To Regulate or Not to Regulate: Assimilation of Dietary Fatty Acids in the Temperate Copepod Temora longicornis
- 1Polar Biological Oceanography, Alfred-Wegener-Institut Helmholtz-Zentrum für Polar- und Meeresforschung, Bremerhaven, Germany
- 2Marine Zoology, University of Bremen, Bremen, Germany
- 3Marine Biology, Ghent University, Gent, Belgium
- 4Biologische Anstalt Helgoland, Alfred-Wegener-Institut Helmholtz-Zentrum für Polar- und Meeresforschung, Helgoland, Germany
- 5Ecological Chemistry, Alfred-Wegener-Institut Helmholtz-Zentrum für Polar- und Meeresforschung, Bremerhaven, Germany
Consumer regulation of lipid composition during assimilation of dietary items is related to their ecology, habitat, and life cycle, and may lead to extra energetic costs associated with the conversion of dietary material into the fatty acids (FAs) necessary to meet metabolic requirements. For example, lipid-rich copepods from temperate and polar latitudes must convert assimilated dietary FAs into wax esters, an efficient type of energy storage which enables them to cope with seasonal food shortages and buoyancy requirements. Lipid-poor copepods, however, tend to not be as constrained by food availability as their lipid-rich counterparts and, thus, should have no need for modifying dietary FAs. Our objective was to test the assumption that Temora longicornis, a proxy species for lipid-poor copepods, does not regulate its lipid composition. Isotopically-enriched (13C) diatoms were fed to copepods during a 5-day laboratory experiment. Compound-specific stable isotope analysis of algae and copepod samples was performed in order to calculate dietary FA assimilation, turnover, and assimilation efficiency into copepod FAs. Approximately 65% of the total dietary lipid carbon (C) assimilated (913 ± 68 ng C ind-1 at the end of the experiment) was recorded as polyunsaturated FAs, with 20 and 15% recorded as saturated and monounsaturated FAs, respectively. As expected, T. longicornis assimilated dietary FAs in an unregulated, non-homeostatic manner, as evidenced by the changes in its FA profile, which became more similar to that of their diet. Copepods assimilated 11% of the total dietary C (or 40% of the dietary lipid C) ingested in the first two days of the experiment. In addition, 34% of their somatic growth (in C) after two days was due to the assimilation of dietary C in FAs. Global warming may lead to increased proportions of smaller copepods in the oceans, and to a lower availability of algae-produced essential FAs. In order for changes in the energy transfer in marine food webs to be better understood, it is important that future investigations assess a broader range of diets as well as lipid-poor zooplankton from oceanographic areas throughout the world’s oceans.
1 Introduction
Conventional methods for estimating the dietary composition of a consumer, such as direct observations of feeding activity and analyses of stomach content and feces, only provide snapshots of its recent diet (Michener and Kaufman, 2007; Newton, 2010). Essential qualitative and quantitative information on pathways of energy flow between prey and predators can, however, be obtained through the combined use of compounds such as fatty acids (FAs) and stable isotopes (Graeve et al., 2005; De Troch et al., 2012; Boissonnot et al., 2016), as these integrate the feeding history of a consumer over a longer time frame.
Compound-specific stable isotope analysis (CSIA) is a state-of-the-art tool ideally suited to measure the stable isotope values of single FAs, which enables its use in the investigation of biochemical processes in detail (Evershed et al., 2007; Guilini et al., 2010; Rix et al., 2018). Interest in the methodology has grown in recent decades. Most studies in the aquatic sciences report natural isotopic abundances and their relevance for food web architecture, movement ecology, and biogeochemical cycling (for examples, see McMahon and Newsome, 2019; Whiteman et al., 2019; and references therein). There are fewer studies in aquatic sciences which are of an experimental (tracer) character. These introduce stable isotopes into a monitored system or compartment and are able to report on labelled/enriched abundances in excess of the natural value (for examples, see Middelburg, 2014; Twining et al., 2020; and references therein). Such tracer studies have, for example, used CSIA to quantify processes such as the assimilation, turnover, and synthesis of important molecules at the plant-animal interface in marine food webs (Graeve et al., 2005; De Troch et al., 2012; Boissonnot et al., 2016; Franco-Santos et al., 2019; Helenius et al., 2019; Graeve et al., 2020; Helenius et al., 2020a; Helenius et al., 2020b). These processes can be investigated by adding compounds enriched in stable isotopes of biogenic elements (e.g., NaH13CO3) to algae cultures, whose isotopic signature will then become enriched with this isotope. Any consumers experimentally fed with labelled algae will also become enriched in relation to natural abundances, making it possible to follow the incorporation and modification of isotopically-enriched dietary molecules into consumer tissues (i.e., specific compounds) using CSIA.
Fatty acid dynamics have been well characterized for larger, mainly herbivorous, Calanus and Neocalanus copepod species that inhabit environments characterized by strong variation of food supply, such as polar and high latitude boreal areas and upwelling systems (Attwood and Peterson, 1989; Ohman et al., 1989; Kattner and Hagen, 1995; Scott et al., 2002; Campbell et al., 2004). The wealth of research conducted into their lipid biochemistry is due to the fact that they are an important source of energy (especially omega-3 and -6 FAs) to their food webs, but also to better understand how they adapt to marked seasonal variations in food supply. Lipid-rich copepods generally exhibit long life cycles, slow growth rates, and few generations per year (Arndt and Swadling, 2006). Given the seasonal character of food availability in high latitudes and upwelling systems, they must store lipids when possible, so that their energy reserves can fuel gonad maturation and, in some cases, pre-bloom spawning in the absence of food (Niehoff, 2007). Furthermore, the matter of whether energy reserves are triacylglycerols (TAGs) or wax esters (WEs) depends on the presence/absence of diapause in their life cycle (Conover, 1988; Lee et al., 2006; Pond, 2012). This is because of the biophysical properties of WEs, which enable copepods to achieve neutral buoyancy and deep-water diapause (Pond, 2012).
In general, fewer studies are available on the lipid biochemistry of smaller copepods (Kattner and Hagen, 2009; Boissonnot et al., 2016; Helenius et al., 2020a), which tend to store little to no energy reserves, to not undergo post-hatching diapause, and to not be as limited by food quantity as their lipid-rich counterparts. One of the species for which information is available is Temora longicornis (Müller, 1785), a small planktonic copepod (~ 1 mm prosome length) which inhabits, and often dominates, temperate and boreal environments (Holste et al., 2009; Martynova et al., 2011; Johnson and Allen, 2012). In the North Sea, this species is abundant and reproduces year-round, and its grazing can have a substantial impact on the phytoplankton standing stock (Maar et al., 2004; Wesche et al., 2007; Gentsch et al., 2009). Like many other small copepods which are typical of highly productive shelf seas, T. longicornis has a limited ability to store energy (lipid) reserves (Evjemo and Olsen, 1997; Kreibich et al., 2008; Niehoff et al., 2015). Studies have shown that, for T. longicornis (a) the FA profile can change within 24h of introduction to a new dietary source (Kreibich et al., 2008; Kreibich et al., 2011), and the response is related to its feeding history (Niehoff et al., 2015); and that (b) egg production rates and hatching success are positively related with the availability of omega-3 (or n-3) FAs (Arendt et al., 2005; Jónasdóttir et al., 2009).
Given the differences in habitat, ecology, and in overall life history strategies between lipid-rich and lipid-poor copepods, it is to be expected that these groups will display distinct FA dynamics (for a review on the topic, see Kattner and Hagen, 2009). As previously stated, the former must store energy in an effective (dense) manner in order to survive food shortages during the winter, to achieve buoyancy, and to fuel annual reproduction (Sargent and Falk-Petersen, 1988). In order to do so, they need to synthesize energy-rich WEs, molecules with a dietary-derived FA component and a de novo biosynthesis-derived fatty alcohol component (Sargent et al., 1981). Lipid-poor copepods, by definition, do not store significant energy reserves and, thus, should have no need to modify dietary FAs upon assimilation. Building upon the results from previous research, the objective of the present study was to test the assumption that T. longicornis, a proxy species for lipid-poor copepods, exhibits mild (if any) lipid homeostasis. We expect, thus, that T. longicornis will assimilate dietary FAs in a mostly unmodified manner; i.e., that the FA profile of experimental copepods should resemble the dietary composition rather than reflect the biochemistry of the field-collected copepods. In order to test this hypothesis, we conducted a feeding tracer experiment, in which the copepods were fed with isotopically-enriched (13C) diatoms and their FA assimilation, turnover rate, and assimilation efficiency (AE) were quantified with CSIA.
2 Methods
2.1 Field Sampling and Experimental Design
On May 17th, 2016, zooplankton were sampled with a 500 µm mesh-size CalCOFI net, which was towed horizontally for 15 minutes at 5 m depth off the German island of Helgoland (54°11’N, 07°54’E), in the southern North Sea. Samples were taken to the laboratory, where intact and active adult females of the copepod T. longicornis were immediately sorted under an Olympus SZX16 stereoscopic microscope. A total of 720 females were sorted, 540 for a feeding experiment and 180 for the determination of in situ elemental and biochemical compositions. This study was conducted concomitantly with that from Franco-Santos et al. (2018b).
The feeding experiment with T. longicornis was initiated immediately after sorting. Copepods were distributed among triplicate 3L plastic beakers fitted with a 300 µm meshed-bottom cylinder (75 females L-1), used to keep females from eating their own eggs and fecal pellets. Food was provided ad libitum (> 350 µg C L-1, Klein Breteler et al., 2002, at a concentration of 8,000 cells mL-1). Feeding occurred on a daily basis for five days after partial water exchanges (approx. two thirds), which removed most of the food from the previous day. The copepods were kept in a dark, temperature-controlled room at 10 ± 0.3°C, simulating the natural environment at the time of sampling. The beakers were gently stirred three times a day for food resuspension in the water.
Sampling was conducted before (day 1 or in situ composition, t0h), during (day 3, t48h), and at the end (day 6, t120h) of the experiment. A total of 10 females per replicate were pooled for elemental analysis (body C and nitrogen (N) contents and molar C:N ratio). Another 50 females per replicate were pooled for biochemical analyses (FA content and profile, and FA-specific content and 13C isotopic enrichment). Copepods were gently washed in distilled water and placed into pre-weighed tin capsules (5x9 mm, IVA Analysentechnik) for elemental analysis or pre-combusted lipid vials for FA analyses. Tin capsules with copepods were dried at 60°C for 48 h and stored in a desiccator until analysis, whereas lipid vials were stored at -80°C until further analyses.
2.2 Prey Culture
The centric diatom Thalassiosira weissflogii (Grunow) (Fryxell and Hasle, 1977), presently accepted as Conticribra weissflogii (Grunow) (Stachura-Suchoples and Williams, 2009), was used in this feeding experiment. This diatom is a common prey for T. longicornis in nature (Evans, 1981), and is well taken up by the copepod under laboratory conditions (Jónasdóttir et al., 2009; Niehoff et al., 2015; Franco-Santos et al., 2018b). Batch cultures of C. weissflogii were started on a daily basis for five consecutive days by diluting a stock solution with fresh f/2 medium (details in Franco-Santos et al., 2018b). Isotopic enrichment of the batch cultures was achieved by adding 13C-enriched sodium bicarbonate (NaH13CO3) powder to the f/2 medium at a concentration of 4 mg L-1. Diatoms were allowed to grow for five days to uptake labeling. Cultures were sampled daily during the experiment for determination of cell elemental (C and N contents and molar C:N ratio) and biochemical (FA content and profile, and FA-specific content and 13C isotopic enrichment) compositions, and subsequently used as food suspension. Cell density in the cultures was determined with a BD Accuri C6 Flow Cytometer. Subsamples of 5.2 *106 cells were filtered through pre-combusted (500°C for 24h) Whatman GF/F filters (0.7 µm pore size, 25 mm diameter). Filters with samples for elemental analysis were dried at 60°C for 48 h, folded inside tin foil, and stored in a desiccator until analysis. Filters with samples for FA analyses were placed into pre-combusted lipid vials and stored at -80°C until analyses.
2.3 Elemental, Biochemical, and Compound-Specific Stable Isotope (CSIA) Analyses
The C and N contents of copepod and prey culture samples were measured with an elemental analyzer (detection limit: 2 µg C/0.5 µg N; maximum error: ± 3%, Euro EA 3000, EuroVector S.P.A., Milan, Italy) using acetanilide as a standard. Prior to C and N determination, tin capsules with copepods were weighed on a microbalance (readability: 0.1 µg, XP6U, Mettler Toledo, Switzerland) in order to obtain copepod dry mass (DM).
The methodology used for lipid extraction (modified after Folch et al., 1957) and subsequent FA identification is described in Boissonnot et al. (2016) and Franco-Santos et al. (2019). Briefly, lipids were extracted from samples after homogenization in a dichloromethane:methanol (2:1, v:v) solution. A known amount of the internal standard tricosanoic acid methyl ester (23:0) was added to each sample in order to calculate the fatty acid methyl ester (FAME) content. A biphasic system was created with the addition of potassium chloride (KCl, 0.88% solution). The (separated) lipid layer was then transesterified for 4 h with sulfuric acid (H2SO4, 3% solution) in methanol at 80°C under nitrogen atmosphere. Cyclohexane was used to extract the FAMEs, which were subsequently determined with a gas chromatograph (HP 6890 N, Agilent Technologies Deutschland GmbH & Co. KG). The software ChemStation (Agilent) was used to evaluate the chromatograms generated. The A:B(n-X) shorthand notation was used to refer to FAs, where A is the number of carbon atoms, B is the number of double bonds, and (n-X) gives the position of the double bond closest to the terminal methyl group. Temora longicornis does not have major storage reserves and exhibits TAG as its primary neutral lipids (Fraser et al., 1989; Peters et al., 2013); therefore, lipid classes were not separated and FAMEs were assumed to be TAG in the present study. The sum of all FAs (TFA) was used to calculate the total lipid content (TLC) of copepods based on the notion that, in species which store FAs as TAG, FAs comprise approximately 80-90% of total lipids (i.e., the saponifiable fraction of lipids; Vogedes et al., 2010).
The 13C isotopic enrichment of FAMEs was measured with a Thermo GC-c-IRMS (gas chromatography-combustion-isotope-ratio mass spectrometry) system, equipped with a Trace GC Ultra gas chromatograph, a GC Isolink operated in combustion mode at 1,000°C and a Delta V Plus isotope ratio mass spectrometer connected via a Conflo IV interface (Thermo Scientific Corporation, Bremen, Germany), according to Boissonnot et al. (2016). The instrument-specific software Isodat 3.0 (Thermo Scientific) was used to generate the chromatograms containing FA peak areas and peak-specific C-isotope ratios. The 14:0 and 18:0 FAME reference standards (Iowa University) with known δ-values were used for further calculations and normalizations (Paul et al., 2007). The equations used by Boissonnot et al. (2016) and Franco-Santos et al. (2019) to calculate lipid C assimilation and turnover were also applied in the present study, and are provided in the supplementary material. Briefly, FA-specific C assimilation was calculated by multiplying the FA mass (in C units) by its excess isotopic signal (atom percent excess, APE) and dividing this number by the average isotopic signal of the dietary FAs. Total lipid C assimilation was, thus, the sum of all FA-specific assimilation values. The exchange of copepod FAs by labeled dietary FAs was obtained by dividing the total lipid C assimilation at t48h or t120h by the absolute mass of TFAs (in C units) at the respective time. Turnover rates were derived from dividing the latter (C exchange) by the number of days passed at the respective time (2 days for t48h or 5 days for t120h).
All raw data and associated equations and calculations can be accessed in the data sets stored with the repository PANGAEA (Franco-Santos et al., 2022). It should be noted that these PANGAEA data sets contain results from two different experiments conducted with Temora longicornis. The data included and analyzed in this study only refer to the experiment which used a nutrient-replete culture of C. weissflogii (identified by the experimental treatment diet abbreviation “Diat+”) to feed the copepods.
2.4 Fatty Acid C Assimilation Efficiencies
Lipid C assimilation efficiency (AE), the percentage of (13C isotopically-enriched) dietary content ingested by copepods that was assimilated into FAs, was calculated for (a) TFA, (b) saturation-specific sums of FAs (saturated, monounsaturated, and polyunsaturated FAs – SFA, MUFA, and PUFA, respectively), and (c) each individual FA that was both available from the diet and assimilated by copepods (> 1% TFA in copepods). It was calculated in two steps:
Ingestion rate of FA-derived dietary C by copepods (FAIngDietC (Y), in µg C ind-1 day-1), which multiplies copepod cell ingestion rates (CellsIng, in cells ind-1 day-1, obtained from Franco-Santos et al., 2018a; Franco-Santos et al., 2018b) by FA-specific dietary C contents (FAdietC (Y), in µg C cell-1):
where “Y” indicates either TFA, the saturation-specific sum of FAs, or the specific FA for which the calculation was carried.
AE of lipid C (%), which divides the C assimilation of “Y” (obtained in the present study, available for t48h and for t120h) by the multiplication of the ingestion rate of FA-derived dietary C by copepods for “Y” and the number of days (∆day) for which C assimilation of “Y” was calculated (2 or 5 days for t48h and t120h, respectively):
The above-mentioned parameter values are available from the PANGAEA data sets (Franco-Santos et al., 2018a; Franco-Santos et al., 2022). In the data sets, they are referred to as “T. longicornis f assim eff FA” (AE), “T. longicornis f IR FA/ind” (FAIngDietC (Y)), “T. longicornis f IR cells/ind” (CellsIng), and “FA C cell” (FAdietC (Y)).
2.5 Statistical Analyses
The statistical significance of differences in DM, C and N contents, molar C:N ratio, and TFA content between the different copepod samples (t0h, t48h, and t120h) was investigated with one-way analyses of variance (ANOVAs). The origin of differences was identified by applying the Tukey HSD (Honestly Significant Difference) post-hoc test with a 95% confidence limit. Prior to the ANOVAs, the data were tested for normality and homogeneity of variances with Shapiro-Wilk and Bartlett tests, as well as graphically tested with histograms/QQ-plots and conditional boxplots (Zuur et al., 2010), respectively. All univariate analyses were performed using R v. 3.4.4 (Team, 2013). Average values given in the text are often accompanied by standard deviation values.
Differences in the FA profile of diatoms and copepods were investigated with multivariate analyses in order to infer copepod lipid accumulation patterns. Data were analyzed as logit-transformed percentage data (as suggested by Warton and Hui, 2011) for FAs with relative content > 1% TFA and for the sum of FAs with < 1% TFA. A principal component analysis (PCA) was computed for visualization of FA profiles. In addition, a similarity percentages (SIMPER) analysis was conducted on the logit-transformed percentage data to identify the specific FAs contributing to the differences visualized in the PCA. Multivariate analyses were performed with PRIMER 7.0 software (Clarke and Gorley, 2015).
3 Results
3.1 Prey Cultures
Cell C, N, and TFA contents in diatom cultures averaged 71 ± 5 pg C cell-1, 14 ± 2 pg N cell-1, and 13 ± 1 pg cell-1, respectively, and molar C:N ratio was 6.2 ± 0.6. The 13C isotopic enrichment was similar for all FAs with the exception of 18:0, as accounted for by the CSIA calculations described in the supplementary material. The average enrichment (L) in all FAs at t120h was 2.63 ± 0.13 atom%, a value which increased to 2.69 ± 0.13 atom% when the FA 18:0 was excluded from the calculations (for FA-specific isotopic enrichment values, please refer to Franco-Santos et al., 2022). The average enrichment for the FA 18:0 at t120h was 1.49 ± 0.15 atom%. A total of 20 FAs were identified in diatom samples, of which nine were present in concentrations > 1% TFA (Table 1). Their FA profile (Table 1, Figure 1) was mostly characterized by PUFAs, which accounted for 60 ± 1% TFA content, whereas SFAs and MUFAs were present in similar quantities (22 ± 1 and 18 ± 0% TFA, respectively). The most abundant FAs were 20:5(n-3) (eicosapentaenoic acid, EPA), 16:3(n-4), 16:1(n-7), and 16:0 (Table 1, Figure 2).
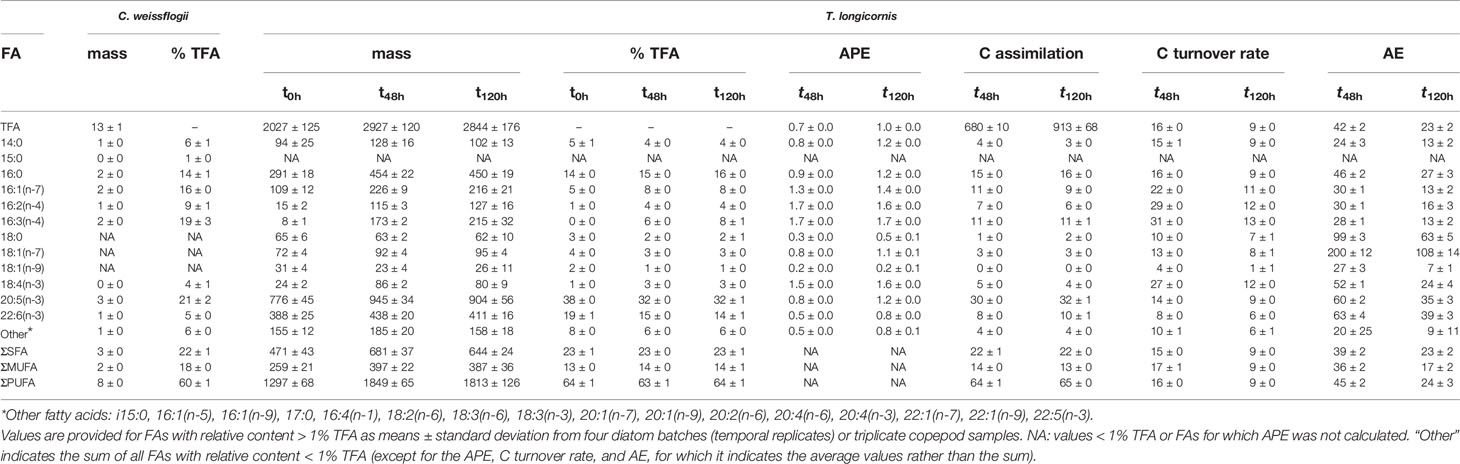
Table 1 Absolute (mass, in pg cell−1 for diatoms and ng ind-1 for copepods) and relative (% TFA) FA contents of diatom cultures and copepod samples; and copepod TFA, FA-specific, and saturation-specific (SFA, MUFA, and PUFA) 13C isotopic enrichment (APE), C assimilation (as ng C ind-1 for TFA and as % TFA assimilated for individual FAs), C turnover rate (as % day1), and C assimilation efficiency (AE, as %) between t0h-t48h and between t0h-t120h.
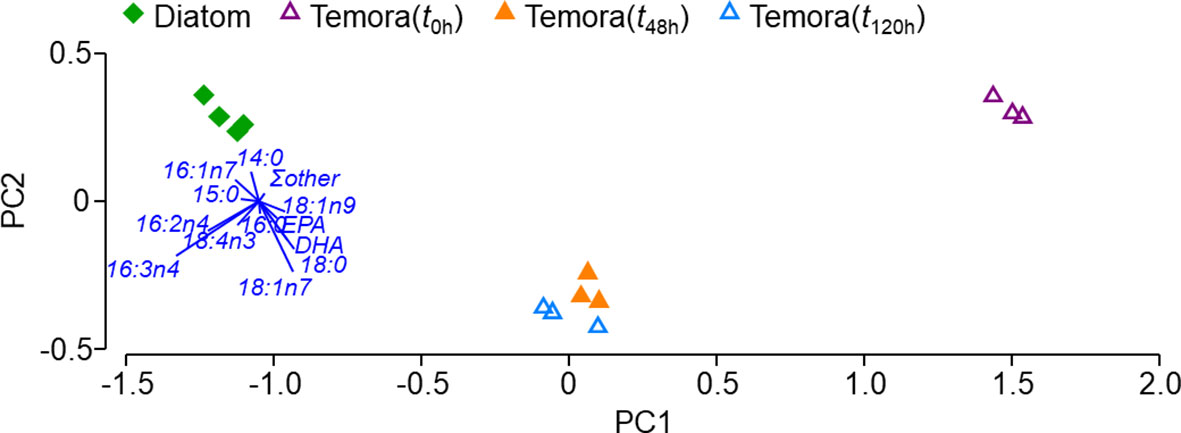
Figure 1 Principal component analysis (PCA) illustrating the relative FA profile (for FAs >1% TFA and the sum of FAs <1% TFA) of copepods (at the three sampling times) and of their diatom prey. Important FAs for characterizing overall FA profile were overlaid as vectors.
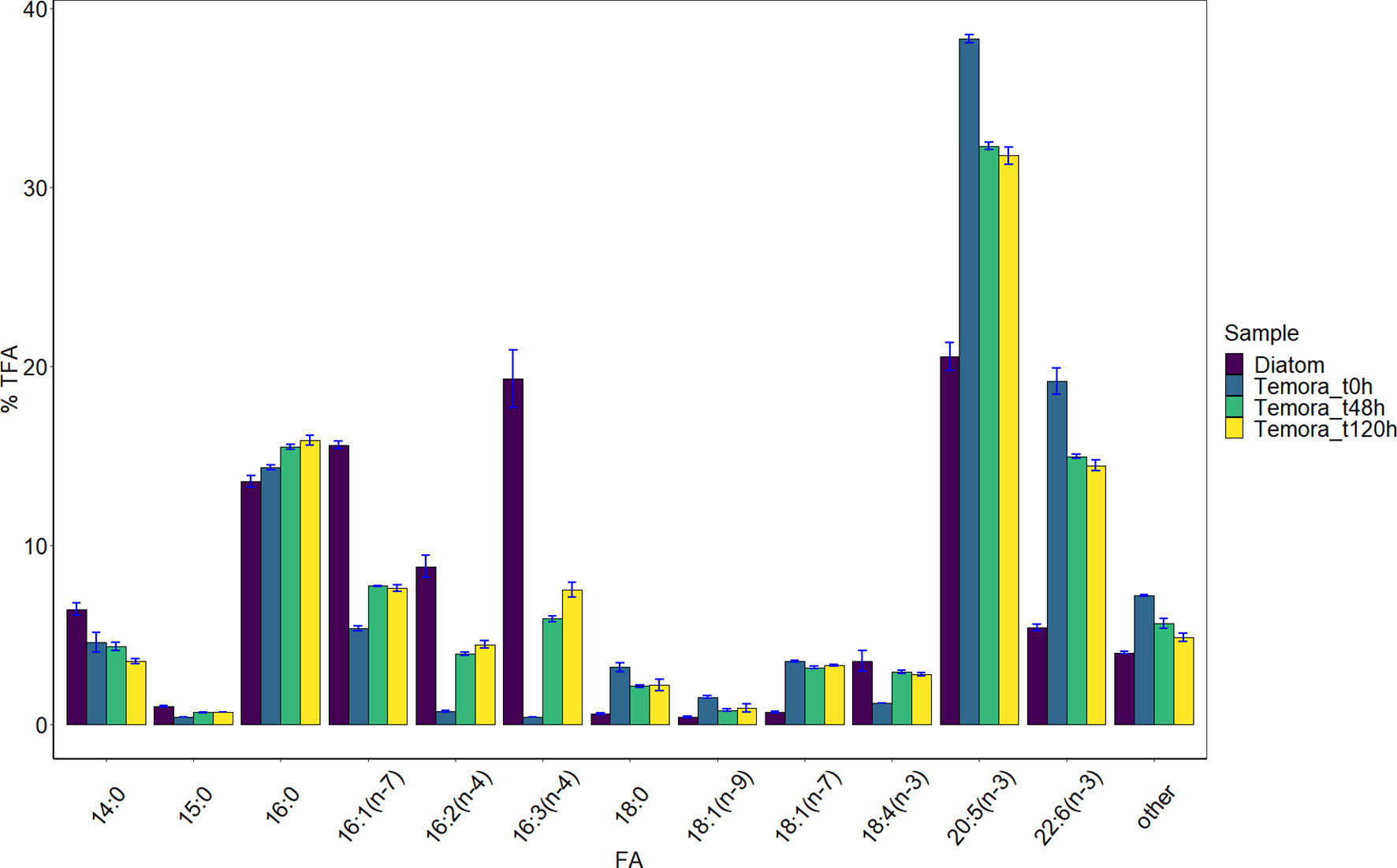
Figure 2 Fatty acid profile of diatom cultures and copepods (at the three sampling times). Mean relative values (% TFA) and standard deviation bars (atop each FA bar) are shown for major FAs (>1% TFA) and for the sum of minor FAs (referred to as “other”).
3.2 Copepods
3.2.1 Elemental and Biochemical Compositions
Copepod DM was significantly lower at t0h (36 ± 2 µg ind-1) than during the experiment (ANOVA; F=53.68; df=2,6; p=1.5*10-4/Tukey; p<0.001 for both tests), when it reached 47 ± 1 and 49 ± 1 µg ind-1 at t48h at t120h, respectively. Average C and N contents of copepods also increased significantly between t0h and t48h, from 16 ± 1 to 20 ± 0 µg C ind-1 and from 4 ± 0 to 5 ± 0 µg N ind-1, and remained the same thereafter (ANOVAs; F=29.63; df=2,6; p=7.8*10-4 for C content and F=16.33; df=2,6; p=3.7*10-3 for N content/Tukey; p<0.01 for both tests). The molar C:N ratio first increased significantly (ANOVA; F=7.125; df=2,6; p=0.026/Tukey; p<0.05) between t0h and t48h, from 4.7 ± 0.1 to 4.9 ± 0.1, and then decreased to 4.7 ± 0.1 (not statistically significant). Average TFA content also increased significantly (ANOVA; F=36.6; df=2,6; p=4.4*10-4/Tukey; p<0.001) from 2.0 ± 0.1 to 2.9 ± 0.1 µg ind-1 between t0h and t48h and then remained stable (2.8 ± 0.2 µg ind-1 at t120h). Using the previously mentioned TFA equivalence to 80-90% of TLC, we estimated that TLC increased from 2.2-2.5 ± 0.2 µg ind-1 (6-7 ± 1% DM) at t0h to 3.2-3.6 ± 0.2 µg ind-1 (7-8 ± 0% DM) at t48h and remained at 3.2-3.6 ± 0.2 µg ind-1 until t120h (6-7 ± 0% DM).
3.2.2 Fatty Acid Profile and 13C Isotopic Enrichment
A total of 27 FAs were identified in copepod samples, though only 11 had a relative abundance >1% TFA (Table 1, Figure 2). The major FAs were EPA and 22:6(n-3) (docosahexaenoic acid, DHA), which accounted for 32-38 and 14-19% TFA, respectively, followed by the FAs 16:0 and 16:1(n-7) (14-16 and 5-8% TFA, respectively) (Table 1). The relative content of the diatom fatty acid trophic markers (FATM), 16:1(n-7), 16:2(n-4), and 16:3(n-4), increased in copepods throughout the experiment, though 16:1(n-7) decreased slightly (< 1% TFA) in the last three days. The relative EPA content, on the other hand, progressively decreased with days (Figure 2). The relative SFA, MUFA, and PUFA contents of copepods remained similar throughout the experiment (23, 13-14, and 63-64% TFA, respectively).
As copepods were fed with diatom cultures, their FA profile changed towards a profile more similar to that of their diet, though not exactly the same (Figures 1 and 2). The PCA in Figure 1 clearly shows a difference in FA profiles between the diatom diet, the field copepods, and the copepods sampled during the experiment. On the horizontal scale, the FA profile of experimental copepods (t48h and t120h) changed from that observed for the individuals in situ (t0h samples, far right on plot) towards that of their prey (diatom samples, far left on plot), being situated approximately half way between these two. On the vertical scale, the PCA seems to indicate that differences in FA profile between the experimental copepods and their prey is likely due to FAs such as 18:1(n-7), 18:0, and DHA. The SIMPER confirmed these patterns, and revealed that differences in FA profile between (a) t0h copepods and diatoms were mainly due to the FAs 16:3(n-4) and 16:2(n-4), which were present in low concentrations in the field copepods and contributed to 23 and 14% of the variation, respectively; (b) field and experimental copepods were due to the FAs 16:3(n-4), 16:2(n-4), and18:4(n-3), which were present in lower concentrations in t0h copepods and contributed to 33, 20, and 10% variation, respectively; and (c) diatoms and experimental (t48h/t120h) copepods were mainly due to the FAs 18:1(n-7), 18:0, 16:3(n-4), and DHA, which were all present in lower relative concentrations in diatoms (except for 16:3(n-4)) and contributed to 16, 14, 12, and 11% of the variation, respectively.
The average 13C isotopic enrichment of copepods, calculated as the difference between the atom% of field and experimental individuals, increased as the experiment progressed, from 0.74 ± 0.03 APE at t48h to 0.95 ± 0.01 APE at t120h. FA-specific 13C isotopic enrichment values are provided in Table 1 (for all FA-specific values please refer to Franco-Santos et al., 2022).
3.2.3 Lipid Carbon Assimilation and Turnover
The average total assimilation of C into copepod FAs was 680 ± 10 ng C ind-1 in the first two days of the experiment and 913 ± 68 ng C ind-1 for the entire experiment duration (Table 1, Figure 3). From the total lipid C assimilated by copepods, approximately 65% were PUFAs, 20% SFAs, and 15% MUFAs (Table 1, Figure 3). Six FAs accounted for approximately 85% of all C assimilated into FAs by copepods - EPA and 16:0 accounted for ~ 30 and 15%, respectively, and DHA for another ~ 10%. Each of the three diatom FATM, 16:1(n-7), 16:2(n-4), and 16:3(n-4), accounted between 6 and 11% of the total C assimilated into FAs (Table 1, Figure 3). Overall, copepod TFA, saturation-specific, and FA-specific C assimilation rates were higher during the first two days of the experiment (steeper slopes in Figure 3) than during the last three days of the experiment (gentler slopes in Figure 3). A total of 11 ± 0% of the dietary C ingested by copepods was assimilated into FAs in the first two days of the experiment (refer to calculations for “T. longicornis f C assim/IR C” in Franco-Santos et al., 2022). In addition, C assimilation into FAs accounted for 34 ± 3% of copepod somatic growth during that same period (refer to calculations for “T. longicornis f C assim/μ C” in Franco-Santos et al., 2022). Furthermore, copepods assimilated 13C isotopically-enriched FAs which were not detected in the diet, namely 16:4(n-1), 17:0, C20 MUFA, 20:2(n-6), C22 MUFA, and 22:5(n-3) (docosapentaenoic acid, DPA); and failed to assimilate the FA 16:1(n-9), which was present in the diatom cultures.
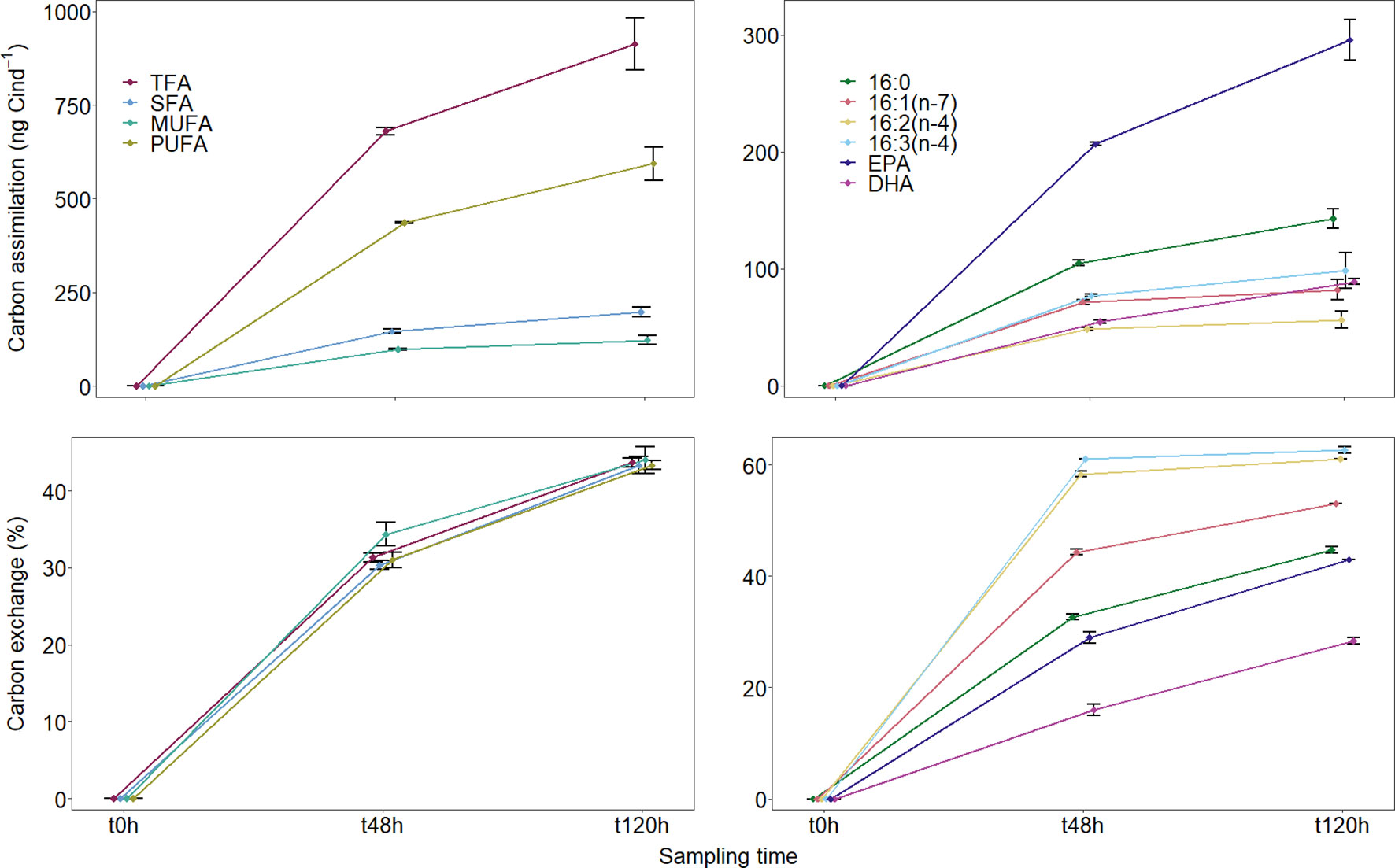
Figure 3 Total (TFA), saturation-specific groups (left column), and FA-specific (right column) values for C assimilation (ng C ind-1, upper row) and exchange (%, lower row) recorded for Temora longicornis during the feeding experiment (at t48h and t120h). Values are mean ± standard deviation of triplicate samples.
The endogenous lipid C content exchanged for dietary FAs in copepods increased from 31 ± 1% TFA on day two of the experiment (t48h) to 43 ± 1% TFA at the end of the experiment (Table 1, Figure 3). The TFA turnover rates, thus, decreased with experimental time, from 16 ± 0% day-1 (t48h) to 9 ± 0% day-1 (t120h), a pattern similar to that shown by the assimilation rates (Table 1, Figure 3). Similar lipid C exchange values were observed for SFA, MUFA, and PUFA at both sampling times (Table 1, Figure 3). The diatom FATMs showed some of the highest FA-specific C exchange, 44-53, 59-61, and 61-63% for 16:1(n-7), 16:2(n-4), and 16:3(n-4), respectively. Although C assimilation values for the FA 18:4(n-3) were lower than those for the above-mentioned FAs (approximately 5% TFA assimilated), almost 60% of the initial 18:4(n-3) content had been replaced with 13C-enriched dietary C by the end of the experiment (Table 1). Exchange of EPA and DHA was slightly lower, varying between 29-43% and 16-28%, respectively.
3.2.4 Lipid Carbon Assimilation Efficiency (AE)
Average AE of dietary C into copepod FAs also decreased in the last three days of the experiment in comparison to the first two days; this was valid whether AE was calculated for TFA, saturation-specific sums of FAs (i.e., SFA, MUFA, or PUFA), or individual FAs (Table 1). The average AE for TFA was approximately 40 and 25% at t48h and t120h, respectively; similar AE values were obtained for SFA and PUFA and slightly lower values for MUFA. Approximately half of the major FAs (>1% TFA) had higher AE values than TFA, and half had lower values. Assimilation efficiency values of 93-212% were recorded for the FA 18:1(n-7), and the FA 18:0 had a mean AE of almost 100% at t48h. Copepods assimilated more than half of the dietary EPA and DHA ingested in the first two days (60 and 63%, respectively), though AE was lower when calculated for t120h. Although the C assimilation of diatom FATMs was among the highest recorded for individual FAs, their AE was among the lowest calculated.
4 Discussion
In the present study we experimentally tested the hypothesis that lipid-poor copepods exhibit little to no homeostatic regulation in their lipid composition. Overall, the results supported our hypothesis, as evidenced by the changes in the FA profile of copepods, which began resembling the FA profile of their experimental diet during the feeding experiment (Figure 1). Although copepods assimilated dietary FA mostly in an unmodified manner, evidence is also provided for minor reworking of dietary FAs during assimilation. Copepods assimilated 11% of the total dietary C (or 40% of the dietary lipid C) ingested in the first two days of the experiment, and 34% of their somatic growth (in C units) at t48h was due to the assimilation of dietary lipid C. Only 11 studies have been published to date which use CSIA to quantify trophodynamics in copepods. Of these, they address: benthic copepods (De Troch et al., 2012; Werbrouck et al., 2017), polar calanoids (Graeve et al., 2005; Helenius et al., 2019; Graeve et al., 2020; Helenius et al., 2020b), T. longicornis (Grice et al., 1998; Klein Breteler et al., 2002), and other non-Temora small, lipid-poor copepods (Boissonnot et al., 2016; Burian et al., 2018; Helenius et al., 2020a). Our results are discussed below in light of relevant published data for pelagic copepods.
4.1 Effects of Experimental Diet on the TFA Content and Profile of Temora Longicornis
Copepods showed an increase in TFA (content and DM) during the experiment in comparison to in situ values, confirming their active uptake of the diatom diet (as also confirmed by ingestion rates reported by Franco-Santos et al., 2018a; Franco-Santos et al., 2018b). This indicates at least one of two possibilities, though more likely a combination of both. First, that copepods may have been C-limited in the field (a condition previously shown for T. longicornis in the same area and month by Niehoff et al., 2015) and, thus, replenished their FA/energy depots when exposed to a C-rich experimental diet. Anderson et al. (2021) calculated that nutrient limitation of marine copepods changes from N- to C-limitation at food C:N ratios lower than 7.3-11.5 (depending on food quantity). At the time of sampling in Helgoland, seston C content was 301 ± 8 µg C L-1, and the molar C:N ratio was 7.8 ± 0.2, so it is possible that copepods may have been C-limited. The chlorophyll a concentration in the seston at the time and location of sampling was 1.5 ± 0.1 µg chl a L-1, a low value in comparison to peak values during spring and summer (Amorim et al., 2021), further indicating limited food quantity in the field. The second possibility is that small copepods such as T. longicornis do have some ability to store energy reserves – from 9.5 to 11.5% body C (this study; Evjemo and Olsen, 1997; Evjemo et al., 2003). These values are within the range of those obtained for another small (~ 5 µg C ind-1) and ubiquitous copepod, Acartia tonsa (Acanthacartia) Dana (1849) (9 - 14.5% body C content; Veloza et al., 2006).
The FA profile of copepods appeared to be influenced by that of the diatom cultures within the first 48h of the experiment (Figure 1), a result in agreement with those from Kreibich et al. (2008; 2011). Copepods assimilated dietary FA in a non-homeostatic manner, and FA-specific trends (increasing and decreasing content) during the laboratory experiment followed the FA-specific availability in the diet. As an example, the absolute content of diatom FATM in copepods soared: that of 16:1(n-7) more than doubled, that of 16:2(n-4) was about 8x as high, and that of 16:3(n-4) increased by 20-25x (~10, 7, and 11% of all FA assimilated, respectively). The EPA and DHA compositions of another small, lipid-poor copepod (Eurytemora herdmani Thompson and Scott, 1897), were also found to be influenced by, though not tightly-coupled with, the content of these FAs in their dietary sources. It should be noted, however, that this trend may not be the same for all lipids. Grice et al. (1998) reported a differentiation in profile between T. longicornis and its diets, Rhodomonas sp. (Karsten, 1898) and Isochrysis galbana (Parke, 1949), when investigating sterols, which are isoprenoid-derived lipids with essential roles in cell structure, function, and physiology. Although the authors did not conduct a labeling tracer experiment, the significant presence of sterols in the copepods which were not observed in their diets cannot be ignored. Similarly, earlier studies with Calanus helgolandicus (Claus, 1863) also reported little overlap between the sterol composition of copepods and that of its food sources, as well as cholesterol production in copepods fed with a cholesterol-free diet (Prahl et al., 1984; Harvey et al., 1987).
Although changes in the FA profile of T. longicornis reflected the dietary FA composition and availability, minor modifications of dietary FAs were also recorded. 13C isotopically-enriched assimilation by copepods during the experiment was registered for half of the minor FAs (<1% TFA), even though these FAs were not detected in the diet. This observation indicates that copepods may have biosynthesized minor FAs from a precursor dietary FA or non-lipoidal material. These modifications were, however, at such low levels that they cannot characterize a pattern of regulated (homeostatic) dietary FA assimilation. In the case of DPA (a precursor of DHA), for example, the incorporation corresponded to only 0.3% of all the 13C isotopically-enriched FAs assimilated by copepods. It is also likely that copepods biosynthesized 18:1(n-7) from any assimilated dietary precursor 16:1(n-7), which lead to the differentiation of their FA profile in relation to that of their prey (Figure 1). The AE values for 18:1(n-7) also support the claim that this FA was at least partially biosynthesized from the diatom FATM precursor 16:1(n-7). The AE of 18:1(n-7) reached more than 100% (up to 252% at t48h), meaning that copepods assimilated more 18:1(n-7) in its 13C isotopically-enriched form than they actually ingested from the diet. Similar results were obtained for EPA from Calanus finmarchicus (Gunnerus, 1770) feeding on dinoflagellates, with gross growth efficiencies (the equivalent to our AE) reported to be well above 100% (Helenius et al., 2020b). Conversely, the dietary FA 16:1(n-9) was not detected in copepods, an indication that it was either metabolized, bioconverted to another FA in its entirety during assimilation, or egested before assimilation. The latter scenario (egestion) was indeed reported for C. finmarchicus feeding on the same diatom species used herein, though this result did not include estimations of the isotopic signal of 16:1(n-9) in fecal pellets (Helenius et al., 2020b).
It is also apparent that copepods selectively assimilated certain dietary FAs. This pattern can be easily identified when two or more FAs are present in similar relative proportions in the diatom cultures but are differentially assimilated by the copepods. In this study, copepods assimilated approximately 2-3x as much DHA and EPA than other FAs available with similar relative proportions in the diet (14:0 and 16:3(n-4), respectively; Table 1). It should be reiterated that this is a minor ‘regulated’ component of overall dietary FA assimilation. It appears that certain dietary FAs (such as PUFAs with 16 C atoms) are used by copepods either to build energy reserves or to provide immediate energy, but not for both, although this pattern is not yet fully understood (Graeve et al., 2020). The assimilation and subsequent storage of dietary C and, more specifically, FATM, into consumer FAs depends on various factors, such as its developmental stage, life history strategies, physiological stress, environmental temperature, among others (Galloway and Budge, 2020; Graeve et al., 2020). C16 PUFAs such as 16:2(n-4) and 16:3(n-4) are not essential for membranes and thought to only be used for obtaining energy (mitochondrial β-oxidation; Leonard et al., 2004). They were not retained in storage lipids of Antarctic copepods (Graeve et al., 2020), and had some of the lowest AE recorded in our study. EPA and DHA, on the other hand, are dominant components in phospholipids (i.e., indispensable for membranes). Thus, it is not surprising that EPA and DHA would be selectively assimilated in relation to C16 PUFAs in copepods, or in any other marine zooplankton for that matter. As a matter of fact, experimental studies have proposed that, in order to maintain EPA and DHA levels constant and to carry out important physiological processes, animals might be able to adjust their retention efficiency of these PUFAs depending on their food quality (Twining et al., 2016).
The above-mentioned results are in agreement with the predictions made by the Lipid Homeostasis Hypothesis (LHH) for holoplankton with intermediate total lipid content (Franco-Santos et al., 2019); as were those of Helenius et al. (2020a). Briefly, the LHH postulates that the strength of lipid homeostasis in zooplankton can be predicted by the size of the lipid reserves (i.e., total lipid content, TLC) of a species and, thus, be separated into three categories. Zooplankton with intermediate TLC (15-25% DM), such as many small copepods from temperate regions, should be more flexible in their FA composition. They will generally assimilate dietary FAs in a mostly unmodified (non-homeostatic) manner and invest this energy into fast growth and reproduction, and they do not produce large lipid reserves. Even though the TLC of T. longicornis herein was < 10% DM, the species can certainly be considered as a representative of holoplankton with intermediate TLC in terms of their life-history strategies. The FA dynamics recorded herein contrast with those observed by Franco-Santos et al. (2019) for the lipid-poor meroplanktonic larvae of the reef-building polychaete Lanice conchilega (Pallas, 1766). According to the LHH, lipid-poor meroplanktonic larvae (TLC < 15% DM) have specific FA requirements to perform body functions. As such, they must modify dietary lipids during assimilation in a regulated (homeostatic) fashion in order to ensure that their functional and structural requirements are met. When fed with the same diatom diet used herein, L. conchilega regulated their lipid assimilation. Unlike the copepods, the polychaetes barely assimilated the diatom FATMs 16:1(n-7), 16:2(n-4), and 16:3(n-4) (7, 2, and 1% of all TFA assimilated, respectively), despite their high availability in the diet. Furthermore, the larvae displayed a more significant (a) modification of dietary FAs (e.g., DPA incorporation corresponded to 13% of all 13C isotopically-enriched FAs assimilated, compared to 0.3% in T. longicornis), and (b) selective assimilation (20x) of EPA in comparison to 16:3(n-4) (for copepods, that was in the order of 3x). The LHH also postulates that lipid-rich zooplankton (TLC > 30% DM), such as polar calanoids, partially regulate their lipid assimilation. Because they must store assimilated dietary energy in a dense and effective configuration in order to survive seasonally low food availability in winter and/or to regulate buoyancy, they partially convert dietary FAs into longer-chain moieties to build up WEs consisting of long-chain FAs and fatty alcohols (>18 C atoms per molecule). According to the LHH, lipid-rich zooplankton should be characterized as semi-homeostatic.
It is also important to highlight that consumers will likely always modify a fraction of the dietary matter assimilated. Whether species-specific lipid homeostatic regulation is weak, strict, or somewhere in between, will depend on how significant and how extensive is the modification of dietary material during or upon assimilation. Therefore, it would be interesting that future experiments consider feeding zooplankton with different diets and, more importantly, with mixed diets of varying nutritional quality. Consumer rates of (prey) ingestion, respiration, excretion, and production of eggs and feces are affected by the nutritional quality of its diet (e.g., Franco-Santos et al., 2018b). If lipid-poor holoplankton were to feed on nutrient-deficient diets, they could potentially need to modify dietary FAs during assimilation to meet their metabolic requirements. If they are able to synthesize the necessary FAs, they will have to spend more energy for lipid homeostasis. If, however, they require FAs which they cannot obtain in sufficient quantities from modified dietary material, such as essential FAs (EFAs), then they will likely eventually die from the lack of these nutrients. In the case of T. longicornis, modification of dietary FAs upon assimilation has not been observed when copepods were fed with single diets of diatoms and dinoflagellates cultured under Nitrogen-depleted conditions (Franco-Santos et al., 2022).
4.2 Lipid C Assimilation, Turnover, and Assimilation Efficiency
The rate of assimilation of dietary C into copepod FAs decreased considerably in the last three days of the experiment in comparison to the first two - by 62-85% (Figure 3). Eurytemora herdmani feeding on the same diatom species used in the present study also showed temporal changes in assimilation of EPA and DHA, though after reaching a maximum value at day six, total FA assimilated actually decreased (i.e., was metabolized and lost) by day eight (Helenius et al., 2020a). The authors found that gross growth efficiency was, thus, higher in the first half of their experiment. The turnover of C in copepod FAs in our study was also significantly lower in the last three days of the experiment. These results suggest that, while copepods were able to accumulate dietary energy when it was made available, the speed with which they did so decreased as saturation was approached and their depots were replenished. Small, temperate copepod species which have high metabolic needs may be able to switch between herbivory and omnivory and, thus, feed year-round. They generally do not depend on the accumulation of large lipid reserves for guaranteed survival and reproduction. Although T. longicornis is omnivorous and feeds opportunistically (Gentsch et al., 2009; Löder et al., 2011; Peters et al., 2013), its ability to store some of its body C content in lipid reserves indicates that energy storage may be important for its life strategy. On a shorter-term scale, stored energy could be mobilized for demanding metabolic processes or during events of food scarcity, thus enabling copepods to cope with environmental changes in feeding conditions (Gentsch et al., 2009; Boissonnot et al., 2016). On a longer-term scale, stored energy might enable this species to actively grow and reproduce during the winter in the North Sea (Wesche et al., 2007). It is also possible that, once energy reserves were rebuilt and secondary production was no longer limited by food quantity, copepods prioritized the investment of dietary energy into reproductive output. These copepods produced 39 ± 9 eggs female-1 day1 (Franco-Santos et al., 2018a; Franco-Santos et al., 2018b) between t48h and t72h, values similar to those reported in the literature for this species (for references, see Franco-Santos et al., 2018b). However, it would be necessary to have egg production rates measured between t0h and t48h in order to confirm which process (building energy reserves or reproduction) was prioritized at each stage of the experiment.
High efficiency in lipid assimilation is usually regarded as an adaptation of herbivorous copepods from higher latitudes to the seasonal fluctuations of food supply (for a review on the topic, see Hagen and Auel, 2001). The lipid C turnover rates deducted from Graeve et al. (2005) for larger calanoids from high latitudes (C. finmarchicus, 5.4% day-1; Calanus glacialis (Jaschnov, 1955), 0.6% day-1), and provided by Boissonnot et al. (2016) for small temperate copepods (Pseudocalanus minutus (Krøyer, 1845), 2.6% day-1; Oithona similis (Claus, 1866), 0.5% day-1) are much lower than the ones obtained in the present study (between 9 and 15% day-1). This is true (for all but C. finmarchicus) even if only the rates for the last three days of the experiment, 4 ± 2% day-1, are considered (one of the replicates had a 1.9% day-1 rate). Only E. herdmani was inferred (from isotopic signal and gross growth efficiency) to exhibit substantial turnover of DHA when fed with a dinoflagellate, but without actual rates we cannot quantitatively compare these results to ours. Together with the C assimilation values of 11% of total C (or 40% of lipid C) ingested, our turnover rates are an indication of how well adapted T. longicornis is to feeding on diatoms and to rapidly assimilating dietary lipids from this diet.
Polyunsaturated omega-3 FAs (such as EPA and DHA) play vital physiological roles, such as structural components of biomembranes, hormone regulation, and immune functions (Brett and Muller-Navarra, 1997; Arts and Kohler, 2009). They are thought to control zooplankton growth and productivity, and their content in dietary items has been shown to be an important food quality indicator (Jónasdóttir et al., 1995; Müller-Navarra, 1995; Müller-Navarra et al., 2000). EPA is a precursor to several molecules involved in reproductive processes; DHA is essential for neural function; and both have an important role in cellular physiology regulating membrane fluidity (Brett and Muller-Navarra, 1997; Sterner and Schulz, 1998; Jónasdóttir et al., 2009). As expected, approximately 65% of all the dietary lipid C assimilated by copepods consisted of PUFAs, mostly EPA and DHA (~ 30 and 10% of TFA assimilated, respectively, reflecting differences in the availability of these FAs from the diet). The assimilation of these EFAs in the first couple of days of our experiment (30 and 8 ng C ind-1, respectively) was higher than that observed for E. herdmani feeding on the same food (approximately 5 and 6 ng C ind-1, respectively) for approximately the same amount of time, though within a few more days the later had assimilated 5-6x more EPA and DHA than T. longicornis (Helenius et al., 2020a). The AE of our copepods for EPA and DHA was among the highest between FAs, approximately 60% at t48h. The average AEs obtained for EPA and DHA in this study were considerably (up to 13x) higher than those recorded for these FAs in C. finmarchicus feeding for 8 days on 13C isotopically-enriched Rhodomonas salina (Wislouch) (Hill and Wetherbee, 1989, Helenius et al., 2019). Our average AE for the FA 18:4(n-3) in T. longicornis was also (up to 3.5x) higher than that presented by Helenius et al. (2019) for C. finmarchicus. To our knowledge these are the only FA-specific AE available in the literature to have been calculated from tracer feeding experiments. Copepods were not only efficient in assimilating EPA and DHA, but also in retaining them, as evidenced by their turnover rates being some of the lowest recorded. Essential FAs such as EPA and DHA are vital for heterotrophs, as previously mentioned, but almost exclusively produced by phytoplankton, such that consumers must assimilate them from dietary sources. Although Kabeya et al. (2018) revealed that de novo biosynthesis of omega-3 PUFAs by marine consumers is more widespread than previously assumed, very few species (if any) are able to biosynthesize these compounds in large enough quantities to meet their own metabolic needs. When the food available is deficient in EFAs, consumers are likely to retain them (Galloway and Budge, 2020), as exemplified by our low turnover rates of DHA (6-8% day-1) in copepods feeding on a DHA-poor (~ 5% TFA) diatom culture. However, when feeding on a DHA-rich diet, such as dinoflagellates, lipid-poor copepods have been observed to quickly metabolize and replace (i.e., high turnover) DHA (Helenius et al., 2020a).
4.3 Future Studies
Although the importance of lipid-rich copepods as vital links in the transfer of energy between primary producers and higher trophic levels in marine food webs has long been recognized, global warming may lead to a greater importance of lipid-poor species in local food webs, even though their energy reserves are not as significant. Increasing temperatures are expected to affect the composition of the zooplankton, potentially resulting in a greater proportion of smaller copepods in the oceans (Richardson, 2008). The upward energy transfer in marine food webs may be further complicated by predictions that the primary production of omega-3 PUFAs, such as EPA and DHA, will decrease with climate warming (Hixson and Arts, 2016). In order for society to be able to better predict and prepare for such changes in marine food web dynamics, it is of the utmost importance that more studies quantify and qualify the assimilation and turnover of energy-rich dietary molecules (such as FAs) in zooplankton, but especially in lipid-poor copepods and other hot climate-favored species. Although our experiment provided important insights into FA dynamics for one of such species, the fact that copepods were fed with a single diet was a limitation of our study. In nature, zooplankton will often have access to several prey, and of varying nutritional quality. It is, thus, important that future studies consider how mixed diets and different prey quality may affect energy transfer between lower trophic levels. Future studies can go one step further and investigate what will happen when consumers are unable to feed. One approach would be to employ isotope tracer additions, CSIA, and lipid fractionation (into neutral and polar fractions) to differentiate between processes related to membrane and storage lipids. Storage lipids are depleted more readily than membrane lipids in conditions of starvation, and both depletions can impact consumer survival, albeit with different intensities. In addition, such studies would also benefit from designs which included different developmental stages and varying seasonal conditions, as a large gap exists in the literature regarding these topics (Kattner and Hagen, 2009). Such an investment in laboratory tracer studies would also generate a side benefit, which is to test and potentially make the LHH more robust and comprehensive.
5 Conclusion
The application of CSIA to samples obtained during this feeding tracer experiment has enabled us to shed light on the FA dynamics (assimilation, turnover, and assimilation efficiency) of the copepod Temora longicornis, which we showed to be non-homeostatic in terms of lipid regulation upon assimilation of dietary material. To our knowledge, only five other publications have offered quantitative (CSIA) data on this topic for lipid-poor copepods, such that our study partly fills a knowledge gap in understanding the bioenergetic structure of lower trophic levels in marine food webs. We also showed that, when C becomes available, C-limited copepods are able to quickly replenish energy stores until depots near saturation, and to replace almost half of their lipid C content in just five days. This strategy likely enables T. longicornis, which seems to be well adapted to feeding on diatoms and to rapidly synthesizing lipids from this food source, to deal with sudden changes in feeding conditions and to actively grow and reproduce year-round. The biochemical importance of (omega-3) PUFAs, especially EPA and DHA, in zooplankton processes was evidenced in this study by the high relative lipid C assimilation and AE and the low turnover rates of these FAs (in comparison to other FAs) in copepods. We should also note that, while this research refers to a specific copepod species and was conducted in a specific location, the ubiquitousness of the species enables the comparison of the results discussed above with those from several small, lipid-poor copepods and zooplankton in general. We strongly encourage future experiments to employ techniques similar to the ones used herein in order to quantify energy transfer between trophic levels. This information will be necessary for the better prediction of and planning for food regime shifts in marine systems in the context of global warming and related environmental changes.
Data Availability Statement
The datasets presented in this study can be found in online repositories. The names of the repository/repositories and accession number(s) can be found below: PANGAEA, https://doi.pangaea.de/10.1594/PANGAEA.940526, https://doi.pangaea.de/10.1594/PANGAEA.940322; https://doi.pangaea.de/10.1594/PANGAEA.940321; https://doi.pangaea.de/10.1594/PANGAEA.940525; https://doi.pangaea.de/10.1594/PANGAEA.940407; https://doi.org/10.1594/PANGAEA.886050 and https://doi.pangaea.de/10.1594/PANGAEA.886018.
Author Contributions
RF-S, BN, CM, and MB contributed to conception and design of the experiment. RF-S conducted the experiment and data analyses, visualization, and curation for PANGAEA. HA, MT, and BN acquired the necessary funds, whereas MB, MG, CM, and BN provided resources for the project. HA, MT, MG, and BN supervised the PhD work. HA, MB, MT, MG, CM, and BN validated the calculations. RF-S wrote the first draft of the manuscript. All authors contributed to manuscript revision, read, and approved the submitted version.
Funding
RF-S was supported during this PhD work by a scholarship from the MARES Doctoral Programme on Marine Ecosystem Health and Conservation (Framework Agreement Number 2011-0016) and by the Alfred Wegener Institute Helmholtz Centre for Polar and Marine Research. The work was further supported by Ghent University (BOF-GOA project 01G02617). CM was supported by the Bundesministerium für Bildung und Forschung (BMBF grant no. 01LN1702A). Open Access Publication costs were supported by the Alfred-Wegener-Institut Helmholtz-Zentrum für Polar- und Meeresforschung.
Conflict of Interest
The authors declare that the research was conducted in the absence of any commercial or financial relationships that could be construed as a potential conflict of interest.
Publisher’s Note
All claims expressed in this article are solely those of the authors and do not necessarily represent those of their affiliated organizations, or those of the publisher, the editors and the reviewers. Any product that may be evaluated in this article, or claim that may be made by its manufacturer, is not guaranteed or endorsed by the publisher.
Acknowledgments
We kindly thank the crew of the Aade research vessel (AWI-BAH) for providing us with zooplankton samples; and Dr Peter Nichols, Dr Eric J. Raes, and Dr Lauris Boissonnot for insightful discussions about the data. We acknowledge support by the Open Access Publication Funds of Alfred-Wegener-Institut Helmholtz-Zentrum für Polar- und Meeresforschung.
Supplementary Material
The Supplementary Material for this article can be found online at: https://www.frontiersin.org/articles/10.3389/fmars.2022.819943/full#supplementary-material
References
Amorim F., de L. L., Rick J., Lohmann G., Wiltshire K. H. (2021). Evaluation of Machine Learning Predictions of a Highly Resolved Time Series of Chlorophyll-a Concentration. Appl. Sci. 11 (16), 7208. doi: 10.3390/app11167208
Anderson T. R., Hessen D. O., Mayor D. J. (2021). Is the Growth of Marine Copepods Limited by Food Quantity or Quality? Limnol. Oceanog. Lett. 6 (3), 127–133. doi: 10.1002/lol2.10184
Arendt K. E., Jónasdóttir S. H., Hansen P. J., Gärtner S. (2005). Effects of Dietary Fatty Acids On The Reproductive Success Of The Calanoid Copepod Temora longicornis. Mar. Biol. 146, 513–530. doi: 10.1007/s00227-004-1457-9
Arndt C. E., Swadling K. M. (2006). Crustacea in Arctic and Antarctic Sea Ice: Distribution, Diet and Life History Strategies. Adv. Mar. Biol., 51, 197–315. doi: 10.1016/S0065-2881(06)51004-1
Arts M. T., Kohler C. C. (2009). Health and condition in fish: the influence of lipids on membrane competency and immune response. In: Kainz, M., Brett, M., Arts, M. (eds) Lipids in Aquatic Ecosystems. Springer, New York, NY. doi: 10.1007/978-0-387-89366-2_10
Attwood C. G., Peterson W. T. (1989). Reduction In Fecundity And Lipids Of The Copepod Calanus australis (Brodskii) by Strongly Pulsed Upwelling. J. Exp. Mar. Biol. Ecol. 129 (2), 121–131. doi: 10.1016/0022-0981(89)90051-8
Boissonnot L., Niehoff B., Hagen W., Søreide J. E., Graeve M. (2016). Lipid Turnover Reflects Life-Cycle Strategies Of Small-Sized Arctic Copepods. J. Plankton. Res 38(6), 1420–1432. doi: 10.1093/plankt/fbw076
Brett M. T., Müller-Navarra D. C. (1997). The Role Of Highly Unsaturated Fatty Acids In Aquatic Foodweb Processes. Freshwater. Biol. 38 (3), 483–499. doi: 10.1046/j.1365-2427.1997.00220.x
Burian A., Grosse J., Winder M., Boschker H. T. S. (2018). Nutrient Deficiencies And The Restriction Of Compensatory Mechanisms In Copepods. Funct. Ecol. 32 (3), 636–647. doi: 10.1111/1365-2435.13016
Campbell R., Boutillier P., Dower J. (2004). Ecophysiology Of Overwintering In The Copepod Neocalanus plumchrus: Changes in Lipid and Protein Contents Over a Seasonal Cycle. Mar. Ecol. Prog. Ser. 280, 211–226. doi: 10.3354/meps280211
Clarke K. R., Gorley R. N. (2015). Getting Started with PRIMER v7. PRIMER-E: Plymouth, Plymouth Marine Laboratory, 20.
Claus C. (1863). Die Frei Lebenden Copepoden Mit besonderer Berucksichtigung Der Fauna Deutschlands, Der Nordsee Und Des Mittelmeeres. Verlag von Wilhelm Engelmann, Leipzig 1–230, pls. 1–37.
Claus C. (1866). Die Copepoden-Fauna von Nizza. Ein Beitrag zur Charakteristik der Formen und deren Abanderungen 'im Sinne Darwin's'. Schriften der Gesellschaft zur Beforderung der Gesmmten Naturwissenschaften zu Marburg. Supplemment 1: 1-34. Pls. 1-5.
Conover R. J. (1988). Comparative Life Histories In The Genera Calanus and Neocalanus in High Latitudes of the Northern Hemisphere. Hydrobiologia 167, 127–142. doi: 10.1007/BF00026299
Dana J.D. (1849). Conspectus Crustaceorum, Quae in Orbis Terrarum Circumnavigatione, Carolo Wilkes, E Classe Reipublicae Foederatae Duce, Lexit Et Descripsit. Am. J. Sci. 8 (2), 276–285.
De Troch M., Boeckx P., Cnudde C., van Gansbeke D., Vanreusel A., Vincx M., et al. (2012). Bioconversion of Fatty Acids at the Basis of Marine Food Webs: Insights From a Compound-Specific Stable Isotope Analysis. Mar. Ecol. Prog. Ser. 465, 53–67. doi: 10.3354/meps09920
Evans F. (1981). An Investigation Into the Relationship of Sea Temperature and Food Supply to the Size of the Planktonic Copepod Temora longicornis Müller in the North Sea. Estua. Coast. Shelf. Sci. 13 (2), 145–158. doi: 10.1016/S0302-3524(81)80072-2
Evershed R. P., Bull I. D., Corr L. T., Crossman Z. M., Van Dongen B. E., Evans C, et al. (2007). Compound-specific stable isotope analysis in ecological research. In R. Michener and K. Lajtha, eds. Stable isotopes in ecology and environmental science. 2nd edition. Blackwell Publishing, Boston, MA. Pages 480–540.
Evjemo J. O., Olsen Y. (1997). Lipid and Fatty Acid Content in Cultivated Live Feed Organisms Compared to Marine Copepods. Hydrobiologia 358, 159–162. doi: 10.1023/A:1003196829109
Evjemo J. O., Reitan K. I., Olsen Y. (2003). Copepods as Live Food Organisms in the Larval Rearing of Halibut Larvae (Hippoglossus hippoglossus L.) With Special Emphasis on the Nutritional Value. Aquaculture 227 (1–4), 191–210. doi: 10.1016/S0044-8486(03)00503-9
Folch J., Lees M., Stanley G. (1957). A Simple Method for the Isolation and Purification of Total Lipides From Animal Tissues. J. Biol. Chem. 226, 497–509. doi: 10.1016/S0021-9258(18)64849-5
Franco-Santos R. M., Auel H., Boersma M., De Troch M., Graeve M., Meunier C. L., et al. (2019). You Are Not Always What You Eat—Fatty Acid Bioconversion and Lipid Homeostasis in the Larvae of the Sand Mason Worm Lanice conchilega. PloS One 14 (6), e0218015. doi: 10.1371/journal.pone.0218015
Franco-Santos R. M., Auel H., Boersma M., De Troch M., Graeve M., Meunier C. L., et al. (2022). Elemental, Biochemical, and Fatty Acid Contents for the Copepod Temora Longicornis Fed Under Laboratory Conditions With Different Nutrient Regimes. PANGAEA. doi: 10.1594/PANGAEA.940526
Franco-Santos R. M., Auel H., Boersma M., De Troch M., Meunier C. L., Niehoff B. (2018a). Vital Rate Measurements and Carbon and Nitrogen Contents of Temora Longicornis Under Different Nutrient Regimes. PANGAEA. doi: 10.1594/PANGAEA.886050
Franco-Santos R. M., Auel H., Boersma M., De Troch M., Meunier C. L., Niehoff B. (2018b). Bioenergetics of the Copepod Temora Longicornis Under Different Nutrient Regimes. J. Plankton. Res. 40 (4), 420–435. doi: 10.1093/plankt/fby016
Fraser A. J., Sargent J. R., Gamble J. C. (1989). Lipid Class and Fatty Acid Composition of Calanus Finmarchicus (Gunnerus), Pseudocalanus Sp. and Temora Longicornis Muller From a Nutrient-Enriched Seawater Enclosure. J. Exp. Mar. Biol. Ecol. 130 (1), 81–92. doi: 10.1016/0022-0981(89)90020-8
Fryxell G. A., Hasle G. R. (1977). The Genus Thalassiosira: Species With a Modified Ring of Central Strutted Processes. In: Simonsen R.. Proceedings of the Fourth Symposium on Recent and Fossil Marine Diatoms, Oslo, August 30 - September 3, 1976. Beihefte zur Nova Hedwigia 54, 67–98.
Galloway Aaron W. E., Budge Suzanne M. (2020). The Critical Importance of Experimentation in Biomarker-Based Trophic Ecology. Phil. Trans. R. Soc. B 375, 20190638. doi: 10.1098/rstb.2019.0638
Gentsch E., Kreibich T., Hagen W., Niehoff B. (2009). Dietary Shifts in the Copepod Temora Longicornis During Spring: Evidence From Stable Isotope Signatures, Fatty Acid Biomarkers and Feeding Experiments. J. Plankton. Res. 31 (1), 45–60. doi: 10.1093/plankt/fbn097
Graeve M., Albers C., Kattner G. (2005). Assimilation and Biosynthesis of Lipids in Arctic Calanus Species Based on Feeding Experiments With a 13C Labelled Diatom. J. Exp. Mar. Biol. Ecol. 317 (1), 109–125. doi: 10.1016/j.jembe.2004.11.016
Graeve M., Boissonnot L., Niehoff B., Hagen W., Kattner G. (2020). Assimilation and Turnover Rates of Lipid Compounds in Dominant Antarctic Copepods Fed With 13C-Enriched Diatoms. Phil. Trans. R. Soc. B 375, 20190647 doi: 10.1098/rstb.2019.0647
Grice K., Klein Breteler W. C. M., Schouten S., Grossi V., de Leeuw J. W., Damsté J. S. S. (1998). Effects of Zooplankton Herbivory on Biomarker Proxy Records. Paleoceanography and Paleoclimatology 13 (6), 686–693. doi: 10.1029/98PA01871
Guilini K., van Oevelen D., Soetaert K., Middelburg J. J., Vanreusel A. (2010). Nutritional Importance of Benthic Bacteria for Deep-Sea Nematodes From the Arctic Ice Margin: Results of an Isotope Tracer Experiment. Limnol. Oceanog. 55 (5), 1977–1989. doi: 10.4319/lo.2010.55.5.1977
Gunnerus J. E. (1770). Nogle Smaa Rare Og Meestendeelen Nye Norske Seedry. Skrifter som udi det Kiobenhavnske Selskab. 10, 166–176.
Hagen W., Auel H. (2001). Seasonal Adaptations and the Role of Lipids in Oceanic Zooplankton. Zoology 104 (3-4), 313–326. doi: 10.1078/0944-2006-00037
Harvey H. R., Eglinton G., O’Hara S. C. M., Corner E. D. S. (1987). Biotransformation and Assimilation of Dietary Lipids by Calanus Feeding on a Dinoflagellate. Geochim. Cosmochimica. Acta 51 (11), 3031–3040. doi: 10.1016/0016-7037(87)90376-0
Helenius L., Budge S., Duerksen S., Devred E., Johnson C. L. (2019). Lipids at the Plant-Animal Interface: A Stable Isotope Labelling Method to Evaluate the Assimilation of Essential Fatty Acids in the Marine Copepod Calanus finmarchicus. J. Plankton. Res. 41 (6), 909–924. doi: 10.1093/plankt/fbz062
Helenius L., Budge S. M., Johnson C. L. (2020a). Stable Isotope Labeling Reveals Patterns in Essential Fatty Acid Growth Efficiency in a Lipid-Poor Coastal Calanoid Copepod. Mar. Biol. 167, 178. doi: 10.1007/s00227-020-03794-8
Helenius L., Budge S. M., Nadeau H., Johnson C. L. (2020b). Ambient Temperature and Algal Prey Type Affect Essential Fatty Acid Incorporation and Trophic Upgrading in a Herbivorous Marine Copepod. Phil. Trans. R. Soc. B 375, 20200039. doi: 10.1098/rstb.2020.0039
Hill D. R., Wetherbee R. (1989). A Reappraisal of the Genus Rhodomonas (Cryptophyceae). Phycologia 28 (2), 143–158
Hixson S. M., Arts M. T. (2016). Climate Warming Is Predicted to Reduce Omega-3, Long-Chain, Polyunsaturated Fatty Acid Production in Phytoplankton. Global Change Biol. 22 (8), 2744–2755. doi: 10.1111/gcb.13295
Holste L., John M. A. S., Peck M. A. (2009). The Effects of Temperature and Salinity on Reproductive Success of Temora Longicornis in the Baltic Sea: A Copepod Coping With a Tough Situation. Mar. Biol. 156, 527–540. doi: 10.1007/s00227-008-1101-1
Jaschnov W. A. (1955). Morphology, Distribution and Systematics of Calanus Finmarchicus Sl [In Russian]. Zool. Zh. 34, 1210–1223.
Johnson W. S., Allen D. M. (2012). Zooplankton of the Atlantic and Gulf Coasts: A Guide to Their Identification and Ecology (2nd Edition) (Baltimore, Maryland, USA: JHU Press).
Jónasdóttir S. H., Fields D., Pantoja S. (1995). Copepod Egg Production in Long Island Sound, USA, as a Function of the Chemical Composition of Seston. Mar. Ecol. Prog. Ser. 119, 87–98. doi: 10.3354/meps119087
Jónasdóttir S. H., Visser A., Jespersen C. (2009). Assessing the Role of Food Quality in the Production and Hatching of Temora Longicornis Eggs. Mar. Ecol. Prog. Ser. 382, 139–150. doi: 10.3354/meps07985
Kabeya N., Fonseca M. M., Ferrier D. E. K., Navarro J. C., Bay L. K., Francis D. S., et al. (2018). Genes for de Novo Biosynthesis of Omega-3 Polyunsaturated Fatty Acids Are Widespread in Animals. Sci. Adv. 4, eaar6849. doi: 10.1126/sciadv.aar6849
Kattner G., Hagen W. (1995). Polar Herbivorous Copepods–different Pathways in Lipid Biosynthesis. ICES. J. Mar. Sci. 52 (3–4), 329–335. doi: 10.1016/1054-3139(95)80048-4
Karsten G. (1898). Rhodomonas Baltica N. G. Et Sp. Wiss. Meeresunters. Abteilung Kiel, N. F. 3, 15–17.
Kattner G., Hagen W. (2009). Lipids in marine copepods: latitudinal characteristics and perspective to global warming. In: Kainz, M., Brett, M., Arts, M. (eds) Lipids in Aquatic Ecosystems. Springer, New York, NY. doi: 10.1007/978-0-387-89366-2_11
Klein Breteler W. C. M., Grice K., Schouten S., Kloosterhuis H. T., Sinninghe Damsté J. S. (2002). Stable Carbon Isotope Fractionation in the Marine Copepod Temora longicornis: Unexpectedly low δ13C value of faecal pellets. Mar. Ecol. Prog. Ser. 240, 195–204. doi: 10.3354/meps240195
Kreibich T., Saborowski R., Hagen W., Niehoff B. (2008). Short-Term Variation of Nutritive and Metabolic Parameters In Temora Longicornis Females (Crustacea, Copepoda) as a Response to Diet Shift and Starvation. Helgoland. Mar. Res. 62, 241–249. doi: 10.1007/s10152-008-0112-0
Kreibich T., Saborowski R., Hagen W., Niehoff B. (2011). Influence of Short-Term Nutritional Variations on Digestive Enzyme and Fatty Acid Patterns of the Calanoid Copepod Temora Longicornis. J. Exp. Mar. Biol. Ecol. 407 (2), 182–189. doi: 10.1016/j.jembe.2011.06.013
Lee R. F., Hagen W., Kattner G. (2006). Lipid Storage in Marine Zooplankton. Mar. Ecol. Prog. Ser. 307, 273–306. doi: 10.3354/Meps307273
Leonard A. E., Pereira S. L., Sprecher H., Huang Y.-S. (2004). Elongation of Long-Chain Fatty Acids. Prog. Lipid Res. 43 (1), 36–54. doi: 10.1016/S0163-7827(03)00040-7
Löder M. G. J., Meunier C., Wiltshire K. H., Boersma M., Aberle N. (2011). The Role of Ciliates, Heterotrophic Dinoflagellates and Copepods in Structuring Spring Plankton Communities at Helgoland Roads, North Sea. Mar. Biol. 158, 1551–1580. doi: 10.1007/s00227-011-1670-2
Maar M., Nielsen T. G., Gooding S., Tönnesson K., Tiselius P., Zervoudaki S., et al. (2004). Trophodynamic Function of Copepods, Appendicularians and Protozooplankton in the Late Summer Zooplankton Community in the Skagerrak. Mar. Biol. 144, 917–933. doi: 10.1007/s00227-003-1263-9
Martynova D. M., Kazus N. A., Bathmann U., Graeve M., Sukhotin A. A. (2011). Seasonal Abundance and Feeding Patterns of Copepods Temora Longicornis, Centropages Hamatus and Acartia Spp. in the White Sea (66 N). Polar. Biol. 34, 1175–1195. doi: 10.1007/s00300-011-0980-7
McMahon K. W., Newsome S. D. (2019). Amino acid isotope analysis: a new frontier in studies of animal migration and foraging ecology. In Hobson, K., and Wassenaar, L. (Eds.), Tracking Animal Migration with Stable Isotopes. Academic Press, London, pp. 173–190.
Michener R. H., Kaufman L. (2007). Stable Isotope Ratios as Tracers in Marine Food Webs: An Update. In R. Michener and K. Lajtha, eds. Stable isotopes in ecology and environmental science. 2nd edition. Blackwell Publishing, Boston, MA. Pages 238–282.
Middelburg J. J. (2014). Stable Isotopes Dissect Aquatic Food Webs From the Top to the Bottom. Biogeosciences 11 (8), 2357–2371. doi: 10.5194/bg-11-2357-2014
Müller O. F. (1785). Entomostraca Seu Insecta Testacea, Quae in Aquis Daniae Et Norvegiae Reperit, Descripsit Et Iconibus Illustravit. F.W. Thiele, Lipsiae & Havniae 1-134, index, pls. 1-21. page(s): 115–116.
Müller-Navarra D. (1995). Evidence That a Highly Unsaturated Fatty Acid Limits Daphnia growth in nature. Archiv. Fur. Hydrobio. 132, 297–307. doi: 10.1127/archiv-hydrobiol/132/1995/297
Müller-Navarra D. C., Brett M. T., Liston A. M., Goldman C. R. (2000). A Highly Unsaturated Fatty Acid Predicts Carbon Transfer Between Primary Producers and Consumers. Nature 403, 74–77. doi: 10.1038/47469
Newton J. (2010). "Stable Isotope Ecology", in Encyclopedia of Life Sciences (ELS) (Chichester:John Wiley & Sons, Ltd). doi: 10.1002/9780470015902.a0021231
Niehoff B. (2007). Life History Strategies in Zooplankton Communities: The Significance of Female Gonad Morphology and Maturation Types for the Reproductive Biology of Marine Calanoid Copepods. Prog. Oceanog. 74 (1), 1–47. doi: 10.1016/j.pocean.2006.05.005
Niehoff B., Kreibich T., Saborowski R., Hagen W. (2015). Feeding History Can Influence Physiological Responses of Copepods: An Experimental Study Comparing Different Cohorts of Temora Longicornis From the Southern North Sea. J. Exp. Mar. Biol. Ecol. 469, 143–149. doi: 10.1016/j.jembe.2015.04.008
Ohman M. D., Bradford J. M., Jillett J. B. (1989). Seasonal Growth and Lipid Storage of the Circumglobal, Subantarctic Copepod, Neocalanus Tonsus (Brady). Deep Sea Research Part A. Oceanog. Res. Papers. 36 (9), 1309–1326. doi: 10.1016/0198-0149(89)90085-X
Pallas P. S. (1766). Miscellanea Zoologica. Quibus Novae Imprimis Atque Obscurae Animalium Species Describuntur Et Observationibus Iconibusque Illustrantur. Petrum van Cleef. Hagí Comitum xii + (224), pp. 14 pls.
Paul D., Skrzypek G., Fórizs I. (2007). Normalization of Measured Stable Isotopic Compositions to Isotope Reference Scales–a Review. Rapid Commun. Mass. Spectro. 21 (18), 3006–3014. doi: 10.1002/rcm.3185
Peters J., Dutz J., Hagen W. (2013). Trophodynamics and Life-Cycle Strategies of the Copepods Temora Longicornis and Acartia Longiremis in the Central Baltic Sea. J. Plankton. Res. 35 (3), 595–609. doi: 10.1093/plankt/fbt004
Pond D. W. (2012). The Physical Properties of Lipids and Their Role in Controlling the Distribution of Zooplankton in the Oceans. J. Plankton. Res. 34 (6), 443–453. doi: 10.1093/plankt/fbs027
Prahl F. G., Eglinton G., Corner E. D. S., O'Hara S. C.M., Forsberg T. E. V. (1984). Changes in Plant Lipids During Passage Through the Gut Of Calanus J. Mar. Biol. Assoc. U. K. 64 (2), 317–334. doi: 10.1017/S0025315400030022
Richardson A. J. (2008). In hot water: zooplankton and climate change. ICES. J. Mar. Sci. 65 (3), 279–295. doi: 10.1093/icesjms/fsn028
Rix L., de Goeij J. M., van Oevelen D., Struck U., Al-Horani F. A., Wild C., et al. (2018). Reef Sponges Facilitate the Transfer of Coral-Derived Organic Matter to Their Associated Fauna via the sponge loop. Mar. Ecol. Prog. Ser. 589, 85–96. doi: 10.3354/meps12443
Sargent J. R., Falk-Petersen S. (1988). The lipid biochemistry of calanoid copepods. In: Boxshall, G.A., Schminke, H.K. (eds) Biology of Copepods. Developments in Hydrobiology, vol 47. Springer, Dordrecht. doi: 10.1007/978-94-009-3103-9_9
Sargent J. R., Gatten R. R., Henderson R. J. (1981). Marine wax esters. Pure. Appl. Chem. 53 (4), 867–871. doi: 10.1351/pac198153040867
Scott C. L., Kwasniewski S., Falk-Petersen S., Sargent J. R. (2002). Species Differences, Origins and Functions of Fatty Alcohols and Fatty Acids in the Wax Esters and Phospholipids of Calanus Hyperboreus, C. Glacialis and C. Finmarchicus From Arctic Waters. Mar. Ecol. Prog. Ser. 235, 127–134. doi: 10.3354/meps235127
Stachura-Suchoples K., Williams D. M. (1949). Description of Conticribra Tricircularis, New Genus and Species of Thalassiosirales, With a Discussion on Its Relationship to Other Continuous Cribra Species of Thalassiosira Cleve (Bacillariophyta) and Its Freshwater Origin. Eur. J. Phycol. 44 (4), 477–486.
Sterner R. W., Schulz K. L. (1998). Zooplankton Nutrition: Recent Progress and a Reality Check. Aquat. Ecol. 32, 261–279. doi: 10.1023/A:1009949400573
Thompson I. C., Scott A. (1897). "Notes on New and Other Copepoda," in: On the Plankton Collected Continuously During Two Traverses of the North Atlantic in the Summer of 1897; With Descriptions of New Species of Copepoda; and an Appendix on Dredging in Puget Sound. Eds. Herdman W. A., Thompson I. C., Scott A. (Proceedings and Transactions of the Liverpool Biological Society). 12, 33–90
Twining C. W., Brenna J. T., Hairston N. G., Flecker A. S. (2016). Highly Unsaturated Fatty Acids in Nature: What We Know and What We Need to Learn. Oikos 125 (6), 749–760. doi: 10.1111/oik.02910
Twining C. W., Taipale S. J., Ruess L., Bec A., Martin-Creuzburg D., Kainz M. J. (2020). Stable Isotopes of Fatty Acids: Current and Future Perspectives for Advancing Trophic Ecology. Philos. Trans. R. Soc. B. 375, 20190641. doi: 10.1098/rstb.2019.0641
Veloza A. J., Chu F. L. E., Tang K. W. (2006). Trophic Modification of Essential Fatty Acids by Heterotrophic Protists and Its Effects on the Fatty Acid Composition of the Copepod Acartia tonsa. Mar. Biol. 148, 779–788. doi: 10.1007/s00227-005-0123-1
Vogedes D., Varpe O., Søreide J. E., Graeve M., Berge J., Falk-Petersen S. (2010). Lipid Sac Area as a Proxy for Individual Lipid Content of Arctic Calanoid Copepods. J. Plankton. Res. 32 (10), 1471–1477. doi: 10.1093/plankt/fbq068
Warton D. I., Hui F. K. C. (2011). The Arcsine Is Asinine: The Analysis of Proportions in Ecology. Ecology 92 (1), 3–10. doi: 10.1890/10-0340.1
Werbrouck E., Bodé S., van Gansbeke D., Vanreusel A., De Troch M. (2017). Fatty Acid Recovery After Starvation: Insights Into the Fatty Acid Conversion Capabilities of a Benthic Copepod (Copepoda, Harpacticoida). Mar. Biol. 164, 151. doi: 10.1007/s00227-017-3181-2
Wesche A., Wiltshire K. H., Hirche H. J. (2007). Overwintering Strategies of Dominant Calanoid Copepods in the German Bight, Southern North Sea. Mar. Biol. 151, 1309–1320. doi: 10.1007/s00227-006-0560-5
Whiteman J., Elliott Smith E., Besser A., Newsome S. (2019). A Guide to Using Compound-Specific Stable Isotope Analysis to Study the Fates of Molecules in Organisms and Ecosystems. Diversity 11 (1), 8. doi: 10.3390/d11010008
Keywords: compound-specific stable isotope analysis (CSIA), fatty acid trophic markers, homeostasis, lipid dynamics, metabolic requirements, omega-3 fatty acids, tracer experiment, 13C labelling
Citation: Franco-Santos RM, Auel H, Boersma M, De Troch M, Graeve M, Meunier CL and Niehoff B (2022) To Regulate or Not to Regulate: Assimilation of Dietary Fatty Acids in the Temperate Copepod Temora longicornis. Front. Mar. Sci. 9:819943. doi: 10.3389/fmars.2022.819943
Received: 23 November 2021; Accepted: 24 March 2022;
Published: 03 May 2022.
Edited by:
Travis Blake Meador, Academy of Sciences of the Czech Republic (ASCR), CzechiaReviewed by:
William Ross Hunter, Queen’s University Belfast, United KingdomCatherine J. Stevens, University of Victoria, Canada
Copyright © 2022 Franco-Santos, Auel, Boersma, De Troch, Graeve, Meunier and Niehoff. This is an open-access article distributed under the terms of the Creative Commons Attribution License (CC BY). The use, distribution or reproduction in other forums is permitted, provided the original author(s) and the copyright owner(s) are credited and that the original publication in this journal is cited, in accordance with accepted academic practice. No use, distribution or reproduction is permitted which does not comply with these terms.
*Correspondence: Rita M. Franco-Santos, rita.franco-santos@outlook.com; Barbara Niehoff, barbara.niehoff@awi.de
†Present address: Rita M. Franco-Santos, Department of Process Engineering and Applied Science, Dalhousie University, Halifax, NS, Canada