Plastic Ingestion in Post-hatchling Sea Turtles: Assessing a Major Threat in Florida Near Shore Waters
- 1Whitney Laboratory for Marine Bioscience and Sea Turtle Hospital, University of Florida, St. Augustine, FL, United States
- 2Department of Biological Sciences, School of Natural Sciences, University of Limerick, Limerick, Ireland
- 3Department of Biology, Hal Marcus College of Science and Engineering, University of West Florida, Pensacola, FL, United States
- 4Guana Tolomato Matanzas National Estuarine Research Reserve, Ponte Vedra Beach, FL, United States
- 5Department of Biology, University of Florida, Gainesville, FL, United States
Pollution from anthropogenic marine debris, particularly buoyant plastics, is ubiquitous across marine ecosystems. Due to the persistent nature of plastics in the environment, their buoyancy characteristics, degradation dynamics, and ability to mimic the behavior of natural prey, there exists significant opportunity for marine organisms to ingest these man-made materials. In this study we examined gastrointestinal (GI) tracts of 42 post-hatchling loggerhead (Caretta caretta) sea turtles stranded in Northeast Florida. Necropsies revealed abundant numbers of plastic fragments ranging from 0.36 to 12.39 mm in size (length), recovered from the GI tracts of 39 of the 42 animals (92.86%), with GI burdens ranging from 0 to 287 fragments with a mass of up to 0.33 g per turtle. Post-hatchlings weighed from 16.0 to 47.59 g yielding a plastic to body weight percentage of up to 1.23%. Several types of plastic fragments were isolated, but hard fragments and sheet plastic were the most common type, while the dominant frequency of fragment color was white. Fragment size and abundance mixed with natural gut contents suggests significant negative health consequences from ingestion in animals at this life stage. Gaining greater insight into the prevalence of plastic ingestion, the types of plastic and the physiological effects of plastic consumption by multiple life-stages of sea turtles will aid the prioritization of mitigation efforts for the growing marine debris problem. This report demonstrates that plastic ingestion is a critical issue for marine turtles from the earliest stages of life.
Introduction
The accumulation of persistent anthropogenic debris in the marine environment in recent years has reached epic proportions. Plastics have become the most ubiquitous form of marine debris globally (Derraik, 2002; Cole et al., 2011), reaching a staggering 299 million tons as of 2013 (Wright et al., 2013). Plastic pollution has been identified in practically every environment (air, soil, freshwater, ice, and marine), from high mountain tops to the deepest oceanic trench (Peeken et al., 2018; Allen et al., 2019; Choy et al., 2019; Brahney et al., 2020; Kane et al., 2020; Kelly et al., 2020). It has even been shown that each human unintentionally ingests 50,000 microplastic particles per year, and also breathes in a similar level (Cox et al., 2019). Originating mostly from land-based sources such as landfills and manufacturing plants, packaging and single-use plastic items comprise 40% of all plastic production (Van Buskirk and Crowder, 1994). One area of particular concern is the long “life” plastics have in the marine environment. Plastics derived from petro-chemicals, which began mass production in the 1950s, continue to persist and accumulate in the marine environment despite weathering, fragmentation, embrittlement, ultraviolet (UV) photolysis, and biodegradation. Plastic items eventually physically disintegrate into smaller sizes, but this does not change their effective mass in the environment, potentially remaining chemically intact for centuries (Worm et al., 2017). The United Nations Joint Group of Experts on the Scientific Aspects of Marine Pollution (GESAMP) determined that land-based sources account for up to 80% of the world’s marine pollution (GESAMP, 1991). The systematic degradation process of plastic products generates distinct size classes of plastic debris (macro, meso, micro, and nano particles), each of which bring their own detrimental biological impacts (Anderson et al., 2015; Rochman et al., 2016) and can affect a wide diversity of marine fauna. Both vertebrate and invertebrate taxa living in a wide range of habitats from oceanic to interstitial are impacted by plastic waste (Wright et al., 2013; Crump et al., 2020). For some species, including sea turtles (Bjorndal et al., 1994; Duffy and Burkhalter, 2020), plastics pose a threat of entanglement, habitat degradation and ingestion. Micro-plastics occupy similar size ranges as some planktonic organisms (≤5 mm) and are consumed by pelagic and benthic marine organisms including planktivores, detritivores, and suspension feeders (Thompson et al., 2004; Li et al., 2016). Another notable concern is that buoyant plastic pieces themselves act as habitats/vectors, creating novel dispersal agents for a variety of microbial species into potentially non-native waters (Law et al., 2010). Similarly, these plastic pieces can provide refuges and places to deposit eggs for pelagic zooplankton where they previously could not, increasing their geographical range (Desforges et al., 2015). Moreover, pelagic zooplankton have been found to ingest microplastics, which could offer another path for further introduction to larger marine species, such as juvenile turtles.
The multi-faceted attributes of plastics such as their long life, chemical additives and changing physical features due to weathering, transport and dispersal contribute to the growing concern of this oceanic pollutant. Approximately 50% of the known floating plastic resides in the subtropical gyres of the North and South Atlantic, North and South Pacific and Indian Oceans (Anderson et al., 2015; Desforges et al., 2015). These currents and convergent zones not only encompass large areas of marine debris, but are also habitat for a vast diversity of biota (Carr, 1987). The North Atlantic Subtropical Gyre is a particularly complex habitat in terms of plastic accumulation due to the presence of the pelagic brown algae Sargassum-dominated drift (i.e., Sargassum natans and Sargassum fluitans) (Witherington, 2002). These floating macroalgal mats provide refugia for a variety of pelagic invertebrates and vertebrates (hydroids, copepods, insects, crabs, shrimp, polychaetes, bryozoans, fishes). The Sargassum community also provides crucial developmental habitat for oceanic-stage neonate sea turtle species. Following an initial period of frenzied swimming, these post-hatchling turtles recruit to the Sargassum from nesting beaches along the southeastern United States Atlantic coast and transit through the neritic zone heading for the oceanic zone (Wyneken and Salmon, 1992; Bolten, 2003a). These oceanic juveniles (Bolten, 2003a) or surface-pelagic juvenile life-stage sea turtles (Witherington et al., 2012) begin to feed on small zooplankton and seek refuge and food in floating Sargassum and flotsam (Bolten, 2003a; Witherington et al., 2012). Where the currents converge at Sargassum zones, marine debris poses a significant threat of ingestion to the immature turtles (Carr, 1987; Witherington et al., 2012). Due to their long complex life histories, migratory behavior and feeding ecology, multiple life stages of sea turtles are particularly vulnerable to marine debris, as they come into contact with and ingest plastic, mistaking it for prey items.
All seven sea turtle species have been shown to ingest plastic (Duncan et al., 2019). Marine debris ingestion and entanglement cause morbidity and mortality in all sea turtle species (Franzen-Klein et al., 2020). Furthermore, earlier life stages appear to be more severely affected than later stages (Campani et al., 2013; Table 1). To determine the prevalence of plastic ingestion in a population of post-hatchling sea turtles we examined the gut contents of 42 Caretta caretta post-hatchlings. These turtles are considered “washbacks” – post-hatchling transitional stage sea turtles that have emerged from the nesting beaches and have begun to feed. This transitional stage ends once the turtles reach the oceanic zone in the western Atlantic that is demarcated by the Gulf Stream (Bolten, 2003a). Early life-stages are potentially vulnerable to marine debris ingestion, but limited debris ingestion data exists for post-hatchling sea turtles. Therefore, we investigated the burden of ingested marine debris in deceased C. caretta post-hatchling washbacks. The behavior of C. caretta post-hatchlings is similar to that of other species of sea turtles (Wyneken and Salmon, 1992; Bolten, 2003b; Mellgren et al., 2003; Schuyler et al., 2012; Mansfield et al., 2014; Putman Nathan and Mansfield Katherine, 2015), therefore, the results revealed here are useful as a proxy to sea turtles in general. Loggerhead and green (Chelonia mydas) post-hatchling turtles, in particular, share similar feeding ecology (Boyle and Limpus, 2008).
Materials and Methods
Necropsies were performed on 42 post-hatchling loggerhead sea turtles that died while at the Whitney Laboratory Sea Turtle Rehabilitation Hospital1. Rehabilitation and necropsy of deceased turtles was conducted in accordance with the high standards of Florida Fish and Wildlife Conservation Commission (FWC) rehabilitation permits MTP-16-228 to MTP-17-228. The post-hatchlings were transported to the University of Florida’s Whitney Laboratory Sea Turtle Rehabilitation Hospital by the FWC during the nesting season from August 2016 to November 2016 and August 2017 to November 2017 (Supplementary Table S1). These post-hatchlings washed ashore in North Florida and later died in rehabilitation. The health condition of post-hatchling washback turtles that strand is already poor (including surviving animals). All post-hatchlings were categorized as thin to emaciated and covered in epibiota. The nature of post-hatchlings stranding is that they were predominantly carried in by strong onshore winds and rough ocean conditions. During the 2016 and 2017 nesting seasons the hospital treated a total of 396 post-hatchlings, 293 of which were successfully released back into the ocean (Figure 1A). The remaining 103 died shortly after being admitted to the hospital or were dead on arrival. A subset (n = 42) of those dead post-hatchlings were necropsied, during which gut content was examined. Post-hatchlings stranded along an approximately 150 km stretch of north Florida coastline, from Nassau County to Flagler County (Supplementary Figure S1A). Deceased individuals were frozen at −20°C until necropsies were performed. Once a batch of carcasses were collected, individuals were thawed under refrigeration at 4°C. Post-hatchling biometric data was recorded as described by Bolten (1999) and the INDICIT Consortium (Bolten, 1999; INDICIT Consortium, 2018). Electronic calipers (iGaging Digital Caliper) were used to measure the maximum straight carapace length (SCLmax), by measuring the distance from the anterior edge of the carapace to the posterior tip of the supracaudals (Figure 1B). Width was measured using electronic calipers at the widest point of the carapace. Mass of the individual post-hatchling was measured using a digital gram scale (Tanita KD-200 Digital Scale).
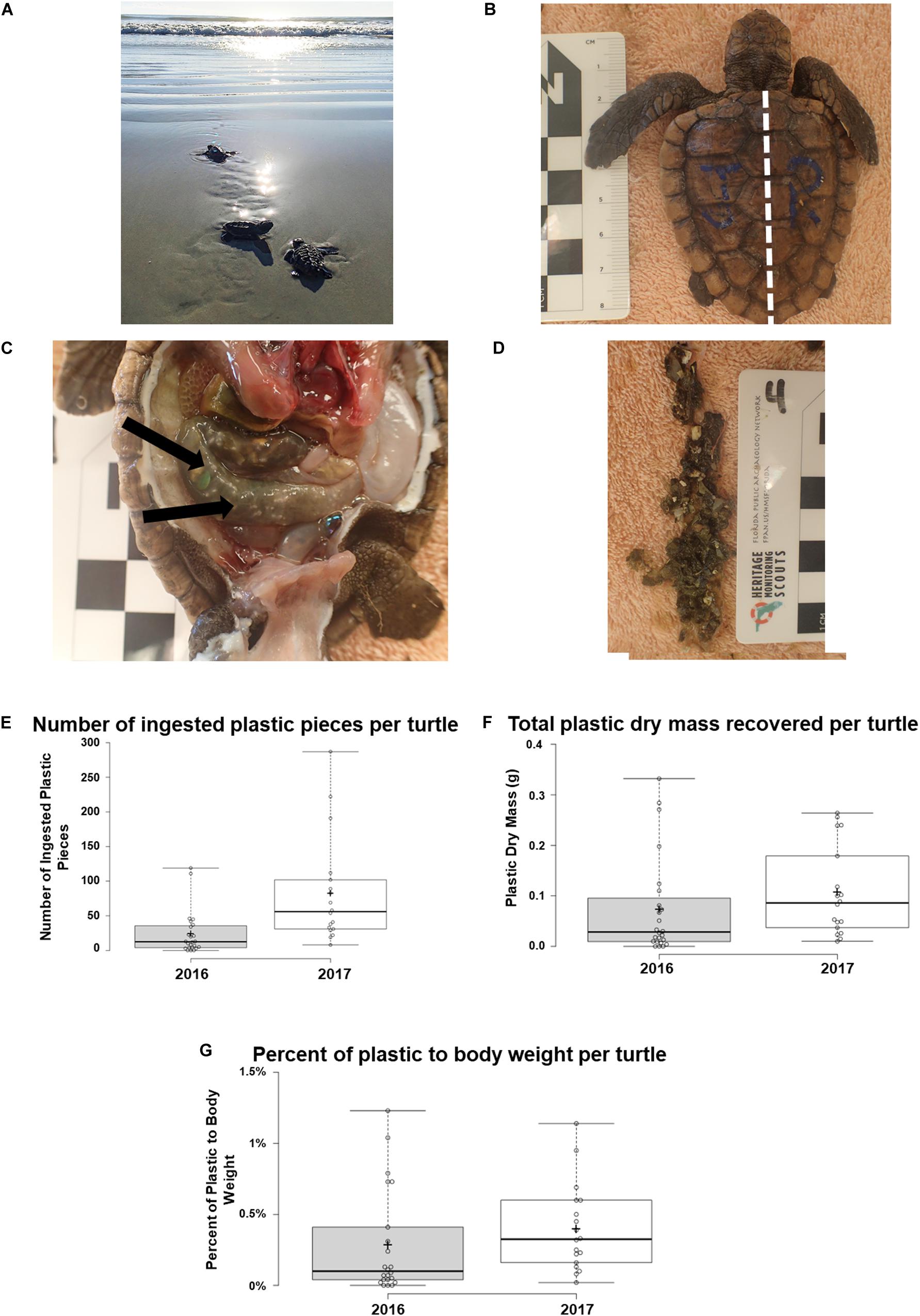
Figure 1. Analysis of plastic pieces identified from post-hatchling washback necropsies. Post-hatchling washback sea turtles. (A) Post-hatchling washback Caretta caretta sea turtles being released back into the Atlantic Ocean, North Florida, after being successfully rehabilitated at the University of Florida’s Whitney Laboratory Sea Turtle Hospital. (B) A deceased loggerhead (C. caretta) washback with scale bar, prior to necropsy. Dashed white line indicates maximum straight carapace length. (C) Intact intestinal tract of a loggerhead washback with arrows indicating presence of plastic. (D) Extracted gastrointestinal tract from a loggerhead necropsy, with scale ruler for size comparison, each box on the scale ruler is 1 cm2. (E) Comparison of the quantity of plastic pieces ingested by washbacks in 2016, compared to 2017. (F) Comparison of the dry mass of ingested plastic pieces in washbacks in 2016, compared to 2017. (G) Comparison of the proportion of ingested plastic to washback weight in 2016 washbacks, compared with 2017 washbacks. Boxplots were generated using BoxPlotR (Spitzer et al., 2014).
A scalpel was used to carefully remove the plastron of each animal (Figure 1C). The GI tract is easily identifiable and was removed in its entirety, from esophagus to cloaca. Scissors were used to incise along the GI tract exposing the contents (Figure 1D) – this method allowed the location of plastics within the GI tract to be observed. For each animal, the intestines and their contents were placed in a dish of deionized fresh water at a depth to fully cover the contents. To separate organic stomach contents from inorganics, forceps were used to massage the material. Organic matter, primarily unidentifiable digested contents, sank to the bottom of the tank while plastics floated to the surface and were collected with forceps. The inorganic material from each animal was cleaned in a 10% H2O2 solution in plastic petri dishes to remove any residual organic matter, utilizing methods adapted from sediment particle size distribution analysis (Osborne and Ellis, 2013). Inorganic material was then rinsed a minimum of three times with deionized water, processed through a vacuum filter [0.7 μm glass fiber filters, in accordance with Lusher and Hernandez-Milian (2018)] to remove moisture, and weighed. Filtered plastics that were observable with the naked eye were analyzed, as sea turtles are considered to be visual feeders (Schuyler et al., 2012). Each piece of plastic was also photographed and categorized under a dissecting microscope into one of three categories; hard plastics, soft plastics, latex. Hardness was determined by manually attempting to flex the material in accordance with MSFD “Guidance on Monitoring of Marine Litter in European Seas” (Galgani et al., 2013). Latex was determined visually to be a bendable, stretchy material. Each piece of hard plastic was measured at the widest point using a dissecting microscope. Color was documented and samples were assigned a color (white, transparent, brown, tan, green, red, blue, black, pink, purple, gray, yellow, and orange) also in accordance with MSFD “Guidance on Monitoring of Marine Litter in European Seas” (Galgani et al., 2013). Distributions and variances of data for all recorded datasets were checked; any datasets not displaying normal distribution (Shapiro–Wilk test) were log transformed. Any datasets not normally distributed (raw data or after log transformation) were analyzed using non-parametric statistical tests. P-values less than 0.05 were considered to be statistically significant.
Results
Near Ubiquitous Plastic Ingestion
The initial health condition of stranded post-hatchling washback turtles was poor (including surviving animals), with all post-hatchlings being categorized as thin to emaciated and covered in epibiota. Out of 42 post-hatchling sea turtles analyzed, plastics were obtained from the gastrointestinal tracts of 39 individuals (92.86%). The mean SCL of these individuals was 57.127 mm (SD 7.648 and SE 1.18), ranging from 45.92 to 76.35 mm. The percent of plastic to body weight for each turtle was variable (Supplementary Table S1). The quantity, type and GI tract location of plastic obtained from each of the necropsied turtles was recorded (Supplementary Table S2).
Quantity, Mass (g) and Percent of Plastic to Body Weight
From the 42 post-hatchling marine turtles 2,068 pieces of plastic were recovered, with plastic burdens ranging from 0 to 287 pieces with an average of 49.24 ± 9.61 (±SE) pieces per individual. A significantly larger quantity of plastic (Mann–Whitney U test, U = 76.5, p = 0.00042) was ingested in 2017 compared to 2016 (Figure 1E). The average dry mass of plastic present was 0.09 ± 0.01 g, ranging from 0.00 to 0.33 g (Supplementary Table S1). There was no significant difference (Mann–Whitney U test, U = 143.5, p = 0.06724) between the dry mass of plastic recovered from 2016 post-hatchlings compared to 2017 post-hatchlings (Figure 1F). The average percent of plastic to body weight was 0.34 ± 0.0005%, ranging from 0.00 to 1.23% (Supplementary Table S1). There was no significant difference (Mann–Whitney U test, U = 127, p = 0.05486) between the average percent of plastic to body weight in 2016 post-hatchlings compared to 2017 post-hatchlings (Figure 1G).
Type of Ingested Plastic
Plastic from each category [as defined by Galgani et al. (2013), Industrial, Hard, Sheet, Balloon, Foam, Thread, Other] was identified with the exception of Foam (Figures 2A,B and Supplementary Table S2). Plastics were predominantly identified as Hard plastic (mean: 30.643 ± 7.482 pieces per turtle), followed by Sheet plastic (14.690 ± 2.619), Thread (2.786 ± 0.613), Balloon (0.929 ± 0.317), Other (0.119 ± 0.070), Industrial (0.071 ± 0.040), and Foam (0) (Figure 2A). There was a significant difference between the amount of plastic recovered from each category [Kruskal–Wallis test, chi-squared = 190.22, df = 6, p-value < 2.2e-16]. The most abundant category was Hard plastic, comprising 62.23% of plastic pieces identified.
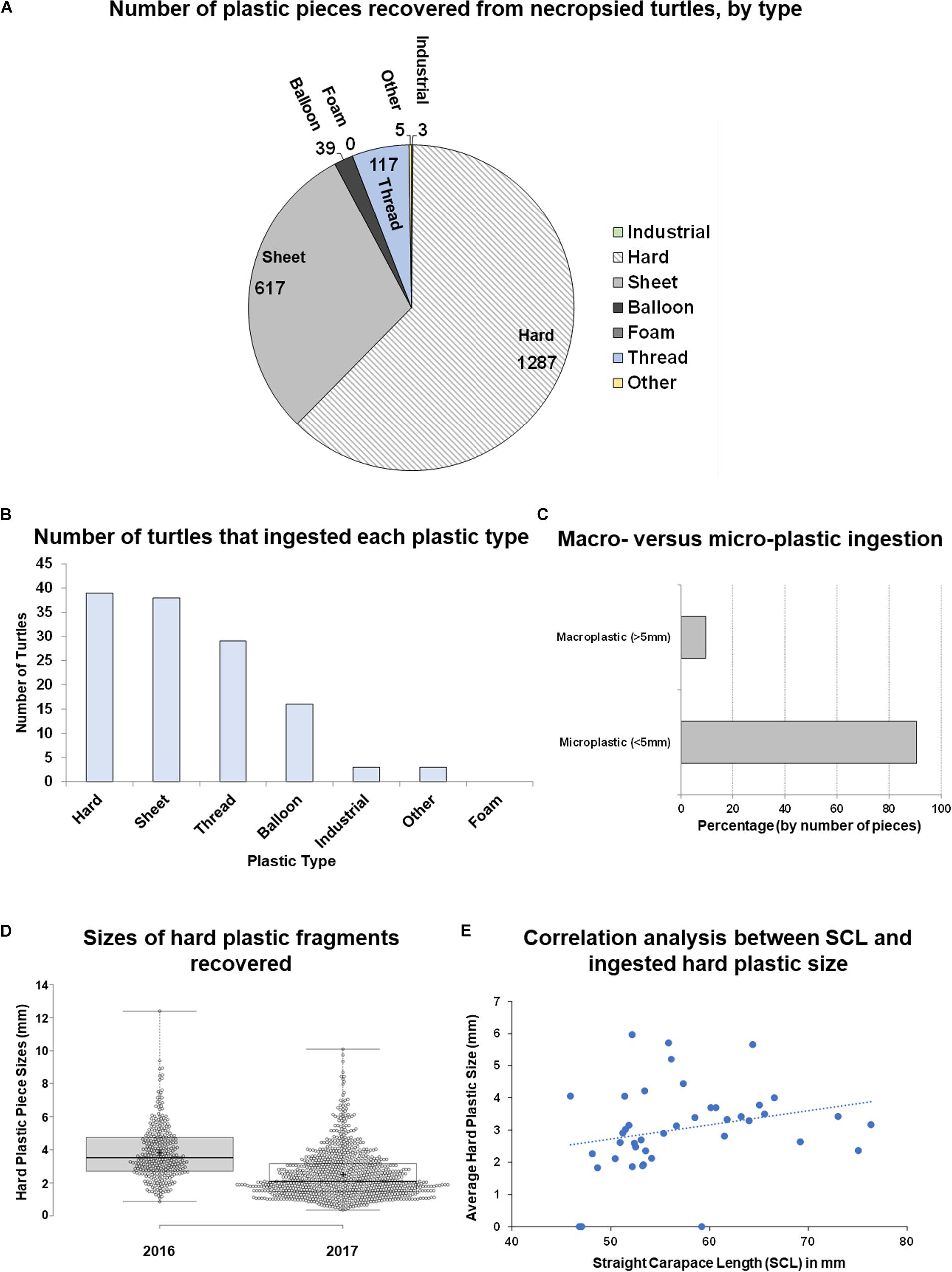
Figure 2. Analysis of plastic types and hard plastic size ingested by post-hatchling washbacks. (A) Quantity of each type of plastic ingested by washbacks (2016 and 2017). (B) Quantity of washbacks that ingested each type of plastic type (2016 and 2017). (C) Percentage of macroplastic versus microplastic ingested (2016 and 2017). (D) Comparison of the maximum size of hard plastic ingested by 2016 washbacks, compared to 2017 washbacks. (E) Correlation of ingested hard plastic size to corresponding washback size (2016 and 2017).
Ingested Plastic Size (mm), Post-hatchling Length Correlations and Plastic Color
With the majority of plastics falling into the Hard category, these fragments were analyzed in more detail to record their size (measured on the longest axis) and color. The majority of plastic recovered from the post-hatchlings was microplastic (<5 mm), with just under 10% being macroplastic (>5 mm, Figure 2C). The maximum length of hard plastic fragments ranged from 0 to 12.393 mm, with an average of 2.825 ± 0.045 mm. Hard plastic fragments recovered from the 2016 post-hatchlings were significantly larger (t-test, p = 6.760E–44, df = 1286) than those recovered from the 2017 post-hatchlings (Figure 2D). There was no correlation between the average length of plastic ingested and the size (SCL, mm) of the post-hatchling (Figure 2E). Similarly, there was no correlation between the total mass of plastic ingested and the size (SCL, mm) of the post-hatchling, or the maximum length of plastic ingested and the size (SCL, mm) of the post-hatchling. Indeed, the longest plastic fragment identified (12.39 mm) was ingested by the smallest turtle (SCL, 45.92 mm). Together, this suggests that even small post-hatchlings are ingesting large pieces of plastic, accumulating high gastrointestinal tract plastic burdens.
In relation to plastic color, white plastics of varying size were determined to occupy the most abundant hard color frequency comprising 70% of the total sample, with other colors ranging from 7 to <1% each (Figure 3A).
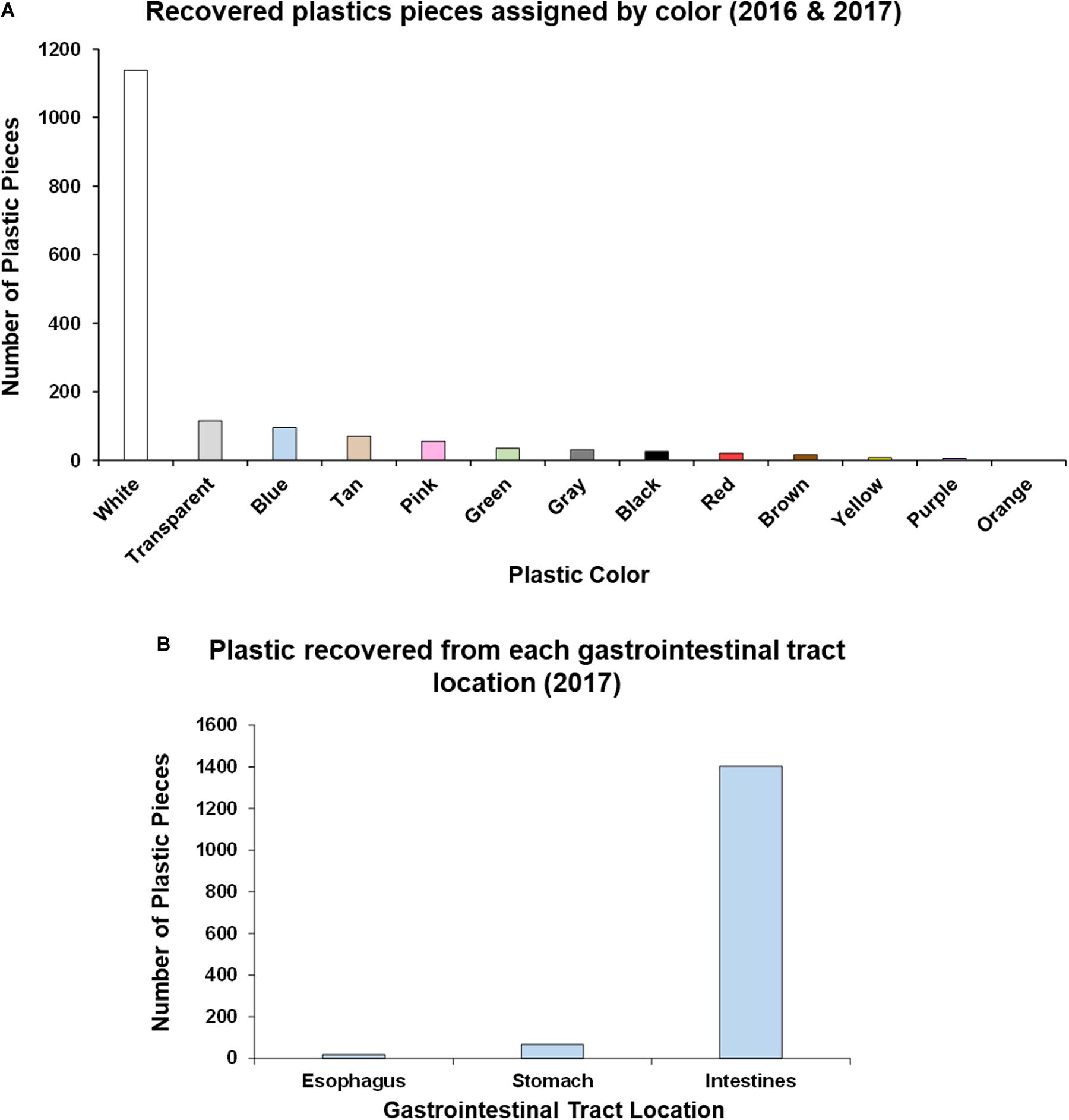
Figure 3. Analysis of plastic ingestion recovery location and color in post-hatchling washbacks. (A) Quantity of plastic pieces of each color recovered from washback necropsies (2016 and 2017). (B) Quantity of plastic pieces recovered from each gastrointestinal tract location (2017).
Gastrointestinal Tract Plastic Location
In the second year of data collection (2017) we implemented a more detailed approach to identifying location within the GI tract (esophagus, stomach, intestine) of these 18 post-hatchlings, as described by Galgani et al. (2013). The majority of plastic (94.29%) was recovered from the intestines, followed by 4.5% from the stomach and 1.21% from the esophagus (Figure 3B and Supplementary Table S2). Significantly more plastic [Kruskal–Wallis test, H(2) = 34.466, p < 0.00001] was recovered from the intestines than the stomach or esophagus. This location trend was also seen in each of the plastic type categories – in each category most of the plastic pieces were recovered from the intestines (Supplementary Table S2).
Discussion
While microplastic ingestion has been shown to be near ubiquitous in older sea turtles the burden of plastic ingestion was not particularly high (Duncan et al., 2019). However, here we demonstrate that marine plastic debris present in the ocean environment is being ingested by post-hatchling (4.6–7.6 cm) loggerhead sea turtles near ubiquitously (92.86% of our deceased post-hatchling sample set) and at high burdens, up to 287 pieces of plastic in a single individual. While this study’s results focused solely on loggerheads, one green sea turtle (C. mydas) and one Kemp’s ridley (Lepidochelys kempii) also stranded and died during the study period. Both of these individuals had also ingested plastic (Supplementary Tables S1, S2), suggesting that plastic ingestion is occurring in post-hatchlings across multiple sea turtle species. With their indiscriminate feeding behavior (Nelms et al., 2016) post-hatchlings may mistakenly ingest plastic debris with likely negative health consequences. Ingested plastic mass accounted for of up to 1.23% of post-hatchling body weight, indicating the strong potential for plastic-related health issues. Marine debris ingestion and entanglement have caused morbidity and mortality in all seven of sea turtle species (Franzen-Klein et al., 2020). The incidence of plastic ingestion in these post-hatchlings is higher than that reported for later life-stages (Campani et al., 2013; Camedda et al., 2014; Vélez-Rubio et al., 2018). However, the incidence of plastic ingestion varies by year and geographic location (Gramentz, 1988; Witherington, 1994; Tomás et al., 2002; Parker et al., 2005; Boyle and Limpus, 2008; Campani et al., 2013; Camedda et al., 2014; Schuyler Q. et al., 2014a; Pham et al., 2017; Vélez-Rubio et al., 2018; Duncan et al., 2019; Table 1).
Entire generations of sea turtles are being impacted by plastic ingestion with no means of egesting larger fragments (hard plastic up to 12.39 mm in length in the post-hatchlings in the current study) (Figure 2D), resulting in accumulation in the GI tract and possible blockage. Studies on early life history stage post-hatchling marine turtles are lacking in the literature, but their small size suggests that they may be at higher risk of mortality due to macro-plastic fragment ingestion (Boyle, 2006; Schuyler et al., 2012; Nelms et al., 2016; White et al., 2018). Thus, exposure to small plastic debris is especially devastating to this size class of three species of sea turtle (Schuyler et al., 2012; Pham et al., 2017). Interestingly, we show that hard plastic fragments recovered from the 2016 post-hatchlings were significantly larger than those recovered from the 2017 post-hatchlings (Figure 2D) despite more plastic pieces being ingested in 2017 (Figure 1E). Additionally, the cohort of post-hatchling washbacks in this study had an average plastic-to-body mass ratio 162% higher than washbacks from southern Florida (White et al., 2018). The average southern Florida ratio was 2.07 mg of ingested plastic per 1 gram of body weight (mg/g, n = 13, sampled 2015–2016), while the average northern Florida ratio was 3.35 mg/g (n = 42, sampled 2016–2017), with the highest northern Florida individual having a ratio of 12.30 mg/g.
Ninety-four percent of plastic (2017) was recovered from the intestine (Figure 3B and Supplementary Table S2), supporting previous findings (Pham et al., 2017). We also found organic material within the GI tracts in addition to plastic contents, indicating that pieces of plastic were likely ingested during active feeding periods. The presence of plastic in the GI tract reduces the ability of the post-hatchling to ingest and efficiently digest natural food items, thus contributing to nutritional dilution, whereby non-nutritive materials fill the gut capacity but lack nutrients, thus impacting decreasing productivity and growth (Bolten and Balazs, 1995; McCauley and Bjorndal, 1999; Bjorndal, 2017; Santos et al., 2020). The cause of mortality was undetermined in these particular sea turtles, however, ingestion of plastics, even when not the causative agent, can have sub-lethal effects (Bjorndal et al., 1994). For example, juvenile loggerheads feed primarily on gelatinous low-nutrient prey and require high volume throughput to obtain the necessary nutrition to maintain normal growth patterns (Bolten and Balazs, 1995; McCauley and Bjorndal, 1999; Bjorndal, 2017). The presence of plastic fragments almost certainly affects nutritional processing through the GI tract and would thus affect normal feeding performance at an already vulnerable life stage (Bolten and Balazs, 1995; Bjorndal, 2017). Furthermore, it is likely that such burdens of plastic in the GI tract also cause some physical discomfort that could affect feeding behavior and even affect buoyancy and swimming performance (White et al., 2018).
In addition to physical blockage and erosion of gut wall epithelia structure, the chronic leaching of chemical additives can also have a multitude of unknown physiological effects on juvenile-stage marine turtles (White et al., 2018). Studies have found that brominated flame retardants (BFRs), which are added to plastic products to reduce flammability, are known endocrine inhibitors and could potentially affect hormone production during early life-stages in turtles (Cohn et al., 2012; Von Moos et al., 2012; Setälä et al., 2014; Cole and Galloway, 2015; Dai et al., 2016; Wang et al., 2016). Similarly, other common chemical additives in plastics are polychlorinated biphenyls (PCB’s), which have been shown to increase the likelihood of developing cancer in mammals and invertebrates (Cohn et al., 2012; Von Moos et al., 2012; Setälä et al., 2014; Cole and Galloway, 2015; Dai et al., 2016; Wang et al., 2016). Chronic exposure to these types of chemicals, especially at a vulnerable developmental stage, could have unknown physiological effects and needs addressing with further research. Recently, this topic has gained traction and it is starting to be researched in turtles. One study observed that a wide variety of chemical additives (PCBs, DDTs, CHLs and BFR’s) were found in three species of sea turtle (C. mydas, C. caretta, and Lepidochelys olivacea), although not all were strictly from plastics (Clukey et al., 2018).
Several studies have attempted to demonstrate a correlation with marine debris and stranding location, but Schuyler Q. et al. (2014a) stated that due to the likelihood of sea turtles encountering marine debris at different life-stages and their inability to regurgitate (Sheavly and Register, 2007), turtles may ingest plastics far from where they actually strand on the shore and can then be retained in the gastrointestinal tract for months (Lutz, 1990). It can be speculated that the plastic fragments from this study were consumed just off the coast of the southeastern United States, since by definition this post-hatchling transitional stage can occur days, weeks, or months after leaving the nesting beach (Bolten, 2003a). The highest concentrations of plastic debris in the Atlantic Ocean occur between 22 degrees and 38 degrees north (Law et al., 2010). This concentration coincides with loggerhead and green turtle developmental habitat, thus further supporting why such a high percentage of our study animals (93%) contained plastic debris in their GI tracts.
Electrophysiological measurements, behavioral studies and the discovery of at least three different retinal photopigments confirms that sea turtles are able to differentiate colors (Fehring, 1972; Granda, 1979; Bartol and Musick, 2003). All colors of plastic have been found to be ingested by C. caretta and C. mydas and neither species discriminates against plastic when feeding (Lutz, 1990). Though sea turtles can and do use olfaction to orient to prey, they are primarily visual feeders (Constantino and Salmon, 2003; Narazaki et al., 2013; Camedda et al., 2014; Fukuoka et al., 2016). The most abundant color of plastic ingested by the post-hatchlings was white (Figure 3A), comprising 70% of the hard plastic fragments and consistent with previous studies (Tourinho et al., 2010; Ryan et al., 2016). This could indicate there is a preferential bias for color selection when foraging in the wild. Interestingly, post-hatchling loggerheads may preferentially forage on different plastic colors than their adult counterparts, in which blue and black are the dominant ingested colors (Duncan et al., 2019). It has been shown that leatherback (Dermochelys coriacea) post-hatchlings primarily rely on visual cues rather than chemical cues to identify food (Constantino and Salmon, 2003). This reliance on visual recognition likely predisposes post-hatchlings to plastic ingestion. Modeling suggests that sea turtles prefer more translucent and flexible debris, mistaking it for prey items such as jellyfish and that they would eat fewer blue debris items (Schuyler Q. A. et al., 2014b). However, this prediction is not what was found for the post-hatchlings in this study (white being the dominant color ingested, outstripping translucent items by over ten-fold), or a study of older life-stages in which blue and black were the dominant ingested colors (Duncan et al., 2019). Benthic phase green and hawksbill (Eretmochelys imbricata) turtles had a strong selectivity for clear plastic (Schuyler et al., 2012). Santos et al. (2016) hypothesize that marine animals that perceive floating plastic from below should preferentially ingest dark plastic fragments, whereas animals that perceive floating plastic from above should select for paler plastic fragments. This hypothesis appears to fit with the differences between post-hatchling sea turtles (white fragment ingestion) which reside near the surface and later life stages (dark fragment ingestion) which dive to deeper depths. Further experimental research is recommended to assess whether the color consumption trend reported here is a reflection of post-hatchling sea turtle selectivity (resembling natural gelatinous prey items) or rather due to the proportion of colors in the environment, arising from manufacturer bias toward producing white/colorless plastics (Schuyler et al., 2012; Camedda et al., 2014; Schuyler Q. et al., 2014a).
Conclusion
Man-made plastic products are continuing to enter the marine environment at unprecedented scales and are dramatically impacting coastal marine life. We have confirmed that vulnerable early life-stage loggerhead post-hatchlings ingest plastic debris in potentially harmful quantities, including fragment sizes that are not easily passed through their GI tract. This is a crucial stage in the life cycle where the turtles are vulnerable to a large number of threats and these Northeastern Florida post-hatchlings must expend large amounts of energy to reach the relative safety of the Sargassum Sea. Therefore, ingestion of plastic may well have a disproportionate effect on the survival of post-hatchling turtles, not only causing direct health implications but indirectly hampering normal feeding and GI activities and reducing the energy available to the turtles to expend on migration, predator evasion and prey capture (McCauley and Bjorndal, 1999). Additional research is needed to fully understand the long-term health and survival impacts of plastic pollution and ingestion, and the associated chemical additives, throughout the entire life-history of these long-lived reptiles. Gaining greater insight into the prevalence of plastic ingestion, the types of plastic, as well as the physiological and toxicological effects of plastic consumption across sea turtle life-stages will elucidate the scale of the problem and help prioritize mitigation efforts for the growing global marine plastic dilemma.
Data Availability Statement
All datasets presented in this study are included in the article/Supplementary Material.
Ethics Statement
Ethical review and approval was not required for the animal study because study was conducted on deceased animals. Animals were not euthanized for the purposes of this study, they died in care during rehabilitation. Rehabilitation activities are covered by Florida Fish and Wildlife Conservation Commission (FWC) rehabilitation permits MTP-16-228 to MTP-17-228.
Author Contributions
CE, TO, and DD designed and supervised the project. JF, DR, RT, and JP generated the data. JF, CE, LW, SE, and DD performed the data analysis and figure preparation. CE, JF, LW, SE, TO, MM, and DD wrote the manuscript. All authors read and approved the final manuscript.
Funding
JF received a Gumbo Limbo Nature Center, Inc d/b/a Friends of Gumbo Limbo (a 501c3 non-profit organization) Grant through a generous donation through their Graduate Research Grant program. LW was funded by an Irish Research Council Government of Ireland Postgraduate Scholarship, under project number GOIPG/2020/1056.
Conflict of Interest
The authors declare that the research was conducted in the absence of any commercial or financial relationships that could be construed as a potential conflict of interest.
Acknowledgments
Warmest thanks to Nancy Condron, and the veterinary and rehabilitation staff and volunteers of the Sea Turtle Hospital at Whitney Laboratory. Thanks also are due to the Florida Fish and Wildlife Conservation Commission’s Meghan Koperski for valuable assistance with hospital permitting.
Supplementary Material
The Supplementary Material for this article can be found online at: https://www.frontiersin.org/articles/10.3389/fmars.2020.00693/full#supplementary-material
FIGURE S1 | (A) Map of the approximately 150 km stretch of north Florida coastline (Nassau County to Flagler County), over which the necropsied post- hatchlings stranded along. Map was modified from one generated by Eric Gaba (https://commons.wikimedia.org/wiki/File:USA_Florida_relief_location_map.jpg) and used under creative commons, CC BY-SA (https://creativecommons.org/licenses/by-sa/3.0).
TABLE S1 | Proportion of mass and weight of ingested plastic to size and weight of corresponding 2016 and 2017 post-hatchling washbacks.
TABLE S2 | Data for the type, location and total plastic ingested by each post-hatchling washback necropsied in 2016 and 2017.
Footnotes
References
Allen, S., Allen, D., Phoenix, V. R., Le Roux, G., Durántez Jiménez, P., Simonneau, A., et al. (2019). Atmospheric transport and deposition of microplastics in a remote mountain catchment. Nat. Geosci. 12, 339–344. doi: 10.1038/s41561-019-0335-5
Anderson, A., Andrady, A., Arthur, C., Baker, J., Bouwman, H., Gall, S., et al. (2015). “Sources, fate and effects of microplastics in the marine environment: a global assessment,” in IMO/FAO/UNESCO-IOC/UNIDO/WMO/IAEA/UN/UNEP/UNDP Joint Group of Experts on the Scientific Aspects of Marine Environmental Protection) Rep Stud GESAMP, ed. P. Kershaw (London: International Maritime Organization).
Bartol, S. M., and Musick, J. A. (2003). Sensory biology of sea turtles. Biol. Sea Turtles 2, 79–102. doi: 10.1201/9781420040807.ch3
Bjorndal, K. A. (2017). “Foraging ecology and nutrition of sea turtles,” in Foraging ecology and nutrition of sea turtles, in The Biology of Sea Turtles, eds L. P. Lutz, and J. A. Musick (Boca Raton, FL: CRC press), 213–246.
Bjorndal, K. A., Bolten, A. B., and Lagueux, C. J. (1994). Ingestion of marine debris by juvenile sea turtles in coastal Florida habitats. Mar. Pollut. Bull. 28, 154–158. doi: 10.1016/0025-326x(94)90391-3
Bolten, A. B. (1999). “Techniques for Measuring Sea Turtles,” in Research and Management Techniques for the Conservation of Sea Turtles, eds K. Eckert, K. Bjorndal, F. Abreu-Grobois, and M. Donnelly (Washington, DC: IUCN/SSC Marine Turtle Specialist Group Publication No. 4).
Bolten, A. B. (2003a). “Active swimmers–passive drifters: the oceanic juvenile stage of loggerheads in the Atlantic system,” in Loggerhead Sea Turtles, eds A. B. Bolten, and B. E. Witherington (Washington, DC: Smithsonian Institution Press), 63–78.
Bolten, A. B. (2003b). “Variation in sea turtle life history patterns: neritic vs. oceanic developmental stages.,” in The Biology of Sea Turtles, eds P. L. Lutz, J. Musick, and J. Wyneken (Boca Raton, FL: CRC Press) 2, 243–257. doi: 10.1201/9781420040807.ch9
Bolten, A., and Balazs, G. (1995). Biology of the Early Pelagic Stage–the “Lost Year.”. Washington DC: Smithsonian Institution Press.
Boyle, M. C., and Limpus, C. J. (2008). The stomach contents of post-hatchling green and loggerhead sea turtles in the southwest Pacific: an insight into habitat association. Mar. Biol. 155, 233–241. doi: 10.1007/s00227-008-1022-z
Brahney, J., Hallerud, M., Heim, E., Hahnenberger, M., and Sukumaran, S. (2020). Plastic rain in protected areas of the United States. Science 368, 1257–1260. doi: 10.1126/science.aaz5819
Camedda, A., Marra, S., Matiddi, M., Massaro, G., Coppa, S., Perilli, A., et al. (2014). Interaction between loggerhead sea turtles (Caretta caretta) and marine litter in Sardinia (Western Mediterranean Sea). Mar. Environ. Res. 100, 25–32. doi: 10.1016/j.marenvres.2013.12.004
Campani, T., Baini, M., Giannetti, M., Cancelli, F., Mancusi, C., Serena, F., et al. (2013). Presence of plastic debris in loggerhead turtle stranded along the tuscany coasts of the pelagos sanctuary for mediterranean marine mammals (Italy). Mar. Pollut. Bull. 74, 225–230. doi: 10.1016/j.marpolbul.2013.06.053
Carr, A. (1987). Impact of nondegradable marine debris on the ecology and survival outlook of sea turtles. Mar Pollut Bull. 18, 352–356. doi: 10.1016/s0025-326x(87)80025-5
Choy, C. A., Robison, B. H., Gagne, T. O., Erwin, B., Firl, E., Halden, R. U., et al. (2019). The vertical distribution and biological transport of marine microplastics across the epipelagic and mesopelagic water column. Sci. Rep. 9:7843. doi: 10.1038/s41598-019-44117-2
Clukey, K. E., Lepczyk, C. A., Balazs, G. H., Work, T. M., Li, Q. X., Bachman, M. J., et al. (2018). Persistent organic pollutants in fat of three species of Pacific pelagic longline caught sea turtles: accumulation in relation to ingested plastic marine debris. Sci. Total Environ. 61, 402–411. doi: 10.1016/j.scitotenv.2017.07.242
Cohn, B. A., Terry, M. B., Plumb, M., and Cirillo, P. M. (2012). Exposure to polychlorinated biphenyl (PCB) congeners measured shortly after giving birth and subsequent risk of maternal breast cancer before age 50. Breast Cancer Res. Treat. 136, 267–275. doi: 10.1007/s10549-012-2257-4
Cole, M., and Galloway, T. S. (2015). Ingestion of nanoplastics and microplastics by pacific oyster larvae. Environ. Sci. Technol. 49, 14625–14632. doi: 10.1021/acs.est.5b04099
Cole, M., Lindeque, P., Halsband, C., and Galloway, T. S. (2011). Microplastics as contaminants in the marine environment: a review. Mar. Pollut. Bull. 62, 2588–2597. doi: 10.1016/j.marpolbul.2011.09.025
Constantino, M. A., and Salmon, M. (2003). Role of chemical and visual cues in food recognition by leatherback posthatchlings (Dermochelys coriacea L). Zoology 106, 173–181. doi: 10.1078/0944-2006-00114
Cox, K. D., Covernton, G. A., Davies, H. L., Dower, J. F., Juanes, F., and Dudas, S. E. (2019). Human consumption of Microplastics. Environ. Sci. Technol. 53, 7068–7074. doi: 10.1021/acs.est.9b01517
Crump, A., Mullens, C., Bethell, E. J., Cunningham, E. M., and Arnott, G. (2020). Microplastics disrupt hermit crab shell selection. Biol. Lett. 16:20200030. doi: 10.1098/rsbl.2020.0030
Dai, Q., Min, X., and Weng, M. (2016). A review of polychlorinated biphenyls (PCBs) pollution in indoor air environment. J. Air. Waste Manage. Assoc. 66, 941–950. doi: 10.1080/10962247.2016.1184193
Derraik, J. G. B. (2002). The pollution of the marine environment by plastic debris: a review. Mar. Pollut. Bull. 44, 842–852. doi: 10.1016/s0025-326x(02)00220-5
Desforges, J.-P. W., Galbraith, M., and Ross, P. S. (2015). Ingestion of microplastics by zooplankton in the Northeast Pacific Ocean. Arch. Environ. Contam. Toxicol. 69, 320–330. doi: 10.1007/s00244-015-0172-5
Duffy, D. J., and Burkhalter, B. (2020). When is a lab animal not a lab animal? Rehabilitation of tumor-aficted sea turtles, and their utilization as a natural model for human and wildlife cancers. Lab. Animal. 49, 95–98. doi: 10.1038/s41684-020-0504-6
Duncan, E. M., Broderick, A. C., Fuller, W. J., Galloway, T. S., Godfrey, M. H., Hamann, M., et al. (2019). Microplastic ingestion ubiquitous in marine turtles. Global Change Biol. 25, 744–752. doi: 10.1111/gcb.14519
Fehring, W. K. (1972). Hue discrimination in hatchling loggerhead turtles (Caretta caretta caretta). Anim. Behav. 20, 632–636. doi: 10.1016/s0003-3472(72)80135-0
Franzen-Klein, D., Burkhalter, B., Sommer, R., Weber, M., Zirkelbach, B., and Norton, T. (2020). Diagnosis and management of marine debris ingestion and entanglement by using advanced imaging and endoscopy in sea turtles. J. Herpetol. Med. Surg. 30:74. doi: 10.5818/17-09-126
Fukuoka, T., Yamane, M., Kinoshita, C., Narazaki, T., Marshall, G. J., Abernathy, K. J., et al. (2016). The feeding habit of sea turtles influences their reaction to artificial marine debris. Sci. Rep. 6:28015. doi: 10.1038/srep28015
Galgani, F., Hanke, G., Werner, S., Oosterbaan, L., Nilsson, P., Fleet, D., et al. (2013). Guidance on Monitoring of Marine Litter in European Seas. Ispra: Publications Office of the European Union.
GESAMP (1991). GESAMP Report of the 21st session. IMO/FAO/UNESCO-IOC/UNIDO/WMO/IAEA/UN/UNEP/UNDP Joint Group of Experts on the Scientific Aspects of Marine Environmental Protection (GESAMP) Rep Stud GESAMP MO/FAO/UNESCO-IOC/UNIDO/WMO/IAEA/UN/UNEP/UNDP), London: International Maritime Organization.
Gramentz, D. (1988). Involvement of loggerhead turtle with the plastic, metal, and hydrocarbon pollution in the central Mediterranean. Mar. Pollut. Bull. 19, 11–13. doi: 10.1016/0025-326x(88)90746-1
Granda, A. (1979). Eyes and their Sensitivity to Light of Differing Wavelengths. New York, NY: John Wiley & Sons, 266.
INDICIT Consortium (2018). Monitoring Marine Litter Impacts on sea Turtles. Protocol for the Collection of data on ingestion and entanglement in the loggerhead turtle (Caretta caretta Linnaeus, 1758). Brussel: European Union.
Kane, I. A., Clare, M. A., Miramontes, E., Wogelius, R., Rothwell, J. J., Garreau, P., et al. (2020). Seafloor microplastic hotspots controlled by deep-sea circulation. Science 68, 1140-1145. doi: 10.1126/science.aba5899
Kelly, A., Lannuzel, D., Rodemann, T., Meiners, K. M., and Auman, H. J. (2020). Microplastic contamination in east Antarctic sea ice. Mar. Pollut. Bull. 154:111130. doi: 10.1016/j.marpolbul.2020.111130
Law, K. L., Morét-Ferguson, S., Maximenko, N. A., Proskurowski, G., Peacock, E. E., Hafner, J., et al. (2010). Plastic accumulation in the North Atlantic subtropical gyre. Science 329, 1185–1188. doi: 10.1126/science.1192321
Li, W. C., Tse, H. F., and Fok, L. (2016). Plastic waste in the marine environment: a review of sources, occurrence and effects. Sci. Total Environ. 56, 333–349. doi: 10.1016/j.scitotenv.2016.05.084
Lusher, A. L., and Hernandez-Milian, G. (2018). Microplastic extraction from marine vertebrate digestive tracts, regurgitates and scats: a protocol for researchers from all experience levels. Bio Protocol. 8:e3087. doi: 10.21769/BioProtoc.3087
Lutz, P. L. (1990). “Studies on the ingestion of plastic and latex by sea turtles,” in Proceedings of the 2nd International Conference on Marine Debris: US Department of Commerce, eds R. S. Shomura, and I. L. Godfrey (Honolulu, HI: NOAA Tech).
Mansfield, K. L., Wyneken, J., Porter, W. P., and Luo, J. (2014). First satellite tracks of neonate sea turtles redefine the ‘lost years’ oceanic niche. Proc. R. Soc. B Biol. Sci. 281:20133039. doi: 10.1098/rspb.2013.3039
McCauley, S. J., and Bjorndal, K. A. (1999). Conservation implications of dietary dilution from debris ingestion: sublethal effects in post−hatchling loggerhead sea turtles. Conserv. Biol. 13, 925–929. doi: 10.1046/j.1523-1739.1999.98264.x
Mellgren, R. L., Mann, M. A., Bushong, M. E., Harkins, S. R., and Keathley, V. L. (2003). Habitat selection and antipredator behavior in three species of hatchling sea turtles. Int. J. Comp. Psychol. 16, 156–117.
Narazaki, T., Sato, K., Abernathy, K. J., Marshall, G. J., and Miyazaki, N. (2013). Loggerhead turtles (Caretta caretta) use vision to forage on gelatinous prey in mid-water. PLoS One 8:e66043. doi: 10.1371/journal.pone.0066043
Nelms, S. E., Duncan, E. M., Broderick, A. C., Galloway, T. S., Godfrey, M. H., Hamann, M., et al. (2016). Plastic and marine turtles: a review and call for research. ICES J. Mar. Sci. 73, 165–181. doi: 10.1093/icesjms/fsv165
Osborne, T. Z., and Ellis, L. R. (2013). Removal of Organic Matter from Hydric Soils for Textural Analysis using Hydrogen Peroxide. Technical Memorandum TM-12-2013 Palatka: St Johns River Water Management District.
Parker, D. M., Cooke, W. J., and Balazs, G. H. (2005). Diet of oceanic loggerhead sea turtles (Caretta caretta) in the central North Pacific. Fish. Bull. 103, 142–152.
Peeken, I., Primpke, S., Beyer, B., Gütermann, J., Katlein, C., Krumpen, T., et al. (2018). Arctic sea ice is an important temporal sink and means of transport for microplastic. Nat. Commun. 9:1505. doi: 10.1038/s41467-018-03825-5
Pham, C. K., Rodríguez, Y., Dauphin, A., Carriço, R., Frias, J. P. G. L., Vandeperre, F., et al. (2017). Plastic ingestion in oceanic-stage loggerhead sea turtles (Caretta caretta) off the North Atlantic subtropical gyre. Mar. Pollut. Bull. 121, 222–229. doi: 10.1016/j.marpolbul.2017.06.008
Putman Nathan, F., and Mansfield Katherine, L. (2015). Direct evidence of swimming demonstrates active dispersal in the sea turtle “Lost Years”. Curr. Biol. 25, 1221–1227. doi: 10.1016/j.cub.2015.03.014
Rochman, C., Andrady, A., Dudas, S., Fabres, J., Galgani, F., Lead, D., et al. (2016). Sources, Fate and Effects of Microplastics in the Marine Environment: Part 2 of a Global Assessment: UNEP/UNDP Joint Group of Experts on the Scientific Aspects of Marine Environmental Protection, London: International Maritime Organization.
Ryan, P. G., Cole, G., Spiby, K., Nel, R., Osborne, A., and Perold, V. (2016). Impacts of plastic ingestion on post-hatchling loggerhead turtles off South Africa. Mar. Pollut. Bull. 107, 155–160. doi: 10.1016/j.marpolbul.2016.04.005
Santos, R. G., Andrades, R., Demetrio, G. R., Kuwai, G. M., and Sobral, M. F. (2020). Vieira JdS and Machovsky-Capuska GE. Exploring plastic-induced satiety in foraging green turtles. Environ Pollut. 265:114918. doi: 10.1016/j.envpol.2020.114918
Santos, R. G., Andrades, R., Fardim, L. M., and Martins, A. S. (2016). Marine debris ingestion and Thayer’s law – The importance of plastic color. Environ. Pollut. 214, 585–588. doi: 10.1016/j.envpol.2016.04.024
Schuyler, Q. A., Wilcox, C., Townsend, K., Hardesty, B. D., and Marshall, N. J. (2014b). Mistaken identity? Visual similarities of marine debris to natural prey items of sea turtles. BMC Ecol. 14:14. doi: 10.1186/1472-6785-14-14
Schuyler, Q., Hardesty, B. D., Wilcox, C., and Townsend, K. (2012). To eat or not to eat? Debris selectivity by marine turtles. PloS One 7:e40884. doi: 10.1371/journal.pone.0040884
Schuyler, Q., Hardesty, B. D., Wilcox, C., and Townsend, K. (2014a). Global analysis of anthropogenic debris ingestion by sea turtles. Conserv. Biol. 28, 129–139. doi: 10.1111/cobi.12126
Setälä, O., Fleming-Lehtinen, V., and Lehtiniemi, M. (2014). Ingestion and transfer of microplastics in the planktonic food web. Environ. Pollut. 185, 77–83. doi: 10.1016/j.envpol.2013.10.013
Sheavly, S. B., and Register, K. M. (2007). Marine debris & plastics: environmental concerns, sources, impacts and solutions. J Polym. Environ. 15, 301–305. doi: 10.1007/s10924-007-0074-3
Spitzer, M., Wildenhain, J., Rappsilber, J., and Tyers, M. (2014). BoxPlotR: a web tool for generation of box plots. Nat. Methods. 11, 121–122. doi: 10.1038/nmeth.2811
Thompson, R. C., Olsen, Y., Mitchell, R. P., Davis, A., Rowland, S. J., John, A. W. G., et al. (2004). Lost at sea: where is all the plastic? Science 304, 838–838. doi: 10.1126/science.1094559
Tomás, J., Guitart, R., Mateo, R., and Raga, J. A. (2002). Marine debris ingestion in loggerhead sea turtles, Caretta caretta, from the Western Mediterranean. Mar. Pollut. Bull. 44, 211–216. doi: 10.1016/s0025-326x(01)00236-3
Tourinho, P. S., do Sul, J. A. I., and Fillmann, G. (2010). Is marine debris ingestion still a problem for the coastal marine biota of southern Brazil? Mar. Pollut. Bull. 60, 396–401. doi: 10.1016/j.marpolbul.2009.10.013
Van Buskirk, J., and Crowder, L. B. (1994). Life-history variation in marine turtles. Copeia 66–81. doi: 10.2307/1446672
Vélez-Rubio, G., Teryda, N., Asaroff, P., Estrades, A., Rodriguez, D., and Tomás, J. (2018). Differential impact of marine debris ingestion during ontogenetic dietary shift of green turtles in Uruguayan waters. Mar. Pollut. Bull. 127, 603–611. doi: 10.1016/j.marpolbul.2017.12.053
Von Moos, N., Burkhardt-Holm, P., and Köhler, A. (2012). Uptake and effects of microplastics on cells and tissue of the blue mussel Mytilus edulis L. after an experimental exposure. Environ. Sci. Technol. 46, 11327–11335. doi: 10.1021/es302332w
Wang, J., Tan, Z., Peng, J., Qiu, Q., and Li, M. (2016). The behaviors of microplastics in the marine environment. Mar. Environ. Res. 113, 7–17. doi: 10.1016/j.marenvres.2015.10.014
White, E. M., Clark, S., Manire, C. A., Crawford, B., Wang, S., Locklin, J., et al. (2018). Ingested micronizing plastic particle compositions and size distributions within stranded post-hatchling sea turtles. Environ. Sci. Technol. 52, 10307–10316. doi: 10.1021/acs.est.8b02776
Witherington, B. (1994). “Flotsam, jetsam, post-hatchling loggerheads, and the advecting surface smorgasbord,” in Proceedings of the 14th Annual Symposium on Sea Turtle Biology and Conservation, eds K. A. Bjorndal, A. B. Bolten, D. A. Johnson, and P. J. Eliazar. (Honolulu, HI: NOAA Tech)
Witherington, B. (2002). Ecology of neonate loggerhead turtles inhabiting lines of downwelling near a Gulf Stream front. Mar. Biol. 140, 843–853. doi: 10.1007/s00227-001-0737-x
Witherington, B., Hirama, S., and Hardy, R. (2012). Young sea turtles of the pelagic Sargassum-dominated drift community: habitat use, population density, and threats. Mar. Ecol. Prog. Ser. 463, 1–22. doi: 10.3354/meps09970
Worm, B., Lotze, H. K., Jubinville, I., Wilcox, C., and Jambeck, J. (2017). Plastic as a persistent marine pollutant. Annu. Rev. Environ. Resour. 42, 1–26. doi: 10.1146/annurev-environ-102016-060700
Wright, S. L., Rowe, D., Thompson, R. C., and Galloway, T. S. (2013). Microplastic ingestion decreases energy reserves in marine worms. Curr. Biol. 23, R1031–R1033. doi: 10.1016/j.cub.2013.10.068
Keywords: plastic, microplastic, marine turtles, pollution, Caretta caretta, marine debris, Lepidochelys kempii
Citation: Eastman CB, Farrell JA, Whitmore L, Rollinson Ramia DR, Thomas RS, Prine J, Eastman SF, Osborne TZ, Martindale MQ and Duffy DJ (2020) Plastic Ingestion in Post-hatchling Sea Turtles: Assessing a Major Threat in Florida Near Shore Waters. Front. Mar. Sci. 7:693. doi: 10.3389/fmars.2020.00693
Received: 07 April 2020; Accepted: 30 July 2020;
Published: 25 August 2020.
Edited by:
Pierluigi Carbonara, COISPA Tecnologia & Ricerca, ItalyReviewed by:
Robson G. Santos, Federal University of Alagoas, BrazilGaelle Darmon, Université Paris Sciences et Lettres, France
Copyright © 2020 Eastman, Farrell, Whitmore, Rollinson Ramia, Thomas, Prine, Eastman, Osborne, Martindale and Duffy. This is an open-access article distributed under the terms of the Creative Commons Attribution License (CC BY). The use, distribution or reproduction in other forums is permitted, provided the original author(s) and the copyright owner(s) are credited and that the original publication in this journal is cited, in accordance with accepted academic practice. No use, distribution or reproduction is permitted which does not comply with these terms.
*Correspondence: Catherine B. Eastman, cbeastman@whitney.ufl.edu
†These authors have contributed equally to this work