Ocean acidification and warming modify stimulatory benthos effects on sediment functioning: An experimental study on two ecosystem engineers
- Biology Department, Marine Biology Research Group, Ghent University, Gent, Belgium
Many macrofauna have a stimulatory effect on sediment functioning through their burrowing, feeding and irrigation activities. Here, we investigated the single and combined effect of ocean acidification and warming on the stimulatory effect of two key-species inhabiting sandy seabeds in the Southern Bight of the North Sea; the bivalve Abra alba and the polychaete Lanice conchilega. The species were separately incubated in natural sediment in the laboratory under ambient, low pH (pH: -0.3), warm (T: + 3°C) and mimicked climate change (pH: -0.3, T: +3°C) conditions. After six weeks of incubation, nutrient and oxygen exchange were measured at the sediment-water interface to estimate aerobic sediment metabolism and nitrogen cycling. Both species facilitate sediment community oxygen consumption, nitrification and denitrification under ambient conditions. The stimulatory effect of A. alba disappeared in a low pH environment and decreased over time in the warmer treatments along with increased mortality. In contrast, L. conchilega stimulated sediment biogeochemical cycling more when seawater becomes acidified (+ 8 to 41%, depending on the function) but warming had no effect. We explain these species-specific climate change effects by different behavioral and physiological coping strategies that cascade on to sediment biogeochemical cycling, especially through altered oxygenation the sediment matrix.
1 Introduction
The important role of macrofauna species as benthic ecosystem engineers has long been recognized. Infaunal macrobenthic species are known to alter both the physical structure of and the chemical fluxes within sediments (Laverock et al., 2011). The foraging movements of macrobenthic species rework the top layer of the sediment [i.e. bioturbation (Meysman et al., 2006; Kristensen et al., 2012)], facilitating transport of oxygen and organic matter into deeper layers and of excretion products in and out of the sediment towards the water column. This altered transport of solutes and solids that is driven by physiological and behavioral processes stimulate microbial activity and organic matter mineralization and thus increase turnover of nutrients (Mermillod-Blondin et al., 2004).
Over the last decades, human activities have strongly increased the atmospheric carbon dioxide (CO2) levels. If no drastic CO2 mitigation measures are taken, this increase is predicted to continue up to atmospheric CO2 concentrations of ca. 940 ppm by the end of this century compared to a current value of ca. 416 ppm (NOAA; Collins et al., 2013). Oceans are counteracting this increase by absorbing CO2 at a current rate of 106 tons per hour (Brewer, 2009). This absorbing capacity, however, has already led to a decrease in surface-ocean pH of 0.1 units since the start of the industrial revolution (Caldeira and Wickett, 2003). A further decline of about 0.29 pH units by the end of the 21st century is predicted for the open ocean (Collins et al., 2013; Bindoff et al., 2019; Allan, 2021). In coastal shallow waters, acidification rates often follow different trends. These areas are subject to other stressors, such as freshwater inputs (Carstensen and Duarte, 2019), eutrophication (Cai et al., 2017) and seasonal variations (Hartman et al., 2019; Omar et al., 2019) which may lead to higher acidification rates (Provoost et al., 2010). On top of their effect on ocean pH, higher concentrations of atmospheric CO2 and methane enhance the greenhouse effect, thus impacting ocean surface temperature. This is expected to lead to an increase of global (0 – 200 m depth) ocean temperature of 1.0 up to 3.2°C by the end of this century (Collins et al., 2013). These alterations in both seawater temperature and pH may challenge marine benthic species and the way in which they affect their environment (Fabry et al., 2008; Byrne, 2011; Dupont and Pörtner, 2013; Nagelkerken and Munday, 2016). Ocean acidification (OA) disrupts acid-base regulations of marine species through the intake of acidified water and calcifying organisms in particular are negatively affected as OA causes the dissolution of their CaCO3 shells (Matoo et al., 2013; Thomsen et al., 2015; Lemasson et al., 2017; Hurd et al., 2019; Figuerola et al., 2021), whereas changing temperatures may move organisms away from their thermal optima (Pörtner et al., 2004; Sokolova et al., 2012; Reddin et al., 2022). The consecutive behavioral and physiological acclimation and adaptation to climate change is well documented for macrobenthic species (e.g. Tuomainen and Candolin, 2011; Ong et al., 2017; Vlaminck et al., 2022a; Vlaminck et al., 2022b). Alma et al. (2020) for example, found reduced shell strength when the purple-hinge rock scallop was exposed to a pCO2 of 1050 µatm, while Steeves et al. (2018) predicted increased growth rates of two bivalve species; Crassostrea virginica and Mytilus edulis, in a warmer climate. Van Colen et al. (2020) identified a shift in feeding mode of Scrobicularia plana from predominantly suspension feeding to deposit feeding, in a warmer and acidified environment. Little research has investigated the cascading effect on ecosystem functioning. For example, previous research has demonstrated that OW increased and OA decreased microbial gene abundance and thus modify structure and functioning of nitrogen cycling microbial communities (Currie et al., 2017; Seidel et al., 2022). However, it remains largely unexplored to what extent climate change induced alteration in macrofauna behavior that shape the habitat conditions for the microbial community, i.e. ecosystem engineering, also affect functioning.
In this study, we aim to improve our understanding of the effects of climate change on single species contributions to ecosystem functioning, with a focus on sediment nitrogen cycling. We studied the effect of climate change on two widely spread key species that are abundant in the Southern Bight of the North Sea: the white furrow shell Abra alba and the sand mason, Lanice conchilega (Tebble, 1976; Hartmann-Schröder, 1996). Abra alba is a tellenid bivalve living in the upper layer of the sediment. In search for food, A. alba randomly reworks the upper layer of the sediment, impacting organic matter sequestration and resuspension (Bernard et al., 2012). Lanice conchilega is a tube-dwelling polychaete that can occur in high densities, forming reef-like structures that increase local biodiversity (Rabaut et al., 2007; De Smet et al., 2015). Lanice conchilega acts as a piston pumper that ventilates the sediment, oxygenating deeper layers and stimulating nitrogen cycling (Forster et al., 1999; Van Hoey et al., 2008). Recently the effect of ocean acidification on the behavior and ecophysiology of these species has been investigated. Vlaminck et al. (2022b) found a decreased energy intake and a higher metabolic loss through increased excretion rates for the bivalve. Another study shows that this decreased energy intake, amongst others resulted from a reduced feeding activity (Vlaminck et al., 2022a). Additionally, L. conchilega increased pumping frequency when pH was lowered (Vlaminck et al., 2022a). Whether this behavioral and physiological plasticity to OA, and possible warming-related acclimation, also translates into altered sediment functioning has hitherto not been investigated.
In the present work, two microcosm experiments were conducted, where sediments and Abra alba and L. conchilega individuals were incubated under four different scenarios; a control environment with ambient temperature and pH (CTRL), a scenario with elevated temperature (OW: ocean warming), a scenario with decreased pH (OA: ocean acidification) and a scenario with both an elevated temperature and a decreased pH (CC: climate change). After 6 weeks, nutrient and oxygen fluxes at the sediment-water interface were measured to investigate the effect of A. alba and L. conchilega on the benthic ecosystem metabolism and biogeochemical cycling under the four scenarios. When pH is lowered, we expect the organisms to cope with hypercapnia according to the previously observed behavioral and physiological changes (Vlaminck et al., 2022a; Vlaminck et al., 2022b). More specifically, as A. alba is expected to lower respiratory activity and feeding under low pH, we hypothesize that this species’ contribution to sediment biogeochemistry will decrease. In contrast, as L. conchilega is expected to increase its pumping frequency when exposed to low pH seawater, we hypothesize that this species’ contribution to sediment ecosystem functioning will increase. In addition, ocean warming may alter the contribution of both species, depending on their physiological responses to temperature variability and the warming effects on the metabolic rate of the sediment microbial community.
2 Material and methods
2.1 Field sampling
Sediment for all experiments was collected in June 2019 at station 780 (N:51°28.292’, E:3°3.523’) in the Belgian part of the North Sea; this station is characterized by fine sandy sediments with an organic carbon content of 0.40% and nitrogen content of 0.04-0.07% (Braeckman et al., 2014). Multiple Van Veen grabs were deployed from the Research Vessel Simon Stevin. On board of the ship, sediment was first sieved over a 1-mm mesh to eliminate all macrofauna. Subsequently, the sediment was thoroughly mixed before placing it in the experimental set-up. Abra alba individuals were collected at the same station and with the same gear on August 27th 2019. Lanice conchilega individuals were collected at an intertidal sandy beach in Boulogne-sur-Mer (Nord Pas-de-Calais, France: N: 50.7345, E:1.5881) on July 4th of the same year; we decided to collect the latter species from intertidal sediments since Van Veen grabs damage these organisms and we wanted to ensure collection of complete, undamaged and similarly sized tubes. Lanice conchilega specimens were transported to the laboratory in buckets with sediment and aerated seawater within 4 h after collection, and were incubated in circulating aerated seawater at in situ conditions in a climate-controlled room (Table 1). Water flow (approximately 500 L.h-1) was established between two tanks; one incubation tank (120 L) holding the cores with sediment and macrofauna (see 2.2), and one storage tank (80 L) with the pump. On a weekly basis, 30 L of seawater was refreshed to maintain ambient salinity and avoid nutrient build-up. All systems were subjected to a 12:12 h light:dark regime in a temperature-controlled room.
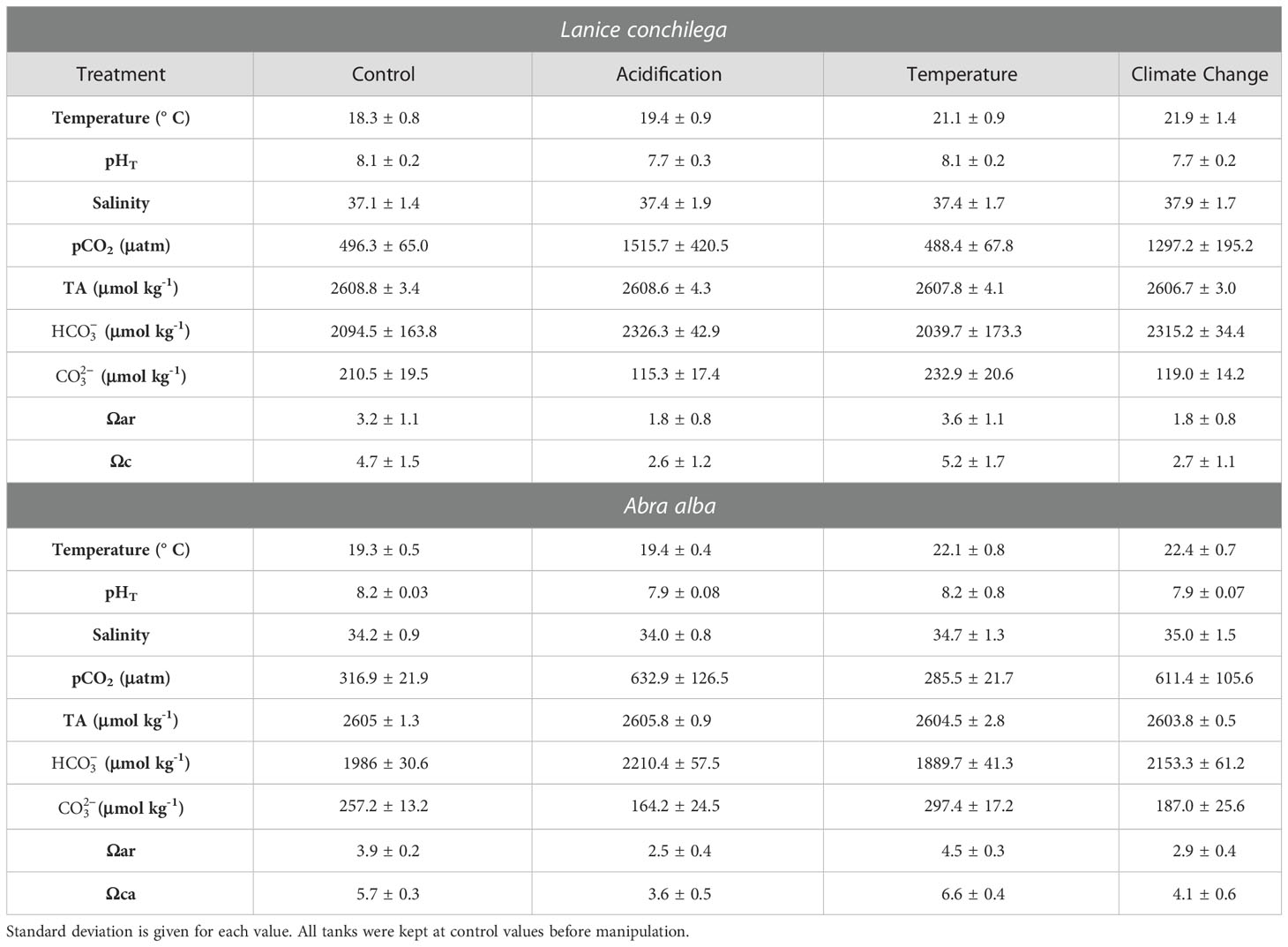
Table 1 Water chemistry over the duration of the experiments: temperature, total pH, salinity, partial pressure of carbon dioxide (pCO2), total alkalinity (TA), bicarbonate concentration (), carbonate concentration (), saturation state of aragonite (Ωar) and saturation state of calcite (Ωc).
2.2 Experimental set-up and seawater manipulation
Separate experiments were conducted with A. alba (from 01/07/2019 to 12/08/2019) and with L. conchilega (from 02/09/2019 to 18/10/2019). 32 Plexiglas cores (10 cm internal diameter) were used in each experiment in order to establish a total of 4 replicates for the 4 seawater condition treatments for each of the two sediment types (with and without macrofauna). All cores were filled with sieved and mixed sediment up to approximately 10 cm and distributed over 16 tank systems, 4 replicate tanks for each of the 4 treatments. Sediment (volume = 0.772 ± 0.006 L) was allowed to settle and stabilize for 7 days, after which A. alba or L. conchilega individuals were added at natural densities (A. alba: n = 9, L. conchilega: n = 6; Van Hoey et al., 2014) to one core in each tank ). A fully crossed experimental design with the factors temperature (ambient or elevated), pH (ambient or lowered) and exposure time (3 or 6 weeks), was followed to investigate the effects of climate change on macrofauna and their effect on sediment biogeochemistry. After one (L. conchilega) and two (A. alba) weeks of acclimatization to ambient conditions, seawater pH was lowered by 0.1 unit per day and temperature was elevated by 1°C per day over a period of three days following a commonly applied gradual change for continental shelf benthos from temperate regions (e.g. Widdicombe and Needham, 2007, Matoo et al., 2013; Voet et al., 2022). Seawater pH manipulation (-0.3 units) was achieved using an IKS Aquastar Industrial control system with pH glass electrodes through the bubbling of pure CO2. PH electrodes were 2-point calibrated on a weekly basis with Hanna instrument buffer solutions (pH 4.01 and 7.01). The climate room was set at ambient temperature and an external heating system (Teco Refrigeration technologies; Model: TK2000) was used to manipulate seawater temperature (+3°C). Temperature and pH were logged every 15 minutes with the IKS Aquastar system. Twice a week, organisms were fed 5 mL of commercial Shellfish Diet 1800, corresponding roughly to 125 mgC m-3 (Reed Mariculture Inc., composed of 40% Isochrysis, 15% Pavlova, 25% Tetraselmis and 20% Thalassiosira weissflogii) diluted in 16 L of seawater and distributed equally in the 16 tanks. On a weekly basis, salinity was measured with a portable conductivity meter (YSI 30M salinity system). Temperature and pH were measured every week with a portable pH/conductivity meter (Metrohm; Model: 914). This pH electrode was calibrated using a TRIS buffer to allow conversion of the continuously measured pH (NBS) data to total (pH) scale (Dickson, 2010). Weekly water samples were taken from each tank and filtered through GF/C filter papers for total alkalinity (TA) measurements. The samples were kept at 4° C until analysis via titration using a HydroFIA TA (CONTROS Systems & Solutions GmbH) at the Flanders Marine Institute (VLIZ). Carbonate chemistry parameters (pHT, pCO2, , , Ωc and Ωa) were derived from the measured values for temperature, pH, salinity and TA via CO2SYS software (Pierrot et al., 2011)(Table 1). Mortality was checked daily and dead organisms (L. conchilega: n = 2, A. alba: n = 61) were removed to avoid nutrient build-up.
2.3 Flux measurements and sampling
After three and six weeks of incubation, the sediment community oxygen consumption (SCOC) and nutrient exchange at the sediment water interface were quantified, using closed dark incubations of the cores. Sediment cores were closed airtight, cutting them off from the circulation system and allowing us to measure changes in oxygen and nutrient concentrations in the water column. The overlaying sea water was mixed to avoid stratification at a rate low enough to avoid resuspension of the sediment. Oxygen concentration in the overlaying sea water was continuously monitored with Robust Oxygen Optodes (OXROB 10, Pyroscience sensor technology). An optical oxygen meter (FirestingO2, Pyroscience) was used to control the sensors. Incubations were run for 3 h, sufficient to measure changes in oxygen and nutrient concentrations while the oxygen concentration of the seawater remained above 60% saturation. Water samples (10 mL) were taken from the total volume of water (0.728 ± 0.006 L) in the cores, and filtered through Whatman GF/F filters, at the start and end of the closed incubation, to determine nutrient concentrations.
Oxygen fluxes were calculated from the regression slopes of the oxygen concentration over time, according to Eq. (1).
where is the change in oxygen concentration in the overlying water (in mmol L−1 d−1), V is the volume of the overlying water (in L), and A is the sediment surface area (in m2).
Nutrient fluxes were calculated as the difference between the nutrient concentrations at the end and start of the incubation. After the closed-core incubations after 3 weeks of incubation, the cores were reconnected to the normal water circulation, in order to be sampled again after 6 weeks of incubation.
Oxygen Penetration Depth (OPD) were measured in the sediment after 5 weeks of incubation. Oxygen microsensors (Unisense OX100, 100 µm tip size) were vertically inserted into the sediment and moved with a Unisense microprofiler at increments of 250 µm (two replicate profiles in each core). SensorTracePro software (Unisense) was used to visualize the output. The O2 microsensor was 2-point calibrated with a 0% oxygen solution (Na-ascorbate solution) and a 100% oxygen solution (obtained through oxygenation of seawater through bubbling).
2.4 Mass budget calculations
Nitrification, denitrification and total mineralization were estimated using the sediment-water exchange fluxes of O2, NOx and NHx. A mass budget of oxygen, nitrate and ammonium in the sediment was constructed as a function of source and sink processes (Soetaert et al., 2001; Braeckman et al., 2010). Oxygen, nitrate and ammonium are exchanged across the sediment-water interface. Oxygen is consumed through the oxidation of organic carbon (oxic mineralization) and of ammonium to nitrate (nitrification), or through the re-oxidation of reduced substances formed through anoxic mineralization. The O:C ratio is assumed to equal 1 (Redfield), while 2 moles of oxygen are required for the oxidation of ammonium to nitrate (Soetaert et al., 2001). Ammonium is the end product of organic nitrogen mineralization and is consumed during denitrification. Nitrification produces nitrate but 0.8 moles of NO3 are being consumed for each mole of carbon that is denitrified. Part of the reduced substances remain buried in the sediment and are therefore not available for re-oxidation (Soetaert et al., 2001).
The rates of change are set to zero, based on the assumption that a geochemical steady state is reached approximately 1 week after the addition of organisms. We assume that the burial of anoxic substances is negligible, allowing us to combine oxic and anoxic mineralization (Braeckman et al., 2010). To solve the model, an extra equation is added, using the N:C ratio (mean value = 0.1351 mol N mol C-1) to appoint the relationship between nitrogen and carbon mineralization, resulting in a set of equations (that were solved using the limSolve package (Soetaert and Van den Meersche, 2017) in the open source software R version 4.0.4 (R Core Team, 2014).
2.5 Statistical analyses
Differences in survival of study organisms between treatments at the end of incubation were analyzed using two-way analysis of variances (ANOVA) with temperature and pH as fixed factors, with two levels each (ambient and increased temperature; ambient and lowered pH). Normal distribution and equal variances were assumed and tested with Shapiro and Levene’s test respectively. If significant effects were found, Tukey HSD tests were performed to identify pairwise differences. To test the effect of A. alba or L. conchilega (present or absent), temperature, pH, and time (three or six weeks of incubation) and their interaction on biogeochemical fluxes, linear mixed effects models were constructed for each factor. We allowed random intercepts for tank identity to take the non-independence of the two cores in each tank into account. Step-by-step deletion of single model terms that were not involved in any significant interaction led to the minimal adequate model. The Kenward-Roger method was used to estimate degrees of freedom and calculate F-statistics and associated p-values. When no warming x pH interaction effects are present, the model compares the combined acidified treatments (OA and CC) with the non-acidified treatments (OW and CTRL) combined and/or the warmed (OW and CC) with the non-warmed (CTRL and OA) treatments. Model assumptions were checked graphically; the normality of residuals was numerically confirmed with a Shapiro-Wilk test. Statistical analysis was conducted in R-software version 4.0.4 (R Core Team, 2014) with packages lme4 (Bates et al., 2014), lmerTest (Kuznetsova et al., 2017) and MuMIn packages (Barton, 2020).
3 Results
3.1 Macrofauna survival
There was no significant temperature, pH or interaction effect on the survival of L. conchilega. Increased seawater temperature did, however, significantly reduce the survival of A. alba (p-value< 0.05), decreasing its survival by 34 (± 0.08) % after 6 weeks of incubation under both warmed conditions (i.e. warming; 50 ± 19%,and climate change: 42 ± 13%) compared to the control (67 ± 15%) and ocean acidification (72 ± 11%) conditions (Figure 1; Table 2).
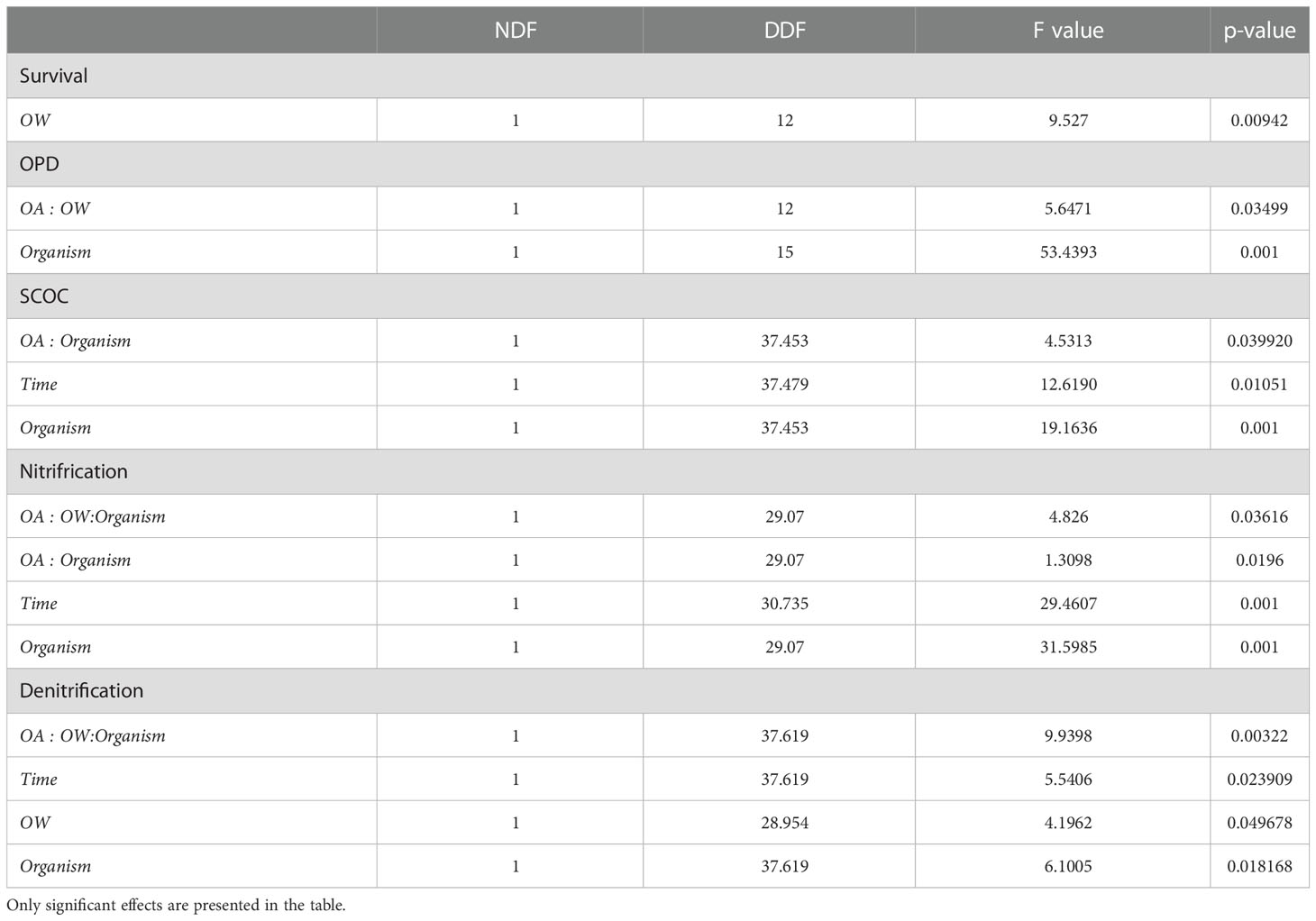
Table 2 Linear mixed effects models comparing OPD and biogeochemical cycling in different treatments; seawater temperature, seawater pH, Abra alba presence and experimental duration.
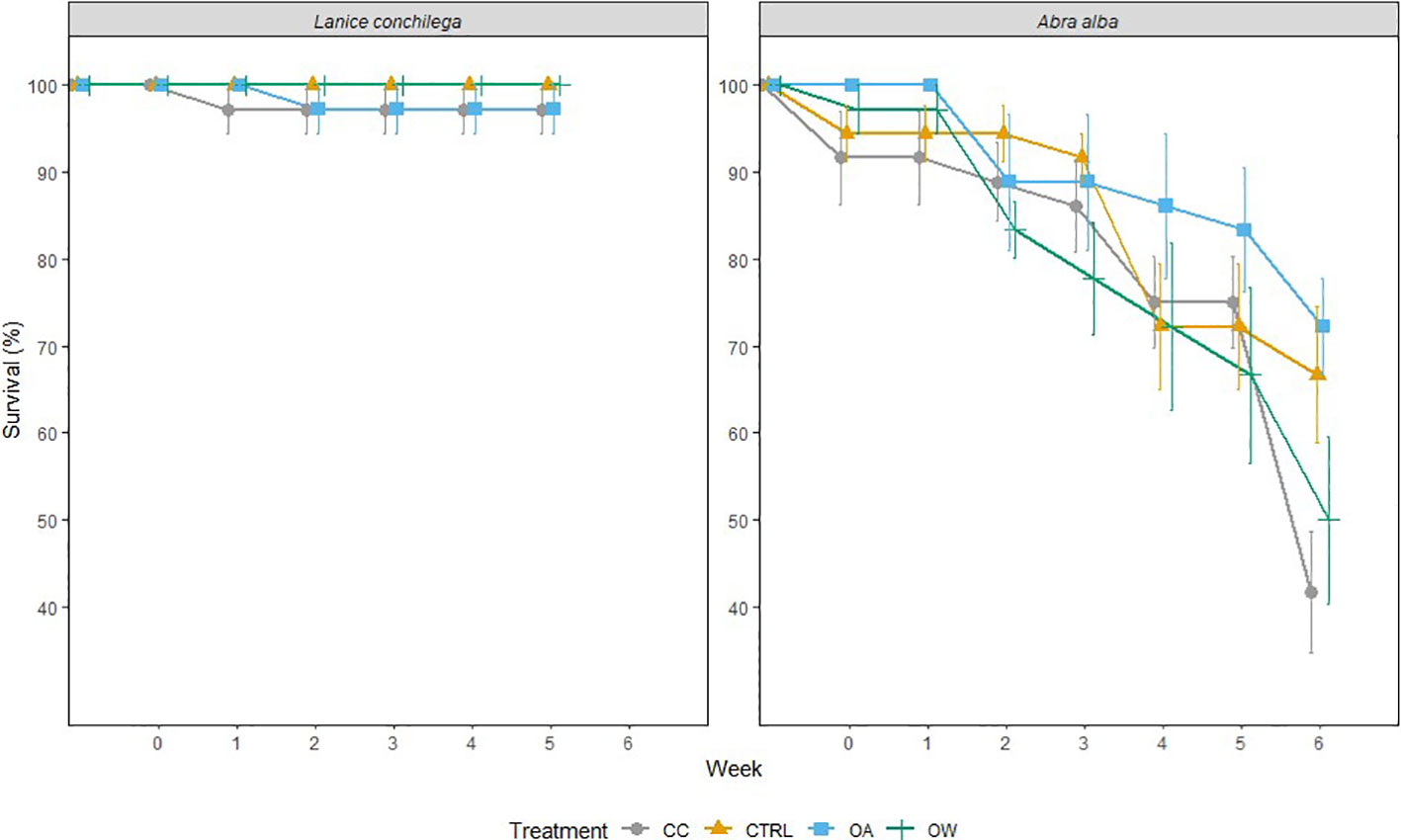
Figure 1 Effects of temperature and pH on the survival rate of A. alba and L. conchilega during the 6-week incubation period. Error bars represent standard errors (± SE, n = 36).
3.2 Nutrient and oxygen fluxes and cycling
3.2.1 Lanice conchilega
The presence of L. conchilega significantly increased OPD by 31 (± 1) %, compared to cores with no L. conchilega individuals present (Figure 2). Furthermore, L. conchilega increased SCOC by 28% under ambient conditions, whereas stimulation in an acidified environment was even greater with a 37 (± 8) % increase (Table 3, interaction effect of OA and organism presence; Figure 3; pairwise p-value< 0.001). Similarly, acidified conditions increased the stimulatory effect of L. conchilega on nitrification and denitrification, with respective increases of 41 (± 7) % and 25 (± 7) %, when comparing all non-acidified treatments with all acidified treatments (Table 3, interaction effect of OA and organism; Figure 3; pairwise p-values< 0.001). On average the highest stimulation was found in the CC treatment, although this effect was not significant (p-value = 0.842). Experimental duration did not affect fluxes, therefore time was pooled in the graphic representation of the results (Figure 3).
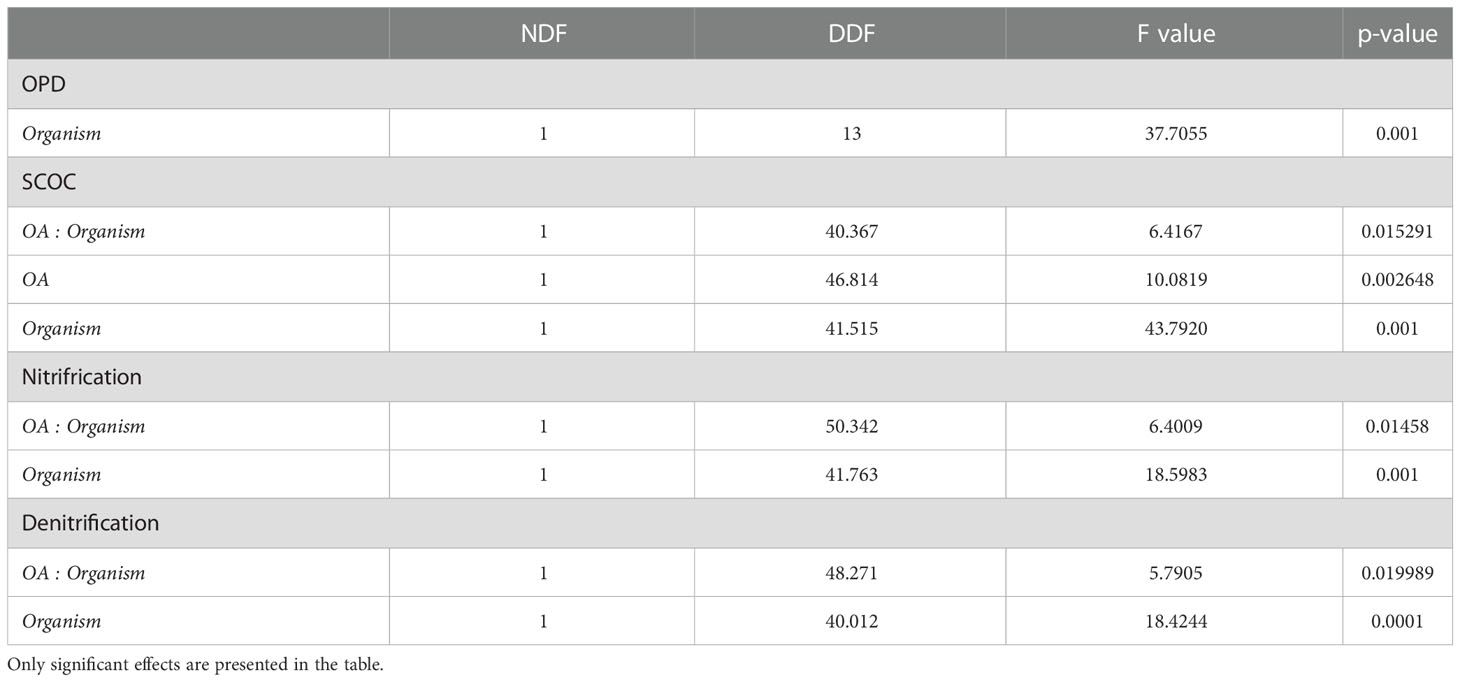
Table 3 Linear mixed effects models comparing OPD and biogeochemical cycling in different treatments; seawater temperature, seawater pH, Lanice conchilega presence and experimental duration.
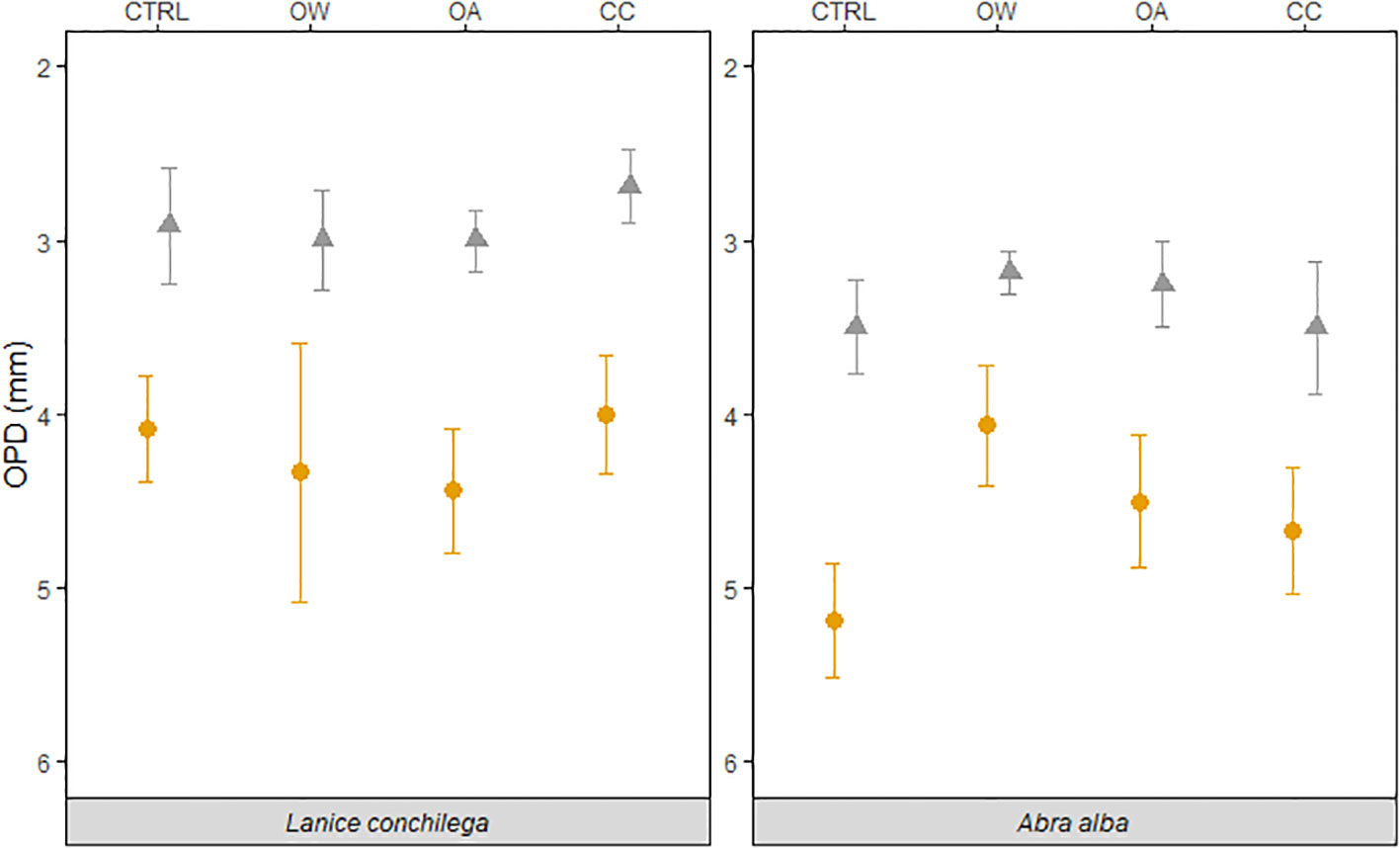
Figure 2 Temperature and pH effect on the oxygen penetration depth in the presence (orange circles) and absence (grey triangles) of L. conchilega and A. alba. Error bars represent the standard errors (± SE, n = 4).
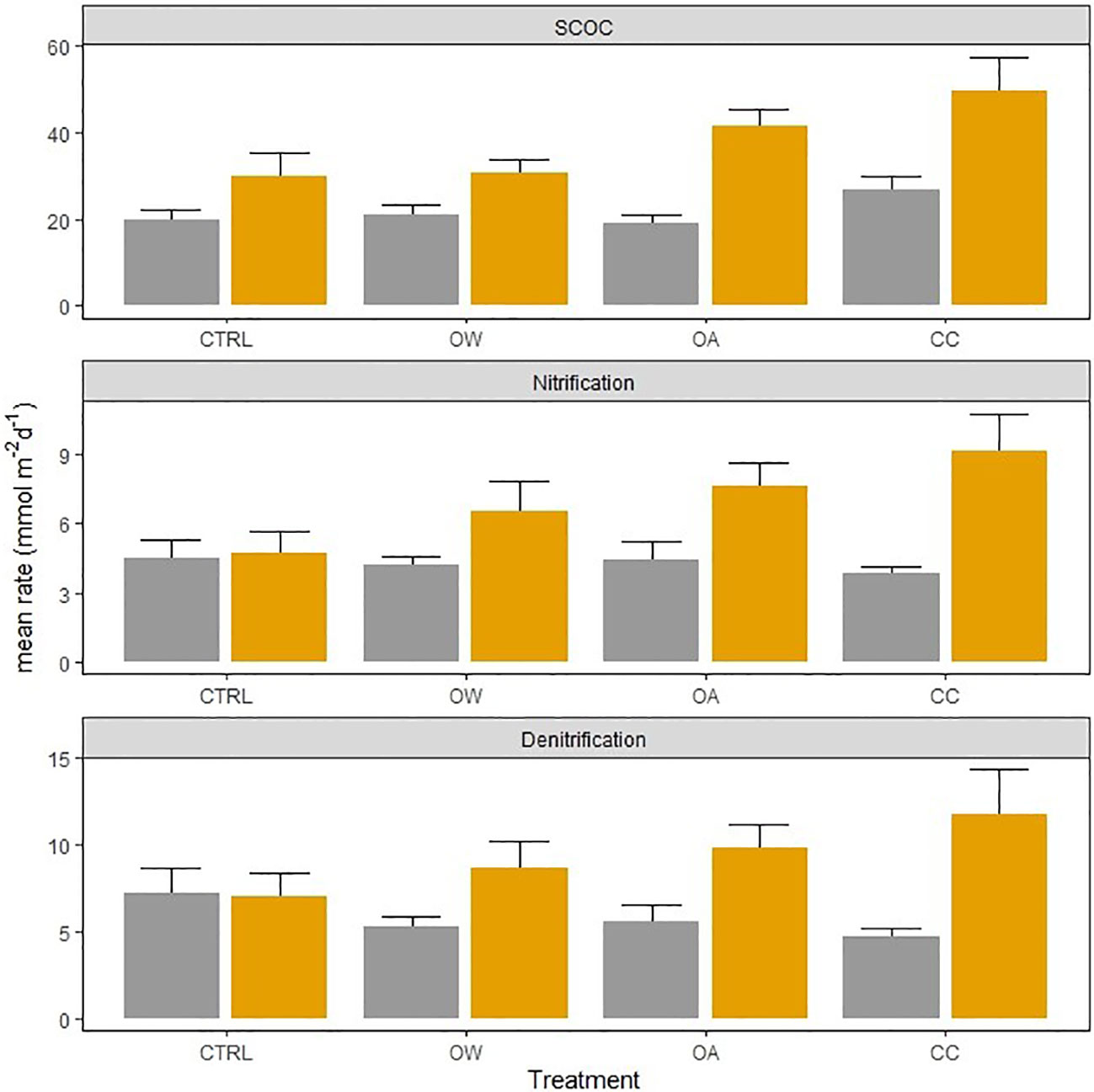
Figure 3 Effect of Lanice conchilega presence (present: orange bars, absent: grey bars) on sediment community oxygen consumption (SCOC, mmol O2 m-2 d-1), nitrification, and denitrification rates (mmol N m-2 d-1), in 4 different treatments, with different times pooled because experiment duration did not affect the fluxes and processes. Error bars represent standard errors (± SE).
3.2.2 Abra alba
Experimental duration significantly affected nitrification and denitrification rates, with respective decreases of 23 (± 6) % and 22 (± 1) % after six weeks of incubation compared to three weeks of incubation (Table 2, Time effect; Figure 1).
Abra alba presence increased OPD by 27 (± 6) % in all treatments, when compared to all cores without A. alba presence (Table 2, organism effect; Figure 2). Irrespective of the presence of A. alba, the interaction of seawater pH and temperature significantly affected OPD (Table 2, interaction effect of OA and OW; Figure 2). On average, OPD in sediments with Abra was deeper in CTRL when compared to OW, OA or CC (17%, 19%, and 6%, respectively).
In non-acidified conditions, A. alba significantly stimulated SCOC resulting in an increase of 35 (± 15) % averaged over time (Table 2, interaction effect of OA and organism; Figure 4; pairwise p-value< 0.001). Although the stimulation was on average larger in the CC treatment with A. alba, the statistical comparison revealed that SCOC rates did not differ significantly between the sediment with and without A.alba in the CC treatment (p = 0.086). This stimulatory effect was absent under acidified conditions. Nitrification and denitrification were both stimulated by the presence of A. alba in a control environment (pairwise p-value = 0.001 and 0.010 respectively). This stimulatory effect was absent when either seawater temperature was raised (OW) or pH was lowered (OA) (pairwise p-values all< 0.05). However, nitrification and denitrification rates were stimulated by the presence of A. alba under combined warming and acidification, i.e. the CC scenario, with an increase of 61 (± 5) % and 110 (± 1) % averaged over time respectively (pairwise p-values: 0.008 and 0.010 respectively; Table 2: OW, OA and organism interaction effect; Figure 4).
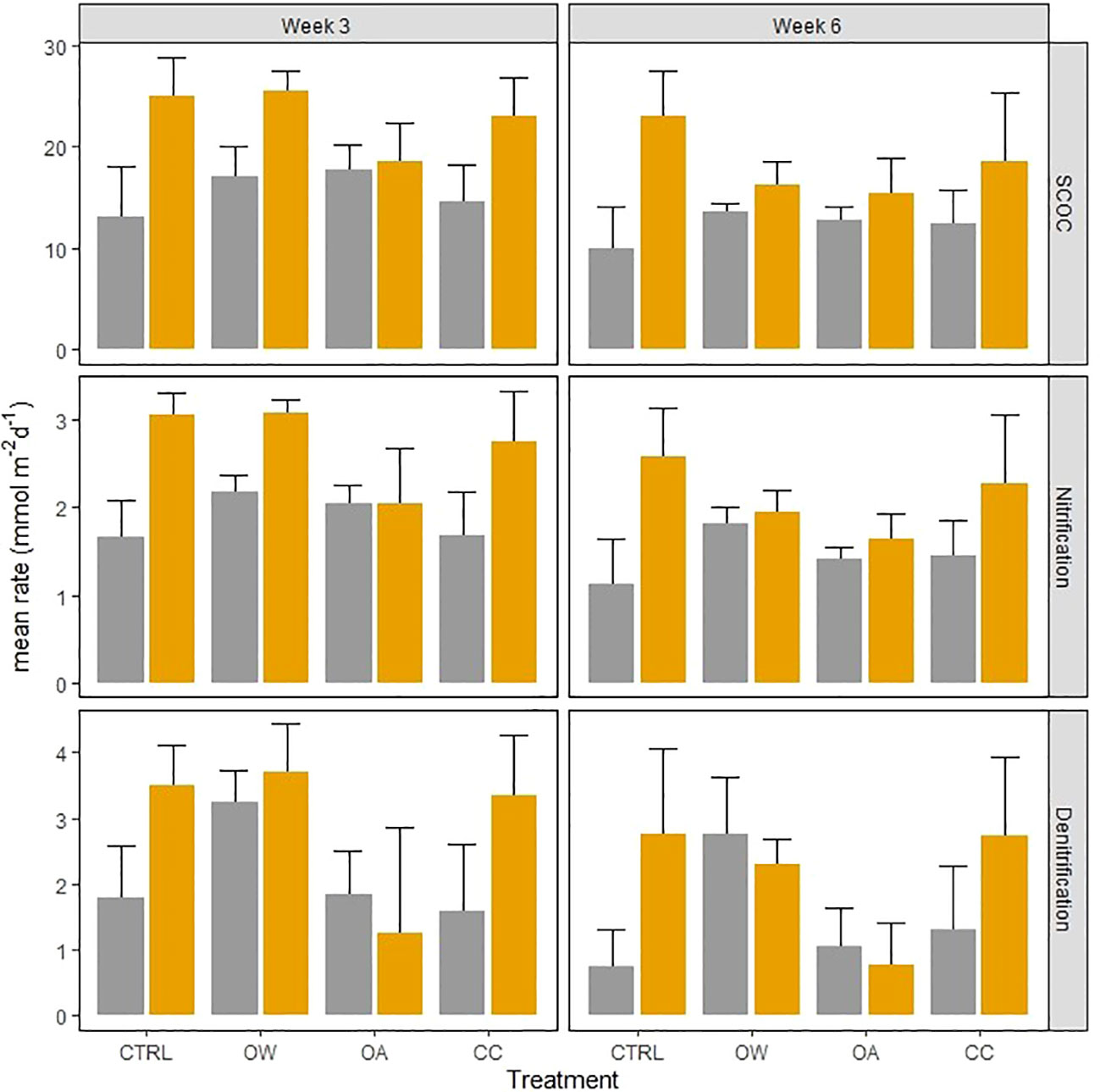
Figure 4 Effect of Abra alba presence (present: orange bars, absent: grey bars) on sediment community oxygen consumption (SCOC, mmol O2 m-2 d-1), nitrification and denitrification rates (mmol N m-2 d-1), in 4 different treatments at 2 different times. Error bars represent standard errors (± SE).
4 Discussion
This experimental study investigated climate change effects on sediment ecosystem functioning, in particular how macrofauna bioturbation affect nitrogen cycling under simulated climate change conditions.”. Interestingly, the effect of climate change differed between the two investigated key species. Both species facilitate biogeochemical cycling under ambient conditions. Lanice conchilega stimulates sediment aerobic metabolism and biogeochemical cycling more when seawater becomes acidified, while warming has no significant effect on the species contribution. Contrary, Abra alba contributes less in an acidified environment and the contribution in the warmer treatments decreases over time along with lower densities. The measured N cycling process rates in this study, using strongly manipulated and reduced communities, are generally slightly lower than the natural rates described by Toussaint et al. (2021) for the same location of sediment collection. Nevertheless, the chosen approach does allow for empirical proof and understanding on how different aspects of climate change, i.e. ocean warming and acidification, modify the contribution of marine benthic fauna to nitrogen cycling.
Laboratory and climate change conditions had no effect on Lanice conchilega survival while Abra alba survival was reduced both by experiment duration and ocean warming. Although both species are widely spread in temperate continental shelf sea beds, the more abundant occurrence of L. conchilega in warmer regions such as the coastlines of Mauritius, Madagascar and Mozambique (Day, 1968; Lewinsohn and Fishelson, 1967) as well as this species’ presence in the lower intertidal zone of estuaries and beaches (Alves et al., 2017) that are prone to strong diurnal fluctuations in temperature and dryness confirms that this species is more tolerant to suboptimal laboratory conditions, as well as to increased temperature in general.
Abra alba and Lanice conchilega individuals increased oxygen penetration depth in an environment with ambient pH and temperature which corroborates the found stimulatory effect on biogeochemical cycling of both species under those conditions. Pumping activity of Lanice conchilega increased oxygen penetration by 1.32 (± 0.16) mm which is similar to the 1.2 mm increase found for similar organism densities by Braeckman et al. (2010). Interestingly, even though L. conchilega is known to increase pumping frequency in a similarly acidified environment by 30% (Vlaminck et al., 2022a), oxygen penetration depth did not differ across treatments suggesting that in this experiment seawater conditions did not affect tube structure or ventilation magnitude which could lead to change in oxygenation depth (Kristensen et al., 2012). Such increased pumping frequency allows the worm to renew the O2 pool faster in order to cope with the higher metabolic cost associated with inhabiting low pH environments (Briffa et al., 2012; Turner et al., 2015). This was for example also observed for the polychaete Alitta virens (Godbold and Solan, 2013) while a similar bio-irrigation response to other environmental stressors, like hypoxia and increased temperature, has been found for different polychaete species (Kristensen, 1983; Forster and Graf, 1995; Ouellette et al., 2004). The pumping of L. conchilega transports nitrate into the sediment and increases the availability of oxygen in deeper layers of the sediment (Forster and Graf, 1995), increasing the surface for coupled nitrification-denitrifcation, stimulating these processes. Foshtomi et al. (2018) furthermore proofs that L. conchilega influences the composition and density of denitrifying communities by changing the availability of nitrate. Higher diversity at depth was observed in L. conchilega patches, resulting in high denitrification rates. Increased irrigation activity under low pH conditions can thus explain the observed increased nitrification and denitrifcation rates under simulated ocean acidification and climate change in our study. Warming did however not significantly affect this species contribution to biogeochemical cycling, most likely due to the abovementioned tolerance to temperature fluctuations.
A. alba did not stimulate nitrogen cycling in ocean acidification or ocean warming conditions as was the case under ambient and climate change conditions. After 6 weeks of incubation, the stimulatory effect was greatest under ambient conditions where sediment oxygenation was, on average, the deepest in comparison to the other treatments. In a similarly acidified environment, A. alba reduced respiration and feeding, especially suspension feeding which is known to create the greatest subsurface advective flows (and hence stimulatory effects via sediment oxygenation) by tellinid bivalves (Volkenborn et al., 2012; Vlaminck et al., 2022a). These behavioral changes reduce the uptake of low-pH seawater, thereby limiting possible physiological costs related to hypercapnia but at the same time also reduce particle mixing and bio-irrigation, which can therefore explain the observed absence of A. alba contribution on all measured functions (SCOC, nitrification and denitrification) under ocean acidification in this study. Interestingly, when combined with warming (i.e. the climate change treatment), A.alba did stimulate nitrogen cycling under reduced pH. This difference can possibly be attributed to the lower costs for calcification with higher temperature (Clarke, 1993; Mancuso et al., 2019) as demonstrated by the higher saturation state of calcite and aragonite in both warmer treatments (Table 1). This may leave more energy for feeding and maneuvering in comparison to the single pH treatment where warming was not present, thereby stimulating biogeochemical cycling. This effect however seems to decrease with time when densities dropped at warmer temperatures, i.e. the ocean warming and climate change treatments. Braeckman et al. (2010) studied the effect of A.alba density on bioturbation and its contribution to sediment biogeochemistry. They found decreasing bioturbation rates and ammonium effluxes with decreasing density of A. alba. After six weeks of incubation only four A. alba individuals remained in the warmer treatments in our study, which is a similar density as the lowest that was used by Braeckman et al. (2010), where bioturbation was 78% lower compared to natural densities. Our study shows that at such reduced densities the stimulatory effect can disappear especially in the warming treatment where biogeochemical cycling is boosted in the absence of A. alba.
In conclusion, we show that ocean warming and acidification change the contribution of macrofauna to benthic ecosystem functioning, i.e. biogeochemical cycling. Declines in macrofauna activity or density, as observed for A. alba, will ultimately lead to major changes in the contribution of benthos to global nitrogen and carbon cycling (Sweetman et al., 2017; Snelgrove et al., 2018). However other effects, such as observed for L. conchilega, where ocean acidification increased the contribution of this species to overall sediment metabolism and biogeochemical cycling, could mitigate such reduced sediment functioning related to climate change. The variable results for both key-species depending on physiological tolerance and behavioral plasticity highlight the difficulty to upscale population studies to the community and ecosystem level (Ehrnsten et al., 2020).
Data availability statement
The raw data supporting the conclusions of this article will be made available by the authors, without undue reservation.
Author contributions
EV and CC contributed to conception and design of the study. EV acquired the data and performed the statistical analysis with the input of UB and CC. EV, CC, UB and TM performed the interpretation of the data. EV wrote the first draft of the manuscript. All authors contributed to the article and approved the submitted version.
Funding
Funding for the research leading to this publication was provided through the BRAIN-be project BR/175/A1/PERSUADE of the Belgian Science Policy Office. Additional funding was obtained from the Special Research Fund (BOF) of Ghent University through GOA project 01G02617. Experiments used infrastructure funded by the Flemish Science Fund FWO through project GOH3817N in the framework of EMBRC-Belgium.
Acknowledgments
Sampling at sea was performed with logistic support from the Research Vessel Simon Stevin. The authors thank Helena Voet, Hannelore Theetaert, Bart Beuselinck, Bruno Vlaeminck, Annick Van Kenhove, Guy De Smet and Elise Toussaint for their practical and analytical support.
Conflict of interest
The authors declare that the research was conducted in the absence of any commercial or financial relationships that could be construed as a potential conflict of interest.
Publisher’s note
All claims expressed in this article are solely those of the authors and do not necessarily represent those of their affiliated organizations, or those of the publisher, the editors and the reviewers. Any product that may be evaluated in this article, or claim that may be made by its manufacturer, is not guaranteed or endorsed by the publisher.
Supplementary material
The Supplementary Material for this article can be found online at: https://www.frontiersin.org/articles/10.3389/fmars.2023.1101972/full#supplementary-material
References
Allan R. P., Hawkins E., Bellouin N., Collins B. (2021). IPCC, 2021: Summary for Policymakers. Global Warming of 1.5 °C. An IPCC Special Report on the Impacts of Global Warming of 1.5 °C above Pre-Industrial Levels and Related Global Greenhouse Gas Emission Pathways, in the Context of Strengthening the Global Res. IPCC: Geneva, Switzerland, 2018.
Alma L., Kram K. E., Holtgrieve G. W., Barbarino A., Fiamengo C. J., Padilla-Gamiño J. L. (2020). Ocean acidification and warming effects on the physiology, skeletal properties, and microbiome of the purple-hinge rock scallop. Comp. Biochem. Physiol. Part A Mol. Integr. Physiol. 240, 110579. doi: 10.1016/j.cbpa.2019.110579
Alves R. M. S., Van Colen C., Vincx M., Vanaverbeke J., De Smet B., Guarini J.-M., et al. (2017). A case study on the growth of lanice conchilega (Pallas 1766) aggregations and their ecosystem engineering impact on sedimentary processes. J. Exp. Mar. Bio. Ecol. 489, 15–23. doi: 10.1016/j.jembe.2017.01.005
Bates D., Mächler M., Bolker B. M., Walker S. C. (2014). Fitting linear mixed-effects models using lme4. J. Stat. Software 67. doi: 10.18637/jss.v067.i01
Bernard G., Grémare A., Maire O., Lecroart P., Meysman F. J. R., Ciutat A., et al. (2012). Experimental assessment of particle mixing fingerprints in the deposit-feeding bivalve abra alba (wood). J. Mar. Res. 70, 689–718. doi: 10.1357/002224012806290705
Bindoff N. L., Cheung W. W. L., Kairo J. G., Arístegui J., Guinder V. A., Hallberg R., et al. (2019). Changing ocean, marine ecosystems, and dependent communities. Eds. Pörtner H.-O., Roberts D., Masson-Delmotte V., Zhai P., Tignor M., Poloczanska E. (Switzerland: Intergovernmental Panel on Climate Change).
Braeckman U., Foshtomi M. Y., Van Gansbeke D., Meysman F., Soetaert K., Vincx M., et al. (2014). Variable importance of macrofaunal functional biodiversity for biogeochemical cycling in temperate coastal sediments. Ecosystems 17, 720–737. doi: 10.1007/S10021-014-9755-7
Braeckman U., Provoost P., Gribsholt B., Van Gansbeke D., Middelburg J. J., Soetaert K., et al. (2010). Role of macrofauna functional traits and density in biogeochemical fluxes and bioturbation. Mar. Ecol. Prog. Ser. 399, 173–186. doi: 10.3354/meps08336
Brewer P. G. (2009). A changing ocean seen with clarity. Proc. Natl. Acad. Sci. U. S. A. 106, 12213–12214. doi: 10.1073/pnas.0906815106
Briffa M., de la Haye K., Munday P. L. (2012). High CO 2 and marine animal behaviour: Potential mechanisms and ecological consequences. Mar. pollut. Bull. 64, 1519–1528. doi: 10.1016/j.marpolbul.2012.05.032
Byrne M. (2011). Impact of ocean warming and acidification on marine invertebrate life histroy stages: vulnerabilities and potential for persistence in a changing ocean. Oceanogr. Mar. Biol. Annu. Rev. 49, 1–42.
Cai W.-J., Huang W.-J., Luther G. W., Pierrot D., Li M., Testa J., et al. (2017). Redox reactions and weak buffering capacity lead to acidification in the Chesapeake bay. Nat. Commun. 8, 1–12. doi: 10.1038/s41467-017-00417-7
Caldeira K., Wickett M. E. M. E. (2003). Anthropogenic carbon and ocean pH. Nature 425, 365. doi: 10.1038/425365a
Carstensen J., Duarte C. M. (2019). Drivers of pH variability in coastal ecosystems. Environ. Sci. Technol. 53, 4020–4029. doi: 10.1021/ACS.EST.8B03655
Clarke A. (1993). Temperature and extinction in the sea: a physiologist’s view. Paleobiology 19, 499–518. doi: 10.1017/S0094837300014111
Collins M., Knutti R., Arblaster J., Dufresne J., Fichefet T., Friedlingstein P., et al. (2013). Long-term climate change: Projections, commitments and irreversibility. clim. chang. 2013 phys. sci. basis work. gr. I contrib. to fifth assess. rep. intergov. panel clim. Chang. 1029–1136.
Currie A. R., Tait K., Parry H., de Francisco-Mora B., Hicks N., Osborn A. M., et al. (2017). Marine microbial gene abundance and community composition in response to ocean acidification and elevated temperature in two contrasting coastal marine sediments. Front. Microbiol. 8, 1599. doi: 10.3389/fmicb.2017.01599
Day J. H. (1968). A Monograph on the Polychaeta of Southern Africa Part 1, Errantia: Part 2, Sedentaria Published by the Trustees of the British Museum (Natural History), London, 1967 Publication no. 656. Pp. viii+ 878. Price£ 15. J Mar Biol Assoc UK 48 (3), 836–836.
De Smet B., D’Hondt A. S., Verhelst P., Fournier J., Godet L., Desroy N., et al. (2015). Biogenic reefs affect multiple components of intertidal soft-bottom benthic assemblages: The lanice conchilega case study. Estuar. Coast. Shelf Sci. 152, 44–55. doi: 10.1016/j.ecss.2014.11.002
Dickson A. G. (2010). The carbon dioxide system in seawater: Equilibrium chemistry and measurements. In Riebesell U., Fabry V. J, Hansson L. Eds. Guide to best practices for ocean acidification research and data reporting (Luxembourg: Publications Office of the European Union) 1, 17–40.
Dupont S., Pörtner H. (2013). Marine science: Get ready for ocean acidification. Nature 498, 429. doi: 10.1038/498429a
Ehrnsten E., Sun X., Humborg C., Norkko A., Savchuk O. P., Slomp C. P., et al. (2020). Understanding environmental changes in temperate coastal seas: Linking models of benthic fauna to carbon and nutrient fluxes. Front. Mar. Sci. 7. doi: 10.3389/FMARS.2020.00450
Fabry V. J., Seibel B. A., Feely R. A., Orr J. C. (2008). Impacts of ocean acidification on marine fauna and ecosystem processes. ICES J. Mar. Sci. 65, 414–432. doi: 10.1093/icesjms/fsn048
Figuerola B., Hancock A. M., Bax N., Cummings V. J., Downey R., Griffiths H. J., et al. (2021). A review and meta-analysis of potential impacts of ocean acidification on marine calcifiers from the southern ocean. Front. Mar. Sci. 8. doi: 10.3389/fmars.2021.584445
Forster S., Glud R. N., Gundersen J. K., Huettel M. (1999). In situ study of bromide tracer and oxygen flux in coastal sediments. Estuar. Coast. Shelf Sci. 49, 813–827. doi: 10.1006/ecss.1999.0557
Forster S., Graf G. (1995). Impact of irrigation on oxygen flux into the sediment: intermittent pumping by callianassa subterranea and “piston-pumping” by lanice conchilega. Mar. Biol. 123, 335–346. doi: 10.1007/BF00353625
Foshtomi M. Y., Leliaert F., Derycke S., Willems A., Vincx M., Vanaverbeke J. (2018). The effect of bio-irrigation by the polychaete Lanice conchilega on active denitrifiers: distribution, diversity and composition of nosZ gene. PLoS One 13 (2), e0192391.
Godbold J. A. J. A., Solan M. (2013). Long-term effects of warming and ocean acidification are modified by seasonal variation in species responses and environmental conditions. Philos. Trans. R. Soc B 368, 20130186. doi: 10.1098/rstb.2013.0186
Hartman S. E., Humphreys M. P., Kivimäe C., Woodward E. M. S., Kitidis V., McGrath T., et al. (2019). Seasonality and spatial heterogeneity of the surface ocean carbonate system in the northwest European continental shelf. Prog. Oceanogr. 177, 101909. doi: 10.1016/j.pocean.2018.02.005
Hartmann-Schröder G. (1996). Annelida, Borstenwürmer, Polychaeta. Jena, Germany: Gustav Fisher Verlag.
Hurd C. L., Beardall J., Comeau S., Cornwall C. E., Havenhand J. N., Munday P. L., et al. (2019). Ocean acidification as a multiple driver: how interactions between changing seawater carbonate parameters affect marine life. Mar. Freshw. Res. 71, 263–274. doi: 10.1071/MF19267
Kristensen E. (1983). Ventilation and oxygen uptake by three species of nereis (Annelida : Polychaeta). 11. effects of temperature and salinity changes. Mar. Ecol. Prog. Ser. 12, 299–306. doi: 10.3354/meps012299
Kristensen E., Penha-Lopes G., Delefosse M., Valdemarsen T., Quintana C. O., Banta G. T. (2012). What is bioturbation? the need for a precise definition for fauna in aquatic sciences. Mar. Ecol. Prog. Ser. 446, 285–302. doi: 10.3354/meps09506
Kuznetsova A., Brockhoff P. B., Christensen R. H. B. (2017). lmerTest package: Tests in linear mixed effects models. J. Stat. Software 82, 1–26. doi: 10.18637/JSS.V082.I13
Laverock B., Gilbert J., Tait K., Osborn A. M., Widdicombe S. (2011). Bioturbation: impact on the marine nitrogen cycle. Biochem. Soc Trans. 39, 315–320. doi: 10.1042/BST0390315
Lemasson A. J., Fletcher S., Hall-Spencer J. M., Knights A. M. (2017). Linking the biological impacts of ocean acidification on oysters to changes in ecosystem services: a review. J. Exp. Mar. Bio. Ecol. 492, 49–62. doi: 10.1016/j.jembe.2017.01.019
Lewinsohn C., Fishelson L. (1967). The second Israel south red Sea expedition 1965, (General report). Isr. J. Zool. 16, 59–68.
Mancuso A., Stagioni M., Prada F., Scarponi D., Piccinetti C., Goffredo S. (2019). Environmental influence on calcification of the bivalve chamelea gallina along a latitudinal gradient in the Adriatic Sea. Sci. Rep. 9, 1–11. doi: 10.1038/s41598-019-47538-1
Matoo O. B., Ivanina A. V., Ullstad C., Beniash E., Sokolova I. M. (2013). Interactive effects of elevated temperature and (CO2) levels on metabolism and oxidative stress in two common marine bivalves (Crassostrea virginica and mercenaria mercenaria). Comp. Biochem. Physiol. Part A Mol. Integr. Physiol. 164, 545–553. doi: 10.1016/j.cbpa.2012.12.025
Mermillod-Blondin F., Rosenberg R., François-Carcaillet F., Norling K., Mauclaire L. (2004). Influence of bioturbation by three benthic infaunal species on microbial communities and biogeochemical processes in marine sediment. Aquat. Microb. Ecol. 36, 271–284. doi: 10.3354/ame036271
Meysman F., Middelburg J., Heip C. (2006). Bioturbation: a fresh look at darwin’s last idea. Trends Ecol. Evol. 21, 688–695. doi: 10.1016/j.tree.2006.08.002
Nagelkerken I., Munday P. L. P. L. (2016). Animal behaviour shapes the ecological effects of ocean acidification and warming: moving from individual to community-level responses. Glob. Change Biol. 22, 974–989. doi: 10.1111/gcb.13167
Omar A. M., Thomas H., Olsen A., Becker M., Skjelvan I., Reverdin G. (2019). Trends of ocean acidification and pCO2 in the northern north Sea 2003–2015. J. Geophys. Res. Biogeosci. 124, 3088–3103. doi: 10.1029/2018JG004992
Ong E. Z., Briffa M., Moens T., Van Colen C. (2017). Physiological responses to ocean acidification and warming synergistically reduce condition of the common cockle cerastoderma edule. Mar. Environ. Res. 130, 38–47. doi: 10.1016/j.marenvres.2017.07.001
Ouellette D., Desrosiers G., Gagne J. P., Gilbert F., Poggiale J. C., Blier P. U., et al. (2004). Effects of temperature on in vitro sediment reworking processes by a gallery biodiffusor, the polychaete neanthes virens. Mar. Ecol. Prog. Ser. 266, 185–193. doi: 10.3354/meps266185
Pierrot D. E., Wallace D. W. R., Lewis E. (2011). MS excel program developed for CO2 system calculations. doi: 10.3334/CDIAC/OTG.CO2SYS_XLS_CDIAC105A
Pörtner H. O., Langenbuch M., Reipschläger A. (2004). Biological impact of elevated ocean CO2 concentrations: Lessons from animal physiology and earth history. J. Oceanogr. 60, 705–718. doi: 10.1007/s10872-004-5763-0
Provoost P., Van Heuven S., Soetaert K., Laane R., Middelburg J. J. (2010). Long-term record of pH in the Dutch coastal zone: a major role for eutrophication-induced changes. Biogeosci. Discuss 7, 4127–4152. doi: 10.5194/bgd-7-4127-2010
Rabaut M., Guilini K., Van Hoey G., Vincx M., Degraer S. (2007). A bio-engineered soft-bottom environment: The impact of lanice conchilega on the benthic species-specific densities and community structure. Estuar. Coast. Shelf Sci. 75, 525–536. doi: 10.1016/j.ecss.2007.05.041
R Core Team (2014). R: A language and environment for statistical computing. Vienna, Austria: R Foundation for Statistical Computing. Available at: http://www.R-project.org/.
Reddin C. J., Aberhan M., Raja N. B., Kocsis Á.T. (2022). Global warming generates predictable extinctions of warm-and cold-water marine benthic invertebrates via thermal habitat loss. Glob. Change Biol. 28, 5793–5807. doi: 10.1111/gcb.16333
Seidel L., Ketzer M., Broman E., Shahabi-Ghahfarokhi S., Rahmati-Abkenar M., Turner S., et al. (2022). Weakened resilience of benthic microbial communities in the face of climate change. ISME Commun. 2, 1–9. doi: 10.1038/s43705-022-00104-9
Snelgrove P. V. R., Soetaert K., Solan M., Thrush S., Wei C.-L., Danovaro R., et al. (2018). Global carbon cycling on a heterogeneous seafloor. Trends Ecol. Evol. 33, 96–105. doi: 10.1016/J.TREE.2017.11.004
Soetaert K., Herman P. M. J., Middelburg J. J., Heip C., Smith C. L., Tett P., et al. (2001). Numerical modelling of the shelf break ecosystem: reproducing benthic and pelagic measurements. Deep Sea Res. Part II Top. Stud. Oceanogr. 48 (14–15), 3141–3177. doi: 10.1016/S0967-0645(01)00035-2
Soetaert K., Van den Meersche K. (2009). Package limSolve, solving linear inverse models in r. sunsite.icm.edu.pl.
Sokolova I. M., Frederich M., Bagwe R., Lannig G., Sukhotin A. A. (2012). Energy homeostasis as an integrative tool for assessing limits of environmental stress tolerance in aquatic invertebrates. Mar. Environ. Res. 79, 1–15. doi: 10.1016/j.marenvres.2012.04.003
Steeves L. E., Filgueira R. R., Guyondet T., Chasse J., Comeau L., Chassé J., et al. (2018). Past, present, and future: performance of two bivalve species under changing environmental conditions. Front. Mar. Sci. 5. doi: 10.3389/FMARS.2018.00184
Sweetman A. K., Thurber A. R., Smith C. R., Levin L. A., Mora C., Wei C.-L., et al. (2017). Major impacts of climate change on deep-sea benthic ecosystems. Elem. Sci. Anthr. 5. doi: 10.1525/ELEMENTA.203
Tebble N. (1976). British Bivalve seashells. a handbook for identification (Edinburgh: British Museum (Natural History), Her Majesty’s Stationary Office).
Thomsen J., Haynert K., Wegner K. M., Melzner F. (2015). Impact of seawater carbonate chemistry on the calcification of marine bivalves. Biogeosciences 12, 4209–4220. doi: 10.5194/bg-12-4209-2015
Toussaint E., De Borger E., Braeckman U., De Backer A., Soetaert K., Vanaverbeke J. (2021). Faunal and environmental drivers of carbon and nitrogen cycling along a permeability gradient in shallow north Sea sediments. Sci. Total Environ. 767, 144994. doi: 10.1016/j.scitotenv.2021.144994
Tuomainen U., Candolin U. (2011). Behavioural responses to human-induced environmental change. Biol. Rev. 86, 640–657. doi: 10.1111/j.1469-185X.2010.00164.x
Turner L. M., Ricevuto E., Massa-Gallucci A., Gambi M. C., Calosi P. (2015). Energy metabolism and cellular homeostasis trade-offs provide the basis for a new type of sensitivity to ocean acidification in a marine polychaete at a high-CO2 vent: Adenylate and phosphagen energy pools versus carbonic anhydrase. J. Exp. Biol. 218, 2148–2151. doi: 10.1242/jeb.117705
Van Colen C., Ong E. Z., Briffa M., Wethey D. S., Abatih E., Moens T., et al. (2020). Clam feeding plasticity reduces herbivore vulnerability to ocean warming and acidification. Nat. Climate Change 10 (2), 162–166. doi: 10.1038/s41558-019-0679-2
Van Hoey G., Birchenough S. N. R., Hostens K. (2014). Estimating the biological value of soft-bottom sediments with sediment profile imaging and grab sampling. J. Sea Res. 86, 1–12. doi: 10.1016/J.SEARES.2013.10.010
Van Hoey G., Guilini K., Rabaut M., Vincx M., Degraer S. (2008). Ecological implications of the presence of the tube-building polychaete lanice conchilega on soft-bottom benthic ecosystems. Mar. Biol. 154, 1009–1019. doi: 10.1007/s00227-008-0992-1
Vlaminck E., Cepeda E., Moens T., Van Colen C. (2022a). Ocean acidification modifies behaviour of shelf seabed macrofauna: A laboratory study on two ecosystem engineers, abra alba and lanice conchilega. J. Exp. Mar. Bio. Ecol. 558, 151831. doi: 10.1016/j.jembe.2022.151831
Vlaminck E., Moens T., Vanaverbeke J., Van Colen C. (2022b). Physiological response to seawater pH of the bivalve abra alba, a benthic ecosystem engineer, is modulated by low pH. Mar. Environ. Res. 179, 105704. doi: 10.1016/j.marenvres.2022.105704
Voet H., Van Colen C., Vanaverbeke J. (2022). Climate change effects on the ecophysiology and ecological functioning of an offshore wind farm artificial hard substrate community. Sci. Total Environ. 810, 152194. doi: 10.1016/j.scitotenv.2021.152194
Volkenborn N., Meile C., Polerecky L., Pilditch C. A., Norkko A., Norkko J., et al. (2012). Intermittent bioirrigation and oxygen dynamics in permeable sediments: An experimental and modeling study of three tellinid bivalves. J. Mar. Res. 70, 794–823. doi: 10.1357/002224012806770955
Keywords: macrofauna, ocean acidification, ocean warming, biogeochemistry, ecosystem functioning
Citation: Vlaminck E, Moens T, Braeckman U and Van Colen C (2023) Ocean acidification and warming modify stimulatory benthos effects on sediment functioning: An experimental study on two ecosystem engineers. Front. Mar. Sci. 10:1101972. doi: 10.3389/fmars.2023.1101972
Received: 18 November 2022; Accepted: 06 February 2023;
Published: 20 February 2023.
Edited by:
Panagiotis D. Dimitriou, University of Crete, GreeceReviewed by:
Eva Chatzinikolaou, Hellenic Centre for Marine Research, GreeceUlisse Cardini, Anton Dohrn Zoological Station Naples, Italy
Copyright © 2023 Vlaminck, Moens, Braeckman and Van Colen. This is an open-access article distributed under the terms of the Creative Commons Attribution License (CC BY). The use, distribution or reproduction in other forums is permitted, provided the original author(s) and the copyright owner(s) are credited and that the original publication in this journal is cited, in accordance with accepted academic practice. No use, distribution or reproduction is permitted which does not comply with these terms.
*Correspondence: Carl Van Colen, carl.vancolen@ugent.be