Shallow Sea Gas Manifestations in the Aegean Sea (Greece) as Natural Analogs to Study Ocean Acidification: First Catalog and Geochemical Characterization
- 1Institute of Geosciences, University of Potsdam, Potsdam Golm, Germany
- 2GFZ German Research Centre for Geosciences, Potsdam, Germany
- 3Istituto Nazionale di Geofisica e Vulcanologia, Sezione di Palermo, Palermo, Italy
- 4Dipartimento di Scienze della Terra e del Mare, Università degli Studi di Palermo, Palermo, Italy
The concepts of CO2 emission, global warming, climate change, and their environmental impacts are of utmost importance for the understanding and protection of the ecosystems. Among the natural sources of gases into the atmosphere, the contribution of geogenic sources plays a crucial role. However, while subaerial emissions are widely studied, submarine outgassing is not yet well understood. In this study, we review and catalog 122 literature and unpublished data of submarine emissions distributed in ten coastal areas of the Aegean Sea. This catalog includes descriptions of the degassing vents through in situ observations, their chemical and isotopic compositions, and flux estimations. Temperatures and pH data of surface seawaters in four areas affected by submarine degassing are also presented. This overview provides useful information to researchers studying the impact of enhanced seawater CO2 concentrations related either to increasing CO2 levels in the atmosphere or leaking carbon capture and storage systems.
Introduction
The concentration of carbon dioxide (CO2) in the atmosphere is increasing mainly due to fossil fuel combustion and industrial processes. Since the beginning of the industrial revolution at the end of the eighteenth century, its level increased from about 280 ppm and exceeded the average yearly value of 413 ppm during the year 2021 (NOAA, 2021). Being one of the major greenhouse gases, such rapid increase has severe consequences on earth’s climate (IPCC, 2021). About one third of the anthropogenic CO2 released into the atmosphere in the past two centuries has been taken up by the ocean (Sabine et al., 2004; Bindoff et al., 2019; Gruber et al., 2019). In aquatic systems CO2 gas dissolves, hydrates and dissociates to form weak carbonic acid (Drever, 1997), and the pH is lowered according to the following reaction:
Current CO2 emission rates exceed the buffering capacity of the oceans and cause a shift of marine carbonate chemistry and a decrease of pH that has been quantified in 0.1 units compared to the pre-industrial period (Haugan and Drange, 1996; Doney et al., 2009; Gattuso and Hansson, 2011; Jiang et al., 2019). Depending on different emission scenarios, models predicted that further CO2 increase would cause an additional reduction of pH between 0.3 and 0.5 units by the end of the century (Caldeira and Wicket, 2005; Joos et al., 2011; Jiang et al., 2019). Business-as-usual CO2 emission scenarios predict that atmospheric CO2 will reach 750 ppm and pH levels will decrease to 7.8 by the year 2100 (Jiang et al., 2019). In addition to this, both surface temperature and heat content of the ocean have increased. Specifically at the ocean surface, temperature increased by 0.88°C on average from 1850–1900 to 2011–2020. Possible future scenarios anticipate that it will arrive at 0.86°C from 1995–2014 to 2081–2100 (IPCC, 2021). Similarly, ocean heat content increased by 0.28–0.55 YJ between 1971 and 2018 and will probably continue to increase until at least 2300 (IPCC, 2021). This projection applies also for low emission scenarios due to the slow circulation of the deep ocean.
Many studies evidenced that ocean acidification (OA) will exert significant and sometimes unexpected effects on marine ecosystems (Jiang et al., 2019). Because these changes decrease the saturation state of the carbonate ion (CO32–) in seawater, organisms relying on calcification for growth or protection are assumed to be most severely affected (Doney et al., 2012). On the contrary, photosynthetic organisms, such as seagrass and algae, may benefit from the increasing pCO2 which is an essential resource for their photosynthesis and survival (Fabricius et al., 2011; Koch et al., 2012; Russell et al., 2013). It should be mentioned that even though laboratory experiments documented the benefits of OA on seagrass growth, anthropogenic stressors might counterbalance positive effects of increased CO2 and have likely blocked potential beneficial responses of OA (Koch et al., 2012; Doo et al., 2020). To face the problem of atmospheric CO2 increase, apart from the most logical solution remaining the strong reduction of anthropogenic CO2 emission, one remedy proposed is the geologic carbon sequestration. CO2 capture and storage (CCS) systems concentrate and transfer liquid CO2 into storage sites, including sub-seabed deep geological formations such as exhausted oil or gas reservoirs. This approach is considered promising, since technically feasible (IPCC, 2005), but as with all other human technologies, it is not exempt from drawbacks. One of these drawbacks is the possibility that the chosen reservoir is not perfectly sealed and undergoes CO2 leakage (Monastersky, 2013). If these reservoirs are offshore, CO2 leakages from CCS can drive strong local seawater acidification (Blackford et al., 2014), exceeding the values predicted by the worst scenario of climate change. Moreover, in the case of a CO2 leak from a storage site, the gas will also acidify the pore water in the sediments surrounding the storage site (Millero et al., 2009). This may also increase the release of harmful elements from the sediments creating an additional negative impact on the marine environment (De Orte et al., 2014; Foo et al., 2018). Flohr et al. (2021) simulated CO2 leakage from an offshore CO2 storage site in the British sector of the central North Sea. The CO2 release experiment (Flohr et al., 2021; Gros et al., 2021) lasted for 1 month and the authors illustrated that different approaches can detect, attribute and quantify the release.
Notwithstanding the increasing number of studies on the ecological consequences of OA and CCS leakage, many issues remain unexplored and, until now, the vast majority of them have been performed in laboratories mainly as short-term and univariate experiments (Cornwall and Hurd, 2016). To have a more realistic picture, experiments should be made on marine organisms in their natural ecosystems. In this sense, areas with natural CO2 vents represent useful experimental locations to investigate the impact of OA on entire ecosystems (Hall-Spencer et al., 2008). Natural underwater vents of volcanic origin release gases composed mainly of CO2 and may therefore represent a natural analog to study the impact of seawater acidification. The CO2 vent areas are also perfect natural laboratories to study the impact of CO2 leakage from CCS systems.
Few of these “natural laboratories” have already been used to study the effects of elevated CO2 on ecosystems (Vizzini et al., 2010; Lauritano et al., 2015; Linares et al., 2015) sometimes evidencing the adaptation of complex ecosystems such as coral reefs (Golbuu et al., 2016; Teixidó et al., 2020).
These vent sites allow to study different habitats, including shallow coral reefs in Papua New Guinea, Japan, and Northern Mariana Islands (Enochs et al., 2016; Golbuu et al., 2016); seagrass meadows, macroalgae stands, and coralligenous in the Mediterranean Sea (Columbretes Islands, Spain—Linares et al., 2015; Ischia, Italy—Hall-Spencer et al., 2008; Vulcano, Italy—Boatta et al., 2013; Panarea, Italy—Rogelja et al., 2016; Methana, Greece—Baggini et al., 2014); as well as in the subtropical North East Atlantic reefs (La Palma, Canary Islands—Hernández et al., 2016). However, a larger representation of environments is needed to predict the biological and ecological consequences of OA.
Our study will give a first catalog of the gas vents within the Aegean Sea comprising a description of the areas. It will provide important information to researchers who study the impact of enhanced seawater CO2 concentrations related to increasing CO2 levels in the atmosphere or even to leaking CCS systems like (i) extension and morphology of the exhaling area; (ii) preliminary gas flux estimations and geochemical characterization of the gases; (iii) presence of possible confounding factors as for example emission of thermal waters and/or hydrogen sulfide, iron oxi-hydroxide flocculation. The geochemical characterization is based almost exclusively on literature data that are gathered together with some new results and are made available to the reader in Supplementary Table 1, while a description of the degassing sites and a rough estimation of the gas fluxes are available in Table 1. We also present unpublished data on pH and temperature measurements of surface seawaters in four areas affected by the submarine degassing (Supplementary Table 2).
Study Area
The Aegean Sea (Figure 1) is located in the eastern Mediterranean and is a rift formed in a “backarc” setting. It is situated in the upper plate of the Hellenic subduction zone and west of Anatolia, where active tectonics is observed. In fact, the northern Aegean Sea is a part of the Eurasian plate and the boundary with the Aegean microplate is called the North Anatolian Trough (NAT). The latter is the continuation of the North Anatolian Fault Zone (NAFZ) and is a ∼300 km long system of tectonically active marine basins, up to 1,000 m deep (Le Pichon et al., 1987; Taymaz et al., 1991; Kreemer et al., 2004).
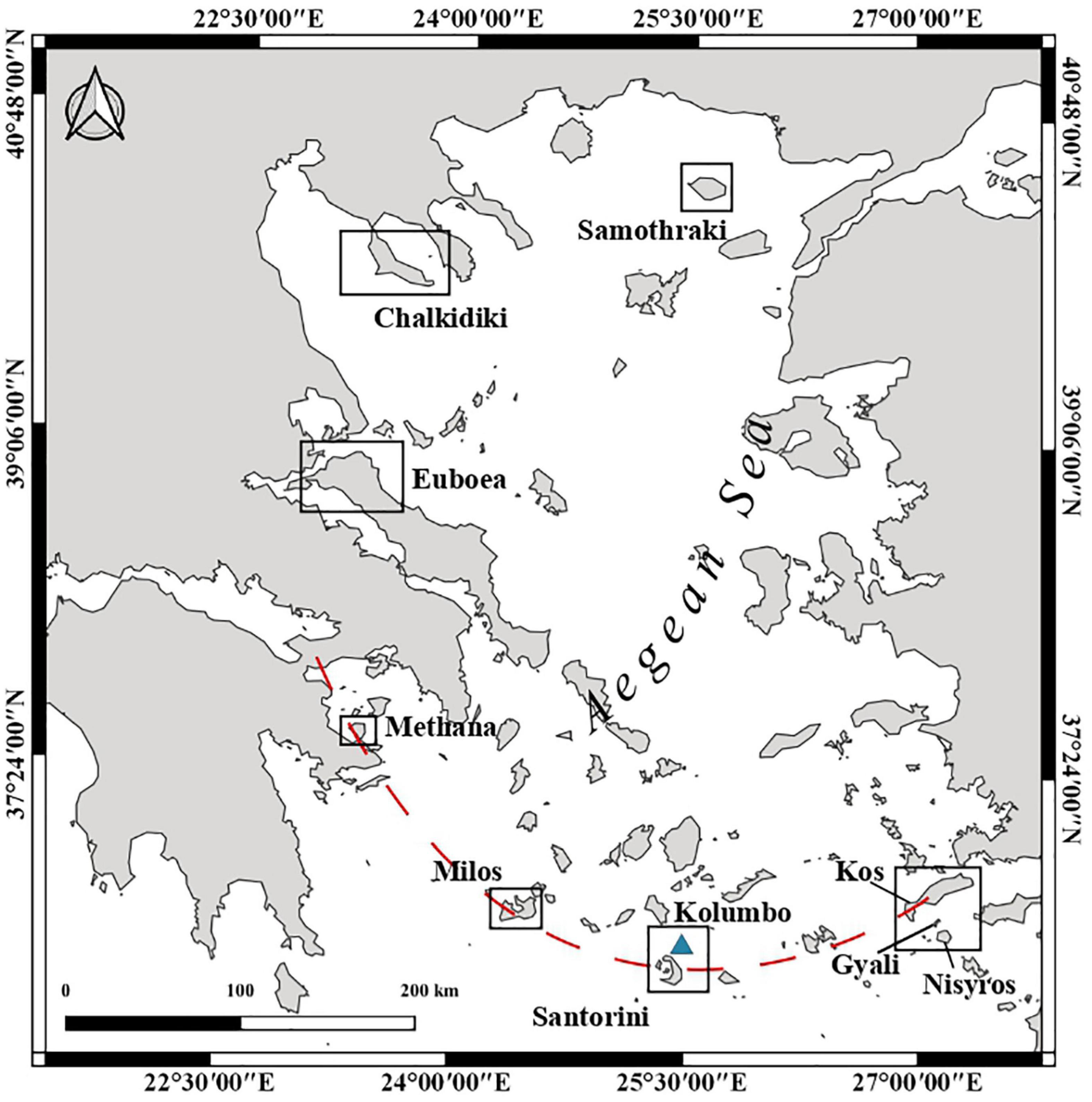
Figure 1. Map of the Aegean sea where the south Aegean active volcanic arc (SAAVA) is drawn with a dashed red line. Study areas are delimited in black squares, while Kolumbo is marked with a light blue triangle. Details on the study areas are found in section “Geological and Geochemical Description of the Submarine Degassing Areas and in situ Observations”.
The thinning of various tectonic units mainly emplaced during the Upper Cretaceous– Paleocene convergence–collision processes has resulted in the creation of the basin (Boccaletti et al., 1974; Robertson et al., 1991). It should be noted that the Hellenic subduction system was active since at least the Late Cretaceous, while the “backarc” rift was developed during Eocene-Early Miocene (Agostini et al., 2010; Jolivet et al., 2013). Despite the long-lasting formation of the Aegean basin (∼40 Ma), the extension rate is relatively low, so that the oceanic crust was not generated (Agostini et al., 2010).
Nowadays, the extension is seemingly localized around the Corinth-Patras rift (southern Greece), however; it was widespread during the Miocene (Sébrier, 1977; Mercier et al., 1979). Oligo-Miocene extensional metamorphic complexes outcrop in the Cyclades archipelago and the northern Aegean Sea (Lister et al., 1984; Gautier et al., 1993). The extension has proceeded from north to south, while the subduction front was retreating southward (Lauritano et al., 2015).
This geodynamically active regime is also characterized by intense seismic activity (Taymaz et al., 2007), by the presence of the south Aegean active volcanic arc (SAAVA) (Fytikas et al., 1984) and anomalous geothermal gradients (Fytikas and Kolios, 1979). Similar to other regions of intense geodynamic activity, extensive geogenic degassing takes place (Daskalopoulou et al., 2018a, 2019a) with gas manifestations being widespread both on land and underwater.
Geological and Geochemical Description of the Submarine Degassing Areas and in situ Observations
A total of 10 areas characterized by submarine degassing were documented and sampled along the Aegean Sea. Table 1 summarizes the general characteristics of the underwater sampling sites. A brief description of the degassing sites and in situ observations documented during the field campaigns are presented in this paragraph. Where possible, underwater filming allowed us to document and describe the degassing areas, and estimate the gas fluxes (as described in Supplementary Material).
Samothraki Island
The island of Samothraki is located at the NE part of the Aegean Sea of Greece (Figure 1) and belongs to the Circum Rhodope Zone (Kauffmann et al., 1976). It comprises five lithological units, which include: (i) low-grade metamorphic rocks (basement unit), (ii) an ophiolitic complex, (iii) a granite intrusion with biotite and a contact metamorphic event, (iv) Cenozoic volcanic rocks, and (v) Quaternary clastic sedimentary rocks (Kotopouli et al., 1989; St. Seymour et al., 1996). The rough relief with steep slopes characterizing the SSE part of the island is the result of the tectonic uplift movements, whereas natural weathering and erosion are responsible for the geomorphology (Pavlidis et al., 2005).
Sparse emission points characterized by ambient temperatures are found within the fisherman port of Therma (Figure 2A). The manifestations are rich in CH4 (72.7% on average-Supplementary Table 1), while CO2 is also present (23.8% on average-Supplementary Table 1). The flux of the bubbles is low (Table 1), and the gas manifestations, which are spread on an area of a few hundred m2, are permanent.
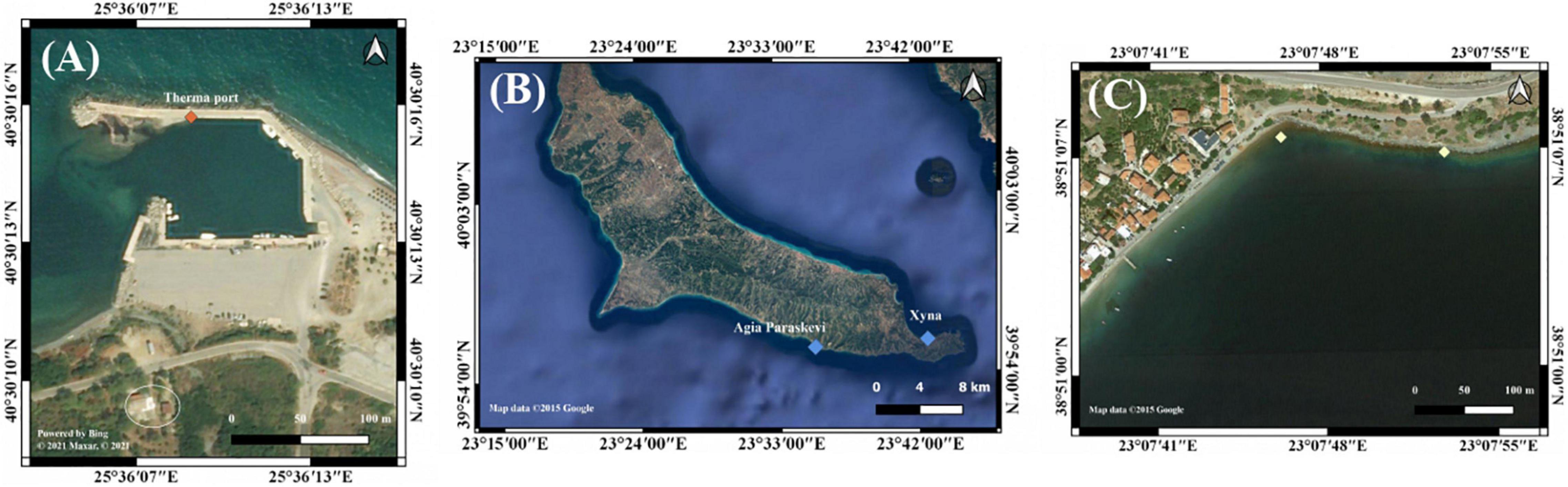
Figure 2. (A) Map of the north section of Samothraki Island that shows the location and name of the emission point. The circled area corresponds to the degassing points of Pigi A and B, and Giotrisi at the hydrothermal system of Therma (Dotsika, 2012; Daskalopoulou et al., 2018a). (B) Map of the Kassandra Peninsula that shows the locations and names of the emission points. (C) Map of the Ilion area (Euboea) that shows the location of the sampling points.
This manifestation seems to have no relationship with the nearby (800 m south) on-land hydrothermal system of Therma (Figure 2A) that reaches emission temperatures up to 74°C (Dotsika, 2012) and whose bubbling gases have CO2-rich composition (Daskalopoulou et al., 2018a). On the contrary, its CH4-rich composition points toward a hydrocarbon reservoir like those that are widespread and exploited in the north Aegean Sea (Rigakis et al., 2001).
Chalkidiki Peninsula
Chalkidiki peninsula is located at NNW part of the Aegean Sea (Figure 1) and is a part of the Vardar-Axios Zone, and the Serbomacedonian and Rhodope Massif (from west to the east) (Kauffmann et al., 1976). The area of interest is situated at Kassandra peninsula in Vardas-Axios zone, with the latter being considered as a narrow fragment of the Serbomacedonian Massif (Kockel et al., 1977). Despite the various metamorphic facies, the zone mainly comprises granitic intrusions of Upper Jurassic age and carbonates of a similar age enclosing bauxite horizons (Mountrakis, 1985). The tectonic regime of the area is mainly influenced by Thermaikos gulf, which is the relic of an older larger elongated tectonic depression trending from NNW to SSE.
Underwater degassing takes place in two areas at the Kassandra Peninsula (Figure 2B). The first emission site is found in Agia Paraskevi in front of “Halkidiki Thermal Spa” hotel. The two neighboring main degassing points can be visually recognized from the hotel due to a lighter color with respect to the main sea body; they appear like large stains in the sea. One of the main degassing areas is next to the coast close to the thermal springs on land. The springs are at sea level within some small caves (Lazaridis et al., 2011). Bubbling gases sometimes occurred also inside the caves but are more widespread in the sea. In this area, the gases come up from a very shallow depth (<1 m) between the boulders that form the shore. Another degassing area is at some tens of meters from the coast. Here intense degassing occurs at about 5 m depth. Gas emission vents form some recognizable alignments and are probably accompanied by thermal water emission because most of the orifices are surrounded by white deposits. It is worth noting that H2S is present both within the cave and in the two underwater degassing areas (Supplementary Table 1). The degassing is constant and the flux elevated (Table 1), especially at the area further from the coast. Here H2S, although below the analytical detection limit (<10 ppm) in the sample collected close to the sea surface, could still be smelled in the atmosphere above the bubbling site. Considering that H2S is highly soluble in water only where bubbling is very intense it may reach the surface after crossing 5 m of seawater.
The second degassing spot is found on the eastern coast of the Chalkidiki peninsula in Xyna. It is near the shoreline at the eastern end of a 3 km long sandy beach at the border with a private luxury resort. The gas flux is very low (Table 1). In correspondence to the bubbling site, on the beach (5 m from the shore) there is a small hypothermal spring (23°C) captured with a shallow well.
Euboea Island
The island of Euboea is found at the western part of the Aegean Sea (Figure 1) and is the second largest island of Greece. It consists of formations from the Sub-Pelagonian structural zone, while its southern part belongs to the Atticocycldic massif. Volcanism of Pliocene and Quaternary age took place in the area (Fytikas et al., 1976; Pe-Piper and Piper, 1989, 2002) contributing to the formation of geothermal fields. The major fault structures of the North Euboean Gulf, where the underwater vents are found (Figure 2C), comprise several segments of normal faults, trending about NW-SE and dipping NE with a total length of about 20–30 km (Pavlides et al., 2004).
Widespread underwater manifestations are found a few meters by the coast in the area of Ilion (Figure 2C). The widespread bubbling is constant and the flux, according to our estimation, is classified intense (Table 1). Hydrogen sulfide is present in minor concentrations (Supplementary Table 1), while the rusty color of the sediments suggests the existence of iron oxides deposition. It is worth noting that low pH values have been documented along the coast, with the lowest values being found in front of a high temperature and intensely degassing spring on land 10 m from the sea (Supplementary Table 2).
Methana Peninsula
Methana peninsula is located in Saronikos Gulf and represents the northwestern, still active part of the SAAVA (Figure 1). It belongs to the Atticocycladic zone and consists of Quaternary calc-alkaline volcanic rocks (andesites to dacites; Fytikas et al., 1986). Two different Pliocene-Quaternary tectonic domains within the Aegean plate are affecting the tectonic regime; a rapid N–S extension to the north and an E–W extension to the south (Jolivet et al., 2013).
Around the peninsula, two areas of submarine gas emissions have been recognized so far (Figure 3A). The first one is located in the northern part of the peninsula, where the “Pausanias baths” are found. These baths are associated with hydrothermal degassing emissions composed of almost pure CO2 (D’Alessandro et al., 2008). They are found at about 3 m from the coast in a depth of 2 m. The seabed is formed of boulders and the flux is considered low (Table 1). Baggini et al. (2014) state that along the entire northern coast, the measured pH values of seawater are lower and highly variable with respect to their reference site. Local fishermen reported intermittent gas bubbling close to the coast about 3 km west of the Pausanias baths. This degassing site has not been confirmed and no gas sample has been collected. It may be related either to the historical eruption of 230 BCE, whose lava flow entered the sea in that area, or to the nearby active submarine volcanic edifice called Pausanias (Foutrakis and Anastasakis, 2018). In any case, the sea close to the Pausanias baths has been the site of many studies to investigate the effect of higher pCO2 values on the local ecosystem (Baggini et al., 2014, 2015; Bray et al., 2014; Triantaphyllou et al., 2018; Patoucheas et al., 2021).
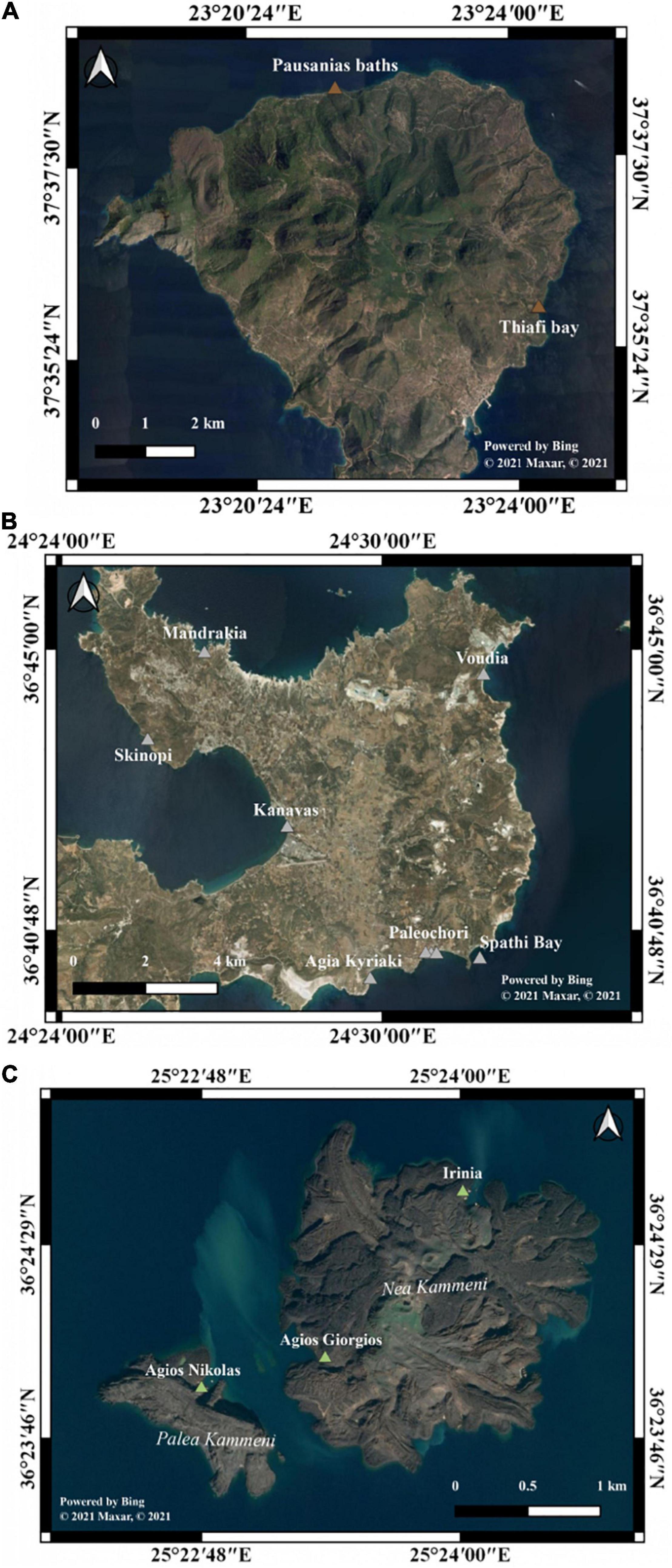
Figure 3. (A) Map of the Methana Peninsula that shows the locations and names of the emission points. (B) Map of the western section of Milos Island that shows the locations and names of the emission points. (C) Map of the Kammeni Islands that shows the locations and names of the emission points.
The second submarine vent is situated in the eastern part of the peninsula in a small bay called by the locals “Thiafi bay.” This area is nearly 300 m long and is demonstrating on the beach widespread alteration from recent fumarolic activity. The alteration is particularly evident at its northern and southern ends, where it is expressed as native S and sulfates (alunite, gypsum, and alunogen) (Rahders et al., 1997). CO2 fluxes on land are sometimes elevated and account for the whole area for about 500 t/a (D’Alessandro et al., 2008). The underwater gas vents show very low fluxes and are visible at shallow depths (<3 m) (Table 1) close to the coast (from the shoreline to distances of a few tens of meters). Few of these vents have a distinguishable orifice, and only some of them are associated with obvious deposits (possibly amorphous silica), whereas no thermal anomaly has been found at the gas vents (D’Alessandro et al., 2008).
In both degassing sites, CO2 is the prevailing gas component (up to ∼98%), while H2S was documented only in Thiafi (Supplementary Table 1).
Milos Island
Milos Island is found in the center of SAAVA (Figure 1) in the convergence zone between the African and the Aegean plates. It belongs in the Atticocycladic zone and comprises Upper Pliocene submarine and Upper Pleistocene to Holocene submarine-to-subaerial calc-alkaline volcanic domes, lavas, and pyroclastic deposits (andesites, dacites, and rhyolites; Fytikas et al., 1986; Stewart and McPhie, 2006). The youngest (Upper Pleistocene) volcanic activity is located in the volcanic centers of Fyriplaka in the south and Trachilas in the north, which are also present-day exhaling areas (Fytikas et al., 1986). However, the seabed around the island is hydrothermally very active. This is particularly noticeable in eight areas, located along the eastern part of the island (Dando et al., 1995; Cronan and Varnavas, 1999; Daskalopoulou et al., 2018b; Ivarsson et al., 2019). Gas emissions have been studied in the following sites (clockwise starting from the north): Mandrakia, Voudia, Paleochori, Spathi Bay, Agia Kyriaki, DEH (Kanavas), Skinopi (Figure 3B).
The little center of Mandrakia stands just on the prominent central part of a gulf oriented to the north. In the eastern part of the gulf, just close to some boulders, in a sandy seabed with a large Posidonia grassland, some sparse emissions are present tens of meters away from the shoreline, at a depth of about 3 m (Table 1). The vents stand exactly on the direction of the impluvium present on land and emit mainly CO2 (about 98%).
Voudia bay has two submarine hydrothermal vents. One of them is situated few meters away from the shoreline aligned with altered rocks on the beach, while the other one is found in the southern part of the bay. According to Megalovasilis (2020), the temperatures of the vents range from 28 to 78°C. Daskalopoulou et al. (2019b) collected samples from the emanations spot and reported a CO2-rich gas (96.6%), with minor contents of H2S (3,100 μmol/mol; Supplementary Table 1). Evidence of sulfur yellow-concretions were noted on the walls of some degassing vents (Supplementary Figure 2). While sampling, the same authors have observed a relatively low flow (Table 1), which was afterwards confirmed by the documentation of Megalovasilis (2020).
Paleochori Bay is an 800 m long bay with apparent fumarolic activity at its eastern and western parts. Numerous intensively degassing seeps of elevated temperatures (up to 122°C; Dando et al., 1995; Khimasia et al., 2020) occur in the area. The emanating gases are rich in CO2 (up to 93%; Supplementary Table 1) with minor enrichments in N2 (up to 14%; Supplementary Table 1). H2S is also present in concentrations up to 3.5% (Supplementary Table 1). The area is characterized by Fe- and S-alteration products (Cronan and Varnavas, 1999; Baltatzis et al., 2001; Voudouris et al., 2021). According to various authors (Dando et al., 1995; Yücel et al., 2013; Khimasia et al., 2020), the sea-bottom of the bay is dominated by areas of white and brown bacterial mat (Supplementary Figures 2C,D). Areas of gray/yellow sand with encrustations and of light brown sand with numerous burrows are also present. Godelitsas et al. (2015) suggested that the reddish or yellow-colored sediment patches in the center of the white mats might have been caused by elemental sulfur (Supplementary Figure 2C) and arsenic sulfides precipitation. It is worth noting that the gas ascends from rock fissures at a depth of about 2–3 m, resulting in bacteria-dominated outlets known as “White Smoker” (Supplementary Figure 2E). This degassing area is distinguished by the highest gas fluxes estimated in this study (about 1.8 L/min) (Table 1). It is important to note that the hydrothermal fluids release to the seawater huge quantities of potentially toxic elements. For example, several studies revealed concentrations of the order of thousands of μg/L of As, Ba, Fe, and Mn, and up to 1 μg/L of Hg (Price et al., 2013; Roberts et al., 2021) leading to the formation of hydrothermal precipitates rich in these and other (Sb, Tl) elements (Voudouris et al., 2021).
Spathi bay is located in the south-eastern sector of the island and extends for about 400 m. The coastline is characterized by pebbles and is bordered to the west by an imposing promontory and to the east by striking stacks. We do not have detailed information on the underwater emissions as the gas sample was kindly taken by colleagues.
Agia Kyriaki is located in the southern sector of Milos, in a bay that stretches for about 500 m. The coast is predominantly sandy and the seabed is characterized by alternating sandy areas and reefs. The only underwater gas manifestation in this area is found at less than 10 m from the shoreline, where a few isolated bubble-trains with a low flux outcome from a substrate of rock blocks, at a depth of about 2–3 m (Table 1). H2S was present in concentrations lower than 10 μmol/mol (Supplementary Table 1).
DEH is located in the sea along Kanavas coast close to the power plant of the Hellenic Public Power Corporation. The degassing area is about 400 m2 with a CO2 output estimated at 1.06 t/d (Daskalopoulou et al., 2018b). The numerous and widespread gas manifestations have low temperatures with H2S being less than 16 μmol/mol (Supplementary Table 1). The sea bottom is sandy and very shallow (about 1–2 m) and clear alignments of high-flux degassing vents from fissures were noted (Supplementary Figure 2A and Table 1). The environment is disturbed by many human activities, i.e., a shipyard 500 m north, abandoned salt flats about 200 m south, and the power plant less than 100 m away which discharges its cooling water about 50 m from the bubbling area.
Skinopi is a small bay with a 100 m long pebble beach on which stand some characteristic houses and small jetties for fishermen’s boats. It is located 1,500 m west of Adamas, the main port of the island. Close to the coast (tens on meters) there are many bubbling areas. The degassing area is shallow (<2 m) and not very active (low fluxes) (Table 1), while the sandy and boulders sea bottom is mostly covered by Posidonia grasslands. The gases are mainly composed of CO2 (>82%), and H2S is undetectable (Supplementary Table 1).
Santorini Island
Santorini volcanic complex is found in the center of the SAAVA (Figure 1). It comprises the islands of Thera, Thirasia, Palea Kammeni, Nea Kammeni, and Aspronisi, and belongs to the Atticocycladic zone. The complex consists of volcanic rocks (mainly pumice and glass) and metamorphic formations (mainly marbles and phyllites) (Druitt et al., 1989, 1999; Oikonomidis and Pavlides, 2017). The evolution of the volcanic centers is associated with two NE-SW faults; the Kammeni Line (Heiken and McCoy, 1984; Druitt et al., 1989, 1999; Parks et al., 2013) and the Columbo Fault Zone (Druitt et al., 1989, 1999; Mountrakis et al., 1998). In addition to this, Tzanis et al. (2020) demonstrated that both volcanism and the shape of the volcanic center are controlled by the tectonics.
Submarine gas vents are located in Palea and Nea Kammeni islets (Figure 3C). The emission point in Palea Kammeni is in the bay of Agios Nikolaos and is located close to the coast in the eastern part of the island. Two emissions have been documented in the Nea Kammeni island. One is called Agios Giorgios and is found on the western side of the island, while Irinia is on the eastern side where most of the island visitors are disembarked. Both Agios Nikolaos and Agios Giorgios are CO2 dominated (Chiodini et al., 1998; Tassi et al., 2013; Daskalopoulou et al., 2018a) and present H2S content up to 26 and 415 μmol/mol, respectively (Supplementary Table 1). The outlet temperatures of the two points are 36 (Agios Nikolaos) and 40°C (Agios Giorgios) (Böstrom and Widenfalk, 1984; Dotsika et al., 2009). On the other hand, Irinia shows a mixed CO2-N2 composition (Daskalopoulou et al., 2018b; Tarchini et al., 2019). All three sites are in protected coves where the seawater is heavily stained by iron oxides due to the input of the hydrothermal fluids. In the deepest parts of the coves, iron concentrations in seawater exceed 1 mg/L (up to 13.7 mg/L; Smith and Cronan, 1983). The iron, solubilized by the low pH CO2-rich hydrothermal fluids, becomes oxidized on mixing with seawater and precipitates accumulating abundantly at the bottom of the exhaling areas (Smith and Cronan, 1983).
Kolumbo
Kolumbo is a submarine volcano found 7 km northeast off Santorini island (Figure 1). It is a high-temperature hydrothermal field (Sigurdsson et al., 2006) characterized by numerous vents of CO2-rich gases (<97%) and fluids of ∼220°C (Carey et al., 2011). Despite its proximity to Santorini, volcanological and petrological evidence suggest the existence of two separate plumbing systems beneath the two volcanic edifices (Francalanci et al., 2005; Dimitriadis et al., 2009; Kilias et al., 2013). In addition to this, Rizzo et al. (2016) has documented a more than 85% mantle contribution for He (the highest across SAAVA). Gases collected in Kolumbo are CO2 dominated (>98% on average; Carey et al., 2011; Rizzo et al., 2016, 2019; Supplementary Table 1).
Kos Island
Kos Island is located in the eastern part of the SAAVA (Figure 1). It comprises alluvial deposits with greenschists and flysch in the north, lacustrine and terrestrial deposits of the Pliocene age in the central part, while tuffs and ignimbrites of the Quaternary age are found in the south (La Ruffa et al., 1999). Faults of WNW-ESE and NE-SW orientation seem to control the tectonic evolution of the island and to be related to extensional processes and volcanic activity during the Pleistocene and Pliocene (Lagios et al., 1998). In the area, four submarine degassing centers have been recognized (Daskalopoulou et al., 2019b). Paradise beach and Kefalos are located in the western part of the island, while Therma and Agia Irini in the eastern (Figure 4A).
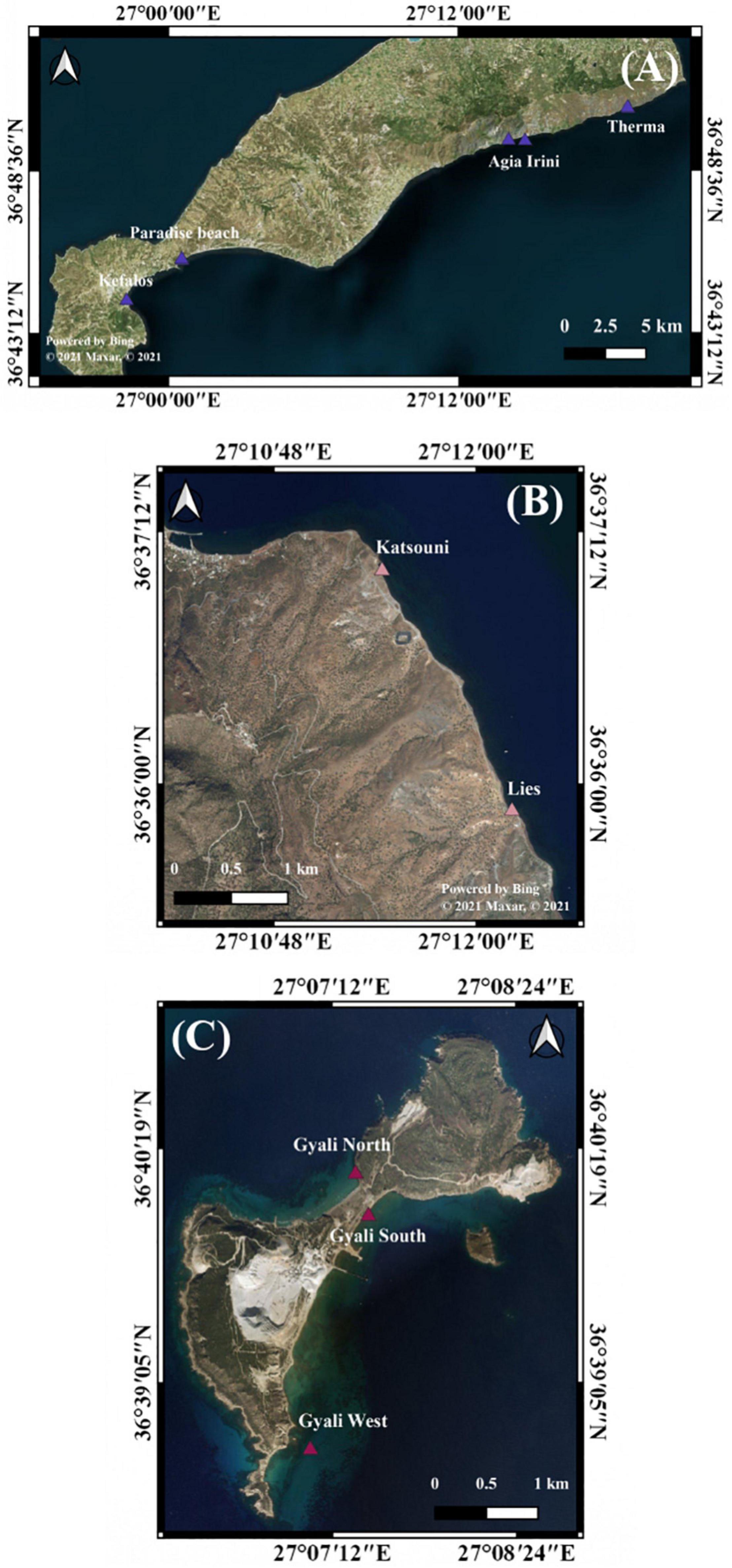
Figure 4. (A) Map of the southern sector of Kos Island that shows the locations and names of the emission points. (B) Map of the NE sector of Nisyros Island that shows the locations and names of the emission points. (C) Map of Gyali Island that shows the locations and names of the emission points.
The submarine emissions of Paradise beach are rich in CO2. H2S is always below detection limit (Supplementary Table 1). The vents are found at approximately 20 m from the coast at 1–1.5 m depth. They are widespread and having elevated gas flows (Table 1).
The marine area of Kefalos at the SW of the island is interested by diffuse degassing, with a lot of bubble streams mainly concentered just to the east of the harbor area. Hundreds of little vents that emit trains of little bubbles are present in a sandy seabed at a depth of few meters.
Submarine gases of Therma present similar chemical characteristics to the gases of Paradise. However, they are found by the coast and are characterized by elevated temperatures (up to 45°C).
Two degassing vents have been recognized in the sea in front of the Agia Irini church. Despite the vicinity of the emission points, their prevailing gas components differ significantly. One (Agia Irini 1) is rich in CO2 (>95%), while the other (Agia Irini 2) is rich in N2 (34–99%). At both sites, H2S is generally below detection limit (Supplementary Table 1).
Nisyros Island
Nisyros Island is found at the eastern end of the SAAVA (Figure 1) and is a quiescent active stratovolcano with intense fumarolic activity that is generated by the presence of a high enthalpy geothermal system (Marini et al., 1993). It belongs to the Atticocycladic unit and consists of Quaternary volcanic rocks and alternations of lava flows, pyroclastic deposits and lava domes. The island has an area of 47 km2 and forms a truncated cone with a base diameter of 8 km and a 4 km wide central caldera (Hunziker and Marini, 2005), known as the Lakki Caldera. Numerous, mostly sub-vertical faults crosscut the island and the caldera. The vertical offsets for the majority of these faults decrease from the caldera rim toward the coast, where they practically disappear (“scissors-type” faults); something that evidences their association with volcano-tectonic effects (Stiros, 2000).
Two points of submarine vents have been recognized in Nisyros (Figure 4B). Lies and Katsouni are two gas manifestations rich in CO2 (Supplementary Table 1). They are found few meters from the coast in < 2 m depth, where the sea bottom is mostly covered by boulders and pebbles. Both sites are characterized by low temperatures and medium to low gas fluxes.
Gyali Island
Gyali Island is located between the Islands of Kos and Nisyros (Figure 1). The small island is uninhabited except by workers for the extraction of pumice and occasional tourists visiting the picturesque bays. It consists of a thick rhyolitic pumice succession to the south (Gyali pumice breccia and overlying units) and rhyolitic lava to the north. These two formations are separated by an isthmus, which is found in the center of the island. According to Allen and McPhie (2000), the pumice breccia of Gyali is the result of a submarine phreatomagmatic eruption.
Three submarine vents have been recognized in the island (Figure 4C), in the area where the fault zones are located (Daskalopoulou et al., 2021a). The gas manifestations are rich in CO2, with H2S being found in minor content (Supplementary Table 1). Intense degassing activity is observed in both Gyali West and Gyali South, where the emissions are widespread at some tens of meters from the shore. In both areas the sea bottom is sandy, but while the former is at about 10 m depth the latter is shallower (1–3 m depth). The third site is less active in terms of degassing and is found very close to the shore. The bubbles rise at shallow depth (about 1 m) between large boulders.
Geochemistry of Submarine Gas Vents
In the current study, a total of 122 data from submarine gas manifestations are presented. This dataset comprises both literature (Chiodini et al., 1998; Shimizu et al., 2005; Kyriakopoulos, 2010; Carey et al., 2013; Tassi et al., 2013; Rizzo et al., 2016, 2019; Daskalopoulou et al., 2018a, b, 2019b, 2021a; Tarchini et al., 2019) and 7 unpublished results from submarine degassing areas distributed in ten areas of the Aegean Sea; seven of which belong to the SAAVA. Gases were collected using the inverted funnel method (Figure 5). Sample IDs, coordinates, references, gas flux estimations and their chemical and isotopic content are found in Supplementary Table 1. pH and temperature data of seawater affected by the submarine degassing are also presented in Supplementary Table 2. Details on the sampling techniques and the laboratory methods used are found in Methods section in Supplementary Data Sheet 1.
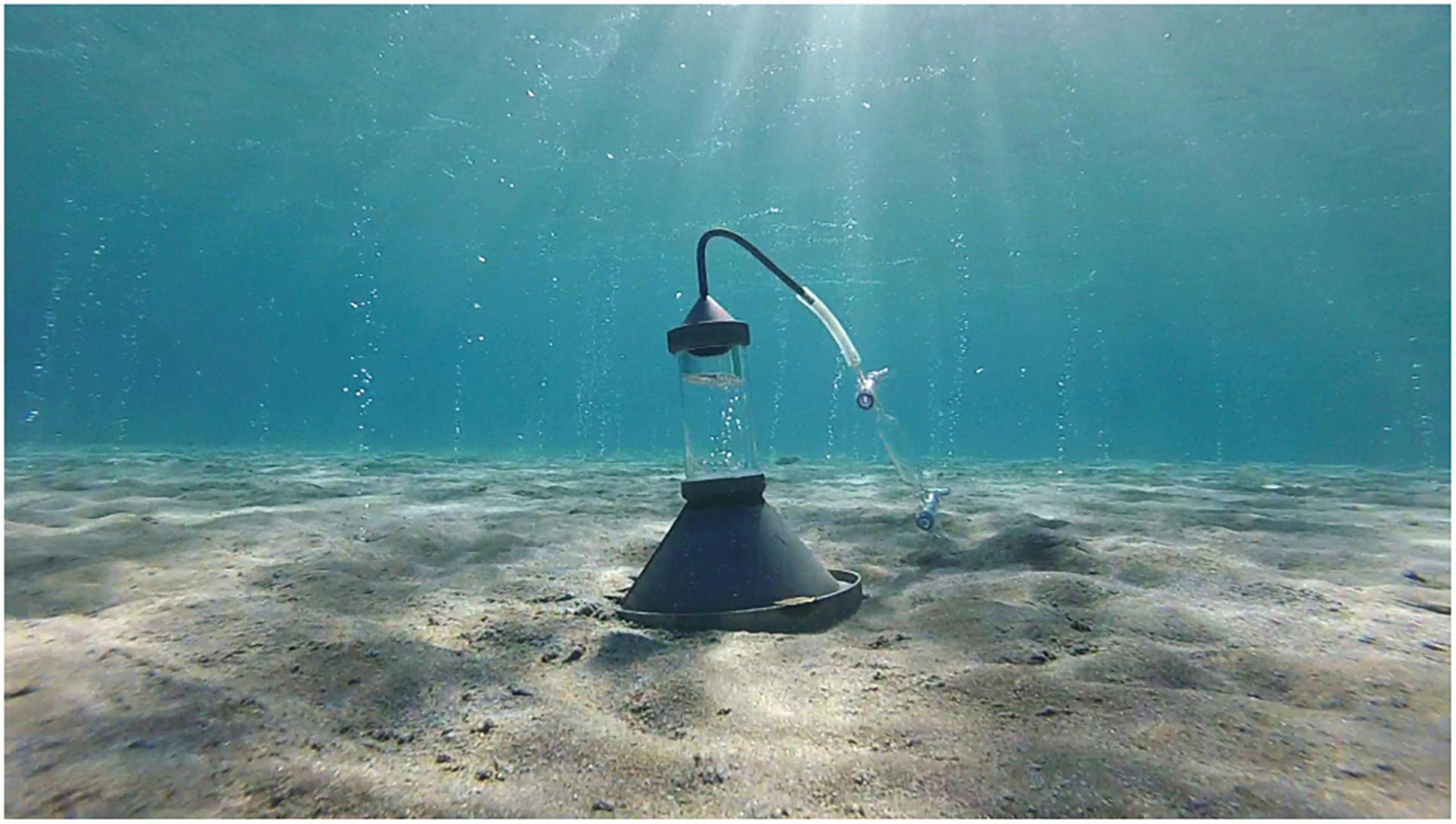
Figure 5. Gas sampling with the use of the inverted funnel method at Kefalos (Kos Island). Note that the funnel was constructed at the mechanical laboratory of Istituto Nazionale di Geofisica e Vulcanologia, Sezione di Palermo (INGV-Palermo). Additional photos regarding the sampling technique are provided in Supplementary Figure 1. Photo courtesy of SC.
No samples plot close to the atmospheric point (Kipfer et al., 2002) in the CO2-N2-O2 ternary diagram (air in Figure 6A), thus excluding important air contaminations. The vast majority of the samples present N2/O2 ratios higher than the ratios of air saturated waters (ASW; Kipfer et al., 2002), suggesting that the atmospheric component deriving from meteoric recharge has been modified by microbial or inorganic redox reactions that took place in the subterranean circuit. In their majority, gases are rich in CO2, while few samples have N2 or CH4 as the prevailing gas species (Figure 6B). Geographically, gases rich in N2 (Santorini, Nisyros, Kos, Methana) are distributed in the SAAVA and just after SAAVA to the north (Euboea), while CH4 prevails in the gas vents of north eastern Aegean Sea (Samothraki) (Daskalopoulou et al., 2018a, 2019a). Figures 6A,B show the occurrence of dissolution processes, with the latter being likely responsible for the CO2 loss and the enrichment of the less soluble gases (CH4, O2, and N2).
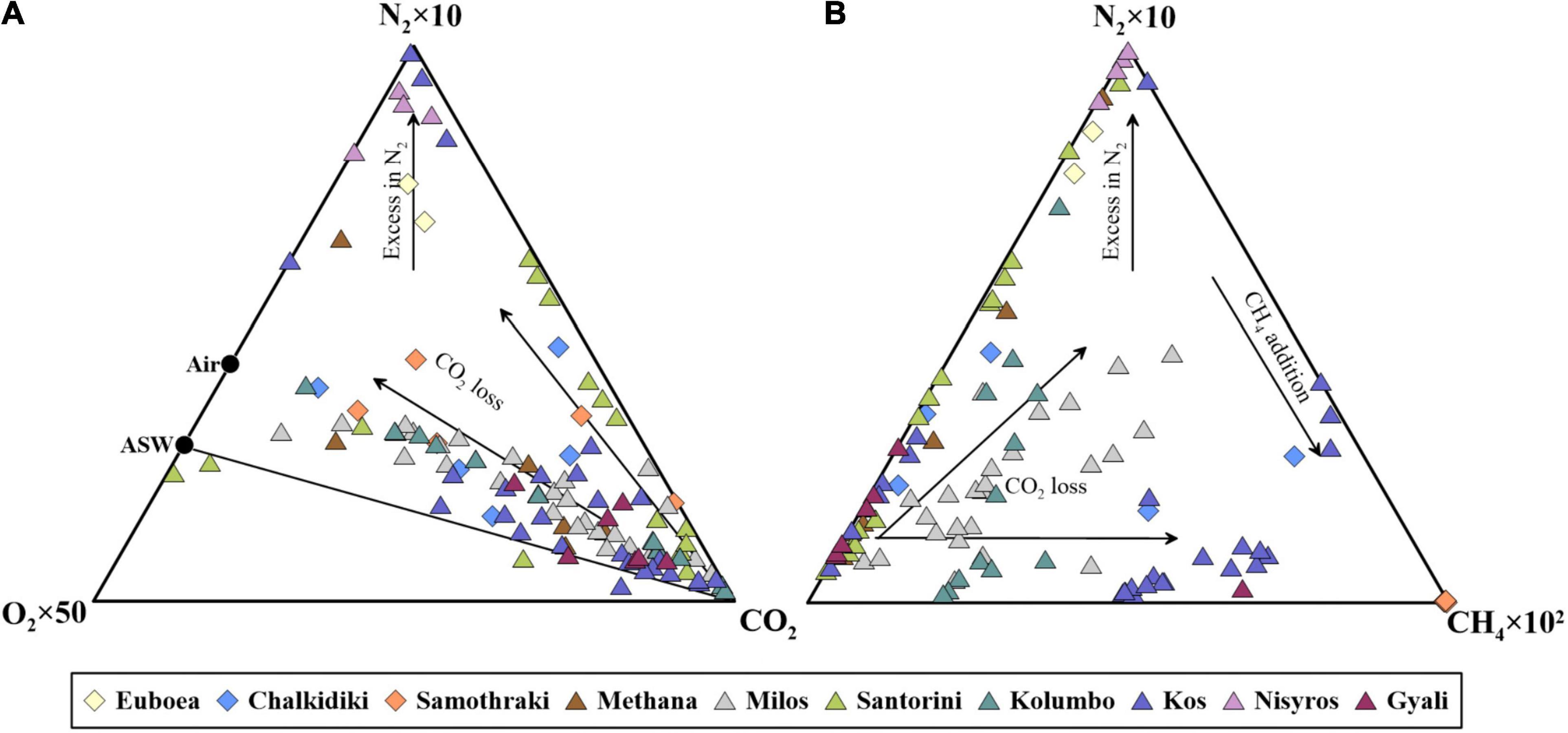
Figure 6. Ternary plot of (A) CO2-N2-O2 and (B) CH4-N2-CO2. Processes impacting the gases are drawn with an arrow. The abbreviation “ASW” stands for air saturated water. Values of air and air saturated water (ASW) after Kipfer et al. (2002). Literature data from Chiodini et al. (1998), Shimizu et al. (2005), Kyriakopoulos (2010), Carey et al. (2013), Tassi et al. (2013), Rizzo et al. (2016, 2019), Daskalopoulou et al. (2018a, b, 2019b, 2021a), and Tarchini et al. (2019).
An important atmospheric contribution for He is noticed for the gases of Samothraki Island as they plot close to the atmospheric point (Figure 7A, after Sano and Wakita, 1985). As expected, the vents located at the SAAVA present an enhanced MORB-type mantle contribution arriving up to ∼90% (Kolumbo), while the gases of non-volcanic areas show a more crustal origin for He (up to ∼95%). Figure 7B (Sano and Marty, 1995) reveals a mixed mantle-limestone origin for C for the great majority of the gases. The contribution of the organic sediment is relatively negligible. Some gases present CO2/3He ratios that fall below the Mantle field, indicating CO2 loss. This is likely due to the dissolution of CO2 in water or to the precipitation of carbonates (Kanellopoulos, 2012; Winkel et al., 2013; Stefánsson et al., 2016, 2017; Kanellopoulos et al., 2017). A change in the flow as observed from Daskalopoulou et al. (2019b) for the sample of Kos presenting a strong decrease in δ13C-CO2 may have also contributed to the dissolution processes. Methane for most submarine gases plots in the field ascribed to abiogenic hydrocarbons emitted from volcanic-geothermal systems (Figure 7C, after Etiope and Schoell, 2014). Contribution from biogenic sources cannot be excluded due to the wide range (from ∼4 to 244) of CH4/(C2H6+C3H8) ratios (Bernard et al., 1978). Some gases exhibit high δ13C-CH4 and δ2H-CH4 values. This points to either organic or inorganic CH4 oxidation processes. It is worth mentioning that isotope fractionation for organic and inorganic processes follow different fractionation paths (details in Daskalopoulou et al., 2018a, 2019b). Low δ2H-CH4 values of Milos can be explained by non-equilibrium fractionation of CH4-H with either H2O or H2 (Botz et al., 1996). Biogenic origin is attributed to CH4 for the area of Samothraki. In particular, low δ13C-CH4 and δ2H-CH4 values as well as intermediate CH4/(C2H6+C3H8) ratio indicate mixing between thermogenic and microbially-derived gases. The latter has been attributed to CO2-reduction by Daskalopoulou et al. (2018a) on the basis of carbon isotope fractionation factor between coexisting CO2 and CH4 (Whiticar et al., 1986).
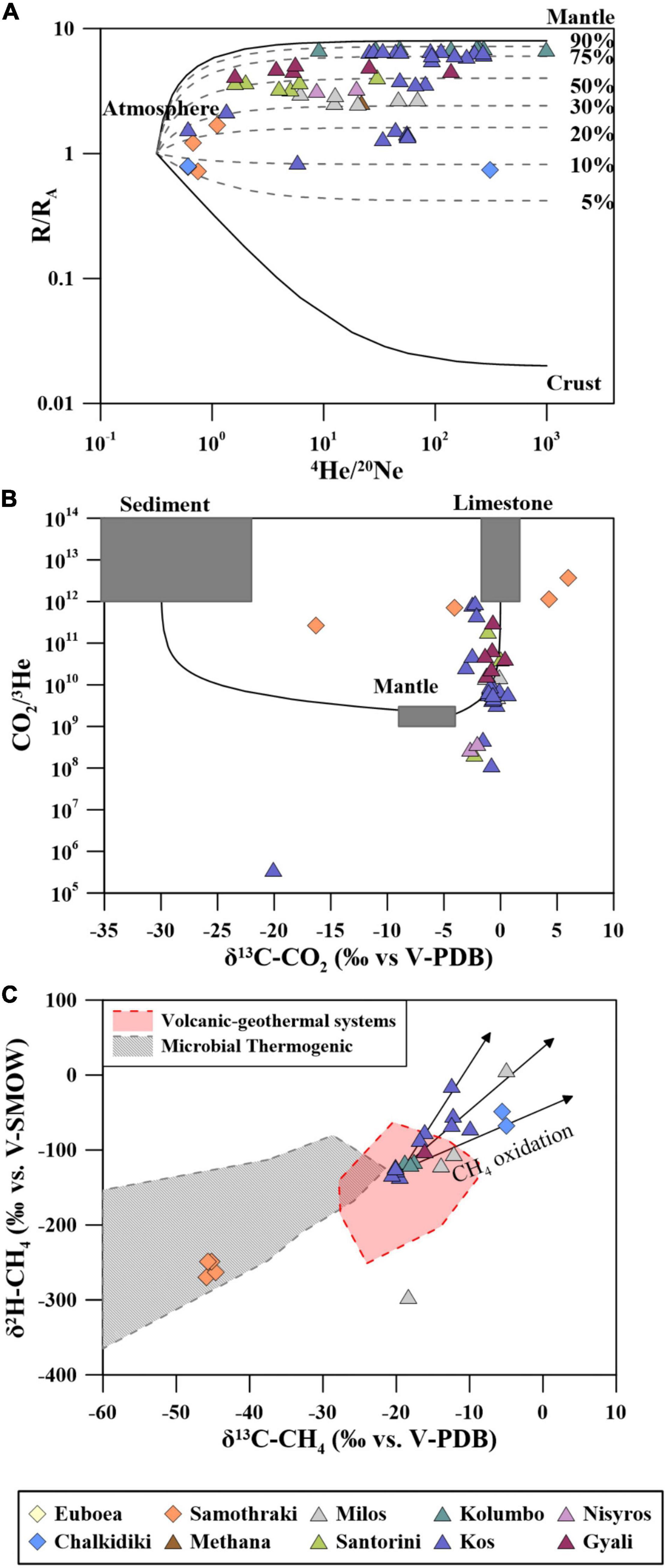
Figure 7. Binary plot of (A) R/RA vs. 4He/20Ne of the Hellenic gas emissions. The mixing lines between Atmosphere and Mantle and between Atmosphere and Crust are also plotted. Dashed lines represent mixing between atmosphere and end-members with different percentages of mantle contribution (after Sano and Wakita, 1985); (B) CO2/3He vs. δ13C-CO2. The composition for Sediments, MORB-like Mantle and Limestones end-members are, as follows: δ13C-CO2 = −30‰, −5‰ and 0‰ and CO2/3He = 1 × 1013, 2 × 109 and 1 × 1013, respectively (after Sano and Marty, 1995); and (C) modified Schoell binary diagram (Etiope and Schoell, 2014) between δ2H-CH4 and δ13C-CH4 ratios for the Aegean submarine gas discharges. Slopes of biogenic and abiogenic oxidation of CH4 are also plotted. Literature data from Chiodini et al. (1998), Shimizu et al. (2005), Kyriakopoulos (2010), Carey et al. (2013), Tassi et al. (2013), Rizzo et al. (2016, 2019), Daskalopoulou et al. (2018a, b, 2019b, 2021a), and Tarchini et al. (2019).
The CO2 loss is more evident in the binary plots of Figure 8. The positive correlation between He and N2 indicates the impact of the CO2 dissolution on the gases (Figure 8A). He and N2, as well as CH4 (Figure 8B), are less soluble respect to CO2, hence the strong solubility difference between the gases in the marine environment may has resulted in CO2 loss (Reid et al., 1987). In fact, D’Alessandro et al. (2014) showed that when a gas mixture ascends through non-saturated waters, solubility contrasts might enrich the less soluble gases. This is specifically applicable in reduced gas upflow conditions (Daskalopoulou et al., 2021b).
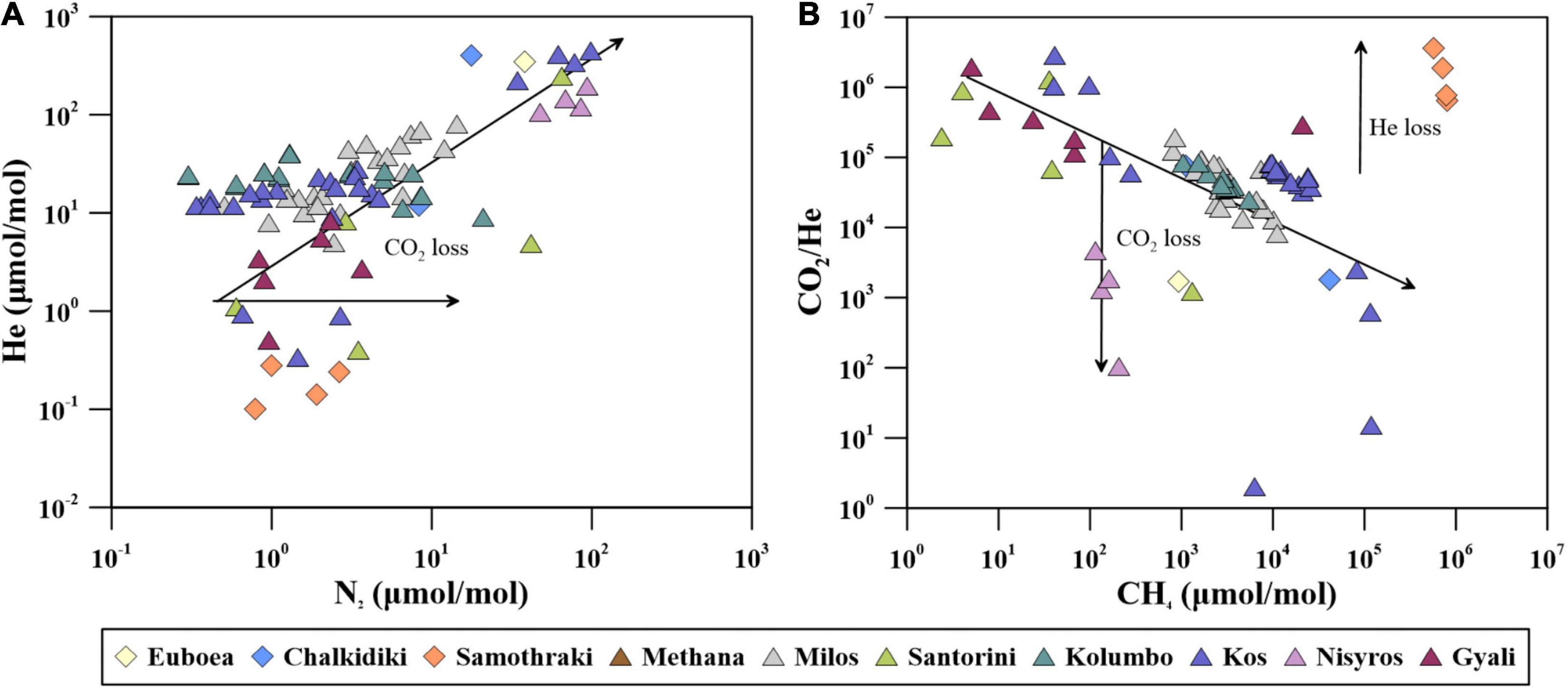
Figure 8. Binary plot of (A) He-N2 and (B) CO2/He-CH4. Processes impacting the gases are drawn with an arrow. Literature data from Chiodini et al. (1998), Shimizu et al. (2005), Kyriakopoulos (2010), Carey et al. (2013), Tassi et al. (2013), Rizzo et al. (2016, 2019), Daskalopoulou et al. (2018a, b, 2019b, 2021a), and Tarchini et al. (2019).
Figure 9 further evidences the impact of solubility-related processes on gas composition. The elevated CO2/H2S ratios of the gases in Kos, Gyali and Santorini demonstrate the interaction between magmatic gases and hydrothermal systems (Supplementary Table 1). This process, known as magmatic scrubbing (Symonds et al., 2001), occurs when ascending gases encounter any aquifer interposed between the source magma stored at depth and the surface (Di Napoli et al., 2016). The more water-soluble gas species dissolve due to the gas-water-rock interactions, thus modifying the composition of the primary magmatic gas phase. In their great majority, gases collected along SAAVA are poor in CH4. This scarcity evidences the lack of underlying organic-rich source rocks in these areas. Few gases of Milos, Kos and Santorini are virtually enriched in CH4 due to loss of CO2 by dissolution as evidenced also in Figure 8B. Finally, some gases of Chalkidiki, Milos, and Euboea plot close to the atmospheric point, further demonstrating the CO2 loss due to dissolution processes, but also indicating some contribution of the atmospheric component.
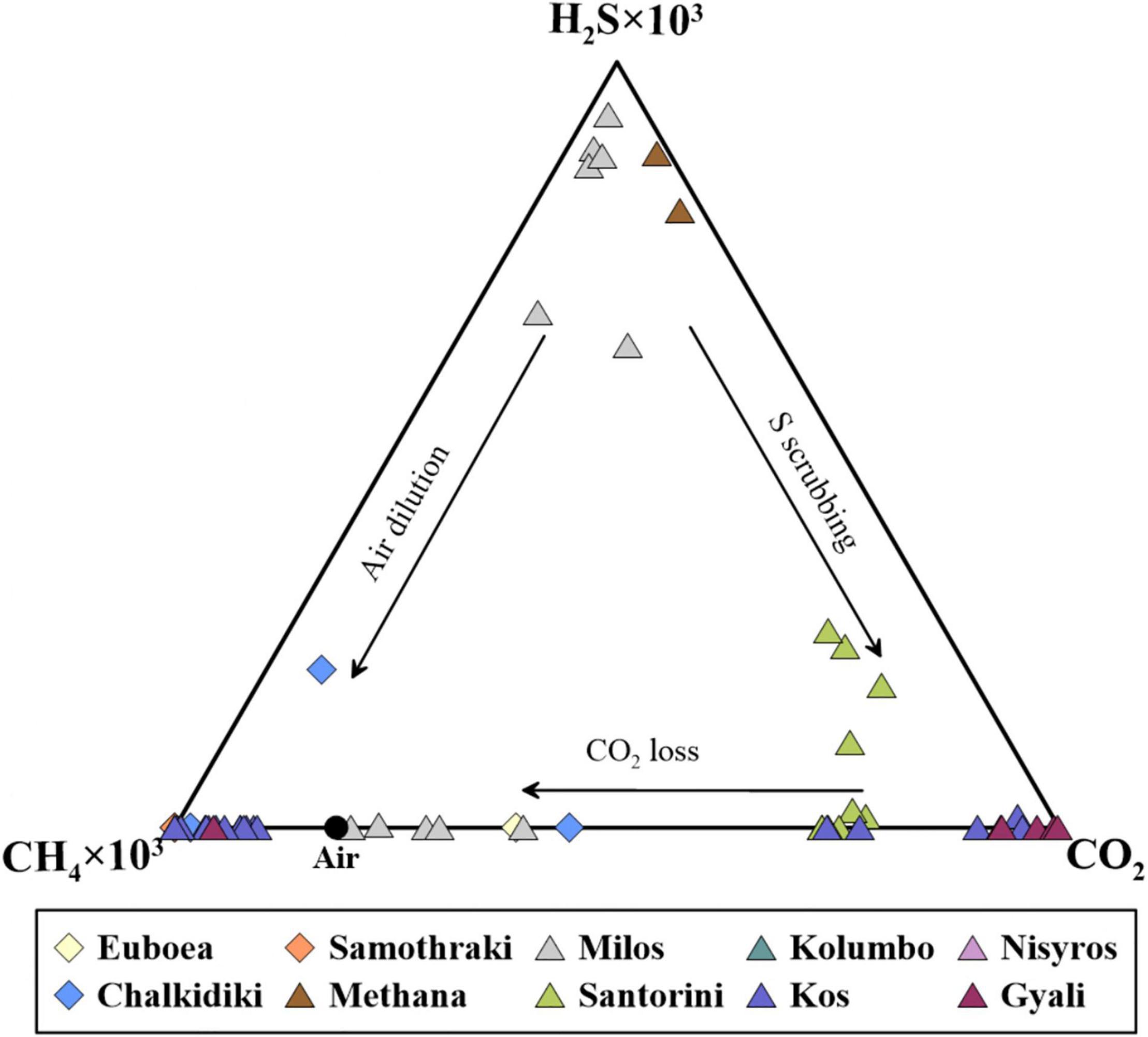
Figure 9. Ternary plot of CO2-H2S-CH4. Processes impacting the gases are drawn with arrows. Values of air after Kipfer et al. (2002). Literature data from Chiodini et al. (1998), Shimizu et al. (2005), Kyriakopoulos (2010), Carey et al. (2013), Tassi et al. (2013), Rizzo et al. (2016, 2019), Daskalopoulou et al. (2018a, b, 2019b, 2021a), and Tarchini et al. (2019).
In order to further demonstrate the impact of water-gas interactions on the gas content, two samples for each site were collected at the degassing centers of Agia Paraskevi (Chalkidiki) and Agia Irini 2 (Kos). One of the two samples was taken in the usual manner from the emission site at sea bottom (for details see “Material and Methods” section in Supplementary Data Sheet 1), while the second at the sea surface after the gas bubbles have risen through the entire water column. Results evidence that sea bottom samples have CO2 as the major component. In one case they show the presence of some H2S. The superficial samples have much lower CO2 concentrations, H2S always below the detection limit, and become enriched in N2, O2, He, and CH4 (Figure 10 and Supplementary Table 1). These, sometimes very strong changes, can be explained by two processes that drive the gas exchanges between the rising bubbles and the seawater: mixing between two end-members and fractionation due to different solubility. The first process accounts for the virtual enrichment of the less soluble gases of hydrothermal origin (He and CH4) with respect to CO2 (Figure 10A). The second process is responsible for the decrease of CO2 and H2S (hydrothermal end-member) and the increase in O2 and N2 (Air-saturated seawater end-member) (Figure 10B). The extent of the changes suffered by the ascending gases depends on many conditions, which are mainly temperature, area of the interaction surface and interaction time (i.e. distance to be covered from the sea bottom to the surface). In the case of dry gases (no water vapor) the temperature is generally that of seawater because even if the emitted gases are hot they rapidly equilibrate with the seawater temperature due to the water/gas mass ratio and the thermal inertia of water. Both the interaction surface area and interaction time strongly depend on gas flux, bubble dimension, and depth of the water column. Higher gas fluxes, greater bubble dimensions, and lower emission depths all reduce gas exchange between bubbles and water, limiting the changes in gas composition. In the case of the above mentioned sites, the high gas flux and shallow depth of Agia Paraskevi prevents strong compositional changes like those registered in the site of Agia Irini 2. For example, while in the first case 78% of the initial CO2 content arrives at the sea surface in the second case less than 1% does (Supplementary Table 1).
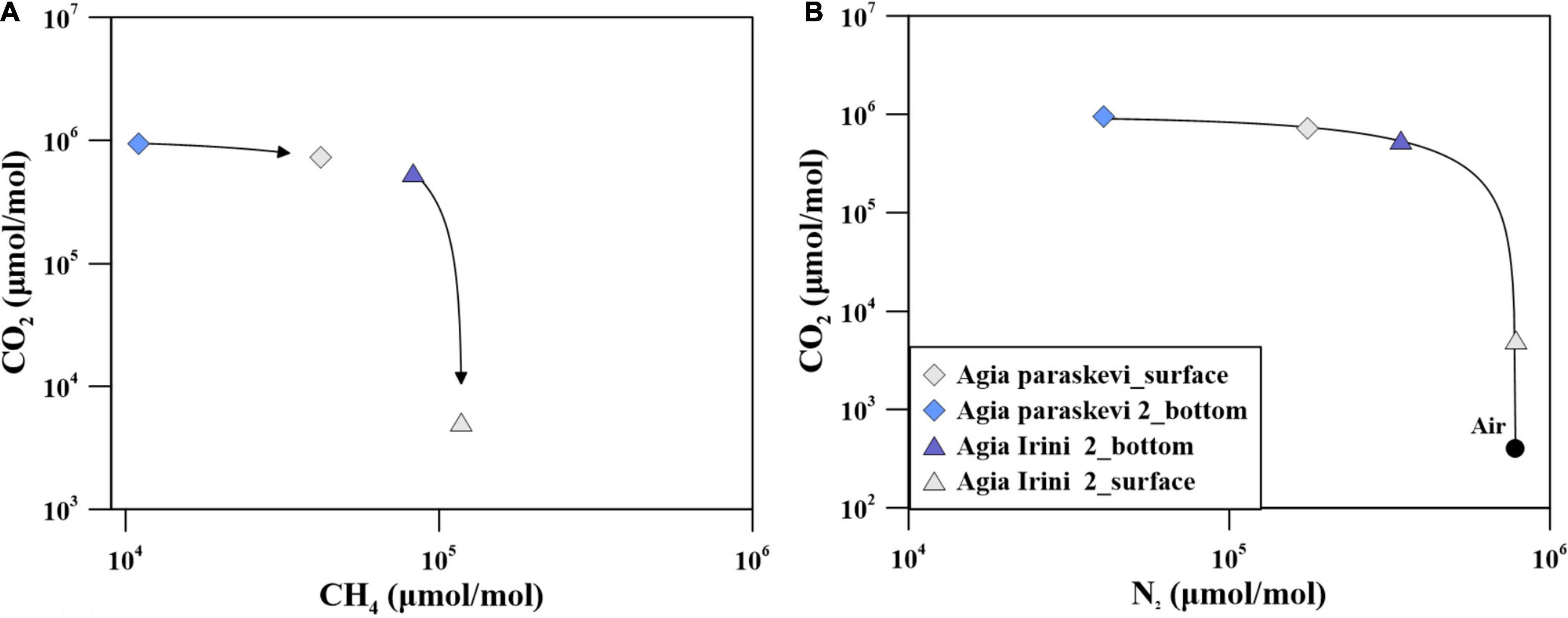
Figure 10. Binary plot of (A) CO2-CH4 and (B) CO2−−N2. Lines in (A) indicate the course toward less soluble gas species, while the line in (B) indicates mixing from the deeper to the shallower gas sample and then to the atmospheric end member. Values of air after Kipfer et al. (2002).
Changes on water characteristics were documented for the areas of Kanavas and Paleochori (Milos), Therma (Kos), and Ilion (Euboea) (Figures 11A–D). These areas comprise widespread degassing vents that are characterized by elevated CO2 contents (>90%) and presence of H2S (Supplementary Table 1). pH transects that were performed along the coast revealed pH values lower than the value of average seawater (Supplementary Table 2). Such lowering of the pH is driven by the dissolution of CO2 in seawater.
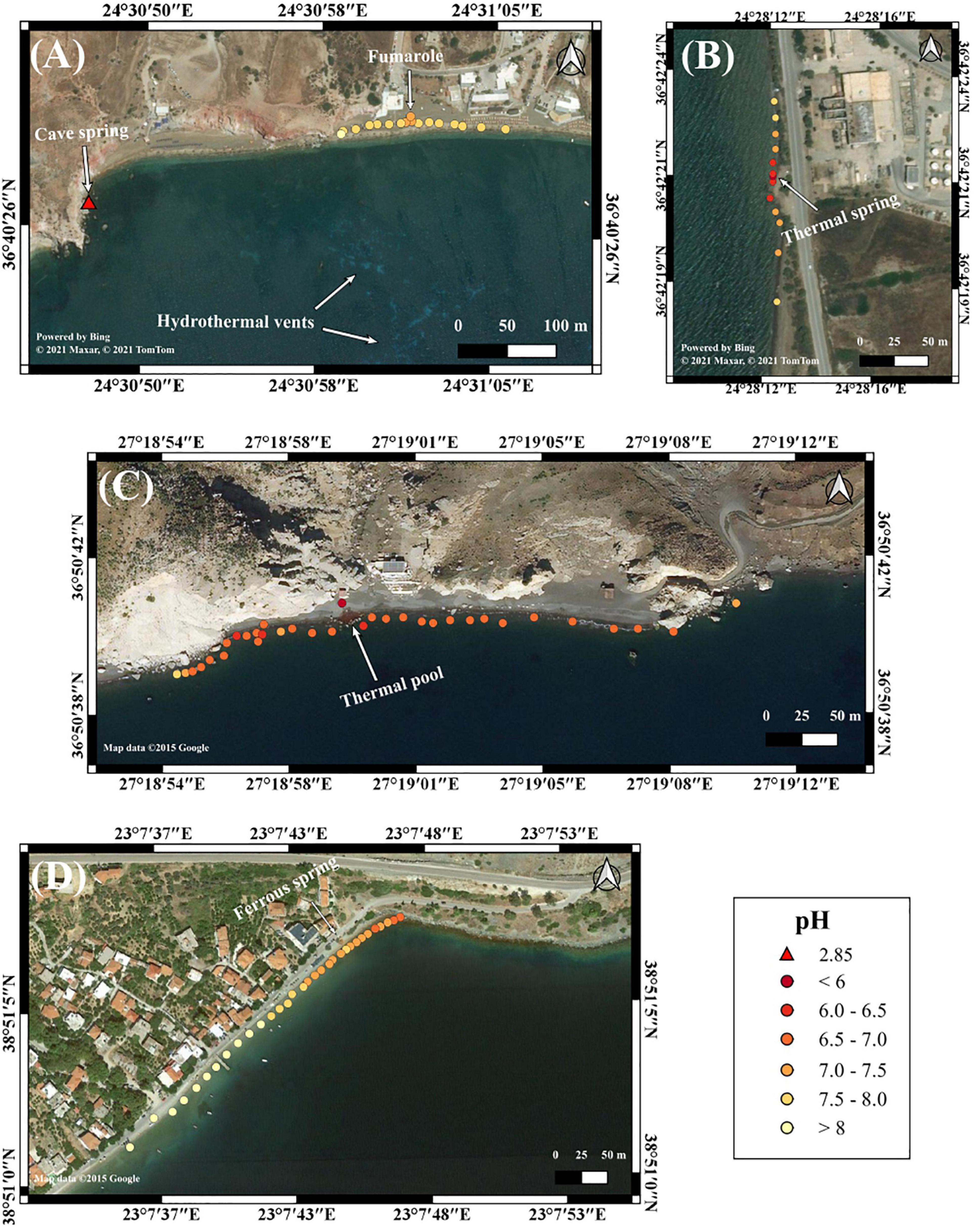
Figure 11. pH transects for the areas of (A) Paleochori and (B) Kanavas at Milos, (C) Therma at Kos, and (D) Ilion at Euboea. Points of particular interest are marked with an arrow. The morphology of the seashore at Paleochori (A) was diverse when the measurements took place.
Daskalopoulou et al. (2018b) identified areas (on-land and in the sea) of intense degassing and anomalous CO2 flux at both Kanavas and Paleochori that correspond to the sites where the lowest pH values are found (Figures 11A,B). These areas are connected to the main fault structures recognized in the island (Stewart and McPhie, 2006) that have likely taken part in the volcanic activity of Milos, acting as pathways for the ascending magma (Kokkalas and Aydin, 2013) and the uprising gases (Dando et al., 1995; Daskalopoulou et al., 2018b). Especially for Paleochori Bay, Aliani et al. (2004) and Khimasia et al. (2021) mapped minor fault structures in the bay with the latter using microbial mats and high temperatures (Khimasia et al., 2020) for fault identification. It is worth mentioning that the temperature profiles by Khimasia et al. (2021) took place at hydrothermal vents within the bacterial mats (Figure 11A). Intense on land and submarine degassing is also identified in the island of Kos at Therma with the CO2 upflow being completely addressed to the hydrothermal component (Daskalopoulou et al., 2019b). Megalovasilis (2020) suggested that the low pH values (Figure 11C) are the result of either fluid mixing with seawater in the substrate or water-gas interactions. It is important to note that the lowest pH values in Milos and Kos are found in emission points characterized by high temperatures (Figure 12). This, combined with the waters’ enriched SO4-Cl content (Li Vigni et al., 2021), evidences the impact of hydrothermal activity on the systems.
In the area of Ilion, the low pH values are concentrated in front and east of a thermal (>62°C; D’Alessandro et al., 2014; Li Vigni et al., 2021) spring at the road (Figure 11D). The spring, as well as many of the thermal water emissions in Euboea, is connected to one of the tectonic structures in the border with the Sperchios Basin-Evoikos Gulf graben. The spring water ascents from the deep and hot geothermal system of the area (Li Vigni et al., 2021), and according to D’Alessandro et al. (2014) is affected by the Quaternary volcanic system. The volcanic impact though is also evident at the eastern side of the coast, where the degassing is diffuse. There, rocks present a rusty color, which is indicative of the emission of iron-rich (probably thermal) groundwater in the area. It is worth mentioning that the mobility of Fe and other trace metals (e.g., Cd, As, Pb) is enhanced by reducing conditions (Tarasov et al., 2005). The main driver is the dissolution of reactive gases (e.g., CO2, H2S, H2) that results in intense rock leaching (Aiuppa et al., 2000).
Overview
Submarine degassing may have an impact on marine environments through ocean acidification. Hence, there is a necessity to study and better understand the ocean and its components. The current work reviews all known submarine gas manifestations of the Aegean Sea and summarizes the geochemical processes taking place in the individual areas.
All in all, degassing occurs in both volcanic and non-volcanic areas and is associated with the complex tectonics of the individual systems. Carbon dioxide is the dominant gas species for most vents and is often related to volcanism, geothermal energy, and elevated heat flow (Fytikas and Kolios, 1979). On the other hand, sites where CH4 is the dominant gas species are likely related to hydrocarbon reservoirs (Rigakis et al., 2001).
The isotope signatures of He for gases found in SAAVA yield an important mantle contribution, while a dominant crustal origin characterizes gases in non-volcanic areas. Carbon dioxide derives from mixed mantle-limestone sources for most samples and in cases exhibits unimportant contributions from organic sediment sources. Methane is attributed to abiogenic hydrocarbons discharged from volcanic-geothermal systems. Inorganic and organic CH4 oxidation processes resulting in isotope fractionation have also been identified (Daskalopoulou et al., 2018a). Only at Samothraki, where it is the main gas species, CH4 is of biogenic origin.
The impact of water-gas-rock interactions on the initial gas phase is evident as soluble gas species dissolve in the water. This results in their depletion and the consequent enrichment of less soluble gas species. This phenomenon was also noticeable while comparing the composition of the gases at the emission point on the seafloor and the sea surface after its rising through the entire sea column. In addition to gas content variations, pH transects were performed in 4 sites. These are characterized by volcanic/geothermal activity, have CO2 as the dominant gas species, and presented lower pH respect to the average marine value.
Even though the impact of gases on marine flora and fauna was not investigated in the current study, it shouldn’t be disregarded. Various researchers (e.g., Boatta et al., 2013; Price and Giovannelli, 2017; Aiuppa et al., 2021; Caramanna et al., 2021) have already highlighted that shallow marine vents can provide us with an accessible and economic way to investigate the effects of CO2 on the whole marine ecosystems. In addition to this, parameters like the presence of light, wave action, tides, the input of meteoric water, salinity variations, etc., can significantly influence the geochemistry of the vents and the microbial diversity and distribution (Giovannelli and Price, 2018).
It is important to note that this is a preliminary catalog of shallow submarine vents found in the Aegean Sea. Springs found in tectonic structures on-land close to the coast (Li Vigni et al., 2021) characterized by strong degassing (Daskalopoulou et al., 2019a) and soil alterations (D’Alessandro et al., 2020) can be good indications of nearby submarine degassing underscoring that the catalog has still to be completed. Nevertheless, we aim that this study will initiate further research on the OA in Greece and in other countries. Following the identification of new emission sites and the quantification of gas flow, research should move towards a bio-, hydro-, and geochemical monitoring direction. As a next step, the anthropogenic input has to be taken into consideration. Understanding and defining the impact of both geogenic and anthropogenic processes affecting the various spheres in systems such as the Mediterranean, will result in the improvement of not only the carbon cycle knowledge but also of the dynamics and vulnerability of individual systems. This research and our group invite researchers from related disciplines to use multiple approaches and investigate other aspects of this problem. Hence, we make results accessible as electronic supplements and we aim to publish them as stand-alone datasets with their own doi.
Author Contributions
SC and KD contributed to the conception and design of this study. ML, GP, and SC collected the samples by diving. ML contributed to the laboratory analyses. KD and WD’A wrote the first draft while all authors contributed to manuscript revision, read and approved the submitted version. All authors were involved in the sampling campaigns.
Conflict of Interest
The authors declare that the research was conducted in the absence of any commercial or financial relationships that could be construed as a potential conflict of interest.
Publisher’s Note
All claims expressed in this article are solely those of the authors and do not necessarily represent those of their affiliated organizations, or those of the publisher, the editors and the reviewers. Any product that may be evaluated in this article, or claim that may be made by its manufacturer, is not guaranteed or endorsed by the publisher.
Acknowledgments
This manuscript comprises literature data collected principally by the authors’ working group. We kindly acknowledge all the friends and colleagues who helped us either in the field or with precious information about the sampling sites. We would like to thank once again: the LAVA Mining and Quarrying SA Company that allowed us to access Gyali Island, offered us lunch and accompanied us around the island with their boat looking for underwater gas emanations (Mr. Diamantis); Sabina Morici who helped us with the pH measurements at Paleochori and DEH (Milos Island) and Roy Price and Thomas Pichler, who collected gas samples at Spathy Bay (Milos Island); Marine warrant officer Ilias Simadakis who gave us the permission to collect samples at Agia Irini (Kos Island), the owner of “Kardamena Watersports Center” Mr. Nikos Nikolakopoulos who gave us one of his boats, and Mr. Yannis Limperis who accompanied us to the points of interest at Agia Irini. For the analyses made at the laboratories of the INGV of Palermo, we are indebted to the heads of the laboratories and technicians: G. Capasso, F. Grassa, M. Martelli, Y. Oliveri, A. Rizzo, F. Salerno, A. Sollami, and M. Tantillo. We are grateful for the insightful comments of the two reviewers and of the editor HR that helped us to improve the manuscript.
Supplementary Material
The Supplementary Material for this article can be found online at: https://www.frontiersin.org/articles/10.3389/fmars.2021.775247/full#supplementary-material
References
Agostini, S., Doglioni, C., Innocenti, F., Manetti, P., and Tonarini, S. (2010). On the geodynamics of the Aegean rift. Tectonophysics 488, 7–21. doi: 10.1016/j.tecto.2009.07.025
Aiuppa, A., Allard, P., D’Alessandro, W., Michel, A., Parello, F., Treuil, M., et al. (2000). Mobility and fluxes of major, minor and trace metals during basalt weathering and groundwater transport at Mt. Etna volcano (Sicily). Geochim. Cosmochim. Acta 64, 1827–1841. doi: 10.1016/s0016-7037(00)00345-8
Aiuppa, A., Hall-Spencer, J. M., Milazzo, M., Turco, G., Caliro, S., and Di Napoli, R. (2021). Volcanic CO2 seep geochemistry and use in understanding ocean acidification. Biogeochemistry 152, 93–115. doi: 10.1007/s10533-020-00737-9
Aliani, S., Meloni, R., and Dando, P. R. (2004). Periodicities in sediment temperature timeseries at a marine shallow water hydrothermal vent in Milos Island (Aegean Volcanic arc, Eastern Mediterranean). J. Mar. Syst. 46, 109–119. doi: 10.1016/j.jmarsys.2003.11.015
Allen, S. R., and McPhie, J. (2000). Water-settling and resedimentation of submarine rhyolitic pumice at Yali eastern Aegean Greece. J. Volcanol. Geotherm. Res. 95, 285–307. doi: 10.1016/S0377-0273(99)00127-4
Baggini, C., Issaris, Y., Salomidi, M., and Hall-Spencer, J. (2015). Herbivore diversity improves benthic community resilience to ocean acidification. J. Exp. Mar. Biol. Ecol. 469, 98–104.
Baggini, C., Salomidi, M., Voutsinas, E., Bray, L., Krasakopoulou, E., and Hall-Spencer, J. M. (2014). Seasonality affects macroalgal community response to increases in pCO2. PLoS One 9:e106520. doi: 10.1371/journal.pone.0106520
Baltatzis, E., Valsami-Jones, E., Magganas, A., and Kati, M. (2001). Tamarugite from Milos Island, Greece. Neues Jahrb. für Mineral. Monatshefte 8, 371–377.
Bernard, B. B., Brooks, J. M., and Sackett, W. M. (1978). “A geochemical model for characterization of hydrocarbon gas sources in marine sediments,” in Proceedings of the Offshore Technology Conference, Houston, TX, 435–438.
Bindoff, N. L., Cheung, W. W. L., Kairo, J. G., Arístegui, J., Guinder, V. A., et al. (2019). “Changing ocean, marine ecosystems, and dependent communities,” in IPCC Special Report on the Ocean and Cryosphere in a Changing Climate, eds H.-O. Pörtner, D. C. Roberts, V. Masson-Delmotte, P. Zhai, M. Tignor, E. Poloczanska, et al. (Geneva: Intergovernmental Panel on Climate Change).
Blackford, J., Stahl, H., Bull, J. M., Berges, B. J. B., Cevatoglu, M., Lichtschlag, A., et al. (2014). Detection and impacts of leakage from sub-seafloor deep geological carbon dioxide storage. Nat. Clim. Change 4, 1011–1016. doi: 10.1038/nclimate2381
Boatta, F., D’Alessandro, W., Gagliano, A. L., Liotta, M., Milazzo, M., Rodolfo-Metalpa, R., et al. (2013). Geochemical survey of Levante Bay, Vulcano Island (Italy), a natural laboratory for the study of ocean acidification. Mar. Pollut. Bull. 73, 485–494. doi: 10.1016/j.marpolbul.2013.01.029
Boccaletti, M., Manetti, P., and Peccerillo, A. (1974). The Balkanids as an instance of back-arc thrust belt: possible relation with the Hellenids. Geol. Soc. Am. Bull. 85, 1077–1084. doi: 10.1130/0016-7606197485<1077:TBAAIO<2.0.CO;2
Böstrom, K., and Widenfalk, L. (1984). The origin of iron-rich muds at the Kameni Islands, Santorini, Greece. Chem. Geol. 42, 203–216. doi: 10.1016/0009-2541(84)90015-9
Botz, R., Stüben, D., Winckler, G., Bayer, R., Schmitt, M., and Faber, E. (1996). Hydrothermal gases from offshore Milos Island, Greece. Chem. Geol. 130, 161–173.
Bray, L., Pancucci-Papadopoulou, M. A., and Hall-Spencer, J. M. (2014). Sea urchin response to rising pCO2 shows ocean acidification may fundamentally alter the chemistry of marine skeletons. Med. Mar. Sci. 15:510. doi: 10.12681/mms.579
Caldeira, K., and Wicket, M. E. (2005). Ocean model predictions of chemistry changes from carbon dioxide emissions to the atmosphere and ocean. J. Geophys. Res. 110:C09S04. doi: 10.1029/2004jc002671
Caramanna, G., Sievert, S. M., and Bühring, S. I. (2021). Submarine shallow-water fluid emissions and their geomicrobiological imprint: a global overview. Front. Mar. Sci. 8:727199. doi: 10.3389/fmars.2021.727199
Carey, S., Bell, K. L. C., Nomikou, P., Vougioukalakis, G., Roman, C., Cantner, K., et al. (2011). Exploration of the Kolumbo volcanic rift zone. “New frontiers in ocean exploration” the E/V Nautilus 2010 field season. Oceanography 24, 24–25.
Carey, S., Nomikou, P., Croff Bell, K., Lilley, M., Lupton, J., Roman, C., et al. (2013). CO2 degassing from hydrothermal vents at Kolumbo submarine volcano, Greece, and the accumulation of acidic crater water. Geology 41, 1035–1038. doi: 10.1130/G34286.1
Chiodini, G., Cioni, R., Di Paola, G. M., Dotsika, E., Fytikas, M., Guidi, M., et al. (1998). “Geochemistry of Santorini fluids,” in Proceedings of the 2nd Workshop, The European laboratory volcanoes. Santorini, Greece, EUR 18161 EN, eds R. Casale, M. Fytikas, G. Sigvaldasson, and G. E. Vougioukalakis (Luxembourg: European Commission), 193–232.
Cornwall, C. E., and Hurd, C. L. (2016). Experimental design in ocean acidification research: problems and solutions. ICES J. Mar. Sci. 73, 572–581. doi: 10.1093/icesjms/fsv118
Cronan, D. S., and Varnavas, S. P. (1999). Metalliferous sediments off Milos, Hellenic Volcanic Arc. Explor. Min. Geol. 8, 289–297.
D’Alessandro, W., Brusca, L., Kyriakopoulos, K., Bellomo, S., and Calabrese, S. (2014). A geochemical traverse along the “Sperchios Basin — Evoikos Gulf” Graben (Central Greece): origin and evolution of the emitted fluids. Mar. Petrol. Geol. 55, 295–308. doi: 10.1016/j.marpetgeo.2013.12.011
D’Alessandro, W., Brusca, L., Kyriakopoulos, K., Michas, G., and Papadakis, G. (2008). Methana, the westernmost active volcanic system of the south Aegean arc (Greece): insight from fluids geochemistry. J. Volcanol. Geotherm. Res. 178, 818–828. doi: 10.1016/j.jvolgeores.2008.09.014
D’Alessandro, W., Calabrese, S., Bellomo, S., Brusca, L., Daskalopoulou, K., Vigni, L. L., et al. (2020). Impact of hydrothermal alteration processes on element mobility and potential environmental implications at the Sousaki solfataric field (Corinthia–Greece). J. Volcanol. Geotherm. Res. 407:107121. doi: 10.1016/j.jvolgeores.2020.107121
Dando, P. R., Hughes, J. A., Leahy, Y., Niven, S. J., Taylor, L. J., and Smith, C. (1995). Gas venting rates from submarine hydrothermal areas around the island of Milos, Hellenic Volcanic Arc. Cont. Shelf Res. 15, 913–929. doi: 10.1016/0278-4343(95)80002-U
Daskalopoulou, K., Calabrese, S., Gagliano, A. L., and D’Alessandro, W. (2019a). Estimation of the geogenic carbon degassing of Greece. Appl. Geochem. 106, 60–74. doi: 10.1016/j.apgeochem.2019.04.018
Daskalopoulou, K., Gagliano, A. L., Calabrese, S., Li Vigni, L., Longo, M., Kyriakopoulos, K., et al. (2019b). Degassing at the volcanic/geothermal system of kos (Greece): geochemical characterization of the released gases and CO2 output estimation. Geofluids 2019:3041037. doi: 10.1155/2019/3041037
Daskalopoulou, K., Calabrese, S., Gagliano, A. L., Kyriakopoulos, K., Li Vigni, L., Longo, M., et al. (2021a). Chemical characterisation of the gases released at Gyali Island, Dodecanese, Greece and preliminary estimation of the CO2 output. It. J. Geosci. 140, 16–28. doi: 10.3301/IJG.2020.18
Daskalopoulou, K., Woith, H., Zimmer, M., Niedermann, S., Barth, J. A. C., Frank, A. H., et al. (2021b). Insight into Hartoušov Mofette, Czech Republic: tales by the fluids. Front. Earth Sci. 9:615766. doi: 10.3389/feart.2021.615766
Daskalopoulou, K., Calabrese, S., Grassa, F., Kyriakopoulos, K., Parello, F., Tassi, F., et al. (2018a). Origin of methane and light hydrocarbons in natural fluid emissions: a key study from Greece. Chem. Geol. 479, 286–301. doi: 10.1016/j.chemgeo.2018.01.027
Daskalopoulou, K., Gagliano, A. L., Calabrese, S., Longo, M., Hantzis, K., Kyriakopoulos, K., et al. (2018b). Gas geochemistry and CO2 output estimation at the island of Milos, Greece. J. Volcanol. Geotherm. Res. 365, 13–22. doi: 10.1016/j.jvolgeores.2018.10.003
De Orte, M. R., Sarmiento, A. M., Basallote, M. D., Rodríguez-Romero, A., Riba, I., and Delvalls, A. (2014). Effects on the mobility of metals from acidification caused by possible CO2 leakage from sub-seabed geological formations. Sci. Total Environ. 470–471, 356–363. doi: 10.1016/j.scitotenv.2013.09.095
Di Napoli, R., Aiuppa, A., Bergsson, B., Ilyinskaya, E., Pfeffer, M. A., Guðjónsdóttir, S. R., et al. (2016). Reaction path models of magmatic gas scrubbing. Chem. Geol. 420, 251–269. doi: 10.1016/j.chemgeo.2015.11.024
Dimitriadis, I., Karagianni, E., Panagiotopoulos, D., Papazachos, C., Hatzidimitriou, P., Bohnhoff, M., et al. (2009). Seismicity and active tectonics at Coloumbo Reef (Aegean Sea, Greece): monitoring an active volcano at Santorini volcanic center using a temporary seismic network. Tectonophysics 465, 136–149. doi: 10.1016/j.tecto.2008.11.005
Doney, S. C., Fabry, V. J., Feely, R. A., and Kleypas, J. A. (2009). Ocean acidification: the other CO2 problem. Annu. Rev. Mar. Sci. 1, 169–192. doi: 10.1146/annurev.marine.010908.163834
Doney, S. C., Ruckelshaus, M., Duffy, J. E., Barry, J. P., Chan, F., English, C. A., et al. (2012). Climate change impacts on marine ecosystems. Ann. Rev. Mar. Sci. 4, 11–37. doi: 10.1146/annurev-marine-041911-111611
Doo, S. S., Kealoha, A., Andersson, A., Cohen, A. L., Hicks, T. L., Johnson, Z. I., et al. (2020). The challenges of detecting and attributing ocean acidification impacts on marine ecosystems. ICES J. Mar. Sci. 77, 2411–2422. doi: 10.1093/icesjms/fsaa094
Dotsika, E. (2012). Isotope and hydrochemical assessment of the Samothraki Island geothermal area, Greece. J. Volcanol. Geotherm. Res. 233–234, 18–26. doi: 10.1016/j.jvolgeores.2012.04.017
Dotsika, E., Poutoukis, D., Michelot, J. L., and Raco, B. (2009). Natural tracers for identifying the origin of the thermal fluids emerging along the Aegean volcanic arc (Greece): evidence of arc-type magmatic water (ATMW) participation. J. Volcanol. Geotherm. Res. 179, 19–32. doi: 10.1016/j.jvolgeores.2008.09.024
Drever, J. I. (1997). The Geochemistry of Natural Waters: Surface and Groundwater Environments, 3rd Edn. Upper Saddle River, NJ: Prentice Hall.
Druitt, T. H., Edwards, L., Mellors, M., Pyle, D. M., Sparks, R. S. J., Lanphere, M., et al. (1999). Santorini Volcano (Geological map of the Santorini islands, Scale 1/20.000). Geological Society Memoir No. 19. London: Geological Society.
Druitt, T. H., Mellors, R. A., Pyle, D. M., and Sparks, R. S. J. (1989). Explosive volcanism on Santorini, Greece. Geol. Mag. 126, 95–126. doi: 10.1017/S0016756800006270
Enochs, I. C., Manzello, D. P., Tribollet, A., Valentino, L., Kolodziej, G., Donham, E. M., et al. (2016). Elevated colonization of microborers at a volcanically acidified coral reef. PLoS One 11:e0159818. doi: 10.1371/journal.pone.0159818
Fabricius, K. E., Langdon, C., Uthicke, S., Humphrey, C., Noonan, S., De’ath, G., et al. (2011). Losers and winners in coral reefs acclimatized to elevated carbon dioxide concentrations. Nat. Clim. Change 1, 165–169. doi: 10.1038/nclimate1122
Flohr, A., Schaap, A., Achterberg, E. P., Alendal, G., Arundell, M., Berndt, C., et al. (2021). Towards improved monitoring of offshore carbon storage: a real-world field experiment detecting a controlled sub-seafloor CO2 release. Int. J. Greenh. Gas Control 106:103237. doi: 10.1016/j.ijggc.2020.103237
Foo, S. A., Byrne, M., Ricevuto, E., and Gambi, M. C. (2018). “The carbon dioxide vents of ischia, italy, a natural system to assess impacts of ocean acidification on marine ecosystems: an overview of research and comparisons with other vent systems,” in Oceanography and Marine Biology, An Annual Review, eds S. J. Hawkins, A. J. Evans, A. C. Dale, L. B. Firth, and I. P. Smith (Boca Raton, FL: CRC Press). doi: 10.1201/9780429454455
Foutrakis, P. M., and Anastasakis, G. (2018). The active submarine NW termination of the south Aegean active volcanic arc: the submarine Pausanias volcanic field. J. Volcanol. Geotherm. Res. 357, 399–417. doi: 10.1016/j.jvolgeores.2018.05.008
Francalanci, L., Vougioukalakis, G. E., Perini, G., and Manetti, P. A. (2005). “West-East traverse along the magmatism of the South Aegean volcanic arc in the light of volcanological, chemical and isotope data,” in The South Aegean Active Volcanic Arc, Present Knowledge and Future Perspectives, Developments in Volcanology, Vol. 7, eds M. Fitykas and G. E. Vougioukalakis (Amsterdam: Elsevier), 65–111. doi: 10.1016/S1871-644X(05)80033-6
Fytikas, M., Giuliani, O., Innocenti, F., Marinelli, G., and Mazzuoli, R. (1976). Geochronological data on recent magmatism of the Aegean Sea. Tectonophysics 31, T29–T34. doi: 10.1016/0040-1951(76)90161-X
Fytikas, M., Innocenti, F., Kolios, N., Manetti, P., and Mazzuoli, R. (1986). The plio-quaternary volcanism of Saronikos area (western part of the active Aegean volcanic arc). Ann. Geol. Pays Hellen. 33, 23–45.
Fytikas, M., Innocenti, F., Manetti, P., Mazuoli, R., Peccerilo, A., and Villari, L. (1984). “Tertiary to quaternary evolution of the volcanism in Aegean Sea,” in The Geological Evolution of the Eastern Mediterranean, Vol. 17, eds J. E. Dixon and A. H. F. Robertson (London: Geological Society of London Special Publications), 687–699.
Fytikas, M., and Kolios, N. (1979). “Preliminary heat flow map of Greece,” in Terrestrial Heat Flow in Europe, eds V. Cermak and L. Rybach (Berlin: Springer-Verlag), 197–205.
Gattuso, J.-P., and Hansson, L. (2011). “Ocean acidification: background and history,” in Ocean Acidification, eds J.-P. Gattuso and L. Hansson (Oxford: Oxford University Press), 1–20.
Gautier, P., Brun, J. P., and Jolivet, L. (1993). Structure and kinematics of upper cenozoic extensional detachment on Naxos and Paros (Cyclades Islands, Greece). Tectonics 12, 1180–1194. doi: 10.1029/93TC01131
Giovannelli, D., and Price, R. E. (2018). “Marine shallow-water hydrothermal vents: microbiology,” in Reference Module in Earth Systems and Environmental Sciences, Vol. 4, (Amsterdam: Elsevier), 353–363. doi: 10.1016/b978-0-12-409548-9.11250-3
Godelitsas, A., Price, R. E., Pichler, T., Amend, J., Gamaletsos, P., and Göttlicher, J. (2015). Amorphous as-sulfide precipitates from the shallow-water hydrothermal vents off Milos Island (Greece). Mar. Chem. 177, 687–696. doi: 10.1016/j.marchem.2015.09.004
Golbuu, Y., Gouezo, M., Kurihara, H., Rehm, L., and Wolanski, E. (2016). Long-term isolation and local adaptation in Palau’s Nikko Bay help corals thrive in acidic waters. Coral Reefs 35, 909–918. doi: 10.1007/s00338-016-1457-5
Gros, J., Schmidt, M., Linke, P., Dötsch, S., Triest, J., Martínez-Cabanas, M., et al. (2021). Quantification of dissolved CO2 plumes at the Goldeneye CO2-release experiment. Int. J. Greenh. Gas Control 109:103387. doi: 10.1016/j.ijggc.2021.103387
Gruber, N., Clement, D., Carter, B. R., Feely, R. A., van Heuven, S., Hoppema, M., et al. (2019). The oceanic sink for anthropogenic CO2 from 1994 to 2007. Science 363, 1193–1199.
Hall-Spencer, J. M., Rodolfo-Metalpa, R., Martin, S., Ransome, E., Fine, M., Turner, S. M., et al. (2008). Volcanic carbon dioxide vents show ecosystem effects of ocean acidification. Nature 454, 96–99. doi: 10.1038/nature07051
HAUGAN, P. M., and Drange, H. (1996). Effects of CO2 on the ocean environment. Energy Convers. Manage. 37, 1019–1022. doi: 10.1016/0196-8904(95)00292-8
Heiken, G., and McCoy, F. (1984). Caldera development during the Minoan eruption, Thera, Cyclades, Greece. J. Geophys. Res. 89, 8441–8462. doi: 10.1029/JB089iB10p08441
Hernández, C. A., Sangil, C., and Hernández, J. C. (2016). A new CO2 vent for the study of ocean acidification in the Atlantic. Mar. Pollut. Bull. 109, 419–426. doi: 10.1016/j.marpolbul.2016.05.040
Hunziker, J. C., and Marini, L. (2005). The Geology, Geochemistry and Evolution of Nisyros Volcano. Implications for the Volcanic Hazard. Lausanne: Memoires Geologie, 44.
IPCC (2005). “IPCC special report on carbon dioxide capture and storage,” in Working Group III of the Intergovernmental Panel on Climate Change, eds B. Metz, O. Davidson, H. C. de Coninck, M. Loos, and L. A. Meyer (Cambridge: Cambridge University Press), 442.
IPCC (2021). Climate Change 2021: The Physical Science Basis. Contribution of Working Group I to the Sixth Assessment Report of the Intergovernmental Panel on Climate Change, eds V. Masson-Delmotte, P. Zhai, A. Pirani, S. L. Connors, C. Péan, S. Berger, et al. (Cambridge: Cambridge University Press).
Ivarsson, M., Kilias, S. P., Broman, C., Neubeck, A., Drake, H., Chi Fru, E., et al. (2019). Exceptional preservation of fungi as H2-bearing fluid inclusions in an early quaternary paleo-hydrothermal system at Cape Vani, Milos, Greece. Minerals 9:749. doi: 10.3390/min9120749
Jiang, L. Q., Carter, B. R., Feely, R. A., Lauvset, S. K., and Olsen, A. (2019). Surface ocean pH and buffer capacity: past, present and future. Sci. Rep. 9:18624. doi: 10.1038/s41598-019-55039-4
Jolivet, L., Faccenna, C., Huet, B., Labrousse, L., Le Pourhiet, L., Lacombe, O., et al. (2013). Aegean tectonics: strain localisation, slab tearing and trench retreat. Tectonophysics 597–598, 1–33. doi: 10.1016/j.tecto.2012.06.011
Joos, F., Frolicher, T. L., Steinacher, M., and Plattner, G.-K. (2011). “Impact of climate change mitigation on ocean acidification projections,” in Ocean Acidification, eds J. P. Gattuso and L. Hansson (Oxford: Oxford University Press), 273–289.
Kanellopoulos, C. (2012). Distribution, lithotypes and mineralogical study of newly formed thermogenic travertines in Northern Euboea and Eastern Central Greece. Centr. Eur. J. Geosci. 4, 545–560. doi: 10.2478/s13533-012-0105-z
Kanellopoulos, C., Mitropoulos, P., Valsami-Jones, E., and Voudouris, P. (2017). A new terrestrial active mineralizing hydrothermal system associated with ore-bearing travertines in Greece (northern Euboea Island and Sperchios area). J. Geochem. Explor. 179, 9–24. doi: 10.1016/j.gexplo.2017.05.003
Kauffmann, G., Kockel, F., and Mollat, H. (1976). Notes on the stratigraphic and paleogeographic position of the svoula formation in the innermost zone of the Hellenides (Nothern Greece). Bull. Soc. Géol. Fr. 7/18, 225–230.
Khimasia, A., Renshaw, C. E., Price, R. E., and Pichler, T. (2021). Hydrothermal flux and porewater geochemistry in Paleochori Bay, Milos, Greece. Chem. Geol. 571:120188. doi: 10.1016/j.chemgeo.2021.120188
Khimasia, A., Rovere, A., and Pichler, T. (2020). Hydrothermal areas, microbial mats and sea grass in Paleochori Bay, Milos, Greece. J. Maps 16, 348–356. doi: 10.1080/17445647.2020.1748131
Kilias, S. P., Nomikou, P., Papanikolaou, D., Polymenakou, P. N., Godelitsas, A., Argyraki, A., et al. (2013). New insights into hydrothermal vent processes in the unique shallowsubmarine arc-volcano, Kolumbo (Santorini), Greece. Sci. Rep. 3:2421. doi: 10.1038/srep02421
Kipfer, R., Aeschbach-Hertig, W., Peeters, F., and Stute, M. (2002). Noble gases in lakes and ground waters. Rev. Mineral. Geochem. 47, 615–700. doi: 10.2138/rmg.2002.47.14
Koch, M., Bowes, G., Ross, C., and Zhang, X.-H. (2012). Climate change and ocean acidification effects on seagrasses and marine macroalgae. Glob. Change Biol. 19, 103–132. doi: 10.1111/j.1365-2486.2012.02791.x
Kockel, F., Mollat, H., and Walther, H. W. (1977). Erlauterungen zur Geologischen Karte der Chalkidiki und Angrenzender Gebiete 1:100.000 (Nord Griechenland). Hannover: Bundesanstalt fur Geowissenschaften und Rohstoffe, 1–119.
Kokkalas, S., and Aydin, A. (2013). Is there a link between faulting and magmatism in the south-central Aegean Sea? Geol. Mag. 150, 193–224. doi: 10.1017/S0016756812000453
Kotopouli, C. N., Hatzipanagiotou, K., and Tsikouras, B. (1989). Petrographic and geochemical characteristics of the ophioltic rocks in Northern Samothrace, Greece. Geol. Balc. 19, 61–67.
Kreemer, C., Chamot-Rooke, N., and Le Pichon, X. (2004). Constraints on the evolution and vertical coherency of deformation in the Northern Aegean from a comparison of geodetic, geologic and seismologic data. Earth Planet. Sci. Lett. 225, 329–346. doi: 10.1016/j.epsl.2004.06.018
Kyriakopoulos, K. (2010). “Natural degassing of carbon dioxide and hydrogen sulphide and its environmental impact at Milos Island, Greece,” in Proceedings of the 12th International Congress, Patras, 2361–2372.
La Ruffa, G., Panichi, C., Kavouridis, T., Liberopoulou, V., Leontiadis, J., and Caprai, A. (1999). Isotope and chemical assessment of geothermal potential of Kos island, Greece. Geothermics 28, 205–217. doi: 10.1016/S0375-6505(99)00004-8
Lagios, E., Galanopoulos, D., Hobbs, B. A., and Dawes, G. J. K. (1998). Two-dimensional magnetotelluric modelling of the Kos island geothermal region (Greece). Tectonophysics 287, 157–172. doi: 10.1016/S0040-1951(98)80066-8
Lauritano, C., Ruocco, M., Dattolo, E., Buia, M. C., Silva, J., Santos, R., et al. (2015). Response of key stress-related genes of the seagrass Posidonia oceanica in the vicinity of submarine volcanic vents. Biogeoscience 12, 4185–4194. doi: 10.5194/bg-12-4185-2015
Lazaridis, G., Melfos, V., and Papadopoulou, L. (2011). The first cave occurrence of orpiment (N. Greece). Int. J. Speleol. 40, 133–139. doi: 10.5038/1827-806X.40.2.6
Le Pichon, X., Lybéris, N., and Alvarez, F. (1987). Discussion on the subsidence of the North Aegean trough: an alternative view. J. Geol. Soc. 144, 349–351. doi: 10.1144/gsjgs.144.2.0349
Li Vigni, L., Daskalopoulou, K., Calabrese, S., Kyriakopoulos, K., Parello, F., Brugnone, F., et al. (2021). Geochemical characterisation of the thermo-mineral waters of Greece. Environ. Geochem. Health doi: 10.1007/s10653-021-01001-1
Linares, C., Vidal, M., Canals, M., Kersting, D. K., Amblas, D., Aspillaga, E., et al. (2015). Persistent natural acidification drives major distribution shifts in marine benthic ecosystems. Proc. R. Soc. B Biol. Sci. 282:20150587. doi: 10.1098/rspb.2015.0587
Lister, G. S., Banga, G., and Feenstra, A. (1984). Metamorphic core complexes of cordilleran type in the Cyclades, Aegean Sea, Greece. Geology 12, 221–225. doi: 10.1130/0091-7613198412<221:MCCOCT<2.0.CO;2
Marini, L., Principe, C., Chiodini, G., Cioni, R., Frytikas, M., and Marinelli, G. (1993). Hydrothermal eruptions of Nisyros (Dodecanese, Greece). Past events and present hazard. J. Volcanol. Geotherm. Res. 56, 71–95. doi: 10.1016/0377-0273(93)90051-R
Megalovasilis, P. (2020). Geochemistry of hydrothermal particles in shallow submarine hydrothermal vents on Milos Island, Aegean Sea East Mediterranean. Geochem. Int. 58, 151–181. doi: 10.1134/s001670292002007x
Mercier, J., Delibassis, N., Gauthier, A., Jarrige, J. J., Lemeille, F., Philip, H., et al. (1979). La néotectonique de l’Arc Egéen. Rev. Géol. Dyn. Géogr. Phys. 21, 67–92.
Millero, F., Woosley, R., DiTrolio, B., and Waters, J. (2009). Effect of ocean acidification on the speciation of metals in seawater. Oceanography 22, 72–85. doi: 10.5670/oceanog.2009.98
Monastersky, R. (2013). Seabed scars raise questions over carbon-storage plan. Nature 504, 339–340. doi: 10.1038/504339a
Mountrakis, D., Pavlides, S., Chatzipetros, A., Meletidis, S., Tranos, M., Vougioukalakis, G., et al. (1998). “Active deformation in Santorini,” in The European Laboratory Volcanoes, eds R. Casale, M. Fytikas, G. Sigvaldarsson, and G. Vougioukalakis (Brussels: European Commission), 13–22.
Mountrakis, D. M. (1985). Geology of Greece. Thessaloniki: University Studio Press, 207. (in Greek).
NOAA (2021). National Oceanic & Atmospheric Administration, Earth System Research Laboratory, Global Monitoring Division, Trends in Atmospheric Carbon Dioxide. Available online at: https://www.esrl.noaa.gov/gmd/ccgg/trends/ (accessed December 2021)
Oikonomidis, D., and Pavlides, S. (2017). Geological mapping of Santorini Volcanic island (Greece), with the combined use of Pleiades 1A and ENVISAT satellite images. Arab. J. Geosci. 10:175. doi: 10.1007/s12517-017-2972-6
Parks, M. M., Caliro, S., Chiodini, G., Pyle, D. M., Mather, T. A., Berlo, K., et al. (2013). Distinguishing contributions to diffuse CO2 emissions in volcanic areas from magmatic degassing and thermal decarbonation using soil gas 222Rn–δ13C systematics: application to Santorin volcano, Greece. Earth Planet. Sci. Let. 377–378, 180–190. doi: 10.1016/j.epsl.2013.06.046
Patoucheas, P., Koukousioura, O., Psarra, S., Aligizaki, K., Dimiza, M. D., Skampa, E., et al. (2021). Phytoplankton community structure changes during autumn and spring in response to environmental variables in Methana, Saronikos Gulf, Greece. Environ. Sci. Pollut. Res. 28, 33854–33865. doi: 10.1007/s11356-020-12272-z
Pavlides, S. B., Valkaniotis, S., Ganas, A., Keramydas, D., and Sboras, S. (2004). The active fault of Atalanti–re-evaluation with new geological data. Bull. Geoll Soc. Greece 36, 1560–1567. (in Greek),
Pavlidis, S., Valkaniotis, S., Kurcel, A., Papathanasiou, Y., and Xatzipetrou, A. (2005). Neotectonic structure of Samothraki Island in relation to the North Anatolia fault. Bull. Greek Geol. Soc. 37, 19–28.
Pe-Piper, G., and Piper, D. J. W. (1989). Spatial and temporal variation in late cenozoic back-arc volcanic rocks, Aegean Sea Region. Tectonophysics 169, 113–134. doi: 10.1016/0040-1951(89)90186-8
Pe-Piper, G., and Piper, D. J. W. (2002). The Igneous Rocks of Greece, The Anatomy of an Orogen. Beiträge zur regionalen Geologie der Erde, Band 30. Berlin: Gebrüder Bornträger.
Price, R. E., and Giovannelli, D. (2017). “A review of the geochemistry and microbiology of marine shallow-water hydrothermal vents,” in Reference Module in Earth Systems and Environmental Sciences (Amsterdam: Elsevier). doi: 10.1016/B978-0-12-409548-9.09523-3
Price, R. E., Savov, I., Planer-Friedrich, B., Bühring, S. I., Amend, J., and Pichler, T. (2013). Processes influencing extreme as enrichment in shallow-sea hydrothermal fluids of Milos island, Greece. Chem. Geol. 348, 15–26. doi: 10.1016/j.chemgeo.2012.06.007
Rahders, E., Halbach, P., Halbach, M., Rahner, S., and Varnavas, S. P. (1997). “Hydrothermal alteration and precipitation processes on Methana peninsula, Greece,” in Mineral Deposits: Research and Exploration, Where Do They Meet?, ed. H. Papunen (Rotterdam: Balkema), 965–966.
Reid, R. C., Prausnitz, J. M., and Poling, B. E. (1987). The Properties of Gases & Liquids, 4th Edn. Boston, MA: McGraw-Hill.
Rigakis, N., Roussos, N., Kamberis, E., and Proedrou, P. (2001). Hydrocarbon gas accumulations in Greece and their origin. Bull. Geol. Soc. Greece 34, 1265–1273.
Rizzo, A. L., Caracausi, A., Chavagnac, V., Nomikou, P., Polymenakou, P. N., Mandalakis, M., et al. (2016). Kolumbo submarine volcano (Greece): an active window into the Aegean subduction system. Sci. Rep. 6:28013. doi: 10.1038/srep28013
Rizzo, A. L., Caracausi, A., Chavagnac, V., Nomikou, P., Polymenakou, P. N., Mandalakis, M., et al. (2019). Geochemistry of CO2-rich gases venting from submarine volcanism: the case of kolumbo (Hellenic Volcanic Arc, Greece). Front. Earth Sci. 7:60. doi: 10.3389/feart.2019.00060
Roberts, H., Price, R., Brombach, C. C., and Pichler, T. (2021). Mercury in the hydrothermal fluids and gases in Paleochori Bay, Milos, Greece. Mar. Chem. 233:103984. doi: 10.1016/j.marchem.2021.103984
Robertson, A. H. F., Clift, P. D., Degnan, P. J., and Jones, G. (1991). “Palaeogeographic and palaeotectonic evolution of the eastern Mediterranean Neotethys,” in Palaeogeography and Paleoceanography of Tethys: Special Issue of Palaeogeography Palaeoecology Palaeoecology, Vol. 87, eds J. E. T. Channell, E. L. Winterer, and L. F. Jansa (Amsterdam: Elsevier), 289–343.
Rogelja, M., Cibic, T., Pennesi, C., and De Vittor, C. (2016). Microphytobenthic community composition and primary production at gas and thermal vents in the Aeolian Islands (Tyrrhenian Sea, Italy). Mar. Environ. Res. 118, 31–44. doi: 10.1016/j.marenvres.2016.04.009
Russell, G. L., Lacis, A. A., Rind, D. H., Colose, C., and Opstbaum, R. F. (2013). Fast atmosphere-ocean model runs with large changes in CO2. Geophys. Res. Lett. 40, 5787–5792. doi: 10.1002/2013GL056755
Sabine, C. L., Feely, R. A., Gruber, N., Key, R. M., Lee, K., Bullister, J. L., et al. (2004). The oceanic sink for anthropogenic CO2. Science 305, 367–371. doi: 10.1126/science.1097403
Sano, Y., and Marty, B. (1995). Origin of carbon in fumarolic gas from island arcs. Chem. Geol. 119, 265–274. doi: 10.1016/0009-2541(94)00097-2
Sano, Y., and Wakita, H. (1985). Geographical distribution of 3He/4He ratios in Japan: implications for arc tectonics and incipient magmatism. J. Geophys. Res. 90, 8729–8741. doi: 10.1029/JB090iB10p08729
Sébrier, M. (1977). Tectonique Récente d’une Transversal à l’arc Égéen. Le golfe de Corinthe et ses Regions Périphériques. Ph.D. thesis. Paris: Université Paris XI-Orsay, 137.
Shimizu, A., Sumino, H., Nagao, K., Notsu, K., and Mitropoulos, P. (2005). Variation in noble gas isotopic composition of gas samples from the Aegean arc, Greece. J. Volcanol. Geotherm. Res. 140, 321–339. doi: 10.1016/j.jvolgeores.2004.08.016
Sigurdsson, H., Carey, S., Alexandri, M., Vougioukalakis, G., Croff, K., Roman, C., et al. (2006). Marine investigations of Greece’s santorini volcanic field. Eos 87:337. doi: 10.1029/2006eo340001
Smith, P. A., and Cronan, D. S. (1983). The geochemistry of metalliferous sediments and waters associated with shallow hydrothermal activity (Santorini, Greece). Mar. Geol. 39, 241–262. doi: 10.1016/0009-2541(83)90017-7
St. Seymour, K., Tsikouras, V., Kotopouli, K., Hatzipanayiotou, K., and Pe-Piper, G. (1996). A window to the operation of microplate tectonics in the tethys ocean: the geochemistry of samothrace granite, Aegean Sea. Mineral. Petrol. 56, 251–272. doi: 10.1007/BF01162606
Stefánsson, A., Lemke, K. H., Bènèzeth, P., and Schott, J. (2017). Magnesium bicarbonate and carbonate interactions in aqueous solutions: an infrared spectroscopic and quantum chemical study. Geochim. Cosmochim. Acta 198, 271–284. doi: 10.1016/j.gca.2016.10.032
Stefánsson, A., Sveinbjörnsdòttir, A. E., Heinemeier, J., Arnòrsson, S., Kjartansdòttir, R., and Kristmannsdòttir, H. (2016). Mantle CO2 degassing through the Icelandic crust: evidence from carbon isotopes in groundwater. Geochim. Cosmochim. Acta 191, 300–319. doi: 10.1016/j.gca.2016.06.038
Stewart, A. L., and McPhie, J. (2006). Facies architecture and Late Ploicene—Pleistocene evolution of a felsic volcanic island, Milo, Greece. Bull. Volcanol. 68, 703–726.
Stiros, S. (2000). “Fault pattern of Nisyros island volcano (Aegean Sea, Greece): structural, coastal and archaeological evidence,” in The Archaeology of Geological Catastrophes, Vol. 171, eds B. McGuire et al. (London: Geological Society of London, Special Publications), 385–397.
Symonds, R. B., Gerlach, T. M., and Reed, M. H. (2001). Magmatic gas scrubbing: implications for volcano monitoring. J. Volcanol. Geotherm. Res. 108, 303–341. doi: 10.1016/S0377-0273(00)00292-4
Tarasov, V. G., Gebruk, A. V., Mironov, A. N., and Moskalev, L. I. (2005). Deep-sea and shallow-water hydrothermal vent communities: two different phenomena? Chem. Geol. 224, 5–39. doi: 10.1016/j.chemgeo.2005.07.021
Tarchini, L., Carapezza, M. L., Ranaldi, M., Sortino, F., Gattuso, A., and Acocella, V. (2019). Fluid geochemistry contribution to the interpretation of the 2011–2012 unrest of Santorini, Greece, in the frame of the dynamics of the Aegean Volcanic Arc. Tectonics 38, 1033–1049. doi: 10.1029/2018TC005377
Tassi, F., Vaselli, O., Papazachos, C. B., Giannini, L., Chiodini, G., Vougioukalakis, G. E., et al. (2013). Geochemical and isotopic changes in the fumarolic and submerged gas discharges during the 2011–2012 unrest at Santorini caldera (Greece). Bull. Volcanol. 75:711. doi: 10.1007/s00445-013-0711-8
Taymaz, T., Jackson, J., and McKenzie, D. (1991). Active tectonics of the north and central Aegean Sea. Geophys. J. Int. 106, 433–490. doi: 10.1111/j.1365-246X.1991.tb03906.x
Taymaz, T., Yilmaz, Y., and Dilek, Y. (2007). The Geodynamics of the Aegean and Anatolia: Introduction, Vol. 291. London: Geological Society of London, Special Publications, 1–16.
Teixidó, N., Caroselli, E., Alliouane, S., Ceccarelli, C., Comeau, S., Gattuso, J. P., et al. (2020). Ocean acidification causes variable trait-shifts in a coral species. Glob. Chang. Biol. 26, 6813–6830. doi: 10.1111/gcb.15372
Triantaphyllou, M. V., Baumann, K.-H., Karatsolis, B.-T., Dimiza, M. D., Psarra, S., Skampa, E., et al. (2018). Coccolithophore community response along a natural CO2 gradient off Methana (SW Saronikos Gulf, Greece, NE Mediterranean). PLoS One 13:e0200012. doi: 10.1371/journal.pone.0200012
Tzanis, A., Chailas, S., Sakkas, V., and Lagios, E. (2020). Tectonic deformation in the Santorini volcanic complex (Greece) as inferred by joint analysis of gravity, magnetotelluric and DGPS observations. Geophys. J. Int. 220, 461–489. doi: 10.1093/gji/ggz461
Vizzini, S., Tomasello, A., Di Maida, G., Pirrotta, M., Mazzola, A., and Calvo, S. (2010). Effect of explosive shallow hydrothermal vents on δ13C and growth performance in the seagrass Posidonia oceanica. J. Ecol. 98, 1284–1291. doi: 10.1111/j.1365-2745.2010.01730.x
Voudouris, P., Kati, M., Magganas, A., Keith, M., Valsami-Jones, E., Haase, K., et al. (2021). Arsenian pyrite and cinnabar from active submarine nearshore vents, Paleochori Bay, Milos Island, Greece. Minerals 11:14. doi: 10.3390/min11010014
Whiticar, M., Faber, E., and Schoell, M. (1986). Biogenic methane formation in marine and freshwater environments: CO2 reduction vs. acetate fermentation—isotope evidence. Geochim. Cosmochim. Acta 50, 693–709. doi: 10.1016/0016-7037(86)90346-7
Winkel, L. H. E., Casentini, B., Bardelli, F., Voegelin, A., Nikolaidis, N. P., and Charlet, L. (2013). Speciation of arsenic in Greek travertine: co-precipitation of arsenate with calcite. Geochim. Cosmochim. Acta 106, 99–110. doi: 10.1016/j.gca.2012.11.049
Keywords: CO2 emissions, submarine gas vents, geogenic degassing, environmental impact, Greek Islands, gas flux
Citation: Daskalopoulou K, D’Alessandro W, Longo M, Pecoraino G and Calabrese S (2022) Shallow Sea Gas Manifestations in the Aegean Sea (Greece) as Natural Analogs to Study Ocean Acidification: First Catalog and Geochemical Characterization. Front. Mar. Sci. 8:775247. doi: 10.3389/fmars.2021.775247
Received: 13 September 2021; Accepted: 13 December 2021;
Published: 31 January 2022.
Edited by:
Henry Ruhl, Central and Northern California Ocean Observing System (CeNCOOS), United StatesReviewed by:
Ting Zou, Memorial University of Newfoundland, CanadaArtur Ionescu, Babeş-Bolyai University, Romania
Copyright © 2022 Daskalopoulou, D’Alessandro, Longo, Pecoraino and Calabrese. This is an open-access article distributed under the terms of the Creative Commons Attribution License (CC BY). The use, distribution or reproduction in other forums is permitted, provided the original author(s) and the copyright owner(s) are credited and that the original publication in this journal is cited, in accordance with accepted academic practice. No use, distribution or reproduction is permitted which does not comply with these terms.
*Correspondence: Kyriaki Daskalopoulou, daskalopoulou@uni-potsdam.de, kikdaskalopoulou@gmail.com