Current Status of the Algae Production Industry in Europe: An Emerging Sector of the Blue Bioeconomy
- 1European Commission, Joint Research Centre (JRC), Ispra, Italy
- 2CIIMAR/CIMAR, Interdisciplinary Centre of Marine and Environmental Research, Novo Edifício do Terminal de Cruzeiros do Porto de Leixões, Matosinhos, Portugal
- 3Department of Bioscience, Centre for Circular Bioeconomy, Aarhus University, Silkeborg, Denmark
- 4Independent Researcher, Weiden am See, Austria
- 5Centro de Investigacións Mariñas (CIMA), Consellería do Mar, Xunta de Galicia, Pedras de Corón, Vilanova de Arousa, Spain
- 6Institute for Inorganic and Analytical Chemistry, Friedrich-Schiller-University Jena, Jena, Germany
- 7Institute of Molecular and Cell Biology, University of Tartu, Tartu, Estonia
- 8Vetik OÜ, Saare County, Estonia
- 9French Research Institute for Exploitation of the Sea (Ifremer), Centre de Brest, Département STH, Plouzané, France
- 10Arramara Teoranta, Oyster Bay, Ireland
- 11Archimede Ricerche Srl, Camporosso, Italy
- 12Seaweeds Center, Marine Culture Unit “El Bocal”, Oceanographic Center of Santander, Spanish Institute of Oceanography (IEO), Santander, Spain
- 13Møreforsking AS, Ålesund, Norway
- 14Algalíf Iceland ehf, Reykjanesbaer, Iceland
- 15Roquette Klötze GmbH & Co., Klötze, Germany
The EU Bioeconomy Strategy aims to support the sustainable growth and development of the EU bio-based sectors while creating jobs, innovation and services. Despite the recognized potential of the algae biomass value chain, significant knowledge gaps still exist regarding the dimension, capability, organization and structure of the algae production in Europe. This study presents and analyses the results of a comprehensive mapping and detailed characterization of the algae production at the European scale, encompassing macroalgae, microalgae, and the cyanobacteria Spirulina. This work mapped 447 algae and Spirulina production units spread between 23 countries, which represents an important addition to the reported number of algae producing countries. More than 50% of these companies produce microalgae and/or Spirulina. Macroalgae production is still depending on harvesting from wild stocks (68% of the macroalgae producing units) but macroalgae aquaculture (land-based and at sea) is developing in several countries in Europe currently representing 32% of the macroalgae production units. France, Ireland, and Spain are the top 3 countries in number of macroalgae production units while Germany, Spain, and Italy stand for the top 3 for microalgae. Spirulina producers are predominantly located in France, Italy, Germany, and Spain. Algae and Spirulina biomass is directed primarily for food and food-related applications including the extraction of high-value products for food supplements and nutraceuticals. Algae production in Europe remains limited by a series of technological, regulatory and market-related barriers. Yet, the results of this study emphasize that the European algae sector has a considerable potential for sustainable development as long as the acknowledged economic, social and environmental challenges are addressed.
Highlights
- 447 algae and Spirulina spp. production units currently exist in Europe.
- A variety of species, production methods and commercial applications have been identified throughout the European countries.
- In Europe, the harvesting of wild stocks is the predominant production system for macroalgae (68% of the production units mapped). In the case of microalgae, photobioreactors are the main production method (71%) while for Spirulina spp., the open ponds prevail (83%).
- Upscaling of the production volumes and technological and market developments are key drivers to boost the growth of the sector in Europe.
- The European algae industry is a promising emerging sector of the EU Blue Bioeconomy with many data gaps still to be filled with, inter alia, studies like our research.
Introduction
Political Context
The current EU political priorities favor a transition to a sustainable economy balancing the growth of economic activities, the protection of natural resources and the needs of a growing world population. The EU Bioeconomy Strategy (adopted in 2012 and updated in 2018) (EC, 2018) aims at implementing a sustainable and circular bioeconomy throughout Europe. Its Action Plan encourages the strengthening and development of the EU bio-based sectors and the pursuit of sustainable food and production systems. In parallel, the EU Blue Growth Strategy (EC, 2012) fosters the creation of jobs in coastal areas and the sustainable growth of Europe’s maritime economy and highlights aquaculture and blue biotechnology sectors among its priority targets.
The new European Commission’s Green Deal targets priority areas where the algae production sector can provide a relevant contribution. These are, for example, the goals of the EU of becoming climate neutral by 2050, the protection of biodiversity (EC, 2020b), the development of a circular economy (EC, 2020a) and the contribution to the farm to fork strategy for sustainable food (EC, 2020c). Specific priorities of the Commissioner for Environment and Oceans are to boost the EU blue economy potential while pursuing the sustainability of fisheries and aquaculture production and their contribution to global food security and sustainability.
Environmental, Social and Economic Potential
Algae are key components of the marine environment and may play a significant role in addressing the EU strategical priorities. Algae provide many essential ecosystem services. Algae contribute significantly to the global primary production while also playing an important role in the uptake of dissolved nutrients from the surrounding environment, coastal defense from hazardous waves and potentially in carbon sequestration (e.g., Dayton, 1985; Chapman, 1995; Steneck et al., 2002; Christie et al., 2009; Teagle et al., 2017). Macroalgae (or seaweeds) are also critical habitat-structuring species in coastal ecosystems (Reisewitz et al., 2006; Bertocci et al., 2015) whereas microalgae, as phytoplankton, constitute the basis of the marine and aquatic food-chain as the prime source of omega 3 fatty acids (Adarme-Vega et al., 2012).
Algae are used in an increasing number of commercial applications. Already widely established in Asia as a foodstuff, algae biomass is increasingly being incorporated in western diets directly for human consumption or used in a variety of food applications (Peteiro, 2018). Interest in including varying algae species in the European gastronomy has increased in recent years due to their nutritional and therapeutic properties and the search for more sustainable and natural food sources (Wells et al., 2016; Rioux et al., 2017; Mouritsen et al., 2019). Moreover, the genus Arthrospira, (commercially known and referred hereafter as Spirulina) (Vonshak and Tomaselli, 2002), is a cyanobacteria that has been traditionally used in Western human nutrition since the 1970s and is currently referred to as a “super food” given its nutritional properties (Jung et al., 2019). Microalgae are also widely used as food and nutritional supplements, with some species being important sources of specific components such as antioxidants, pigments, oils, and vitamins (Hemantkumar and Rahimbhai, 2019; Niccolai et al., 2019). Additionally, some seaweed species collected along the European coasts such as Laminaria digitata, L. hyperborea, Ascophyllum nodosum, and Gelidium corneum are used as feedstock for the extraction of food, pharmaceutical, biomedical or biotechnological grades of the hydrocolloids alginate and agar (Kraan, 2012; Peteiro, 2018). Algae biomass is also used in feed for aquaculture (Makkar et al., 2016; Wan et al., 2019) and recently explored as a feed supplement for cattle to improve weight gain while reducing enteric methane emissions (Machado et al., 2014; Roque et al., 2019; Kinley et al., 2020). Further uses include fertilizer and plant biostimulant applications as well as a source of high quality and high-value bioactive products for cosmetics and nutraceuticals (Thomas and Kim, 2013; Milledge et al., 2015; Chatterjee et al., 2017).
New applications of algae biomass are currently being explored for bioremediation and biomonitoring (Deng et al., 2007; Reis et al., 2016; Zeraatkar et al., 2016), biofuel production (Darda et al., 2019; Ravanal et al., 2019) and biopolymers (e.g., bioplastics) (Abdul Khalil et al., 2017; Zhang et al., 2019). Recent studies, some of which still at an early experimental phase, have emphasized the potential interest of these marine bioresources for innovative health products (Bogie et al., 2019; Kwon et al., 2020). Despite the growing interest and the potential of the algae production as an innovative sector within the EU bioeconomy, the current status of the algae industry in Europe remains largely unknown.
Robust and comprehensive information on the European algae sector is needed to adequately guide knowledge-based decisions and strategies supporting the socio-economic growth and environmental sustainability of the activity in Europe.
Available Knowledge
Data on the amount of algae biomass produced in Europe is reported under different EU level collection frameworks for catch and aquaculture statistics. This data is based on Member States and European Economic Area (EEA) member countries submissions (EC 762/2008, 2008; EC 216/2009, 2009; EC 217/2009, 2009; EC 218/2009, 2009; EU 2019/909, 2019) and centralized in Eurostat fisheries statistics and the European Commission’s Joint Research Centre (JRC). At the global level, the Food and Agricultural Organization of the United Nations (FAO) also provides data for algae biomass (FAO, 2020). However, the available data are fragmented, incomplete and generally of low quality, which prevents a robust analysis of the sector. Due to the small volume of the algae production compared to other biomass sources, frequently there are no national legal obligations to report detailed data and the production is given as part of a total value or in an aggregated form. Additionally, data on microalgae production are almost non-existent and information is scattered and difficult to access (Vigani et al., 2015). A lack of accurate figures on the seaweed resource production has been identified as one of the main constraints to the development of the seaweed harvesting and farming industry in Europe (Mac Monagail et al., 2017) while an absence of knowledge on the production of microalgae has been recognized as a limiting factor for evaluating the potential of this sector in Europe (Vigani et al., 2015).
According to the available statistics, algae biomass production was increasing worldwide and reached 32.67 Mt [Fresh weight (FW)] in 2016 from which 0.57% of the volume was produced in Europe (including EU 27 + United Kingdom + Iceland + Norway) (Araújo et al., 2019a based on data from FAO). At the global level, algae biomass is mostly supplied by aquaculture (96.5% in 2016) while in Europe harvesting from wild stocks contributed to 98% of the total algae production volume in the same period (Araújo et al., 2019a). Significant knowledge gaps exist with this data.
In addition to the data on the production volumes by species, country and production method, other categories of information are relevant to understand the current landscape, the interplay of stakeholders and potential for growth of the algae sector in Europe. Successful stories from scattered industry players, including examples of research-industry partnerships, illustrate the potential of the sector for innovation in mitigating environmental pressures and bioprospecting and as a sustainable source for food (Blue Bioeconomy Forum, 2019). Simultaneously, challenges and constraints to the sector’s development have also been repeatedly highlighted in different forums (Araújo et al., 2019b; Blue Bioeconomy Forum, 2019). Commonly referred to examples include regulatory barriers and the complexity of the administrative procedures and regulations, the still low consumer’s awareness, the small market size, the sustainability of the production chain and the lack of credits for the environmental services provided by algae production. The access to good-quality data is also one of the gaps listed (Araújo et al., 2019b).
Objectives of the Study
This paper presents the findings from different collaborative initiatives aiming at increasing the information and knowledge on the algae sector in Europe. These findings are based on the outcomes of a workshop and the comprehensive collection of information from different sources and stakeholders. Based on the analysis of the information compiled, our research provides a comprehensive description of the algae production sector at the European level which is, to the best of our knowledge, not available elsewhere. The resulting analysis is based on original data and describes the main characteristics and geographical distribution of the algae biomass production units. The compiled information fills existing knowledge gaps in order to support the development and implementation of evidence-based initiatives and strategies for a European-wide development of the sector.
Materials and Methods
For the purpose of this study the term “algae” includes macroalgae and microalgae. The production of Spirulina will also be addressed, given that this group of organisms is frequently considered, from the industry and consumer perspective, under similar frameworks as “algae” (e.g., CEN/TC454, 2020).
Identification of Constraints and Ways Forward
A workshop to discuss the gaps and priorities of the knowledge on algae biomass in Europe was organized by JRC in collaboration with FAO and The European Cooperation in Science and Technology (COST) with the participation of stakeholders from the research, data collection and management, industry and policy sectors (Figure 1). Twenty-four attendees were divided into four discussion groups, designed to balance the expertise and working field of participants. The same four different questions regarding algae biomass data reporting were discussed by each of the 4 groups (Figure 1). The common answers to 4 and 3 of the discussion groups are presented (Table 1). The participants identified the harmonization of data reporting formats and content as the most important action to increase the quality of data on algae biomass. The availability of information about screening and characterization of new species and the support to the expansion of the EU market were identified as priority needs for the sector (Table 1).
Collection of Data
The discussions held during the workshop reinforced the need to collect additional and comprehensive information about the algae production industry in Europe (Table 1).
To fulfill this knowledge gap, the algae producers in Europe (EU 27 + United Kingdom + EEA countries + Switzerland) were mapped and different categories of information collected. Only companies with production units in Europe were considered. Algae companies were considered as producers even if additionally carrying out other activities (e.g., biomass processing or research).
For the European macro- and microalgae producers the list was initially based on the information provided by the European Algae Biomass Association (EABA), which was revised and further developed with data and information collected from different sources. At the first stage, information was gathered from websites, reports, meetings with stakeholders and expert consultation. The information from the identified companies was verified through desktop research into their websites or through direct contact via email or phone. Further information on their operational status, location of the production facilities and production methods were also requested. From this exercise, a first list of producing companies was obtained with three categories of data: Level 1: Companies that confirmed the information through contact; Level 2: Companies that did not reply to the contact but with web information of sufficient quality to be included in the database; Level 3: Companies that did not reply to the contact and for which the information retrieved from the website was not of sufficient quality to be included in the database. A second round of web research was conducted and 25 of the investigated companies were identified as “not biomass producers.” A consultation involving experts from 11 algae producing countries in Europe was carried out to review the identified list of producers critically. A final list of 225 producers was obtained and the companies on this list contacted to participate in an online survey collecting information on the following topics: geographical location of the production units, organism produced (macroalgae or microalgae), production method, year of establishment of the company, identity of the species produced and commercial uses of the produced biomass. A response rate of 40% was obtained in this survey. For the remaining companies (Level 2 companies) the information was obtained from the web or through direct contact with individual stakeholders. The analysis presented in this study corresponds to the information collected from Level 1 and Level 2 companies which is equivalent to 90% of the companies identified as algae biomass producers in Europe.
For Spirulina, the information was collected from the lists of EABA and the Fédération des Spiruliniers de France. The combined list was completed with information coming from the JRC microalgae database (several companies produce both organisms), the web and consultations with stakeholders.
This data was organized in a database, stored by JRC and used as underlying information source for the analyses conducted on the current status of the algae production sector in Europe.
Data Visualization
The most relevant data from the JRC database were visualized and made publicly available through two platforms:
– An algae industry directory hosted by the European Marine Observation and Data Network (EMODnet) Human Activities Portal1 : algae companies are displayed in the EMODnet mapping based on the information on location of the production unit, group of organism and species produced, and production method.
– Country dashboards: as part of the efforts to gather, synthesize and disseminate the large and scattered information on the bioeconomy, the European Commission’s Knowledge Centre for Bioeconomy is producing and populating the bioeconomy country dashboard with bioeconomy relevant data and information at the national level. Thanks to the JRC Algae Database, the dashboard now also shows data on the European algae sector2.
Results and Discussion: Current Status of the Algae Production Sector in Europe
According to the results of this study, the European algae sector amounts to 225 macroalgae (67%) and microalgae (33%) producing companies. Additionally, 222 Spirulina producers were identified, 15% of which are also microalgae producers (and included in the microalgae accounting), spread between 23 countries (Figures 2, 3). Spain, France and Ireland support the largest number of macro- and microalgae companies followed by Norway, the United Kingdom, Germany and Portugal (Figures 2, 4). The largest seaweed biomass volumes in Europe are produced by Norway, France and Ireland (Araújo et al., 2019a).
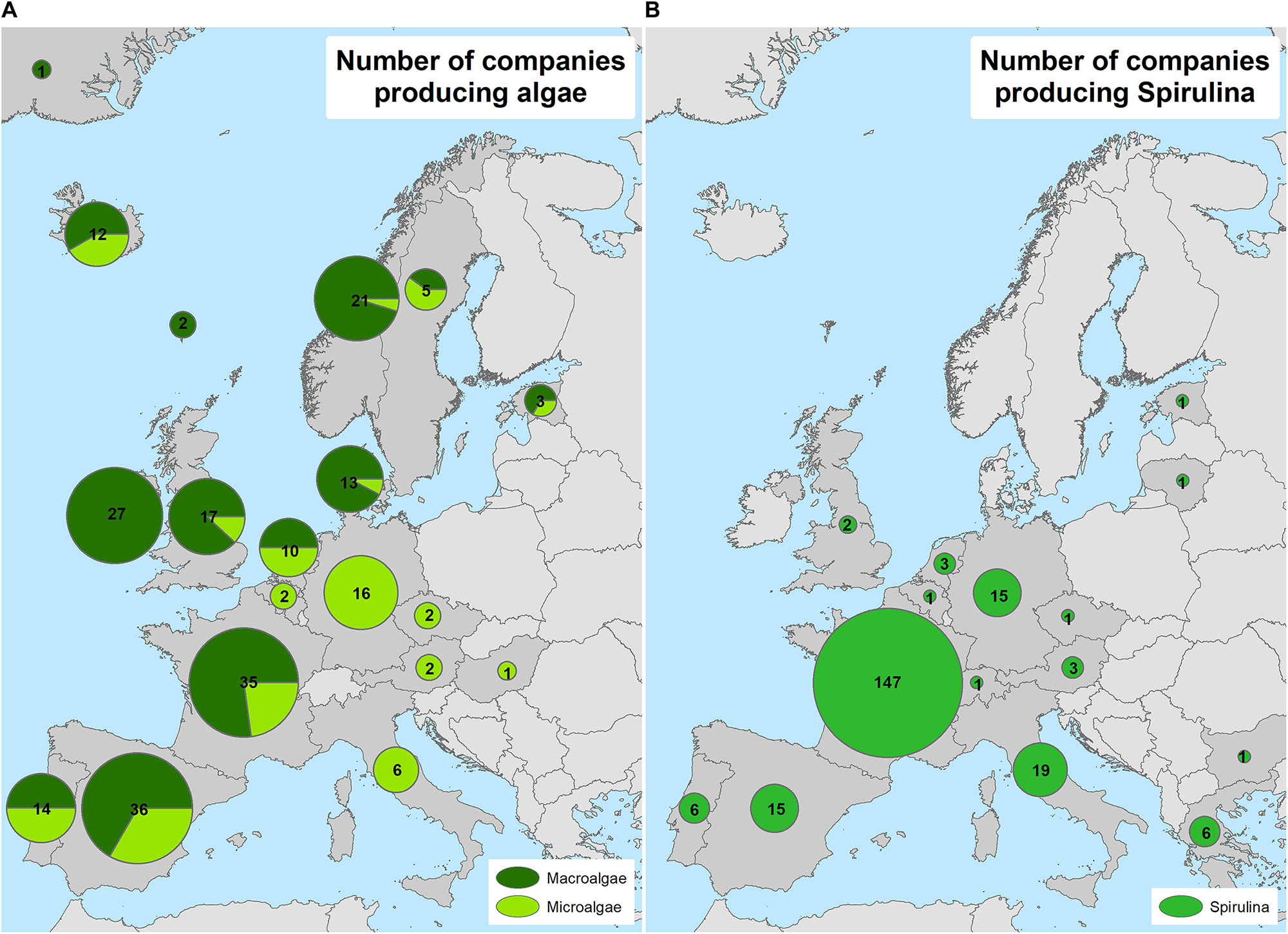
Figure 2. Number and relative distribution between macro- and microalgae (A) and Spirulina (B) production companies by country.
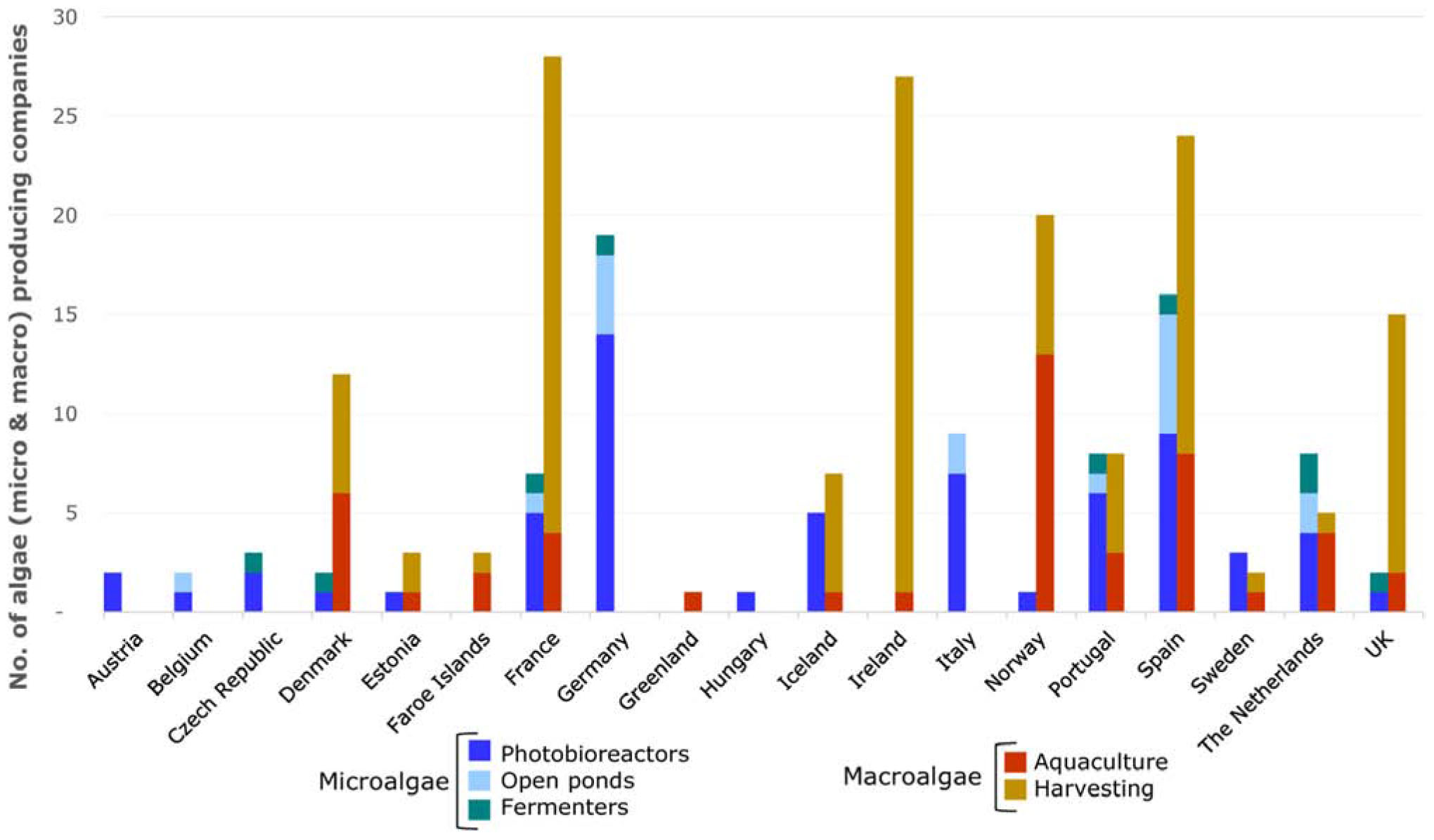
Figure 4. Numbers of macro- and microalgae producing companies in Europe broken down by production technology and country.
Information about the starting year of production activities was retrieved for 215 companies. When solely considering the new companies starting the activity in the last decade an increase of 150% in the number of new algae producing companies was registered and 10 of the current companies were already operating before 1980 (Figure 5). This trend evidences the existing interest in the development of this sector in Europe and demonstrates the temporal stability of some of the companies. For less than 10% of these companies the algae production is, however, not the main business activity.
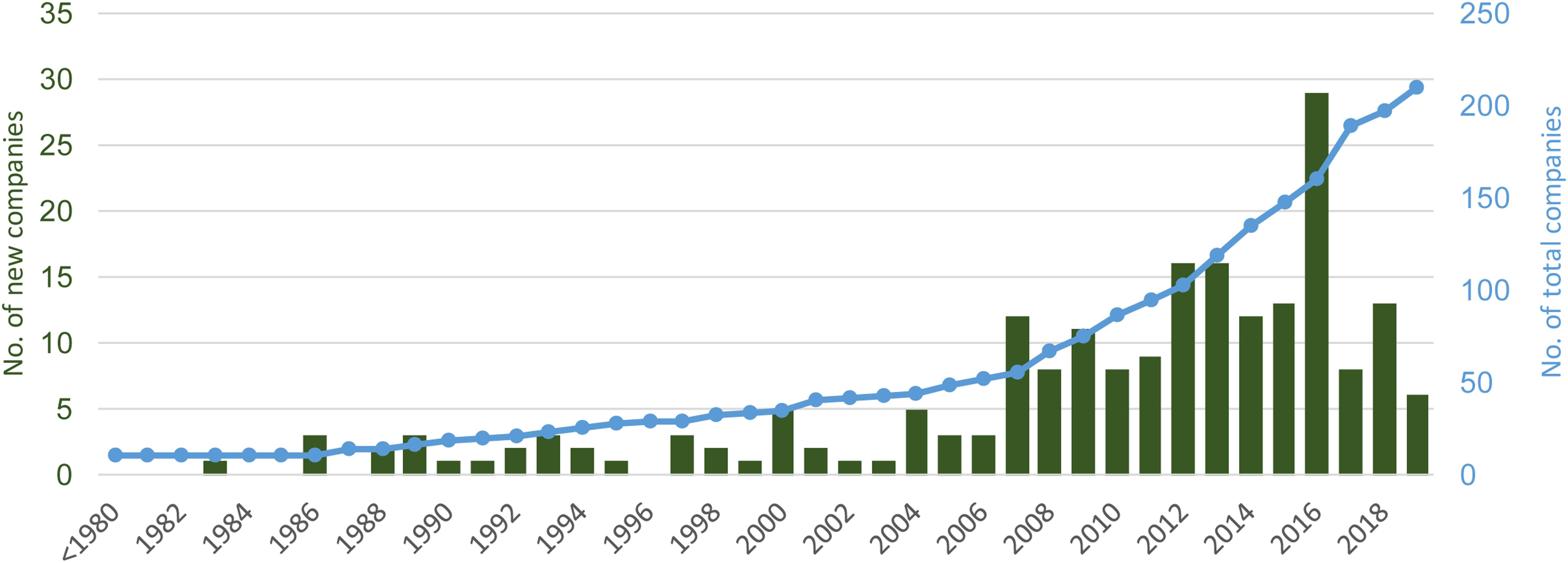
Figure 5. Number of algae producing companies currently operating in Europe (starting activity since 1926). The values shown represent the number (left axis) and the accumulated (right axis) number of companies per year from the companies currently active.
The increasing temporal trend in the number of new algae production companies established since 1926 shows both the dynamics and the potential for growth of the sector. This trend needs to be critically analyzed since many other companies have closed or diverted their activities over the years.
Different studies have highlighted the potential of algae biomass production as an economically sustainable activity in the European bio-based industry landscape (Buschmann et al., 2017; Hasselström et al., 2018). Several constraints, however, still limit the sector expansion, the primary constraints being, but not limited to, the small market size for algae commodities in Europe, the variability in the annual biomass supply, the current state of technological development in the production and processing of biomass. The complexity and/or inexistence of some EU and national regulations in aspects such as cultivation licenses as well as definition of threshold values and measuring variables for harmful metal in food and the lack of harmonization of guidelines for organic certification between countries represent also a burden (Hasselström et al., 2018; Araújo et al., 2019b; Barbier et al., 2020).
MACROALGAE
Spain, France, and Ireland are the countries in Europe with the largest number of macroalgae companies (more than 20 producers each) (Figure 2). Macroalgae production is being developed in a total of 13 countries with Norway, the United Kingdom and Denmark having more than 10 production units. The activities connected to the seaweed production represent an essential livelihood and source of income for some coastal and rural communities and are part of a cultural heritage that is important to preserve (Frangoudes, 2011; Mac Monagail et al., 2017). In some European coastal areas, seaweeds were traditionally gathered by coastal communities for centuries for food, cattle feed and fertilizer and from around the middle of the 20th century, were also used for hydrocolloids extraction (García Tasende and Rodríguez González, 2003; Frangoudes, 2011; Guiry and Morrisson, 2013; Mouritsen et al., 2013; García Tasende and Peteiro, 2015; Peteiro, 2018). Currently, the market for seaweed biomass is much more diversified and the technologies for biomass gathering more advanced. Yet, in some European regions, seaweed gathering still represents a family activity passed on for generations (Alban et al., 2011).
Production Methods
Seaweed production in Europe (considering both harvesting from wild stocks and aquaculture) is primarily concentrated in the Atlantic region with very few companies producing macroalgae in the Mediterranean area (Figure 3A). This is related to the geographical distribution, the larger extension of the intertidal area and a higher abundance and dimension of seaweed species traditionally exploited at an industrial scale on the Atlantic coasts. These factors historically facilitated the expansion of an algae industry based on the mechanical and manual harvesting of wild resources. Some companies are cultivating species that are native to the Mediterranean (e.g., Ulva sp., Gracilaria sp.) but these units correspond to a minority of the European production (Figure 3A). Some initiatives at the European and regional level are now trying to identify the factors limiting the expansion of this activity in the Mediterranean and discussing measures that could promote the cultivation of seaweeds in this area.
(a) Harvesting From Wild Stocks
As anticipated by the data on production volumes (Araújo et al., 2019a), harvesting from wild stocks is the primary production method for macroalgae in Europe, and this has not changed appreciably over the past two decades (Araújo et al., 2019a). Harvesting is the production technology used by 68% of the macroalgae production units (excluding pilot plants) covering 11 European countries (Figures 4, 6). Among these, 85% of the producers harvest the biomass by hand (Figure 6). Mechanical harvesting is usually carried out by companies running a fleet of vessels. Hence, mechanical harvesting, although representing only 15% of the production units (Figure 6), presents a greater potential in terms of biomass removal compared to manual harvesting. Spain, France and Ireland are the countries with the highest number of seaweed harvesting companies (Figure 4). With exception of Denmark, Norway, and Netherlands, harvesting (either manual or mechanical) is the dominant method for all the macroalgae producing countries when considering the number of production units (Figure 4). Countries along the Atlantic coastline, like Ireland and Spain, have a long tradition for hand harvesting of seaweeds (García Tasende and Rodríguez González, 2003; García Tasende and Peteiro, 2015; Mac Monagail and Morrison, 2020). Although used by only a minority of the harvesting companies in Europe, mechanical harvesting potentially corresponds to higher incomes and removal of larger algal volumes (Alban et al., 2004). The species collected by each harvesting method differ with only a few species being harvested mechanically (Frangoudes, 2011; Rebours et al., 2014).
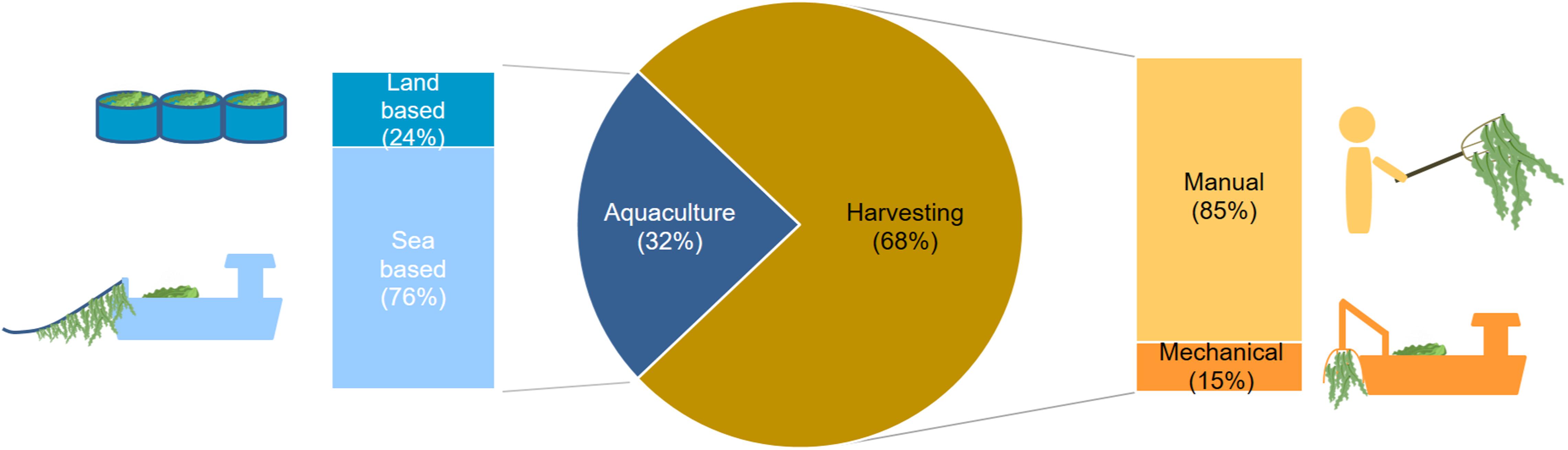
Figure 6. Macroalgae production methods in Europe (share by the number of companies using these methods).
In France, seaweed biomass is harvested by hand and mechanically from boats (using scoubidou devices or large rake-like implements, depending on the target species) (Davoult et al., 2011; Frangoudes, 2011). In Brittany, the most critical seaweed production region in France (Figure 3A), kelp species harvesting is traditionally carried out by a fleet of small vessels operating seasonally. Laminaria digitata is traditionally harvested from May to September following quotas established by management plans. These plans are established in accordance to the available annual biomass and regulate the number of vessels by harvesting area, the starting period of the harvesting season and the number of harvesting days per week. Similarly, the harvesting of L. hyperborea is also managed by quotas established for areas which open every 3 years. These areas are harvested between the beginning of September and mid-May, but the autumn biomass removals represent 75 to 80% of the total annual volume of harvested algae biomass. Recently all the fleet was equipped with Vessel Monitoring Systems (VMS) which will improve harvesting management practices. During the winter, some vessels target shellfish, but the main activity of this fleet is kelp harvesting (Alban et al., 2011). Maerl was harvested mechanically in many parts of Brittany using a “sablier” suction dredge (Mac Monagail and Morrison, 2020), but this activity is banned since 2011 in France. In large parts of the coast, particularly in Brittany, seaweed is harvested by hand (a traditional economic activity running for more than 300 years). Fucus sp., A. nodosum, and Chondrus crispus are the most harvested species followed by an extensive list of edible species e.g., Palmaria palmata, Porphyra sp., Ulva sp. or Himanthalia elongata. The harvesting of these edible species is a recent activity but the economic importance of this sector is increasing over time.
On the Atlantic coast of Spain, seaweeds with economic interest [mainly edible species such as Undaria pinnatifida, L. ochroleuca, Ulva spp., H. elongata but also C. crispus, and Mastocarpus stellatus which are used for the extraction of carrageenans (García Tasende et al., 2012, 2013)] are harvested manually from shore, on foot, during low tides or from vessels by diving (García Tasende and Rodríguez González, 2003; García Tasende and Peteiro, 2015). The agarophyte G. corneum, exploited on the Cantabrian coast (Asturias, Cantabria, and Basque Country), is traditionally collected from beach-cast, and more recently by harvesting using hand-plucking or cutting. Beach-cast Gelidium biomass is harvested with the traditional use of rakes and forks or more recently with buck rakes operated with tractor, while the harvesting of the subtidal Gelidium beds is carried out by diving from a vessel (Juanes and Sosa, 1998).
Norway is the number one seaweed biomass producer (in terms of volume) in Europe (Araújo et al., 2019a) mostly by mechanical harvest of kelp and Fucales. A limited number of other species such as Ulva spp., Porphyra spp., and P. palmata are also handpicked for high-end users such as restaurants in the recent decades. The main volumes are harvested with the use of seaweed trawlers and mechanical cutting, an activity that started already in the 1960s and targets two main species: L. hyperborea and A. nodosum (Vea and Ask, 2011; Mac Monagail et al., 2017). The same two species are harvested by hand in Ireland using traditional techniques that differ among regions (Mac Monagail and Morrison, 2020).
In Ireland, new harvesting methods have been introduced since 2015 in particular the use of rakes from boats for A. nodosum harvesting and the use of mechanical harvesters for removing kelp biomass (Mac Monagail and Morrison, 2020).
In Iceland mechanical harvesters equipped with adjustable rotating cutting blades are used to collect A. nodosum (Mac Monagail and Morrison, 2020) while Lithothamnion sp. is harvested using a vessel that pumps the algae onto the shore where it is then further processed and dried.
In countries like Portugal or Estonia, few companies harvest seaweeds. The harvested biomass is restricted to a small diversity of species (Gelidium sp. in Portugal and Furcellaria lumbricalis in Estonia) which is used as a feedstock for hydrocolloid production.
In the United Kingdom and Denmark manual harvesting is also the primary harvesting method, although mechanical harvesting of Ulva sp. green tides is being developed.
Harvesting of seaweed wild stocks faces the challenge of balancing the socio-economic and environmental sustainability of the activity. The sustainability of harvesting practices has been under debate in several regions (Callaway, 2015; García Tasende and Peteiro, 2015; Marine Scotland Directorate, 2016; Mac Monagail et al., 2017; Burrows et al., 2018). Strict management plans for harvested resources are frequently put in place by the regional authorities, harvesters’ associations or harvesting companies (García Tasende and Rodríguez González, 2003; Frangoudes, 2011). The recent trend showing a reduction in the abundance of some of the harvested species in some coastal areas (Araújo et al., 2016; Krumhansl et al., 2016; Filbee-Dexter and Wernberg, 2018; Casado-Amezúa et al., 2019; Wilson et al., 2019) raises additional concerns on the sustainability of harvesting from wild stocks, which potentially has an impact on the natural communities (Lorentsen et al., 2010; Davoult et al., 2011). Management plans and monitoring programs should take into consideration the harvesting methods, species identity and geographical location of the harvested resources (García Tasende and Peteiro, 2015; Burrows et al., 2018; Lotze et al., 2019).
(b) Aquaculture
Aquaculture production of macroalgae, presently ongoing in 13 European countries, is at an early stage of development in Europe in terms of production volumes and number of production units (Figure 4). According to the official statistics, seaweed aquaculture production contributes to less than 1% of the total European seaweed biomass production (Araújo et al., 2019a), accounting in this study for 32% of the mapped macroalgae production units (Figure 6). Most of the production units are located at sea (offshore or in coastal waters) with only 24% of the companies conducting land-based activities (Figure 6).
Seaweed aquaculture is seen as a possible way to meet the increasing demand from the processing industry for traceable, high quality and predictable yields of biomass. Furthermore, it is widely recognized that a transition from wild stock seaweed harvesting to aquaculture is needed to meet the growing demand while avoiding the overexploitation of wild seaweed resources. Moreover, there has been increasing interest in environmentally friendly farming practices where macroalgae will play a key role in the emerging development of integrated multi-trophic aquaculture (IMTA), which is currently promoted at both European and national level (Alexander et al., 2016; Buck et al., 2018; Kleitou et al., 2018). In Europe, Norway is the country with the highest number of seaweed aquaculture companies (Figure 4). This is a result of the recent Norwegian strategy encouraging research institutions, industries and public authorities to develop a bioeconomy based on the production and processing of cultivated seaweeds (Stévant et al., 2017). The first licenses for seaweed cultivation at sea in Norway were granted in 2014. In 2019 the surface allocated to seaweed cultivation was 834 ha, which corresponds to a production potential of approximately 48,000 tons (FW), with a registered production of 111 tons for a value of about EUR 0.43 million (Directorate of Fisheries, 2020). The estimated potential has not been reached yet since only part of the companies holding a permit are currently in operation and most have still reduced production capacity (Broch et al., 2019). Although a large-scale cultivation is not yet a reality, the Norwegian coast has the optimal conditions to expand further the area and location of the cultivation sites (Stévant et al., 2017).
Other countries, like Denmark, currently have an equal share of aquaculture and harvesting companies (Figure 4), with commercial companies dedicated to large-scale kelp production as part of IMTA since 2012 (Marinho et al., 2015).
In countries like France, Spain, and Portugal some aquaculture companies are already established at a fully commercial scale with sea-based (coastal) or land-based production facilities. In Spain, the development of commercial-scale aquaculture of edible kelp species has been receiving increasing interest since the beginning of the 2000s (Peteiro et al., 2016 and references therein). This attention boosted the development of commercial sea farming initiatives for two seaweed species: Saccharina latissima, and U. pinnatifida, the latter being banned by the national environmental regulation on alien invasive species. Currently there are some licenses granted (at least four authorizations) for the commercial cultivation of S. latissima at sea- and land-based systems. Although the number of licenses is still small, it is expected that seaweed aquaculture will grow significantly during the coming years, particularly the cultivation of species with high demand and high commercial value and for which the extraction from wild stocks is not currently authorized due to environmental protective measures (e.g., for S. latissima and P. palmata) or the low availability of wild biomass (e.g., Porphyra sensu lato). Other seaweed species such as Ulva spp. and Gracilaria/Gracilariopsis spp. are also currently cultivated on land-based tanks. Other countries with cultivation facilities are Estonia, Faroe Islands, Iceland, Ireland, Sweden, the Netherlands, and the United Kingdom (Figure 4).
Different cultivation techniques are in use depending on the target species and available conditions. Each cultivation technology has its own advantages and challenges (Barbier et al., 2019).
Land-based cultivation involves higher availability of land space, and it is associated with high infrastructural and operational costs. It is the most suitable method for certain species and for high-value applications (e.g., functional products for human consumption, cosmetics, and pharmaceutics). Land-based cultivation offers the possibility of producing biomass in a more controlled environment obtaining a more consistent supply throughout the year and thus better control of the biomass quality, standardization, traceability, security, and composition (Hafting et al., 2012, 2015; Pereira et al., 2013; Barbier et al., 2019).
Sea-based cultivation, which presents a higher potential for scaling up the production volumes, is the dominating production method for kelp species. However, it results in more variable yields, less control of the quality of the biomass (Titlyanov and Titlyanova, 2010), higher risks of diseases and pests (Ward et al., 2020) and vulnerability to environmental conditions (e.g., major storms, torrential rains) (Peteiro et al., 2014). Adequate site-selection of the production facilities to guarantee the suitable environmental conditions for biomass growth and cultivation is also challenging (Bruhn et al., 2016; Peteiro et al., 2016; Barbier et al., 2019; Visch et al., 2020a). Most of the current sea-based seaweed cultivation systems in the world are located in coastal areas and shallow oceans (Buschmann et al., 2017).
Offshore aquaculture is estimated to have the advantage of reducing conflicts in the use of space and consequently has a higher potential for upscaling of the production facilities (Barbier et al., 2019). In some regions, offshore cultivation also gives a higher guarantee of stable and suitable environmental conditions (Broch et al., 2019). The main challenge to this production method is the need to develop cultivation methods and innovative solutions to withstand the forces of offshore seas, to reduce the currently high infrastructural and logistics costs associated with offshore operations and to increase the biomass yields (Buck and Buchholz, 2004; Peteiro et al., 2014; Zuniga-Jara et al., 2016; Bak et al., 2018; Buck et al., 2018). At present, innovative projects seek for the development of new techniques that may increase the profitability of this production method (Bak et al., 2018) and for the design of multifunctional structures combining other economic activities, for example wind farms with seaweed aquaculture facilities (Jansen et al., 2016; Buck and Grote, 2018; van den Burg et al., 2020). Offshore production still represents a minority of the aquaculture units in Europe, but cultivation systems adapted to highly exposed seas have been developed and tested for several years at the Faroe Islands (Bak et al., 2018). Increased modeling complexity and accuracy will support aquaculture development. Recent modeling studies show spatial variations in the cultivation potential of S. latissima in Norway (Broch et al., 2019), the role of environmental nutrient concentrations in the biochemical composition of algae biomass (van der Molen et al., 2018) and the growth dynamics of S. latissima with variable environmental parameters (Venolia et al., 2020).
Integrated Multi-Trophic Aquaculture (IMTA) systems, already used by approximately 10% of the European aquaculture companies, may represent an opportunity to increase the economic and environmental sustainability of the production of all the involved cultures. The IMTA concept is based on the co-cultivation of species from different trophic levels (2 or more) (Figure 7) and is regarded as a potential mitigation approach, reducing the nutrients and organic matter inputs from finfish aquaculture (e.g., Chopin et al., 2001; Neori et al., 2004; Troell et al., 2009; Buck et al., 2018). In some coastal areas, such as Norway, the ubiquitous presence of fish aquaculture units is regarded as an opportunity to develop a production system based on the co-cultivation of fish and seaweed, the latter benefiting from the nutrient-enriched effluent waters from fish aquaculture (e.g., Handå et al., 2013; Marinho et al., 2015; Stévant et al., 2017) (see Figure 7). This is similar to other aquaculture practices in north-western Spain (Galicia) where commercial kelp farming is developed in culture areas with a high density of mussel floating rafts (Peteiro and Freire, 2013; Peteiro et al., 2016).
Current efforts to upscale the industrial production of seaweeds have focused on implementing efficient farming strategies and developing technologies to optimize the cultivar production and deployment, biomass harvest and crop handling logistics (Peteiro et al., 2019; Stévant, 2019; Goecke et al., 2020). Innovation in automation of processes during seeding, biomass monitoring, harvesting and processing is needed to improve economic sustainability. Recent developments in this field include automated evaluation of biomass density in land-based systems through spectral reflectance imagery (Praeger et al., 2020) or the use of intelligent management systems in IMTA by using underwater drones for biomass monitoring at sea (IMPAQT project3). The development of the sector will also greatly depend on the increasing demand for certain species and the expansion of specific market segments. Production of species currently with high demand but low biomass supply (e.g., P. palmata, Porphyra spp., Ulva spp.) needs to be increased (Barbier et al., 2019).
The aquaculture sector still faces negative public perception related to the management of space and the social acceptability of the aquaculture practices and products. Several additional challenges also need to be solved: control of pests, diseases, genetic contamination, selection of strains, fundamental knowledge on different aspects of seaweed biology and physiology, threats from climate change and herbivory as well as an understanding of the impact of aquaculture in the surrounding ecosystems (Buschmann et al., 2017; Stévant et al., 2017; Campbell et al., 2019). Recently, the environmental risks associated with seaweed farming have been assessed on various ways in different environments (Cabral et al., 2016; de Carvalho et al., 2017; Walls et al., 2017; Hasselström et al., 2018; Visch et al., 2020b). Biomass production from seaweeds at the global level is still far from being comparable to land-based agriculture. However, recent exploratory studies have shown promising resource efficiencies from aquaculture production (Taelman et al., 2015), which highlights the potential of seaweed aquaculture to contribute to solving societal challenges such as climate change (Duarte et al., 2017; Philis et al., 2018), eutrophication of coastal waters (Duarte and Krause-Jensen, 2018) and well-being of local communities including women empowerment (Rebours et al., 2014). Given the limitations to the increase in food production from agricultural systems, aquaculture contribution to global food security is expected to progressively increase. This development will entail additional challenges to the interplay of the aquaculture’s multiple sustainability dimensions (Loureiro et al., 2015; Cottier-Cook et al., 2016; Buschmann et al., 2017).
Species Produced
Although there are many seaweed species distributed in European waters [estimated to be around 1700 species (Costello et al., 2006)], only a small part of these are commercially exploited (Mac Monagail and Morrison, 2020) and, for many of them, only in some coastal areas.
(a) Aquaculture
Seaweed aquaculture production is currently limited to a reduced number of species from the total which are commercially exploited in Europe. From the available data, the seaweed volumes produced by aquaculture represent a minor fraction of the wild seaweed stock harvested. Figure 8 shows the share in the number of companies producing each species in Europe. For some of the species the combined yearly production volumes at the European level are presented (when available). Saccharina latissima is the most produced species by aquaculture (both considering production volume and number of companies) with an estimated annual production in Europe of 376 tons (FW) (Figure 8). This species has been the main target in the large-scale sea-based cultivation systems in Europe due to several reasons: broad geographical distribution (Muller et al., 2009), early availability of kelp production protocols (Edwards and Watson, 2011; Yarish et al., 2017; Forbord et al., 2018; Peteiro, 2018), the potential for higher biomass yields (Kraan, 2010; Peteiro et al., 2016) and rich nutritional content for human food and animal feed (Schiener et al., 2015, 2016). According to the number of seaweed producing companies by target species (Figure 8), Alaria esculenta, Ulva spp., Laminaria sp. (mainly L. digitata) and P. palmata are also important species in the European aquaculture sector. Yet, comprehensive data on volumes produced for certain seaweed species are not available (Figure 8). Other examples of cultivated species in European waters are Asparagopsis sp., Codium sp., Gracilaria sp., Gracilariopsis longissima, Porphyra spp., and Undaria sp.
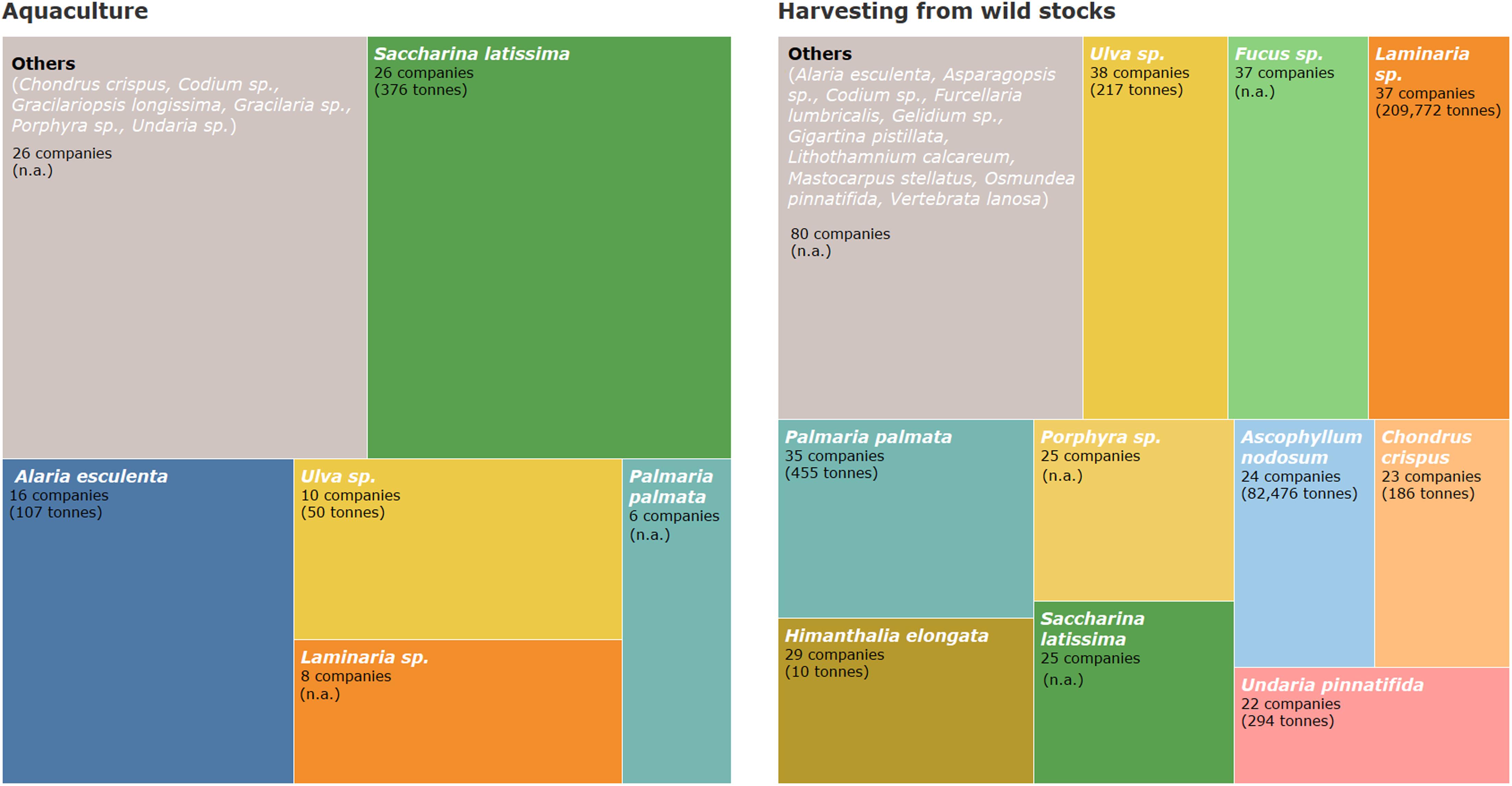
Figure 8. Share of number of companies by macroalgae species produced in aquaculture and wild stock harvesting systems in Europe. Examples of the produced species included in the category “Others” are given. Production volumes per year by species are given whenever available (all numbers are in fresh weight). Volumes of production are estimated based on FAO (2020), national and regional statistics authorities and information from the industry.
In Norway, at sea licenses for cultivation of P. palmata, Ulva spp., L. digitata and Porphyra spp. have been issued but these units are still not operational (Stévant et al., 2017). In Ireland there is a commercial demand from other industries for the cultivated biomass of P. palmata and L. digitata and there are currently 17 applications submitted to cultivate a variety of seaweed species including A. esculenta, S. latissima, L. digitata, P. palmata, Porphyra spp., and M. stellatus (Mac Monagail and Morrison, 2020).
(b) Harvesting From Wild Stocks
Harvesting from wild stocks targets a much wider variety of species in comparison to seaweed aquaculture and the volumes produced are also higher (Figure 8). The most collected seaweed in Europe in terms of volume is Laminaria spp. (including L. hyperborea, L. digitata, and a very small proportion of L. ochroleuca), with more than 0.2 Mt of harvested biomass. L. digitata is the main exploited species in France where it is collected seasonally (Alban et al., 2011; Davoult et al., 2011), while L. hyperborea is the main species exploited in areas such as Norway where it is harvested all year-round (Frangoudes, 2011; Vea and Ask, 2011). Laminaria hyperborea and L. digitata are also harvested to a lower extent in Ireland (Mac Monagail and Morrison, 2020), and in Iceland, respectively. Laminaria ochroleuca is harvested in Spain (Galicia), where species such as L. hyperborea and S. latissima are no longer approved by the regional seaweed exploitation plans (García Tasende and Peteiro, 2015).
Ascophyllum nodosum is harvested in Europe at an annual rate of approximately 0.1 Mt (FW) per year (Figure 8), being one of the main species traditionally collected in countries like Ireland, Iceland, and Norway (Frangoudes, 2011; Mac Monagail and Morrison, 2020) and, to a lesser extent, in France.
A variety of other species are collected in different countries but contribute with much smaller biomass volumes (Figure 8). This is related to the commercial interest of the species as well as to the morphology and the commercial applications of the produced biomass. Species like Porphyra spp., Ulva spp. or P. palmata have much lower individual biomass than kelp species or fucoids like Laminaria spp., A. nodosum and Fucus spp. Additionally, as a result of the natural availability of stocks, species like A. nodosum or Laminaria spp. are primarily used for low-value, high volume applications like hydrocolloid extraction and fertilizers. Seaweed biomass used for food or the extraction of high added-value bioactive compounds is needed in much smaller quantities but represents a higher value per unit of biomass (Hafting et al., 2015). Another critical factor is that the production of some species is limited to particular geographic regions. This is the case of the harvesting of agarophytes (G. corneum and Pterocladiella capillacea) in the Iberian Peninsula (center-south of Portugal, Azores archipelago and the north of Spain) which accounted for approximately 5000 tons (FW) in 2018 (these values may be even higher given that data from some northern Spain regions are not available). This is also the case for the harvesting of F. lumbricalis in Estonia, which reached 60 tons (FW) in 2019 (Ilmjärv personal communication).
Biomass Applications
Most of the seaweed companies in Europe direct their biomass production to food (36%), food- related uses (15%) i.e., food supplements, nutraceuticals and hydrocolloid production and, to feed (10%), accounting for 61% of the total uses (Figure 9). Cosmetics and well-being products also contribute to a significant share of the biomass uses (17%) while all other applications (e.g., fertilizers and biostimulants) contribute individually less than 11% of the total share (Figure 9). Commercial applications such as biofuels, bioremediation or biomaterials (listed under “Others”) or pharmaceuticals have only a small share at the European scale (Figure 9). These values refer to the number of companies directing the produced biomass to each of the uses which do not necessarily reflect the volumes allocated for each application. Besides, companies that are only involved in processing are not accounted for in this work; thus, the picture may be incomplete for some of the commercial uses. This is the case of the hydrocolloids sector using Gelidium sp., Laminaria hyperborea and A. nodosum as feedstock which requires high biomass volumes. Several of these hydrocolloid producing companies are among the largest in Europe exploiting a significant share of the harvested resources (Peteiro, 2018). It is to be noted that companies sourcing biomass from outside Europe and purely transforming companies were excluded from this analysis.
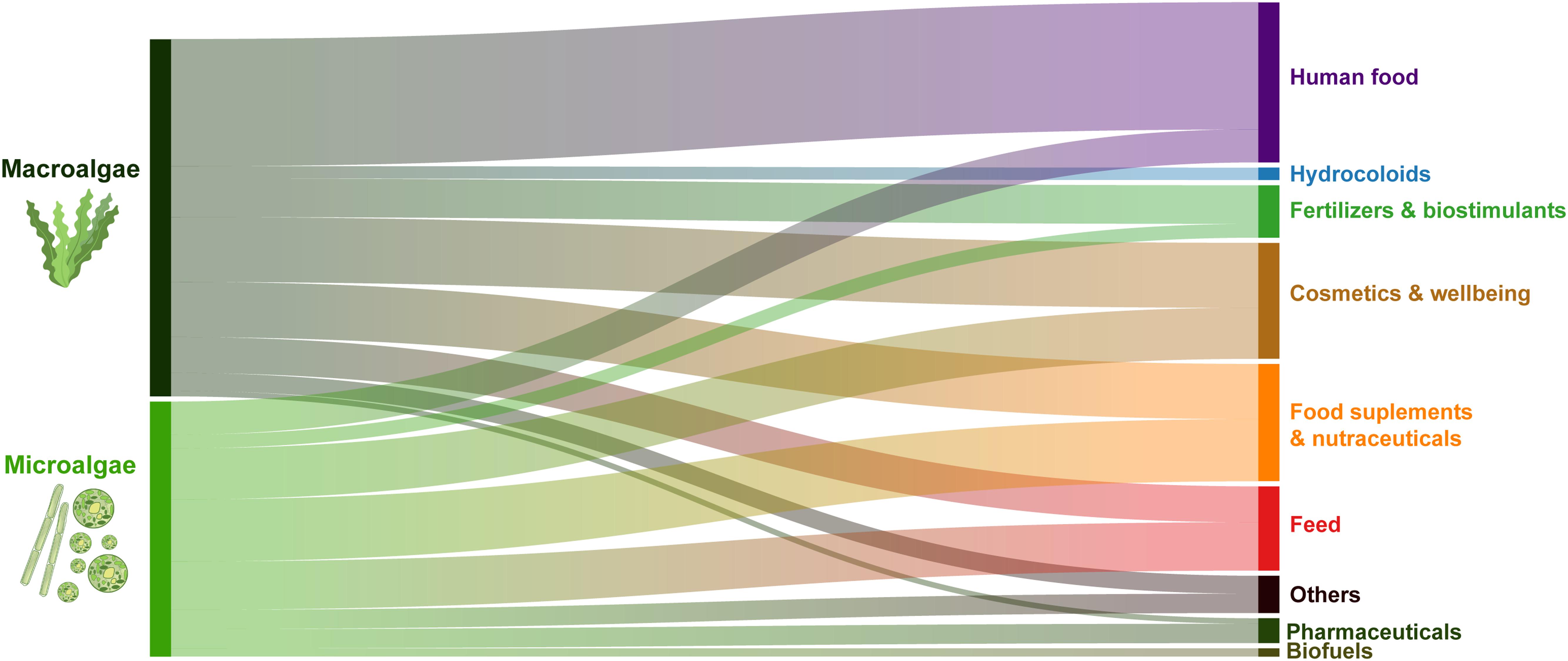
Figure 9. Share of commercial biomass applications by macroalgae and microalgae production company. These results are based on the share in the number of companies (not by volume).
Laminaria hyperborea biomass in Europe is mainly allocated to the production of extracts used for alginates, supplying 25% of the world alginates manufacturing (Frangoudes, 2011; Rebours et al., 2014; Stévant et al., 2017). The alginate industry also uses A. nodosum biomass, however, in countries like Ireland, the current production is mainly used as horticultural products (feeds, fertilizers, and biostimulants) (Guiry and Morrisson, 2013; Mac Monagail and Morrison, 2020). G. corneum, collected in Portugal and Spain, accounts for a large volume of the annual algae national production being among the top suppliers of the agar manufacturing industry (Callaway, 2015).
Food is the primary market in several companies for the seaweed biomass in Europe (Figure 9). The volumes produced are much smaller than the ones directed to hydrocolloid manufacturing but the prices per biomass unit are higher and there are many European companies specializing in selling seaweed products for the food market. Currently, several edible seaweed species are sold fresh or dried for direct consumption, used as condiments or incorporated in other formulations (e.g., dressings, sauces, canned seafood, bread, pasta, salt) in several European countries (Mouritsen et al., 2013; Le Bras et al., 2014, 2015; García Tasende and Peteiro, 2015). Some species like L. digitata, C. crispus, and P. palmata have an historical record of consumption as food (García Tasende and Rodríguez González, 2003; Mouritsen et al., 2013; Mac Monagail et al., 2017). Himanthalia elongata, harvested in Europe for centuries and used as fertilizer, food and for hydrocolloid extraction, is currently collected in France, Ireland and Spain mainly for human consumption (Stagnol et al., 2016). Alaria esculenta and S. latissima are often sold directly for human consumption or as high added-value food ingredients (Stévant et al., 2017). Palmaria palmata is considered a food delicacy in Ireland with most of the produced biomass consumed internally since the demand is higher than the supply (Mac Monagail and Morrison, 2020). The historical consumption of this species is documented in France, Iceland, and Ireland and it is currently one of the most popular species used for human consumption in Europe (Mouritsen et al., 2013). Fucus spiralis, Porphyra spp., and Osmundea pinnatifida are consumed traditionally in the Azores archipelago (Patarra et al., 2011). In Spain, other kelp species such as L. ochroleuca and U. pinnatifida and the kelp-like species Saccorhiza polyschides have also been exploited for human food since the 1990s. More recently, other edible species are also used such as Codium spp., Gigartina pistillata, Dilsea carnosa, O. pinnatifida or Nemalion helminthoides (García Tasende and Rodríguez González, 2003; García Tasende and Peteiro, 2015). Yet, in some countries such as Norway, Greece, Estonia or Portugal (except for some islands of the Azores archipelago) seaweed consumption is marginal.
The retail price of seaweed biomass used for direct consumption varies with the product formulation (dry seaweeds have usually lower prices), species identity, production method (biomass from aquaculture generally entails higher production costs) and the amount of seaweed used. The market value is generally high, i.e., on average more than 100 € kg–1 for dried and flaked seaweeds (Le Bras et al., 2015). In the current study the prices from online shops were consulted for a total of 12 dried seaweed species from 20 different companies spread by four countries. The average price was estimated at 107 € kg–1 of dry seaweed. A closer outlook to the online algae market in Spain illustrates that the edible seaweeds with the highest market value (average value on dry weight) are Porphyra spp. (commercialized as nori) [251 € kg–1 (ranging from 383 € kg1 for nori sheets and 179 € kg–1 for nori flakes)], Codium spp. (155 € kg–1), Gracilaria sp. or Gracilariopsis longissima (155 € kg–1), P. palmata (114 € kg–1), U. pinnatifida (108 € kg1), Laminaria spp. or S. latissima (88 € kg1), H. elongata (86 € kg–1), C. crispus (72 € kg–1), and Fucus spp. (25 € kg–1).
The nutritional properties of some seaweeds species make them an exciting feedstock as a source of food and food applications: the relatively high content in protein [generally higher in red and green algae (up to 44% of dry mass) than in brown algae (10–24% dry weight)] and dietary fibers (33–62% dry weight) (Holdt and Kraan, 2011; Patarra et al., 2011; Wells et al., 2016), the high-quality profiles of amino-acids (with glutamic and aspartic acids as the most abundant) (Holdt and Kraan, 2011; Maehre et al., 2014) and the high concentration of polysaccharides (e.g., laminarin, fucoidans, mannitol; 4–76% dry weight) and minerals (Cofrades et al., 2008; López-López et al., 2009; Holdt and Kraan, 2011). Seaweeds have a low lipid content (usually less than 5% dry weight) being the long chain polyunsaturated fatty acids (PUFA) and carotenoids the most relevant for nutrition (Holdt and Kraan, 2011). Seaweeds are increasingly used in the food supplements and nutraceuticals market and in cosmetics formulation. Bioactive compounds from brown algae (e.g., fucoidans, fucoxanthin, laminarin, polyphenols) and red algae (e.g., phycoerythrin) are used as colorants, antioxidants and recognized by their nutritional properties. Currently, research findings on the potential bioactive and functional properties of extracted seaweed compounds suggest positive effects on human health from its anti-bacterial, anti-fungal and anti-inflammatory activity (Holdt and Kraan, 2011; Hafting et al., 2015). However, the extraction of these high value-added substances involves specific technologies with associated high investment costs (Stévant et al., 2017). The quantification of the beneficial claims and digestibility and bioavailability pathways of the use of these products (for macro and microalgae) still needs further evidence support (Wells et al., 2016). Additionally, the role of seasonal, environmental and geographical variation in the nutritional properties of seaweeds needs to be better documented to support nutritional claims (Wells et al., 2016). Seaweed biomolecular composition can change markedly in response to seasonal variation in environmental factors such as salinity, nitrogen content and water temperature (Marinho-Soriano et al., 2006; Zhang and Thomsen, 2019).
Seaweed biomass has also been used in feed meals for marine and terrestrial organisms. Its positive role in reducing enteric methane emissions from cattle was in the spotlight as a result of recent studies (Maia et al., 2016; Roque et al., 2019; Kinley et al., 2020). Species such as A. nodosum are also extensively used as feed ingredients for livestock and pets (Frangoudes, 2011; Holdt and Kraan, 2011). Saccharina latissima has also been integrated as meal in animal feed (Stévant et al., 2017) and the use of kelp meals for abalone feed is common in some European countries (e.g., Ireland) (O’Mahoney et al., 2014). Currently, the cost and scale of producing seaweed-based protein for fish feed applications is not yet competitive with other protein sources with a high environmental footprint such as soy. The upscaling of the production and optimization of the processing methods is expected to place seaweed biomass in a better market position for feed applications in the future (Emblemsvåg et al., 2020).
MICROALGAE and SPIRULINA spp.
Germany, France and Spain host the largest number of microalgae producers in Europe (Figure 2). The same countries (together with Italy) have the largest number of Spirulina producers but France dominates the production landscape with 65% of the mapped production units in Europe (Figure 2). Microalgae and Spirulina production is located mainly on inland sites (Figure 3). From the European countries included in this study, 16 have microalgae and 15 have Spirulina production plants (Figure 2).
Production Methods
Microalgae are widely cultivated by different production methods and used in several commercial applications. Some production plants combine different production systems, e.g., photobioreactors (PBR) with fermenters or open ponds. Overall, PBR are the most common system used for microalgae production (71%), while open ponds and fermenters represent 19 and 10% of the total production units, respectively (Figure 10). For Spirulina the primary production method used is open ponds (83% of the companies) (Figure 10). All of the microalgae producing countries dominantly use PBR although some (e.g., Spain) also have a high share of production in open ponds (Figure 4). In eight out of the 16 countries producing microalgae, fermenters are also used for the biomass cultivation although the share of this method is relatively low (10%) (Figure 4).
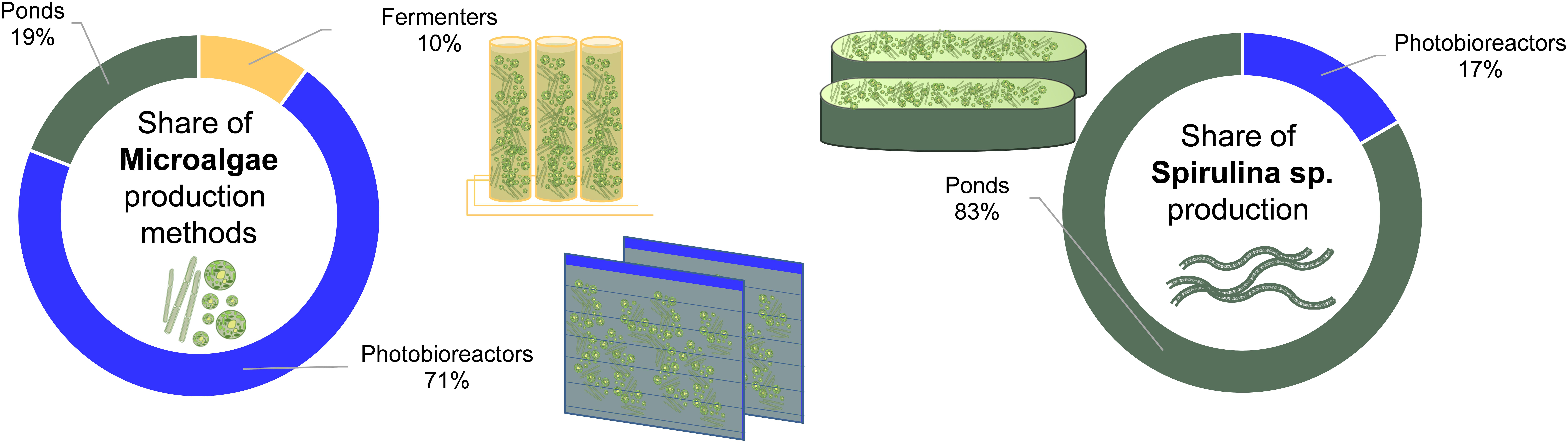
Figure 10. Microalgae and Spirulina share of production methods by production company. The category “Ponds” includes both open and semi-open ponds.
Different cultivation methods have specific features that make them more adequate for the production of certain species and commercial applications. Factors like land requirements, construction and operational costs, technological development, maintenance and control of environmental parameters vary according to the cultivation method used (Zhu, 2015).
Photobioreactors, which is the most commonly used method of microalgae production in Europe (Figure 10), have associated high investment and operating costs as well as large energy requirements. However, within this technology significant differences are dependent on the type of PBR used. The closed PBR technology offers a variety of systems – from green wall to tubular PBR systems made of glass or plastic where the tubes are installed in horizontal or vertical arrays, each of the layouts having benefits and drawbacks in terms of cost and production stability. Closed PBR is the production method used for the production of high-value low-volume products for nutraceuticals, cosmetics, and pharmaceuticals. This technology allows for stricter control of the environmental factors and biomass quality and increases the photosynthetic efficiency and productivity of the production systems (Narala et al., 2016; Acién et al., 2017).
Cultivation in open ponds involves lower investment, operational and energy costs and has the potential to produce higher biomass volumes (Narala et al., 2016). This production method is thus more commonly used in the production of biomass for low-value applications, although some technical issues still need to be solved to unfold its potential in the upscaling of algae biomass production for uses such as biofuel (Prussi et al., 2011; Narala et al., 2016; Acién et al., 2017). The disadvantages of open cultivation systems are the increased risk of contamination, lower control of the environmental conditions and greater land and water requirements (Murthy, 2011; Mayers et al., 2016; Costa et al., 2019). Additionally, most of the European countries have sub-optimal climate conditions for large-scale outdoor microalgae production (Vigani et al., 2015). In fact open ponds are the preferred method for Spirulina production in Europe (Figure 10). Actually, open ponds were the first microalgae industrial cultivation systems widely applied, and are used by 83% of the companies operating large-scale Spirulina cultivation plants. This species (together with Chlorella spp.) is the most commonly cultivated in open ponds worldwide (Mobin and Alam, 2017; Costa et al., 2019).
Hybrid systems combining open and closed cultivation methods are also used, allowing for a first cultivation step where contaminations and biomass traits are better controlled in photobioreactors with the subsequent transference of the inoculum to open ponds for increased biomass growth and lipid accumulation (Murthy, 2011).
Fermenters are currently used by a minority of the European microalgae producers (10%, Figure 10). They are a more recently developed method that allows for higher productivity and densities, control of contamination and manipulation of the biomass quality and profiles during the first growth phases of microalgae cultures (Barros et al., 2019). With this method microalgae cultures are grown heterotrophically using organic compounds such as sugars as carbon source. Production in fermenters can be combined with other methods such as autotrophic systems (photobioreactors or open ponds) since the highly concentrated inoculum obtained can be used to minimize the limitations (e.g., contamination, scalability) of these systems (Barros et al., 2019). Even if not as common as heterotrophically grown microalgae fermenters, it is also possible, although not as common, to grow algae autotrophically in fermenters by using artificial light or in mixotrophy (light and sugar). Fermentation technology has the potential for upscaling the production volumes while reducing production costs. The long-term sustainability of this production method needs to be better studied since the use of organic carbon substrates has been argued to have a higher carbon footprint than autotrophic production (Murthy, 2011).
Microalgae production has proven environmental benefits but also potential adverse impacts related to the high water and energy demand, management of wastewater, emission control, land use and risk of microbial contamination (Usher et al., 2014; Mayers et al., 2016; Béchet et al., 2017). More empirical evidence is needed to compare the environmental footprint of this production method with other feedstocks for commodities such as land-based cultures.
Phototrophic production of microalgae in any kind of pond system or photobioreactor is facing the main challenges of light limitation and a missing scalability of reactors. Hence, there is a need of technological and material innovation, a need for adapted strains as well as a rethinking about the use of waste streams (e.g., special waste waters, CO2 emissions, heat) and its impact on production costs. A global mapping of the main productions costs (energy, labor costs, water/waste water) together with climate data could help to estimate the economic efficiency of an algae farm according to the technology, scale and algae strain on a certain location. As microalgae are always produced in containment, nutrition monitoring is more feasible, but it is still in its infancy. More advanced in-line measurement and dosing devices for monitoring and adjusting the concentrations of single elements in the culture media would greatly enhance productivity as depletion of even one single component strongly decreases biomass production or reduces compound contents. Similarly, nutrition monitoring would also help to reduce production cost as well as the environmental load caused by the amount of nutrition-loaded waste water (Jaywant and Arif, 2019).
Species Produced
Only a minor share of the naturally occurring microalgae species are exploited commercially. In Europe it has been argued that the rate of exploitation of new species is also hindered by administrative burdens, namely the need for any novel species to go through the Novel Food regulation (EU, 2015) before it can be placed on the food market. The stakeholders from the algae industry claim that these procedures represent an expensive and time- consuming endeavor (Araújo et al., 2019b). Some of these species are already extensively cultivated at the industrial scale and have a well-established market (Carrasco et al., 2018).
The market value for these species at the moment is very much based on their potential as a source of high-value bioactive and functional compounds such as pigments (e.g., carotenoids such as β-carotene and fucoxanthin), anti-oxidants (e.g., astaxanthin, fucoxanthin), long-chain polyunsaturated omega-3 fatty-acids [e.g., docosahexaenoic acid (DHA), eicosapentaenoic acid (EPA)], phycobiliproteins (phycocyanin) and polysaccharides (Marcati et al., 2014; Sun et al., 2014; Mobin and Alam, 2017).
According to the results of this study, the most cultivated microalgae species in Europe in terms of the number of companies are Chlorella spp., Nannochloropsis spp., and Haematococcus pluvialis (Figure 11) together with Spirulina. These species are also reported among the most widely exploited species worldwide over the last decades for biotechnology applications (Mobin and Alam, 2017). Indeed, Chlorella and Spirulina are the most produced species in terms of dried algae volumes (Vigani et al., 2015). Other species such as Tetraselmis sp., Tisochrysis lutea (formerly Isochrysis galbana), Dunaliella salina, Phaeodactylum tricornutum, Porphyridium sp., and Scenedesmus sp. are also among the top produced species in Europe (7 or more companies) (Figure 11). Some other species such as Thalassiosira sp., Acutodesmus obliquus, and Chaetoceros muelleri show less interest from an industrial point of view (in terms of the number of companies) but they are still produced in Europe (Figure 11).
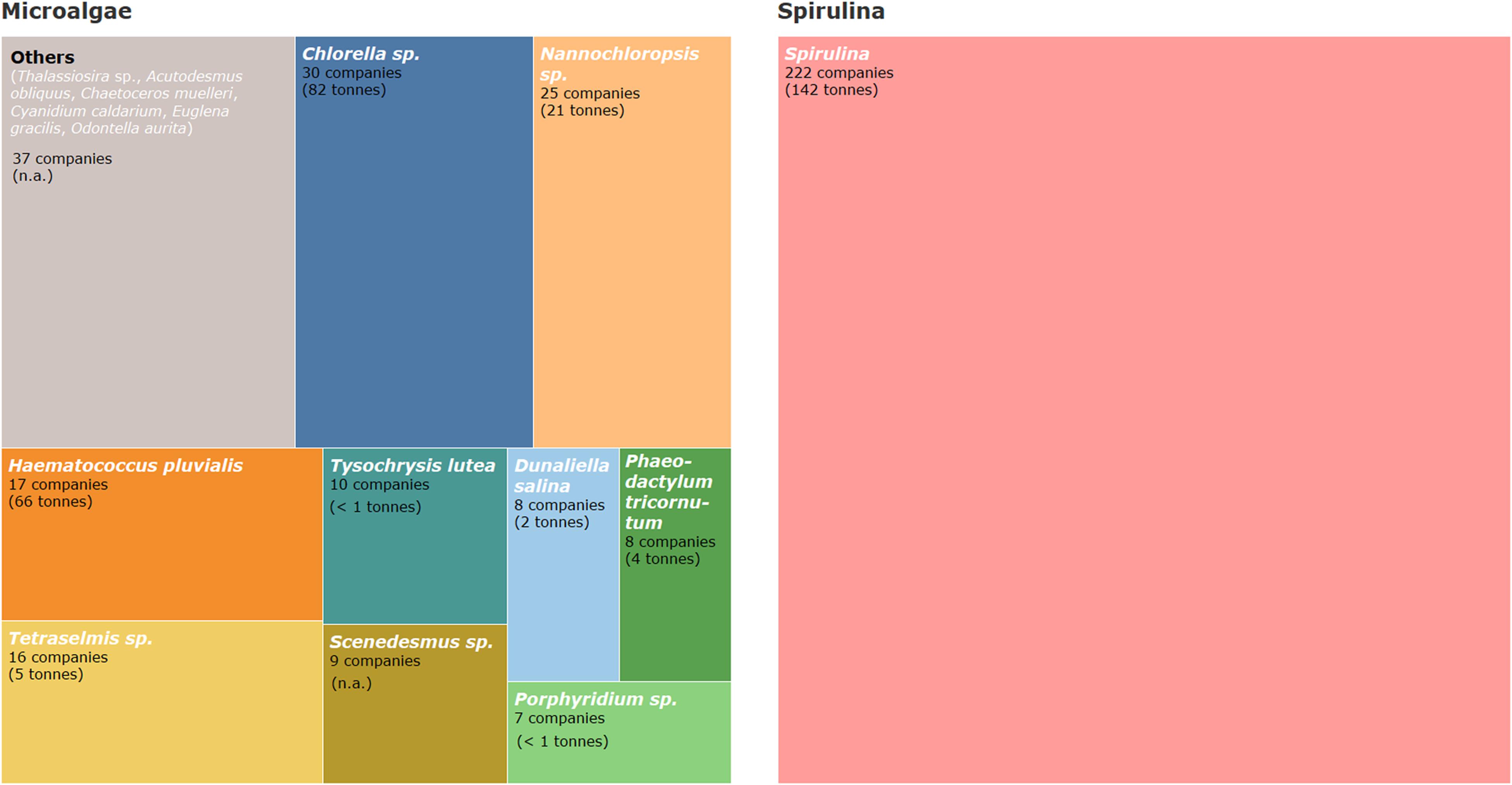
Figure 11. Share of number of companies by microalgae species and Spirulina produced in Europe. Examples of the produced species included in the category “Others” are given. Production volumes are given per year by species at the European level whenever available (all numbers are in dry weight).
Official statistics on microalgae production volumes are almost non-existent at the European scale and the data available from FAO or Eurostat are limited and fragmented. In this study several data were gathered on the production volumes at the European scale showing an approximate production of 182 tons dry weight (DW) of microalgae and 142 tons (DW) of Spirulina. Chlorella spp. and H. pluvialis are the most produced microalgae in terms of volumes, corresponding to more than 80% of the total values gathered in this study (Figure 11). This information on produced volumes needs to be taken with caution because data reported refer in many cases to estimates of production values and some countries and companies producing microalgae biomass were not included in this sum.
Biomass Applications
Microalgae species are sources of different bioactive compounds and thus used in a variety of commercial applications.
Chlorella spp. was the first species to be cultivated on an industrial scale in the 1960s in Japan. According to our study, this species is produced in 11 European countries. This species has a high protein (up to 60%) and carbohydrates content and it is also rich in B vitamins (Costa et al., 2019). Nannochloropsis sp. is an important source of EPA (Mobin and Alam, 2017). Haematococcus pluvialis has the capacity, under stressful conditions, to accumulate high quantities of astaxanthin a high-value antioxidant carotenoid. Indeed, H. pluvialis is the leading natural source of commercial astaxanthin (Mobin and Alam, 2017) which is currently the most produced carotenoid (Borowitzka, 2013). Spirulina is very rich in proteins and antioxidant compounds. It is used for the extraction of pigments such as phycocyanin, a blue photosynthetic pigment which is used in health, cosmetics and food applications (Mobin and Alam, 2017). Dunaliella salina is an excellent natural source of the antioxidant β-carotene (Borowitzka, 1999; Mobin and Alam, 2017; Costa et al., 2019). Porphyridium sp. is used for the extraction of exopolysaccharides and pigments (phycoerythrin).
Food supplements and nutraceuticals (24%), cosmetics (24%), and feed (19%) are the main applications of microalgae biomass, contributing together to 67% of the total uses (Figure 9).
Spirulina production is mainly directed to food and food supplements and nutraceuticals, contributing to 75% of the reported uses (data not shown).
Although industrial microalgae production was already established a long time ago the high production costs and technological constraints as well as gaps in the scientific understanding of large scale algae cultivation, limit the commercialization of the biomass as high-value products excluding large scale low-cost applications such as fuel (Caporgno and Mathys, 2018; Kiesenhofer and Fluch, 2018; Barros et al., 2019).
Microalgae biomass from species such as Chlorella spp. and Spirulina has historically been used in the food industry as dietary supplements providing vitamins, highly digestive proteins, polysaccharides and lipids (e.g., PUFA) (Stanic-Vucinic et al., 2018). It is estimated that these 2 groups have up to 70% of protein content (Wells et al., 2016; Niccolai et al., 2019) and a well-balanced amino acid profile (Becker, 2007). Spirulina is also regarded as a good source of vitamins, macro-and micro-elements essential fatty acids (Stanic-Vucinic et al., 2018). The use of microalgae as a sustainable alternative to animal-based food to fulfill the proteins demand of the growing global population has been discussed in recent studies (Caporgno and Mathys, 2018). These and other species such as Dunaliella sp. and H. pluvialis can be used as a source of nutrients and high-value extracts by adding them to food products and feed and increase their nutritional value. They can also be used as natural colorants in a variety of food products (e.g., bread, pasta, cookies, ice cream, candy, and beverages).
Some species (e.g., Chlorella spp., Spirulina, Dunaliella sp., H. pluvialis) produce compounds associated with potentially beneficial health bio-activity, for example anti-fungal, anti-viral, anti-carcinogenic, anti-inflammatory, anti-oxidant, and immune system stimulant. Thus, they are used in a variety of health products, including tablets and capsules. Additionally, some species contain a high content of dietary fibers which have positive health benefits (Niccolai et al., 2019). However, further scientific evidence is needed to understand the health-related benefits of microalgae extracts and the bioavailability of the bioactive compounds (Caporgno and Mathys, 2018).
Chlorella spp., Nannochloropsis sp., and T. lutea are among the microalgae species used as feed in aquaculture and animal farming. In the aquaculture industry microalgae have been regarded as a sustainable alternative source to omega-3 from fish oils while the market for microalgae biomass as animal feed is already of considerable size (Carrasco et al., 2018). However, microalgae cannot yet be regarded as an alternative to vegetables or cereals as protein source for the feed market (Vigani et al., 2015). Microalgae are an important source of EPA and DHA (products with high commercial value) and can be directly incorporated in formulations for larvae and juvenile feed (mainly for rotifers that are then used for breeding crustaceans and fish). Astaxanthin from H. pluvialis was used as coloring pigment in aquaculture and the antioxidant activities have positive health effects and enhance the immunological system.
The market value for microalgae biomass varies depending on several factors such as the production system, production costs (energy and work force), geographical origin, certification schemes (e.g., organic production) and step of the value chain [e.g., Business to Business (B2B) or Business to Consumers (B2C)]. Based on consultation with algae producers, B2B values (on dry weight) for Chlorella sp. and Spirulina (the 2 main species marketed for food and food supplements), vary between 25–50 € kg–1 and 30–70 € kg–1, respectively. In contrast, B2C values for both species range between 150 and 280 € kg–1 (higher values for small package sizes, finished products). For Nannochloropsis sp., the most relevant species for feed, B2B price values are in the range of 30–110 € kg–1 and B2C market value (as marine phytoplankton) can go up to 1000€ kg–1. Speciality algae as well as algae used for the extraction of high value products are in a different price range. For example H. pluvialis B2C price varies between 150 and 300 € kg–1 while B2B price for astaxanthin oleoresin, based in pure astaxanthin, is in the range of 6.000–8.000 € kg–1. The emerging applications of microalgae biomass face an increasing market demand and have still room for a strong increase in the production volumes.
The extracts of some microalgae species (e.g., Nannochloropsis sp., Chlorella spp., Spirulina, Dunaliella sp., Schizochytrium sp., Tetraselmis sp., Porphyridium sp., P. tricornutum, and H. pluvialis) have been used in the cosmetic industry for skin regeneration, moisturizing, anti-aging, and protection from UV radiation (Ariede et al., 2017).
The use of microalgae as a feedstock for biofuel (biogas, biodiesel, and hydrogen) production has been the focus of several research and industrial efforts over the last decades. Microalgae have been identified as a promising feedstock for biofuel production since they do not compete for arable land with food and feed crops. They also show a high lipid content producing high oil yields. However, further technological developments are needed to upscale the production volumes and reduce the production costs that can turn the biofuel production from microalgae biomass a reality in the near future (Zhang et al., 2014).
Over the past decade the algal biorefineries have been investigated as an approach to optimize the use of multiple components of the feedstock for different applications. In addition, if connected to other industrial infrastructures, the algae production plants can benefit from the associated resources (e.g., water, heat) and function as a source of additional services such as wastewater treatment (Mayers et al., 2016). This synergy is expected to reduce the costs and increase the environmental sustainability of microalgae production but further research is needed on the optimization of such approach (Béchet et al., 2017).
Conclusion and Future Directions
This study illustrates the European algae industry as a bio-based sector with a considerable potential to further develop and contribute to critical societal challenges such as the EU carbon neutrality, an innovative food system that ensures access to nutritious and sustainable food, and, ultimately, the support to a sustainable and circular European bioeconomy. This potential is illustrated by the number of algae biomass production units mapped, spread across 23 European countries and covering different environments and production methods. Although many of the compiled production units are of small size, the activities related to the sector represent an important source of livelihood in several coastal areas in Europe. The production of seaweed is still very dependent on the harvesting of wild stocks, but the growing trend in seaweed aquaculture demonstrated by the number of operating companies mapped, represents new opportunities for the multiuse of the maritime space and the sustainable production of algae biomass while providing a series of ecosystem services such as bioremediation and carbon uptake. In contrast, the main production method of microalgae is photobioreactors which illustrates the potential of the European microalgae biomass in the market of high value products but also the non-readiness of this production system to support large scale biofuel production from algae biomass. In terms of the number of production units, the sector is well balanced between Spirulina (49.7%) and algae production (50.3%). For the latter, the microalgae production units are still a minority (33%). Further, the algae sector in Europe currently relies on the production of large biomass volumes of a reduced number of species. The results of this study also show that a variety of other species is currently produced which can be an opportunity to upscale the production volumes and meet increasing market demands or explore other high-value commercial applications based on low biomass volumes. Although currently the algae production in Europe is mainly directed to food and food- related uses, this study shows a variety of other applications being progressively established in the market. In contrast, less commercially important species are being researched, showing the innovative and wide range of influence and possibilities for the sector.
In Europe the algae and Spirulina sector is still immature and thus relatively small. Its future growth relies on technical innovations to upscale the production, while reducing the production costs. Additional key needs are to expand and diversify the market for algae and Spirulina commodities and to develop uniform standards for existing products and production methods while informing customers and simplifying regulatory and administrative procedures (e.g., for novel algae-based products, licensing of production units).
The biorefinery approach (algae biofactory) is currently being investigated as a mean to increase the environmental sustainability and economic feasibility of existing conventional industrial processes. Different potential conversion pathways are being researched for the use, extraction and valorization of algae biomass biorefinery products (Zhang and Thomsen, 2019). Additionally, technological improvements in the biomass cultivation systems are intensively researched (e.g., offshore aquaculture, biomass optimization practices, technology readiness for microalgae production and processing, incorporation of algae production in wastewater treatment and CO2 mitigation).
Yet, the expected boost in the development of the algae and Spirulina sector in Europe in the coming years needs also to be coupled with the protection of the environmental and recreational services provided by these coastal and fresh water-based ecosystems in order to guaranty the establishment of sustainable and responsible industries.
Data Availability Statement
The datasets presented in this article are not readily available because part of the data are confidential. Requests to access the datasets should be directed to RA, rita.araujo@ec.europa.eu.
Author Contributions
RA: conceptualization, methodology, investigation, writing-original draft, project administration, and supervision. FV: investigation, data curation, validation, writing-review and editing. JS: data visualization, data curation, writing-review and editing. IA, AB, SF, MG, TI, ML, MM, SM, CR, TS, and JU: investigation, writing-review and editing. FG: investigation, data curation, writing-review and editing. CP: investigation, data visualization, writing-review and editing. All authors contributed to the article and approved the submitted version.
Conflict of Interest
TI was employed by Vetik OÜ, MM was employed by Arramara Teoranta, SM was employed by Archimede Ricerche Srl, TS was employed by Algalíf Iceland ehf., and JU was employed by Roquette Klötze GmbH & Co.
The remaining authors declare that the research was conducted in the absence of any commercial or financial relationships that could be construed as a potential conflict of interest.
Acknowledgments
The authors acknowledge the following persons and institutions for providing data, comments or information to support and increase the quality of this manuscript: Adrian MacLeod, Haik van Exel, Helena Abreu, Vitor Verdelho, Jean Paul Cadoret, Fédération des Spiruliniers de France. (1) Spain: Ministry of Agriculture, Fisheries and Food (MAPA); the Autonomous Governments of Galicia, Asturias, Cantabria, and Andalusia; (2) Norway: Directorate of Fisheries; (3) France: Ministère de L’Ágriculture et de l’Alimentation. The description of the Norwegian industry and its data collections were conducted under series of projects and through annual updates to better understand the industry structure. In the frame of these projects, the European Union by the Atlantic Area operational programme, through the European Regional Development Fund (ERDF) and Nordland County Council (Norway) financially supported the initial investigation conducted during the NETALGAE project. Then, the Norwegian Research Council (244244 PROMAC, 294946SBP-N, and 55031Blå-Grønn), the Møre and Romsdal County Council and Møreforsking AS financially supported the latest data updates for Norway.
Footnotes
- ^ https://www.emodnet-humanactivities.eu/view-data.php
- ^ https://knowledge4policy.ec.europa.eu/visualisation/bioeconomy-different-countries_en#algae_prod_plants
- ^ https://impaqtproject.eu/
References
Abdul Khalil, H. P. S., Saurabh, C. K., Tye, Y. Y., Lai, T. K., Easa, A. M., Rosamah, E., et al. (2017). Seaweed based sustainable films and composites for food and pharmaceutical applications: a review. Renew. Sust. Energ. Rev. 77, 353–362. doi: 10.1016/j.rser.2017.04.025
Acién, F. G., Molina, E., Reis, A., Torzillo, G., Zittelli, G. C., Sepúlveda, C., et al. (2017). “Photobioreactors for the production of microalgae,” in Microalgae-Based Biofuels and Bioproducts, eds C. Gonzalez-Fernandez and R. Muñoz (Amsterdam: Elsevier Ltd; Woodhead Publishing Series in Energy), 1–44. doi: 10.1016/b978-0-08-101023-5.00001-7
Adarme-Vega, T. C., Lim, D. K. Y., Timmins, M., Vernen, F., Li, Y., and Schenk, P. (2012). Microalgal biofactories: a promising approach towards sustainable omega-3 fatty acid production. Microb. Cell Fact 11:96. doi: 10.1186/1475-2859-11-96
Alban, F., Frangoudes, K., and Fresard, M. (2011). Kelp harvesting fleet dynamics and the fleet’s dependence on Laminaria forest in the Iroise Sea (North Finistère, France). Cah. Biol. Mar. 52, 507–516.
Alban, F., Le Floc’h, P., and Boncoeur, J. (2004). The impact of economic and regulatory factors on the relative profitability of fishing boats. A case study of the seaweed harvesting fleet of Northwest Brittany (France). Aquat. Living Resour. 17, 185–193. doi: 10.1051/alr:2004013
Alexander, K. A., Angel, D., Freeman, S., Israel, D., Johansen, J., Kletou, D., et al. (2016). Improving sustainability of aquaculture in Europe: stakeholder dialogues on Integrated Multi-trophic Aquaculture (IMTA). Environ. Sci. Policy 55, 96–106. doi: 10.1016/j.envsci.2015.09.006
Araújo, R., Lusser, M., Sanchez Lopez, J., and Avraamides, M. (2019a). Brief on Algae Biomass Production. Luxembourg: Publications Office of the European Union.
Araújo, R., Sanchez Lopez, J., Landa, L., and Lusser, M. (2019b). Report on the community of practice workshop: algae production in europe: status, challenges and future developments. ARES 2019:3524950.
Araújo, R. M., Assis, J., Aguillar, R., Airoldi, L., Bárbara, I., Bartsch, I., et al. (2016). Status, trends and drivers of kelp forests in Europe: an expert assessment. Biod. Conserv. 25, 1319–1348. doi: 10.1007/s10531-016-1141-7
Ariede, M. B., Candido, T. M., Jacome, A. L. M., Velasco, M. V. R., Carvalho, J. C. M., and Baby, A. R. (2017). Cosmetic attributes of algae. Algal. Res. 25, 483–487. doi: 10.1016/j.algal.2017.05.019
Bak, U. G., Mols-Mortensen, A., and Gregersen, O. (2018). Production method and cost of commercial-scale offshore cultivation of kelp in the Faroe Islands using multiple partial harvesting. Algal. Res. 33, 36–47. doi: 10.1016/j.algal.2018.05.001
Barbier, M., Araújo, R., Rebours, C., Jacquemin, B., Holdt, R., and Charrier, B. (2020). Development and objectives of the Phycomorph European Guidelines for Sustainable Aquaculture of Seaweeds (PEGASUS). Bot. Mar. 63, 5–16. doi: 10.1515/bot-2019-0051
Barbier, M., Charrier, B., Araujo, R., Holdt, S. L., Jacquemin, B., and Rebours, C. (2019). “PEGASUS - PHYCOMORPH European guidelines for a sustainable aquaculture of seaweeds,” in COST Action FA1406 Phycomorph, eds M. Barbier and B. Charrier (France: European Commission), doi: 10.21411/2c3w-yc73
Barros, A., Pereira, H., Campos, J., Marques, A., Varela, J., and Silva, J. (2019). Heterotrophy as a tool to overcome the long and costly autotrophic scale-up process for large scale production of microalgae. Sci. Rep. 9:13935. doi: 10.1038/s41598-019-50206-z
Béchet, Q., Plouviez, M., Chambonnière, P., and Guieysse, B. (2017). “Environmental impacts of full-scale algae cultivation,” in Microalgae-Based Biofuels and Bioproducts, eds C. Gonzalez-Fernandez and R. Muñoz (Amsterdam: Elsevier Ltd.; Woodhead Publishing Series in Energy), 505–525.
Becker, E. W. (2007). Microalgae as a source of protein. Biotechnol. Adv. 25, 207–210. doi: 10.1016/j.biotechadv.2006.11.002
Bertocci, I., Araújo, R., Oliveira, P., and Sousa-Pinto, I. (2015). Potential effects of kelp species on local fisheries. J. Appl. Ecol. 52, 1216–1226. doi: 10.1111/1365-2664.12483
Blue Bioeconomy Forum (2019). Highlights. Synthesis of the Roadmap and a Selection of Viable and Innovative Projects. Luxembourg: European Commission. Publications Office of the European Union.
Bogie, J., Hoeks, C., Schepers, M., Tiane, A., Cuypers, A., and Leijten, F. (2019). Dietary Sargassum fusiforme improves memory and reduces amyloid plaque load in an Alzheimer’s disease mouse model. Sci. Rep. 9:4908. doi: 10.1038/s41598-019-41399-4
Borowitzka, M. A. (1999). Commercial production of microalgae: ponds, tanks, tubes and Fermenters. J. Biotechnol. 70, 313–321. doi: 10.1016/s0168-1656(99)00083-8
Borowitzka, M. A. (2013). “Dunaliella: biology, production, and markets,” in Handbook of Microalgal Culture, eds A. Richmond and Q. Hu (Chichester: Wiley-Blackwell), 359–368. doi: 10.1002/9781118567166.ch18
Broch, O. J., Alver, M. O., Bekkby, T., Gundersen, H., Forbord, S., and Handå, A. (2019). The kelp cultivation potential in coastal and offshore regions of Norway. Front. Mar. Sci. 5:529. doi: 10.3389/fmars.2018.00529
Bruhn, A., Tørring, D. B., Thomsen, M., Vergés, P. C., Nielsen, M. M., and Rasmussen, M. B. (2016). Impact of environmental conditions on biomass yield, quality, and bio-mitigation of Saccharina latissima. Aquacult. Environ. Interact. 8, 619–636. doi: 10.3354/aei00200
Buck, B. H., and Buchholz, C. M. (2004). The offshore-ring: a new system design for the open ocean aquaculture of macroalgae. J. Appl. Phycol. 16, 355–368. doi: 10.1023/B:JAPH.0000047947.96231.ea
Buck, B. H., and Grote, B. (2018). “Seaweed in high-energy environments: protocol to move Saccharina cultivation offshore,” in Protocols for Macroalgae Research, eds B. Charrier, T. Wichard, and C. R. K. Reddy (Boca Raton, MA: CRC Press), 3–36. doi: 10.1201/b21460-1
Buck, B. H., Troell, M. F., Krause, G., Angel, D. L., Grote, B., and Chopin, T. (2018). State of the art and challenges for offshore integrated multi-trophic aquaculture (IMTA). Front. Mar. Sci. 5:165. doi: 10.3389/fmars.2018.00165
Burrows, M. T., Fox, C. J., Moore, P., Smale, D., Sotheran, I., Benson, A., et al. (2018). Seaweed Harvesting as a Diversification Opportunity for Fishermen. A Report by SRSL for HIE. London: Highlands and Islands Enterprise, 171.
Buschmann, A. H., Camus, C., Infante, J., Neori, A., Israel, Á, Hernández-González, M. C., et al. (2017). Seaweed production: overview of the global state of exploitation, farming and emerging research activity. Eur. J. Phycol. 52, 391–406. doi: 10.1080/09670262.2017.1365175
Cabral, P., Levrel, H., Viard, F., Frangoudes, K., Girard, S., and Scemama, P. (2016). Ecosystem services assessment and compensation costs for installing seaweed farms. Mar. Policy 71, 157–165. doi: 10.1016/j.marpol.2016.05.031
Callaway, E. (2015). Lab staple agar runs low. Dwindling seaweed harvest imperils reagent essential for culturing microbes. News in focus. Nature 528, 171–172. doi: 10.1038/528171a
Campbell, I., Macleod, A., Sahlmann, C., Neves, L., Funderud, J., Øverland, M., et al. (2019). The environmental risks associated with the development of seaweed farming in Europe - Prioritizing key knowledge gaps. Front. Mar. Sci. 6:107. doi: 10.3389/fmars.2019.00107
Caporgno, M. P., and Mathys, A. (2018). Trends in microalgae incorporation into innovative food products with potential health benefits. Front. Nutr. 5:58. doi: 10.3389/fnut.2018.00058
Carrasco, R., Fajardo, C., Guarnizo, P., Vallejo, R. A., and Fernandez-Acero, F. J. (2018). Review: biotechnology applications of microalgae in the context of EU “Blue Growth” initiatives. JMGE 118:7371.
Casado-Amezúa, P., Araujo, R., Bárbara, I., Bermejo, R., Borja, Á, and Díez, I. (2019). Distributional shifts of canopy-forming seaweeds from the Atlantic coast of Southern Europe. Biodivers. Conserv. 28, 1151–1172. doi: 10.1007/s10531-019-01716-9
CEN/TC454 (2020). EN 17399: 2020 “Algae and Algae Products-Terms and Definitions”. CEN/TC 454 - Algae and Algae Products. European Committee for Standardization.
Chapman, A. R. O. (1995). Functional ecology of fucoid algae: twenty-three years of progress. Phycologia 34, 1–32. doi: 10.2216/i0031-8884-34-1-1.1
Chatterjee, A., Singh, S., Agrawal, C., Yadav, S., Rai, R., and Rai, L. C. (2017). “Role of algae as a biofertilizer: recent progress in biotechnology,” in Algal Green Chemistry, eds R. P. Rastogi, D. Madamwar, and A. Pandey (Amsterdam: Elsevier), 189–200. doi: 10.1016/B978-0-444-63784-0.00010-2
Chopin, T., Buschmann, A., Hulling, C., Troell, M., Kautsky, N., and Neori, A. (2001). Integrating seaweeds into marine aquaculture systems: a key toward sustainability. J. Phycol. 37, 975–986. doi: 10.1046/j.1529-8817.2001.01137.x
Christie, H., Norderhaug, K. M., and Fredriksen, S. (2009). Macrophytes as habitat for fauna. Mar. Ecol. Prog. Ser. 396, 221–233. doi: 10.3354/meps08351
Cofrades, S., López-López, I., Solas, M. T., Bravo, L., and Jiménez-Colmenero, F. (2008). Influence of different types and proportions of added edible seaweeds on characteristics of low salt gel/emulsion meat systems. Meat Sci. 79, 767–776. doi: 10.1016/j.meatsci.2007.11.010
Costa, J. A. V., Freitas, B. C. B., Santos, T. D., Mitchell, B. G., and Morais, M. G. (2019). “Open pond system for microalgae culture,” in Biomass, Biofuels and Bioechamicals, Biofuels from Algae, eds A. Pandey, J.-S. Chang, C. R. Soccol, D.-J. Lee, and Y. Chist (Amsterdam: Elsevier), 199–223.
Costello, M. J., Bouchet, P., Emblow, C. S., and Legakis, A. (2006). European marine biodiversity inventory and taxonomic resources: state of the art and gaps in knowledge. Mar. Ecol. Prog. Ser. 316, 257–268. doi: 10.3354/meps316257
Cottier-Cook, E., Nagabhatla, N., Badis, Y., Campbell, M. L., Chopin, T., Dai, W., et al. (2016). Safeguarding the Future of the Global Seaweed Aquaculture Industry. United Nations University Policy-brief, Safeguarding the Future of the Global Seaweed Aquaculture Industry. Available online at: https://www.sams.ac.uk/t4-media/sams/pdf/globalseaweed-policy-brief.pdf (accessed May, 2020).
Darda, S., Papalas, T., and Zabaniotou, A. (2019). Biofuels journey in Europe: currently the way to low carbon economy sustainability is still a challenge. J. Clean Prod. 208, 575–588. doi: 10.1016/j.jclepro.2018.10.147
Davoult, D., Engel, C. R., Arzel, P., Knoch, D., and Laurans, M. (2011). Environmental factors and commercial harvesting: exploring possible links behind the decline of the kelp Laminaria digitata in Brittany, France. Cah. Biol. Mar. 52, 429–434.
Dayton, P. K. (1985). Ecology of kelp communities. Annu. Rev. Ecol. Syst. 16, 215–245. doi: 10.1146/annurev.es.16.110185.001243
de Carvalho, L. L., de Souza, E. G. A., da Mata Júnior, M. R., and Villaça, R. C. (2017). Assessment of rocky reef fish assemblages close to seaweed farming. Aquac. Res. 48, 481–493. doi: 10.1111/are.12896
Deng, L. P., Sua, Y. Y., Sua, H., Wang, X. T., and Zhua, X. B. (2007). Sorption and desorption of lead (II) from wastewater by green algae Cladophora fascicularis. J. Hazard Mater. 143:2225.
Directorate of Fisheries (2020). Available online at: https://www.fiskeridir.no/Akvakultur/Tall-og-analyse/Akvakulturstatistikk-tidsserier/Alger (accessed August, 2020).
Duarte, C. M., and Krause-Jensen, D. (2018). Intervention options to accelerate ecosystem recovery from coastal eutrophication. Front. Mar. Sci. 5:470. doi: 10.3389/fmars.2018.00470
Duarte, C. M., Wu, J., Xiao, X., Bruhn, A., and Krause-Jensen, D. (2017). Can seaweed farming play a a role in climate change mitigation and adaptation? Front. Mar. Sci. 4:100. doi: 10.3389/fmars.2017.00100
EC (2012). Commission Communication COM/2012/494. Blue Growth Opportunities for Marine and Maritime Sustainable Growth. Available online at: https://ec.europa.eu/maritimeaffairs/sites/maritimeaffairs/files/docs/body/com_2012_494_en.pdf (accessed May, 2020).
EC (2018). Commission Communication COM/2018/673. A Sustainable Bioeconomy for Europe: Strengthening the Connection between Economy, Society and the Environment. Available online at: https://eur-lex.europa.eu/legal-content/en/ALL/?uri=CELEX%3A52018DC0673 (accessed May, 2020).
EC (2020a). Commission Communication COM/2020/28. A New Circular Economy Action Plan for a Cleaner and More Competitive Europe. Available online at: https://eur-lex.europa.eu/legal-content/EN/TXT/?uri=COM%3A2020%3A98%3AFIN (accessed May, 2020).
EC (2020b). Commission Communication COM/2020/380. EU Biodiversity Strategy for 2030. Bringing Nature Back into Our Lives. Available online at: https://eur-lex.europa.eu/legal-content/EN/TXT/?qid=1590574123338&uri=CELEX:52020DC0380 (accessed May, 2020).
EC (2020c). Commission Communication COM/2020/381. A Farm to Fork Strategy for a Fair, Healthy and Environmentally-Friendly Food System. Available online at: https://eur-lex.europa.eu/legal-content/EN/TXT/?uri=CELEX:52020DC0381 (accessed May, 2020).
EC 216/2009 (2009). Regulation (EC) No 216/2009 of the European Parliament and of the Council of 11 March 2009 on the Submission of Nominal catch Statistics by Member States Fishing in Certain Areas other Than those of the North Atlantic (Recast). Available online at: https://eur-lex.europa.eu/legal-content/EN/TXT/PDF/?uri=CELEX:32009R0216&from=en (accessed May, 2020).
EC 217/2009 (2009). Regulation (EC) No 217/2009 of the European Parliament and of the Council of 11 March 2009 on the Submission of Catch and Activity Statistics by Member States Fishing in the North-West Atlantic (Recast). Available online at: https://eur-lex.europa.eu/legal-content/EN/TXT/PDF/?uri=CELEX:32009R0217&from=EN (accessed May, 2020).
EC 218/2009 (2009). Regulation (EC) No 218/2009 of the European Parliament and of the Council of 11 March 2009 on the Submission of Nominal Catch Statistics by Member States Fishing in the North-East Atlantic (Recast). Available online at: https://eur-lex.europa.eu/legal-content/EN/TXT/PDF/?uri=CELEX:32009R0218&from=EN (accessed May, 2020).
EC 762/2008 (2008). Regulation (EC) No 762/2008 of the European Parliament and of the Council of 9 July 2008 on the Submission by Member States of Statistics on Aquaculture and Repealing Council Regulation (EC) No 788/96. Available online at: https://eur-lex.europa.eu/legal-content/EN/TXT/PDF/?uri=CELEX:32008R0762&from=EN (accessed May, 2020).
Edwards, M., and Watson, L. (2011). Cultivating Laminaria digitata. Aquaculture Explained No. 26. Bord lascaigh Mhara. Dún Laoghaire: Irish Sea Fisheries Board, 72.
Emblemsvåg, J., Kvadsheim, N. P., Halfdanarson, J., Koesling, M., Nystrand, B. T., Sunde, J., et al. (2020). Strategic considerations for establishing a large-scale seaweed industry based on fish feed application: a Norwegian case study. J. Appl. Phycol. 32, 4159–4169. doi: 10.1007/s10811-020-02234-w
EU (2015). Regulation 2015/2283 of the European Parliament and of the Council of 25 November 2015 on Novel Foods, Amending Regulation (EU) No 1169/2011 of the European Parliament and of the Council and repealing Regulation (EC) No 258/97 of the European Parliament and of the Council and Commission Regulation (EC) No 1852/2001. Available online at: https://eur-lex.europa.eu/legal-content/EN/TXT/?uri=CELEX:32015R2283 (accessed May, 2020).
EU 2019/909 (2019). Commission Implementing Decision (EU) 2019/909 of 18 February 2019 Establishing the List of Mandatory Research Surveys and Thresholds for the Purposes of the Multiannual Union Programme for the Collection and Management of data in the Fisheries and Aquaculture Sectors. Available online at: http://dcf.mir.gdynia.pl/wp-content/uploads/2019/10/COM_Impl-Decision_2019-909_EU-MAP_surveys-thresholds.pdf (accessed May, 2020).
FAO (2020). FishStatJ - Software for Fishery and Aquaculture Statistical Time Series. Rome: Food and Agricultural Organization of the United Nations.
Filbee-Dexter, K., and Wernberg, T. (2018). Rise of turfs: a new battlefront for globally declining kelp forests. Bioscience 68, 64–76. doi: 10.1093/biosci/bix147
Forbord, S., Steinhovden, K. B., Rød, K. K., Handå, A., and Skjermo, J. (2018). “Cultivation protocol for Saccharina latissima,” in Protocols for Macroalgae Research, eds B. Charrier, T. Wichard, and C. R. K. Reddy (Boca Raton, MA: CRC Press), 37–60. doi: 10.1201/b21460-2
Frangoudes, K. (2011). Seaweeds fisheries management in France, Japan, Chile and Norway. Cah. Biol. Mar. 52, 517–525.
García Tasende, M., Cid, M., and Fraga, M. I. (2012). Spatial and temporal variations of Chondrus crispus (Gigartinaceae, Rhodophyta) carrageenan content in natural populations from Galicia (NW Spain). J. Appl. Phycol. 24, 941–951. doi: 10.1007/s10811-011-9715-y
García Tasende, M., Cid, M., and Fraga, M. I. (2013). Qualitative and quantitative analysis of carrageenan content in gametophytes of Mastocarpus stellatus (Stackhouse) Guiry along Galician coast (NW Spain). J. Appl. Phycol. 25, 587–596. doi: 10.1007/s10811-012-9893-2
García Tasende, M., and Peteiro, C. (2015). Explotación de las macroalgas marinas: galicia como caso de estudio hacia una gestión sostenible de los recursos. Rev. Ambien. 111, 116–132.
García Tasende, M., and Rodríguez González, L. M. (2003). Economic seaweeds of Galicia (NW Spain). Thalassas 19, 17–25. doi: 10.1127/anthranz/56/1998/17
Goecke, F., Klemetsdal, G., and Ergon, A. (2020). Cultivar development of kelps for commercial cultivation-past lessons and future prospects. Front. Mar. Sci. 8:110. doi: 10.3389/fmars.2020.00110
Guiry, M. D., and Morrisson, L. (2013). The sustainable harvesting of Ascophyllum nodosum (Fucaceae, Phaeophyceae) in Ireland, with notes on the collection and use of some other brown algae. J. Appl. Phycol. 25, 1823–1830. doi: 10.1007/s10811-013-0027-2
Hafting, J. T., Craigie, J. S., Stengel, D. B., Loureiro, R. R., Buschmann, A. H., Charles, Y., et al. (2015). Prospects and challenges for industrial production of seaweed bioactives. J. Phycol. 51, 821–837. doi: 10.1111/jpy.12326
Hafting, J. T., Critchley, A. T., Cornish, M. L., Hubley, S. A., and Archibald, A. F. (2012). On-land cultivation of functional seaweed products for human usage. J. Appl. Phycol. 24, 385–392. doi: 10.1007/s10811-011-9720-1
Handå, A., Forbord, S., Wang, X. X., Broch, O. J., Dahle, S. W., Trond, R. S., et al. (2013). Seasonal- and depth-dependent growth of cultivated kelp (Saccharina latissima) in close proximity to salmon (Salmo salar) aquaculture in Norway. Aquaculture 414, 191–201. doi: 10.1016/j.aquaculture.2013.08.006
Hasselström, L., Visch, W., Gröndahl, F., Nylund, G. M., and Pavia, H. (2018). The impact of seaweed cultivation on ecosystem services - a case study from the west coast of Sweden. Mar. Pollut. Bull. 133, 53–64. doi: 10.1016/j.marpolbul.2018.05.005
Hemantkumar, J. N., and Rahimbhai, M. I. (2019). “Microalgae and its use in nutraceuticals and food supplments,” in Microalgae: from Physiology to Application, ed. M. Vítová (London: IntechOpen).
Holdt, S. L., and Kraan, S. (2011). Bioactive compounds in seaweed: functional food applications and legislation. J. Appl. Phycol. 23, 543–597. doi: 10.1007/s10811-010-9632-5
Jansen, H. M., Van Den Burg, S., Bolman, B., Jak, R. G., Kamermans, P., Marnix, P., et al. (2016). The feasibility of offshore aquaculture and its potential for multi-use in the North Sea. Aquacult. Int. 24, 735–756. doi: 10.1007/s10499-016-9987-y
Jaywant, S. A., and Arif, K. M. (2019). A comprehensive review of microfluidic water quality monitoring sensors. Sensors 19:4781. doi: 10.3390/s19214781
Juanes, J. J., and Sosa, P. A. (1998). “The seaweed resources of Spain,” in Seaweed Resources of the World, eds A. T. Critchley and M. Ohno (Jokosuka: Japan International Cooperation Agency (JICA)), 164–175.
Jung, F., Krüger-Genge, A., Waldeck, P., and Küpper, J.-H. (2019). Spirulina platensis, a super food? J. Cell. Biotech. 5, 43–54. doi: 10.3233/jcb-189012
Kiesenhofer, D. P., and Fluch, S. (2018). The promises of microalgae-still a long way to go. FEMS Microbiol. Lett. 365:fnx257. doi: 10.1093/femsle/fnx257
Kinley, R. D., Martinez-Fernandez, G., Matthews, M. K., de Nys, R., and Magnusson, M. (2020). Mitigating the carbon footprint and improving productivity of ruminant livestock agriculture using a red seaweed. J. Clean. Prod. 259:120836. doi: 10.1016/j.jclepro.2020.120836
Kleitou, P., Kletou, D., and David, J. (2018). Is Europe ready for integrated multi-trophic aquaculture? A survey on the perspectives of European farmers and scientists with IMTA experience. Aquaculture 490, 136–148. doi: 10.1016/j.aquaculture.2018.02.035
Kraan, S. (2010). Mass-cultivation of carbohydrate rich macroalgae, a possible solution for sustainable biofuel production. Mitig. Adapt. Strat. Gl. 18, 1–20. doi: 10.1007/s11027-010-9275-5
Kraan, S. (2012). “Algal polysaccharides, novel applications and outlook,” in Carbohydrates: Comprehensive Studies on Glycobiology and Glycotechnology, ed. C.-F. Chang (London: InTechOpen), 489–532. doi: 10.5772/51572
Krumhansl, K. A., Okamoto, D. K., Rassweiler, A., Novak, M., Bolton, J. J., Sean, D. C., et al. (2016). Global patterns of kelp forest change over the past half-century. Proc. Natl. Acad. Sci. U.S.A. 113, 13785–13790. doi: 10.1073/pnas.1606102113
Kwon, P. S., Oh, H., Kwon, S., Jin, W., Zhang, F., and Fraser, K. (2020). Sulfated polysaccharides effectively inhibit SARS-CoV-2 in vitro. Cell Discov. 6:50. doi: 10.1038/s41421-020-00192-8
Le Bras, Q., Lesueur, M., Lucas, S., and Gouin, S. (2015). Etude du Marché Français des algues Alimentaires. Panorama de la Distribution. Programme IDEALG Phase 2. Les Publications du Pôle Halieutique Agrocampus Ouest n° 36. Saint-Brieuc: AGROCAMPUS WEST Fisheries Center, Cellule Études et Transfert. 42.
Le Bras, Q., Ritter, L., Fasquel, D., Lesuer, M., Lucas, S., and Gouin, S. (2014). Etude de la Consommation des Algues Alimentaires em France. Programme Idealg Phase 1: Etude Nationale. Les Publications du Pôle Halieutique Agrocampus Ouest n° 35, Cellule Études et Transfert. 72.
López-López, I., Cofrades, S., Ruiz-Capillas, C., and Jiménez-Colmenero, F. (2009). Design and nutritional properties of potential functional frankfurters based on lipid formulation, added seaweed and low salt content. Meat Sci. 83, 255–262. doi: 10.1016/j.meatsci.2009.05.014
Lorentsen, S.-H., Sjøtun, K., and Gremillet, D. (2010). Multi-trophic consequences of kelp harvest. Biol. Conserv. 143, 2054–2062. doi: 10.1016/j.biocon.2010.05.013
Lotze, H. K., Derek, P., Tittensor, D. P., Bryndum-Buchholz, A., Eddy, T. D., and Cheung, W. W. L. (2019). Global ensemble projections reveal trophic amplification of ocean biomass declines with climate change. Proc. Natl. Acad. Sci. U.S.A. 116, 12907–12912. doi: 10.1073/pnas.1900194116
Loureiro, R., Gachon, M. M., and Rebours, C. (2015). Seaweed cultivation: potential and challenges of crop domestication at an unprecented pace. New. Phytol. 2, 489–492. doi: 10.1111/nph.13278
Mac Monagail, M., Cornish, L., Morrisson, L., Araújo, R., and Critchley, A. T. (2017). Sustainable harvesting of wild seaweed resources. Eur. J. Phycol. 52, 371–390. doi: 10.1080/09670262.2017.1365273
Mac Monagail, M., and Morrison, L. (2020). The seaweed resources of Ireland: a twenty-first century perspective. J. Appl. Phycol. 32, 1287–1300. doi: 10.1007/s10811-020-02067-7
Machado, L., Magnusson, M., Paul, N. A., de Nys, R., and Tomkins, N. (2014). Effects of marine and freshwater macroalgae on in vitro total gas and methane production. PLoS One 9:e85289. doi: 10.1371/journal.pone.0085289
Maehre, H. K., Malde, M. K., Eilertsen, K. E., and Elvevoll, E. O. (2014). Characterization of protein, lipid and mineral content in common Norwegian seaweeds and evaluation of their potential as food and feed. J. Sci. Food. Agric. 94, 3281–3290. doi: 10.1002/jsfa.6681
Maia, M. R. G., Fonseca, A. J. M., Oliveira, H. M., Mendonça, C., and Cabrita, A. R. J. (2016). The potential role of seaweeds in the natural manipulation of rumen fermentation and methane production. Sci. Rep. 6:32321. doi: 10.1038/srep32321
Makkar, H. P. S., Tran, G., Heuzé, V., Giger-Reverdin, S., Lessire, M., and Lebas, F. (2016). Seaweeds for livestock diets: a review. Anim. Feed Sci. Technol. 212, 1–17. doi: 10.1016/j.anifeedsci.2015.09.018
Marcati, A., Ursu, A. V., Laroche, C., Soanen, N., Marchal, L., and Jubeau, S. (2014). Extraction and fractionation of polysaccharides and B-phycoerythrin from the microalgae Porphyridium cruentum by membrane technology. Algal. Res. 5, 258–263. doi: 10.1016/j.algal.2014.03.006
Marine Scotland Directorate (2016). Wild Seaweed Harvesting: Strategic Environmental Assessment - Environmental Report. St Andrew’s House: Scotish Government.
Marinho, G., Holdt, S., Birkeland, M., and Angelidaki, I. (2015). Commercial cultivation and bioremediation potential of sugar kelp, Saccharina latissima, in Danish waters. J. Appl. Phycol. 27, 1963–1973. doi: 10.1007/s10811-014-0519-8
Marinho-Soriano, E., Fonseca, P. C., Carneiro, M. A. A., and Moreira, W. S. C. (2006). Seasonal variation in the chemical composition of two tropical seaweeds. Biores. Tech. 97, 2402–2406. doi: 10.1016/j.biortech.2005.10.014
Mayers, J. J., Nilsson, A. E., Svensson, E., and Albers, E. (2016). Integrating microalgae production with industrial outputs-reducing process inputs and quantifying the benefits. Indust. Biotechnol. 12:4.
Milledge, J. J., Nielsen, B. V., and Bailey, D. (2015). High-value products from macroalgae: the potential uses of the invasive brown seaweed, Sargassum muticum. Rev. Environ. Sci. Biotechnol. 15, 67–88. doi: 10.1007/s11157-015-9381-7
Mobin, S., and Alam, F. (2017). Some promising microalgal species for commercial applications: a review. Energy Proc. 110, 510–517. doi: 10.1016/j.egypro.2017.03.177
Mouritsen, O. G., Dawczynski, C., Duelund, L., Jahreis, G., Vetter, W., and Schröder, M. (2013). On the human consumption of the red seaweed dulse (Palmaria palmata (L.) Weber & Mohr). J. Appl. Phycol. 25, 1777–1791. doi: 10.1007/s10811-013-0014-7
Mouritsen, O. G., Rhatigan, P., and Pérez-Lloréns, J. L. (2019). The rise of seaweed gastronomy: phycogastronomy. Bot. Mar. 62, 195–209. doi: 10.1515/bot-2018-0041
Muller, R., Laepple, T., Bartsch, I., and Wiencke, C. (2009). Impact of oceanic warming on the distribution of seaweeds in polar and cold-temperate waters. Bot. Mar. 52, 617–638.
Murthy, G. S. (2011). “Overview and assessment of algal biofuels production technologies,” in Biofuels: Alternative Feedstocks and Conversion Processes, eds A. Pandey, C. Larroche, S. C. Ricke, C.-G. Dussap, and E. Gnansounou (Amsterdam: Elsevier Inc), 415–437. doi: 10.1016/b978-0-12-385099-7.00019-x
Narala, R. R., Garg, S., Sharma, K. K., Thomas-Hall, S. R., Deme, M., Li, Y., et al. (2016). Comparison of microalgae cultivation in photobioreactor, open raceway pond and a two-stage hybrid system. Front. Energy Res. 4:29. doi: 10.3389/fenrg.2016.00029
Neori, A., Chopin, T., Troell, M., Buschmann, A. H., Kraemer, G. P., and Halling, C. (2004). Integrated aquaculture: rationale, evolution and state of the art emphasizing seaweed biofiltration in modem mariculture. Aquaculture 231, 361–391. doi: 10.1016/j.aquaculture.2003.11.015
Niccolai, A., Zittelli, G. C., Rodolfi, L., Biondi, N., and Tredici, M. R. (2019). Microalgae of interest as food source: biochemical composition and digestibility. Algal Res. 42:101617. doi: 10.1016/j.algal.2019.101617
O’Mahoney, M., Rice, O., Mouzakitis, G., and Burnell, G. (2014). Towards sustainable feeds for abalone culture: evaluating the use of mixed species seaweed meal in formulated feds for the Japanese abalone, Haliotis discus hannai. Aquaculture 430, 9–16. doi: 10.1016/j.aquaculture.2014.02.036
Patarra, R. F., Paiva, L., Neto, A. I., Lima, E., and Baptista, J. (2011). Nutritional value of selected macroalgae. J. Appl. Phycol. 23, 205–208. doi: 10.1007/s10811-010-9556-0
Pereira, R., Yarish, C., and Critchley, A. T. (2013). “Seaweed aquaculture for human foods in land-based and IMTA systems,” in Sustainable Food Production, eds P. Christou, R. Savin, B. A. Costa-Pierce, I. Misztal, and C. B. A. Whitelaw (Berlin: Springer), 1405–1424. doi: 10.1007/978-1-4614-5797-8_189
Peteiro, C. (2018). “Alginate production from marine macroalgae, with emphasis on kelp farming,” in Alginates and their Biomedical Applications, eds B. H. A. Rehm and F. Moradali (Singapore: Springer), 27–66. doi: 10.1007/978-981-10-6910-9_2
Peteiro, C., Bidegain, G., and Sánchez, N. (2019). Experimental evaluation of the effect of water velocity on the development of string-attached kelp seedlings (Laminariales) with implications for hatchery and nursery production. Algal Res. 44:101678. doi: 10.1016/j.algal.2019.101678
Peteiro, C., and Freire, Ó (2013). Epiphytism on blades of the edible kelps Undaria pinnatifida and Saccharina latissima farmed under different abiotic conditions. J. World. Aquacult. Soc. 44, 706–715. doi: 10.1111/jwas.12065
Peteiro, C., Sánchez, N., Dueñas-Liaño, C., and Martínez, B. (2014). Open-sea cultivation by transplanting young fronds of the kelp Saccharina latissima. J. Appl. Phycol. 26, 519–528. doi: 10.1007/s10811-013-0096-2
Peteiro, C., Sánchez, N., and Martínez, B. (2016). Mariculture of the Asian kelp Undaria pinnatifida and the native kelp Saccharina lattisima along the Atlantic coast of southern Europe: an overview. Algal Res. 15, 9–23. doi: 10.1016/j.algal.2016.01.012
Philis, G., Gracey, E. O., Gansel, L. C., Fet, A. M., and Rebours, C. (2018). Comparing the primary energy and phosphorus consumption of soybean and seaweed-based aquafeed proteins-a material and substance flow analysis. J. Clean. Prod. 200, 1142–1153. doi: 10.1016/j.jclepro.2018.07.247
Praeger, C., Vucko, M. J., McKinna, L., de Nys, R., and Cole, A. (2020). Estimating the biomass density of macroalgae in land-based cultivation systems using spectral reflectance imagery. Algal Res. 50:102009. doi: 10.1016/j.algal.2020.102009
Prussi, M., Chiaramonti, D., Casini, D., Rodolfi, L., Bassi, N., and Bacci, F. (2011). “Improved raceway ponds for microalgae production,” in Proceedings of the Conference Paper: Atti 19th International Symposium on Alcohol Fuels, Verona.
Ravanal, M. C., Camus, C., Buschmann, A. H., Gimpel, J., Olivera-Nappa, A., and Salazar, O. (2019). “Production of bioethanol from brown algae,” in Advances in Feedstock Conversion Technologies for Alternative Fuels and Bioproducts, ed. M. Hosseini (Cambridge: Woodhead Publishing Series in Energy), 69–88. doi: 10.1016/B978-0-12-817937-6.00004-7
Rebours, C., Marinho-Soriano, E., Zertuche-González, J. A., Hayashi, L., Vásquez, J. Á, and Kradolfer, P. (2014). Seaweeds: an opportunity for wealth and sustainable livelihood for coastal communities. J. Appl. Phycol. 26, 1939–1951. doi: 10.1007/s10811-014-0304-8
Reis, P. A., Gonçalves, J., Abreu, H., Pereira, R., Benoit, M., O’Mahony, F., et al. (2016). Seaweed Alaria esculenta as a biomonitor species of metal contamination in Aughinish Bay (Ireland)). Ecol. Indic. 69, 19–25. doi: 10.1016/j.ecolind.2016.03.041
Reisewitz, S. E., Estes, J. A., and Simenstad, C. A. (2006). Indirect food web interactions: sea otters and kelp forest fishes in the Aleutian archipelago. Oecologia 146, 623–631. doi: 10.1007/s00442-005-0230-1
Rioux, L.-E., Beaulieu, L., and Turgeon, S. L. (2017). Seaweeds: a traditional ingredients for new gastronomic sensation. Food Hydrocoll. 68, 255–265. doi: 10.1016/j.foodhyd.2017.02.005
Roque, B. M., Brooke, C. G., Ladau, J., Polley, T., Marsh, L. J., Najafi, N., et al. (2019). Effects of the macroalgae Asparagopsis taxiformis on methane production and rumen microbiome assemblage. Anim. Microb. 1, 1–14.
Schiener, P., Black, K. D., Stanley, M. S., and Green, D. H. (2015). The seasonal variation in the chemical composition of the kelp species Laminaria digitata, Laminaria hyperborea, Saccharina latissima and Alaria esculenta. J. Appl. Phyc. 27, 363–373. doi: 10.1007/s10811-014-0327-1
Schiener, P., Zhao, S., Theodoridou, K., Carey, M., Mooney-McAuley, K., and Greenwell, C. (2016). The nutritional aspects of biorefined Saccharina latissima, Ascophyllum nodosum and Palmaria palmata. Biomass Convers. Biorefin. 7, 221–235. doi: 10.1007/s13399-016-0227-5
Stagnol, D., Michel, R., and Davoult, D. (2016). Population dynamics of the brown alga Himanthalia elongata under harvesting pressure. Estuar. Coast. Shelf. S 174, 65–70. doi: 10.1016/j.ecss.2016.03.014
Stanic-Vucinic, D., Minic, S., Nikolic, M. R., and Velickovic, T. C. (2018). “Spirulina Phycobiliproteins as food components and complements,” in Microalgal Biotechnology, eds E. Jacob-Lopes, L. Q. Zepka, and M. I. Queiroz (London: IntechOpen).
Steneck, R. S., Graham, M. H., Bourque, B. J., Corbett, D., Erlandson, J. M., Estes, J. A., et al. (2002). Kelp forest ecosystems: biodiversity, stability, resilience and future. Environ. Conserv. 29, 436–459. doi: 10.1017/s0376892902000322
Stévant, P. (2019). Seaweeds in Food Applications: Effects of Processing on Product Quality. Trondheim, NO: Norwegian University of Science and Technology.
Stévant, P., Rebours, C., and Chapman, A. (2017). Seaweed aquaculture in Norway: recent industrial developments and future perspectives. Aquacult. Int. 25, 1373–1390. doi: 10.1007/s10499-017-0120-7
Sun, Y., Wang, H., Guo, G., Yinfang, P., and Binlun, Y. (2014). The isolation and antioxidant activity of polysaccharides from the marine microalgae Isochrysis galbana. Carbohyd. Polym. 113, 22–31. doi: 10.1016/j.carbpol.2014.06.058
Taelman, S. E., Champenois, J., Edwards, M. D., De Meester, S., and Dewulf, J. (2015). Comparative environmental life cycle assessment of two seaweed cultivation systems in North West Europe with a focus on quantifying sea surface occupation. Algal Res. 11, 173–183. doi: 10.1016/j.algal.2015.06.018
Teagle, H., Hawkins, S. J., Moore, P. J., and Smale, D. A. (2017). The role of kelp species as biogenic habitat formers in coastal marine ecosystems. J. Exp. Mar. Biol. Ecol. 492, 81–98. doi: 10.1016/j.jembe.2017.01.017
Thomas, N., and Kim, S.-K. (2013). Beneficial effects of marine algal compounds in cosmeceuticals. Mar. Drugs 11, 146–164. doi: 10.3390/md11010146
Titlyanov, E. A., and Titlyanova, T. V. (2010). Seaweed cultivation: methods and problems. Russ. J. Mar. Biol. 36, 227–242. doi: 10.1134/S1063074010040012
Troell, M., Joyce, A., Chopin, T., Neori, A., Buschmann, A. H., and Fang, J. G. (2009). Ecological engineering in aquaculture - Potential for integrated multi-trophic aquaculture (IMTA) in marine offshore systems. Aquaculture 297, 1–9. doi: 10.1016/j.aquaculture.2009.09.010
Usher, P. K., Ross, A. B., Camargo-Valeo, M. A., Tomlin, A. S., and Gale, W. F. (2014). An overview of the potential environmental impacts of large-scale microalgae cultivation. Biofuels 5, 331–349. doi: 10.1080/17597269.2014.913925
van den Burg, S. W. K., Rockmann, C., Banach, J. L., and van Hoof, L. (2020). Governing risks of multi-use: seaweed aquaculture at offshore wind farms. Front. Mar. Sci. 7:60. doi: 10.3389/fmars.2020.00060
van der Molen, J., Ruardlj, P., Mooney, K., Kerrison, P., O’Connor, N. E., and Gorman, E. (2018). Modelling potential production of macroalgae farms in UK and Dutch coastal waters. Biogeosciences 15, 1123–1147. doi: 10.5194/bg-15-1123-2018
Vea, J., and Ask, E. I. (2011). Creating a sustainable commercial harvest of Laminaria hyperborea, in Norway. J. Appl. Phyc. 23, 489–594. doi: 10.1007/s10811-010-9610-y
Venolia, C. T., Lavaud, R., Green-Gavrielidis, L. A., Thornber, C., and Humphries, A. T. (2020). Modeling the growth of sugar kelp (Saccharina latissima) in aquaculture systems using dynamic energy budget theory. Ecol. Mod. 430:109151. doi: 10.1016/j.ecolmodel.2020.109151
Vigani, M., Parisi, C., Rodríguez-Cerezo, E., Barbosa, M. J., Sijtsma, L., and Ploeg, M. (2015). Food and feed products from micro-algae: market opportunities and challenges for the EU. Food Sci. Tech. 42, 81–92. doi: 10.1016/j.tifs.2014.12.004
Visch, W., Kononets, M., Hall, P. O. J., Nylund, G. M., and Pavia, H. (2020a). Environmental impact of kelp (Saccharina latissima) aquaculture. Mar. Pollut. Bull. 155:110962. doi: 10.1016/j.marpolbul.2020.110962
Visch, W., Nylund, G. M., and Pavia, H. (2020b). Growth and biofouling in kelp aquaculture (Saccharina latissima): the effect of location and wave exposure. J. Appl. Phycol. 32, 3199–3209. doi: 10.1007/s10811-020-02201-5
Vonshak, A., and Tomaselli, L. (2002). “Arthrospira (Spirulina): systematics and ecophysioiogy,” in The Ecology of Cyanobacteria: Their Diversity in Time and Space, eds B. A. Whitton and M. Potts (Dordrecht: Springer), 505–522. doi: 10.1007/0-306-46855-7_18
Walls, A. M., Kennedy, R., Edwards, M. D., and Johnson, M. P. (2017). Impact of kelp cultivation on the ecological status of benthic habitats and Zostera marina seagrass biomass. Mar. Pollut. Bull. 123, 19–27. doi: 10.1016/j.marpolbul.2017.07.048
Wan, A. H. L., Davies, S. J., Soler-Vila, A., Fitzgerald, R., and Johnson, M. P. (2019). Macroalgae as a sustainable aquafeed ingredient. Rev. Aquac. 11, 458–492. doi: 10.1111/raq.12241
Ward, G. M., Faisan, J. P., Cottier-Cook, E. J., Gachon, C., Hurtado, A. Q., and Lim, P. E. (2020). A review of reported seaweed diseases and pests in aquaculture in Asia. J. World Aquacult. Soc. 51, 815–828. doi: 10.1111/jwas.12649
Wells, M. L., Potin, P., Craigie, J. S., Raven, J. A., and Merchant, S. S. (2016). Algae as nutritional and functional food sources: revisiting our understanding. J. Appl. Phycol. 29, 949–982. doi: 10.1007/s10811-016-0974-5
Wilson, K. L., Skinner, M. A., Lotze, H. K., and Sorte, C. (2019). Projected 21st-century distribution of canopy-forming seaweeds in the Northwest Atlantic with climate change. Divers. Distrib. 25, 582–602. doi: 10.1111/ddi.12897
Yarish, C., Kim, J. K., Lindell, S., and Kite-Powell, H. (2017). Developing an Environmentally and Economically Sustainable Sugar Kelp Aquaculture Industry in Southern New England: from Seed to Market. Mansfield, CO: Department of Ecology and Evolutionary Biology, University of Connecticut.
Zeraatkar, A. K., Ahmadzadeh, H., Talebi, A. F., Moheimani, N. R., and McHenry, M. P. (2016). Potential use of algae for heavy metal bioremediation, a critical review. J. Environ. Manag. 181, 817–831. doi: 10.1016/j.jenvman.2016.06.059
Zhang, C., Show, P.-L., and Ho, S.-H. (2019). Progress and perspective on algal plastics - A critical review. Bioresour. Technol. 289:121700. doi: 10.1016/j.biortech.2019.121700
Zhang, X., Rong, J., Chen, H., He, C., and Wang, Q. (2014). Current status and outlook in the application of microalgae in biodiesel production and environmental protection. Front. Energy Res. 2:32. doi: 10.3389/fenrg.2014.00032
Zhang, X., and Thomsen, M. (2019). Biomolecular composition and revenue explained by interactions between extrinsic factors and endogenous rhythms of Saccharina latissima. Mar. Drugs 17:107. doi: 10.3390/md17020107
Zhu, L. (2015). Biorefinery as a promising approach to promote microalgae industry: an innovative framework. Renew. Sust. Energy Rev. 41, 1376–1384. doi: 10.1016/j.rser.2014.09.040
Keywords: Blue growth, seaweed, bioeconomy, biomass, production, algae
Citation: Araújo R, Vázquez Calderón F, Sánchez López J, Azevedo IC, Bruhn A, Fluch S, Garcia Tasende M, Ghaderiardakani F, Ilmjärv T, Laurans M, Mac Monagail M, Mangini S, Peteiro C, Rebours C, Stefansson T and Ullmann J (2021) Current Status of the Algae Production Industry in Europe: An Emerging Sector of the Blue Bioeconomy. Front. Mar. Sci. 7:626389. doi: 10.3389/fmars.2020.626389
Received: 05 November 2020; Accepted: 28 December 2020;
Published: 27 January 2021.
Edited by:
Seyed Hossein Hoseinifar, Gorgan University of Agricultural Sciences and Natural Resources, IranReviewed by:
Kit Wayne Chew, Xiamen University Malaysia, MalaysiaLuciane Maria Colla, The University of Passo Fundo, Brazil
Copyright © 2021 Araújo, Vázquez Calderón, Sánchez López, Azevedo, Bruhn, Fluch, Garcia Tasende, Ghaderiardakani, Ilmjärv, Laurans, Mac Monagail, Mangini, Peteiro, Rebours, Stefansson and Ullmann. This is an open-access article distributed under the terms of the Creative Commons Attribution License (CC BY). The use, distribution or reproduction in other forums is permitted, provided the original author(s) and the copyright owner(s) are credited and that the original publication in this journal is cited, in accordance with accepted academic practice. No use, distribution or reproduction is permitted which does not comply with these terms.
*Correspondence: Rita Araújo, rita.araujo@ec.europa.eu