Changes in the Bacterial Community Associated With Experimental Symbiont Loss in the Mucus Layer of Cassiopea xamachana Jellyfish
- 1Posgrado en Ciencias del Mar y Limnología, Posgrado UNAM, Universidad Nacional Autónoma de México, Ciudad de México, Mexico
- 2Unidad Académica de Sistemas Arrecifales, Instituto de Ciencias del Mar y Limnología-UNAM, Puerto Morelos, Mexico
- 3Red de Manejo Biorracional de Plagas y Vectores, Instituto de Ecología, AC–INECOL, Clúster Científico y Tecnológico BioMimic®, Xalapa, Mexico
- 4Departamento de Recursos del Mar, Centro de Investigación y de Estudios Avanzados del Instituto Politécnico Nacional (CINVESTAV) Unidad Mérida, Mérida, Mexico
Cassiopea xamachana is a model system for studies in animal symbiosis with algal symbionts. This medusa is also associated with a microbial community that can impact its health, but this community has not been thoroughly studied. Shifts in the bacterial community following the loss of symbionts involving stress, environmental changes, or seasonal fluctuations can be complex, as the role of symbionts in structuring this community is not well established. To understand the interplay among microbial associates with this host, we explored the experimental diminishing of algal symbionts, and the influence of seasonal fluctuations over the structure of the bacterial community, through 16S rRNA gene high-throughput sequencing. Results showed that Gammaproteobacteria, Bacteroidia, and Alphaproteobacteria were dominant in all the mucus samples at the beginning of the experiments. However, after 28 days, bleached medusas showed a marked increase in Gammaproteobacteria, specifically in the genus Vibrio, as evidenced by Linear Discriminant Analysis of Effect Size (LEfSe). Seasons also resulted in shifts of the bacterial community, although bacterial genera were distinct from those found in bleached medusas, suggesting temporal associations with the host. According to PERMANOVA analysis, seasonal fluctuations affected the dominant bacterial members (p = 0.07), but symbiont presence was a more significant driver (p=0.001). We found the bacterial community of C. xamachana is like that of other jellyfish and corals, which furthers the interest in this animal as a study model. Defining relevant bacterial genera can help us understand the functional role of the holobiont members that assemble and maintain a healthy microbial community. Also, studies in other regions where C. xamachana distributes can help us define a core bacterial community for this medusa.
Introduction
Cnidarian-microbe interactions are ecologically important (Pitt et al., 2005; Tinta et al., 2012; Glasl et al., 2016; Röthig et al., 2017; Webster and Reusch, 2017), facilitating energy acquisition and potentially contributing to the nutrition (Lesser et al., 2004; Rädecker et al., 2015; Röthig et al., 2021), health (Bourne et al., 2016; Hernandez-Agreda et al., 2017), immunity (Bosch, 2013), and defense of the hosts (Ritchie, 2006; Krediet et al., 2012; Glasl et al., 2016). A surface mucus layer (SML) covers the Cnidarian body and protects their live tissues from physical, chemical, and biological impacts (Brown and Bythell, 2005). Due to its chemical composition, the SML sustains a diverse microbial community of Bacteria, Archaea, Microalgae, and Fungi, many of which are host specific (Rohwer et al., 2002; Lampert et al., 2008; Carlos et al., 2013; Cleary et al., 2016). Considering the sparse distribution of nutrients in oligotrophic waters, where many Cnidarians develop, the SML represents a rich substrate that stimulates bacterial growth (Hansson and Norrman, 1995; Wild et al., 2004; Bythell and Wild, 2011). The composition of Cnidaria-associated bacterial communities is dynamic, displaying seasonal and geographic variations (Morrow et al., 2012; Sharp et al., 2017), particularly in short-term studies (Yang et al., 2017). However, the mechanisms that structure and regulate this community remain poorly understood.
As in corals, some Scyphozoan jellyfishes contain symbiotic dinoflagellate algae, like species in the genera Cassiopea, Mastigias, Linuchae, and Phyllorhiza (reviewed in Djeghri et al., 2019). The medusae complete their energetic demands with photosynthetic products supplied by the symbiotic algae, which also contribute to the production of the SML (Hofmann and Kremer, 1981; McCloskey et al., 1994; Freeman et al., 2016). Jellyfish may also lose their symbionts and bleach after heat and light stress (McGill and Pomoroy, 2008; Newkirk et al., 2018). The disruption of the symbiosis can affect the SML secretion and modify its biochemical properties (Lee et al., 2016; Rivera-Ortega and Thomé, 2018), which may be followed by changes in the associated bacteria, as occurs with corals (Bourne et al., 2007; Glasl et al., 2016; Wright et al., 2019). Symbionts can contribute significantly to mucus production in symbiotic corals (Brown and Bythell, 2005). A fraction of the carbon translocated to hosts gets lost from the association as dissolved and particulate organic carbon (mucus); this loss represents, for example, between 5% and 50% in 48 h incubations in the coral Stylophora pistillata (Tremblay et al., 2012), or 20-45% of daily net photosynthate in corals (as reviewed by Brown and Byhtell, 2005). But unlike corals, soft-body Cnidarians can directly use the energy from the translocation of organic carbon from the algal symbionts for biomass increase and mucus production. Environmental variations that affect the photosynthesis of symbionts can thus affect mucus production. Therefore, soft-body symbiotic Cnidarians like the jellyfish C. xamachana and the sea anemone Exaiptasia diaphana, represent an opportunity to directly study the interaction of algal symbionts with the animal host, and with the commensal/opportunistic bacteria that live in the mucus layer. But unlike E. diaphana, C. xamachana maintains a closer symbiotic relationship (McGill and Pomoroy, 2008; Voolstra, 2013; Freeman et al., 2016).
The Bacteria-Cnidaria-Symbiodiniaceae must be studied as consortia since the exchange and recycling of fundamental nutrients influence its health and stability (Bourne et al., 2016; Webster and Reusch, 2017; Goulet et al., 2020). However, such exchange can be affected by bleaching, disease, and colonization by opportunistic pathogens (Matthews et al., 2020). Shifts in the bacterial community following the loss of symbionts involving stress, environmental changes, or seasonal fluctuations can be complex, considering that the Bacteria-Symbiodiniaceae association is just beginning to be studied. Some studies have postulated the existence of bacteria closely associated with the surface layer of the symbiont that can help it tolerate physiological stress (as ROS) or variations in environmental conditions (Matthews et al., 2020; Garrido et al., 2021; Maire et al., 2021). A model system such as the jellyfish C. xamachana may prove helpful to define the role of algal symbionts as drivers of the bacterial community in symbiotic medusae.
Cassiopea medusae are Scyphozoans (Order Rhizostomeae) common in shallow waters of tropical and subtropical ecosystems like mangroves, seagrasses, and coral reefs (Niggl et al., 2010; Ohdera et al., 2018). The planktonic medusae shift from a benthic polyp stage (Colley and Trench, 1983), which may bring changes in the associated microbial community. Also, the medusa stage moves with marine currents, potentially affecting its distribution and microbiome. Cassiopea jellyfish increase their abundance and size in areas where human population density is high (Stoner et al., 2011). Nutrient loading can lead to blooms of epibenthic jellyfish which can have consequences to the ecology of the impacted area, competing with other filter-feeding consumers, and locking the biomass that can alter community structure and ecosystem function. Further, metamorphosis of the jellyfish larvae occurs only after the symbiont has been collected by the polyp and temperatures rise above 20°C (Fitt and Costley, 1998). With global change advancing, temperatures that used to limit the reproduction of the jellyfish to the summer months might be surpassed during colder months and exacerbate the density of these jellyfish (Aljbour et al., 2019). A limited number of studies have focused on the bacterial community of symbiotic Rhizostomeae jellyfishes. For example, the bacterial community of C. xamachana held in captivity was studied, showing the dominance of two families, Moxarellaceae and Pseduomonadaceae (Röthig et al., 2021). In the abundant Pacific jellyfish Mastigias papua, the associated bacterial community displayed high variation within the species, low diversity, and the highest bacterial abundance related to members of the Endozoicomonadaceae family (Cleary et al., 2016).
Among non-symbiotic Rhizostomeae jellyfishes, the moon jellyfish Aurelia aurita is one of the best-studied. Its bacterial community is similar throughout its pelagic developmental stages, only the polyp stage being different (Weiland-Bräuer et al., 2015). Also, reports indicate differences associated with geographic location (Kramar et al., 2019). After a jellyfish bloom, in the senescence phase, the bacterial community of A. aurita was characterized by an increase in Gammaproteobacteria, specifically Vibrionaceae and Alteromonadaceae (Kramar et al., 2019). Among other species studied, Cyanea lamarckii displayed significant differences in the bacterial community of the umbrella in contrast to other body parts (Hao et al., 2019). The authors also assessed the bacterial community in different life stages that in general, increased in diversity from larval to adult stages. A recent study of four jellyfish species that form large blooms indicated species-specific differences in their bacterial communities (Peng et al., 2021); Vibrio was the bacterial genus dominant in Aurelia coerulea, as in other Aurelia species (Weiland-Bräuer et al., 2015). Mycoplasma dominated the Rhopilema esculentum and Nemopilema nomurai jellyfishes, but in Cyanea nozakii its bacterial community was equally abundant in Sphingomonas, Phyllobacterium, and Ralstonia (Peng et al., 2021).
Cassiopea xamachana is a benthic jellyfish that lives in ecologically obligate symbiosis with the photosynthetic dinoflagellate Symbiodinium microadriaticum (Colley and Trench, 1983; Lampert, 2016). The adult medusa rests on the sea bottom, their convex umbrella and oral arms facing upwards to allow the capture of light by their symbionts (Lampert, 2016; Ohdera et al., 2018). The colonization by algal symbionts supports the transition from polyp to adult medusa (Hofmann et al., 1996; Newkirk et al., 2018). The relative simplicity of culturing polyps and adults of C. xamachana in laboratory conditions, makes this symbiotic jellyfish an ideal model system to study the mechanisms that regulate the colonization of the host by their symbionts. However, it is not clear how much C. xamachana depends on its algal symbionts, nor if a decrease in algal symbionts can restructure its associated bacterial community. In this study, we characterized the bacterial community of the SML of this symbiotic jellyfish while in captivity, contrasting summer and winter seasons, and the effect of a significant loss of symbionts (bleaching).
Materials and Methods
Organisms and Experimental Conditions
Adult medusas of C. xamachana previously collected from Nichupté lagoon in NE Quintana Roo, Mexico were used for this study. The organisms were maintained in captivity in an open system pond (100 L seawater), under natural light and temperature conditions for more than a year prior to the experiments. For the experimental procedures, medusas were taken from the farming pond and maintained individually in one Liter beakers with 500 mL of seawater from the farming pond, with bubbling air and daily seawater replacements for the duration of the experiments (28 days). All medusas in beakers were maintained under a roof with indirect ambient light. Medusas were sampled in two seasons, in summer (late August 2019) and winter (early March 2020) with mean temperatures of ~ 29.02°C and ~ 26.05°C, respectively (SAMMO, 2018-2019). Symbiotic medusas (n = 6) were maintained as controls and compared to bleached medusas before bleaching (n = 3) and after bleaching (n = 3). Samples were taken at day zero and after 28 days each season (total number of samples = 24). The organisms were not fed during the experimental procedures.
Artificial Bleaching of Medusas
Medusas with a similar umbrella size (6 cm in diameter) were selected. A procedure was chosen to avoid high-temperature bleaching, which can significantly modify the bacterial community, including an increase in heterotrophy and virulence genes (Littman et al., 2011). The experimental bleaching of medusas was achieved by adding a mixture of monosaccharides, following Pogoreutz et al. (2017). The mixture of sugars contained: (D+) glucose (4.14 mg L-1), (D+) galactose (3.59 mg L-1), and mannitol (2.25 mg L-1), dissolved in 200 mL of distilled water. Seawater (500 mL) containing 3 mL of this mixture was used daily to replace the seawater of the beaker containing each medusa for 28 consecutive days, with a final mixed sugar concentration of 0.3 mg L-1. Every day, measurements of the umbrella diameter in all medusas were taken to evaluate the loss of biomass during the experiments.
The bleaching success was evaluated by weekly sampling a tentacle fragment from each medusa, using sterilized scissors. Each tentacle fragment was weighed and ground with a Dounce homogenizer. The homogenate was centrifuged at 13,000 RPM for 5 min and the supernatant removed. The pellet was transferred to a microcentrifuge tube, washed twice with milli-Q water, and resuspended in 500 µL of sterile seawater, adding Lugol (30%) to aid in symbiont counting. Symbiont density was quantified with a hemocytometer in an optic microscope (3 replicate counts per sample) and the data normalized to the wet weight (g) of the tentacle. Further, we photographed a portion of a tentacle of each medusa with a fluorescence microscope (Axioskop 40 with aim 20X Tex Red Fs 15) at the beginning and at the end of the 28-day treatment.
SML and Seawater Sampling for DNA Extraction
We collected samples from the SML of symbiotic medusas maintained as controls and bleached medusas, at the start and end of 28 days. All sampling was repeated for summer and winter. For the collection of the SML, the medusas were brought to the lab and individually placed in a sterile beaker with 200 mL of sterilized seawater. After twenty minutes, the released mucus (500 µl per medusa) was collected with a transfer pipette and was processed immediately for total DNA extraction.
We also sampled seawater from the farming pond, at the beginning (n = 6, each season) and at the end of the experimental time (n = 6, each season). Seawater samples were pre-filtered through 2.5 µm (Whatman ™) to remove large debris, followed by filtering with Durapore® membranes (0.45 µm and 0.22 µm). These last two filters were conserved for immediate DNA extraction. DNA extraction from the SML and seawater samples was carried out with DNeasy® Power Biofilm Kit following the manufacturer’s instructions. DNA integrity was evaluated by electrophoresis in 1% agarose gels. DNA concentration and quality were assessed with a BioSpectrometer (Eppendorf).
16S rRNA Libraries Preparation and Sequencing
PCR amplification of the hypervariable V3 and V4 regions of 16S rRNA gene was performed using the primers 515F = 5’-TCGTCGGCAGCGTCAGATGTGTATAAGAGACAGCCTACGGGNGGCWGCAG - 3’ and 806R = 5’-GTCTCGTGGGCTCGGAGATGTGTATAAGAGACAGGACTACHVGGGTATCTAATC- 3’ and the conditions suggested by Klindworth et al. (2013). The PCR mixture of each 20 µl reaction contained 2 µl DNA, 200 U DreamTaq (ThermoFisher Scientific), 2.5 µl 10X buffer, 1µl 25 mM MgCl2, 0.4 µl deoxynucleotide triphosphate mix (dNTPs, 10 mM), and 0.2 µM for each primer. The amplification reaction was performed in an Applied Biosystems thermal cycler (Veriti 96) with an initial denaturalization of 3 min at 95°C, followed by 25 cycles of 95°C (30 s), 55°C (45 s), and 72°C (30 s), and a final extension of 5 min at 72°C. PCR products were verified in a 1% agarose gel-TAE (Tris-acetate-EDTA) and 1 µl of the PCR reaction was evaluated with a Bioanalyzer DNA 1000 chip. A first purification of the PCR amplicons was performed with AMpure XP Illumina adapters, and a second PCR was used for barcoding with the Nextera XT Index kit, having an initial denaturation of 3 min at 95°C, followed by 8 cycles of 95°C (30 s), 55°C (30 s) and 72°C (30 s), with a final extension of 5 min at 72°C. PCR products were also purified with AMpure XP. Indexed PCR products were quantified with a Qubit® 3.0 Fluorometer (Life Technologies, USA). Finally, the library was diluted to 4 nM using Tris pH 8.5. For sequencing, the library was denatured with fresh NaOH, diluted with hybridization buffer, and heated. Twenty % of PhiX was used as an internal control. Illumina sequencing was carried out in CINVESTAV-Mérida using an Illumina-MiSeq platform (Illumina, San Diego, CA, USA), with a MiSeq reagent nano kit V2 (2 × 250).
Bioinformatic Analysis
We processed the 2 x 250 paired-end reads with the QIIME2 pipeline (Caporaso et al., 2010; Bolyen et al., 2019). Both forward and reverse reads were trimmed in position 40 from the 5’ end and truncated to a length of 240. For denoising and resolving the ASVs we used the DADA2 plugin, removing chimeric sequences with the “consensus” method (Callahan et al., 2016). For the taxonomic classification we used SILVA 132 as a reference database using the classify-consensus-vsearch plugin (Rognes et al., 2016). The sequences were deposited in the NCBI database under BioProject ID, PRJNA810909. Representative sequences were aligned and masked with MAFFT (Katoh and Standley, 2013), and a phylogenetic tree was built with FasterTree 2 (Price et al., 2010). Chloroplast, mitochondrial ASVs, and unknowns were pruned, and the feature table exported to the R environment for statistical analysis performed with phyloseq (McMurdie and Holmes, 2013) using Vegan (Oksanen et al., 2007) and ggplot2 v3.1.0 (Wickham, 2016) libraries.
Statistical Analyses
To analyze the bacterial diversity present within each individual SML sample, the feature table with all samples was normalized with the CSS (Cumulative Sum Scaling) method. The observed species alpha diversity indexes were then calculated. Beta-diversity metrics were calculated for all samples, using the weighed UniFrac distance. A principal coordinate analysis (PCoA) with weighted UniFrac distance was applied in R to visualize beta diversity variations. A nested PERMANOVA according to season and condition was used to calculate the significant differences between groups (control and bleached) using 999 permutations. A Linear Discriminant Analysis Effect Size (LEfSe; Segata et al., 2011) was performed after removing samples with lower than 16,900 reads by rarefaction, to identify bacterial taxa with differential relative abundances between seasons and for the bleached condition, with a cutoff of LDA>2 and a p-value <0.05. The relative abundances were plotted in R with significant LEfSe genera in each category (condition and season). Symbiont density of control and bleached medusas was assessed with an ANOVA test in SigmaPlot V.12.1.
Results
Experimentally Bleached Cassiopea xamachana
Bleached C. xamachana was successfully obtained by adding a mixture of sugars. Cellular counts showed a significant reduction of symbiont density in bleached medusas in summer and winter after seven days of treatment (Figure 1A, p = 0.001; Table S1, Supplementary Material 1). After 14 days, and up to the 28 days of treatment, symbiont density remained low and constant, with a total loss of symbionts of 84-99% for bleached medusas in summer and 89-99% in winter. Control medusas preserved their symbionts after 28 days (Figure 1A). We observed a diminishing size of the umbrella in all medusas possibly due to the lack of enough food (Figure S1). Tentacles of bleached medusas were checked with a fluorescence microscope at day 0 and after 28 days of treatment (Figures 1B–E); phenotypic characteristics of the tentacles of control medusas showed fluorescence and brownish color during the 28 days of treatment (Figure 1F) and bleached medusas at day 28 showed the absence of brownish color that gives the presence of symbionts (Figure 1G).
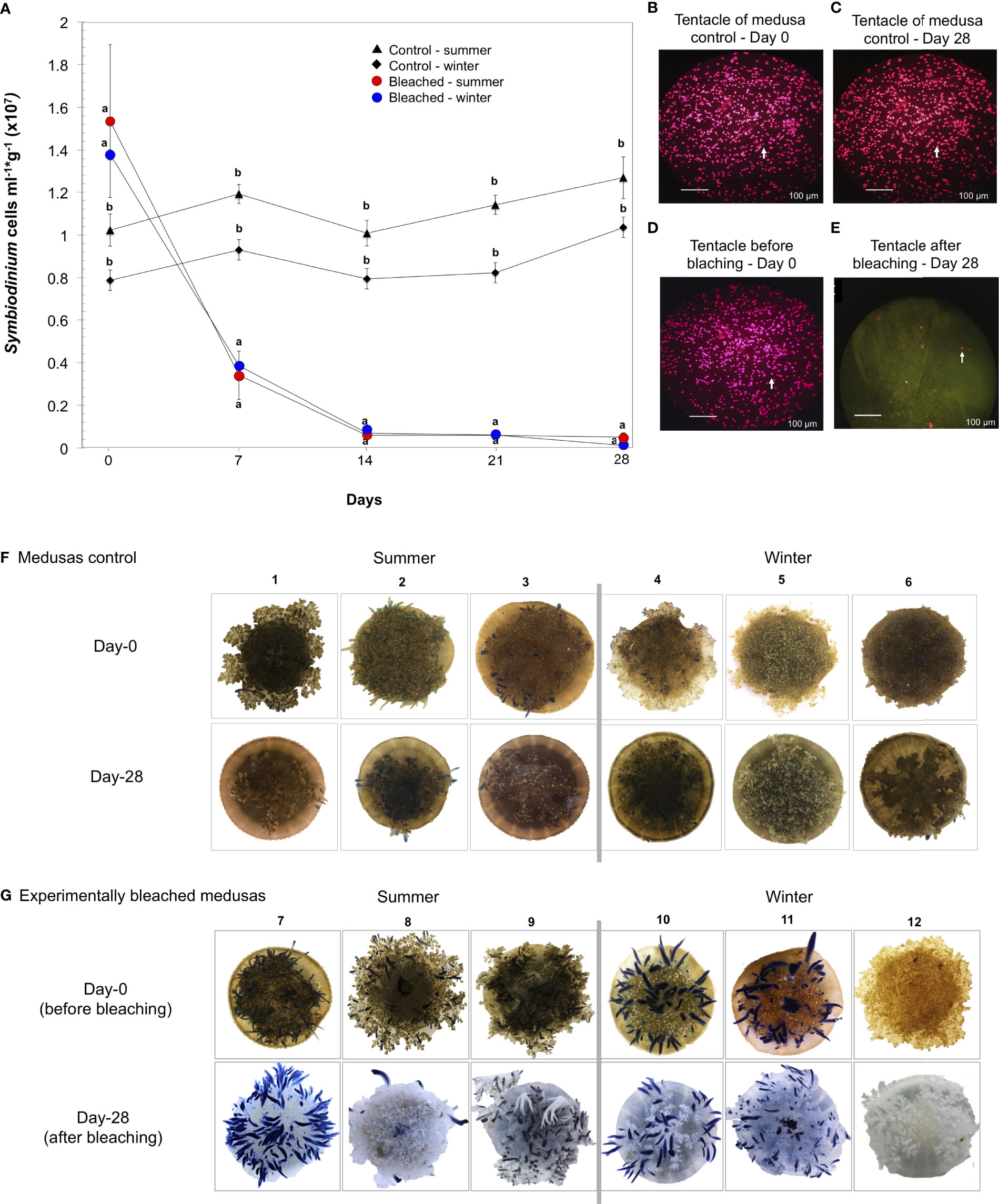
Figure 1 Experimental bleaching and its effects on symbiont density in C. xamachana. Medusas were bleached by daily additions of a mixture of sugars (see Methods). (A) Symbiont cell density (cells ml-1g-1) in medusas treated for 28 days in summer (red circles) and winter (blue circles); control medusas in summer (triangles) and winter (rhombs). Values expressed as the mean ± s.e.m. Letters a and b show significant differences between the density of symbionts from bleached medusas against control medusas. See Supplementary Material 1. (B, C) Confocal fluorescence microscopy images of C. xamachana tentacles of symbiont density in control medusas at day 0 and day 28 and (D, E) symbiont density at the initial (day 0) and end of bleaching treatment (day 28). White arrows indicate symbiont cells in host tentacles, scale bars are 100 μm. (F) Photographs of control medusas from day-0 to 28 days in summer (1-3) and winter (4-6). (G) Photographs of the phenotypic response of medusas at day-0 and after 28 days of bleaching treatment in summer (7-9) and winter (10-12). The numbers on top of the photographs are the identity for each medusa.
Community Composition and Diversity
A total of 24 SML and 11 seawater samples were sequenced. DADA2 plugin analysis resolved 12,854 ASVs (Amplicon Sequences Variants). The sequencing resulted in 1,611,190 high-quality sequence reads (Table S2 and Supplementary Material 2). A total of 610, 883 reads were assigned for seawater and 1,000,307 reads for mucus samples. Control samples in both seasons showed the lowest coverage with 138,376 reads. The analysis of ASVs for all samples indicated 12,654 ASVs affiliated with the domain Bacteria, and only the ASVs with a relative abundance equal or less than 1% were collapsed into “Other” (Supplementary Material 3).
Principal Coordinates Analysis (PCoA) indicated a differential clustering of ASVs from SML and those from seawater samples (Figure S2). Therefore, seawater samples were excluded from subsequent analyses to emphasize the fine-scale differences of ASVs associated with the SML of medusas. The PCoA analysis of control and bleached samples at day-0 showed a close distance among independent replicates in both seasons, except for winter controls (Figure 2). After 28 days in the vessels, control samples were separated according to season, while bleached samples remained grouped according to symbiotic condition (Figure 2). This analysis suggested the effect of the season on the clustering of control samples and the effect of the bleached condition on the clustering of mucus samples after 28 days.
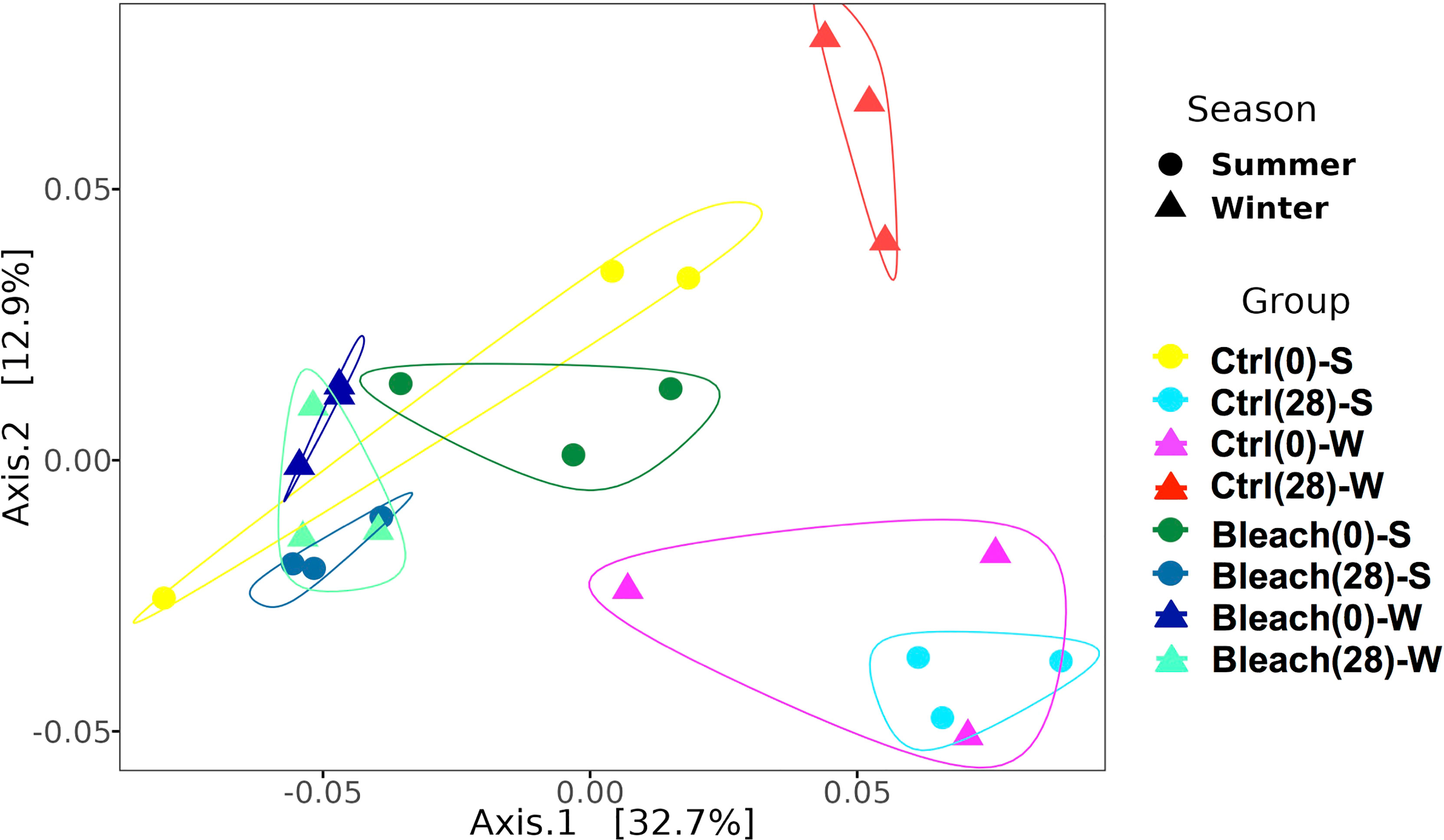
Figure 2 Weighted Unifrac distance PCoA plot for SML samples. Increasing distance between points equates to decreasing similarity between samples from control (Ctrl) and bleached (Bleach) medusas at day-0 and day-28 in summer (S) and winter (W). The polygons denote groups of samples formed. Circles represent a single sample of summer and triangles represent a single sample of winter; the same color represents independent samples per condition or season. Significant differences were found for season (p = 0.017) and symbiotic condition (p = 0.001) after a PERMANOVA comparison (see Table 2).
Control medusas at day-0 and day-28 in both seasons showed a similar number of observed ASVs and alpha diversity parameters, although no statistical analysis was performed due to the low number of samples (Figure 3 and Table S3, Supplementary Material 4). After 28 days of manipulation, bleached medusas in both seasons had a lower number of observed ASVs and alpha diversity parameters.
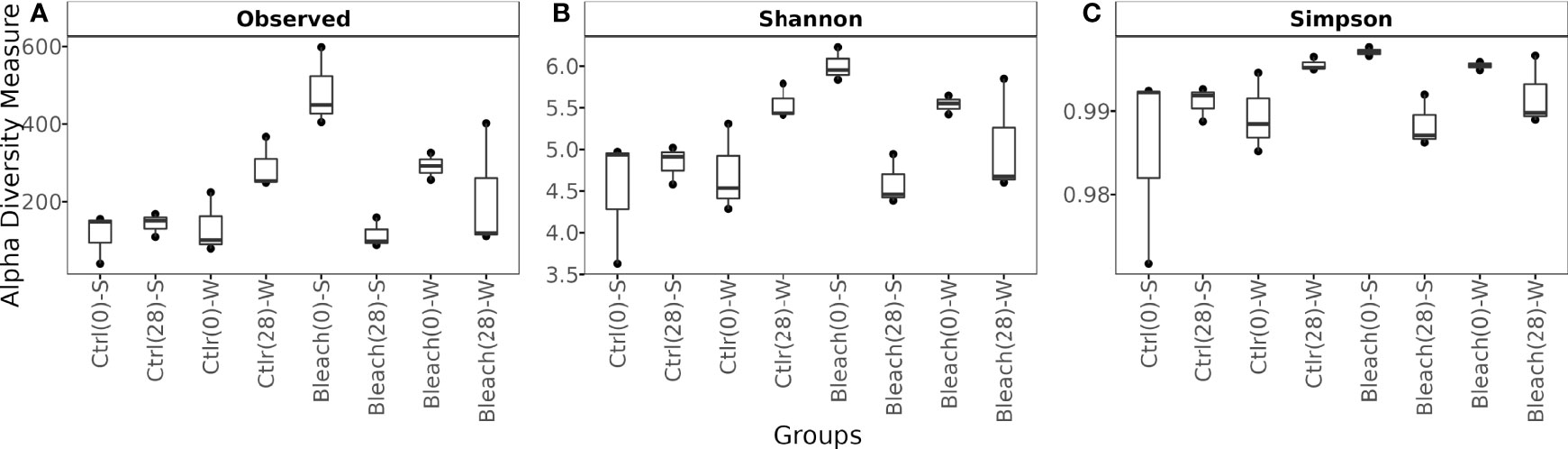
Figure 3 Box plot showing observed ASVs and Alpha diversity metrics. (A) Number of observed ASVs, (B) Shannon (H’), and (C) Simpson diversity indexes of the bacterial community in mucus samples from control (Ctrl) and bleached (Bleach) medusas in summer (S) and winter (W). Values are from 3 samples per symbiotic condition and season (at the start (0) and end (28) of 28 days of manipulations). See Supplementary Material 4 for raw data.
SML Bacterial Community by Symbiotic Condition and Season
Gammaproteobacteria, Bacteroidia, and Alphaproteobacteria were observed as initially dominant classes in all the mucus samples at the beginning of the experiment (Figure S3). However, after 28 days, bleached medusas showed a marked increase in Gammaproteobacteria, specifically in the genus Vibrio for summer samples (Figure S3 and Figure 4). Linear discriminant effect size (LEfSe) analysis identified within bleached medusas initially, Endozoicomonas, Tenacibaculum, and 21 other genera as significantly different, but after 28 days, differentially abundant genera were Vibrio, Sphingobium, and Simiduia (LDA score>2, p <0.05) (Figure 5A). Detailed differences in the relative abundance of bacterial genera that were significantly different in bleached medusas between day-0 and day-28, corroborated these significant shifts, in particular for the more abundant Endozoicomonas and Tenacibaculum (Figure 5B). Seasons also resulted in significant differences although genera were different from those underlined in bleached medusas; in summer were Pir4_lineage, Rheinheimera, Sphingomonas, and others, and in winter Pseudoalteromonas, Enhydrobacter, Acinetobacter, and others (LDA score>2, p <0.05) (Figure 6A), showing differences in the relative abundance according to season (Figure 6B).
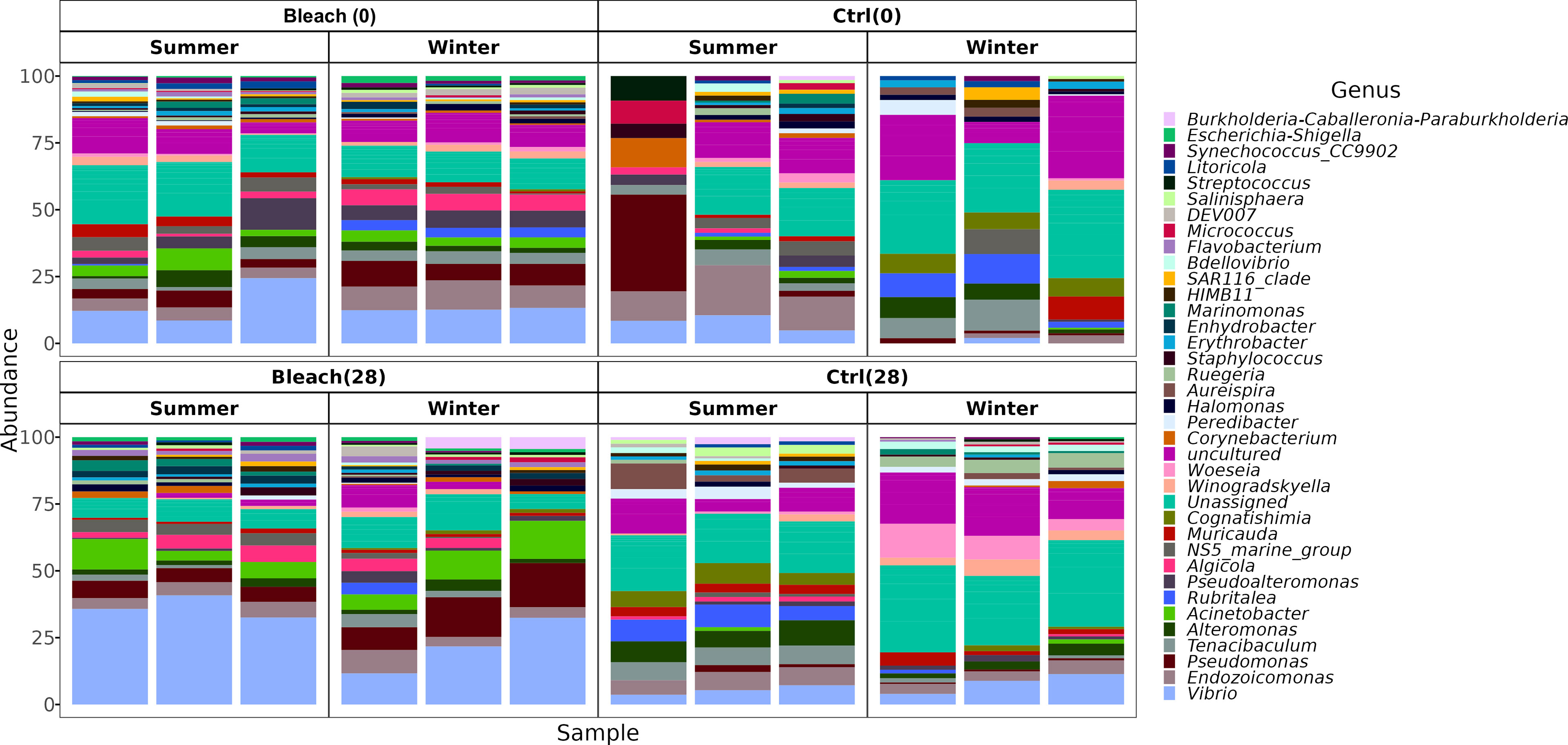
Figure 4 The bacterial composition of medusas at the genus taxonomic level. Bar plots display the bacterial composition in the mucus of all samples (Control and bleached medusas, at days 0 and 28) in summer and winter. The 40 most abundant genera were plotted and distinguished by color. For a relative abundance of all genera, see Supplementary Material 3.
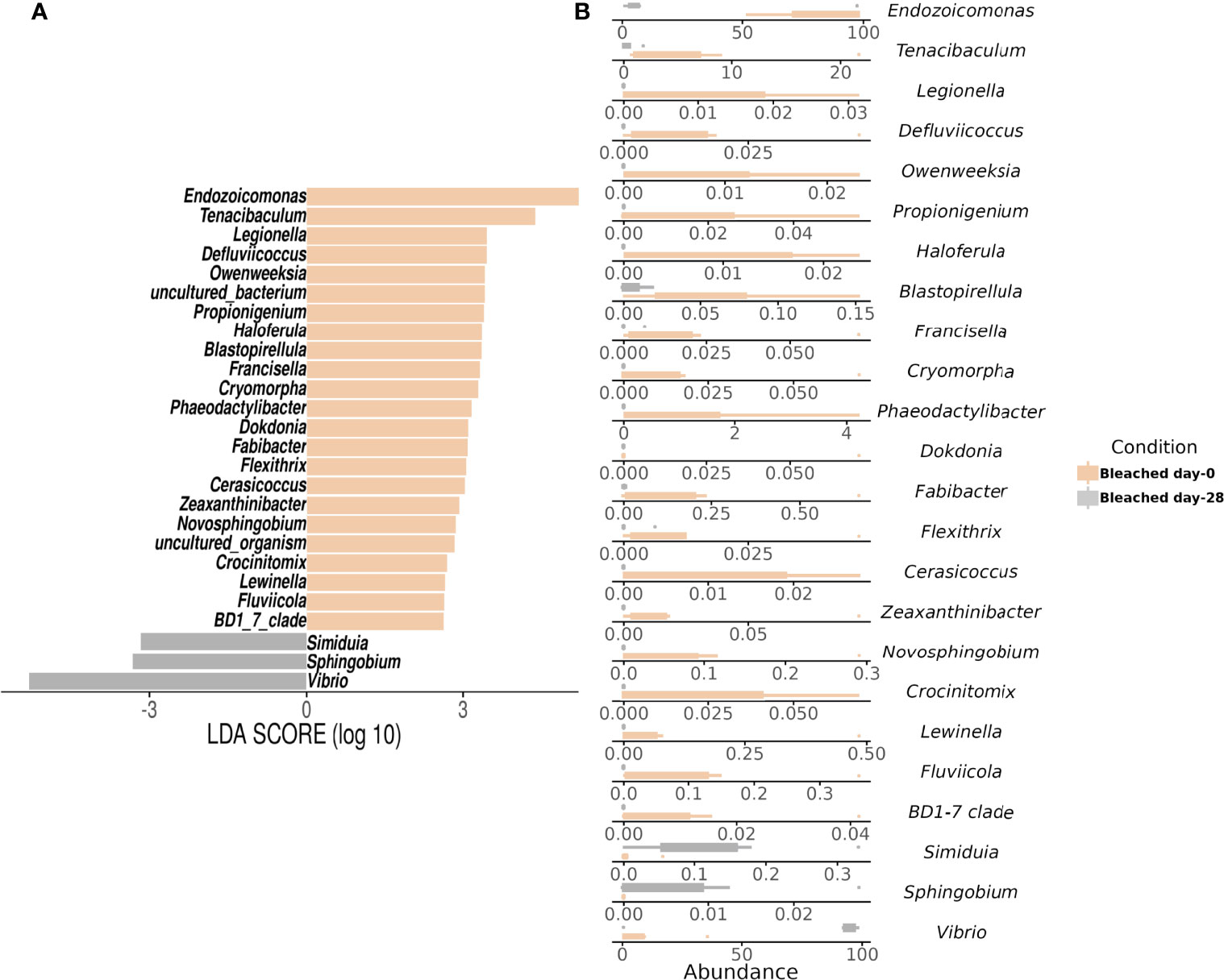
Figure 5 Linear Discriminant Analysis of Effect Size (LEfSe) at the genus level by symbiotic condition in bleached medusas. The analysis shows (A) significant differences in dominant bacterial genera in Bleached medusas between day-0 (beige bars) and day-28 (gray bars) (LDA score>2, p <0.05). Horizontal columns represent the relative abundance of bacteria that were significantly different between symbiotic conditions. (B) Abundance for the genera highlighted by the LEfSe analysis for both sampling times. Note the different values for the x-axis.
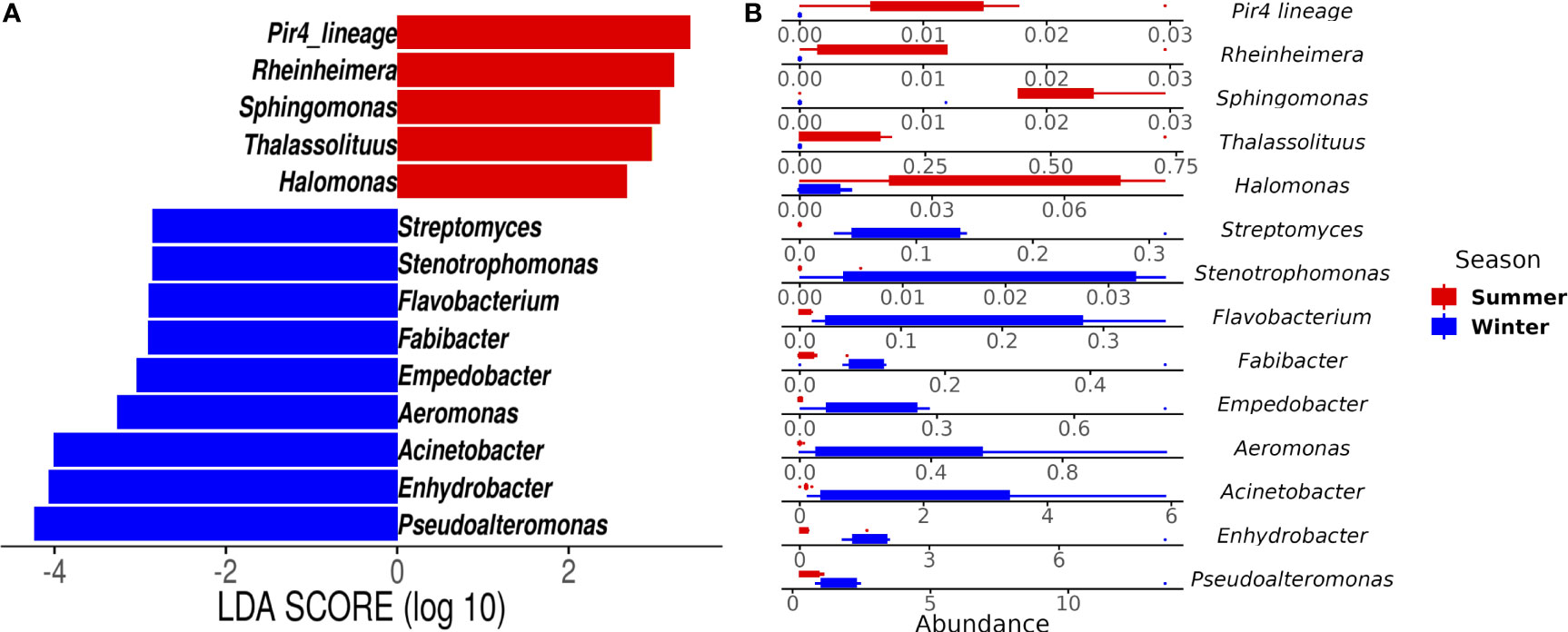
Figure 6 Linear Discriminant Analysis of Effect Size (LEfSe) at the genus level by season. The analysis shows (A) genera that differed significantly in abundance in summer (red bars) and winter samples (blue bars) (LDA score > 2; p < 0.05). (B) Abundance for the genera highlighted by the LEfSe analysis for both seasons. Note the different values for the x-axis.
Pairwise-PERMANOVA analysis indicated significant differences in the bacterial composition of bleached medusas in samples from day-0 and day-28, regardless of the season (F = 5.424345, R = 0.3516743, p = 0.0318; Table 1). Also, this analysis indicated that control and bleached medusas on day-28 were significantly different (F = 6.591283, R= 0.397273, p = 0.0078; Table 1). PERMANOVA covariate analysis showed that the bleached condition was significantly important in defining the bacterial community in the mucus of C. xamachana (F = 5.8289, R = 0.40364, p = 0.001), although the season also presented a value of significance (F = 3.2240, R = 3.2240, p = 0.017; Table 2).
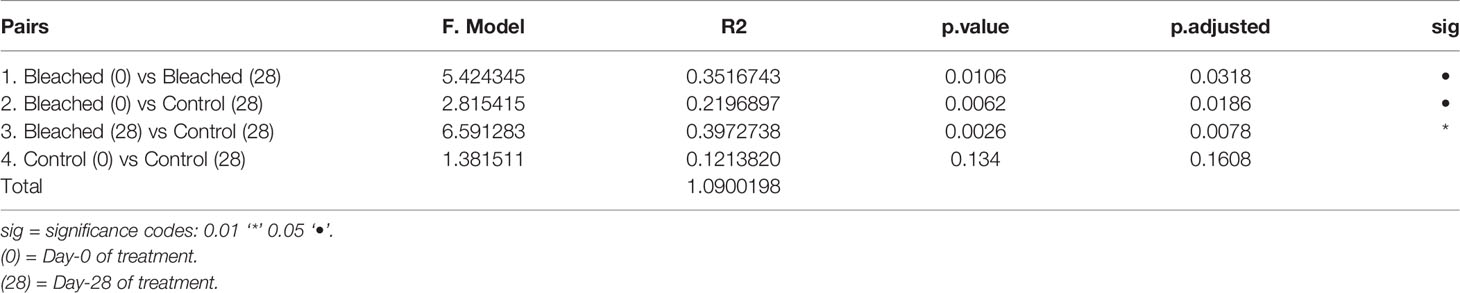
Table 1 Pairwise PERMANOVA analysis of the bacterial community of mucus between Control and Bleached samples.

Table 2 PERMANOVA covariate analysis based on nMDS dissimilarities of the phylogenetic distance of matrix data of the bacterial community of SML by symbiotic condition (control, bleached) and season (summer, winter).
Discussion
Cassiopea xamachana jellyfish naturally harbor symbiotic algae and associate with a surface bacterial community. We have studied this bacterial community by comparing symbiotic and bleached medusas in two seasons. Bleached medusas were obtained by additions of a labile sugar mix (Figure 1) (Pogoreutz et al., 2017). The disruption of the symbiotic relationship in corals by increased organic carbon load is well established, including the expression of bacterial virulence genes and the formation of a hypoxic layer on the coral surface (Vega-Thurber et al., 2009). Elevated levels of dissolved organic carbon (DOC) (e. g. 25 mg L-1) have also been shown to accelerate bacterial growth rates associated with the SML of corals, potentially disrupting the balance of the microbial community (Kline et al., 2006). However, an experimental study showed that the bacterial community from coral fragments treated with enriched DOC levels (e. g. 10 mg L-1), remained stable after 28 days, did not show an increase in virulent bacteria, but caused bleaching of the coral fragments (Pogoreutz et al., 2017); these authors also observed the proliferation of diazotrophs. By using a lower concentration of DOC (0.3 mg L-1) we also induced bleaching of the jellyfish but perhaps with milder effects over the SML-associated bacterial community (Figure 2). It is not clear if moderate additions of labile carbon can promote the abundance of specific bacterial groups that may lead to changes in key members of this community (Kline et al., 2006; Vega-Thurber et al., 2009). For example, Vibrio species highly increase their abundance during coral bleaching events and become dominant (Bourne et al., 2007; Tout et al., 2015; McDevitt-Irwin et al., 2017), possibly due to a reduction of the antibiotic activity of bacteria found in the mucus of corals (Ritchie, 2006), and the ability of Vibrio to utilize a wide variety of sugars (Lee et al., 2016). Also, a study of the jellyfish Aurelia aurita reported an increase in Vibrionaceae bacteria at the end of the jellyfish bloom (Kramar et al., 2019). Further, bacterial groups associated with corals that potentially fix nitrogen (diazotrophs) are common and diverse. These may include Cyanobacteria, Alphaproteobacteria, and Deltaproteobacteria species (Olson et al., 2009). Although our results showed the occurrence of these bacteria, to better distinguish diazotrophs a specific study of nifH genes would have been necessary, considering that such genes correlate with the presence of algal symbionts (Olson et al., 2009).
Bleached medusas showed a lower diversity of bacterial taxa (Figure 3), consistent with reports in stressed corals (McDevitt-Irwin et al., 2017). Medusas sampled during the summer showed a decrease of bacterial diversity after 28 days of incubation but during winter, the bacterial diversity of medusas remained without significant change over time (Figures 3, 4). This contrasts with results reported by Pogoreutz et al. (2017) in corals exposed to high DOC, where they observed an overall decrease of bacterial diversity over time. Our results indicated the dominance of Gammaproteobacteria, Bacteroidia, and Alphaproteobacteria in all mucus samples (Figure S3). Jellyfish species from Indonesia, like Mastigias papua and Tripedalia cystophora, were also characterized by the higher abundance of Gammaproteobacteria class in bleached medusas and the abundant Endozoicomonas genus (Cleary et al., 2016). However, Rhizosotma pulmo, an endemic and common jellyfish of the Mediterranean Sea, has a completely different bacterial community to the one we observed in C. xamachana, even at the Phylum level (Cleary et al., 2016). Kelly et al. (2014) found that algae-dominated coral reefs from the Pacific, correlate with higher abundances of Gammaproteobacteria (such as Alteromonadales, Psuedomonadales, and Vibrionales), Betaproteobacteria, and Bacteriodetes; while reefs with high coral cover, correlate with higher abundances of Alphaproteobacteria (such as Rhodobacteriales and Sphongomonadales). Similar results have been obtained for symbiotic and non-symbiotic reef invertebrates, the former hosting Gammaproteobacteria and the latter Alphaproteobacteria (Bourne et al., 2013). These same bacterial classes have been identified in the gastric cavity of other non-symbiotic marine jellyfish and have been reported with potential in bioremediation, the degradation of polycyclic aromatic hydrocarbons, and the synthesis of antimicrobial compounds (Tinta et al., 2019).
In the present study, the initial abundance of Endozoicomonas (Figure 5) was consistent with previous studies that report a high frequency of this genus and with the highest abundance among reef-forming corals (Bayer et al., 2013; Glasl et al., 2016; Neave et al., 2017a; Pogoreutz et al., 2017). However, Shiu et al. (2020) indicated that the relative abundance of Endozoicomonas usually decreases with heat-induced coral bleaching and perhaps positively correlates with Symbiodiniaceae abundance. It remains unclear whether this phenomenon of decreased Endozoicomonas abundance is caused by temperature stress or a decreased abundance of Symbiodiniaceae. In this respect, our results also suggest a correlation of Endozoicomonas with the presence of symbionts, and in the absence of heat stress. Additionally, Endozoicomonas has been recognized as an important, common bacterial genus, extremely flexible and diverse, associated with a high variety of marine organisms (Morrow et al., 2012; Forget and Juniper, 2013; Katharios et al., 2015; Neave et al., 2017b). Even though their role is not clearly defined yet, it has been proposed that some of their functions are related to nutrient acquisition and recycling of carbon and nitrogen (Morrow et al., 2015), antibiotic production, and the structuring of the microbiota (Bayer et al., 2013; Jessen et al., 2013; Rua et al., 2014). Endozoicomonas has also been found in high abundances and associated with other non-symbiotic marine jellyfish, being considered for its possible application in biotechnology and as a candidate for studies in probiotic applications (Mansson et al., 2011; Deering et al., 2016; Viver et al., 2017; Tinta et al., 2019).
The experimental bleaching of C. xamachana was difficult to achieve. We tried different bleaching methods (low temperature, menthol, DCMU addition) with poor success; medusas either lost a considerable amount of mass or died. The addition of sugars successfully caused the loss of algal symbionts and, even though the animals lost mass (≈ 30%), they were kept alive and active. Sugar addition has the downside of altering the associated bacterial community, increasing diazotroph abundance (Pogoreutz et al., 2017), which we may have noted as an increase in the relative abundance of Vibrio (Figures 4, 5). However, the relative abundance of Vibrio also increases with environmental deterioration and bleaching (Vega-Thurber et al., 2009; Kelly et al., 2014). A different bleaching method could help distinguish between these two factors (lack of symbionts and sugar addition) but is methodologically challenging. Considering that (1) we used a low sugar concentration for the experimental bleaching of the medusas, and (2) our results showed a significant effect of the loss of symbionts on the associated bacterial community, we may suggest it is plausible that algal symbionts may influence the structuring of the associated bacterial community.
The bacterial community of medusas like C. xamachana and other symbiotic model organisms has only begun to be studied over the past few years. Some studies have attempted to demonstrate cooperation between the associated microorganisms, and that the host may be able to select them (Fraune et al., 2015; Har et al., 2015). In our study the bleaching stress was unavoidable but there is still a lack of experimental evidence linking specific factors and the increase of Vibrio during bleaching. Overall, studies about the functional capabilities of the bacterial community of C. xamachana, can help us identify specific roles of certain members of this community that change in response to a loss of symbionts. Similar studies have identified other dominant families like Moraxellaceae and Pseudomonadaceae in C. xamachana when the nitrogen conditions are not limiting for the algal symbiont (Röthig et al., 2021). The associated bacteria participate in the elimination of dissolved inorganic nitrogen (DIN) to overcome the nitrogen limitation of the symbiotic algae, a mechanism that has been proposed to help stabilize the host-algae relationship (Röthig et al., 2021). However, functional studies of bacterial members will further help understand how biological factors, like alterations of the algal symbionts, may interact with this community and the key role of the photosynthetic activity of Symbiodiniaceae in the maintenance of the bacterial community, as previously reported (Littman et al., 2010).
Some bacterial members in the mucus in C. xamachana were enriched for specific taxa at each season (Figure 6). Studies confirm that seasonal fluctuations include changes in the physiology of the associated host, in the months of low sea temperatures (winter); it has been documented those corals such as Astrangia poculata decrease their metabolic functions, retract their polyps, and feed less, losing biomass in consequence (Grace, 2017). As temperatures increase, the metabolic activity of the holobiont resumes, as occurs in tropical corals (Mouchka et al., 2010; Zaneveld et al., 2016; Sharp et al., 2017). In summer, the coral holobiont consumes more food and possibly supplies more substrates that promote bacterial growth following a restructuring of the bacterial members (Burmester et al., 2017; Grace, 2017). The variation in certain bacterial groups by seasonality suggests that the bacterial communities undergo a succession, that is, changes in the bacterial community can occur during the colder winter months, followed by a restructuring of the bacterial community in summer. Lastly, the genetic variation of the host may also play a role. According to several studies (Arai, 2001; Holland et al., 2004; Arai et al., 2017; Ohdera et al., 2018) molecular phylogenetic analyses indicate individuals of Cassiopea analyzed from the same geographic area corresponding with different clades.
Conclusions
The addition of sugars was confirmed to have considerable potential as a method for rapid and effective bleaching in C. xamachana. This method can broaden the study of symbiont-related questions like carbon metabolism and limitation, dissociation of host and algae, conditions for reestablishing this relationship, or capacities for symbiont cooperation. Endozoicomonas, Tenacibaculum, and 21other bacterial genera were abundant in medusas at day-0; however, it is not clear if DOC addition, symbiont loss, or both, were significant in provoking the shift of the bacterial community in the bleached condition, increasing the abundance of Vibrio. Seasonal fluctuations did not cause significant variations in dominant bacterial members; such changes were better associated with symbiont presence. However, given the low number of replicates we used, and the natural variation of the bacteria associated with the medusae, probably also dependent on a genetic variation of the host, more studies will be needed to ascertain this claim. At the class level, the bacteria in the mucus of C. xamachana were similar to bacterial classes found in other tropical, symbiotic Rhizostomeae jellyfish, in the model organism Exaiptasia anemone, and in reef-building corals, mainly dominated by Gammaproteobacteria and Alphaproteobacteria. Future studies with this model organism can enlighten the role of symbionts in driving the stability of the associated bacterial community. Such studies may include seasonal fluctuations and bleaching events, triggered by seawater temperature, nutrient levels, and climate change, all associated with diseases and mortality in symbiotic corals. Also, studies in other regions where C. xamachana distributes, can help us identify stable and functional associations of bacteria that may contribute to the fitness of this jellyfish.
Data Availability Statement
The original contributions presented in the study are included in the article/Supplementary Material and the NCBI (https://www.ncbi.nlm.nih.gov/) under accession no. PRJNA810909. Further inquiries can be directed to the corresponding author/s.
Author Contributions
NC, PT contributed to the conception and design of the study. NC, DC-G organized the databases. NC, DC-G performed the statistical analysis. NC wrote the first draft of the manuscript. PT, JG-M wrote and edited sections of the manuscript. All authors contributed to manuscript revision, read, and approved the submitted version.
Funding
This work was supported by PAPIIT-DGAPA-UNAM [grant IN204318] to PET. ICML-UNAM provided funds for open access publication fees.
Conflict of Interest
The authors declare that the research was conducted in the absence of any commercial or financial relationships that could be construed as a potential conflict of interest.
Publisher’s Note
All claims expressed in this article are solely those of the authors and do not necessarily represent those of their affiliated organizations, or those of the publisher, the editors and the reviewers. Any product that may be evaluated in this article, or claim that may be made by its manufacturer, is not guaranteed or endorsed by the publisher.
Acknowledgments
We thank the support of Posgrado en Ciencias del Mar y Limnología, UNAM, and a scholarship to NC from CONACYT [CVU 544689].
Supplementary Material
The Supplementary Material for this article can be found online at: https://www.frontiersin.org/articles/10.3389/fmars.2022.879184/full#supplementary-material
References
Aljbour S. M., Zimmer M., Al-Horani F. A., Kunzmann A. (2019). Metabolic and Oxidative Stress Responses of the Jellyfish Cassiopea Sp. To Changes in Seawater Temperature. J. Sea Res. 145, 1–7. doi: 10.1016/j.seares.2018.12.002
Arai M. N. (2001). Pelagic Coelenterates and Eutrophication: A Review. Hydrobiologia 451, 69–87. doi: 10.1023/A:1011840123140
Arai Y., Gotoh R. O., Yokoyama J., Sato C., Okuizumi K., Hanzawa N. (2017). Phylogenetic Relationships and Morphological Variations of Upside-Down Jellyfishes, Cassiopea Spp. Inhabiting Palau Island. Biogeography 19, 133–141. doi: 10.11358/biogeo.19.133
Bayer T., Arif C., Ferrier-Pagès C., Zoccola D., Aranda-Lastra M., Voolstra C. R. (2013). Bacteria of the Genus Endozoicomonas Dominate the Microbiome of the Mediterranean Gorgonian Coral Eunicella Cavolini. Mar. Ecol. Prog. Ser. 479, 75–84. doi: 10.3354/meps10197
Bolyen E., Rideout J. R., Dillon M. R., Bokulich N. A., Abnet C. C., Al-Ghalith G. A., et al. (2019). Reproducible, Interactive, Scalable and Extensible Microbiome Data Science Using QIIME 2. Nat. Biotechnol. 37, 852–857. doi: 10.1038/s41587-019-0209-9
Bosch T. C. (2013). Cnidarian-Microbe Interactions and the Origin of Innate Immunity in Metazoans. Annu. Rev. Microbiol. 67, 499–518. doi: 10.1146/annurev-micro-092412-155626
Bourne D. G., Dennis P. G., Uthicke S., Soo R. M., Tyson G. W., Webster N. (2013). Coral Reef Invertebrate Microbiomes Correlate With the Presence of Photosymbionts. ISME J. 7, 1452–1458. doi: 10.1038/ismej.2012.172
Bourne D., Iida Y., Uthicke S., Smith-Keune C. (2007). Changes in Coral-Associated Microbial Communities During a Bleaching Event. ISME J. 2, 350–363. doi: 10.1038/ismej.2007.112
Bourne D. G., Morrow K. M., Webster N. S. (2016). Insights Into the Coral Microbiome: Underpinning the Health and Resilience of Reef Ecosystems. Annu. Rev. Microbiol. 70, 317–340. doi: 10.1146/annurev-micro-102215-095440
Brown B. E., Bythell J. C. (2005). Perspectives on Mucus Secretion in Reef Corals. Mar. Ecol. Prog. Ser. 296, 291–309. doi: 10.3354/meps296291
Burmester E. M., Finnerty J. R., Kaufman L., Rotjan R. D. (2017). Temperature and Symbiosis Affect Lesion Recovery in Experimentally Wounded, Facultatively Symbiotic Temperate Corals. Mar. Ecol. Prog. Ser. 570, 87–99. doi: 10.3354/meps12114
Bythell J. C., Wild C. (2011). Biology and Ecology of Coral Mucus Release. J. Exp. Mar. Biol. Ecol. 408, 88–93. doi: 10.1016/j.jembe.2011.07.028
Callahan B. J., McMurdie P. J., Rosen M. J., Han A. W., Johnson A. M. J. A., Holmes S. P. (2016). DADA2: High Resolution Sample Inference From Illumina Amplicon Data. Nat. Methods 13, 581–583. doi: 10.1038/nmeth.3869
Caporaso J. G., Kuczynski J., Stombaugh J., Bittinger K., Bushman F. D., Costello E. K. (2010). QIIME Allows Analysis of High-Throughput Community Sequencing Data. Nat. Methods 7, 335–336. doi: 10.1038/nmeth.f.303
Carlos C., Torres T. T., Ottoboni L. M. M. (2013). Bacterial Communities and Species-Specific Associations With the Mucus of Brazilian Coral Species. Sci. Rep. 3, 1624. doi: 10.1038/srep01624
Cleary D. R., Becking L. E., Polónia A. M., Freitas R. M., Gomes N. M. (2016). Jellyfish Associated Bacterial Communities and Bacterioplankton in Indonesian Marine Lakes. FEMS Microbiol. Ecol. 92, 1–14. doi: 10.1093/femsec/fiw064
Colley N. J., Trench R. K. (1983). Selectivity in Phagocytosis and Persistence of Symbiotic Algae by the Scyphistoma Stage of the Jellyfish Cassiopea Xamachana. Proc. R. Soc. London B 219, 61–82. doi: 10.1098/rspb.1983.0059
Deering R. W., Chen J., Sun J., Ma H., Dubert J., Barja J. L., et al. (2016). N-Acyl Dehydrotyrosines, Tyrosinase Inhibitors From the Marine Bacterium Thalassotalea Sp. J. Nat. Prod. 79, 447–450. doi: 10.1021/acs.jnatprod.5b00972
Djeghri N., Pondaven P., Stibor H., Dawson M. N. (2019). Review of the Diversity, Traits, and Ecology of Zooxanthellate Jellyfishes. Mar. Biol. 166, 147. doi: 10.1007/s00227-019-3581-6
Fitt W. K., Costley K. (1998). The Role of Temperature in Survival of the Polyp Stage of the Tropical Rhizostome Jellyfish Cassiopea Xamachana. J. Exp. Mar. Biol. Ecol. 222, 79–91. doi: 10.1016/S0022-0981(97)00139-1
Forget N. L., Juniper S. K. (2013). Free-Living Bacterial Communities Associated With Tubeworm (Ridgeia Piscesae) Aggregations in Contrasting Diffuse Flow Hydrothermal Vent Habitats at the Main Endeavour Field, Juan De Fuca Ridge. Microbiol. Open 2, 259–275. doi: 10.1002/mbo3.70
Fraune S., Anton-Erxleben F., Augustin R., Franzenburg S., Knop M., Schröder K., et al. (2015). Bacteria-Bacteria Interactions Within the Microbiota of the Ancestral Metazoan Hydra Contribute to Fungal Resistance. ISME J. 9, 1543–1556. doi: 10.1038/ismej.2014.239
Freeman C. J., Stoner E. W., Easson C. G., Matterson K. O., Baker D. M. (2016). Symbiont Carbon and Nitrogen Assimilation in the Cassiopea-Symbiodinium Mutualism. Mar. Ecol. Pror. Ser. 544, 281–286. doi: 10.3354/meps11605
Garrido A. G., Machado L. F., Zilberberg C., Assis L. D. C. (2021). Insights Into ‘Symbiodiniaceae Phycosphere’ in a Coral Holobiont. Symbiosis 83, 25–39. doi: 10.1007/s13199-020-00735-3
Glasl B., Herndl G. J., Frade P. R. (2016). The Microbiome of Coral Surface Mucus has a Key Role in Mediating Holobiont Health and Survival Upon Disturbance. ISME J. 10, 2280–2290. doi: 10.1038/ismej.2016.9
Goulet T. L., Erill I., Ascunce M. S., Finley S. J., Javan G. T. (2020). Conceptualization of the Holobiont Paradigm as It Pertains to Corals. Front. Physiol. 11, 566968. doi: 10.3389/fphys.2020.566968
Grace S. (2017). Winter Quiescence, Growth Rate, and the Release From Competition in the Temperate Scleractinian Coral Astrangia Poculata (Ellis & Solander 1786). Northeast. Nat. 24, 119–134. doi: 10.1656/045.024.s715
Hansson L. J., Norrman B. (1995). Release of Dissolved Organic Carbon (DOC) by the Scyphozoan Jellyfish Aurelia Aurita and Its Potential Influence on the Production of Planktic Bacteria. Mar. Biol. 121, 527–532. doi: 10.1007/bf00349462
Hao W., Gerdts G., Holst S., Wichels A. (2019). Bacterial Communities Associated With Scyphomedusae at Helgoland Roads. Mar. Biodiv. 49, 1489–1503. doi: 10.1007/s12526-018-0923-4
Har J. Y., Helbig T., Lim J. H., Fernando S. C., Reitzel A. M., Penn K., et al. (2015). Microbial Diversity and Activity in the Nematostella Vectensis Holobiont: Insights From 16S rRNA Gene Sequencing, Isolate Genomes, and a Pilot-Scale Survey of Gene Expression. Front. Microbiol. 6, 818. doi: 10.3389/fmicb.2015.00818
Hernandez-Agreda A., Gates R. D., Ainsworth T. D. (2017). Defining the Core Microbiome in Corals' Microbial Soup. Trends. Microbiol. 25, 125–140. doi: 10.1016/j.tim.2016.11.003
Hofmann D. K., Fitt W. K., Fleck J. (1996). Checkpoints in the Life-Cycle of Cassiopea Spp.: Control of Metagenesis and Metamorphosis in a Tropical Jellyfish. Int. J. Dev. Biol. 40, 331–338.
Hofmann D. K., Kremer B. P. (1981). Carbon Metabolism and Strobilation in Cassiopea Andromeda (Cnidaria: Scyphozoa): Significance of Endosymbiotic Dinoflagellates. Mar. Biol. 65, 25–33. doi: 10.1007/BF00397064
Holland B. S., Dawson M. N., Crow G. L., Hofmann D. K. (2004). Global Phylogeography of Cassiopea (Scyphozoa: Rhizostomeae): Molecular Evidence for Cryptic Species and Multiple Invasions of the Hawaiian Islands. Mar. Biol. 145, 1119–1128. doi: 10.1007/s00227-004-1409-4
Jessen C., Villa Lizcano J. F., Bayer T., Roder C., Aranda M., Wild C., et al. (2013). In-Situ Effects of Eutrophication and Overfishing on Physiology and Bacterial Diversity of the Red Sea Coral Acropora Hemprichii. PLos One 8, e62091. doi: 10.1371/journal.pone.0062091
Katharios P., Seth-Smith H. M. B., Fehr A., Mateos J. M., Qi W., Richter D., et al. (2015). Environmental Marine Pathogen Isolation Using Mesocosm Culture of Sharpsnout Seabream: Striking Genomic and Morphological Features of Novel Endozoicomonas Sp. Sci. Rep. 5, 17609. doi: 10.1038/srep17609
Katoh K., Standley D. M. (2013). MAFFT Multiple Sequence Alignment Software Versión 7: Improvements in Performance and Usability. Mol. Biol. Evol. 30, 772–780. doi: 10.1093/molbev/mst010
Kelly L. W., Williams G. J., Barott K. L., Carlson C. A., Dinsdale E. A., Edwards R. A., et al. (2014). Local Genomic Adaptation of Coral Reef-Associated Microbiomes to Gradients of Natural Variability and Anthropogenic Stressors. Proc. Natl. Acad. Sci. U. S. A. 111, 10227–10232. doi: 10.1073/pnas.1403319111
Klindworth A., Pruesse E., Schweer T., Peplies J., Quast C., Horn M., et al. (2013). Evaluation of General 16S Ribosomal RNA Gene PCR Primers for Classical and Next-Generation Sequencing-Based Diversity Studies. Nucl. Acid Res. 41, e1. doi: 10.1093/nar/gks808
Kline D. I., Kuntz N. M., Breitbart M., Knowlton N., Rowher F. (2006). Role of Elevated Organic Carbon Levels and Microbial Activity in Coral Mortality. Mar. Ecol. Prog. Ser. 314, 119–125. doi: 10.3354/meps314119
Kramar M. K., Tinta T., Lucic D., Malej A., Turk V. (2019). Bacteria Associated With Moon Jellyfish During Bloom and Post-Bloom Periods in the Gulf of Trieste (Northern Adriatic). PLos One 14, e0198056. doi: 10.1371/journal.pone.0198056
Krediet C. J., Ritchie K. B., Alagely A., Teplitski M. (2012). Members of Native Coral Microbiota Inhibit Glycosidases and Thwart Colonization of Coral Mucus by an Opportunistic Pathogen. ISME J. 7, 980–990. doi: 10.1038/ismej.2012.164
Lampert K. P. (2016). “Cassiopea and Its Zooxanthellae,” in The Cnidaria, Past, Present and Future: The World of Medusa and Her Sisters. Eds. Goffredo S., Dubinsky Z. (Switzerland: Springer International Publishing), 415–423.
Lampert Y., Kelman D., Nitzan Y., Dubinsky Z., Behar A., Hill R. T. (2008). Phylogenetic Diversity of Bacteria Associated With the Mucus of Red Sea Corals. FEMS Microbiol. Ecol. 64, 187–198. doi: 10.1111/j.1574-6941.2008.00458.x
Lee S. T. M., Davy S. K., Tang S. L., Kench P. S. (2016). Mucus Sugar Content Shapes the Bacterial Community Structure in Thermally Stressed Acropora Muricata. Front. Microbiol. 7, 371. doi: 10.3389/fmicb.2016.00371
Lesser M. P., Mazel C. H., Gorbunov M. Y., Falkowski P. G. (2004). Discovery of Symbiotic Nitrogen-Fixing Cyanobacteria in Corals. Science 305, 997–1000. doi: 10.1126/science.1099128
Littman R. A., Bourne D. G., Willis B. L. (2010). Responses of Coral-Associated Bacterial Communities to Heat Stress Differ With Symbiodinium Type on the Same Coral Host. Mol. Ecol. 19, 1978–1990. doi: 10.1111/j.1365-294X.2010.04620.x
Littman R. A., Willis B. L., Bourne D. G. (2011). Metagenomic Analysis of the Coral Holobiont During a Natural Bleaching Event on the Great Barrier Reef. Environ. Microbiol. Rep. 3, 651–660. doi: 10.1111/j.1758-2229.2010.00234.x
Maire J., Girvan S. K., Barkla S. E. (2021). Intracellular Bacteria Are Common and Taxonomically Diverse in Cultured and in Hospite Algal Endosymbionts of Coral Reefs. ISME J. 15, 2028–2042. doi: 10.1038/s41396-021-00902-4
Mansson M., Gram L., Larsen A. (2011). Production of Bioactive Secondary Metabolites by Marine Vibrionaceae. Mar. Drugs 9, 1440–1468. doi: 10.3390/md9091440
Matthews J. L., Raina J. B., Kahlke T., Seymour J. R., van Oppen M. J. H., Suggett D. J. (2020). Symbiodiniaceae-Bacteria Interactions: Rethinking Metabolite Exchange in Reef-Building Corals as Multi-Partner Metabolic Networks. Environ. Microbiol. 22, 1675–1687. doi: 10.1111/1462-2920.14918
McCloskey L. R., Muscatine L., Wilkerson F. P. (1994). Daily Photosynthesis, Respiration, and Carbon Budgets in a Tropical Marine Jellyfish (Mastigias Sp.). Mar. Biol. 119, 13–22. doi: 10.1007/BF00350101
McDevitt-Irwin J. M., Baum J. K., Garren M., Vega Thurber R. L. (2017). Responses of Coral-Associated Bacterial Communities to Local and Global Stressors. Front. Mar. Sci. 4, 262. doi: 10.3389/fmars.2017.00262
McGill C. J., Pomoroy C. M. (2008). Effects of Bleaching and Nutrient Supplementation on Wet Weight in the Jellyfish Cassiopea Xamachana (Bigelow) (Cnidaria: Scyphozoa). Mar. Freshw. Behav. Physiol. 41, 179–189. doi: 10.1080/10236240802369899
McMurdie P. J., Holmes S. (2013). Phyloseq: An R Package for Reproducible Interactive Analysis and Graphics of Microbiome Census Data. PLos One 8, e61217. doi: 10.1371/journal.pone.0061217
Morrow K. M., Bourne D. G., Humphrey C., Botté E. S., Laffy P., Zaneveld J., et al. (2015). Natural Volcanic CO2 Seeps Reveal Future Trajectories for Host-Microbial Associations in Corals and Sponges. ISME J. 9, 894–908. doi: 10.1038/ismej.2014.188
Morrow K. M., Moss A. G., Chadwick N. E., Liles M. R. (2012). Bacterial Associates of Two Caribbean Coral Species Reveal Species-Specific Distribution and Geographic Variability. Appl. Environ. Microb. 78, 6438–6449. doi: 10.1128/AEM.01162-12
Mouchka M. E., Hewson I., Harvell I. D. (2010). Coral-Associated Bacterial Assemblages: Current Knowledge and the Potential for Climate-Driven Impacts. Integr. Comp. Biol. 50, 662–674. doi: 10.1093/icb/icq061
Neave M. J., Michell C. T., Apprill A., Voolstra C. R. (2017a). Endozoicomonas Genomes Reveal Functional Adaptation and Plasticity in Bacterial Strains Symbiotically Associated With Diverse Marine Hosts. Sci. Rep. 7, 40579. doi: 10.1038/srep40579
Neave M. J., Rachmawati R., Xun L., Michell C. T., Bourne D. G., Aprill A., et al. (2017b). Differential Specificity Between Closely Related Corals and Abundant Endozoicomonas Endosymbionts Across Global Scales. ISME J. 11, 186–200. doi: 10.1038/ismej.2016.95
Newkirk C. R., Frazer T. K., Martindale M. Q. (2018). Acquisition and Proliferation of Algal Symbionts in Bleached Polyps of the Upside-Down Jellyfish, Cassiopea Xamachana. J. Exp. Mar. Biol. Ecol. 508, 44–51. doi: 10.1016/j.jembe.2018.08.010
Niggl W., Naumann M. S., Struck U., Manasrah R., Wild C. (2010). Organic Matter Release by the Benthic Upside-Down Jellyfish Cassiopea Sp. Fuels Pelagic Food Webs in Coral Reefs. J. Exp. Mar. Biol. Ecol. 384, 99–106. doi: 10.1016/j.jembe.2010.01.011
Ohdera A. H., Abrams M. J., Ames C. L., Baker D. M., Suescún-Bolívar L. P., Collins A. G., et al. (2018). Upside-Down But Headed in the Right Direction: Review of the Highly Versatile Cassiopea Xamachana System. Front. Ecol. Evol. 6, 35. doi: 10.3389/fevo.2018.00035
Oksanen J., Kindt R., Legendre P., O’Hara B., Stevens M. H. H., Oksanen M. J., et al. (2007). The Vegan Package. Community Ecol. Package 10 (631-637), 719.
Olson N. D., Ainsworth T. D., Gates R. D., Takabayashi M. (2009). Diazotrophic Bacteria Associated With Hawaiian Montipora Corals: Diversity and Abundance in Correlation With Symbiotic Dinoflagellates. J. Exp. Mar. Biol. Ecol. 371, 140–146. doi: 10.1016/j.jembe.2009.01.012
Peng S., Hao W., Li Y., Wang L., Sun T., Zhao J., et al. (2021). Bacterial Communities Associated With Four Blooming Scyphozoan Jellyfish: Potential Species-Specific Consequences for Marine Organisms and Human Health. Front. Microbiol. 12, 647089. doi: 10.3389/fmicb.2021.647089
Pitt K. A., Koop K., Rissik D. (2005). Contrasting Contributions to Inorganic Nutrient Recycling by the Co-Occurring Jellyfishes, Catostylus Mosaicus and Phyllorhiza Punctata (Scyphozoa, Rhizostomeae). J. Exp. Mar. Biol. Ecol. 315, 71–86. doi: 10.1016/j.jembe.2004.09.007
Pogoreutz C., Rädecker N., Cárdenas A., Gärdes A., Voolstra C. R., Wild C. (2017). Sugar Enrichment Provides Evidence for a Role of Nitrogen Fixation in Coral Bleaching. Glob. Change Biol. 23, 3838–3848. doi: 10.1111/gcb.13695
Price M. N., Dehal P. S., Arkin A. P. (2010). FastTree 2-Approximately Maximum-Likelihood Trees for Large Alignments. PLos One 5, e9490. doi: 10.1371/journal.pone.0009490
Rädecker N., Pogoreutz C., Voolstra C. R., Wiedenmann J., Wild C. (2015). Nitrogen Cycling in Corals: The Key to Understanding Holobiont Functioning? Trends Microbiol. 23, 490–497. doi: 10.1016/j.tim.2015.03.008
Ritchie K. B. (2006). Regulation of Microbial Populations by Coral Surface Mucus and Mucus -Associated Bacteria. Mar. Ecol. Prog. Ser. 322, 1–14. doi: 10.3354/meps322001
Rivera-Ortega J., Thomé P. E. (2018). Contrasting Antibacterial Capabilities of the Surface Mucus Layer From Three Symbiotic Cnidarians. Front. Mar. Sci. 5, 392. doi: 10.3389/fmars.2018.00392
Rognes T., Flouri T., Nichols B., Quince C., Mahé F. (2016). VSEARCH: A Versatile Open Source Tool for Metagenomics. PeerJ 4, e2584. doi: 10.7717/peerj.2584
Rohwer F., Seguritan V., Azam F., Knowlton N. (2002). Diversity and Distribution of Coral-Associated Bacteria. Mar. Ecol. Prog. Ser. 243, 1–10. doi: 10.3354/meps243001
Röthig T., Puntin G., Wong J. C. Y., Burain A., McLeod W., Baker D. M. (2021). Holobiont Nitrogen Control and Its Potential for Eutrophication Resistance in an Obligate Photosymbiotic Jellyfish. Microbiome 9, 127. doi: 10.1186/s40168-021-01075-0
Röthig T., Roik A., Yum L., Voolstra C. R. (2017). Distinct Bacterial Microbiomes Associate With the Deep-Sea Coral Eguchipsammia Fistula From the Red Sea and From Aquaria Settings. Front. Mar. Sci. 4, 259. doi: 10.3389/fmars.2017.00259
Rua C. P. J., Trindade-Silva A. E., Appolinario L. R., Venas T. M., Garcia G. D., Carvalho L. S., et al. (2014). Diversity and Antimicrobial Potential of Culturable Heterotrophic Bacteria Associated With the Endemic Marine Sponge Arenosclera Brasiliensis. PeerJ 2, e419. doi: 10.7717/peerj.419
SAMMO (2018-2019) Universidad Nacional Autónoma De México, Instituto De Ciencias Del Mar Y Limnología, Servicio Académico De Monitoreo Meteorológico Y Oceanográfico, Puerto Morelos Q. Roo México. Available at: www.sammo.icmyl.unam.mx (Accessed 15 October, 2021).
Segata N., Izard J., Waldron L., Gevers D., Miropolsky L., Garret W. S., et al. (2011). Metagenomic Biomarker Discovery and Explanation. Genome Biol. 12, R60. doi: 10.1186/gb-2011-12-6-r60
Sharp K. H., Pratte Z. A., Kerwin A. H., Rotjan R. D., Stewart F. J. (2017). Season, But Not Symbiont State, Drives Microbiome Structure in the Temperate Coral Astrangia Poculata. Microbiome 5, 120. doi: 10.1186/s40168-017-0329-8
Shiu J.-H., Yu S.-P., Fong C.-L., Ding J.-Y., Tan C.-J., Fan T.-Y., et al. (2020). Shifting in the Dominant Bacterial Group Endozoicomonas Is Independent of the Dissociation With Coral Symbiont Algae. Front. Microbiol. 11, 1791. doi: 10.3389/fmicb.2020.01791
Stoner E. W., Layman C. A., Yager L. A., Hassett H. M. (2011). Effects of Anthropogenic Disturbance on the Abundance and Size of Epibenthic Jellyfish Cassiopea Spp. Mar. Poll. Bull. 62, 1109–1114. doi: 10.1016/j.marpollbul.2011.03.023
Tinta T., Kogovšek T., Klun K., Malej A., Herndl G. J., Turk V. (2019). Jellyfish-Associated Microbiome in the Marine Environment: Exploring Its Biotechnological Potential. Mar. Drugs 17, 94. doi: 10.3390/md17020094
Tinta T., Kogovšek T., Malej A., Turk V. (2012). Jellyfish Modulate Bacterial Dynamic and Community Structure. PLos One 7, e39274. doi: 10.1371/journal.pone.0039274
Tout J., Siboni N., Messer L. F., Garren M., Stocker R., Webster N. S., et al. (2015). Increased Seawater Temperature Increases the Abundance and Alters the Structure of Natural Vibrio Populations Associated With the Coral Pocillopora Damicornis. Front. Microbiol. 6, 432. doi: 10.3389/fmicb.2015.00432
Tremblay P., Grover R., Maguer J. F., Legendre L., Ferrier-Pagès C. (2012). Autotrophic Carbon Budget in Coral Tissue: A New 13C-Based Model of Photosynthate Translocation. J. Exp. Biol. 215, 1384–1393. doi: 10.1242/jeb.065201
Vega-Thurber R., Willner-Hall D., Rodriguez-Mueller B., Rodriguez-Mueller B., Desnues C., Edwards R. A., et al. (2009). Metagenomic Analysis of Stressed Coral Holobionts. Environ. Microbiol. 11, 2148–2163. doi: 10.1111/j.1462-2920.2009.01935.x
Viver T., Orellana L. H., Hatt J. K., Urdiain M., Díaz S., Richter M., et al. (2017). The Low Diverse Gastric Microbiome of the Jellyfish Cotylorhiza Tuberculata Is Dominated by Four Novel Taxa. Environ. Microbiol. 19, 3039–3058. doi: 10.1111/1462-2920.13763
Voolstra C. R. (2013). A Journey Into the Wild of the Cnidarian Model System Aiptasia and Its Symbionts. Mol. Ecol. 22, 4366. doi: 10.1111/mec.12464
Webster N. S., Reusch T. B. H. (2017). Microbial Contributions to the Persistence of Coral Reefs. ISME J. 11, 2167–2174. doi: 10.1038/ismej.2017.66
Weiland-Bräuer N., Neulinger S. C., Pinnow N., Künzel S., Baines J. F., Schmitz R. A. (2015). Composition of Bacterial Communities Associated With Aurelia Aurita Changes With Compartment, Life Stage, and Population. Appl. Environ. Microbiol. 81, 6038–6052. doi: 10.1128/AEM.01601-15
Wickham H. (2016). Ggplot2: Elegant Graphics for Data Analysis. J. Stat. Software 35, 216. doi: 10.1007/978-3-319-24277-4
Wild C., Huettel M., Klueter A., Kremb S. G., Rasheed M. Y. M., Jørgensen B. B. (2004). Coral Mucus Functions as an Energy Carrier and Particle Trap in the Reef Ecosystem. Nature 428, 66–70. doi: 10.1038/nature02344
Wright R. M., Strader M. E., Genuise H. M., Matz M. (2019). Effects of Thermal Stress on Amount, Composition, and Antibacterial Properties of Coral Mucus. PeerJ 7, e6849. doi: 10.7717/peerj.6849
Yang S.-H., Tseng C.-H., Huang C.-R., Chen C.-P., Tandon K., Lee S. T. M., et al. (2017). Long-Term Survey Is Necessary to Reveal Various Shifts of Microbial Composition in Corals. Front. Microbiol. 8, 1094. doi: 10.3389/fmicb.2017.01094
Keywords: symbiotic jellyfish, 16S rRNA, surface mucus layer, medusa, microbiome
Citation: Carabantes N, Cerqueda-García D, García-Maldonado JQ and Thomé PE (2022) Changes in the Bacterial Community Associated With Experimental Symbiont Loss in the Mucus Layer of Cassiopea xamachana Jellyfish. Front. Mar. Sci. 9:879184. doi: 10.3389/fmars.2022.879184
Received: 18 February 2022; Accepted: 22 April 2022;
Published: 19 May 2022.
Edited by:
Xavier Pochon, The University of Auckland, New ZealandReviewed by:
Nicola Gabriele Kriefall, Boston University, United StatesEmily Aguirre, University of Southern California, United States
Copyright © 2022 Carabantes, Cerqueda-García, García-Maldonado and Thomé. This is an open-access article distributed under the terms of the Creative Commons Attribution License (CC BY). The use, distribution or reproduction in other forums is permitted, provided the original author(s) and the copyright owner(s) are credited and that the original publication in this journal is cited, in accordance with accepted academic practice. No use, distribution or reproduction is permitted which does not comply with these terms.
*Correspondence: Patricia E. Thomé, thome@cmarl.unam.mx