The evolution of predator avoidance in cephalopods: A case of brain over brawn?
- 1Centre for Ecology and Conservation, University of Exeter Penryn Campus, Penryn, United Kingdom
- 2Baltic Sea Centre, Stockholm University, Stockholm, Sweden
- 3Department of Biology, Centre for Animal Movement Research (CAnMove), Lund University, Lund, Sweden
- 4Department of Zoology, Stockholm University, Stockholm, Sweden
- 5Animal Physiology and Biochemistry, Zoological Institute and Museum, University of Greifswald, Greifswald, Germany
Predation is a major evolutionary driver of animal adaptation. However, understanding of anti-predator evolution is biased toward vertebrate taxa. Cephalopoda, a class in the invertebrate phylum Mollusca, are known for their diverse anti-predator strategies, characterised by their behavioural flexibility. While ancestral cephalopods were protected by a hard outer shell, extant cephalopods have greatly reduced their reliance on physical defences. Instead, cephalopods have evolved highly developed senses to identify potential threats, cryptic skin patterns to avoid detection, startle responses to deter attack, and elaborate means of escape. While cephalopod anti-predator repertoires are relatively well described, their evolution, and the selective pressures that shaped them, have received much less attention. This is despite their potential relevance, in turn, to elucidate evolution of the remarkable cognitive abilities of cephalopods. Here, we review cephalopod anti-predator evolution, considering four key aspects: (i) shell reduction and loss; (ii) the skin patterning system; (iii) the ecological context accompanying the evolution of advanced cognit.ive abilities; (iv) why the evolutionary trajectory taken by cephalopods is so unique among invertebrates. In doing so, we consider the unique physiology of cephalopods and discuss how this may have constrained or aided the development of their anti-predator repertoire. In particular, cephalopods are poorly equipped to defend themselves physically and escape predation by fish, due to a lack of comparable weaponry or musculature. We argue that this may have selected for alternative forms of defence, driving an evolutionary trajectory favouring crypsis and complex behaviours, and the promotion of sensory and cognitive adaptations. Unravelling the complexities of cephalopod anti-predator evolution remains challenging. However, recent technological developments available for cephalopod field and laboratory studies, coupled with new genomic data and analysis approaches, offer great scope to generate novel insights.
1 Introduction
Excluding a small number of apex predators, individuals of most species face a constant threat of predation. Consequently, predation and the acquisition of defences to avoid it, are a major driver of evolution (Vermeij, 1978; Dawkins and Krebs, 1979; Langerhans, 2007). The range of anti-predatory adaptations that have arisen during animal evolution are extremely diverse and varied (Edmunds, 1974; Vannier et al., 2006). These range from relatively simple defences, such as the use of protective shells and refuges, to the evolution of complex anti-predator warning systems in group-living mammals (Palmer, 1979; Bednekoff, 2015). While the use of complex behavioural strategies to protect against predation has received much attention among vertebrates, invertebrate taxa are less well studied. Vertebrates share much evolutionary history and many underlying biological traits that separate them from other animal taxa. Thus, to improve understanding of predator avoidance among animals more generally, it is necessary to expand consideration of invertebrate lineages. A particularly interesting invertebrate group to examine anti-predator strategies is Cephalopoda, given evolutionary transitions from shelled lineages towards shell reduction and loss, with accompanying investment into larger brains, more advanced neurological systems, and the expression of complex behaviours.
Cephalopoda is an ecologically diverse class of molluscs containing ∼1,000 described species, which occupy varied marine habitats from intertidal rockpools to abyssal thermal vents (Roper et al., 1984; Horton et al., 2019). Cephalopods are divided into two subclasses, Nautiloidea and Coleoidea (Figure 1). Nautiloidea thrived during the early Palaeozoic, but most taxa in the subclass are now extinct, and today it contains just six extant species in the genera Nautilus and Allonautilus. Thus, the subclass Coleoidea contains the vast majority of extant cephalopod species. Coleoidea is divided into two superorders, Octopodiformes, including octopuses and vampire squid, and Decapodiformes, including squid and cuttlefish. Considerable phylogenetic uncertainty surrounds relationships among orders in the Decapodiformes (Sanchez et al., 2018; Anderson and Lindgren, 2021). In particular, use of the term ‘squid’ is taxonomically problematic, since the superorder Decapodiformes contains cuttlefish (Sepiida), but the remaining orders are not consistently recovered as a monophyletic group (Anderson and Lindgren, 2021) (Figure 1).
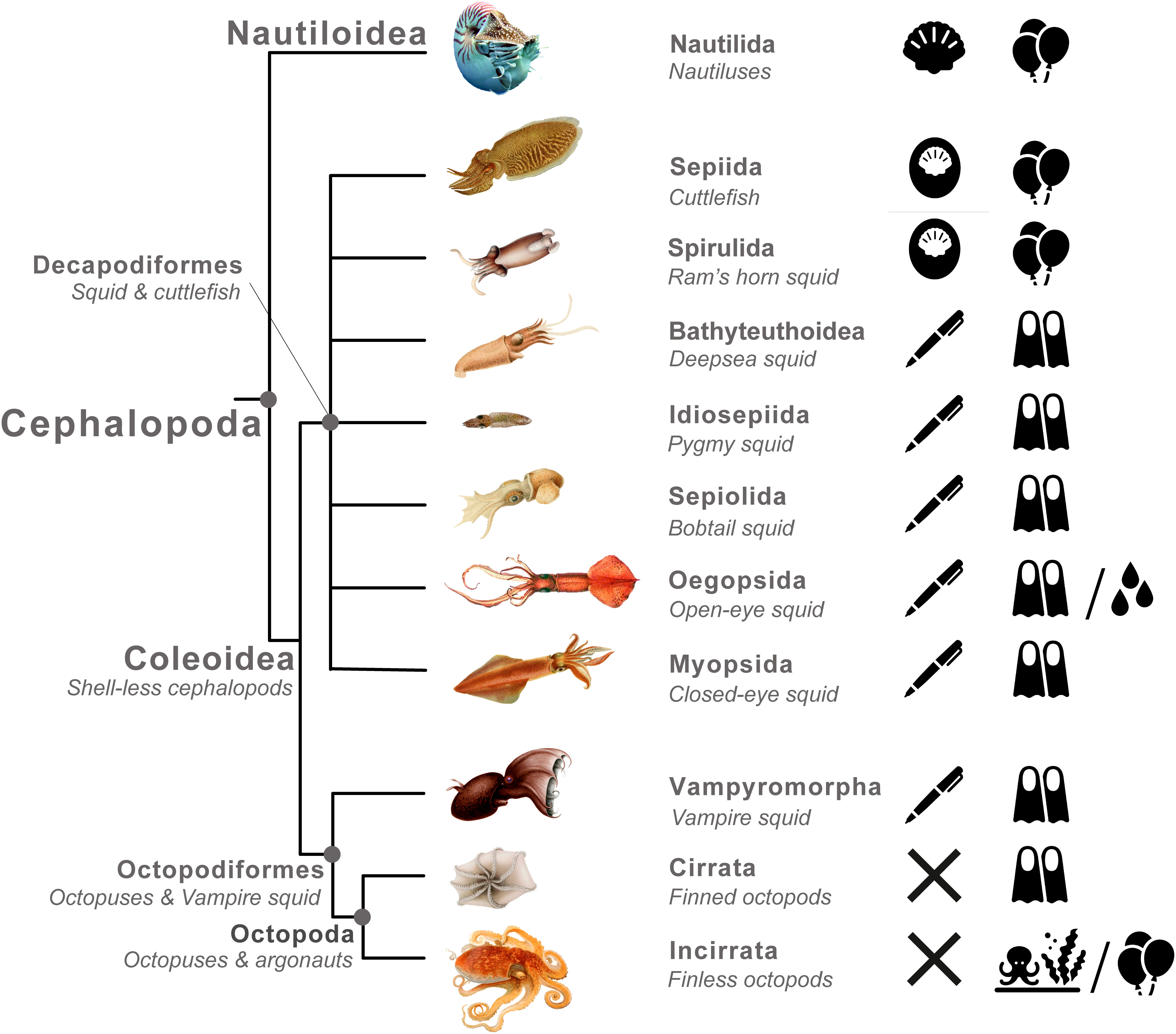
Figure 1 Phylogenetic tree illustrated with shell structures and buoyancy mechanisms exhibited by cephalopods. The shell is an important character in the evolution of anti-predator strategies in Cephalopoda. There has been a transition from a protective outer structure to a buoyancy device, or loss in some groups, corresponding with major transitions in lifestyle and the way that different cephalopod lineages interact with predators. Nautiluses, cuttlefish and ram’s horn squid possess a shell containing gas filled chambers used to modify buoyancy, that is either: external in nautiluses, or internal in cuttlefish and ram’s horn squid; Decapod squid and the vampire squid possess a greatly reduced shell called a pen (or ‘gladius’) and use either: (i) low density fluid or (ii) hydrodynamic lift generated by finning to modulate buoyancy; Octopuses and bobtail squid have completely lost the ancestral cephalopod shell and have either: (iii) adapted to a benthic lifestyle, (iv) evolved to use a swim bladder (in the ‘football octopus’), or (v) evolved use of the egg case to generate buoyancy (in ‘paper nautiluses’). (Illustrations from Jatta, 1896; Chun, 1910 & Meyer, 1913). Icons on the right of the figure represent the following: black shell – presence of an external shell; white shell within black oval – presence of a reduced internal shell; pen – presence of a pen or gladius; cross – complete absence of a shell; balloons – neutral buoyancy achieved via gas filled chambers or spaces; fins– neutral achieved via hydrodynamic lift; water droplets – neutral buoyancy achieved via low density fluid filled chambers; benthic octopus – evolved a benthic lifestyle. Cephalopod illustration credits are as follows: Nautilus, Lee R Berger, CC BY-SA 3.0, https://en.wikipedia.org/wiki/File:Nautilus_profile.jpg; Ocythoe tuberculata, Eledone aldrovandi, Argonauta argo, Jatta, 1896; Rossia mastigophora, Mastigoteuthis glaukopis, Vampyroteuthis infernalis, Cirruthauma murrayi, Spirula spirula, Chun, 1910; Sepia officianalis, Loligo vulgaris, Meyer, 1913.
All cephalopods share a distinctive bauplan consisting of a mantle, which comprises a muscular body wall that encloses the visceral mass, and a ring of tentacles surrounding the mouth. Yet, cephalopods show great variability in size and in morphology, ranging from ~8mm to at least 10m in length, and from shelled nautiluses with their numerous cirri to completely shell-less eight-armed octopuses (Hanlon and Messenger, 2018). As with other bilaterian animals, cephalopods share certain fundamental underlying physiological traits with vertebrates (Willmer et al., 2009; Carroll et al., 2013). However, given that cephalopods diverged from other molluscs ~530 million years ago (Kröger et al., 2011), the group represents a deeply divergent lineage, that is distantly separated from even their closest living molluscan relatives. Since their ancient divergence from other molluscs, cephalopods have evolved highly complex nervous systems comparable to those of vertebrates, from an ancestral state where the ‘brain’ consisted of a simple ring of paired ganglia (Shigeno et al., 2018). This semi-independent evolution of sophisticated brains and eyes and accompanying behavioural complexity provides an evolutionary contrast to the vertebrates, and adds significant interest to the evolution of anti-predator behaviour in cephalopods.
From a predator’s perspective, cephalopods can be considered a high reward food source, being rich in proteins, minerals, and omega-3 fatty acids (Mouritsen and Styrbæk, 2018). Additionally, excluding nautiluses, cephalopods are relatively easily to process as a prey item by predators, given the lack of a hard external carapace or the shell that serves to protect their similarly nutritious bivalve and gastropod relatives. Cephalopods also lack substantial physical weaponry, such as hard and sharp teeth, fangs, or claws. Instead, cephalopods depend heavily on their highly sophisticated brains and sensory systems to avoid predation, possessing a level of behavioural complexity otherwise unseen outside of vertebrates (Young, 1988; Grasso and Basil, 2009; Bush, 2012). Recent reviews have examined many aspects of cephalopod biology including anatomy, behaviour and cognition (Derby, 2007; Marini et al., 2016; Mather and Dickel, 2017; Villanueva et al., 2017; O’Brien et al., 2018; Schnell and Clayton, 2019; Schnell et al., 2020). However, relatively limited attention has been given to the evolution of anti-predator adaptations among cephalopods. Here, we explore the evolution of anti-predator adaptations and strategies in cephalopods. The primary strategy of defence in the major lineage of extant cephalopods, the coleoids, is a reliance on crypsis and vigilance, followed by behaviourally complex secondary defences if the cephalopod is detected (Hanlon and Messenger, 2018). A hallmark of these anti-predatory strategies is their dependence upon highly developed nervous systems and cognitive abilities. Consequently, we focus on four fundamental outstanding questions regarding the evolution of advanced cognitive abilities in cephalopods and their significance for predator avoidance:
1. Was shell loss linked to cognitive evolution in cephalopods, and if so, how?
2. Did evolution of the cephalopod skin patterning system facilitate further cognitive advances?
3. What was the ecological context leading to the evolution of advanced cognitive abilities in cephalopods?
4. Given that other invertebrate groups have not undergone similar cognitive evolutionary trajectories, what is special about cephalopods?
Ecological pressure arising from the diversification of bony fish (teleosts) is postulated to have provided an important context for the evolution of cognitive advances in modern cephalopods (Packard, 1972; House et al., 1988). Thus, we divide question three further, to provide a detailed examination of competing hypotheses regarding the role of bony fish in cephalopod anti-predator evolution. Specifically, we ask if adaptations during this period enabled cephalopods to: (i) compete more effectively for prey items with fish; (ii) avoid predation by fish; (iii) predate upon fish; or, (iv) potentially all three of these ecological pressures acting in concert.
We discuss each of the above questions in turn below, and end by considering the future outlook for research into cephalopod anti-predator biology.
2 Was shell loss linked to cognitive evolution in cephalopods, and if so, how?
Tough external body coverings such as shells and exoskeletons are a basic form of passive, physical defence against predation (Palmer, 1979). Consequently, an obvious question is why was the protective shell lost in most extant cephalopod lineages? The earliest known fossil cephalopods had shells, as did their most closely related mollusc relatives, and the resemblance between cephalopod and shelled gastropod embryos also suggests that ancestral cephalopods had shells (Kröger et al., 2011). Yet, only the six extant species of nautiloids retain an external shell. No cephalopod shells are known to show the complex spines or nodes present on many gastropod shells, which serve to deter, gape-limit, and generally resist predation (Palmer, 1979; Bertness and Cunningham, 1981; Lowell et al., 1994). Instead, coleoid cephalopods have greatly reduced their reliance on a shell, with three main variants existing today: the cuttlefish cuttlebone, the squid pen, and complete shell loss in octopuses (Figure 1; Box 1). Without the protection of a hard outer shell, cephalopods are physically vulnerable to predation. Consequently, key questions in exploring the evolution of anti-predator avoidance in cephalopods are when, why, and how did they evolve shell reduction and internalisation?
Box 1 Variants of the shell in modern coleoids.
Among modern coleoids, the main shell conditions are possession of a cuttlebone, squid pen, or loss of the shell altogether. The cuttlebone is an internalised shell present in all cuttlefish and is primarily composed of the crystal form of calcium carbonate, aragonite. It retains a buoyancy control function and possesses both the ancestral phragmocone and a highly modified siphuncle on the ventral surface (Packard, 1972). Hydrostatic pressure acting on the gas filled chambers of the cuttlebone limits the depth to which cuttlefish can descend to <200m (Sherrard, 2000). Ram’s horn squid (Spirula spirula) also possess an internal open coiled mineralized shell that acts to control buoyancy in a similar manner (Lindsay et al., 2020).
The squid pen is an internalised leaf-like vestige of the former shell and is composed primarily of chitin. The pen is present in many species within the superorder Decapodiformes, and a single species in the superorder Octopodiformes (the vampire squid, Vampyroteuthis infernalis) (Bizikov, 2004; Clements et al., 2017). In most species the pen no longer serves as a flotation device, instead providing muscle attachment points and support for the mantle (Young et al., 1998). Consequently, cephalopods with a pen must either fin or jet to generate hydrodynamic lift (Packard, 1972). Certain bathypelagic squid (e.g. Cranchiidae: glass squid) have specialised ammonia filled tissues, which, since ammonia is less dense than water, counteract their bodyweight (Clarke et al., 1979).
The remaining extant coleoids, composed primarily of octopuses and sepiolids (bobtail squid), lack a shell and possess no buoyancy control device, apart from football octopuses (Ocythoe tuberculata), and argonauts (Argonauta spp.) which are pelagic octopuses also known as paper nautiluses. Like fish, football octopuses possess a gas-filled swim bladder (Packard and Wurtz, 1994). Meanwhile, female argonauts secrete a thin shell-like egg case composed of calcite (the crystal form of calcium carbonate) from greatly expanded dorsal tentacle tips, which functions as a brood chamber and floatation device (Packard & Wurtz, 1994; Finn and Norman, 2010). Apertures at the top of the egg case trap an air pocket at the ocean surface, allowing the argonaut to descend to a depth where the compressed gas provides neutral buoyancy, conserving energy otherwise required to maintain a constant depth (Finn and Norman, 2010). While fragile and not a true cephalopod shell, the argonaut egg case facilitates descent to a safe distance below the water’s surface to avoid detection and shallow dives by avian predators (Finn and Norman, 2010).
Cephalopods are considered to have evolved from monoplacophoran molluscs, similar to the fossil Knightoconus antarcticus from the Antarctic Cambrian Minaret Formation (Yochelson et al., 1973; Webers and Yochelson, 1989). The earliest unambiguous cephalopod fossil is the Cambrian nautiloid Plectronoceras cambria, from the lower Fengshan Formation, North China (Walcott, 1905; Hildenbrand et al., 2021). P. cambria was a very small cephalopod with an external horn-shaped shell (Fang et al., 2019), which shares greater similarity with the shells of gastropod and monoplacophoran-like molluscs than with modern cephalopods. In line with this, the proposed ancestral state for cephalopods is a benthic lifestyle (Kröger et al., 2011). However, in contrast to other molluscs and in common with extant nautiloids, early cephalopods possessed a phragmocone; a shell structure composed of a series of simple partitions (septa) separating hollow chambers (camerae) (Kröger et al., 2011). A thin tube of body tissue (the siphuncle) extends through the partitions of the phragmocone, allowing gas to diffuse into the chambers for buoyancy adjustment via osmotic control.
It is debated whether P. cambria could regulate its buoyancy. Some authors suggest the chambers of the shell of P. cambria contained liquid (Webers and Yochelson, 1989), while others argue for nautilus-type buoyancy regulation based on shell morphology (Kröger et al., 2011). Nevertheless, it is generally agreed that buoyancy almost immediately became a feature of cephalopod biology, and was present in younger species of Plectronoceras and other Cambrian cephalopods (Webers and Yochelson, 1989; Kröger et al., 2011). This adaptation of the phragmocone into a buoyancy device greatly aided energy-efficient mobility and ultimately enabled cephalopods to ascend from the sea floor, opening up life within the water column (Kröger et al., 2011). Thus, while the cephalopod shell most likely fulfilled an important defensive role during the very early stages of cephalopod evolution, a key difference between cephalopod shells compared to other mollusc shells is their function as a flotation device.
Fossil evidence suggests that cephalopod shell morphology diversified rapidly after P. cambria, resulting in a radiation of small cephalopod species with tall curved shells (Landing and Kröger, 2009). Subsequently, in the early Ordovician, cephalopod shell shape evolved substantially, and long straight-shelled orthocerids and coiled-shelled nautiloids appeared (Kröger et al., 2011). Later, during the Devonian, ammonites independently evolved coiled shells (Kröger, 2005). It is unclear whether coiling enabled the shell to withstand higher pressures, such as those applied by predators, or those exerted hydrostatically under greater depths, or if it provided greater buoyancy control and mobility (Lewy, 2002; Kröger, 2005; Pérez-Claros et al., 2007). In contrast, belemnites possessed elongated linear shells that had an inorganic lining which increased their strength, enabling them to exploit deep ocean habitats (Doguzhaeva et al., 2014), which probably contained lower predator densities. By the Early Carboniferous, stem group coleoids had internalized their shells (Kröger et al., 2011).
Fossil evidence concerning early coleoid evolution is sparse, but the available record suggests that the transition from an external to an internal shell led to an adaptive radiation in cephalopods during the later Palaeozoic (Young et al., 1998; Kröger et al., 2011). Recent molecular clock-based estimates have corroborated this hypothesis, implying that crown group coleoids emerged during the final stages of the Palaeozoic, followed by diversification during the ‘Mesozoic Marine Revolution’ in the Jurassic and Cretaceous (Tanner et al., 2017). Thus, there are postulated to have been three main ecological phases of cephalopod shell evolution: 1. Molluscan-like shells accompanying a benthic lifestyle; 2. Adaptation of the shell into a buoyancy device, resulting in free-swimming forms escaping the sea floor; 3. Internalization and reduction of the shell leading to expansion into alternative ecological niches among coleoid cephalopods (Kröger et al., 2011; Tanner et al., 2017) (Figure 2).
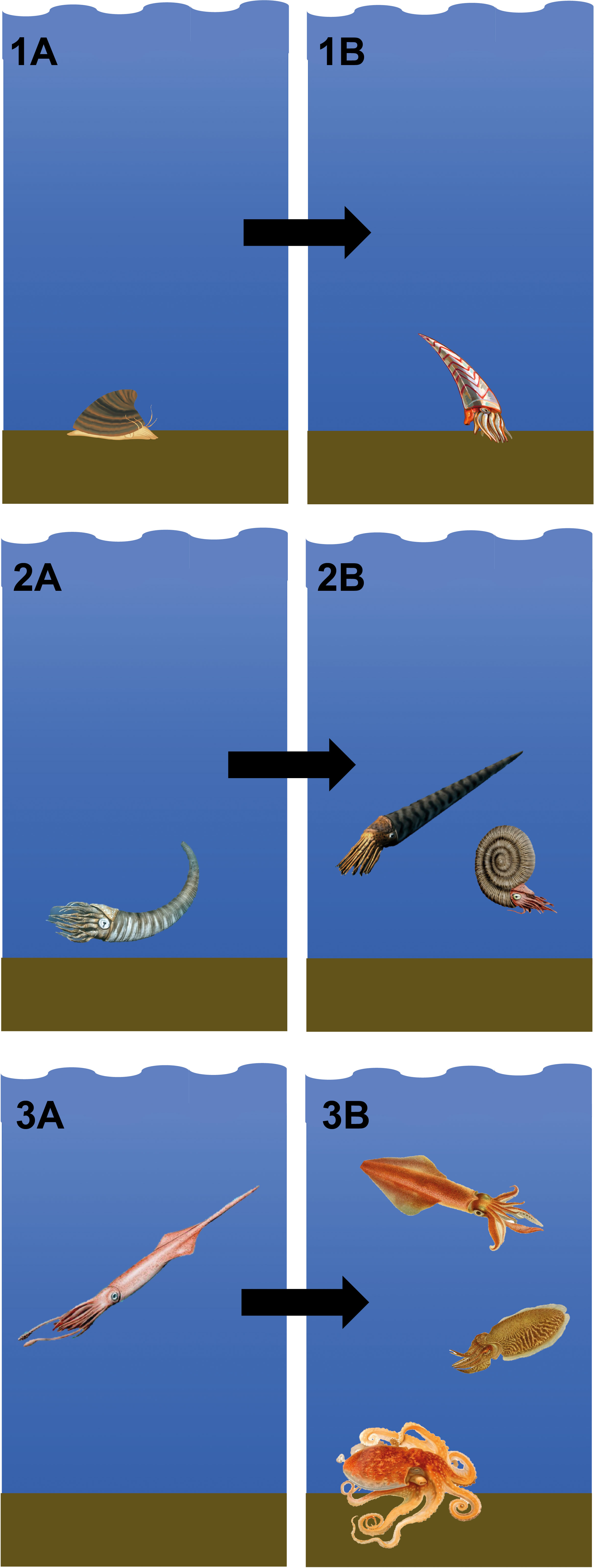
Figure 2 Transition from ancestral benthic shelled molluscs to modern cephalopods with a reduced and internalised or absent shell: (1A). The ancestor of cephalopods is generally considered to be a monoplacophoran mollusc, similar to fossil Knightoconus antarcticus from the Anarctic Cambrian Minaret Formation (image shows a reconstruction of Knightoconus antarcticus, image credit: HannahMoss, CC BY-SA 4.0); (1B). Subsequently, the first cephalopods appeared, with the earliest currently accepted cephalopod fossil being Plectronoceras cambria (image shows a reconstruction of Plectronoceras cambria, image credit: Entelognathus, CC BY-SA 4.0); (2A). From the Late Cambrian, cephalopods had achieved buoyancy and could ascend from the sea floor, coinciding with a radiation of small cephalopod species with tall, curved shells (image shows a reconstruction of Cyrtoceras, image credit: Nobu Tamura, CC BY-NC-ND 3.0); (2B). From the Early Ordocivian, shell morphology diverged considerably, with the emergence of long straight shells in orthocerids, and coiled shells, firstly in nautiloids and later in ammonoids (image shows a reconstruction of Orthoceras regulare, a middle Ordovician orthocerid, and Dactylioceras commune, an early Jurassic ammonoid, image credit: Nobu Tamura, CC BY-NC-ND 3.0); (3A). From the Early Carboniferous, stem group coleoids internalized their shells (image shows a reconstruction of Youngibelus tubularis, a Jurassic belemnite, image credit: Nobu Tamura, CC BY-NC-ND 3.0); (3B). The majority of modern coleoids originated during the Jurassic and Cretaceous, after which the shell was further reduced or lost entirely (image credit: see Figure 1).
It is unclear if coleoids lost their shells before or after evolving their advanced neurological and behavioural complexity. Loss of an external shell may have strongly selected for cognitive evolution in cephalopods, providing a need to cope with challenging novel environments because of dramatically increased predation pressure, while simultaneously allowing colonisation of a wider variety of ecological niches (Amodio et al., 2018). However, a ‘post-adaptive’ hypothesis for cognitive advancement has faced criticism, since it implies that cephalopods were left relatively defenceless before evolution of alternative strategies of predator avoidance (Mollo et al., 2019). The alternative ‘pre-adaptive’ hypothesis for shell loss postulates the evolution of advanced sensory, motor, and cognitive abilities in cephalopods prior to shell loss (Mollo et al., 2019), in turn reducing the need for armoury. A potential defence of the post-adaptive hypothesis invokes internalisation of the shell while cephalopods occupied deep offshore waters, where predation pressure is considerably lower than in shallow waters, with jet-propulsion (which evolved prior to shell loss) providing a means of escape in higher predation habitats (Amodio et al., 2019).
3 Did evolution of the cephalopod skin patterning system facilitate further cognitive advances?
One of the most remarkable features of cephalopod biology is an ability to modify skin colouration and patterning rapidly, dynamically, and at very fine scale (Box 2). In modern coleoids, skin patterning is used: (i) for camouflage to ambush prey and hide from predators, (ii) in startle displays to confuse or scare off predators (i.e. deimatic displays, see section 3.3.1 below), and, (iii) as a means of communication (Moynihan and Rodaniche, 1982; Hanlon and Messenger, 1988; Palmer et al., 2006). Additionally, octopuses and cuttlefish can also change their skin texture from smooth to ridged or spiky to further modify their appearance, using circular muscles just under the skin’s surface (Panetta et al., 2017). Below we review aspects of skin patterning used by cephalopods for crypsis, which forms the primary anti-predatory strategy utilised by the group (Mcfall-Ngai, 1990; Hanlon et al., 1999; Staudinger et al., 2011; Amodio et al., 2018).
Box 2 Cephalopod skin patterning.
The complex system of skin patterning in cephalopods is produced primarily by three layers of cells at the surface of the skin (Osorio, 2014). The layer closest to the surface is composed of pigment containing sacks called chromatophores, that can expand and contract under muscular control. Each chromatophore contains either red, yellow, or brown pigment, and coordination of the system can alter the overall colour and patterns present on the skin extremely rapidly. When the chromatophores are retracted, the pigment containing area is reduced to the extent that the tissue becomes translucent, allowing light to pass through the animal (Mäthger and Hanlon, 2012). Below the chromatophores are a layer of iridophores, which are pigment containing cells that refract light to enable iridescent displays, or reflect light to match complex backgrounds (Messenger, 2009). The third layer is composed of a further type of diffuse white pigment containing cells called leucophores, which are used to control the brightness and contrast of the display (these are generally not found in squid, with the exception of several Sepioteuthis spp.) (Mäthger et al., 2009).
Surprisingly given their remarkable colour changing capabilities, cephalopods are generally thought to be colour blind, due to only possessing a single visual pigment (the firefly squid (Watasenia scintillans) is the only cephalopod known to possess more than a single visual pigment (it has three) (Messenger, 1977; Seidou et al., 1990; Marshall and Messenger, 1996; Kröger and Gislén, 2004). This is not necessarily a problem for startle displays, provided that the display causes the predator to recoil. However, an inability to detect colour presents challenges for achieving camouflage by background matching (Messenger, 2009). One apparent solution is that cephalopods use their iridophores to reflect incoming light to match their surroundings. Relaxing circular muscles around the chromatophore reduces the area of pigmentation and reveals the reflective cells underneath. These cells reflect ambient light enabling cephalopods to display colours on their skin.
Another explanation is that cephalopods may use light intensity rather than wavelength to estimate background colour. Experimental evidence suggests that cuttlefish are capable of detecting differences in the intensity of light emitted from different parts of a substrate and using this information to produce an adaptive cryptic display (Marshall and Messenger, 1996). It has also been proposed that cephalopods may see colour using a phenomenon known as chromatic aberration (Gagnon et al., 2016; Stubbs and Stubbs, 2016a, b). Cephalopod pupils are irregularly shaped, allowing light to enter the eye in several places. This results in a slight loss of visual acuity, but may enable the distinction of different wavelengths of light (Stubbs and Stubbs, 2016b).
Finally, there is some evidence that cephalopod skin possesses photoreceptors which are sensitive to different wavelengths of light. Chromatophores from the two-spot octopus (Octopus bimaculoides) have been shown to expand in response to light in a phenomenon termed ‘light-activated chromatophore expansion’ (LACE) and was fastest in response to blue light (Desmond Ramirez and Oakley, 2015). By combining some or all of these mechanisms, cephalopods may be capable of detecting colour in their surroundings to some extent, despite possessing only a single visual pigment.
Crypsis in cephalopods is well-studied and is particularly prevalent in shallow water species (Marshall and Messenger, 1996; Zylinski et al., 2009; Buresch et al., 2011; Staudinger et al., 2011; Murali et al., 2019). Perhaps the most familiar means of avoiding detection in cephalopods is matching the surroundings. One of the best studied species displaying the incredible flexibility of cephalopod background matching is the common octopus (Octopus vulgaris), which uses papillate textures and mottle patterns to imitate a wide variety of substrates, rocks, corals and other objects (Hanlon et al., 2009).
Even where an animal’s colouration provides a near-perfect match to a background, an outline may persist that can give-away a hiding individual. To counteract this, many animals adopt disruptive patterning to break up outlines and distinctive features by including bold patterns at the body periphery (Stevens and Merilaita, 2009). Octopuses have an advantage in this regard due their highly flexible body, meaning their ‘typical outline’ is more varied than many other animals. The common octopus takes this even further and has been observed to pick up rocks and lumps of coral with their arms to further break up their outline (Norman et al., 2001). In contrast, common and pharaoh cuttlefishes (Sepia officinalis, Sepia pharaonis) are known to display bold square patterns which act as disruptive components on pebbly substrates. The common cuttlefish has a particularly noteworthy display on sandy substrates with sparsely dispersed black and white pebbles, featuring a mottle pattern over most of its body and a large white square in the middle of its back (Hanlon et al., 2009).
Some cephalopods make use of their flexible bodies to further enhance their camouflage. The Caribbean reef squid (Sepioteuthis sepioidea) is frequently observed to hide amongst seaweeds whilst displaying a ‘plaid’ pattern of stripes and bars, orientating its body to be parallel with the direction of the seaweed growth and waving its arms in the water current (Moynihan and Rodaniche, 1982). This alignment of body parts with background stimuli has also been observed in the two-toned pygmy squid (Idiosepius pygmaeus) and experimentally tested in the European common cuttlefish (Sepia officinalis), which preferentially holds its arms parallel with stripes of varying orientations (Moynihan, 1983; Barbosa et al., 2012).
For many organisms, especially those inhabiting three dimensional environments, countershading is a common basic form of crypsis (Ferguson and Messenger, 1991; Cuthill et al., 2016). By darkening the dorsal surface, and lightening the ventral surface, animals can negate a dark outline against the sky from below, and a light outline against the sea or seafloor from above (Ferguson and Messenger, 1991). Through the capacity for fine-scale neurally controlled polymorphism over their body surface, many cephalopod species are able to take this a step further, and alter their countershading according to their orientation in the water column (Ferguson and Messenger, 1991; Ferguson et al., 1994). Thus, rather than their dorsal side always appearing darker, they can ensure that the surface of their body closest to the water surface is darkest. Another particularly noteworthy example of shadow concealment is seen in the Hawaiian bobtail squid (Euprymna scolopes), which light up their ventral surface via a mutualism with an endosymbiotic bioluminescent bacterium, Vibrio fischeri, that creates counter-illumination, which has an analogous effect to countershading (Jones and Nishiguchi, 2004).
It is worth noting that adaptations which disguise an animal from potential predators can also serve to disguise it from potential prey (Pembury Smith and Ruxton, 2020). This is undoubtedly true in cephalopods, which make use of their abilities to blend in with their surroundings to ambush prey (Moynihan and Rodaniche, 1982; Roper and Hochberg, 1988). From an anti-predator perspective, the ability to blend in with their surroundings was presumably of key value to cephalopods once they had begun to internalise their shells. The internalisation of the shell meant that cephalopods were not only more vulnerable to attack physically, but also that their entire body surface was now available as a substrate for crypsis. Further, the dynamic nature of cephalopod skin pigmentation meant that this crypsis could be highly plastic, offering the potential to hide from predators across varied backgrounds. While much is known about the components of cephalopod skin patterning and how they function individually (Gilmore et al., 2016), remarkably little is known about how and when it evolved. Extant nautiloids do not possess the ability to change their skin patterning, and presumably the ability evolved subsequently to the internalisation of the shell, but beyond this the situation is unclear.
An improved knowledge of the evolution of cephalopod skin patterning has implications for our understanding of cephalopod cognitive evolution, and its involvement in anti-predator strategies. The different mechanisms used to control the various components of cephalopod skin patterning systems necessitate the integration of different forms of visual information (Gilmore et al., 2016; Hanlon and Messenger, 2018). This requirement to process complex information regarding the appearance of their surroundings and to coordinate this with an appropriate cryptic response is neurologically costly (Zylinski and Osorio, 2011), and presumably strongly selected for improvements in visual and cognitive systems (if improved abilities increased fitness). In song birds, it has been suggested that the complexity of song repertoire is linked to cognitive evolution (Boogert et al., 2008; Searcy and Nowicki, 2019; Williams and Lachlan, 2022). Similarly, it is possible that the evolution of the complex cephalopod skin patterning system was linked to the evolution of larger and more sophisticated brains, able to cope with the challenges of deploying crypsis and other anti-predatory behaviours effectively (as well as various forms of interspecies communicative displays).
Additionally, the switch from a reliance on external mechanical defences towards advanced forms of crypsis accompanied by vigilance, is likely to have selected for further cognitive advances. Specifically, a strategy of crypsis and vigilance promote ‘wait-and-see’ assessments of predators (Moynihan and Rodaniche, 1982; Hanlon and Messenger, 2018). Under such a strategy, more accurate assessments of the type of predator and level of threat it poses, coupled with selection of the most appropriate response, would lead to higher survival. Consequently, evolutionary pressure to enhance anti-predatory judgements made while employing crypsis and vigilance may have contributed to the evolution of cognitive development in Cephalopods, potentially giving rise to an evolutionary arms race with the predators seeking to detect and consume them.
4 What was the ecological context leading to the evolution of advanced cognitive abilities in cephalopods?
Modern cephalopods face a great diversity of predators (Box 3), partially reflecting the wide variety of habitats that they inhabit. Of these, fish are considered the most important, and are hypothesized to have played the greatest role in shaping cephalopod evolution, during two main phases (Randall, 1967; Clarke and Stevens, 1974; Staudinger et al., 2013; Hanlon and Messenger, 2018): (i) Firstly, during the Devonian ‘age of fish’, which saw a considerable increase in fish diversity, following the rise of nekton (actively swimming organisms, as opposed to those carried passively by ocean currents) (Near et al., 2012); (ii) Secondly, during the Jurassic/Cretaceous ‘Mesozoic Marine Revolution’ (Vermeij, 1977), concomitant with the radiation of the majority of modern bony fish groups (Near et al., 2012; Tanner et al., 2017). The first period is considered to have coincided with a major shift in predator-prey dynamics in the world’s oceans, which saw an increase in higher-metabolism predation and the predation of hard-shelled organisms, and coincided with the appearance of the cephalopod beak (Tanner et al., 2017). Meanwhile, the second period accompanied further reduction of the cephalopod shell and the emergence of the majority of modern coleoid lineages (Tanner et al., 2017).
Box 3 Modern predators of cephalopods.
Sea birds predate cephalopods using their keen eyesight, including king penguins (Aptenodytes forsten) which can hunt squid at depths of 250m, and albatrosses which forage for squid at the ocean surface (Imber, 1973; Berruti and Harcus, 1978; Kooyman et al., 1982). Many mammals frequently predate cephalopods using diverse strategies. The sperm whale (Physeter macrocephalus) is a voracious hunter of squid, and is estimated to catch 150 million tonnes per year; nearly three times the catch of human fisheries (Clarke, 1977; Clarke, 1983; Clarke, 1996). Both pinnipeds and mustelids regularly predate cephalopods. For example, elephant seals (Mirounga sp.) regularly dive below 1,000m to catch octopuses, cuttlefish, and squid, while sea otters (Enhydra lutris) search out octopuses within their dens (McCleneghan and Ames, 1976). Interspecies predation and intraspecies cannibalism are both reportedly widespread in cephalopods (Boyle, 1983). Sharks, rays and skates use olfactory and electrical cues to prey on cephalopods in many habitats with some species, such as the sicklefin weasel shark (Hemigaleus microstoma) specialising on them over other prey items (Clarke and Stevens, 1974; Stevens and Cuthbert, 1983; Smale, 1996). However, it is the great diversity of bony fish, with which cephalopods share many of their habitats, which are considered to be the greatest predators of cephalopods (Randall, 1967; Smale, 1996; Staudinger et al., 2013; Hanlon and Messenger, 2018). Cephalopods form part of the diet of most carnivorous reef fish from eels flushing octopuses out crevices to barracuda and jacks hunting reef squid (MacGinitie and MacGinitie, 1968; Moynihan and Rodaniche, 1982). Decapitated heads of pelagic squid species have been found in the stomachs of swordfish (Xiphias gladius) from which it has been inferred that cephalopods form a large part of their diet (Scott and Tibbo, 1968; Bello, 1991; Bower and Ichii, 2005). Pelagic squid and argonauts are hunted by many scombrid and gadid fish, with tuna in particular preying on a wide range of species (Royer et al., 1998; Staudinger et al., 2013). One study found that cephalopods made up more than 50% of the diet by mass of adult Cape dory (Zeus capensis) (Meyer and Smale, 1991). Groupers such as dusky groupers (Epinephelus marginatus) hunt cuttlefish, octopuses and squid across their range (Smale, 1996).
While it is generally agreed that the powerful jaws, keen eyesight, and muscular bodies of fish presented strong selective pressures on cephalopod evolution (Packard, 1972; Klug et al., 2010; Bush and Bambach, 2011; Tanner et al., 2017), it is not obvious what form of selection exerted the dominant effect. Major cognitive advances and the complex behaviours present in modern cephalopods were presumably selected for because they enabled cephalopods to: (i) compete more effectively for prey items with fish; (ii) avoid predation by fish; (iii) predate upon fish; or (iv) potentially all three of these ecological pressures acting in concert. Below we consider anti-predator strategies in cephalopods (Figure 3), examining primary and secondary defences in detail, and we examine how ecological pressure from fish may have acted on their evolution. We argue that constraints on cephalopod physiology limited the potential for flight or physical confrontation to be dominant strategies in cephalopods. Instead, primary defences to avoid detection and secondary responses to startle or confuse predators were selected for, driving the evolution of the complex anti-predator repertoires present in extant coleoids.
4.1 An emphasis on primary defences – not getting seen in the first place
In general, cephalopods rely on primary defences as a strategy to avoid predation. Primary anti-predator defences involve attempting to avoid the attention of predators (Staudinger et al., 2011). This is typically achieved by either using aspects of the environment for concealment (Box 4), or actively attempting to resemble the environment or other organisms within it. Adaptations which help animals to ‘blend in’ with their surroundings, also known as camouflage, can take three main forms (Stevens and Merilaita, 2009): (i) crypsis, whereby animals attempt to avoid being noticed at all, (see section 2 above); (ii) masquerade, whereby animals attempt to resemble other objects in their environment so that they are categorised incorrectly; (iii) motion camouflage, whereby animals attempt to disguise their normal movement patterns (rather than just their bodies), to avoid recognition by a potential predator. Cephalopods have been observed to employ all three strategies (Zylinski et al., 2009; Buresch et al., 2011; Panetta et al., 2017), and camouflage is a major anti-predatory strategy in many cephalopods (Figure 4). While these behaviours are also beneficial for hunting, their frequency across coleoid diversity, and their adoption outside of foraging activities, suggest a dominant role in avoiding predation. Below we provide an overview of masquerade and motion camouflage in cephalopods (we describe crypsis in section 2 above, when discussing the cephalopod skin patterning system), and the use of mimicry to avoid detection by predators.
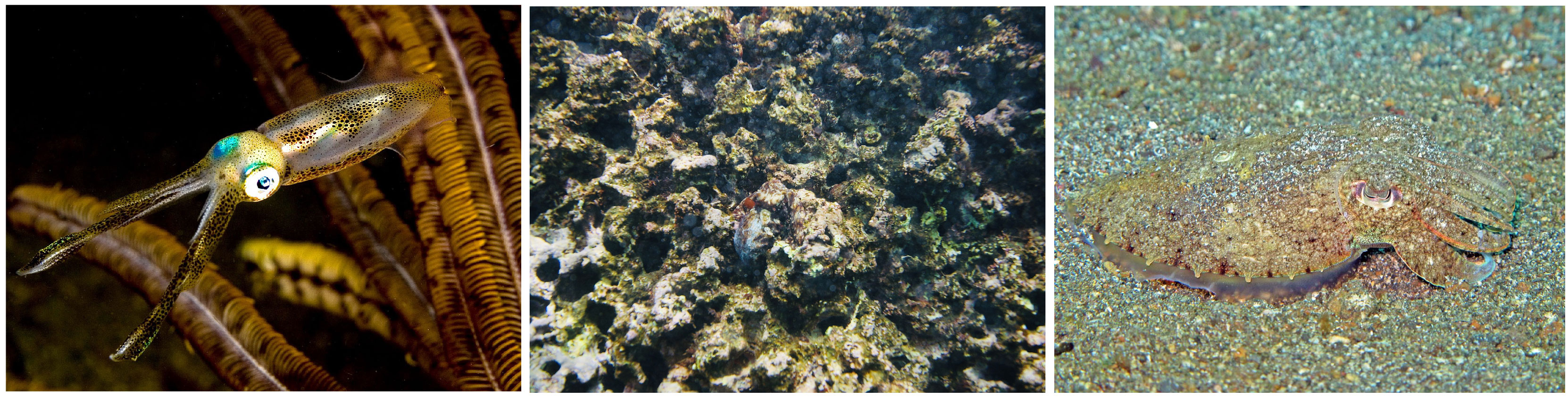
Figure 4 Cephalopod camouflage (L-R) Bigfin reef squid (Sepioteuthis lessoniana) masquerading as seaweed, Octopus sp. background matching against coral, and broadclub cuttlefish (Sepia latimanus) displaying sand-like coloration. Image credits in order are: Nick Hobgood, CC BY SA 3.0, https://commons.wikimedia.org/wiki/File:Sepioteuthis_lessoniana_(Bigfin_reef_squid).jpg; Alana Barthel, CC BY 4.0, https://commons.wikimedia.org/wiki/File:Camouflage_octopus.jpg; Lakshmi Sawitri, CC BY 2.0, https://commons.wikimedia.org/wiki/File:Lembeh81_5-12-11_-_43_cuttlefish_1_looking_like_sand_%286569438067%29.jpg.
Box 4 Use of environmental features for concealment.
Many cephalopods make use of their environment to hide and so avoid being detected by predators. The relative lack of solid internal structures (aside from the beak and eye lenses) in octopuses enables them to squeeze through and into small spaces, helping them to both hide from predators and capture prey (Young et al., 1998). Many octopus species are known to use dens, which they habitually return to, in some cases for more than five months (Ambrose, 1982; Ambrose, 1983; Hartwick et al., 1984). The den can take many forms, from ‘wells’ dug in the sand, to carefully constructed structures of coral rubble or even discarded cans or other anthropogenic waste, and are often marked by middens of discarded shells (Ambrose, 1983; Katsanevakis and Verriopoulos, 2004). Certain octopus species plug the entrance of their den with a rock or lump of coral once inside (Woods, 1965). The mimic octopus (Thaumoctopus mimicus) and the Atlantic longarm octopus (Macrotritopus defilippi) have both been observed burrowing into sand and then tunnelling horizontally before surfacing over 50cm away several minutes later (Hanlon et al., 2008; Hanlon and Messenger, 2018). Being largely pelagic, most squid do not generally exhibit hiding behaviour. However, bobtail squid (Order: Sepiolida) bury themselves in the sand, and some species, such as Hawaiian bobtail squid (Euprymna scolopes) secrete a mucous coat which causes sand to stick to their body surface, further enhancing their cover (Shears, 1988). Meanwhile, as noted above, Caribbean reef squid conceal themselves among coral and seaweed to hide from potential predators. Young fourhorn octopus (Pteroctopus tetracirrhus) still in their pelagic phase are thought to gain some level of protection from hiding inside the cylindrical tunicate Pyrosoma atlanticum (Villanueva et al., 2020). Additionally, several species of octopus use objects that they find on the seabed as tools to hide from predators. Tool use is defined as “the use of an external object as a functional extension of mouth or beak, hand or claw, in the attainment of an immediate goal” (Van Lawick-Goodall, 1971). Consistent with this, the veined octopus (Amphioctopus marginatus) has been observed to carry empty coconut shells, and to hide inside these when predators approach, while similar use of clam shells has been observed in the common octopus (Octopus vulgaris) (Mather, 1994; Finn et al., 2009).
Cephalopods commonly display nocturnal or crepuscular activity patterns, which may reduce the likelihood of attracting predators (Hanlon and Messenger, 2018), but could also aid hunting. Cephalopod activity patterns vary throughout the day with many species active primarily at night, or at dawn and dusk (Meisel et al., 2006; Schaffeld et al., 2016). The timing of maximum activity throughout the day can be influenced by a variety of factors including intra- and interspecific competition, light intensity, tidal state and, perhaps the most influential factor, food availability (Meisel et al., 2003; Hanlon and Messenger, 2018). Reduced diurnal activity has the added benefit of making primary defences more effective during the peak activity time of many visually orientated predators, although nocturnal predation still appears to be a key selection pressure, as many cephalopods continue to display cryptic behaviours in very low light levels (Allen et al., 2010).
Masquerade is particularly useful when an organism attempts to ‘blend in’ with an otherwise homogenous environment. Imitating objects in the environment rather than trying to match the background substrate makes an animal more likely to be detected as an object, but can reduce the likelihood of being correctly recognised as prey (Stevens and Merilaita, 2009; Buresch et al., 2011). For example, common cuttlefish (Sepia officinalis) will preferentially disguise themselves as a conspicuous but inanimate object rather than attempt cryptic behaviour when in low contrast environments (e.g. sandflats), but will revert to cryptic behaviour in high contrast environments (e.g. reefs) (Buresch et al., 2011). Indeed, common cuttlefish have been documented masquerading as pebbles, coral and other inanimate objects against otherwise uniform backgrounds (Hanlon et al., 2009; Panetta et al., 2017). Juvenile Caribbean reef squid have also been observed imitating coral, as well as seaweed and even their own ink (Moynihan and Rodaniche, 1982).
If an animal attempting camouflage moves, it will often give away its position (Hall et al., 2013). Motion camouflage is where an animal moves in a particular manner or whilst displaying certain patterns, such that it avoids detection whilst travelling. This is distinct from crypsis in that it is specifically the movement of the animal which is disguised. However, motion camouflage is often combined with cryptic behaviour to enhance the overall effect. For example, the veined octopus (Amphioctopus marginatus) has been observed ‘walking’ bipedally along the seabed with its other arms tucked in under its body (Huffard, 2006). By moving in this way, the animal apparently resembles objects such as coconut shells, rocks and sponges being pushed around on the seabed by currents and wave action and is thus able to escape the vicinity of a predator without detection. Underwater caustics are particularly helpful in camouflaging movement in marine environments (Cuthill et al., 2019). For example, the big blue octopus (Octopus cyanea) may slowly crawl across open spaces while curled up to resemble a rock, with movement barely distinguishable from the pattern of light refracting through ripples on the water’s surface (Hanlon et al., 1999).
Cephalopods also use mimicry to avoid recognition. Animals attempting to imitate other organisms are said to be employing mimicry. The majority of defensive mimicry in cephalopods is categorised as Batesian mimicry, whereby a harmless organism mimics a dangerous model (Bates, 1981). The mimic octopus (Thaumoctopus mimicus) famously mimics several venomous species, such as sea snakes, eels, and lionfish, not only by changing colour, but also by mimicking body postures and movement of the model (Norman et al., 2001; Hanlon et al., 2010). Both the mimic octopus and the Atlantic longarm octopus (Macrotritopus defilippi) mimic local species of flatfish to disguise their movement (Norman et al., 2001; Hanlon et al., 2010). This likeness is thought to deter gape-limited predators that would be unable to successfully attack a larger target such as a flatfish. The pharaoh cuttlefish (Sepia pharaonis) has been observed to mimic hermit crabs in captivity, with Smith’s cuttlefish (Sepia smithi) showing the same behaviour in the wild, presumably to present a less appealing target to potential predators, especially in flat, homogenous environments (Okamoto et al., 2017; van Elden and Meeuwig, 2020). Cephalopods also use mimicry to assist in prey capture, mimicking benign species such as parrotfish or crustaceans to approach their prey closely without causing alarm (Moynihan and Rodaniche, 1982; Nakajima and Ikeda, 2017). Consequently, many behaviours where cephalopods appear to be utilising mimicry require further research, as it is not always clear whether the behaviour is primarily defensive or aggressive (i.e. used for prey-capture).
4.2 The significance of the ‘wait and see’ strategy
As described above, many cephalopods exhibit cryptic behaviour, and they often employ a ‘wait and see’ strategy regarding their response to potential predators (Moynihan and Rodaniche, 1982; Hanlon and Messenger, 2018). This involves selecting an appropriate secondary defence only once it is apparent that they have been detected by a potential predator, and sometimes delaying further until the predator approaches within a certain distance (Moynihan and Rodaniche, 1982). This can involve careful observation of the potential predator and complex neurological calculations based on previous experience, facilitated by cephalopods’ well-developed eyes and brains (Amodio et al., 2018; Mezrai et al., 2020). Thus, memory and learning are important traits for predator avoidance in cephalopods (Box 5), to remember and recognise predator types and learn correspondingly effective means of response.
Box 5 Memory and learning in cephalopods.
All cephalopods (including nautiluses) possess long-term memory, with octopuses in particular possessing learning capabilities that are often described as ‘vertebrate-like’ (Basil et al., 2011; Gutnick et al., 2021; Schnell and Clayton, 2021). Associative learning mechanisms are common across coleoids, with octopuses, cuttlefish and squid all capable of discriminating between the cause and effect of different stimuli, and even re-learning associations when patterns are reversed (discrimination reversal) (Schnell et al., 2020). For example, embryos of pharaoh cuttlefish (Sepia pharaonis) reduce their ventilation rate and activity levels in response to either visual or chemical stimuli of a potential predator, or conspecific ink, but not to that of a non-threatening species of clownfish (Mezrai et al., 2020). However, when clownfish are presented alongside conspecific ink, subsequent trials with only clownfish stimuli elicit a defensive response, demonstrating a capacity for associative learning (Mezrai et al., 2020).
Some coleoid cephalopods appear capable of social learning (Amodio and Fiorito, 2013; Sampaio et al., 2020). In one experiment, common octopuses learnt to discriminate between two coloured balls faster after having viewed a demonstration by a conspecific (Fiorito and Scotto, 1992). Further experimentation with both octopuses and cuttlefish have confirmed that the capacity to learn socially is present in cephalopods (Sampaio et al., 2020). The asocial nature of many cephalopod species will restrict opportunities for social learning, however, in species such as Caribbean reef squid (Sepioteuthis sepioidea), where individuals regularly congregate in groups and perform complex anti-predator behaviours together, it is certainly plausible that social learning could play an important role in behavioural transmission of strategies to avoid predation.
Memory may help to categorize predatory species by threat level, and even potentially behavioural syndromes (i.e. animal personality) displayed by individual predators within a species. Cephalopods may also utilise differences in fish face shape to help identify predatory species, although this requires experimental validation (Karplus and Algom, 1981). However, alternative possibilities exist: The common octopus (Octopus vulgaris) is able to recognise conspecifics with which it has previously come into contact (Tricarico et al., 2011), whilst male blue-ringed octopuses recognise and retreat faster from females with which they have previously mated (Morse and Huffard, 2022).
The role of predators in driving the evolution of behavioural complexity in cephalopods is potentially supported by detailed observations of reef squid. Reef squid (Sepioteuthis, a genus of pencil squid) live in clear-water predator-rich reef environments, and have one of the highest predator encounter rates, and also some of the most sophisticated anti-predator responses, recorded among cephalopods (Moynihan and Rodaniche, 1982; Hanlon and Messenger, 2018). Caribbean reef squid (Sepioteuthis sepioidea), are typically found in shoals of 4-30 individuals of mixed sexes and sizes, with remarkably limited evidence of cannibalism (Moynihan and Rodaniche, 1982). Observations suggest that these shoals respond to predators collectively as a group, and that they may be able to identify the level of threat posed by the approach of different predators, and alter and coordinate their defensive responses accordingly (Moynihan and Rodaniche, 1982). The detection of high threat predators results in flight, while deimatic responses are shown to medium or low threat predators, including chromatic displays and defensive line formations, which have been described as being similar to fighter jet formations (Moynihan and Rodaniche, 1982).
It has been suggested that individuals in Caribbean reef squid shoals act as sentinels for the group, that keep watch for predators (Hanlon and Budelmann, 1987). Sentinel behaviour is defined by having a high level of coordination; i.e. the sentinel individual will change according to regular ‘shifts’, and provide an alarm signal to the rest of the group when a predator is detected, which would not be given if the individual were alone (Bednekoff, 2015). Sentinel behaviour is often associated with mammals, especially meerkats (Suricata suricatta), although it is also present in many bird species and a few species of fish (Bednekoff, 2015). If Caribbean reef squid do make use of sentinels, they would be the first species of invertebrate currently known to do so. Whilst this would be a remarkable discovery, it would not necessarily represent evidence of cooperation, as sentinel behaviour can evolve entirely through selfish motivations (Clutton-Brock et al., 1999; Ridley et al., 2013). The system of predator detection and defence in Caribbean reef squid is so effective that many small fish (especially goatfish – Family: Mullidae) and some other squid species such as the slender inshore squid (or arrow squid, Doryteuthis plei) have been observed to actively associate with shoals of Caribbean reef squid, purportedly to benefit from the added protection (Moynihan and Rodaniche, 1982; Nunes et al., 2007).
Most cephalopods appear to lack the complex communication systems that typically accompany animals with elaborate social structures. However, there is evidence of what may be syntactic communication (where separate elements are combined into complex structures, enhancing their meaning) in at least three species of cephalopod, the Caribbean reef squid (Sepioteuthis sepioidea), the bigfin reef squid (or oval squid, Sepioteuthis lessoniana) and the Humboldt squid (Dosidicus gigas) (Moynihan and Rodaniche, 1982; Lin et al., 2017; Burford and Robison, 2020). These communication systems appear to be used in a variety of contexts, from mating behaviour to coordinating anti-predator responses, but much research remains to elucidate this.
4.3 Secondary defences – strategies to escape predation once detected
Secondary defences involve any form of defence employed after recognition by a potential predator. Secondary induced defences can be split into four main categories in cephalopods: (i) deimatic responses, (ii) protean responses, (iii) flight responses, and (iv) retaliatory responses. We discuss these in turn below.
4.3.1 Deimatic responses – startling predators to facilitate escape
Deimatic responses aim to startle or surprise a predator, making it recoil or abort an attack (Umbers et al., 2017). This provides time for the prey to escape and potentially prevents further predation attempts. Cephalopods show a vast range of deimatic displays which include combining neurally controlled chromatophore elements with tentacle displays, changes in skin texture, and formation swimming in social species (Byrne et al., 2003; Macia et al., 2004). The common octopus (Octopus vulgaris) pales completely when threatened whilst ‘puffing up’ its web (the skin between the base of the arms) to give an illusion of increased size, which is typical of many cephalopod deimatic responses (Hanlon, 2007). Meanwhile, the flamboyant cuttlefish (Metasepia pfefferi) is renowned for its striking colouration, which is usually employed during mating behaviour, but is also used as a deimatic response (Hanlon and McManus, 2020). The vampire squid (Vampyroteuthis infernalis) has organs at the tips of its arms capable of bioluminescence, which may function both to startle a predator and act as deflective marks, directing the attack away from the centre of the body towards the arms, which can be regenerated (Robison et al., 2003).
Some cephalopods, including the common cuttlefish (Sepia officinalis) and the Atlantic white-spotted octopus (Callistoctopus macropus), include distinctive mantle spots in their deimatic displays which are directed towards attackers (Hanlon and Messenger, 2018). Spots like this, found in many taxa across the animal kingdom, are often termed ‘eyespots’ due to their resemblance (at least to human observers) to eyes (Stevens and Ruxton, 2014). It is hypothesised that this resemblance causes predators to falter or desist in an attack (Stevens, 2005; Kodandaramaiah et al., 2009). However, whilst eyespots have a demonstrated deimatic effect, the exact mechanism behind this is not always clear (Stevens, 2005; Stevens and Ruxton, 2014).
Deimatic responses represent a gamble since the time they take to perform could instead be spent fleeing. Therefore, as a strategy deimatic displays should only be performed to predators they are effective against, which appears to be the case in cephalopods. For example, the common cuttlefish (Sepia officinalis) has been shown to preferentially display deimatic responses to low threat predatory teleost fish, whilst fleeing from larger predators (Langridge, 2009), and Caribbean reef squid (Sepioteuthis sepioidea) are known to make similar distinctions (Moynihan and Rodaniche, 1982; Hanlon and Messenger, 2018).
4.3.2 Protean responses – confusing predators to facilitate escape
Protean responses prevent or impede predators from tracking or predicting the position or actions of their prey (Humphries and Driver, 1970). For example, protean responses include fleeing in zigzags rather than in a straight line. Perhaps the best-known cephalopod defence, inking, is also considered to be a protean response (Wood et al., 2008; Staudinger et al., 2011) (Box 6). Another protean response used by cephalopods is autotomy (Wood and Wood, 1932; Fleming et al., 2007; Bush, 2012), whereby an animal deliberately sheds part of its own body, usually to elude attack (Emberts et al., 2019), such as tail loss in lizards. The oceanic squid, Octopoteuthis deletron, has several well defined fracture planes along the length of its arms, although it is able to autotomise its arms anywhere along their length (Bush, 2012). This makes it the only cephalopod known to exhibit ‘economy of autotomy’, where an individual possesses multiple fracture planes and will selectively autotomise the smallest segment necessary to escape (Emberts et al., 2019). The autotomised arm of the oceanic squid also bioluminesces, and in some cases even attacks the predator, heightening its distracting effect (Bush, 2012). Cephalopods are able to regrow both autotomised arms and tentacles (Aldrich and Aldrich, 1968; Bello, 1995; Shaw et al., 2016; Imperadore et al., 2017). For example, common and pharaoh cuttlefishes (Sepia officinalis and Sepia pharaonis respectively) are able to regenerate 95% of an autotomised arm within 39 days (Tressler et al., 2014). Another notable protean defence in cephalopods is interference colouration, whereby an animal’s colour appears to change with the angle of illumination or observation (Osorio and Ham, 2002). This occurs with the components of cephalopod colouration created by multilayer reflectance (Mäthger et al., 2009). Thus, as a predator approaches, the organism appears to constantly change colour, causing the predator to lose their search image, sometimes resulting in prey effectively disappearing against the background (Pike, 2015).
Box 6 Inking in cephalopods.
Nearly all coleoid cephalopods produce a melanin-based ink, which they often eject in a cloud or in strands when startled (Bush and Robison, 2007; Wood et al., 2008). Inking may obscure the cephalopod from view, enabling them to make their escape, or may distract the predator’s attention and even deceive them into attacking the ink cloud instead (Hanlon and Messenger, 2018). Almost all coleoid cephalopods are able to produce ink composed of mucus secretions from the funnel organ combined with pigment compounds, particularly melanin, from the ink sac (Derby, 2014). The composition of cephalopod ink can be altered by the individual: Ink with a high mucus content forms long strung-out ‘ropes’, whilst ink with a low mucus content results in a large cloud (Derby, 2014). Cephalopods are also able to produce ‘pseudomorphs’, blobs of ink of similar size and shape to their body (Derby, 2014). Some deep water cephalopods, such as deep-sea bobtail squid (Heteroteuthis spp.), expel a luminous ink visible in poor light conditions (Herring, 1977).
Experiments on French grunts (Haemulon flavolineatum) have demonstrated that the presence of squid ink between the fish and a food item significantly delayed capture time compared to an uncoloured control (Wood et al., 2010). Additionally, inking of live longfin inshore squid (Doryteuthis pealeii) caused bluefish (Pomatomus saltatrix) to startle and abandon attacks, while summer flounders (Paralichthys dentatus) misdirected attacks towards ink pseudomorphs (Staudinger et al., 2011). Ink also appears to be unpalatable to some predators. In both summer flounders and sea catfish (Ariopsis felis), palatability of otherwise identical food pellets decreased with the addition of squid ink, suggesting that inking continues to be an effective defence even if a cephalopod is captured (Derby et al., 2013). Additionally, inking serves a secondary function in schooling species as a visual, but notably not chemical, alarm to other individuals (Wood et al., 2008). However, melanin and other protein components, which comprise over 20% of the mass of cephalopod ink, are energetically expensive and time consuming to produce (Derby, 2014). Consequently, despite its efficacy, cephalopods cannot rely on inking as a frequent means of defence, especially in environments with high predator interaction rates such as coral reefs.
4.3.3 Flight responses – a limited ability to outswim attack
Flight is a commonplace anti-predator response across the animal kingdom (Edmunds, 1974). As with many fish adapted to bursts of speed, locomotor and ventilator processes are linked in cephalopods, whereby the increased rate of mantle contractions that occurs during jetting (Box 7), also increases water flow over the gills (Wells, 1990). Internalisation and reduction of the shell in coleoids permitted expansion of the mantle cavity, providing a major boost to jet propulsion (Wells and O’Dor, 1991; Kröger et al., 2011). But generating speeds necessary to outmanoeuvre fish, even over short distances, put high energetic demands on the underlying molluscan physiology of cephalopods. To support increased oxygen demands required for greater mobility, cephalopods evolved a considerable expansion of their circulatory system. Unlike many invertebrates, cephalopods possess a closed circulatory system, and enlargement of the mantle cavity was coupled with supplementation of the systemic heart through the addition of twin accessory branchial (gill) hearts, originating from the nautilus pericardial glands (Bourne et al., 1977; Wells, 1992), resulting in the advanced circulatory system present in modern coleoids. Together with efficient oxygen uptake through gills and skin (Birk et al., 2018), these adaptations pushed back the respiratory limitations of cephalopod‘s molluscan ancestry, facilitating a shift towards a more active lifestyle (Seibel, 2016; Hochachka, 1994). Thus, concerted physiological adaptations facilitated an ecological transition in cephalopods from heavy-shelled relatively cumbersome organisms to more streamlined forms, with greatly reduced or completely absent shells, better tailored for agility and speed (Klug et al., 2016). As noted above, these changes coincided with a general shift from Palaeozoic ecologies, towards higher-metabolism predation and durophagy (predation of hard-shelled organisms), in particular due to the success of the jawed fishes, and a general escalation of predator-prey interactions (Klug et al., 2010; Bush and Bambach, 2011; Tanner et al., 2017).
Box 7 Jetting in cephalopods.
The primary flight mechanism in cephalopods is jetting. Cephalopods with mantle fins (decapods, cirrate octopuses, vampire squid) can propel themselves by ‘finning’, but this is primarily used for slower movements rather than rapid propulsion (Aitken and O’Dor, 2004). Indeed, cephalopods were the originators of the world’s first jet propulsion system (Wells and O’Dor, 1991). To jet, cephalopods use their circular mantle muscles to draw water into the mantle cavity, which is then forcibly expelled through the siphon, generating thrust (O’Dor, 2002). Cephalopods can alter the direction of thrust by changing the position of the siphon, making jetting a highly manoeuvrable form of locomotion. To generate impulses that travel along their unmyelenated nerves rapidly enough to facilitate jetting as a response to danger, cephalopods have evolved nerve axons with a very large diameter (see Box 8 below). But while jetting can provide a rapid escape from immediate danger, it is energetically costly, and can result in bodily damage or arrival into even greater danger.
Some cephalopods are capable of jetting into the air to temporarily avoid predators (Muramatsu et al., 2013). This may involve simply flopping back down into the water without travelling very far (Macia et al., 2004), although some oceanic squid species are capable of more sophisticated aerial gliding. There are reports suggesting that certain oceanic squid can glide for considerable distances, with observations of the common clubhook squid (Onychoteuthis banksii) gliding more for than 40m (Macia et al., 2004; Muramatsu et al., 2013).
Ultimately, physiological limitations constrained the potential of cephalopods to engage in sustained flight when compared to fish. Oxygen transport and carrying capacity is lower in cephalopods compared to fish, due to their smaller hearts relative to body size, and their use of the copper-based respiratory pigment haemocyanin (as for other molluscs) rather than haemoglobin (the equivalent protein in vertebrates) (O’Dor, 1988; O’Dor, 2002; Seibel, 2016). Haemocyanin has a much lower oxygen carrying capacity than haemoglobin (<50% of the O2% by volume), and it is extracellular in cephalopods and so limited to low blood concentrations due to viscosity constraints (Seibel, 2016). Cephalopods also have little or no venous oxygen reserves (Seibel, 2016). Thus, under conditions of high oxygen demand, cephalopods must make maximal use of blood-borne oxygen at every circulatory cycle, as facilitated by increased gill ventilation, heart rate and stroke volume (Seibel, 2016). Jet propulsion is also highly inefficient relative to the caudal fin swimming of fish. For example, tunnel respirometry experiments suggest that short finned squid (Illex illecebrosus) use six times as much energy per unit distance, and have a Froude efficiency (a ratio of power output compared to energy input) three times lower than sockeye salmon of a similar size (Oncorhynchus nerka) (Webber and O’Dor, 1985; O’Dor and Webber, 1986). Meanwhile, since most cephalopod predators are larger fish (Randall, 1967; Staudinger et al., 2013), these limitations mean that cephalopods are not well suited to out-swimming fish. Thus, while flight can be effective over short distances to escape immediate threat, in cephalopods it is typically used in tandem with other anti-predator responses (particularly protean responses) and is generally reserved for the most dangerous predators or where other strategies have failed (Hanlon and Messenger, 2018).
4.3.4 Retaliatory responses – a last resort
Cephalopods possess several forms of weaponry that can be used in retaliation. However, these defences appear to be of limited effectiveness for fighting off determined, larger fish predators, and generally appear only to be used as a last resort against attack, once other options have been exhausted (Hanlon and Messenger, 2018). We review the physical defences of cephalopods below.
Coleoid cephalopods possess muscular arms lined with suckers, and in many cases, sharply toothed chitinous sucker rings, which can be used for defence. For example, scarring found on the heads of many sperm whales is believed to be caused by giant squid (Architeuthis dux) defending themselves against predation (Roper and Boss, 1982). Reports of similar markings on an oceanic whitetip shark (Carcharinus longimanus) may have been caused by flying squid (Thysanoteuthis spp.), glass squid (Megalocranchia spp.), or giant squid (Papastamatiou et al., 2020). The colossal squid (Mesonychoteuthis hamiltoni) has hooks along the length of its arms and larger, revolving ‘club hooks’ at the tips of its tentacles, which are believed to be used in prey capture, but may also be used in self-defence (Rosa et al., 2017).
Cephalopods lack rigid skeletal elements and instead depend on a form of bodily support called a muscular hydrostat, whereby muscles provide both force generation and the support for movement (Kier, 2016). This system allows incredible flexibility, aiding the ability of the arms to grasp an attacker. Many species of octopus have been observed to fold their arms back around themselves in defensive postures, presenting their suckers as a physical defence against attack (Mather, 1998). When captured in the mouth of a pyjama shark (Poroderma africanum), common octopuses (Octopus vulgaris) have been observed to force their arms into the shark’s gills, disrupting breathing, and facilitating release (Jeffs and Brownlow, 2017).
Cephalopod beaks appear in the fossil record during the Devonian period, coinciding with the radiation of jawed-vertebrates and the Devonian Nekton Revolution (Klug et al., 2010). Cephalopod beaks are composed of chitin, and together with the radula and salivary glands, form part of the buccal mass (Uyeno and Kier, 2007). The beak comprises an upper and lower mandible and is articulated by four muscles in a flexible joint, which lacks a rigid anchor point for muscle attachment, and so constrains bite strength. In combination with the relative softness of chitin, this may explain why cephalopods do not commonly bite in self-defence, unlike vertebrates, which possess enamel coated teeth and powerful jaws.
Many cephalopods use venom during the capture of prey (Fry et al., 2009; Cooke et al., 2017). For example, blue ringed octopuses (Hapalochlaena spp.) possess an extremely potent tetrodotoxin venom (TTX), similar to that of pufferfish, that causes paralysis when injected via a bite (Whitelaw et al., 2019). The threat is signposted to potential predators by an aposematic display featuring 40-60 bright blue rings or stripes (Roper and Hochberg, 1988; Kawashima et al., 2019). Yet despite the prevalence of venom for predation among cephalopods (Fry et al., 2009; Cooke et al., 2017), there is limited evidence, as with the cephalopod beak and arms, that venom is a common defensive strategy in most cephalopod species. However, some cephalopods make use of venom borrowed from other species to ward off threats. For example, the blanket octopus (Tremoctopus violaceus) has been observed collecting and carrying venomous tentacles from the Portuguese man o’war (Physalia physalis) in its arms, which it presents to incoming threats by curling its arms over its head (Norman et al., 2002).
In summary, since modern cephalopods share considerable niche overlap with fish (Packard, 1972; House et al., 1988), and both widely predate upon and are predated by fish, it is likely that all three forms of ecological pressure mentioned above played roles in cephalopod evolution. However, the diversification of large, fast, fishes with strong teeth and jaws and keen eyesight, may have resulted in predation providing the strongest selection pressure, with intense predator-prey arms races driving coleoid cognitive evolution. Specifically, a relative deficiency in mechanical defences and the limitations of cephalopod aerobic capacity compared to fish, cut off roads to outright confrontation and the option to out-swim many fish predators, and so may have favoured cognitive adaptations to avoid predation, rather than other forms of defence. However, we add the caveat that defensive behaviours, such as fighting predators off, remain relatively overlooked in cephalopods, and have not been widely analysed in explicit study designs across cephalopod diversity. Thus, in contrast to the large body of literature on cephalopod cognitive abilities, knowledge of the frequency and distribution of cephalopod defensive behaviours remains relatively scarce.
5 Given that other invertebrate groups have not undergone similar cognitive evolutionary trajectories, what is special about cephalopods?
There is a great diversity of invertebrate lineages, yet only cephalopods possess anything like the advanced cognitive abilities and nervous systems (Box 8) of vertebrates. This raises the major outstanding question, what is special about cephalopods? Like many invertebrates, cephalopods have a fast life history, and relatively simple social environments, with the opposite states (i.e. long life history and/or complex social environments) being implicated in vertebrate cognitive evolution (Amodio et al., 2019). The answer is also unlikely to be disproportionate predation pressure, as many other invertebrate lineages experience similar or higher levels of predation to cephalopods. Meanwhile other invertebrate groups lack shells or hard exoskeletons but have not evolved the advanced cognitive abilities of cephalopods. For example, multiple lineages of sea slug (Order Opisthobranchia) have independently lost a protective shell, but none have undergone pronounced cognitive evolution (Amodio et al., 2018; Mollo et al., 2019).
Box 8 The eyes, nerves, and brains of cephalopods.
The eyes of cephalopods famously bear great structural and functional resemblance to those of vertebrates, and provide high visual acuity, which is an extremely important aspect of cephalopod anti-predator responses (Sweeney et al., 2007). Most cephalopods are also visual predators, and as such, investment in energetically expensive eyes has a two-fold benefit. Cephalopod eyes are often larger than those of an equivalently sized vertebrate (Packard, 1972). This is partially an adaptation to low light levels, as cephalopods lack the tapetum lucidum present in many nocturnal vertebrates. However, there is evidence that large eyes may have evolved to facilitate predator detection. Experiments on giant squid suggest that their huge eyes enable the detection of a large predator, such as a sperm whale (Physeter macrocephalus), from over 120m away, providing valuable additional time for escape (Nilsson et al., 2012).
Another famous component of the cephalopod nervous system is the squid giant axon (Young, 1938; Hodgkin and Huxley, 1939). Cephalopods, like other molluscs, lack myelin or equivalent myelin-like sheaths found in certain other invertebrates. Consequently, the conduction velocity of cephalopod nerves is much lower than equivalently sized nerves in vertebrates. To counteract this and enable conduction velocities equivalent to those observed in myelinated vertebrate nerves, cephalopods have evolved axons with a very large diameter. Since action potentials travel much faster in axons with a larger diameter, these giant axons improve the speed of cephalopod responses, and are important in permitting a fast escape response from fish predators.
Like vertebrates, cephalopods have complex multi-lobed brains. Indeed, among invertebrates cephalopods possess the most complex and centralized brains, which show a variety of ‘vertebrate-like’ adaptations (Gutnick et al., 2021). In particular, coleoid cephalopods have the largest brain to body size ratio of any invertebrate, and their relative brain size is larger than that of many reptiles and fish (Packard, 1972; Schnell et al., 2020), while the hundreds of millions of neurons in the octopus brain rivals the size and complexity of many mammalian brains (Katz, 2016). The large size of cephalopod brains is reflected by their high level of behavioural flexibility, with octopuses and cuttlefish demonstrating the most vertebrate-like intelligence among invertebrates (Katz, 2016). Octopuses are famously capable of solving complex mazes and being able to open closed jars, but cephalopod cognition extends beyond problem solving (Amodio and Fiorito, 2013; Schnell et al., 2020). For example, cuttlefish understand object permanence (i.e. just because an object ceases to be detectable, it does not cease to exist), which is an important precursor to many more complex processes (Schnell et al., 2020).
Cephalopods also possess a lateral line system, analogous to that used by fish to detect vibrations in the water (Budelmann and Bleckmann, 1988). This enables them to detect both predators and prey in conditions where vision may be compromised.
Despite the many similarities between cephalopod and vertebrate nervous systems there are also some key differences. For example, in octopuses, the peripheral nervous system is so well developed that more than half of octopus neurons are found outside of the brain (Young, 1971). This devolved nature of octopus nervous systems enables octopuses to ‘delegate’ control of processes such as crawling to the arms themselves freeing the brain from needing to coordinate this computationally complex process (Levy et al., 2015; Zullo et al., 2019). Severed octopus arms will even continue to exhibit natural movement patterns when stimulated artificially (Sumbre et al., 2001).
Timing may have played an important role in the unique cognitive evolution of cephalopods, considering a two-step model, involving initial cognitive priming resulting from the need to navigate three dimensional environments, followed by secondary augmentation under intense predator-prey coevolutionary dynamics (Grasso and Basil, 2009; Amodio et al., 2019). According to this model, ascension from the sea floor selected for a first suite of sensory, motor, and cognitive adaptations in early cephalopods at a time when the water column remained relatively free from predators. These initial adaptations laid the foundation for subsequent neurological advances during intense co-evolution arms races with bony fishes. Indeed, nautiluses, a lineage of primitive shelled cephalopods, possess a centralised brain, relatively complex eyes, and basic cognitive abilities (Young, 1965). Consequently, cephalopods may have been in the right place at the right time and with a sufficient degree of existing cognitive abilities, to experience a form of cognitive runaway selection imposed by evolving teleost predators.
We argue that vigilance and a wait-and-see strategy of avoiding predation likely provided a favourable context for further sensory and cognitive advancements in cephalopods. We also suggest that this trajectory was directed by constraints on cephalopods that prevented them from using flight or physical confrontation as the main form of defence against fish predators. Such an emphasis may have favoured advanced learning abilities to respond appropriately to different types of predator and classes of threat, and sensory abilities to detect predators and hide. Meanwhile, the effectiveness of the wait-and-see strategy was likely facilitated by the skin patterning system, the control and deployment of which could have provided a further favourable context for cognitive advancements. Under this scenario, initial steps towards crypsis following the internalisation of the shell provided a platform for the selection of adaptations that enhanced camouflage, predator recognition, memory, and in some cases the expression of context-specific anti-predatory behaviours depending on perceived threat-levels judged from past experience.
6 Future research directions
A major outstanding challenge to gain an improved understanding of the evolution of anti-predator strategies in cephalopods is disentangling the extent to which different anti-predatory responses evolved directly due to predation pressure, versus other forms of selection. Most important among these is the evolution of the complex cognitive and sensory systems of cephalopods.
Increased cognitive capabilities are often attributed to either the ecological hypothesis, whereby individuals must contend with varying types and availability of food, or the social hypothesis, whereby group-living individuals must maintain, monitor, and react to their own relationships and those of their groupmates (DeCasien et al., 2017; Johnson-Ulrich, 2017; Rosati, 2017; Ashton et al., 2018). While most cephalopods do not have to contend with the challenges of a social environment, they certainly do have to overcome patchiness in prey distribution and a large diversity of potential prey (and so often require diverse prey capture and handling techniques), which frequently occur within structurally and ecologically complex environments (Schnell et al., 2020). Cephalopods also frequently have challenging reproductive systems, which can pose significant risks to fitness (Amodio et al., 2018). Thus, selection for increasing survivability against predator attack likely occurred concurrently with several other pressures (Amodio et al., 2019). Predation pressure is less frequently considered as a driver of the evolution of complex cognition, and yet, predation risk can provide strong selective pressure due to the high fitness cost of being caught (van der Bijl and Kolm, 2016; Amodio et al., 2018). Meanwhile, there is a need to further disentangle the role of competition for prey with other predators, including fish and other cephalopods (Amodio et al., 2020; Schnell et al., 2020), in shaping the evolution of cephalopods.
Comparative phylogenetic analyses, offer a means of teasing apart correlations among complex anti-predator traits and other ecological traits, to access general questions relating to the evolution of anti-predator behaviour in cephalopods. However, at present such analyses remain hampered by uncertainty regarding the relationships among many higher-level cephalopod lineages. Greater taxon sampling and utilisation of genome-level datasets offers a potential way forward to resolve the taxonomy of the group and facilitate detailed analyses. Meanwhile, genomic approaches also offer alternative perspectives from which to study cephalopod behaviour. Such methods are likely to offer considerable scope over coming years, given the dense taxonomic sampling and high quality genomes being rapidly generated by projects such as the Darwin Tree of Life Project (Blaxter, 2022) and the Earth Biogenome Project (Lewin et al., 2022).
Skin patterning plays a major role in many cephalopod behaviours and further research is required to examine the evolution of the skin patterning system, the extent to which cephalopods can interpret skin patterning, and how the displays they employ are interpreted by potential predators. The impact of colouration in many terrestrial organisms has been tested by artificially manipulating the organisms themselves or by employing realistic models (Cuthill et al., 2005). The dynamic nature of cephalopod colouration has made similar experiments complicated. However, methods of modelling and replicating cephalopod skin have now been developed (Sutherland et al., 2008b; Sutherland et al., 2008a; Fishman et al., 2015), and offer potential for experimental approaches to further explore the cephalopod skin patterning system.
Many cephalopod behaviours have proven difficult to study in the field, but technological advances such as remotely operated submersibles and vastly improved and low-cost video recorders, are greatly facilitating the study of cephalopod behaviour. New technologies also facilitate exploration of pelagic and bathypelagic regions of the ocean. Most of the anti-predator adaptations discussed here are utilised best against visually orientated predators, and in turn, visual stimuli appear very important for cephalopods to escape predation. Consequently, it will be interesting to examine whether cephalopods living in the deep sea, where light is greatly reduced, exhibit alternative strategies to their shallow water relatives. Meanwhile, the development of the cephalopod experimental projected habitat (CEPH) which projects a virtual habitat onto a fibreglass tank could help replicate the cephalopod’s natural environment in situations where field studies are restrictive (Josef, 2018). Additionally, as mentioned above, several aspects of cephalopod anti-predator behaviour remain poorly explored. For example, the type, relative frequency, and phylogenetic distribution of defence behaviours displayed by cephalopods requires further elucidation, as well as the specific contexts in which these are expressed to predators. Research towards this aim can make use of both improved technology for conducting field observations and experimental analyses.
Parallels in behavioural complexity enable comparisons to be drawn between cephalopods and vertebrate systems, providing a valuable semi-independent study system. From such comparisons, further insights could be gained into the conditions required for the evolution of behavioural and cognitive complexity. In this respect, the potential for syntactic communication, the use of sentinels, and cooperative or altruistic behaviour in cephalopod anti-predator behaviour are particularly fascinating and represent exciting opportunities for further study.
Author contributions
AH and RJ drafted the manuscript and figures. All authors provided edits and comments on the manuscript.
Funding
University of Exeter covered the OA 1688 publication fee. The University of Exeter has an institutional account with Frontiers, so are entitled to get a 10% discount to the APC. AH was supported by a Biotechnology and Biological Sciences Research Council (BBSRC) David Phillips Fellowship (BB/ N020146/1). RJ would like to thank Gary Knowles and Peter Parkin for their support of his studies.
Acknowledgments
The authors are very grateful for comments from three anonymous reviewers, whose feedback greatly helped to improve the manuscript.
Conflict of interest
The authors declare that the research was conducted in the absence of any commercial or financial relationships that could be construed as a potential conflict of interest.
Publisher’s note
All claims expressed in this article are solely those of the authors and do not necessarily represent those of their affiliated organizations, or those of the publisher, the editors and the reviewers. Any product that may be evaluated in this article, or claim that may be made by its manufacturer, is not guaranteed or endorsed by the publisher.
References
Aitken J. P., O’Dor R. K. (2004). Respirometry and swimming dynamics of the giant australian cuttlefish, sepia apama (mollusca, cephalopoda). Mar. Freshw. Behav. Physiol. 37, 217–234. doi: 10.1080/10236240400016579
Aldrich F. A., Aldrich M. M. (1968). On regeneration ot the tentacular arm of the giant squid architeuthis dux steenstrup (Decapoda, architeuthidae). Can. J. Zool. 46, 845–847. doi: 10.1139/z68-120
Allen J. J., Mathger L. M., Buresch K. C., Fetchko T., Gardner M., Hanlon R. T. (2010). Night vision by cuttlefish enables changeable camouflage. J. Exp. Biol. 213, 3953–3960. doi: 10.1242/jeb.044750
Ambrose R. (1982). Shelter utilization by the molluscan cephalopod octopus bimaculatus. Mar. Ecol. Prog. Ser. 7, 67–73. doi: 10.3354/meps007067
Ambrose R. F. (1983). Midden formation by octopuses: The role of biotic and abiotic factors. Mar. Behav. Physiol. 10, 137–144. doi: 10.1080/10236248309378613
Amodio P., Boeckle M., Schnell A. K., Ostojić L., Fiorito G., Clayton N. S. (2018). Grow smart and die young: Why did cephalopods evolve intelligence? Trends Ecol. Evol. 34, 45–56. doi: 10.1016/j.tree.2018.10.010
Amodio P., Boeckle M., Schnell A. K., Ostojic L., Fiorito G., Clayton N. S. (2019). Shell loss in cephalopods: Trigger for, or by-product of, the evolution of intelligence? a reply to mollo et al. Trends Ecol. Evol. 34, 690–692. doi: 10.1016/j.tree.2019.05.005
Amodio P., Fiorito G. (2013). “Observational and other types of learning in octopus,” in Handbook of behavioral neuroscience (Elsevier B.V.), 293–302.
Amodio P., Shigeno S., Ostojić L. (2020). “Evolution of intelligence in cephalopods,” in eLS (Wiley), 77–84.
Anderson F. E., Lindgren A. R. (2021). Phylogenomic analyses recover a clade of large-bodied decapodiform cephalopods. Mol. Phylogenet. Evol. 156, 107038. doi: 10.1016/j.ympev.2020.107038
Ashton B. J., Thornton A., Ridley A. R. (2018). An intraspecific appraisal of the social intelligence hypothesis. Philos. Trans. R. Soc B Biol. Sci. 373, 01–11. doi: 10.1098/rstb.2017.0288
Barbosa A., Allen J. J., Mäthger L. M., Hanlon R. T. (2012). Cuttlefish use visual cues to determine arm postures for camouflage. Proc. R. Soc B Biol. Sci. 279, 84–90. doi: 10.1098/rspb.2011.0196
Basil J., Barord G., Crok R., Derman R., Hui Ju C., Travis L., et al. (2011). A synthetic approach to the study of learning and memory in chambered nautilus, l. (Cephalopoda, nautiloidea). Vie Milieu 61, 231–242.
Bates H. W. (1981). Contributions to an insect fauna of the Amazon valley (Lepidoptera: Heliconidae). Biol. J. Linn. Soc 16, 41–54. doi: 10.1111/j.1095-8312.1981.tb01842.x
Bednekoff P. A. (2015). Sentinel behavior: A review and prospectus. Adv. Stud. Behav. 47, 115–145. doi: 10.1016/bs.asb.2015.02.001
Bello G. (1991). Role of cephalopods in the diet of the swordfish, xiphias gladius, from the eastern Mediterranean Sea. Bull. Mar. Sci. 49, 312–324.
Bello G. (1995). Hectocotylus regeneration in wild-caught sepiolid squids. J. Mar. Biol. Assoc. Unite. Kingdom 75, 491–494. doi: 10.1017/S0025315400018336
Berruti A., Harcus T. (1978). Cephalopod prey of the sooty albatrosses phoebetria fusca and p. palpebrata at Marion island. South Afr. J. Antarct. Res. 4, 99–103.
Bertness M. D., Cunningham C. (1981). Crab shell-crushing predation and gastropod architectural defense. J. Exp. Mar. Bio. Ecol. 50, 213–230. doi: 10.1016/0022-0981(81)90051-4
Birk M. A., Dymowska A. K., Seibel B. A. (2018). Do squid breathe through their skin? J. Exp. Biol. 221. doi: 10.1242/jeb.185553
Bizikov V. A. (2004). The shell in vampyropoda (Cephalopoda): morphology, functional role and evolution. Ruthenica 3, 1–88.
Blaxter M. L. (2022). Sequence locally, think globally: The Darwin tree of life project. Proc. Natl. Acad. Sci. U. S. A. 119, e2115642118. doi: 10.1073/pnas.2115642118
Boogert N. J., Giraldeau L. A., Lefebvre L. (2008). Song complexity correlates with learning ability in zebra finch males. Anim. Behav. 76, 1735–1741. doi: 10.1016/j.anbehav.2008.08.009
Bourne G. B., Redmond J. R., Johansen K. (1977). Nautilus pompilius: Branchial circulation enhanced by an auxiliary pumping mechanism. Experientia 33, 1453. doi: 10.1007/BF01918802
Bower J. R., Ichii T. (2005). The red flying squid (Ommastrephes bartramii): A review of recent research and the fishery in Japan. Fish. Res. 76, 39–55. doi: 10.1016/j.fishres.2005.05.009
Budelmann B. U., Bleckmann H. (1988). A lateral line analogue in cephalopods: water waves generate microphonic potentials in the epidermal head lines of sepia and lolliguncula. J. Comp. Physiol. A 164, 1–5. doi: 10.1007/BF00612711
Buresch K. C., Mäthger L. M., Allen J. J., Bennice C., Smith N., Schram J., et al. (2011). The use of background matching vs. masquerade for camouflage in cuttlefish sepia officinalis. Vision Res. 51, 2362–2368. doi: 10.1016/j.visres.2011.09.009
Burford B. P., Robison B. H. (2020). Bioluminescent backlighting illuminates the complex visual signals of a social squid in the deep sea. Proc. Natl. Acad. Sci. U. S. A. 117, 8524–8531. doi: 10.1073/pnas.1920875117
Bush S. L. (2012). Economy of arm autotomy in the mesopelagic squid octopoteuthis deletron. Mar. Ecol. Prog. Ser. 458, 133–140. doi: 10.3354/meps09714
Bush A. M., Bambach R. K. (2011) Paleoecologic megatrends in marine metazoa. Available at: http://dx.doi.org/10.1146/annurev-earth-040809-152556.
Bush S. L., Robison B. H. (2007). Ink utilization by mesopelagic squid. Mar. Biol. 152, 485–494. doi: 10.1007/s00227-007-0684-2
Byrne R. A., Griebel U., Wood J. B., Mather J. A. (2003). Squid say it with skin: a graphic model for skin displays in caribbean reef squid (Sepioteuthis sepioidea). Berliner Paläobiol. Abh. 3, 29–35.
Carroll S. B., Grenier J. K., Weatherbee S. D. (2013). From DNA to diversity: Molecular genetics and the evolution of animal design. Wiley, Hoboken, NJ
Clarke M. R. (1983). Cephalopod biomass – estimates from predation. Mem. Natl. Museum Victoria 44, 95–107. doi: 10.24199/j.mmv.1983.44.09
Clarke M. R. (1996). Cephalopods as prey. III. cetaceans. Philos. Trans. R. Soc B Biol. Sci. 351, 1053–1065. doi: 10.1098/rstb.1996.0093
Clarke M. R., Denton E. J., Gilpin-Brown J. B. (1979). On the use of ammonium for buoyancy in squids. J. Mar. Biol. Assoc. Unite. Kingdom 59, 259–276. doi: 10.1017/S0025315400042570
Clarke M. R., Stevens J. D. (1974). Cephalopods, blue sharks and migration. J. Mar. Biol. Assoc. Unite. Kingdom 54, 949–957. doi: 10.1017/S0025315400057672
Clements T., Colleary C., De Baets K., Vinther J. (2017). Buoyancy mechanisms limit preservation of coleoid cephalopod soft tissues in mesozoic lagerstätten. Palaeontology 60, 1–14. doi: 10.1111/pala.12267
Clutton-Brock T. H., O’Riain M. J., Brotherton P. N. M., Gaynor D., Kansky R., Griffin A. S., et al. (1999). Selfish sentinels in cooperative mammals. Sci. (80-.). 284, 1640–1644. doi: 10.1126/science.284.5420.1640
Cooke I. R., Whitelaw B., Norman M., Caruana N., Strugnell J. M. (2017). “Toxicity in cephalopods,” in Evolution of venomous animals and their toxins. toxinology. Ed. Malhotra A. (Dordrecht: Springer).
Cuthill I. C., Matchette S. R., Scott-Samuel N. E. (2019). Camouflage in a dynamic world. Curr. Opin. Behav. Sci. 30, 109–115. doi: 10.1016/j.cobeha.2019.07.007
Cuthill I. C., Sanghera N. S., Penacchio O., Lovell P. G., Ruxton G. D., Harris J. M. (2016). Optimizing countershading camouflage. Proc. Natl. Acad. Sci. U. S. A. 113, 13093–13097. doi: 10.1073/pnas.1611589113
Cuthill I. C., Stevens M., Sheppard J., Maddocks T., Párraga C. A., Troscianko T. S. (2005). Disruptive coloration and background pattern matching. Nature 434, 72–74. doi: 10.1038/nature03312
Dawkins R., Krebs J. R. (1979). Arms races between and within species. Proc. R. Soc London. Ser. B. Biol. Sci. 205, 489–511. doi: 10.1098/rspb.1979.0081
DeCasien A. R., Williams S. A., Higham J. P. (2017). Primate brain size is predicted by diet but not sociality. Nat. Ecol. Evol. 1, 0112. doi: 10.1038/s41559-017-0112
Derby C. D. (2007). Escape by inking and secreting: Marine molluscs avoid predators through a rich array of chemicals and mechanisms. 213, 274–289. doi: 10.2307/25066645
Derby C. (2014). Cephalopod ink: Production, chemistry, functions and applications. Mar. Drugs 12, 2700–2730. doi: 10.3390/md12052700
Derby C. D., Tottempudi M., Love-Chezem T., Wolfe L. S. (2013). Ink from longfin inshore squid, Doryteuthis pealeii, as a chemical and visual defense against two predatory fishes, summer flounder, Paralichthys dentatus, and Sea catfish, Ariopsis felis. Biol. Bull. 225, 152–160. doi: 10.1086/BBLv225n3p152
Desmond Ramirez M., Oakley T. H. (2015). Eye-independent, light-activated chromatophore expansion (LACE) and expression of phototransduction genes in the skin of octopus bimaculoides. J. Exp. Biol. 218, 1513–1520. doi: 10.1242/jeb.110908
Doguzhaeva L. A., Weis R., Delsate D., Mariotti N. (2014). Embryonic shell structure of early-middle Jurassic belemnites, and its significance for belemnite expansion and diversification in the Jurassic. Lethaia 47, 49–65. doi: 10.1111/let.12037
Edmunds M. (1974). Defence in animals: a survey of anti-predator defences. Longman. Longman, London, UK
Emberts Z., Escalante I., Bateman P. W. (2019). The ecology and evolution of autotomy. Biol. Rev. 94, 1881–1896. doi: 10.1111/brv.12539
Fang X., Kröger B., Zhang Y. D., Zhang Y. B., Chen T. E. (2019). Palaeogeographic distribution and diversity of cephalopods during the Cambrian–Ordovician transition. Palaeoworld 28, 51–57. doi: 10.1016/j.palwor.2018.08.007
Ferguson G. P., Messenger J. B. (1991). A countershading reflex in cephalopods. Proc. R. Soc B Biol. Sci. 243, 63–67. doi: 10.1098/rspb.1991.0011
Ferguson G. P., Messenger J. B., Budelmann B. U. (1994). Gravity and light influence the countershading reflexes of the cuttlefish sepia officinalis. J. Exp. Biol. 191, 247–256. doi: 10.1242/jeb.191.1.247
Finn J. K., Norman M. D. (2010). “The argonaut shell: Gas-mediated buoyancy control in a pelagic octopus,” in Proceedings of the royal society b: Biological sciences (Royal Society) 277, 2967–2971. doi: 10.1098/rspb.2010.0155
Finn J. K., Tregenza T., Norman M. D. (2009). Defensive tool use in a coconut-carrying octopus. Curr. Biol. 19, R1069–R1070. doi: 10.1016/j.cub.2009.10.052
Fiorito G., Scotto P. (1992). Observational learning in octopus vulgaris. Sci. (80-.). 256, 545–547. doi: 10.1126/science.256.5056.545
Fishman A., Rossiter J., Homer M. (2015). Hiding the squid: patterns in artificial cephalopod skin. J. R. Soc Interface 12, 20150281. doi: 10.1098/rsif.2015.0281
Fleming P. A., Muller D., Bateman P. W. (2007). Leave it all behind: A taxonomic perspective of autotomy in invertebrates. Biol. Rev. 82, 481–510. doi: 10.1111/j.1469-185X.2007.00020.x
Fry B. G., Roelants K., Norman J. A. (2009). Tentacles of venom: Toxic protein convergence in the kingdom animalia. J. Mol. Evol. 68, 311–321. doi: 10.1007/s00239-009-9223-8
Gagnon Y. L., Osorio D. C., Wardill T. J., Marshall N. J., Chung W. S., Temple S. E. (2016). Can chromatic aberration enable color vision in natural environments? Proc. Natl. Acad. Sci. U. S. A. 113, E6908–E6909. doi: 10.1073/pnas.1612239113
Gilmore R., Crook R., Krans J. (2016). Cephalopod camouflage: Cells and organs of the skin | learn science at scitable. Nat. Educ. 9, 1.
Grasso F. W., Basil J. A. (2009). The evolution of flexible behavioral repertoires in cephalopod molluscs. Brain. Behav. Evol. 74, 231–245. doi: 10.1159/000258669
Gutnick T., Shomrat T., Mather J. A., Kuba M. J. (2021). “The cephalopod brain: Motion control, learning, and cognition,” in Physiology of molluscs: A collection of selected reviews. Eds. Saleuddin S., Mukai S. Apple Academic Press, New York NY, 137–178. doi: 10.1201/9781315207483
Hall J. R., Cuthill I. C., Baddeley R., Shohet A. J., Scott-Samuel N. E. (2013). Camouflage, detection and identification of moving targets. Proc. R. Soc B Biol. Sci. 280, 20130064. doi: 10.1098/rspb.2013.0064
Hanlon R. T. (2007). Cephalopod dynamic camouflage. Curr. Biol. 17, R400–R404. doi: 10.1016/j.cub.2007.03.034
Hanlon R. T., Budelmann B. U. (1987). Why cephalopods are probably not “Deaf”. Am. Nat. 129, 312–317. doi: 10.1086/284637
Hanlon R. T., Chiao C. C., Mäthger L. M., Barbosa A., Buresch K. C., Chubb C. (2009). Cephalopod dynamic camouflage: Bridging the continuum between background matching and disruptive coloration. Philos. Trans. R. Soc B Biol. Sci. 364, 429–437. doi: 10.1098/rstb.2008.0270
Hanlon R. T., Conroy L. A., Forsythe J. W. (2008). Mimicry and foraging behaviour of two tropical sand-flat octopus species off north sulawesi, Indonesia. Biol. J. Linn. Soc 93, 23–38. doi: 10.1111/j.1095-8312.2007.00948.x
Hanlon R. T., Forsythe J. W., Joneschild D. E. (1999). Crypsis, conspicuousness, mimicry and polyphenism as anti-predator defences of foraging octopuses on indo-pacific coral reefs, with a method of quantifying crypsis from video tapes. Biol. J. Linn. Soc 66, 1–22. doi: 10.1111/j.1095-8312.1999.tb01914.x
Hanlon R. T., McManus G. (2020). Flamboyant cuttlefish behavior: Camouflage tactics and complex colorful reproductive behavior assessed during field studies at lembeh strait, Indonesia. J. Exp. Mar. Bio. Ecol. 529, 151397. doi: 10.1016/j.jembe.2020.151397
Hanlon R. T., Messenger J. B. (1988). Adaptive coloration in young cuttlefish (Sepia officinalis l.): the morphology and development of body patterns and their relation to behaviour. Philos. Trans. R. Soc London. B Biol. Sci. 320, 437–487.
Hanlon R. T., Messenger J. B. (2018). Cephalopod behaviour. 2nd edn (Cambridge University Press, Cambridge, UK). doi: 10.1017/9780511843600
Hanlon R. T., Watson A. C., Barbosa A. (2010). A “Mimic Octopus” in the Atlantic: Flatfish mimicry and camouflage by macrotritopus defilippi. Biol. Bull. 218, 15–24. doi: 10.1086/BBLv218n1p15
Hartwick E. B., Ambrose R. F., Robinson S. M. C. (1984). Den utilization and the movements of tagged octopus dofleini. Mar. Behav. Physiol. 11, 95–110. doi: 10.1080/10236248409387038
Hildenbrand A., Austermann G., Fuchs D., Bengtson P., Stinnesbeck W. (2021). A potential cephalopod from the early Cambrian of eastern Newfoundland, Canada. Commun. Biol. 4, 1–11. doi: 10.1038/s42003-021-01885-w
Hochachka P. W. (1994). Oxygen efficient design of cephalopod muscle metabolism. Mar. Fresh Behav. Physiol. 25, 61–67. doi: 10.1080/10236249409378908
Hodgkin A. L., Huxley A. F. (1939). Action potentials recorded from inside a nerve fibre. Nat. 1939 1443651 144, 710–711. doi: 10.1038/144710a0
Horton T., Kroh A., Ahyong S., Bailly N., Boyko C. B., Brandão S. N., et al. (2019) WoRMS - world register of marine species - Cephalopoda. Available at: http://marinespecies.org/aphia.php?p=taxdetailsandid=11707 (Accessed 5 July 2019).
House M., Wiedmann J., Kullmann J. (1988). “Major features of cephalopod evolution,” in Cephalopods–present and past (Stuttgart: Schweizerbart’sche Verlagsbuchhandlung), 1–16.
Huffard C. L. (2006). Locomotion by abdopus aculeatus (Cephalopoda: Octopodidae): Walking the line between primary and secondary defenses. J. Exp. Biol. 209, 3697–3707. doi: 10.1242/jeb.02435
Humphries D. A., Driver P. M. (1970). Protean defence by prey animals. Oecologia 5, 285–302. doi: 10.1007/BF00815496
Imber M. J. (1973). The food of grey-faced petrels (Pterodroma macroptera gouldi (Hutton)), with special reference to diurnal vertical migration of their prey. J. Anim. Ecol. 42, 645. doi: 10.2307/3130
Imperadore P., Shah S. B., Makarenkova H. P., Fiorito G. (2017). Nerve degeneration and regeneration in the cephalopod mollusc octopus vulgaris: The case of the pallial nerve. Sci. Rep. 7, 1–15. doi: 10.1038/srep46564
Jatta G. (1896). I Cefalopodi viventi nel golfo di napoli (sistematica): monografia/ (Berlin: R. Friedländer and Sohn).
Johnson-Ulrich L. (2017). “The social intelligence hypothesis,” in Encyclopedia of evolutionary psychological science (Cham: Springer International Publishing), 1–7.
Jones B. W., Nishiguchi M. K. (2004). Counterillumination in the Hawaiian bobtail squid, euprymna scolopes berry (Mollusca: Cephalopoda). Mar. Biol. 144, 1151–1155. doi: 10.1007/s00227-003-1285-3
Josef N. (2018). Cephalopod experimental projected habitat (CEPH): Virtual reality for underwater organisms. Front. Mar. Sci. 5. doi: 10.3389/fmars.2018.00073
Karplus I., Algom D. (1981). Visual cues for predator face recognition by reef fishes. Z. Tierpsychol. 55, 343–364. doi: 10.1111/j.1439-0310.1981.tb01277.x
Katsanevakis S., Verriopoulos G. (2004). Den ecology of octopus vulgaris cuvie On soft sediment: Availability and types of shelter. Sci. Mar. 68, 147–157. doi: 10.3989/scimar.2004.68n1147
Katz P. S. (2016). Phylogenetic plasticity in the evolution of molluscan neural circuits. Curr. Opin. Neurobiol. 41, 8–16. doi: 10.1016/j.conb.2016.07.004
Kawashima S., Amida A., Ikeda Y. (2019). Preliminary report of specific behaviours of juvenile greater blue-ringed octopus hapalochlaena lunulata (Quoy and gaimard 1832). Molluscan Res. 39, 291–295. doi: 10.1080/13235818.2019.1645633
Kier W. M. (2016). The musculature of coleoid cephalopod arms and tentacles. Front. Cell Dev. Biol. 4, 10. doi: 10.3389/fcell.2016.00010
Klug C., Kröger B., Kiessling W., Mullins G. L., Servais T., Frýda J., et al. (2010). The Devonian nekton revolution. Lethaia 43, 465–477. doi: 10.1111/j.1502-3931.2009.00206.x
Klug C., Schweigert G., Fuchs D., Kruta I., Tischlinger H. (2016). Adaptations to squid-style high-speed swimming in Jurassic belemnitids. Biol. Lett. 12, 20150877. doi: 10.1098/rsbl.2015.0877
Kodandaramaiah U., Vallin A., Wiklund C. (2009). Fixed eyespot display in a butterfly thwarts attacking birds. Anim. Behav. 77, 1415–1419. doi: 10.1016/j.anbehav.2009.02.018
Kooyman G. L., Davis R. W., Croxall J. P., Costa D. P. (1982). Diving depths and energy requirements of king penguins. Science 217, 726–727. doi: 10.1126/science.7100916
Kröger B. (2005). Adaptive evolution in Paleozoic coiled cephalopods. Paleobiology 31, 253–268. doi: 10.1666/0094-8373(2005)031[0253:AEIPCC]2.0.CO;2
Kröger R. H. H., Gislén A. (2004). Compensation for longitudinal chromatic aberration in the eye of the firefly squid, watasenia scintillans. Vision Res. 44, 2129–2134. doi: 10.1016/j.visres.2004.04.004
Kröger B., Vinther J., Fuchs D. (2011). Cephalopod origin and evolution: A congruent picture emerging from fossils, development and molecules. BioEssays 33, 602–613. doi: 10.1002/bies.201100001
Landing E., Kröger B. (2009). The oldest cephalopods from east laurentia. J. Paleontol. 83, 123–127. doi: 10.1666/08-078R.1
Langerhans R. B. (2007). “Evolutionary consequences of predation: avoidance, escape, reproduction, and diversification,” in Predation in organisms (Berlin, Heidelberg: Springer Berlin Heidelberg), 177–220.
Langridge K. V. (2009). Cuttlefish use startle displays, but not against large predators. Anim. Behav. 77, 847–856. doi: 10.1016/j.anbehav.2008.11.023
Levy G., Flash T., Hochner B. (2015). Arm coordination in octopus crawling involves unique motor control strategies. Curr. Biol. 25, 1195–1200. doi: 10.1016/j.cub.2015.02.064
Lewin H. A., Richards S., Aiden E. L., Allende M. L., Archibald J. M., Bálint M., et al. (2022). The earth BioGenome project 2020: Starting the clock. Proc. Natl. Acad. Sci. U. S. A. 119, e2115635118. doi: 10.1073/pnas.2115635118
Lewy Z. (2002). The function of the ammonite fluted septal margins. J. Paleontol. 76, 63–69. doi: 10.1666/0022-3360(2002)076<0063:TFOTAF>2.0.CO;2
Lindsay D. J., Hunt J. C., McNeil M., Beaman R. J., Vecchione M. (2020). The first In situ observation of the ram’s horn squid spirula spirula turns “Common knowledge” upside down. Divers. 2020 12, 449. doi: 10.3390/D12120449
Lin C.-Y., Tsai Y.-C., Chiao C.-C. (2017). Quantitative analysis of dynamic body patterning reveals the grammar of visual signals during the reproductive behavior of the oval squid sepioteuthis lessoniana. Front. Ecol. Evol. 5, 30. doi: 10.3389/fevo.2017.00030
Lowell R. B., Fletcher C. R., Grahame J., Mill P. J. (1994). Ontogeny of shell morphology and shell strength of the marine snails Littorina obtusata and Littorina mariae: different defence strategies in a pair of sympatric, sibling species. J. Zool. 234, 149–164. doi: 10.1111/j.1469-7998.1994.tb06062.x
MacGinitie G. E., MacGinitie N. (1968). Natural history of marine animals. 2d ed (McGraw-Hill, New York NY).
Macia S., Robinson M. P., Craze P., Dalton R., Thomas J. D. (2004). New observations on airborne jet propulsion (flight) in squid, with a review of previous reports. J. Molluscan Stud. 70, 297–299. doi: 10.1093/mollus/70.3.297
Marini G., De Sio F., Ponte G., Fiorito G. (2016). “Behavioral analysis of learning and memory in cephalopods,” in The curated reference collection in neuroscience and biobehavioral psychology (Academic Press, Cambridge, MA), 441–462.
Marshall N. J., Messenger J. B. (1996). Colour-blind camouflage. Nature. 382, 408–409. doi: 10.1038/382408b0
Mather J. A. (1994). ‘Home’ choice and modification by juvenile Octopus vulgaris (Mollusca: Cephalopoda): specialized intelligence and tool use? J. Zool. 233, 359–368. doi: 10.1111/j.1469-7998.1994.tb05270.x
Mather J. A. (1998). How do octopuses use their arms? J. Comp. Psychol. 112, 306–316. doi: 10.1037/0735-7036.112.3.306
Mather J. A., Dickel L. (2017). Cephalopod complex cognition. Curr. Opin. Behav. Sci. 16, 131–137. doi: 10.1016/j.cobeha.2017.06.008
Mäthger L. M., Denton E. J., Marshall N. J., Hanlon R. T. (2009). Mechanisms and behavioural functions of structural coloration in cephalopods. J. R. Soc Interface.
Mäthger L. M., Hanlon R. T. (2012). Pigmentation or transparency? camouflage tactics in deep-sea cephalopods. Pigment Cell Melanoma Res. 25, 295–296. doi: 10.1111/j.1755-148X.2012.00985.x
McCleneghan K., Ames J. A. (1976). A unique method of prey capture by a Sea otter, enhydra lutris. J. Mammal. 57, 410–412. doi: 10.2307/1379710
Mcfall-Ngai M. J. (1990). Crypsis in the pelagic environment. Integr. Comp. Biol. 30, 175–188. doi: 10.1093/icb/30.1.175
Meisel D. V., Byrne R. A., Kuba M., Griebel U., Mather J. A. (2003). Circadian rhythms in octopus vulgaris. Berliner Paläobiol. Abh 03, 171–177.
Meisel D. V., Byrne R. A., Kuba M., Mather J., Ploberger W., Reschenhofer E. (2006). Contrasting activity patterns of two related octopus species, octopus macropus and octopus vulgaris. J. Comp. Psychol. 120, 191–197. doi: 10.1037/0735-7036.120.3.191
Messenger J. B. (1977). Evidence that octopus is colourblind. J. Exp. Biol. 70, 49–55. doi: 10.1242/jeb.70.1.49
Messenger J. B. (2009). Reflecting elements in cephalopod skin and their importance for camouflage. J. Zool. 174, 387–395. doi: 10.1111/j.1469-7998.1974.tb03166.x
Meyer W. T. (1913). Tintenfische; mit besonderer berücksichtigung von sepia und octopus, von dr. Werner th. Meyer … mit einer farbigen tafel und 81 abbildungen im text. tintenfische; mit bes. berücksichtigung von sepia und octopus, von dr. Werner th. Meyer … mit einer farbigen tafel und 81 abbildungen im text (Leipzig: Dr. Werner Klinkhardt).
Meyer M., Smale M. J. (1991). Predation patterns of demersal teleosts from the cape south and west coasts of south africa. 1. pelagic predators. South Afr. J. Mar. Sci. 10, 173–191. doi: 10.2989/02577619109504630
Mezrai N., Arduini L., Dickel L., Chiao C.-C., Darmaillacq A.-S. (2020). Awareness of danger inside the egg: Evidence of innate and learned predator recognition in cuttlefish embryos. Learn. Behav. 48, 401–410. doi: 10.3758/s13420-020-00424-7
Mollo E., Amodeo P., Ghiselin M. T. (2019). Can intelligence gradually evolve in a shell? Trends Ecol. Evol. 34, 689–690. doi: 10.1016/j.tree.2019.04.014
Morse P., Huffard C. L. (2022). Chemotactile social recognition in the blue-ringed octopus, hapalochlaena maculosa. Mar. Biol. 169, 99. doi: 10.1007/s00227-022-04087-y
Mouritsen O. G., Styrbæk K. (2018). Cephalopod gastronomy - a promise for the future. Front. Commun. 3, 38. doi: 10.3389/fcomm.2018.00038
Moynihan M. (1983). Notes on the behavior of idiosepius pygmaeus (Cephalopoda; idiosepiidae). Behaviour 85, 42–57. doi: 10.1163/156853983X00039
Moynihan M., Rodaniche A. F. (1982). The behavior and natural history of the Caribbean reef squid sepioteuthis sepioidea with a consideration of social, signal, and defensive patterns for difficult and dangerous environments. Adv. Ethol. 25, 1–151.
Murali G., Kumari K., Kodandaramaiah U. (2019). Dynamic colour change and the confusion effect against predation. Sci. Rep. 9, 274. doi: 10.1038/s41598-018-36541-7
Muramatsu K., Yamamoto J., Abe T., Sekiguchi K., Hoshi N., Sakurai Y. (2013). Oceanic squid do fly. Mar. Biol. 160 (5), 1171–1175. doi: 10.1007/s00227-013-2169-9
Nakajima R., Ikeda Y. (2017). A catalog of the chromatic, postural, and locomotor behaviors of the pharaoh cuttlefish (Sepia pharaonis) from Okinawa island, Japan. Mar. Biodivers. 47, 735–753. doi: 10.1007/s12526-017-0649-8
Near T. J., Eytan R. I., Dornburg A., Kuhn K. L., Moore J. A., Davis M. P., et al. (2012). Resolution of ray-finned fish phylogeny and timing of diversification. Proc. Natl. Acad. Sci. U. S. A. 109, 13698–13703. doi: 10.1073/pnas.1206625109
Nilsson D. E., Warrant E. J., Johnsen S., Hanlon R., Shashar N. (2012). A unique advantage for giant eyes in giant squid. Curr. Biol. 22, 683–688. doi: 10.1016/j.cub.2012.02.031
Norman M. D., Finn J., Tregenza T. (2001). Dynamic mimicry in an indo-Malayan octopus. Proceedings of the Royal Society B: Biological Sciences 268, 1755–1758. doi: 10.1098/rspb.2001.1708
Norman M. D., Paul D., Finn J., Tregenza T. (2002). First encounter with a live male blanket octopus: The world’s most sexually size-dimorphic large animal. New Zeal. J. Mar. Freshw. Res. 36, 733–736. doi: 10.1080/00288330.2002.9517126
Nunes J. D. A. C. C., Chaves L. D. C. T., Maia-Nogueira R., Sampaio C. L. S. (2007). Association between juvenile reef fish and the Caribbean reef squid sepioteuthis sepioidea on north-eastern Brazilian coastal reefs. J. Mar. Biol. Assoc. Unite. Kingdom 87, 761–762. doi: 10.1017/S0025315407055130
O’Brien C. E., Roumbedakis K., Winkelmann I. E. (2018). The current state of cephalopod science and perspectives on the most critical challenges ahead from three early-career researchers. Front. Physiol. 9, 700. doi: 10.3389/fphys.2018.00700
O’Dor R. K. (1988). Limitations on locomotor performance in squid. J. Appl. Physiol. 64, 128–134. doi: 10.1152/jappl.1988.64.1.128
O’Dor R. (2002). “Telemetered cephalopod energetics: Swimming, soaring, and blimping,” in Integrative and comparative biology (Society for Integrative and Comparative Biology) 42, 1065–1070. doi: 10.1093/icb/42.5.1065
O’Dor R. K., Webber D. M. (1986). The constraints on cephalopods: why squid aren’t fish. Can. J. Zool. 64, 1591–1605. doi: 10.1139/z86-241
Okamoto K., Yasumuro H., Mori A., Ikeda Y. (2017). Unique arm-flapping behavior of the pharaoh cuttlefish, sepia pharaonis: putative mimicry of a hermit crab. J. Ethol. 35, 307–311. doi: 10.1007/s10164-017-0519-7
Osorio D. (2014). Cephalopod behaviour: Skin flicks. Curr. Biol. 24, R684–R685. doi: 10.1016/j.cub.2014.06.066
Osorio D., Ham A. D. (2002). Spectral reflectance and directional properties of structural coloration in bird plumage. J. Exp. Biol. 205, 2017–2027. doi: 10.1242/jeb.205.14.2017
Packard A. (1972). Cephalopods and fish: The limits of convergence. Biol. Rev. 47, 241–307. doi: 10.1111/j.1469-185X.1972.tb00975.x
Packard A., Wurtz M. (1994). An octopus, ocythoe, with a swimbladder and triple jets. Philos. Trans. R. Soc London. Ser. B Biol. Sci. 344, 261–275. doi: 10.1098/rstb.1994.0065
Palmer A. R. (1979). Fish predation and the evolution of gastropod shell sculpture: Experimental and geographic evidence. Evol. (N. Y). 33, 697. doi: 10.2307/2407792
Palmer M. E., Calvé M. R., Adamo S. A. (2006). Response of female cuttlefish sepia officinalis (Cephalopoda) to mirrors and conspecifics: Evidence for signaling in female cuttlefish. Anim. Cogn. 9, 151–155. doi: 10.1007/s10071-005-0009-0
Panetta D., Buresch K., Hanlon R. T. (2017). Dynamic masquerade with morphing three-dimensional skin in cuttlefish. Biol. Lett. 13, 20170070. doi: 10.1098/rsbl.2017.0070
Papastamatiou Y. P., Verbeck D., Hutchinson M., Bracken-Grissom H. D., Chapman D. (2020). An encounter between a pelagic shark and giant cephalopod. J. Fish Biol. 97, 588–589. doi: 10.1111/jfb.14415
Pembury Smith M. Q. R., Ruxton G. D. (2020). Camouflage in predators. Biol. Rev. 95, 1325–1340. doi: 10.1111/brv.12612
Pérez-Claros J. A., Olóriz F., Palmqvist P. (2007). Sutural complexity in late Jurassic ammonites and its relationship with phragmocone size and shape: A multidimensional approach using fractal analysis. Lethaia 40, 253–272. doi: 10.1111/j.1502-3931.2007.00022.x
Pike T. W. (2015). Interference coloration as an anti-predator defence. Biol. Lett. 11, 20150159. doi: 10.1098/rsbl.2015.0159
Ridley A. R., Nelson-Flower M. J., Thompson A. M. (2013). Is sentinel behaviour safe? an experimental investigation. Anim. Behav. 85, 137–142. doi: 10.1016/j.anbehav.2012.10.017
Robison B. H., Reisenbichler K. R., Hunt J. C., Haddock S. H. D. (2003). Light production by the arm tips of the deep-Sea cephalopod vampyroteuthis infernalis. Biol. Bull. 205, 102–109. doi: 10.2307/1543231
Roper C. F. E., Boss K. J. (1982). The giant squid. Scientific American 246, 96–105. doi: 10.1038/scientificamerican0482-96
Roper C. F. E., Hochberg F. G. (1988). Behaviour and systematics of cephalopods from lizard island, Australia, based on color and body patterns. Malacologia 29, 153–193.
Rosa R., Lopes V. M., Guerreiro M., Bolstad K., Xavier J. C. (2017). Biology and ecology of the world’s largest invertebrate, the colossal squid (Mesonychoteuthis hamiltoni): a short review. Polar Biol. 40, 1871–1883. doi: 10.1007/s00300-017-2104-5
Rosati A. G. (2017). Foraging cognition: Reviving the ecological intelligence hypothesis. Trends Cogn. Sci. 21, 691–702. doi: 10.1016/j.tics.2017.05.011
Royer J., Santos M. B., Cho S. K., Stowasser G., Pierce G. J., Daly H. I., et al. (1998). Cephalopod consumption by fish in English channel and Scottish waters. Int. Counc. Explor. Sea Impact Cephalopods Food Chain Their Interact. with Environ. 23, Routledge, Oxford UK
Sampaio E., Ramos C. S., Bernardino B. L. M., Bleunven M., Augustin M. L., Moura É., et al. (2020). Neurally underdeveloped cuttlefish newborns exhibit social learning. Anim. Cogn. 24, 23–32. doi: 10.1007/s10071-020-01411-1
Sanchez G., Setiamarga D. H. E., Tuanapaya S., Tongtherm K., Winkelmann I. E., Schmidbaur H., et al. (2018). Genus-level phylogeny of cephalopods using molecular markers: Current status and problematic areas. PeerJ 2018, e4331. doi: 10.7717/peerj.4331
Schaffeld T., Bräger S., Gallus A., Dähne M., Krügel K., Herrmann A., et al. (2016). Diel and seasonal patterns in acoustic presence and foraging behaviour of free-ranging harbour porpoises. Mar. Ecol. Prog. Ser. 547, 257–272. doi: 10.3354/meps11627
Schnell A. K., Amodio P., Boeckle M., Clayton N. S. (2020). How intelligent is a cephalopod? lessons from comparative cognition. Biol. Rev. 96, 162–178. doi: 10.1111/brv.12651
Schnell A. K., Clayton N. S. (2019). Cephalopod cognition. Curr. Biol. 29, R726–R732. doi: 10.1016/j.cub.2019.06.049
Schnell A. K., Clayton N. S. (2021). Cephalopods: Ambassadors for rethinking cognition. Biochem. Biophys. Res. Commun. 564, 27–36. doi: 10.1016/j.bbrc.2020.12.062
Scott W. B., Tibbo S. N. (1968). Food and feeding habits of swordfish, xiphias gladius, in the Western north Atlantic. J. Fish. Res. Board Canada 25, 903–919. doi: 10.1139/f68-084
Searcy W. A., Nowicki S. (2019). Birdsong learning, avian cognition and the evolution of language. Anim. Behav. 151, 217–227. doi: 10.1016/j.anbehav.2019.01.015
Seibel B. A. (2016). Cephalopod susceptibility to asphyxiation via ocean incalescence, deoxygenation, and acidification. Physiology 31, 418–429. doi: 10.1152/physiol.00061.2015
Seidou M., Sugahara M., Uchiyama H., Hiraki K., Hamanaka T., Michinomae M., et al. (1990). On the three visual pigments in the retina of the firefly squid, watasenia scintillans. J. Comp. Physiol. A 166, 769–773. doi: 10.1007/BF00187321
Shaw T. J., Osborne M., Ponte G., Fiorito G., Andrews P. L. R. (2016). Mechanisms of wound closure following acute arm injury in octopus vulgaris. Zool. Lett. 2, 1–10. doi: 10.1186/s40851-016-0044-5
Shears J. (1988). The use of a sand coat in relation to feeding and diel activity in the sepiolid squid euprymna scolopes. Malacologia 29, 121–133.
Sherrard K. M. (2000). Cuttlebone morphology limits habitat depth in eleven species of sepia (Cephalopoda: Sepiidae). Biol. Bull. 198, 404–414. doi: 10.2307/1542696
Shigeno S., Andrews P. L. R., Ponte G., Fiorito G. (2018). Cephalopod brains: An overview of current knowledge to facilitate comparison with vertebrates. Front. Physiol. 9, 952. doi: 10.3389/fphys.2018.00952
Smale M. J. (1996). Cephalopods as prey. IV. fishes. Philos. Trans. R. Soc B Biol. Sci. 351, 1067–1081. doi: 10.1098/rstb.1996.0094
Staudinger M. D., Hanlon R. T., Juanes F. (2011). Primary and secondary defences of squid to cruising and ambush fish predators: Variable tactics and their survival value. Anim. Behav. 81, 585–594. doi: 10.1016/j.anbehav.2010.12.002
Staudinger M. D., Juanes F., Salmon B., Teffer A. K. (2013). The distribution, diversity, and importance of cephalopods in top predator diets from offshore habitats of the Northwest Atlantic ocean. Deep. Res. Part II Top. Stud. Oceanogr. 95, 182–192. doi: 10.1016/j.dsr2.2012.06.004
Stevens M. (2005). The role of eyespots as anti-predator mechanisms, principally demonstrated in the Lepidoptera. Biol. Rev. Camb. Philos. Soc. 80, 573–588. doi: 10.1017/S1464793105006810
Stevens J. D., Cuthbert G. J. (1983). Observations on the identification and biology of hemigaleus (Selachii: Carcharhinidae) from Australian waters. Copeia 1983, 487. doi: 10.2307/1444394
Stevens M., Merilaita S. (2009). Animal camouflage: Current issues and new perspectives. Philos. Trans. R. Soc B Biol. Sci. 364, 423–427. doi: 10.1098/rstb.2008.0217
Stevens M., Ruxton G. D. (2014). Do animal eyespots really mimic eyes? Curr. Zool. 60, 26–36. doi: 10.1093/czoolo/60.1.26
Stubbs A. L., Stubbs C. W. (2016a). Reply to gagnon et al.: All color vision is more difficult in turbid water. Proc. Natl. Acad. Sci. 113, E6910–E6910. doi: 10.1073/pnas.1614994113
Stubbs A. L., Stubbs C. W. (2016b). Spectral discrimination in color blind animals via chromatic aberration and pupil shape. Proc. Natl. Acad. Sci. 113, 8206–8211. doi: 10.1073/pnas.1524578113
Sumbre G., Gutfreund Y., Fiorito G., Flash T., Hochner B. (2001). Control of octopus arm extension by a peripheral motor program. Sci. (80-.). 293, 1845–1848. doi: 10.1126/science.1060976
Sutherland R. L., Mäthger L. M., Hanlon R. T., Urbas A. M., Stone M. O. (2008a). Cephalopod coloration model I squid chromatophores and iridophores. J. Opt. Soc Am. A 25, 588. doi: 10.1364/JOSAA.25.000588
Sutherland R. L., Mäthger L. M., Hanlon R. T., Urbas A. M., Stone M. O. (2008b). Cephalopod coloration model II multiple layer skin effects. J. Opt. Soc Am. A 25, 2044. doi: 10.1364/JOSAA.25.002044
Sweeney A. M., Haddock S. H. D., Johnsen S. (2007). Comparative visual acuity of coleoid cephalopods. Integr. Comp. Biol. 47, 808–814. doi: 10.1093/icb/icm092
Tanner A. R., Fuchs D., Winkelmann I. E., Gilbert M. T. P., Pankey M. S., Ribeiro Â. M., et al. (2017). Molecular clocks indicate turnover and diversification of modern coleoid cephalopods during the mesozoic marine revolution. Proc. R. Soc B Biol. Sci. 284, 20162818. doi: 10.1098/rspb.2016.2818
Tressler J., Maddox F., Goodwin E., Zhang Z., Tublitz N. J. (2014). Arm regeneration in two species of cuttlefish sepia officinalis and sepia pharaonis. Invertebr. Neurosci. 14, 37–49. doi: 10.1007/s10158-013-0159-8
Tricarico E., Borrelli L., Gherardi F., Fiorito G. (2011). I Know my neighbour: Individual recognition in octopus vulgaris. PloS One 6, e18710. doi: 10.1371/journal.pone.0018710
Umbers K. D. L., De Bona S., White T. E., Lehtonen J., Mappes J., Endler J. A. (2017). Deimatism: A neglected component of anti-predator defence. Biol. Lett. 13, 20160936. doi: 10.1098/rsbl.2016.0936
Uyeno T. A., Kier W. M. (2007). Electromyography of the buccal musculature of octopus (Octopus bimaculoides): A test of the function of the muscle articulation in support and movement. J. Exp. Biol. 210, 118–128. doi: 10.1242/jeb.02600
van der Bijl W., Kolm N. (2016). Why direct effects of predation complicate the social brain hypothesis. BioEssays 38, 568–577. doi: 10.1002/bies.201500166
van Elden S., Meeuwig J. J. (2020). Wild observation of putative dynamic decapod mimicry by a cuttlefish (Sepia cf. smithi). Mar. Biodivers. 50, 1–6. doi: 10.1007/s12526-020-01117-0
Van Lawick-Goodall J. (1971). Tool-using in primates and other vertebrates. Adv. Stud. Behav. 3, 195–249. doi: 10.1016/S0065-3454(08)60157-6
Vannier J., Steiner M., Renvoisé E., Hu S. X., Casanova J. P. (2006). Early Cambrian origin of modern food webs: evidence from predator arrow worms. Proc. R. Soc B Biol. Sci. 274, 627–633. doi: 10.1098/rspb.2006.3761
Vermeij G. J. (1977). The mesozoic marine revolution: Evidence from snails, predators and grazers. Paleobiology 3, 245–258. doi: 10.1017/S0094837300005352
Vermeij G. J. (1978). Biogeography and adaptation: patterns of marine life (Harvard University Press). 3, 245–258. doi: 10.1017/S0094837300005352
Villanueva R., Laptikhovsky V. V., Piertney S. B., Fernández-Álvarez F. Á., Collins M. A., Ablett J. D., et al. (2020). Extended pelagic life in a bathybenthic octopus. Front. Mar. Sci. 7, 1009. doi: 10.3389/fmars.2020.561125
Villanueva R., Perricone V., Fiorito G. (2017). Cephalopods as predators: A short journey among behavioral flexibilities, adaptions, and feeding habits. Front. Physiol. 8, 598. doi: 10.3389/fphys.2017.00598
Walcott C. D. (1905). Cambrian Faunas of China. Proc. Unite. States Natl. Museum 29, 1–106. doi: 10.5479/si.00963801.1415
Webber D. M., O’Dor R. K. (1985). Respiration and swimming performance of short-finned squid (Illex illecebrosus). NAFO Sci. Coun. Stud. 9, 133–138.
Webers G. F., Yochelson E. L. (1989). Late Cambrian molluscan faunas and the origin of the Cephalopoda. Geol. Soc Spec. Publ. 47, 29–42. doi: 10.1144/GSL.SP.1989.047.01.04
Wells M. J. (1990). Oxygen extraction and jet propulsion in cephalopods. Can. J. Zool. 68, 815–824. doi: 10.1139/z90-117
Wells M. J. (1992). The cephalopod heart: The evolution of a high-performance invertebrate pump. Experientia. 48, 800–808. doi: 10.1007/BF02118412
Wells M. J., O’Dor R. K. (1991). Jet propulsion and the evolution of the cephalopods. Bull. Mar. Sci. 49, 419–432.
Whitelaw B. L., Cooke I. R., Finn J., Zenger K., Strugnell J. M. (2019). The evolution and origin of tetrodotoxin acquisition in the blue-ringed octopus (genus hapalochlaena). Aquat. Toxicol. 206, 114–122. doi: 10.1016/j.aquatox.2018.10.012
Williams H., Lachlan R. F. (2022). Evidence for cumulative cultural evolution in bird song. Philos. Trans. R. Soc B 377, 20200322. doi: 10.1098/rstb.2020.0322
Willmer P., Stone G., Johnston I. A. (2009). Environmental physiology of animals. 2nd edn (John Wiley and Sons, Ltd, Hoboken, NJ).
Wood J. B., Maynard A. E., Lawlor A. G., Sawyer E. K., Simmons D. M., Pennoyer K. E., et al. (2010). Caribbean Reef squid, sepioteuthis sepioidea, use ink as a defense against predatory French grunts, haemulon flavolineatum. J. Exp. Mar. Bio. Ecol. 388, 20–27. doi: 10.1016/j.jembe.2010.03.010
Wood J. B., Pennoyer K. E., Derby C. D. (2008). Ink is a conspecific alarm cue in the Caribbean reef squid, sepioteuthis sepioidea. J. Exp. Mar. Bio. Ecol. 367, 11–16. doi: 10.1016/j.jembe.2008.08.004
Wood F. D., Wood H. E. (1932). Autotomy in decapod Crustacea. J. Exp. Zool. 62, 1–55. doi: 10.1002/jez.1400620102
Yochelson E. L., Flower R. H., Webers G. F. (1973). The bearing of the new late Cambrian monoplacophoran genus knightoconus upon the origin of the Cephalopoda. Lethaia 6, 275–309. doi: 10.1111/j.1502-3931.1973.tb01199.x
Young J. Z. (1938). The functioning of the giant nerve fibres of the squid. J. Exp. Biol. 15, 170–185. doi: 10.1242/jeb.15.2.170
Young J. Z. (1965). The central nervous system of nautilus. Philos. Trans. R. Soc Lond. B. Biol. Sci. 249, 27–44. doi: 10.1098/RSTB.1965.0007
Young J. Z. (1971). Anatomy of the nervous system of octopus vulgaris (Oxford University Press, Oxford UK).
Young J. Z. (1988). “Evolution of the cephalopod brain,” in Paleontology and neontology of cephalopods Academic Press, Cambridge, MA. 215–228. doi: 10.1016/B978-0-12-751412-3.50019-X
Young R. E., Vecchione M., Donovan D. T. (1998). The evolution of coleoid cephalopods and their present biodiversity and ecology | African journal of marine science. Afr. J. Mar. Sci. 20, 393–420.
Zullo L., Eichenstein H., Maiole F., Hochner B. (2019). Motor control pathways in the nervous system of octopus vulgaris arm. J. Comp. Physiol. A Neuroethol. Sens. Neural Behav. Physiol. 2, 271–279. doi: 10.1007/s00359-019-01332-6
Zylinski S., Osorio D. (2011). “What can camouflage tell us about non-human visual perception? a case study of multiple cue use in cuttlefish (Sepia spp.),” in Animal camouflage: Mechanisms and function. Eds. Stevens M., Merilaita S. (Cambridge University Press, Cambridge UK), 164–185.
Keywords: squid, octopus, cuttlefish, prey, anti-predator, evolution, Cephalopoda
Citation: Jaitly R, Ehrnsten E, Hedlund J, Cant M, Lehmann P and Hayward A (2022) The evolution of predator avoidance in cephalopods: A case of brain over brawn? Front. Mar. Sci. 9:909192. doi: 10.3389/fmars.2022.909192
Received: 31 March 2022; Accepted: 31 August 2022;
Published: 23 September 2022.
Edited by:
Graziano Fiorito, Stazione Zoologica Anton Dohrn, ItalyReviewed by:
Yuzuru Ikeda, University of the Ryukyus, JapanFrancesca Raffini, University of Ferrara, Italy
Copyright © 2022 Jaitly, Ehrnsten, Hedlund, Cant, Lehmann and Hayward. This is an open-access article distributed under the terms of the Creative Commons Attribution License (CC BY). The use, distribution or reproduction in other forums is permitted, provided the original author(s) and the copyright owner(s) are credited and that the original publication in this journal is cited, in accordance with accepted academic practice. No use, distribution or reproduction is permitted which does not comply with these terms.
*Correspondence: Alexander Hayward, alex.hayward@exeter.ac.uk