), the residual HCl was dried with an N2 purge at 70°C. In the case of the O. similis fixed in Dess-Martin solution, an additional purification procedure was needed due to the interference of residual ions dissolved in the solution. We therefore used cation-exchange chromatography for those samples before derivatization, following a method described by Takano et al. (2010). All samples were derivatized with 1:4 thionyl chloride/2-propanol (v/v) and 1:4 pivaloyl chloride/dichloromethane (v/v) in sequence. Amino acid derivatives were extracted with 3:2 n-hexane/dichloromethane (v/v) and stored at −20°C in a refrigerator before analysis. The nitrogen isotope composition of each AA was analyzed by gas chromatography (HP 6890N, Agilent) connected to a combustion furnace (GC5 interface, Elementar) and isotope ratio mass spectrometry (Isoprime, Elementar). A derivative of a standard mixture of 11 AAs obtained from SHOKO-Science and Indiana University (alanine, glycine, valine, leucine, norleucine, proline, aspartic acid, methionine, glutamic acid, phenylalanine, and hydroxyproline) was used to confirm δ15N measurements. During the calibration procedure, δ15N values of the AA standards displayed fine standard deviation of less than ±1‰. Trophic positions were estimated using an equation proposed by Chikaraishi et al. (2009):
where δ15NGlu and δ15NPhe are the measured nitrogen isotope values of glutamic acid and phenylalanine of consumers, respectively. The value 3.4 indicates the isotopic difference between the glutamic acid and phenylalanine in primary producers (β value) and 7.6 is the trophic discrimination factor (TDF) for δ15N enrichment of glutamic acid relative to phenylalanine with each trophic transfer.
Statistical Analysis
Hierarchical clustering using Bray–Curtis similarity (Bray and Curtis, 1957) was applied to zooplankton abundance and relative contributions to determine similarity among sampling stations. Dominance (Simpson, 1949), diversity (Shannon and Weaver, 1949), evenness (Buzas and Gibson, 1969), and richness (Margalef, 1958) were calculated using the zooplankton abundance values. Species diversity values were analyzed by permutation tests to compare spatial differences among sampling stations. All statistical and ecological analysis related to zooplankton abundance and relative contributions were conducted by paleontological statistics (PAST) software (Hammer et al., 2001). We used independent t-tests for abundance and ecological parameters between sampling periods. Independent t-tests were also used for δ15NAAs and TP values between sampling periods and species body sizes. All independent t-tests were performed using IBM SPSS software v23.0.
Results
Hydrography
Vertical profiles of temperature and salinity are displayed in Figure 2. In October 2017, a pycnocline was generated by freshwater run-off from glaciers and/or land, with low salinity of surface water (<33.5 psu) across the stations. By April, most of the water column was homogenized, with low water temperatures and a weak pycnocline. St. 01 and St. 02 showed slightly lower temperatures and less salinity than other stations, suggesting that strong winds and cold freshwater influenced the stations adjacent to land.
Zooplankton Community
Integrated abundance and relative contribution of the zooplankton community are shown in Figure 3. Zooplankton abundance and ecological parameters are provided in Table 1 and Supplementary Table S1. Copepods were the most dominant taxon, representing more than 95% of the zooplankton community. Total abundance of zooplankton in October was greater than 10 times that of the following April. In the copepod group, the most abundant species was O. similis, which was also the most dominant cyclopoid, accounting for 85.21 ± 6.78% of copepods on average in October and 43.54 ± 7.51% in April. In the calanoid group, members of the genus Pseudocalanus were the most abundant. The Calanus copepodite was the most abundant within Calanus, but Calanus species represented less than 5% all of zooplankton. A Bray–Curtis similarity test of zooplankton composition showed a clear division between October and April (Supplementary Figure S1). Analysis of spatial distributions showed that zooplankton composition in October was less clearly separated between the inner (St. 01–05) and outer fjord (St. 07–10). In contrast, a significant separation was evident in zooplankton composition between the inner and middle-to-outer part of the fjord in April. Calanus species showed higher average abundance in the inner fjord (34.55 ± 8.19 individuals/m3 in October and 47.14 ± 24.37 individuals/m3 for April) compared with the outer fjord (14.31 ± 4.55 individuals/m3 in October and 11.27 ± 4.21 individuals/m3 for April). However, the relative contribution of the genus Calanus was lower in the inner fjord (1.37 ± 0.40% for October and 5.33 ± 0.42 for April) than in the outer fjord (2.34 ± 1.14% for October and 14.44 ± 5.04% for April). In both sampling periods, the number of taxon was similar. However, species diversity parameters showed significant differences in both October and April (Table 2). Average species diversity, evenness, and richness in April were higher than those in October. Species dominance in April was lower than that in October. More details of the spatial variation of species diversity as revealed by a permutation test was shown in Supplementary Table S2. In October, most sampling stations exhibited significantly different species diversity. In contrast, species diversity at each station in April was nearly homogeneous, except for St. 08.
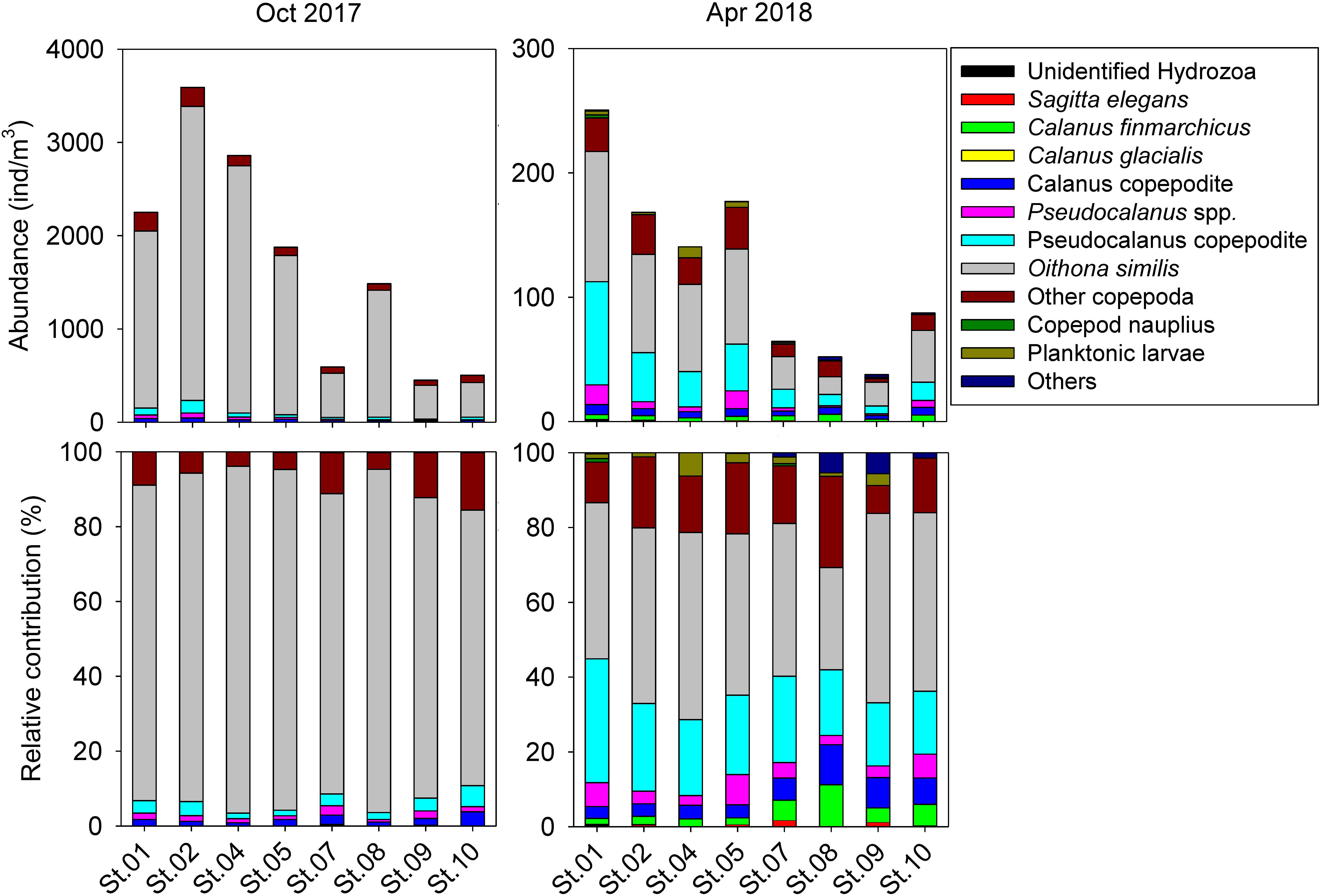
Figure 3. Abundance and relative contribution of zooplankton of Kongsfjorden in October 2017 and April 2018.
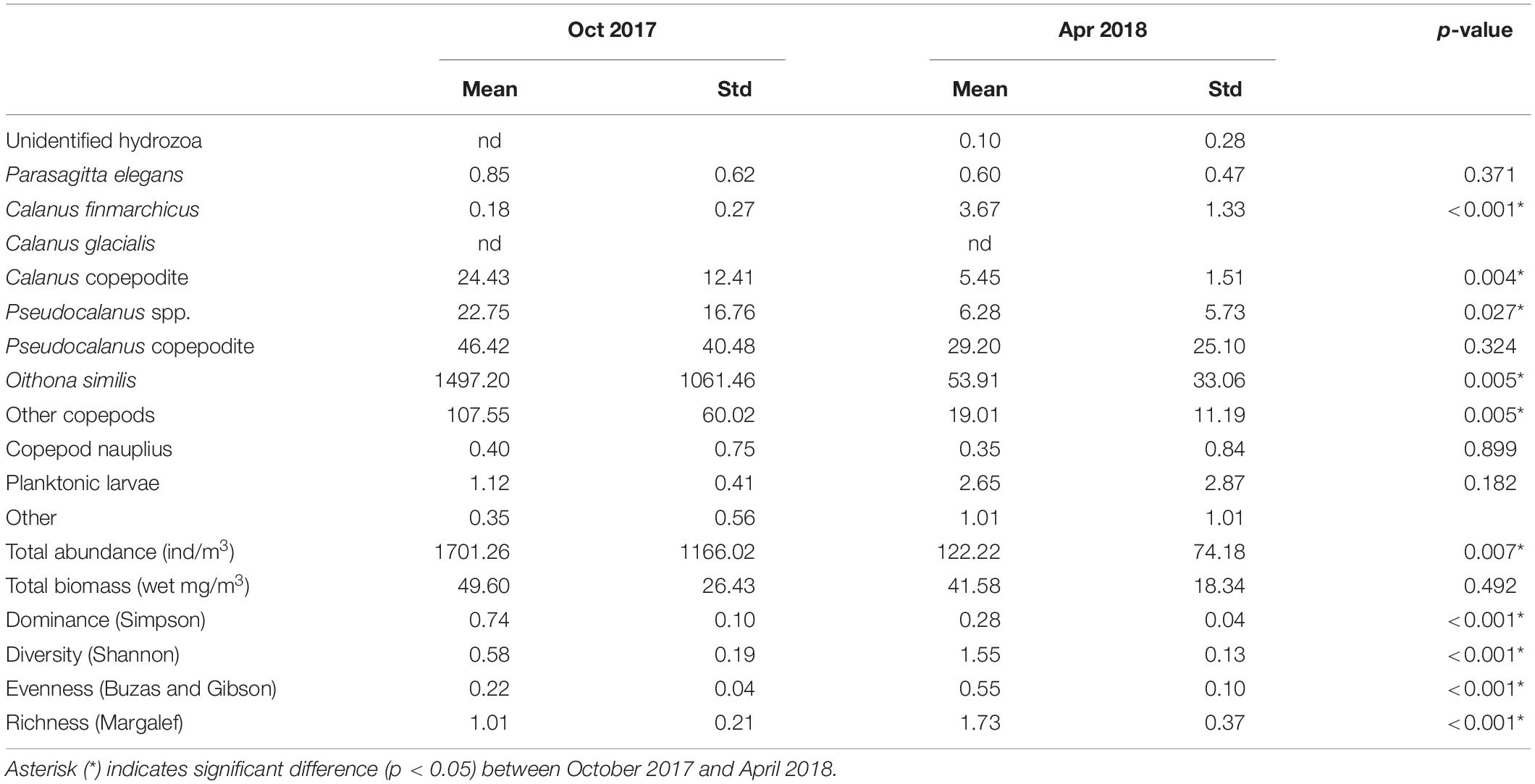
Table 2. Mean abundance, biomass, and ecological parameters for zooplankton collected in October 2017 and April 2018.
Preservation Effect of Dess-Martin
The δ15NAAs values of Paratya spp. are displayed in Supplementary Tables S3, S4. As reported by a previous study, formalin fixation did not appear to influence δ15NAAs values, showing less than a ±1‰ difference from the control (Bämstedt, 1976; Ogawa et al., 2013; Berge et al., 2015a). In our results, a Dess-Martin solution also showed less than a ±1‰ difference in δ15NAAs from the control, confirming that Dess-Martin preservation had little isotopic effect on biota samples.
AA δ15N Values and Trophic Position
δ15NAAs values and the TP of zooplankton are shown in Table 2. No significant spatial trend was observed in δ15NPhe values of zooplankton. For O. similis, a significant decrease was seen in δ15NPhe values. This suggests the δ15N isotopic baseline of O. similis changed during polar night, as a result of changing diets. In Calanus species, a slight decrease in δ15NPhe values (1.2‰ on average) between the two seasons was apparent. For P. elegans, no significant difference in δ15N between size cohorts in glutamic acid (t0.05,4, p = 0.68) and phenylalanine (t0.05,4, p = 0.42) in δ15NAAs trend was evident, nor between the two seasons (δ15NGlu t0.05,10, p = 0.15; δ15NPhe t0.05,10, p = 0.34).
Among all zooplankton, T. abyssorum exhibited the highest TP in our dataset. Trophic position values of three zooplankton collected in both sampling periods are displayed in Figure 4. P. elegans had a consistent TP temporally (t0.05,10, p = 0.87) regardless of body size (t0.05,10, p = 0.71). Calanus species showed an average TP increase of 0.2 from October to April but the difference was not statistically significant (t0.05,4, p = 0.14). O. similis had the largest variation in TP, with a decrease of 0.5 from October to April.
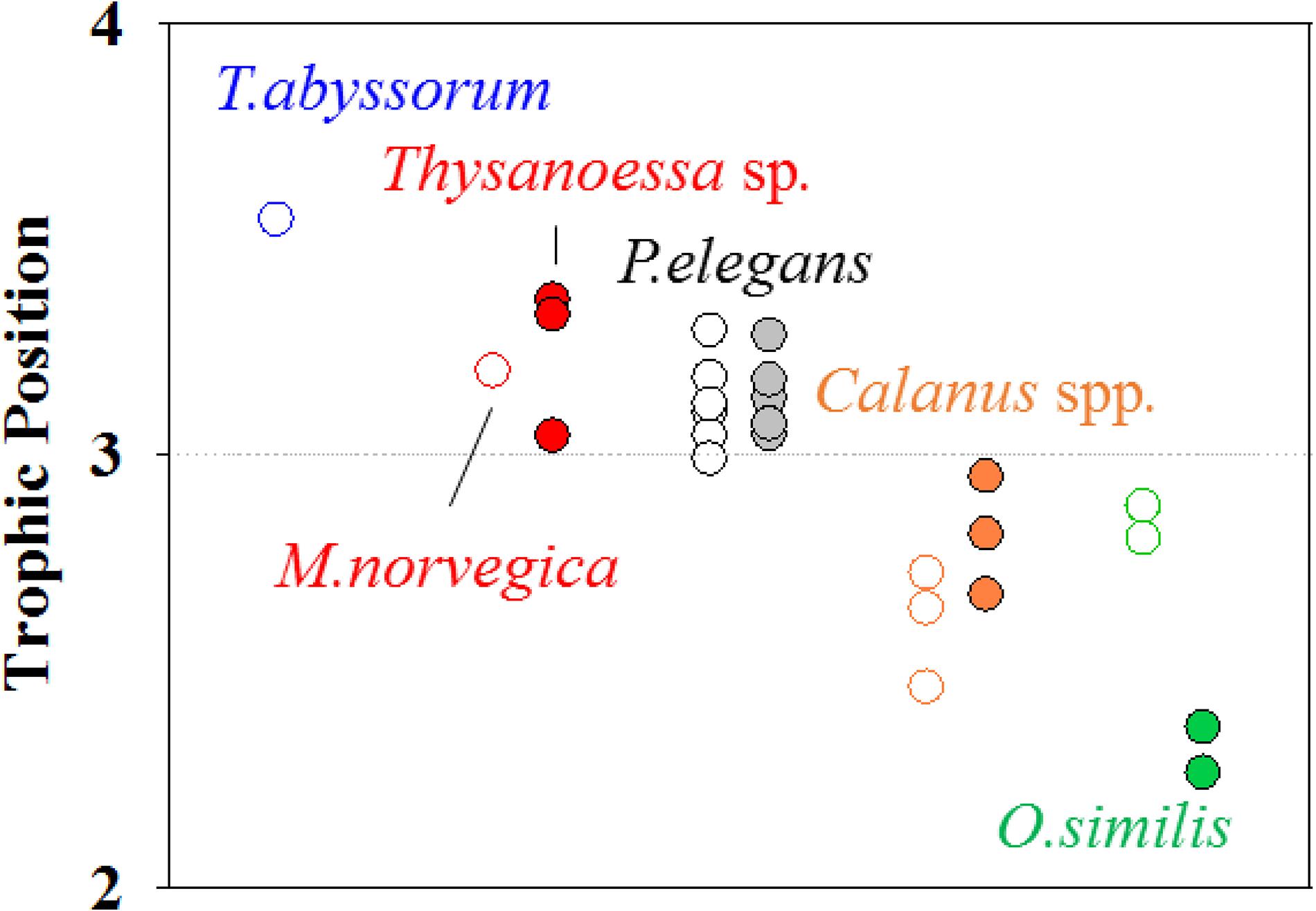
Figure 4. The difference of the TP values of zooplankton species before (open circle) and after polar night (closed circle).
Discussion
Arctic zooplankton have evolved a variety of survival strategies, most of which are related to energy supply during polar night (Hagen and Auel, 2001; Berge et al., 2015b). Energy can be managed through storage (as well as diapause), and flexible feeding behavior (Hagen, 1999). The three zooplankton collected in this study, O. similis, P. elegans, and Calanus spp., exhibit species-specific over-wintering strategies. Such strategies allow researchers to track changing diet sources, and precise TP values can be applied to identify changes in feeding activity. Trophic dynamics in zooplankton species may indicate changes in integrated diets before and after polar night. Cases of temporal TP variation before and after polar night are displayed in Figure 5 and inform the following discussion.
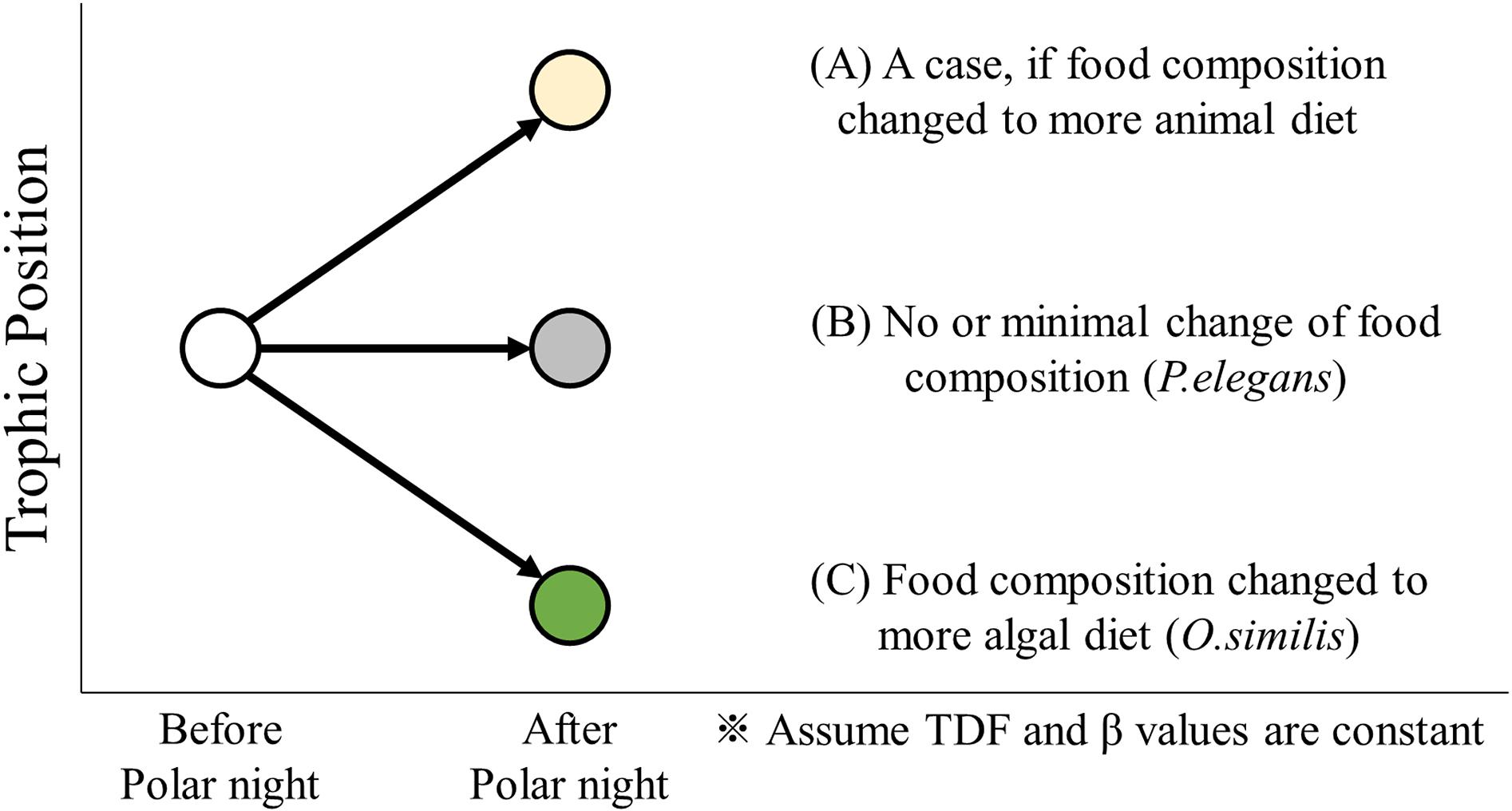
Figure 5. A conceptual illustration of TP variation before and after polar night. The trophic position in zooplankton species can increase or decrease according to the change of dominant diet composition to more animal (A) or more algal diet (C), respectively. But for some carnivorous zooplankton (e.g. P. elegans), it indicates almost constant trophic position (B) before and after polar night.”
Hydrography between two sampling periods showed different patterns of water masses. In October, bottom water, probably indicating the intrusion of the North Atlantic Ocean, displayed relatively high salinity and temperature compared with the surface water, which has a low salinity and temperature. In contrast, in April, the entire water column showed a homogeneous temperature and salinity. Our result showed those hydrographic properties were not clearly related to TP values of zooplankton species but were instead closely influenced to zooplankton community composition. Temporal variation of the zooplankton community in Kongsfjorden, which was caused by seasonal hydrography, was similar to results found in previous studies (Willis et al., 2006; Walkusz et al., 2009). In April, the total zooplankton abundance was much smaller than it was in the preceding October. As the April sampling period took place early in the month, which is considered the pre-bloom season, zooplankton was not yet abundant. With the benefit of two sampling periods, zooplankton communities were clearly separated. In both sampling periods, zooplankton abundance showed a continuous gradient from the inner to outer fjord. The inner fjord usually has the highest abundance of zooplankton, as reported by Walkusz et al. (2009).
In both sampling periods, O. similis was the dominant zooplankton across all sampling stations, and our findings were similar to those of previous studies (Hop et al., 2002; Walkusz et al., 2009). Group Oithona was described as a cosmopolitan copepod group, suggesting a wide range of habitat throughout tropical to polar regions (Fransz and Gonzalez, 1995; Castellani et al., 2005). Thus, their population would be mainly dependent on seasonal reproduction rates, rather than hydrography. In October in particular, the relative contribution of O. similis was greater than 80% at all stations. A comparably high abundance in the late autumn was previously reported in Kongsfjorden, as a result of reproduction from May to September (Lischka and Hagen, 2005). The contribution to the zooplankton community from O. similis was weaker in April, with increasing abundances of other copepod groups as a result of spring reproduction (Berge et al., 2015b). Although both sampling periods showed different spatial patterns of species diversity, which are estimated by permutation tests for the zooplankton community (Supplementary Table S2), spatial variation of TP values in zooplankton species (Table 3) were not related to species diversity.
Continuous energy supply based on flexible feeding behaviors may enable year-round reproduction of O. similis (Berge et al., 2015b). Cultivation experiments with O. similis have revealed a high clearance rate for ciliates, dinoflagellates (Atkinson, 1996; Zamora-Terol et al., 2013), and diatoms (Atienza et al., 2006; Pond and Ward, 2010). This flexible feeding by O. similis is likely connected to seasonally significant TP variation. In this study, as the TP value of O. similis decreased, their dominant diet appeared to shift after polar night from consumers to primary producers, with a case (C) in Figure 5. As a result, O. similis appeared to be connected with protozooplankton, making the dietary composition primarily carnivorous in October and herbivorous in April. Iversen and Seuthe (2011) reported the integrated biomass of protozooplankton and phytoplankton were similar in pre-bloom seasons and before polar night in Kongsfjorden. When both diet sources are available to O. similis, it is likely that phytoplankton would be less preferred due to their low nutritional content compared with protozooplankton (Stoecker and Capuzzo, 1990; Atkinson, 1996). We found that the low solar elevation and short daylengths in October resulted in a diet dominated by protozooplankton. In contrast, when solar elevations were higher and days were longer in April, more phytoplankton was available.
Omnivorous zooplankton has different TP variations according to its habitat. For example, the copepod Pleuromamma xiphias is an opportunistic omnivore from the Sargasso Sea with a seasonal shift between carnivorous and herbivorous diets (Schnetzer and Steinberg, 2002). But in the north of Hawaii, P.xiphias showed similar TP values between summer and winter, as estimated by the CSIA-AAs method [2.8 ± 0.08, Hannides et al. (2009)]. In addition, Hannides et al. (2009) reported that Oithona spp. in the north of Hawaii showed TP close to 2.0, with small seasonal variation. Their study suggested the diet of Oithona spp. would not consist solely of primary producers, in spite of a TP of 2.0, due to small 15N discrimination in the rapid and tightly coupled microbial food web. Although our study produced results in opposition to those of Hannides et al. (2009), we were unable to directly compare TP values for Oithona between low and high latitudes. Because the environmental conditions differed between the two locations, the TDF could have changed, even by species, due to diet quality (Chikaraishi et al., 2015; McMahon et al., 2015). Opportunistic feeding activities of Oithona in various environments would therefore produce different TP values based on available diets and the magnitude of 15N isotopic fractionation. Additional study would be required to determine the ecological relationship between Oithona and protozooplankton, and ciliates and dinoflagellates in particular.
Parasagitta elegans is a major carnivorous zooplankton in the Arctic, and its diet reportedly consists primarily of copepods (Samemoto, 1987; Terazaki, 2004). In this study, abundance of most copepods decreased with the onset of polar night, and the amount of available P. elegans consumption was therefore also lower. However, we found the abundance of P. elegans was constant between the two sampling periods, although temporal and spatial TP variations for the species were unclear. P. elegans requires additional lipids to achieve sexual maturity during spring algal blooms (Choe et al., 2003). Although growth rates and the magnitude of feeding activity of P. elegans decreased during winter compared with spring and summer, feeding activity continued (Grigor et al., 2014). Trophic position values of 2.9–3.0 for P. elegans, as estimated by bulk nitrogen isotope in January Grigor et al. (2015), were similar to our results. The constant TP before and after polar night can be explained by continuous feeding activity on copepods, as a case (B) in Figure 5. This also implies that the TP of copepods, which are available for consumption by P. elegans, would be constant before, during, and after polar night.
As a result of the TP estimates by CSIA-AAs, T. abyssorum was identified as a carnivore in this study. T.abyssorum feeds primarily on Calanus (Kraft et al., 2013). But T. abyssorum was collected in the harbor only in October, making temporal comparisons of TP values impossible. The euphausids M. norvegica and Thysanoessa sp. were collected during different sampling periods, and their TP values ranged from 3.0 to 3.4. The genus Thysanoessa has been reported as a herbivore or omnivore (Wold et al., 2011; Huenerlage et al., 2016). Similarly, M. norvegica is an omnivore, showing seasonally different diet preferences for diatoms and copepods in spring/summer and fall/winter, respectively (Schmidt, 2010). Trophic position values of euphausids in this study suggest their diet was composed primarily of other zooplankton during our sampling periods.
In this study, copepodite was dominant in October and adult C. finmarchicus increased in April, showing similar results to a previous study (Willis et al., 2006). Continuous intrusion of North Atlantic waters into Kongsfjorden would result in a high contribution of C. finmarchicus to the group Calanus in October. In April, when winter-cooled water was dominant, the population of Calanus decreased and most individuals were resting in the deep layer (Conover, 1988). Previous studies have reported that the Calanus species store high-energy wax esters and utilize them during polar night (Scott et al., 2000). The wax material enhances survival over polar night, assuming minimal metabolic demands (Falk-Petersen et al., 2007). In our results, the TP values of Calanus spp. ranged from 2.5 to 3.0, suggesting a high contribution from primary consumers, and is in agreement with a reported gut-content analysis of C. glacialis (Cleary et al., 2017). Calanus spp. filter-feed mainly on phytoplankton, sea ice algae, detritus, protists, and small zooplankton (Frost, 1972; Levinsen et al., 2000; Falk-Petersen et al., 2007; Cleary et al., 2017). Their diets are therefore closely coupled with the composition of organic particles (both living and non-living) in the water mass, and all available diet components would be integrated and assimilated. According to previous studies, their TP values, as estimated by bulk isotope analysis, varied seasonally, ranging from the herbivorous to omnivorous position, and likely depend on diet available in the water column (Søreide et al., 2006, 2008). However, Calanus spp. appear to be omnivorous and carnivorous organisms, feeding on more heterotrophic species during the two non-productive seasons in Kongsfjorden, based on the TP values of 2.5 to 3.0 found in this study.
Compared with TP variation in O. similis, the average TP values of Calanus spp. showed little increase (0.2) after polar night, indicating no statistically significant difference. It is possible that different isotope turnover rates can be applied to Calanus spp. and O similis. Both copepods showed high clearances on similar size classes (from 20 to 40 μm) of dinoflagellates and ciliates, suggesting available diet sizes for both copepods were not substantially different (Levinsen et al., 2000; Zamora-Terol et al., 2013). Instead, the slow growth rate of Calanus spp. may cause slow isotope turnover (Tamelander et al., 2006). The TP value of O. similis could shift earlier to a phytoplankton diet compared to the TP of Calanus spp. during the cold-water conditions of the early spring.
Conclusion
The TPs of representative zooplankton species were measured to determine their feeding activity before and after polar night. We found that temporal TP variation of zooplankton reflected species-specific feeding strategies. In this study, nitrogen isotope analysis of AAs proved to be a useful approach to understanding changes in TPs of zooplankton species before and after polar night. For further study, additional application of CSIA-AAs methods, including carbon isotope analysis of AAs, would help clarify the feeding strategy of zooplankton species under extreme environmental conditions, such as polar night.
Data Availability Statement
All datasets generated for this study are included in the article/Supplementary Material.
Author Contributions
HC, S-YH, SL, and J-HK participated in the field work. HC analyzed the nitrogen isotopes of amino acids in the samples and wrote the manuscript. SL and J-HK collected and sorted zooplankton samples for analysis. S-YH and K-HS designed the experiments and revised the manuscript. All authors contributed to the article and approved the submitted version.
Funding
This research was a part of a project titled “Carbon assimilation rate and organic carbon cycle in sea ice-algal ecosystem (PE19170),” which was funded by the Korea Polar Research Institute.
Conflict of Interest
The authors declare that the research was conducted in the absence of any commercial or financial relationships that could be construed as a potential conflict of interest.
Acknowledgments
We appreciate the contributions of members of the field team in sample collection. The captain of the research vessel (MS Teisten) carefully supported and scheduled our field work. We also thank the Marine Laboratory in Ny-Ålesund, which provided space and equipment for our research.
Supplementary Material
The Supplementary Material for this article can be found online at: https://www.frontiersin.org/articles/10.3389/fmars.2020.00489/full#supplementary-material
References
Atienza, D., Calbet, A., Saiz, E., Alcaraz, M., and Trepat, I. (2006). Trophic impact, metabolism, and biogeochemical role of the marine cladoceran Penilia avirostris and the co-dominant copepod Oithona nana in NW Mediterranean coastal waters. Mar. Biol. 150, 221–235. doi: 10.1007/s00227-006-0351-z
Atkinson, A. (1996). Subantarctic copepods in an oceanic, low chlorophyll environment: ciliate predation, food selectivity and impact on prey populations. Mar. Ecol. Progr. Ser. 130, 85–96. doi: 10.3354/meps130085
Bämstedt, U. (1976). Studies on the deep-water pelagic community of Korsfjqrden, Western Norway Changes in the size and biochemical composition of Meganycitiphanes norvegica (Euphausiacea) in relation to its life cycle. Sarsia 61, 15–30. doi: 10.1080/00364827.1976.10411298
Berge, J., Daase, M., Renaud, P. E., Ambrose, W. G. Jr., Darnis, G., Last, K. S., et al. (2015a). Unexpected levels of biological activity during the polar night offer new perspectives on a warming Arctic. Curr. Biol. 25, 2555–2561. doi: 10.1016/j.cub.2015.08.024
Berge, J., Renaud, P. E., Darnis, G., Cottier, F., Last, K., Gabrielsen, T. M., et al. (2015b). In the dark: a review of ecosystem processes during the Arctic polar night. Progr. Oceanogr. 139, 258–271. doi: 10.1016/j.pocean.2015.08.005
Bowes, R. E., and Thorp, J. H. (2015). Consequences of employing amino acid vs. bulk-tissue, stable isotope analysis: a laboratory trophic position experiment. Ecosphere 6, 1–12.
Branch, T. A., Watson, R., Fulton, E. A., Jennings, S., McGilliard, C. R., Pablico, G. T., et al. (2010). The trophic fingerprint of marine fisheries. Nature 468, 431–435. doi: 10.1038/nature09528
Bray, J., and Curtis, J. (1957). An Ordination Of Upland Forest Communities Of Southern Wisconsin. Washington, DC: Ecological Monographs, 325–349.
Buzas, M. A., and Gibson, T. G. (1969). Species diversity: benthonic foraminifera in western North Atlantic. Science 163, 72–75. doi: 10.1126/science.163.3862.72
Castellani, C., Irigoien, X., Harris, R. P., and Lampitt, R. S. (2005). Feeding and egg production of Oithona similis in the North Atlantic. Mar. Ecol. Progr. Ser. 288, 173–182. doi: 10.3354/meps288173
Chikaraishi, Y., Ogawa, N. O., Kashiyama, Y., Takano, Y., Suga, H., Tomitani, A., et al. (2009). Determination of aquatic food-web structure based on compound-specific nitrogen isotopic composition of amino acids. Limnol. Oceanogr. 7, 740–750. doi: 10.4319/lom.2009.7.740
Chikaraishi, Y., Steffan, S. A., Takano, Y., and Ohkouchi, N. (2015). Diet quality influences isotopic discrimination among amino acids in an aquatic vertebrate. Ecol. Evol. 5, 2048–2059. doi: 10.1002/ece3.1491
Choe, N., Deibel, D., Thompson, R. J., Lee, S. H., and Bushell, V. K. (2003). Seasonal variation in the biochemical composition of the chaetognath Parasagitta elegans from the hyperbenthic zone of Conception Bay, Newfoundland. Mar. Ecol. Progr. Ser. 251, 191–200. doi: 10.3354/meps251191
Cleary, A. C., Søreide, J. E., Freese, D., Niehoff, B., and Gabrielsen, T. M. (2017). Feeding by Calanus glacialis in a high arctic fjord: potential seasonal importance of alternative prey. ICES J. Mar. Sci. 74, 1937–1946. doi: 10.1093/icesjms/fsx106
Conover, R. (1988). Comparative life histories in the genera Calanus and Neocalanus in high latitudes of the northern hemisphere. Hydrobiologia 167, 127–142. doi: 10.1007/978-94-009-3103-9_11
Falk-Petersen, S., Pavlov, V., Timofeev, S., and Sargent, J. R. (2007). “Climate variability and possible effects on arctic food chains: the role of Calanus,” in Arctic Alpine Ecosystems and People in a Changing Environment, eds R. Kallenborn, I. Tombre, J. B. Ørbaek, S. Falk-Petersen, E. N. Hegseth, and A. H. Hoel (Berlin: Springer), 147–166. doi: 10.1007/978-3-540-48514-8_9
Fransz, H., and Gonzalez, S. (1995). The production of Oithona similis (Copepoda: Cyclopoida) in the Southern Ocean. ICES J. Mar. Sci. 52, 549–555. doi: 10.1016/1054-3139(95)80069-7
Frost, B. (1972). Effects of size and concentration of food particles on the feeding behavior of the marine planktonic copepod Calanus pacificus 1. Limnol. Oceanogr. 17, 805–815. doi: 10.4319/lo.1972.17.6.0805
Grigor, J. J., Marais, A. E., Falk-Petersen, S., and Varpe, Ø (2015). Polar night ecology of a pelagic predator, the chaetognath Parasagitta elegans. Polar Biol. 38, 87–98. doi: 10.1007/s00300-014-1577-8
Grigor, J. J., Søreide, J. E., and Varpe, Ø (2014). Seasonal ecology and life-history strategy of the high-latitude predatory zooplankter Parasagitta elegans. Mar. Ecol. Progr. Ser. 499, 77–88. doi: 10.3354/meps10676
Hagen, W. (1999). Reproductive strategies and energetic adaptations of polar zooplankton. Invertebr. Reproduct. Dev. 36, 25–34. doi: 10.1080/07924259.1999.9652674
Hagen, W., and Auel, H. (2001). Seasonal adaptations and the role of lipids in oceanic zooplankton. Zoology 104, 313–326. doi: 10.1078/0944-2006-00037
Hammer, Ø, Harper, D. A., and Ryan, P. D. (2001). PAST: paleontological statistics software package for education and data analysis. Palaeontol. Electr. 4:9.
Hannides, C. C., Popp, B. N., Landry, M. R., and Graham, B. S. (2009). Quantification of zooplankton trophic position in the North Pacific Subtropical Gyre using stable nitrogen isotopes. Limnol. Oceanogr. 54, 50–61. doi: 10.4319/lo.2009.54.1.0050
Hop, H., Pearson, T., Hegseth, E. N., Kovacs, K. M., Wiencke, C., Kwasniewski, S., et al. (2002). The marine ecosystem of Kongsfjorden, Svalbard. Polar Res. 21, 167–208.
Huenerlage, K., Graeve, M., and Buchholz, F. (2016). Lipid composition and trophic relationships of krill species in a high Arctic fjord. Polar Biol. 39, 1803–1817. doi: 10.1007/s00300-014-1607-6
Iversen, K. R., and Seuthe, L. (2011). Seasonal microbial processes in a high-latitude fjord (Kongsfjorden, Svalbard): I. Heterotrophic bacteria, picoplankton and nanoflagellates. Polar Biol. 34, 731–749. doi: 10.1007/s00300-010-0929-2
Kraft, A., Berge, J., Varpe, Ø, and Falk-Petersen, S. (2013). Feeding in Arctic darkness: mid-winter diet of the pelagic amphipods Themisto abyssorum and T. libellula. Mar. Biol. 160, 241–248. doi: 10.1007/s00227-012-2065-8
Kruse, S., Hagen, W., and Bathmann, U. (2010). Feeding ecology and energetics of the Antarctic chaetognaths Eukrohnia hamata, E. bathypelagica and E. bathyantarctica. Mar. Biol. 157, 2289–2302. doi: 10.1007/s00227-010-1496-3
Levinsen, H., Turner, J. T., Nielsen, T. G., and Hansen, B. W. (2000). On the trophic coupling between protists and copepods in arctic marine ecosystems. Mar. Ecol. Progr. Ser. 204, 65–77. doi: 10.3354/meps204065
Lischka, S., and Hagen, W. (2005). Life histories of the copepods Pseudocalanus minutus, P. acuspes (Calanoida) and Oithona similis (Cyclopoida) in the Arctic Kongsfjorden (Svalbard). Polar Biol. 28, 910–921. doi: 10.1007/s00300-005-0017-1
Margalef, D. (1958). Information theory in ecology, General systems. Transl, from Mem. Real Acad. Cienc. Arts. Barcelona 32, 373–449.
McMahon, K. W., and McCarthy, M. D. (2016). Embracing variability in amino acid δ15N fractionation: mechanisms, implications, and applications for trophic ecology. Ecosphere 7:e01511.
McMahon, K. W., Thorrold, S. R., Elsdon, T. S., and McCarthy, M. D. (2015). Trophic discrimination of nitrogen stable isotopes in amino acids varies with diet quality in a marine fish. Limnol. Oceanogr. 60, 1076–1087. doi: 10.1002/lno.10081
Ogawa, N. O., Chikaraishi, Y., and Ohkouchi, N. (2013). Trophic position estimates of formalin-fixed samples with nitrogen isotopic compositions of amino acids: an application to gobiid fish (Isaza) in Lake Biwa. Japan. Ecol. Res. 28, 697–702. doi: 10.1007/s11284-012-0967-z
Pauly, D., and Watson, R. (2005). Background and interpretation of the ‘Marine Trophic Index’as a measure of biodiversity. Philos. Trans. R. Soc. Lond. B 360, 415–423. doi: 10.1098/rstb.2004.1597
Pond, D. W., and Ward, P. (2010). Importance of diatoms for Oithona in Antarctic waters. J. Plankton Res. 33, 105–118. doi: 10.1093/plankt/fbq089
Samemoto, D. (1987). Vertical distribution and ecological significance of chaetognaths in the Arctic environment of Baffin Bay. Polar Biol. 7, 317–328. doi: 10.1007/bf00293222
Schmidt, K. (2010). Food and feeding in Northern krill (Meganyctiphanes norvegica Sars). Adv. Mar. Biol. 57, 127–171. doi: 10.1016/b978-0-12-381308-4.00005-4
Schnetzer, A., and Steinberg, D. (2002). Natural diets of vertically migrating zooplankton in the Sargasso Sea. Mar. Biol. 141, 89–99. doi: 10.1007/s00227-002-0815-8
Scott, C. L., Kwasniewski, S., Falk-Petersen, S., and Sargent, J. R. (2000). Lipids and life strategies of Calanus finmarchicus, Calanus glacialis and Calanus hyperboreus in late autumn, Kongsfjorden, Svalbard. Polar Biol. 23, 510–516. doi: 10.1007/s003000000114
Seuthe, L., Iversen, K. R., and Narcy, F. (2011). Microbial processes in a high-latitude fjord (Kongsfjorden, Svalbard): II. Ciliates and dinoflagellates. Polar Biol. 34, 751–766. doi: 10.1007/s00300-010-0930-9
Seutin, G., White, B. N., and Boag, P. T. (1991). Preservation of avian blood and tissue samples for DNA analyses. Can. J. Zool. 69, 82–90. doi: 10.1139/z91-013
Shannon, C. E., and Weaver, W. (1949). The Mathematical Theory of Communication. Urbana: University of Illinois, 117.
Søreide, J. E., Falk-Petersen, S., Hegseth, E. N., Hop, H., Carroll, M. L., Hobson, K. A., et al. (2008). Seasonal feeding strategies of Calanus in the high-Arctic Svalbard region. Deep Sea Res. Part II Top. Stud. Oceanogr. 55, 2225–2244. doi: 10.1016/j.dsr2.2008.05.024
Søreide, J. E., Hop, H., Carroll, M. L., Falk-Petersen, S., and Hegseth, E. N. (2006). Seasonal food web structures and sympagic–pelagic coupling in the European Arctic revealed by stable isotopes and a two-source food web model. Progr. Oceanogr. 71, 59–87. doi: 10.1016/j.pocean.2006.06.001
Stoecker, D. K., and Capuzzo, J. M. (1990). Predation on protozoa: its importance to zooplankton. J. Plankton Res. 12, 891–908. doi: 10.1093/plankt/12.5.891
Svendsen, H., Beszczynska-Møller, A., Hagen, J. O., Lefauconnier, B., Tverberg, V., Gerland, S., et al. (2002). The physical environment of Kongsfjorden–Krossfjorden, an Arctic fjord system in Svalbard. Polar Res. 21, 133–166. doi: 10.1111/j.1751-8369.2002.tb00072.x
Takano, Y., Kashiyama, Y., Ogawa, N. O., Chikaraishi, Y., and Ohkouchi, N. (2010). Isolation and desalting with cation-exchange chromatography for compound-specific nitrogen isotope analysis of amino acids: application to biogeochemical samples. Rapid Commun. Mass Spectr. 24, 2317–2323. doi: 10.1002/rcm.4651
Tamelander, T., Søreide, J. E., Hop, H., and Carroll, M. L. (2006). Fractionation of stable isotopes in the Arctic marine copepod Calanus glacialis: effects on the isotopic composition of marine particulate organic matter. J. Exp. Mar. Biol. Ecol. 333, 231–240. doi: 10.1016/j.jembe.2006.01.001
Terazaki, M. (2004). Life history strategy of the chaetognath Sagitta elegans in the World Oceans. Coastal Mar. Sci. 29, 1–12.
Vander Zanden, M. J., Casselman, J. M., and Rasmussen, J. B. (1999). Stable isotope evidence for the food web consequences of species invasions in lakes. Nature 401, 464–467. doi: 10.1038/46762
Varpe, Ø (2012). Fitness and phenology: annual routines and zooplankton adaptations to seasonal cycles. J. Plankton Res. 34, 267–276. doi: 10.1093/plankt/fbr108
Walkusz, W., Kwasniewski, S., Petersen, S. F., Hop, H., Tverberg, V., Wieczorek, P., et al. (2009). Seasonal and spatial changes in the zooplankton community of Kongsfjorden. Svalbard. Polar Res. 28, 254–281. doi: 10.1111/j.1751-8369.2009.00107.x
Willis, K., Cottier, F., Kwasniewski, S., Wold, A., and Falk-Petersen, S. (2006). The influence of advection on zooplankton community composition in an Arctic fjord (Kongsfjorden, Svalbard). J. Mar. Syst. 61, 39–54. doi: 10.1016/j.jmarsys.2005.11.013
Wold, A., Jaeger, I., Hop, H., Gabrielsen, G. W., and Falk-Petersen, S. (2011). Arctic seabird food chains explored by fatty acid composition and stable isotopes in Kongsfjorden, Svalbard. Polar Biol. 34, 1147–1155. doi: 10.1007/s00300-011-0975-4
Yoder, M., King, I. W., De Ley, I. T., Mann, J., Mundo-Ocampo, M., Poiras, L., et al. (2006). DESS: a versatile solution for preserving morphology and extractable DNA of nematodes. Nematology 8, 367–376. doi: 10.1163/156854106778493448
Keywords: trophic position, nitrogen isotope, amino acid, zooplankton, Kongsfjorden, Svalbard
Citation: Choi H, Ha S-Y, Lee S, Kim J-H and Shin K-H (2020) Trophic Dynamics of Zooplankton Before and After Polar Night in the Kongsfjorden (Svalbard): Evidence of Trophic Position Estimated by δ15N Analysis of Amino Acids. Front. Mar. Sci. 7:489. doi: 10.3389/fmars.2020.00489
Received: 28 November 2019; Accepted: 02 June 2020;
Published: 21 July 2020.
Edited by:
Alberto Basset, University of Salento, ItalyReviewed by:
Edoardo Calizza, Sapienza University of Rome, ItalyElizaveta Ershova, UiT Arctic University of Norway, Norway
Copyright © 2020 Choi, Ha, Lee, Kim and Shin. This is an open-access article distributed under the terms of the Creative Commons Attribution License (CC BY). The use, distribution or reproduction in other forums is permitted, provided the original author(s) and the copyright owner(s) are credited and that the original publication in this journal is cited, in accordance with accepted academic practice. No use, distribution or reproduction is permitted which does not comply with these terms.
*Correspondence: Kyung-Hoon Shin, shinkh@hanyang.ac.kr