Climate-driven changes to taste and aroma determining metabolites in an economically valuable portunid (Portunus armatus) have implications for future harvesting
- 1Fisheries Research, NSW Department of Primary Industries, Coffs Harbour, NSW, Australia
- 2National Marine Science Centre, Southern Cross University, Coffs Harbour, NSW, Australia
- 3Commonwealth Scientific and Industrial Research Organisation (CSIRO) Agriculture and Food, North Ryde, NSW, Australia
- 4Centre for Advanced Food Engineering, The University of Sydney, Darlington, NSW, Australia
- 5CSIRO Land and Water, Canberra, ACT, Australia
The effects of climate change on the distribution and biology of fisheries species have received substantial attention, but quantitative assessments of changes to taste and aroma determining compounds remain limited—despite sensory quality being a key driver of demand for most harvested species. Utilising the economically important blue swimmer crab (Portunus armatus), we tested the effects of temperature and salinity treatments aligned with near-future climate change projections on volatile and non-volatile sensory compounds that determine seafood flavour. Volatile compounds were analysed using solid phase microextraction and gas chromatography-mass spectrometry and non-volatiles were identified using liquid chromatography (mass spectrometry analysis). Multivariate analyses revealed that temperature, but not salinity, significantly affected the compositions of both volatile and non-volatile compounds in crab meat following a 30-day exposure period. Univariate analyses highlighted significant reductions in amino acids and amines associated with bitter and sweet organoleptic properties following exposure to elevated temperature. These results imply the potential for climate change to alter taste and aroma determining compounds in seafood, which could affect future harvesting priorities. Assessments of seafood sensory quality under climate change can produce valuable information to help predict shifts in fishing effort for harvested species that form the basis of important global fisheries.
Introduction
The impacts of climate change on harvested marine resources are evident throughout the global ocean (Weatherdon et al., 2016; Free et al., 2019). Climate-driven changes to species geographic distributions (Pinsky et al., 2019; Gervais et al., 2021), phenology (Poloczanska et al., 2013) and temporal persistence within fishing regions (Champion et al., 2019; Rogers et al., 2019) presently challenge the sustainable harvest of seafood and the economic viability of fisheries (Sumaila et al., 2011). In addition to impacting the sustainable and profitable harvest of marine resources, studies suggest the environmental effects of climate change can also alter the quality of seafood, including nutritional (e.g. protein and fatty acids) and sensory properties (e.g. metabolites that contribute to flavour) (Dupont et al., 2014; Tate et al., 2017; Ab Lah et al., 2018; Maire et al., 2021). Given the large and growing global dependence on seafood (Gerland et al., 2014; FAO, 2020), understanding the effects of climate change on seafood quality is a priority for climate-impact research that intersects biological, economic and social issues.
The health benefits that humans derive from consuming bioavailable nutrients contained within seafood are immense (Lund, 2013). For example, seafood accounts for approximately 17% of the total animal protein consumed globally (FAO, 2020). Further, micronutrients (e.g. vitamin A, iron, calcium and zinc) essential for cognitive function and hormone regulation are highly concentrated in seafood, which is particularly important for many developing coastal regions that rely on ocean harvests (Hicks et al., 2019). Given the provision of nutrients through seafood is integral to the future of human health globally (Golden et al., 2021), it is concerning that some studies have noted the nutritional quality of seafood may be vulnerable to climate change (Valles-Regino et al., 2015; Lemasson et al., 2019; Alma et al., 2020; Lan et al., 2020). Also concerning is the potential for seafood sensory quality (determined by attributes such as taste, smell and texture) to be affected by climate-driven environmental change, considering sensory attributes contribute to consumer choices regarding seafood consumption and, therefore, the derived health benefits.
Despite the crucial role that sensory quality plays in seafood consumer appeal, studies testing the effects of climate-driven environmental change on seafood flavour remain limited. Of those that have, results vary among the species studied, environmental factors tested and exposure durations assessed. For example, simulated ocean acidification significantly reduced the taste and appearance of northern shrimp (Pandalus borealis), with individuals exposed to lowered pH more likely to score lower in sensory quality assessments than those exposed to higher pH (Dupont et al., 2014). In the context of climate-driven extreme events, simulated marine heatwaves were found to significantly reduce the moisture content of the harvested gastropod Turbo militaris, which could compromise the desirable meat texture (Mamo et al., 2019). Conversely, exposure to climate-driven environmental change can improve the sensory quality of seafood. For example, Ran et al. (2019) found that volatile compounds pertaining to the aroma of both raw and cooked Chinese razor clams (Sinonovacula constricta) significantly improved following exposure to elevated salinity. There is also evidence for no detected effects of simulated climate change on seafood sensory properties, with the appearance, aroma and taste of the Pacific oyster (Magallana gigas) not significantly affected following exposure to ocean acidification and warming treatments (Lemasson et al., 2017). Collectively, these examples demonstrate that, for some seafood species, climate-driven environmental change has been found to have a various of effects on the sensory properties of seafood.
Volatile and non-volatile compounds underpin food sensory quality and represent valuable responses to assess when testing for environmentally induced changes to seafood aroma and taste (Wang et al., 2004; Sarower et al., 2012; Liu et al., 2018; Ran et al., 2019; Duan et al., 2021). Volatile organic compounds, comprised mainly of aldehydes, ketones, and alcohols, occur in gaseous form at room temperature and are therefore key determinates of seafood aroma (Frank et al., 2009). Non-volatile compounds are associated with relatively low vapour pressures and do not similarly evaporate. Therefore, non-volatile metabolites, such as free amino acids (FAAs), primarily determine the taste of seafood (Chen and Zhang, 2007; Liu et al., 2018; Zhang et al., 2019). When taste receptors sense non-volatile compounds, the combination and concentration of these metabolites determine the tastes associated with seafood, such as umami, sweetness, saltiness and bitterness (Chen and Zhang, 2007; Fuentes et al., 2010; Sarower et al., 2012). For example, FAAs play an important role in the umami taste of seafood, while sweetness (a desired trait of many harvested crustaceans) is determined by soluble sugars, including betaine and multiple AAs (e.g. threonine, serine, glycine, alanine, arginine and proline). In addition to being reliable indicators of seafood aroma and taste for quantifying any effects of climate change on seafood sensory quality, the analyses of volatile and non-volatile compounds also reduces limitations associated with sensory panel analyses, including access to adequately trained and calibrated panellists (Mabuchi et al., 2019).
Estuarine systems are vulnerable to the environmental effects of climate change (Scanes et al., 2020) due to their interconnectedness with regional oceanographic processes and terrestrial climate dynamics (Gillanders et al., 2011). Estuaries are crucial habitats that support the recruitment, growth and maturation of harvested marine resources (Beck et al., 2001). With >500 species globally, portunids are a key group of economically important species that recruit and mature within tropical and temperate estuaries (Kailola et al., 1993). In Australia, blue swimmer crabs (Portunus armatus) are the most economically important portunid, supporting a national harvest (commercial and recreational) of 2500 t per annum in the lower reaches of saline estuaries throughout subtropical and temperate latitudes. Given the Australian distribution of P. armatus encompasses two marine climate change hotspots that are warming at rates within the top 10% of coastal ocean regions globally (Hobday and Pecl, 2014), this species is ideal for investigating any potential effects of climate-induced changes to sensory compounds responsible for seafood aroma and taste.
We aimed to test if the concentration of volatile and non-volatile compounds pertaining to seafood aroma and taste significantly differed in P. armatus exposed to temperature and salinity treatments that align with near-future climate change projections, relative to individuals exposed to present-day environmental conditions. By testing the responses of compounds that determine decapod sensory quality to environmental treatments aligned with projected environmental change, we contribute to an emerging understanding of the likely impacts of climate change on seafood consumer appeal.
Material and methods
Portunus armatus collection
Portunus armatus were collected from Wallis Lake, New South Wales (NSW; 32.271°S, 152.471°E) using commercial round traps following Broadhurst et al. (2017). One-hundred live individuals were transported in an aerated fish transporter from Wallis Lake to the National Marine Science Centre (NMSC), Coffs Harbour, Australia. Intermoult crabs (determined following Hay et al. [2005]) with no physical damage were randomly selected as experimental replicates, with carapace length (CL; nearest mm) and mass (nearest 0.01 g) measured immediately prior to their acclimation and experimental exposure period.
Experimental design
Fifty-six polyvinyl chloride experimental tanks (15 l) were partitioned into an orthogonal combination of salinity (~35 and ~40 practical salinity units [PSU]) and temperature treatments (~21 and ~27°C; n = 14 tanks per treatment; Figure 1). Each tank contained one crab (to avoid competitive interactions) acclimated to experimental treatment levels over four days prior to a 30-day experimental exposure period. Treatment levels were strategically selected to reflect present-day environmental conditions experienced by P. armatus throughout their temperate Australian distribution and conditions predicted under near-future climate change (Gillanders et al., 2011; Hobday and Lough, 2011). Each experimental treatment contained an approximate 1:1 sex ratio. This experimental design was previously utilised to test for the effects of climate change on the nutritional quality (e.g. protein, lipids, and fatty acids) of P. armatus (Champion et al., 2020).
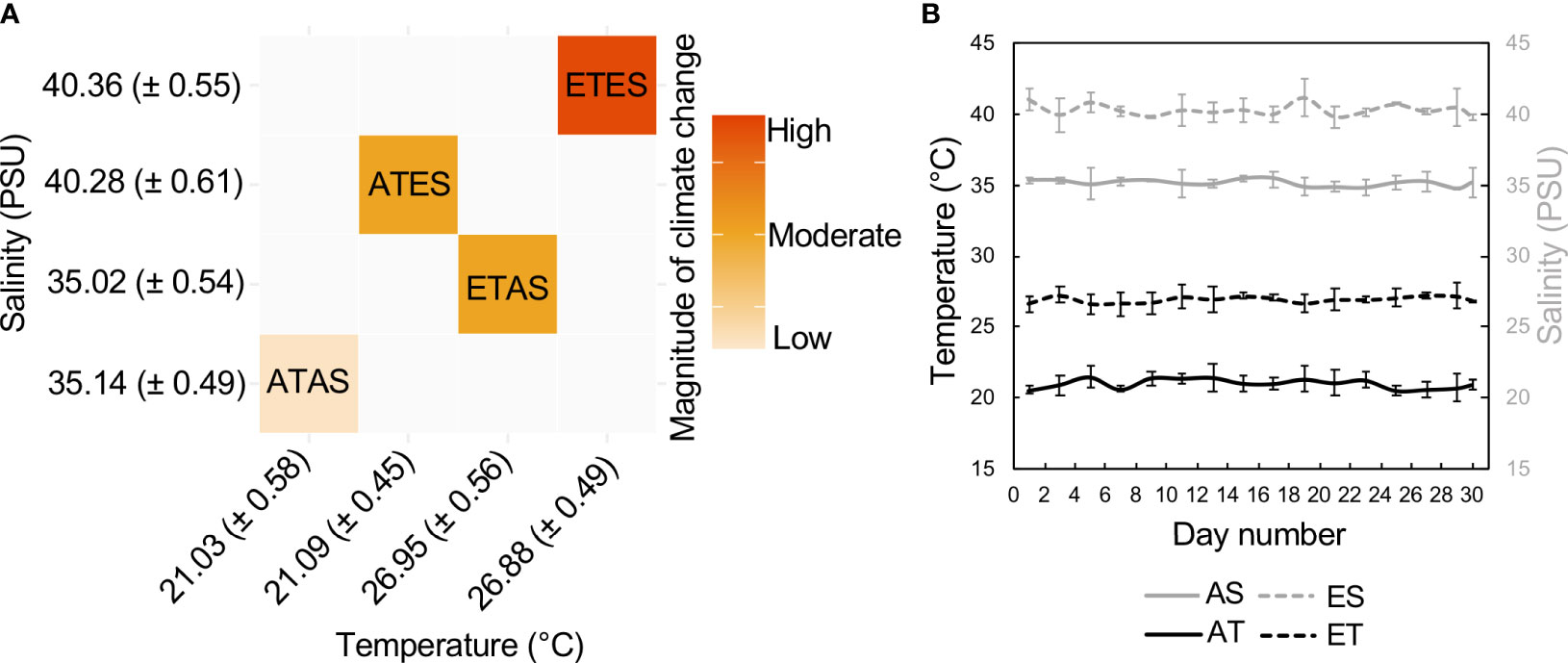
Figure 1 (A) Mean water temperature and salinity (± SD, n = 14 per treatment) of treatments during the 30-day experimental period. (B) Time-series of temperature and salinity levels measured every second day during the experiment (bars denote ± SD). Treatments range from a low magnitude climate change scenario to moderate and high magnitude scenarios, and are coded as follows herein: ATAS, ambient temperature and salinity; ATES, ambient temperature and elevated salinity; ETAS, elevated temperature and ambient salinity; and ETES, elevated temperature and salinity. Dissolved oxygen (mg l-1) was measured every second day and remained consistent among replicates during the experimental period: ATAS = 8.62 ( ± 0.34), ATES = 8.78 ( ± 0.40), ETAS = 8.46 ( ± 0.47), ETES = 8.88 ( ± 0.39).
Experimental maintenance
Flow-through seawater from the adjacent ocean was supplied directly to the experimental system and adjusted to treatment levels in four separate 400 l header tanks prior to entering experimental tanks at a rate of 0.1 l min–1. Water temperature was controlled by heater-chiller units (Aquahort Ltd., Omana Beach, New Zealand) in header tanks and temperature stabilising water baths that housed individual experimental tanks. Ambient salinity from incoming seawater was stable during the experiment (i.e. ~35 PSU; Figure 1). Elevated salinity representative of future estuarine conditions was achieved by supplying hypersaline seawater (created by dissolving aquarium-grade crystallised sea salt at 150 g l–1 [Cheetham Salt, South Australia]) from a sub-header tank into the primary header tanks that serviced relevant treatments.
During a one-week trial of the experimental system without live animals, heater-chiller units and the flow of hypersaline seawater into primary header tanks were adjusted and monitored to confirm stable treatment levels. Temperature and salinity within each experimental replicate were measured once every two days during the experiment using a TP20 digital thermometer (ThermPro, Toronto, Canada) and Vital Sine™ SR6 refractometer (Apopka, Florida, USA), respectively (Figure 1). The experiment took place in an enclosed room with a 12:12 h photoperiod. Throughout the experiment, P. armatus were fed school prawns (Metapenaeus macleayi) as per Uhlmann et al. (2009), with faeces routinely sieved from experimental tanks 14 h after feeding. The status of each crab (i.e. dead or alive) was monitored daily, after which individuals were euthanised by cutting along the ventral longitudinal midline, and the edible abdomen tissue (~20 g) was snap-frozen prior to volatile and non-volatile metabolite analyses.
Volatile metabolite analyses
Solid phase microextraction (SPME) and gas chromatography-mass spectrometry (GC-MS) were used to analyse volatile compounds from experimental replicates. Crab meat samples (~ 10 g) were thawed and homogenised using an Ultra-Turrax (IKA-works, Malaysia). A subsample (2 g) was homogenised with water at a ratio of 1:2 in a 20 ml glass headspace vial, and the slurry (3 g) was transferred into headspace glass vials with an internal standard (4-methyl-1-pentanol; 0.5 µg g-1). Headspace extraction of volatiles was conducted at 40°C for 60 min and desorbed into the GC injector in splitless mode at 240°C for 5 min. Divynylbenzene/carboxen/polydimethylsiloxane solid phase microextraction (SPME) fibres (23-gauge, 2 cm; Agilent Technologies, Bellefonte, USA) were used for the extraction.
Headspace analysis of samples was undertaken using SPME and GC-MS (Schimadzu QP-2010 Plus, Tokyo, Japan) and an auto-sampler (AOC-5000, Schimadzu). Compounds were separated on a Zebron-WAX column (length 30 m; I.D. 0.25 mm and thickness 0.5 µm; Phenomenex, Lane Cove West, Australia). The carrier gas was helium (flow rate = 1.04 ml min-1). The initial column temperature was held at 35°C for 5 min, then increased to 250°C at 5°C min–1 and held for 5 min. Detection of volatiles was performed in electron ionisation mode (EI), 70 eV over a mass range m/z 40-250. Kovats retention indices (RI), EI mass spectral library matches and, in most cases reference chemicals (RI), were used to identify volatile metabolites. Volatile data were semi-quantitated using the IS and expressed as µg g-1.
Non-volatile metabolite analyses
A sample of thawed homogenised crab meat (500 mg) was combined with 1 ml of 1:2 methanol:water solution and further homogenised for 10 min using a TissueLyser (Qiagen Retsch MM300, Haan, Germany). Samples were then centrifuged (Model 1-15, Sigma Laborzentrifugen, Germany) at 18,000 relative centrifugal force for 15 min. The supernatant was collected, and the residue re-extracted under the same conditions. The supernatants were pooled and filtered using a nylon filter (Phenex, 0.2 µm, Phenomenex, Lane Cove West, NSW, Australia).
Identification of non-volatile metabolites was achieved using liquid chromatography – mass spectrometry (LC-MS) analysis undertaken using a Dionex liquid chromatograph equipped with a pump, autosampler and heated column compartment (Ultimate 3000RS, Thermo Scientific, San Jose, CA, USA). Chromatographic separation of compounds was achieved using an Intrada Amino Acid column (length 150 mm x I.D. 3 mm; particle size 3 µm; Imtakt, Portland, OR, USA). The mobile phase consisted of acetonitrile/0.1% formic acid (solvent A) and 100 mM ammonium formate (solvent B). A constant flow rate of 0.6 ml min–1 was used with a gradient elusion program, with an initial gradient of 14% B held constant for 3 minutes before being increased to 100% B for 10 minutes. At 12.5 minutes the gradient was returned to 14% B. An injection volume of 5 µl was used.
Accurate mass measurement of non-volatile metabolites was conducted on a Q-Exactive Orbitrap LC-MS (Thermo Scientific, Scoresby, VIC, Australia) equipped with a heated electrospray ionization (H-ESI) source. The source conditions were as follows: spray voltage (positive ion 3.9 kV), sheath gas 60 (arbitrary units), auxiliary gas 10 (arbitrary units) and sweep gas 1 (arbitrary units), capillary temperature of 350°C and auxiliary gas heating temperature of 400°C. Mass spectra were acquired in positive ionisation mode and recorded over the mass range of 70 – 500 m/z at 70 000 resolution (Xcalibur 4.3, Thermo Fisher Scientific, USA). A data-dependant workflow was used to acquire MS/MS spectra using a stepped collision energy of 15, 30 and 100 eV in the HCD collision cell. The top five most abundant ions were selected for MS/MS scans with a dynamic exclusion of 10.0 seconds to prevent re-analysis. Compound Discoverer Ver 3.1 (Thermo Fisher Scientific, San Jose, CA, USA) and m/zCloud™ (https://www.mzcloud.org/) were used for identification of untargeted metabolites using a standard workflow template for food science. Aggregated computational toxicology resource, (ACToR, USA), FDA UNII – NLM (Maryland, USA), FooDB (The Food Database, Canada) and peptides databases were utilised for identification of metabolites. Only compounds with a high m/zCloud match (>60 with majority >80) were assigned an identity and subjected to statistical analyses.
Statistical analyses
Curation of volatile and non-volatile compound data was conducted using Microsoft Excel and the ‘tidyverse’ package (Wickham et al., 2019) in R version 4.0.1 (R Core Team, 2020). The non-volatile dataset was further filtered for compounds that were in >75% of samples. Semi-quantitative volatile data were calculated using the IS and normalised prior to analysis. Non-volatile compound data were normalised prior to analysis using partial quotient normalisation (Dieterle et al., 2006). Permutational multivariate analysis of variance (PERMANOVA) testing for effects of temperature, salinity and their interaction on the composition of compounds was applied to volatile and non-volatile datasets in R using the ‘vegan’ package (Oksanen et al., 2020). These analyses utilised 999 permutations and similarity matrices based on Euclidean distance. A permutational distance-based analysis was selected over parametric analyses (e.g. multivariate analysis of variance) to ensure rank deficiency of models fitted would not cause unequal residuals between groups within variance-covariance matrices. Principal components analysis (PCA) was also applied to volatile and non-volatile multivariate data to assess for similarity in the composition of compounds among experimental treatments and identify compounds most correlated with principal components explaining the greatest variation in compounds among treatments.
Two-factor analyses of variance (ANOVA) were applied to test for effects of temperature, salinity and their interaction on the univariate responses of volatile and non-volatile compounds. All ANOVAs utilised type III sums of squares as appropriate for unbalanced data (Shaw and Mitchell-Olds, 1993; Queen et al., 2002), which resulted from crab mortalities during the experiment. Tukey’s HSD post-hoc pairwise tests were used to separate significant treatment-level effects. Shapiro-Wilk and Levene tests were applied to confirm that normalised volatile and non-volatile response variables satisfied assumptions of normality and homogeneity of variance. Finally, a binomial generalised linear model (GLM) using a logit link function was applied in R using the ‘MASS’ package (Ripley and Venables, 2002) to test if the proportion of crab mortalities recorded throughout the experiment was significantly related to treatment.
Results
Portunus armatus mortality
Mortalities were between 21.4 and 28.6% among treatments during the experiment (mortalities per treatment = ATAS: 3, ATES: 3, ETAS: 4, and ETES: 3). Neither temperature, salinity nor their interaction significantly affected mortalities (GLM, p > 0.05).
Volatile compounds
A total of 48 volatile compounds were identified and semi-quantified by the SPME method (Table 1; Figure 2A). Permutational multivariate analysis revealed the composition of volatile compounds in P. armatus meat significantly differed between crabs exposed to 21 and 27°C for 30 days (pseudo-F = 1.97, p = 0.02; Figure 2B). However, no significant differences in the composition of volatile compounds were identified between crabs exposed to ambient (35 PSU) or elevated (40 PSU) salinities (pseudo-F = 0.67, p = 0.86; Figure 2C), nor was there a significant interaction between temperature and salinity (at the treatment levels investigated) on the composition of volatile compounds in P. armatus meat (pseudo-F = 1.05, p = 0.37).
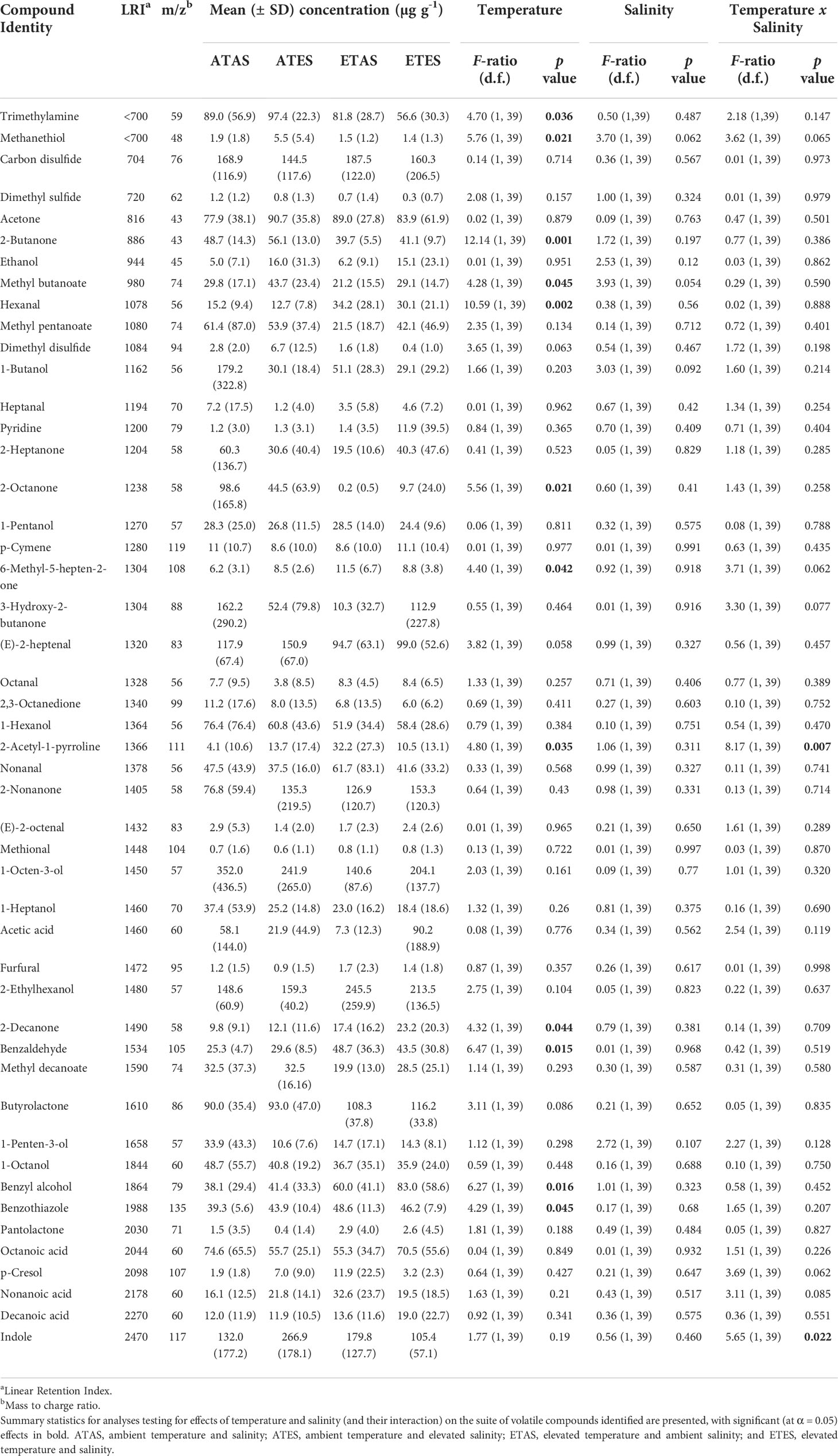
Table 1 Summary of volatile compounds (n = 48) identified in the meat of blue swimmer crabs (Portunus armatus) exposed to elevated temperature and salinity treatments for 30 days.
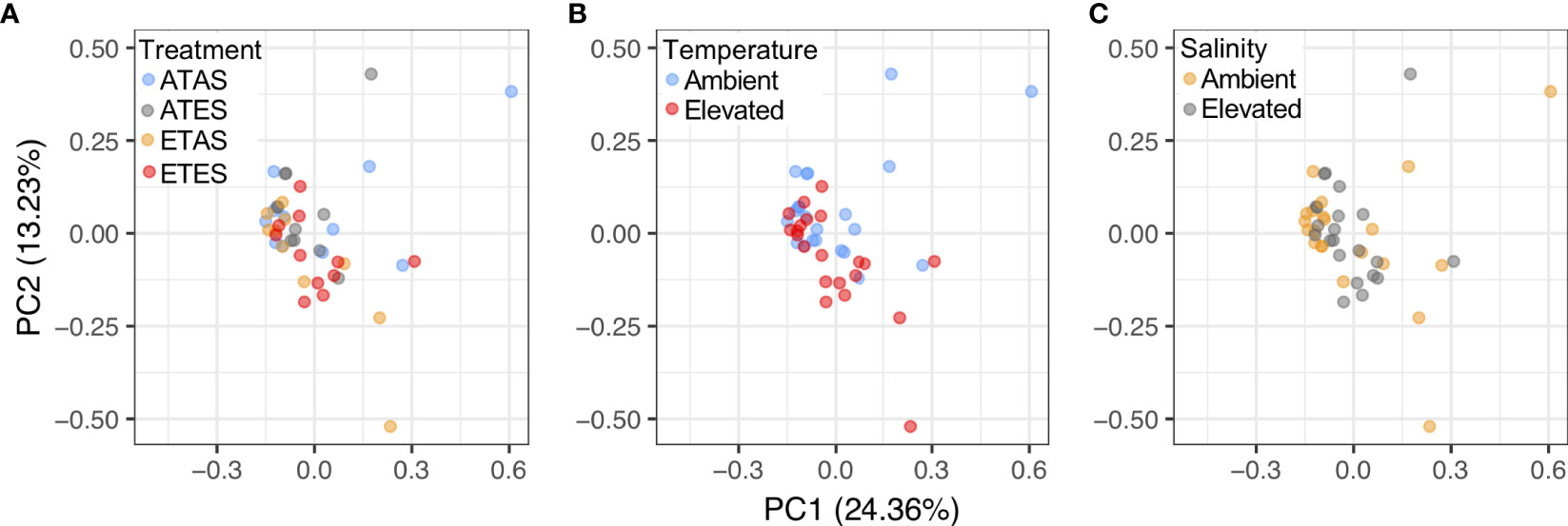
Figure 2 Principal components analysis biplots showing the similarity in composition of volatile compounds in blue swimmer crab (Portunus armatus) meat following experimentation (A) among all treatments, and between levels within the (B) temperature, and (C) salinity treatments. ATAS, ambient temperature and salinity; ATES, ambient temperature and elevated salinity; ETAS, elevated temperature and ambient salinity; and ETES, elevated temperature and salinity.
Principal components analysis identified that 24.4 and 13.2% of variation in volatile compounds among treatments was explained by principal components 1 (PC1) and 2 (PC2), respectively, with PC1 and PC2 separating the composition of volatile compounds in crabs exposed to ambient and elevated temperatures (Figure 2B). The volatile compounds most positively correlated with PC1 were 1-hexanol (loading = 0.23) and 1-octanol (loading = 0.23). Dimethyl disulfide (loading = 0.20) and benzaldehyde (loading = –0.22) were most positively and negatively correlated with PC2, highlighting that differences in the concentration of these compounds among treatments primarily drove the overarching responses in the multivariate data.
Of the 48 volatile compounds identified and semi-quantified, 12 (25%) were significantly affected by temperature (p < 0.05; Table 1). Trimethylamine, methanethiol, 2-butanone, methyl butanoate and 2-octanone were significantly lower following exposure to elevated temperature (Table 1). Conversely, hexanal, 6-methyl-5-hepten-2-one, 2-acetyl-1-pyrroline, 2-decanone, benzaldehyde, benzyl alcohol, benzothiazole were significantly higher in the meat of crabs exposed to elevated temperature. Elevated salinity did not significantly affect the concentration of volatile compounds alone (p > 0.05), however the interaction between temperature and salinity treatments significantly affected 2-acetyl-1-pyrroline and indole (p < 0.05; Table 1). At ambient temperature, 2-acetyl-1-pyrroline and indole were significantly higher when salinity was elevated, with the opposite effect of elevated salinity observed at the elevated temperature level (p < 0.05).
Non-volatile compounds
Non-volatile compounds analysed using LC-MS/MS presented a total of 294 mass features in at least 75% of the samples and 104 of these were positively identified using ms/ms search of mzCloud (https://doi.org/10.5281/zenodo.6775167). Consistent with the results for volatile compounds, the composition of non-volatile compounds in P. armatus meat significantly differed between crabs exposed to 21 and 27°C for a period of 30 days (pseudo-F = 6.13, p = 0.003; Figure 3B). No significant differences in the composition of non-volatile compounds were found between crabs exposed to ambient or elevated salinity levels (pseudo-F = 0.70, p = 0.57; Figure 2C) and there was no evidence of a significant interaction between temperature and salinity on the composition of non-volatile compounds (pseudo-F = 1.02, p = 0.38). PC1 and PC2 explained 26.0 and 12.9% of variation in non-volatile compounds among treatments, respective (Figure 3). Non-volatile compounds most positively correlated with PC1 were lysine (loading = 0.15), histidine (loading = 0.15) and serine (loading = 0.15). Prolyl-glycine (loading = 0.18) was most positively correlated with PC2, while proline (loading = –0.08) was most negatively correlated with this principal component.
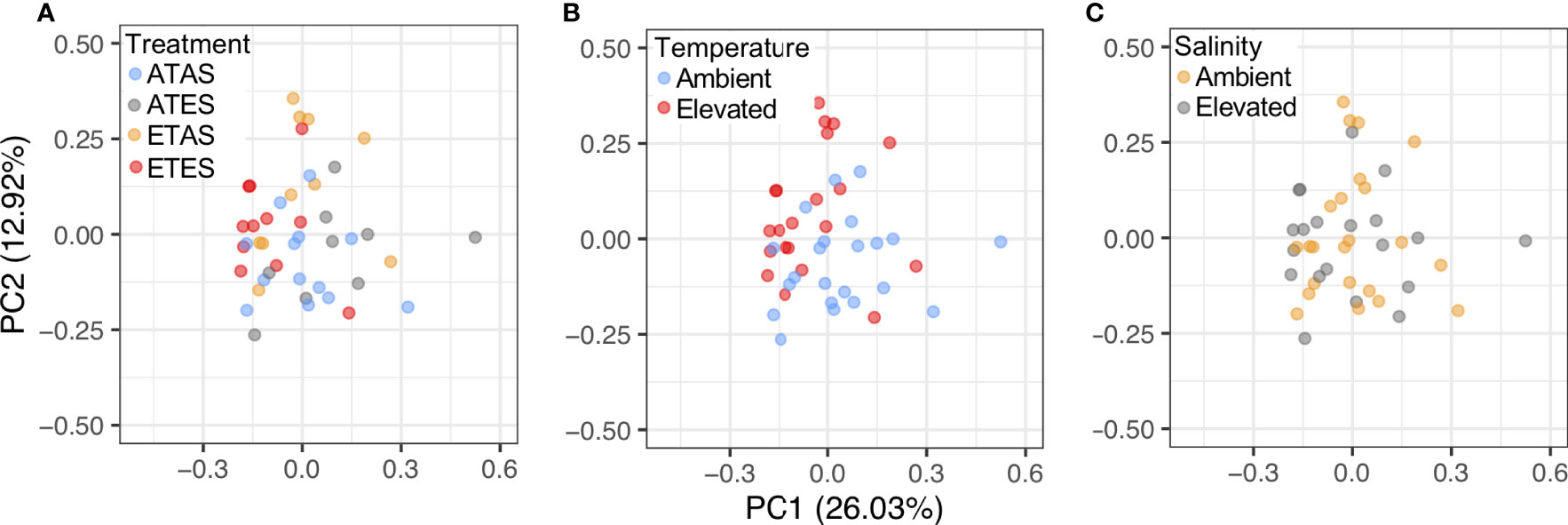
Figure 3 Principal components analysis biplots showing the similarity in composition of non-volatile compounds in blue swimmer crab (Portunus armatus) meat following experimentation (A) among all treatments, and between levels within the (B) temperature, and (C) salinity treatments. ATAS, ambient temperature and salinity; ATES, ambient temperature and elevated salinity; ETAS, elevated temperature and ambient salinity; and ETES, elevated temperature and salinity.
Temperature, salinity or their interaction caused significant differences in the concentration of nineteen unique non-volatile compounds that were confidently identified following manual curation (p < 0.05; Table 2). Of these, temperature alone was responsible for 83% of significant changes to the concentration of non-volatiles. The bitter tasting amino acids and amines tyrosine, iso-leucine, taurine and prolyl-leucine were present in significantly lower concentrations following exposure to the elevated temperature treatment, while the sweet tasting amino acid proline was also less abundant under the elevated temperature (Table 2).
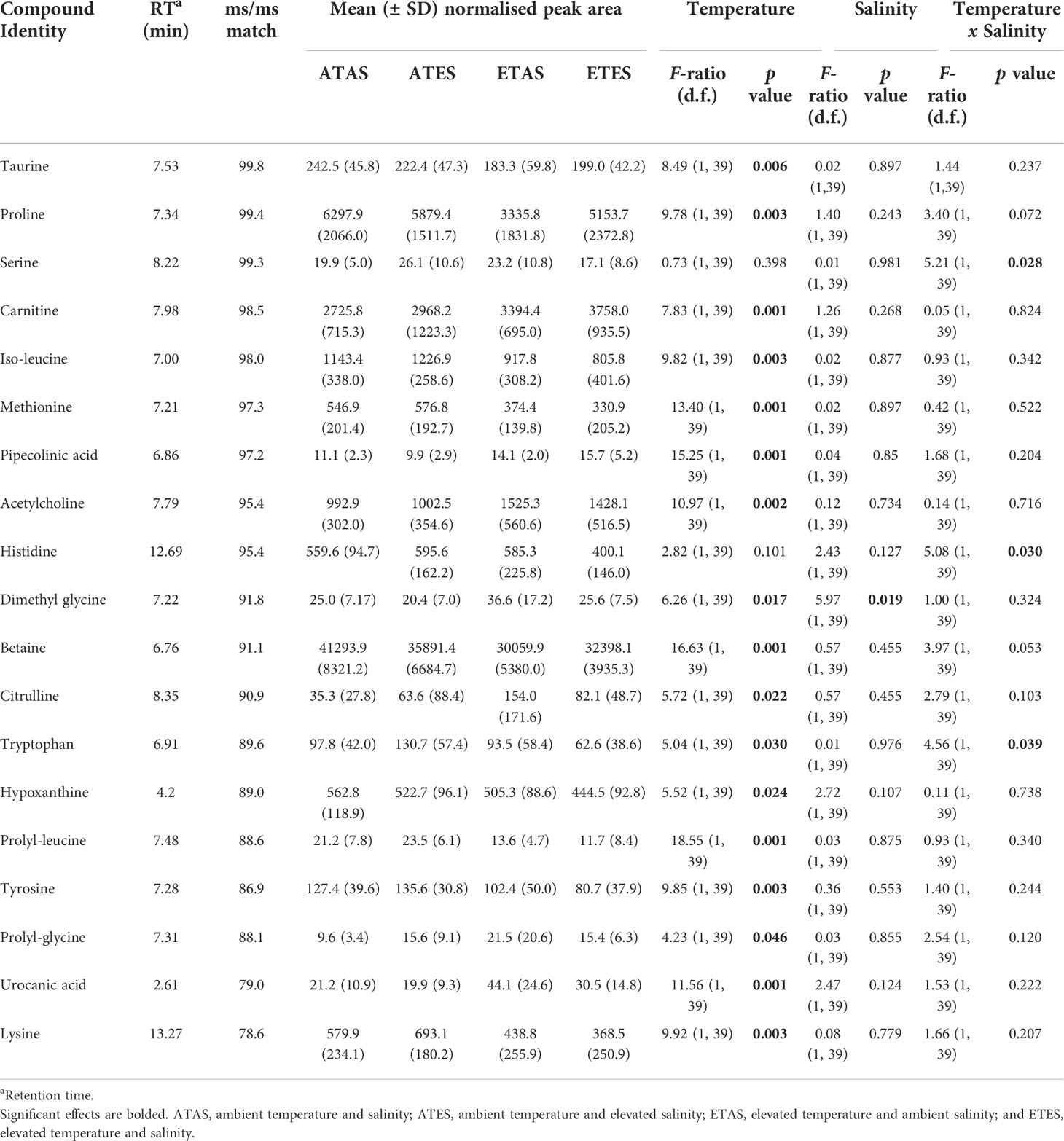
Table 2 Non-volatile compounds (n = 19) positively identified in the meat of blue swimmer crabs (Portunus armatus) that were significantly (at α = 0.05) affected by temperature or salinity treatments (or their interaction) after 30 days of experimental exposure.
A significant decrease in methionine, which is a major flavour precursor as well as an important amino acid, was found in the evaluated temperature treatment (F1,39 = 13.40, p < 0.001). A significant decrease in betaine was also identified under elevated temperature conditions (F1,39 = 16.62, p < 0.001). Betaine is involved in the methylation of cystathionin to form methionine and in so doing forms dimethyl glycine, which was found to be significantly elevated under elevated temperature and salinity conditions (Table 2). Histidine was on average lower in the elevated temperature treatment and this coincided with a significant increase in its breakdown metabolite urocanic acid (F1,39 = 11.56, p < 0.001). Finally, amino acid degradation was also apparent in the elevated temperature treatment, as evidenced by a significant decrease in lysine and a significant increase in its metabolite pipecolinic acid (Table 2).
Discussion
The effects of climate-driven environmental change on the sensory quality of seafood have considerable implications for consumer appeal and human health, but remain largely understudied. Here we tested the effects of simulated climate change on the composition of compounds that determine seafood aroma and flavour in the widely harvested P. armatus. We show the compositions of volatile and non-volatile compounds were significantly altered in crab meat following 30 days of exposure to elevated temperature. However, no overarching effects of elevated salinity on the compositions of volatile and non-volatile compounds were identified, nor was there a significant interaction between temperature and salinity on the composition of these compounds. This dominant effect of temperature, but not of salinity, on the composition of aroma- and taste-active compounds in crab meat suggests a relatively greater sensitivity of P. armatus flavour to changes in temperature than salinity. For example, significant reductions in amino acids and amines associated with bitter and sweet organoleptic properties (e.g. tyrosine, isoleucine, taurine and proline) were identified following exposure to elevated temperature but not salinity. These results support the hypothesis that the warming of marine and estuarine environments expected under near-future climate change could alter the composition of compounds that determine the aroma and flavour of seafood. Given that seafood flavour is inextricably linked with consumer choices and market demand, climate-driven changes to aroma- and taste-active metabolites may manifest as changes in fishing effort for impacted species and, in extreme instances, the economic viability of fisheries.
Changes to volatile and non-volatile compounds affect the flavour of Portunus armatus meat
The sensory attributes of numerous volatile compounds significantly affected by the experimental treatments applied here have been previously reported for some crustaceans (Matiella and Hsieh, 1990; Chung and Cadwallader, 1993; Chung, 1999; Sarnoski et al., 2010; Gu et al., 2013; Mall and Schieberle, 2016; Mall and Schieberle, 2017; Nanda et al., 2021). For example, trimethylamine and methanethiol are known to make important contributions to the aroma of crustaceans (Giri et al., 2010), with a decrease in these volatiles under elevated temperatures (as found here) likely to contribute to changes in the overall organoleptic quality of crab meat. Further, benzaldehyde produces a sweet odour through the degradation of unsaturated lipids, and a significant difference in the concentration of this compound is indicative of a different lipid composition and reduced meat sweetness in crabs exposed to ambient relative to elevated temperatures. 2-Acetyl-1-pyrroline has also been previously identified as an important odour-impacting compound in cooked crustaceans (Mall and Schieberle, 2016; Mall and Schieberle, 2017). The concentration of this compound within crab meat varied in response to the interaction between temperature and salinity treatments, with significantly higher concentrations found when salinity was elevated and the opposite effect observed at elevated temperature. Although we generally did not find significant interactive effects between temperature and salinity on odour-impacting compounds, this result demonstrates that interactive environmental effects can make important contributions to the aroma of seafood.
In addition to volatile odour-impacting compounds, the significant effect of temperature on the composition of non-volatile taste-active compounds further implies changes to the flavour of P. armatus meat under ocean warming. Betaine is a modified amino acid known to make important contributions to sweetness and umami flavours of crustacean meat (Sarower et al., 2012; Liu et al., 2018) and was significantly lower in P. armatus exposed to elevated temperatures for 30 days. Given this compound is commonly associated with high taste activity values (indicative of compounds that make substantial contributions to food taste; Meyer et al., 2016), the reduction of betaine observed here may be a primary mechanism contributing to changes in seafood taste under climate change. Further, lysine is also known to influence sweet and bitter attributes associated with crustacean meat and products (e.g. sauces), and its reduction here could manifest as relatively bitter P. armatus meat under ocean warming. (Liu et al., 2019). Lysine is also a building block for protein and, unlike other amino acids, cannot be produced by the human body and must be consumed. Consequently, reductions in this amino acid under elevated temperature might also reduce the nutritional quality of P. armatus meat.
Linking changes in individual volatiles and non-volatiles to specific changes in sensory attributes (e.g. sweetness, bitterness, umami) is complicated by the multivariate nature of metabolite data. While our findings support the potential for near-future climate change to alter the composition of aroma- and taste-active compounds in seafood, confirmation of such changes requires sensory panel analyses. For example, Lemasson et al. (2017) utilised an expert panel to assess the appearance, aroma, taste and overall acceptability of M. gigas following exposure to elevated temperature and ocean acidification, finding non-significant trends towards improved sensory qualities under acidification and warming scenarios. Furthermore, Dupont et al. (2014) also used an expert panel to identify that the appearance and taste, but not texture, of P. borealis is likely to significantly decline in response to ocean acidification. However, studies integrating analyses of aroma- and taste-active compounds with formal assessments of seafood appearance, aroma and taste by trained assessors are required to provide a mechanistic understanding of the impacts of near-future climate change on the sensory quality of seafood. A recent study by Hsieh et al. (2021) found that exposing tiger shrimp (Penaeus monodon) to lowered pH reduced production of the amino acids glutamate and aspartic acid, which provide a umami flavour in seafood. These changes in amino acid production were subsequently supported by a blind sensory quality test, where P. monodon that had been exposed to lower pH received lower scores for flavour, appearance and texture. Further integrating sensory panel assessments with analyses of aroma- and taste-active compounds is an important future direction for fisheries climate-impact research and a logical next step for understanding how multivariate changes in P. armatus sensory metabolites manifest in changes to flavour attributes.
Resilience of Portunus armatus flavour active compounds to elevated salinity
An important finding of this study was the apparent resilience of volatile and non-volatile compounds in P. armatus meat to altered salinity, which is consistent with previous research showing minimal effects of altered salinity on nutritional properties (e.g. protein and fatty acid composition) contained in P. armatus meat (Champion et al., 2020). Exposure to different salinities is known to affect crab tissue by altering the concentrations of free amino acids and other metabolites to maintain cells in an iso-osmotic condition and the null result here is contrary to other studies (Holt and Kinsey, 2002). For example, Chinese razor clams (Sinonovacula constricta)—a burrowing bivalve occurring in soft sediment environments throughout eastern Asia—cultured under elevated salinity conditions (PSU = 23) had more abundant volatiles than those cultured under lower salinities (PSU = 13; Ran et al., 2019). Furthermore, lowered salinity evoked softer meat texture and weaker flavour characteristics, including umami taste, in green mud crabs (Scylla paramamosain; Luo et al., 2021).
It is possible the trend toward an effect of temperature, but not salinity, on compounds pertaining to the flavour of P. armatus was due to the selection of the treatment levels. Presently, temperatures of 27°C (i.e. the elevated temperature treatment) approximate, or exceed, the upper thermal limit experienced by P. armatus throughout its temperate eastern Australian distribution, particularly at the site of collection for our experiment (Fiebig, 2010). By comparison, salinity levels within estuarine habitats throughout this region vary considerably in response to drought and rainfall events, and are known to exceed the elevated salinity treatment level applied here (PSU = 40) Wallis Lake (Fiebig, 2010). Therefore, the physiology of P. armatus may be better adapted to cope with changes in salinity, relative to the changes in temperature, at the levels applied. Nevertheless, our experimental treatment levels were selected to align with projections of climate-driven environment change in eastern Australia (Gillanders et al., 2011; Hobday and Lough, 2011), and so, for P. armatus, future changes in temperature are likely to make greater contributions to altered sensory quality than changes in salinity.
We also acknowledge salinity levels in estuarine environments are projected to become increasingly variable under climate change, with elevated levels predicted during intense drought events, and reduced levels in response to increasing flooding events. While the magnitude of variation in salinities experienced in estuarine environments is expected to increase under climate change, here we have only considered the elevated salinity scenario likely to occur during periods of drought. Testing the effects of lower salinities is also important for fully addressing the effects of future salinity levels on aroma- and taste-active compounds pertaining to the flavour of P. armatus under climate change.
In addition to coherent choices of the upper bounds of environmental change, careful consideration of experimental durations is essential when testing the effects of climate change on seafood sensory properties. Predicted increases in the magnitude of short-term environmental extremes and the frequency of conditions that are outside species’ tolerance ranges under climate change (Kerr, 2011) highlight the importance of short-term (i.e. weeks to months) experiments for quantifying species-specific responses (as applied here; Jentsch et al., 2007; Thompson et al., 2013). However, the physical effects of climate change on marine systems also include underlying shifts in mean environmental conditions. Selecting experimental treatment levels and durations that align with the projected effects of climate change for specific study systems and species are necessary to ensure results are indicative of likely future changes (Shalders et al., 2022). Moreover, incorporating the effects of climate change across trophic levels is an additional consideration for future research. Here P. armatus were M. macleaya that had not been exposed to experimental treatments. Thus, it remains unknown how the potential effects of environmental change on prey contribute to changes in the composition of volatile and non-volatile compounds in P. armatus meat.
Implications of climate-driven changes to seafood flavour
Seafood flavour is inextricably linked with consumer choices and market demand (Birch and Lawley, 2012; Murray et al., 2017). In Australia and Canada, seafood taste is a leading driver of appeal and a key determinant of the amount of seafood that humans consume (Christenson et al., 2017; Murray et al., 2017). By influencing the amounts and types of seafood that humans consume, flavour characteristics also contribute to the associated health benefits derived through seafood consumption (Christenson et al., 2017). In this way, climate-driven changes to the sensory quality of seafood have the potential to impact consumer preferences for harvested species, and also affect the nutritional benefits humans derive from seafood consumption.
Climate-change impacts may be positive or negative depending on the magnitude and direction of the effects of environmental change on seafood sensory properties. If the magnitude of climate-driven changes to seafood sensory properties are large enough, there is potential for the economic viability of fisheries to become impacted through reductions in the value and/or market demand for their products. However, such impacts will depend on the relative vulnerability of harvested species to climate change, which will be determined by relative physiological sensitivities and exposures (Foden et al., 2019). While experimental assessments are required to quantify the sensitivity of sensory properties in harvested species to environmental change, regions of the global ocean where species are most exposed to the effects of climate-driven ocean warming are already apparent (Hobday and Pecl, 2014; Pecl et al., 2014). It is within these regions, which include the Sea of Japan, south-eastern Australia and the Bering Sea among others, that seafood industries should be most aware of the potential for climate-driven changes to seafood aroma and taste.
Data availability statement
The datasets presented in this study can be found in online repositories. The names of the repository/repositories and accession number(s) can be found below: https://doi.org/10.5281/zenodo.6775167.
Author contributions
CC, MB and MC conceptualised the research question and designed the experimental approach. DF, MT, KK and UP undertook laboratory analysis of volatile and non-volatile metabolites. CC, DF and MT statistically analysed the data. CC and TS wrote the manuscript with input from all authors. All authors contributed to the article and approved the submitted version.
Acknowledgments
We thank Sean Blake for leading the collection of blue swimmer crabs and installing the experimental system. This work is part of the NSW Primary Industries Climate Change Research Strategy, funded by the NSW Climate Change Fund.
Conflict of interest
The authors declare that the research was conducted in the absence of any commercial or financial relationships that could be construed as a potential conflict of interest.
Publisher’s note
All claims expressed in this article are solely those of the authors and do not necessarily represent those of their affiliated organizations, or those of the publisher, the editors and the reviewers. Any product that may be evaluated in this article, or claim that may be made by its manufacturer, is not guaranteed or endorsed by the publisher.
References
Ab Lah R., Kelaher B. P., Bucher D., Benkendorff K. (2018). Ocean warming and acidification affect the nutritional quality of the commercially-harvested turbinid snail Turbo militaris. Mar. Environ. Res. 141, 100–108. doi: 10.1016/j.marenvres.2018.08.009
Alma L., Kram K. E., Holtgrieve G. W., Barbarino A., Fiamengo C. J., Padilla-Gamiño J. L. (2020). Ocean acidification and warming effects on the physiology, skeletal properties, and microbiome of the purple-hinge rock scallop. Comp. Biochem. Physiol. Part A.: Mol. Integr. Physiol. 240, 110579. doi: 10.1016/j.cbpa.2019.110579
Beck M. W., Heck K. L., Able K. W., Childers D. L., Eggleston D. B., Gillanders B. M., et al. (2001). The identification, conservation, and management of estuarine and marine nurseries for fish and invertebrates: a better understanding of the habitats that serve as nurseries for marine species and the factors that create site-specific variability in nursery quality will improve conservation and management of these areas. BioScience 51 (8), 633–641. doi: 10.1641/0006-3568(2001)051[0633:TICAMO]2.0.CO;2
Birch D., Lawley M. (2012). Buying seafood: Understanding barriers to purchase across consumption segments. Food Qual. Preference. 26 (1), 12–21. doi: 10.1016/j.foodqual.2012.03.004
Broadhurst M. K., Millar R. B., Hughes B. (2017). Performance of industry-developed escape gaps in Australian portunus pelagicus traps. Fisheries. Res. 187, 120–126. doi: 10.1016/j.fishres.2016.11.013
Champion C., Broadhurst M. K., Ewere E. E., Benkendorff K., Butcherine P., Wolfe K., et al. (2020). Resilience to the interactive effects of climate change and discard stress in the commercially important blue swimmer crab (Portunus armatus). Mar. Environ. Res. 159, 105009. doi: 10.1016/j.marenvres.2020.105009
Champion C., Hobday A. J., Zhang X., Pecl G. T., Tracey S. R. (2019). Changing windows of opportunity: past and future climate-driven shifts in temporal persistence of kingfish (Seriola lalandi) oceanographic habitat within south-eastern Australian bioregions. Mar. Freshw. Res. 70 (1), 33–42. doi: 10.1071/MF17387
Chen D. W., Zhang M. (2007). Non-volatile taste active compounds in the meat of Chinese mitten crab (Eriocheir sinensis). Food Chem. 104 (3), 1200–1205. doi: 10.1016/j.foodchem.2007.01.042
Christenson J. K., O'Kane G. M., Farmery A. K., McManus A. (2017). The barriers and drivers of seafood consumption in Australia: a narrative literature review. Int. J. Consumer. Stud. 41 (3), 299–311. doi: 10.1111/ijcs.12342
Chung H. Y. (1999). Volatile components in crabmeats of charybdis feriatus. J. Agric. Food Chem. 47 (6), 2280–2287. doi: 10.1021/jf981027t
Chung H. Y., Cadwallader K. R. (1993). Volatile components in blue crab (Callinectes sapidus) meat and processing by-product. J. Food Sci. 58, 1203–1207. doi: 10.1111/j.1365-2621.1993.tb06148.x
Dieterle F., Ross A., Schlotterbeck G., Senn H. (2006). Probabilistic quotient normalization as robust method to account for dilution of complex biological mixtures. application in 1H NMR metabonomics. Analytical. Chem. 78 (13), 4281–4290. doi: 10.1021/ac051632c
Duan Z., Dong S., Sun Y., Dong Y., Gao Q. (2021). Response of Atlantic salmon (Salmo salar) flavor to environmental salinity while culturing between freshwater and seawater. Aquaculture 530, 735953. doi: 10.1016/j.aquaculture.2020.735953
Dupont S., Hall E., Calosi P., Lundve B. (2014). First evidence of altered sensory quality in a shellfish exposed to decreased pH relevant to ocean acidification. J. Shellfish. Res. 33 (3), 857–861. doi: 10.2983/035.033.0320
FAO (2020). “The state of world fisheries and aquaculture 2020,” in Sustainability in action (Rome: Food and Agriculture Organization of the United Nations).
Fiebig S. (2010). Summary of ecological information for the Wallis lake potential RAMSAR site (NSW: Department of Environment and Climate Change).
Foden W. B., Young B. E., Akçakaya H. R., Garcia R. A., Hoffmann A. A., Stein B. A., et al. (2019). Climate change vulnerability assessment of species. Wiley. Interdiscip. Reviews.: Climate Change 10 (1), e551. doi: 10.1002/wcc.551
Frank D., Poole S., Kirchhoff S., Forde C. N. (2009). Investigation of sensory and volatile characteristics of farmed and wild barramundi (Lates calcarifer) using gas chromatography–olfactometry mass spectrometry and descriptive sensory analysis. J. Agric. Food Chem. 57 (21), 10302–10312. doi: 10.1021/jf902030y
Free C. M., Thorson J. T., Pinsky M. L., Oken K. L., Wiedenmann J., Jensen O. P. (2019). Impacts of historical warming on marine fisheries production. Science 363 (6430), 979–983. doi: 10.1126/science.aau1758
Fuentes A., Fernández-Segovia I., Serra J. A., Barat J. M. (2010). Comparison of wild and cultured sea bass (Dicentrarchus labrax) quality. Food Chem. 119 (4), 1514–1518. doi: 10.1016/j.foodchem.2009.09.036
Gerland P., Raftery A. E., Ševčíková H., Li N., Gu D., Spoorenberg T., et al. (2014). World population stabilization unlikely this century. Science 346 (6206), 234–237. doi: 10.1126/science.1257469
Gervais C. R., Champion C., Pecl G. T. (2021). Species on the move around the Australian coastline: a continental scale review of climate-driven species redistribution in marine systems. Global Change Biol. 27, 3200–3217. doi: 10.1111/gcb.15634
Gillanders B. M., Elsdon T. S., Halliday I. A., Jenkins G. P., Robins J. B., Valesini F. J. (2011). Potential effects of climate change on Australian estuaries and fish utilising estuaries: a review. Mar. Freshw. Res. 62 (9), 1115–1131. doi: 10.1071/MF11047
Giri A., Osako K., Okamoto A., Ohshima T. (2010). Olfactometric characterization of aroma active compounds in fermented fish paste in comparison with fish sauce, fermented soy paste and sauce products. Food Res. Int. 43 (4), 1027–1040. doi: 10.1016/j.foodres.2010.01.012
Golden C. D., Koehn J. Z., Shepon A., Passarelli S., Free C. M., Viana D. F., et al. (2021). Aquatic foods to nourish nations. Nature 598, 315–320. doi: 10.1038/s41586-021-03917-1
Gu S. Q., Wang X. C., Tao N. P., Wu N. (2013). Characterization of volatile compounds in different edible parts of steamed Chinese mitten crab (Eriocheir sinensis). Food Res. Int. 54 (1), 81–92. doi: 10.1016/j.foodres.2013.05.018
Hay T., Gribble N., de Vries C., Danaher K., Dunning M., Hearnden M., et al. (2005). Methods for monitoring the abundance and habitat of the northern Australian mud crab Scylla serrata. fishery report 80 (Darwin: Northern Territory Department of Business, Industry and Resource Development).
Hicks C. C., Cohen P. J., Graham N. A., Nash K. L., Allison E. H., D’Lima C., et al. (2019). Harnessing global fisheries to tackle micronutrient deficiencies. Nature 574 (7776), 95–98. doi: 10.1038/s41586-019-1592-6
Hobday A. J., Lough J. M. (2011). Projected climate change in Australian marine and freshwater environments. Mar. Freshw. Res. 62 (9), 1000–1014. doi: 10.1071/MF10302
Hobday A. J., Pecl G. T. (2014). Identification of global marine hotspots: sentinels for change and vanguards for adaptation action. Rev. Fish. Biol. Fisheries. 24 (2), 415–425. doi: 10.1007/s11160-013-9326-6
Holt S. M., Kinsey S. T. (2002). Osmotic effects on arginine kinase function in living muscle of the blue crab callinectes sapidus. J. Exp. Biol. 205 (12), 1775–1785. doi: 10.1242/jeb.205.12.1775
Hsieh H. H., Weerathunga V., Weerakkody W. S., Huang W. J., Muller F. L., Benfield M. C., et al. (2021). The effects of low pH on the taste and amino acid composition of tiger shrimp. Sci. Rep. 11 (1), 1–9. doi: 10.1038/s41598-021-00612-z
Jentsch A., Kreyling J., Beierkuhnlein C. (2007). A new generation of climate-change experiments: events, not trends. Front. Ecol. Environ. 5 (7), 365–374. doi: 10.1890/1540-9295(2007)5[365:ANGOCE]2.0.CO;2
Kailola P. J., Williamson M. J., Stewart P. C., Reichelt R. E., McNee A. (1993). Australian Fisheries resources. Bureau. Resource. Sciences. Canberra. p, 422.
Kerr R. A. (2011). Humans are driving extreme weather; time to prepare. Science 334 (6059), 1040–1040. doi: 10.1126/science.334.6059.1040
Lan W. R., Huang X. G., Lin L. X., Li S. X., Liu F. J. (2020). Thermal discharge influences the bioaccumulation and bioavailability of metals in oysters: Implications of ocean warming. Environ. pollut. 259, 113821. doi: 10.1016/j.envpol.2019.113821
Lemasson A., Hall-Spencer J., Kuri V., Knights A. (2019). Changes in the biochemical and nutrient composition of seafood due to ocean acidification and warming. Mar. Environ. Res. 143, 82–92. doi: 10.1016/j.marenvres.2018.11.006
Lemasson A. J., Kuri V., Hall-Spencer J. M., Fletcher S., Moate R., Knights A. M. (2017). Sensory qualities of oysters unaltered by a short exposure to combined elevated pCO2 and temperature. Front. Mar. Sci. 4, 352. doi: 10.3389/fmars.2017.00352
Liu C., Meng F., Tang X., Shi Y., Wang A., Gu Z., et al. (2018). Comparison of nonvolatile taste active compounds of wild and cultured mud crab Scylla paramamosain. Fisheries. Sci. 84 (5), 897–907. doi: 10.1007/s12562-018-1227-0
Liu T.-T., Xia N., Wang Q.-Z., Chen D.-W. (2019). Identification of the non-volatile taste-active components in crab sauce. Foods 8 (8), 324. doi: 10.3390/foods8080324
Lund E. K. (2013). Health benefits of seafood; is it just the fatty acids? Food Chem. 140 (3), 413–420. doi: 10.3390/foods8080324
Luo J., Monroig Ó., Zhou Q., Tocher D. R., Yuan Y., Zhu T., et al. (2021). Environmental salinity and dietary lipid nutrition strategy: Effects on flesh quality of the marine euryhaline crab Scylla paramamosain. Food Chem. 361, 130160. doi: 10.1016/j.foodchem.2021.130160
Mabuchi R., Ishimaru A., Tanaka M., Kawaguchi O., Tanimoto S. (2019). Metabolic profiling of fish meat by GC-MS analysis, and correlations with taste attributes obtained using an electronic tongue. Metabolites 9 (1), 1. doi: 10.3390/metabo9010001
Maire E., Graham N. A. J., MacNeil M. A., Lam V. W. Y., Robinson J. P. W., Cheung W. W. L., et al. (2021). Micronutrient supply from global marine fisheries under climate change and overfishing. Curr. Biol. 31 (18), 4132–4138. doi: 10.1016/j.cub.2021.06.067
Mall V., Schieberle P. (2016). Characterization of key aroma compounds in raw and thermally processed prawns and thermally processed lobsters by application of aroma extract dilution analysis. J. Agric. Food Chem. 64 (33), 6433–6442. doi: 10.1021/acs.jafc.6b02728
Mall V., Schieberle P. (2017). Evaluation of key aroma compounds in processed prawns (Whiteleg shrimp) by quantitation and aroma recombination experiments. J. Agric. Food Chem. 65 (13), 2776–2783. doi: 10.1021/acs.jafc.7b00636
Mamo L. T., Benkendorff K., Butcherine P., Coleman M. A., Ewere E. E., Miranda R. J., et al. (2019). Resilience of a harvested gastropod, Turbo militaris, to marine heatwaves. Mar. Environ. Res. 151, 104769. doi: 10.1016/j.marenvres.2019.104769
Matiella J. E., Hsieh T. C. Y. (1990). Analysis of crabmeat volatile compounds. J. Food Sci. 55 (4), 962–966. doi: 10.1111/j.1365-2621.1990.tb01575.x
Meyer S., Dunkel A., Hofmann T. (2016). Sensomics-assisted elucidation of the tastant code of cooked crustaceans and taste reconstruction experiments. J. Agric. Food Chem. 64 (5), 1164–1175. doi: 10.1021/acs.jafc.5b06069
Murray G., Wolff K., Patterson M. (2017). Why eat fish? factors influencing seafood consumer choices in British Columbia, Canada. Ocean. Coast. Manage. 100 (144), 16–22. doi: 10.1016/j.ocecoaman.2017.04.007
Nanda P. K., Das A. K., Dandapat P., Dhar P., Bandyopadhyay S., Dib A. L., et al. (2021). Nutritional aspects, flavour profile and health benefits of crab meat based novel food products and valorisation of processing waste to wealth: A review. Trends Food Sci. Technol. 112, 252–267. doi: 10.1016/j.tifs.2021.03.059
Oksanen J., Blanchet F. G., Friendly M., Kindt R., Legendre P., McGlinn D., et al. (2020) Vegan: Community ecology package. r package version 2.5-7. Available at: https://CRAN.R-project.org/package=vegan.
Pecl G. T., Hobday A. J., Frusher S., Warwick H., Sauer H., Bates A. E. (2014). Ocean warming hotspots provide early warning laboratories for climate change impacts. Rev. Fish. Biol. Fisheries. 24 (2), 409–413. doi: 10.1007/s11160-014-9355-9
Pinsky M. L., Selden R. L., Kitchel Z. J. (2019). Climate-driven shifts in marine species ranges: Scaling from organisms to communities. Annu. Rev. Mar. Sci. 12, 153–179. doi: 10.1146/annurev-marine-010419-010916
Poloczanska E. S., Brown C. J., Sydeman W. J., Kiessling W., Schoeman D. S., Moore P. J., et al. (2013). Global imprint of climate change on marine life. Nat. Climate Change 3 (10), 919–925. doi: 10.1038/nclimate1958
Queen J. P., Quinn G. P., Keough M. J. (2002). Experimental design and data analysis for biologists. Cambridge. Univ. Press. doi: 10.1017/CBO9780511806384
Ran Z., Zhang S., Zhu Y., Ke A., Xu J., Li Y., et al. (2019). Effect of salinity on volatiles in the razor clam investigated by head space-solid phase microextraction/gas chromatography-mass spectrometry. Fisheries. Sci. 85 (1), 137–146. doi: 10.1007/s12562-018-1260-z
R Core Team .(2020). R: A language and environment for statistical computing (Vienna: R Foundation for Statistical Computing).
Rogers L. A., Griffin R., Young T., Fuller E., Martin K. S., Pinsky M. L. (2019). Shifting habitats expose fishing communities to risk under climate change. Nat. Climate Change 9 (7), 512–516. doi: 10.1038/s41558-019-0503-z
Sarnoski P. J., O’Keefe S. F., Jahncke M. L., Mallikarjunan P., Flick G. J. (2010). Analysis of crab meat volatiles as possible spoilage indicators for blue crab (Callinectes sapidus) meat by gas chromatography–mass spectrometry. Food Chem. 122 (3), 930–935. doi: 10.1016/j.foodchem.2010.03.069
Sarower M. G., Hasanuzzaman A. F. M., Biswas B., Abe H. (2012). Taste producing components in fish and fisheries products: A review. Int. J. Food Fermentation. Technol. 2 (2), 113–121.
Scanes E., Scanes P. R., Ross P. M. (2020). Climate change rapidly warms and acidifies Australian estuaries. Nat. Commun. 11 (1), 1803. doi: 10.1038/s41467-020-15550-z
Shalders T. C., Champion C., Coleman M. A., Benkendorff K. (2022). The nutritional and sensory quality of seafood in a changing climate. Mar. Environ. Res. 176, 105590. doi: 10.1016/j.marenvres.2022.105590
Shaw R. G., Mitchell-Olds T. (1993). ANOVA for unbalanced data: an overview. Ecology 74 (6), 1638–1645. doi: 10.2307/1939922
Sumaila U. R., Cheung W. W., Lam V. W., Pauly D., Herrick S. (2011). Climate change impacts on the biophysics and economics of world fisheries. Nat. Climate Change 1 (9), 449–456. doi: 10.1038/nclimate1301
Tate R. D., Benkendorff K., Ab Lah R., Kelaher B. P. (2017). Ocean acidification and warming impacts the nutritional properties of the predatory whelk, Dicathais orbita. J. Exp. Mar. Biol. Ecol. 493, 7–13. doi: 10.1016/j.jembe.2017.03.006
Thompson R. M., Beardall J., Beringer J., Grace M., Sardina P. (2013). Means and extremes: building variability into community-level climate change experiments. Ecol. Lett. 16 (6), 799–806. doi: 10.1111/ele.12095
Uhlmann S. S., Broadhurst M. K., Paterson B. D., Mayer D. G., Butcher P., Brand C. P. (2009). Mortality and blood loss by blue swimmer crabs (Portunus pelagicus) after simulated capture and discarding from gillnets. ICES. J. Mar. Sci. 66 (3), 455–461. doi: 10.1093/icesjms/fsn222
Valles-Regino R., Tate R., Kelaher B., Savins D., Dowell A., Benkendorff K. (2015). Ocean warming and CO2-induced acidification impact the lipid content of a marine predatory gastropod. Mar. Drugs 13 (10), 6019–6037. doi: 10.3390/md13106019
Wang W. N., Wang A. L., Bao L., Wang J. P., Liu Y., Sun R. Y. (2004). Changes of protein-bound and free amino acids in the muscle of the freshwater prawn Macrobrachium nipponense in different salinities. Aquaculture 233 (1-4), 561–571. doi: 10.1016/j.aquaculture.2003.09.042
Weatherdon L. V., Magnan A. K., Rogers A. D., Sumaila U. R., Cheung W. W. (2016). Observed and projected impacts of climate change on marine fisheries, aquaculture, coastal tourism, and human health: An update. Front. Mar. Sci. 3, 48. doi: 10.3389/fmars.2016.00048
Wickham H., Averick M., Bryan J., Chang W., McGowan L. D. A., Francois R., et al. (2019). Welcome to tidyverse. J. Open Source Software. 4 (43), 1686. doi: 10.21105/joss.01686
Keywords: climate change, non-volatile, Portunus armatus, seafood flavour, volatile
Citation: Champion C, Frank D, Taylor MC, Kaczmarska K, Piyasiri U, Broadhurst MK, Shalders TC and Coleman MA (2022) Climate-driven changes to taste and aroma determining metabolites in an economically valuable portunid (Portunus armatus) have implications for future harvesting. Front. Mar. Sci. 9:973801. doi: 10.3389/fmars.2022.973801
Received: 20 June 2022; Accepted: 21 July 2022;
Published: 11 August 2022.
Edited by:
Bayden D. Russell, Swire Institute of Marine Science, School of Biological Sciences, Faculty of Science, The University of Hong Kong, Hong Kong SAR, ChinaReviewed by:
Mohamad N. Azra, Universiti Malaysia Terengganu, MalaysiaLaura Ramajo, Universidad Católica del Norte, Chile
Copyright © 2022 Champion, Frank, Taylor, Kaczmarska, Piyasiri, Broadhurst, Shalders and Coleman. This is an open-access article distributed under the terms of the Creative Commons Attribution License (CC BY). The use, distribution or reproduction in other forums is permitted, provided the original author(s) and the copyright owner(s) are credited and that the original publication in this journal is cited, in accordance with accepted academic practice. No use, distribution or reproduction is permitted which does not comply with these terms.
*Correspondence: Curtis Champion, curtis.champion@dpi.nsw.gov.au