Study on the inactivation and reactivation mechanism of pathogenic bacteria in aquaculture by UVC-LED
- 1College of Marine Science and Environment, Dalian Ocean University, Dalian, China
- 2Key Laboratory of Environment Controlled Aquaculture, Ministry of Education, Dalian Ocean University, Dalian, China
- 3College of Biosystems Engineering and Food Science, Zhejiang University, Hangzhou, China
Ultraviolet disinfection is an important method for controlling the large-scale outbreaks of diseases in aquaculture. As a novel and promising light source, ultraviolet light-emitting diode (UV-LED) has the advantages of safety, high efficiency and no environmental pollution risks. However, it remains unclear whether UV-LEDs can replace traditional UV light sources for aquaculture water treatment processes. Present study aimed to investigate the efficacy of UVC-LEDs (265 nm) on pathogenic bacteria, specifically Aeromonas salmonicida and Escherichia coli. The effects of UVC-LED dose, light conditions, and temperature on bacterial reactivation were also investigated. The results showed that exposure to UVC-LED effectively inactivated both types of bacteria. To achieve 4.5-log inactivation of A. salmonicida and E. coli, 24 mJ/cm2 and 28 mJ/cm2 UVC-LED irradiation were required, and the inactivation rate increased with increasing UVC-LED fluence. Both A. salmonicida and E. coli were revived after UVC-LED disinfection, and photoreactivation was significantly higher than dark reactivation. Bacterial reactivation rate due to high-dose UVC-LED treatment was significantly lower than that of low-dose. After 72 h of reactivation, photoreactivation and dark reactivation rates were 1 ± 0.4% and 2.2 ± 0.2%for A. salmonicida, and 0.02% and 0% for E. coli, respectively. Besides, the photoreactivation rates for the two bacteria exhibited different correlations with temperature. The highest photoreactivation rate for A. salmonicida was 68.7 ± 4% at 20°C, while the highest photoreactivation rate for E. coli was 53.98 ± 2.9% at 15°C for 48 h. This study reveals the rapid and efficient inactivation of bacteria by UVC-LED, and elucidates the mechanism and influencing factors for inactivation and reactivation by UVC-LED. The study also highlights that adequate UVC-LED irradiation and avoidance of visible light after UVC-LED disinfection can effectively inhibit bacterial reactivation. Our findings form a reference for the design and operation of UV disinfection in aquaculture.
1 Introduction
Due to the rapid development of aquaculture industries, the pressure on the treatment of aquaculture wastewater discharge has been increased. The direct discharge of aquaculture wastewater water containing a large amount of residual bait feces and bacterial viruses will lead to water environmental pollution, which enhances the necessity and urgency of aquaculture wastewater treatment. (Wang et al., 2022). Without effective treatment, excrements from culture organisms as well as residues of chemicals and feeds in aquaculture wastewater leads to increases in nitrogen, phosphorus, and other nutrients, bacterial proliferation, as well as disease outbreaks, which severely affects aquaculture development (Hu et al., 2019; Shu et al., 2022). In 2021, the pollution area of aquaculture reached 58.98 km2 in China, which caused the death of aquaculture animals (1.01×107 kg), economic losses of more than 20 million dollars and the proliferation of bacteria and viruses (China Fisher Statistical Yearbook, 2022). Therefore, there is a need to develop an effective and safe disinfection technology for removal of pathogenic microorganisms and pollutants in aquaculture.
In traditional wastewater treatment, chlorine, ozone and UV disinfection are the most commonly used methods for disinfection. Mechanistically, chlorine and ozone produce strong oxidizing substances to destroy cell membranes, remove pathogenic bacteria and decompose organic matter from wastewater (Deborde and Von Gunten, 2008; Lee and Von Gunten, 2016; Li et al., 2017b). As chemical disinfectants, addition of chlorine and ozone to water produces by-products and residues that threaten the safety of aquatic animals. Ultraviolet (UV) disinfection is associated with the absence of harmful by-products, high sterilization efficiencies, simplified operation and convenient management among others (Zheng et al., 2011). Reducing the use of chemical inputs in the breeding process meets the requirements for green aquaculture in China. Instead of chemical addition, UV sterilization is becoming common in water treatment and aquaculture (Guo et al., 2009b). The UVC wavelengths between 240-280 nm have the best disinfectant effects on bacteria, especially at 253.7 nm, since the maximum absorbance of DNA and protein for most microorganisms is around 260 nm and below 280 nm, respectively (Beck et al., 2017). The low-pressure (LP: 254 nm) and medium-pressure (MP: 240-580 nm) mercury lamps have been widely used in drinking water and sewage treatment plants (Oguma et al., 2004). UVC directly affects DNA by disrupting its transcription and replication processes, causing damage to the double-stranded DNA structure and forming cyclobutane pyrimidine dimers. Since they are mercury-free, have flexible wavelengths, compactness and low energy consumption, ultraviolet light-emitting diodes (UV-LED) are novel and reliable UV light sources to replace the traditional mercury lamps (Shen et al., 2020). It has been reported that UVC-LED has a great effect in the disinfection process of recirculation aquaculture systems (Moreno-Andrés et al., 2020). In the study of Nyhan et al., the inactivation efficiency of traditional light source and UVC-LED on Escherichia coli, Bacillus subtilis, Salmonella Typhimurium and Listeria monocytogenes were compared. It was found that the disinfection effectiveness of UVC-LED on bacteria is either equal to or superior to that of low pressure mercury lamps (Nyhan et al., 2021). At 275 nm, UVC-LED has a better inactivation efficiency on E. coli, than 253.7 nm LP-UV, indicating that UVC-LED has better disinfection effects (Green et al., 2018). Nevertheless, there is no fundamental distinction between traditional mercury lamps and UVC-LEDs, as traditional mercury lamps also emit the UV wavelength which is absorbed by DNA. It has also been reported that UVC-LEDs at 265 nm have higher antibacterial efficacies than LP-UV, but are limited by the higher costs of disinfection temporarily. (Chatterley and Linden, 2010). The majority of UVC disinfection devices are designed as pipeline systems, which allows optical path of UVC can be controlled and ensure the efficiency of disinfection. Moreover, physical filtration often set prior to UVC treatment to remove suspended solids and particulate matter in the process of wastewater disinfection, which helps to enhance the effectiveness of subsequent UVC treatment.
After UV irradiation, some bacteria can repair their damaged DNA via photoreactivation and dark repair. It is necessary to focus on the variations of reactivation and influencing factors of bacteria after UV irradiation. Dark repair is a multistep process, where damaged or abnormal DNA bases are removed by base excision repair (BER) and nucleotide excision repair (NER) subpathways (Nyangaresi et al., 2018). Photoreactivation is a highly specific process for damaged DNA repair. Photolyase absorbs energy from visible light and participates in bacterial reactivation (Ramírez et al., 2021). Besides, photoreactivation is affected by repair time and UV radiation dose among others. It was found that the amount of E. coli increased as the reactivation duration extended, and the photoreactivation was positively correlated with the duration of recovery, since light provides energy for photolytic enzymes involved in repairing DNA damage (Locas et al., 2008). Since photoreactivation is a photochemical reaction, its rate is greatly affected by temperature. Photoreactivation rate has been shown to increase with increasing temperature (Kelner, 1949). However, in another study, after UV irradiation, the photoreactivation rate of E. coli rapidly decreased with increasing temperature and the highest photoreactivation rate appeared at 10°C. Current studies on temperature are focused on E. coli, with studies on aquatic pathogens being few. Therefore, there is a need to elucidate on bacterial reactivation in aquaculture wastewater after ultraviolet disinfection.
We investigated the feasibility of UVC-LED as novel light sources for bacterial disinfection in aquaculture, and focused on the reactivated effects and influencing factors of bacterial after UV irradiation. Two common gram-negative bacteria (E. coli and A. salmonicida) in aquaculture were inactivated by UVC-LED at an emission wavelength of 265 nm. The effects of UVC-LED dose, light conditions and temperature on bacterial reactivation were further investigated, the mechanisms and influencing factors of inactivation and reactivation supported the application of UVC-LED and operation of UV disinfection processes in aquaculture.
2 Materials and methods
2.1 Microbial cultivation
To clarify the potential application of UVC-LED disinfection in aquaculture, Aeromonas salmonicida (A. salmonicida) and Escherichia coli ATCC 8099 (E. coli) were selected as the representatives of common pathogens in aquaculture. A. salmonicida is a prevalent pathogenic bacterium that can cause significant economic losses in fish diseases (Coscelli et al., 2015), whereas Escherichia coli is often utilized as a model bacterium for the safety evaluation (Okeke et al., 2011). The strain A. salmonicida was granted by Professor Feng (Institute of Microbiology, Chinese Academy of Sciences) which was inoculated in Luria-Bertani (LB) medium and incubated in the shaker incubator at 28°C, 120 r/min for 48 h. E. coli was purchased from Guangdong Huankai Microbial Technology Company, the strain was inoculated in Luria-Bertani (LB) medium and incubated in a shaker incubator at 37°C for 24 h. The cells were collected by centrifugation (6000 rpm, 5 min and 4°C) and suspended in sterile phosphate buffered saline (PBS, 0.1 M) to yield a concentration of approximately 107-108 CFU/mL. Enumeration was performed by the spread plate method, 50 μL serially diluted samples were spread on agar plates in triplicate and incubated at the corresponding culture temperature. The colonies on the plates between10-300 were counted and calculated as CFU/mL.
2.2 UVC-LEDs and experimental setup
UVC-LED devices (peak wavelength at 265 nm, Shenzhen Fluence Technology PLC) were applied as the light source in present study. Twenty-five UVC-LED lamps were connected and fixed on a circular board (40 mm diameter, 12.6 cm2 area). A metal radiating fin was installed above the circular board to protect the UVC-LED from overheating and magnetic stirrer was used to mix the solution during the experiment. The average UVC-LED irradiance was 0.20 mW/cm2 during all experiments. Light intensity was adjusted by changing the current of the DC power supply and measured by digital handheld optical power and energy meter console (PM100D, THORLABS) with a probe (S120VC). The irradiation device (a) and the UVC-LED spectrogram (b) were illustrated in Figure 1
2.3 Irradiation experiment
Taken 20 mL of the above diluted bacterial solution was placed in the petri dish (60 mm diameter, 15 mm height) and irradiated at 20 mm from UVC-LED. Before irradiation, the UVC-LED modules were preheated and reached the stable emission stage. A cylindrical device was used to cover the outside of the ultraviolet device to ensure that the UV beam remains parallel. During the whole experimental process, the shading curtain was used to avoid the interference of other surrounding light. The disinfection effects on A. salmonicida and E. coli under the treatment of different UVC-LED dosages were compared by changing the exposure duration. The UVC-LED treatment duration were set as 0, 20, 40, 60, 80, 100, 120, 140, 160 and 180 s. The UVC-LED treatment dosages were set as 0, 4, 8, 12, 16, 20, 24, 28, 32 and 36 mJ/cm2, respectively. After UVC-LED treatment, 1 mL sample was taken immediately and diluted to a series of gradients for the enumeration. Agar plating method is a commonly used technique for bacterial counting, and it is also widely employed in the evaluation of disinfection efficacy. The inactivation efficiency of bacteria was analyzed by calculating log inactivation using Eq. (1) (Lu et al., 2021).
in which, N0 and N were the colony counts (CFU/mL) before and immediately after disinfection, k was the UV inactivation rate constant (cm2/mJ), and D was the dosage of UV treatment received by the bacteria (mJ/cm2).
2.4 Photoreactivation and dark reactivation
According to the above experiment results, the inactivation of 3-log and 5-log of A. salmonicida and E. coli were selected for photoreactivation and dark repair experiments. Thus, UVC-LED irradiation at 12 mJ/cm2, 24 mJ/cm2 for A. salmonicida, and 20 mJ/cm2, 36 mJ/cm2 for E. coli were used. As described above, 30 mL of microbial suspension was prepared before UVC-LED irradiation, half of the microbial suspension was taken as the control group and the rest was placed in a petri dish for UVC-LED irradiation. Take 5 mL irradiated samples into sterile centrifuge tubes and transferred them to a light incubator (Temperature: 25 ± 0.5°C; Light intensity: 93.8 μmol/m2/s) for photoreactivation and dark reactivation. Dark reactivation samples were taken into tubes covered with aluminum foil to avoid light exposure. Samples were taken at 0, 12, 24, 48 and 72 h for enumeration, respectively. Agar plating methods were used to enumerate these microorganisms in suspension. The rate of photoreactivation and dark repair was quantified using Eq. (2) (Lindenauer and Darby, 1994).
in which, Nt is the concentration of microorganisms after photoreactivation/dark repair for a period of time, t (h) (CFU/mL). N0 and N were the colony counts (CFU/mL) before and immediately after disinfection.
To indicate the regrowth potential of bacteria after irradiation and reactivation, the growth ratio was expressed as Eq. (3) (Kashimada et al., 1996).
in which, N0 is the colony count (CFU/mL) before UVC-LED irradiation, NT is the concentration of microorganisms after photoreactivation/dark repair for 72 h.
2.5 Impact of temperature on bacterial photoreactivation
To investigate the effects of different temperatures on the photoreactivation of the irradiated bacteria, 15°C, 20°C, 25°C were set up to simulate actual culture temperature in aquaculture (Wan et al., 2022). Using water bath devices to control the stability of temperature and monitor temperature variations in real-time. The temperature control devices were preheated at least 20 min before the experiment to remain the water temperature stable. A. salmonicida and E. coli were irradiated by UVC-LED at 12 mJ/cm2 and 20 mJ/cm2, respectively. Then the irradiated samples were immediately floated in water bath devices and made sure the bacterial suspension was in the centrifuge tube below the water surface. Sampling and counting are the same as described above. The rate of photoreactivation at different temperatures was quantified using Eq. (2).
2.6 Statistical analysis
All experiments were conducted in triplicates, and the results were expressed as mean ± standard deviation. One-way ANOVA was performed with SPSS 21.0 software, and the results were considered statistically significant when p< 0.05. Pearson correlation analysis was performed to display the correlations between UV fluence, repair mode, repair time, temperature and reactivation rate. Significant correlations were considered when p< 0.05, and highly significant correlations were considered when p< 0.01.
3 Results
3.1 UVC-LED inactivation of A. salmonicida and E. coli
Inactivation of A. salmonicida and E. coli at different UVC-LED irradiation fluences are shown in Figure 2. At 12 mJ/cm2, UVC-LED irradiation yielded a 2.83-log inactivation of A. salmonicida, while completely inactivation (4-log reduction) was achieved at 24 mJ/cm2 with the bacteria entering the non-culturable state. Inactivation of A. salmonicida stopped increasing as the UVC-LED irradiation fluence increased. For E. coli, 20 mJ/cm2 was able to yield a 3-log inactivation, while 28 mJ/cm2 UVC-LED was required to obtain a 4.4-log inactivation. A 36 mJ/cm2 treatment was able to yield about 5-log inactivation of E. coli, but they were still culturable. Even though the inactivation curves of the two bacteria were comparable, the inactivation rates slowed down when UVC-LED irradiation exceeded a certain fluence. The inactivation rate constant for A. salmonicida increased (kd=0.24) when the UVC-LED irradiation fluence was less than 16 mJ/cm2, and the inactivation rate (kd=0.04) decreased significantly when the fluence of UVC-LED treatment was higher than 16 mJ/cm2. When UVC-LED fluence exceeded 24 mJ/cm2, A. salmonicida was no longer culturable (kd= 0). In contrast, a shoulder effect was observed in E. coli inactivation when UVC-LED irradiation was less than 8 mJ/cm2 (kd=0.05). The inactivation rate for E. coli (kd=0.23) improved with increasing UVC-LED irradiation fluence at the range of 8-24 mJ/cm2, while it decreased (kd=0.08) when UVC-LED irradiation was above 24 mJ/cm2. These results indicate that a tailing stage might exist in bacterial inactivation when UVC-LED treatment exceeds a certain fluence, which may be attributed to self-aggregation caused by UV irradiation, leading to changes in bacterial surface properties (Kollu and Ormeci, 2015). Similar phenomena were also found in inactivation of isolated Bacillus subtilis spores by a low-pressure mercury lamp (253.7 nm) (Mamane-Gravetz and Linden, 2005).
3.2 Impact of UVC-LED fluences on photoreactivation
After UVC-LED inactivation, microorganisms can be repaired via different reactivation mechanisms, and the photoreactivation was significantly correlated with UVC-LED fluences. To assess the differences between sublethal and complete inactivation of bacteria, the inactivation of 3-log and 5-log of A. salmonicida and E. coli were selected for photoreactivation and dark repair. Based on this, UVC-LED at 12 mJ/cm2 and 24 mJ/cm2 or 20 mJ/cm2 and 36 mJ/cm2 were used for A. salmonicida and E. coli inactivation. Both irradiated A. salmonicida and irradiated E. coli exhibited obvious photoreactivations. The reactivation rate was positive correlated with reactivation duration and significantly negative correlated with UVC-LED irradiation fluence (p< 0.05) both for A. salmonicida and E. coli. The higher the applied fluence of UVC-LED, the less the photoreactivation rate (Figure 3). Generally, after 12 mJ/cm2 or 20 mJ/cm2 UVC-LED irradiation, photoreactivation rates for both A. salmonicida and E. coli increased first, and then decreased with increasing reactivation time, but they were difficult to be revived after high fluence UVC-LED irradiation (24 mJ/cm2 or 36 mJ/cm2). After 12 mJ/cm2 and 24 mJ/cm2 UVC-LED irradiation, the A. salmonicida concentrations were 3×104 CFU/mL and 4.83×103 CFU/mL, respectively. The reactivation rates and bacterial concentrations were maximum after 48 h of reactivation (Figure 3A). Therefore, UVC-LED irradiation reduced the culturability of A. salmonicida. Compared to A. salmonicida, the photoreactivation rate for irradiated E. coli was relatively low, and after UVC-LED irradiations of 20 mJ/cm2 and 36 mJ/cm2, the concentrations of E. coli were 1.29×106 CFU/mL and 1.06×107 CFU/mL, respectively. After photoreactivation for 72 h in 36 mJ/cm2 UVC-LED irradiation group, the highest photoreactivation rate and bacterial concentration for E. coli were 0.02% and 1.44×105 CFU/mL, respectively (Figure 3B).
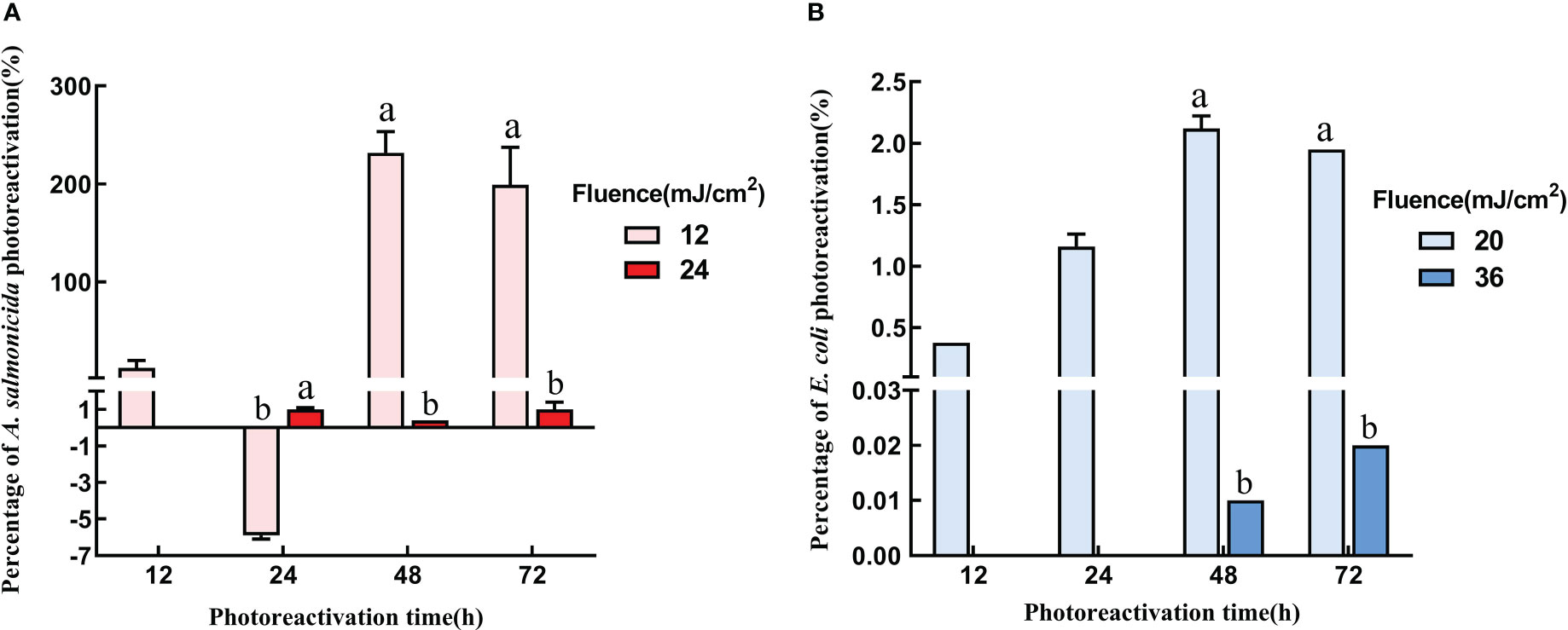
Figure 3 Photoreactivation with different UVC fluence (A) A salmonicida; (B) E coli). Note: Different lowercase letters in the figure indicate that bacterial reactivation is significantly different under different conditions. (p< 0.05).
3.3 Impact of UVC-LED fluences on dark repair
Apart from photoreactivation, microorganisms can also be repaired in dark conditions. In Figure 4, dark reactivations of A. salmonicida and E. coli differed from photoreactivation. The dark reactivation rate of A. salmonicida was extremely low, and the bacterial concentration continued to decrease at the early stage of dark reactivation. Only 0.8 ± 0.63% and 2.2 ± 0.24% of A. salmonicida had reactivated after revival for 72 h in 12 mJ/cm2 and 24 mJ/cm2 UVC-LED irradiated group, respectively. Therefore, higher fluences of UVC-LED irradiation and longer reactivation times improved the dark repair capacity of A. salmonicida. The dark reactivation rate at 72 h was higher than that at 48 h, and extended dark might contribute to A. salmonicida reactivation (Figure 4A). Compared to A. salmonicida, E. coli exhibited a different consistency with regards to dark repair and photoactivation of E. coli. The dark reactivation rate of E. coli increased with increasing reactivation time, and was delayed with a high dose of UVC-LED irradiation. After 20 mJ/cm2 UVC-LED irradiation, there were 1.99×106 CFU/mL of E. coli in dark reactivation for 48 h and the dark repair rate reached a maximum of 0.13 ± 0.01%. There was no dark reactivation of E. coli after 36 mJ/cm2 UVC-LED irradiation (Figure 4B). The dark reactivation rate of bacterial reactivation in the water sample reduced with increasing UVC-LED fluence, consistent with findings from other studies (Nebot Sanz et al., 2007). Thus, a higher fluence of UVC-LED irradiation might cause serious bacterial damage, leading to a loss of reactivation capacity.
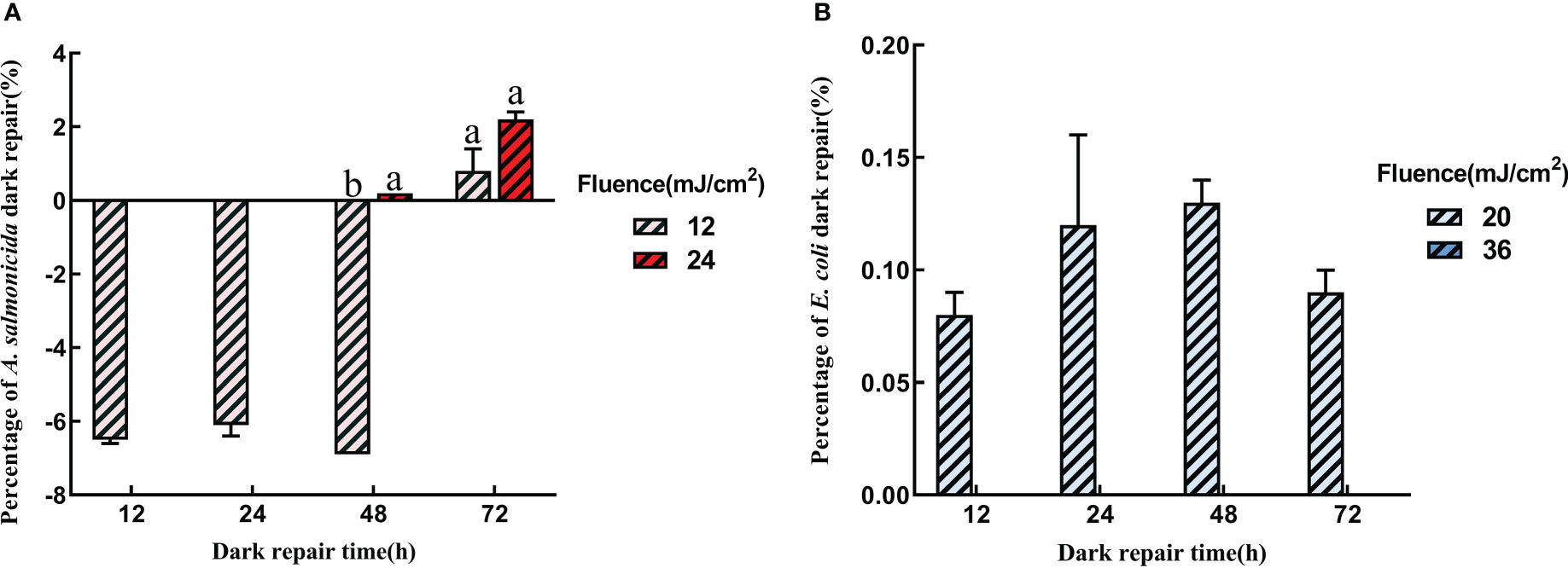
Figure 4 Dark repair with different UVC fluence (A) A salmonicida; (B) E coli). Different lowercase letters in the figure indicate that bacterial reactivation is significantly different under different conditions. (p< 0.05).
Both strains showed better growth rates in light and dark conditions without UVC-LED irradiation. The growth rate of unirradiated E. coli under light conditions was 154.55 ± 6.94% while that of A. salmonicida under dark conditions was 2318.8 ± 227.8%, respectively (Figure 5). We postulated that reactivated light might inhibit bacterial growth when the cellular structure of A. salmonicida had not been destroyed by UV irradiation. The fluence of UVC-LED irradiation significantly affected A. salmonicida growth, which was enhanced by 12 mJ/cm2 UVC-LED irradiation (achieved a growth rate of 192.8 ± 35.9%) while it was inhibited after 24 mJ/cm2 UVC-LED irradiation (a growth rate of only 1 ± 0.4%). However, under both conditions of photoreactivation and dark reactivation, the growth rates of E. coli after UVC-LED irradiation were very low. Differences in outcomes between the two strains indicate differences in reactivation mechanisms of E. coli and A. salmonicida.
3.4 Effects of temperature on photoreactivation
From the above findings, photoreactivation was the main pathway for reactivation for A. salmonicida and E. coli after UVC-LED irradiation. Photoreactivation is a photochemical reaction that maybe be affected by reactivation temperatures. Temperature gradients were established considering the water temperature variations in different seasons during mariculture, as well as the temperature tolerance of the cultured organisms, to clarify whether different culture temperatures will have an impact on bacterial inactivation. The common aquaculture temperatures (15°C, 20°C, 25°C) were selected to investigate the effects of temperature on bacterial photoreactivation.
Photoreactivation of A. salmonicida exhibited the same trend under different reactivation durations and temperatures (Figure 6A). The photoreactivation rate of A. salmonicida increased with increasing temperature and prolongation of resurrection duration. The highest photoreactivation rates of 9.7 ± 2.5%, 68.7 ± 4% and 67.1 ± 10% at 15°C, 20°C, 25°C were achieved when UVC-LED irradiated A. salmonicida had been cultured for 72 h. The photoreactivation capacity of A. salmonicida at 15°C was significantly lower than those at 20°C and 25°C (p< 0.05), and after 72 h of photoreactivation, the bacterial concentrations were 2.02×107 CFU/mL, 1.42×108 CFU/mL and 1.39×108 CFU/mL, respectively. Therefore, higher temperatures were better for A. salmonicida photoreactivation, and the photoreactivation rate increased with time. On the contrary, the photoreactivation rate for E. coli decreased with increasing temperature, and the order of temperature effects on E. coli photoreactivation was 15°C >20°C >25°C. Under the three temperature conditions, E. coli reactivation reached the maximum value after culture for 48 h, which were 9.13×107 CFU/mL, 6.60×107 CFU/mL and 7.73×106 CFU/mL, respectively (Figure 6B). Therefore, the variation in temperature and repair rate between the two bacteria highlights the importance of considering the impact of temperature during UVC-LED disinfection
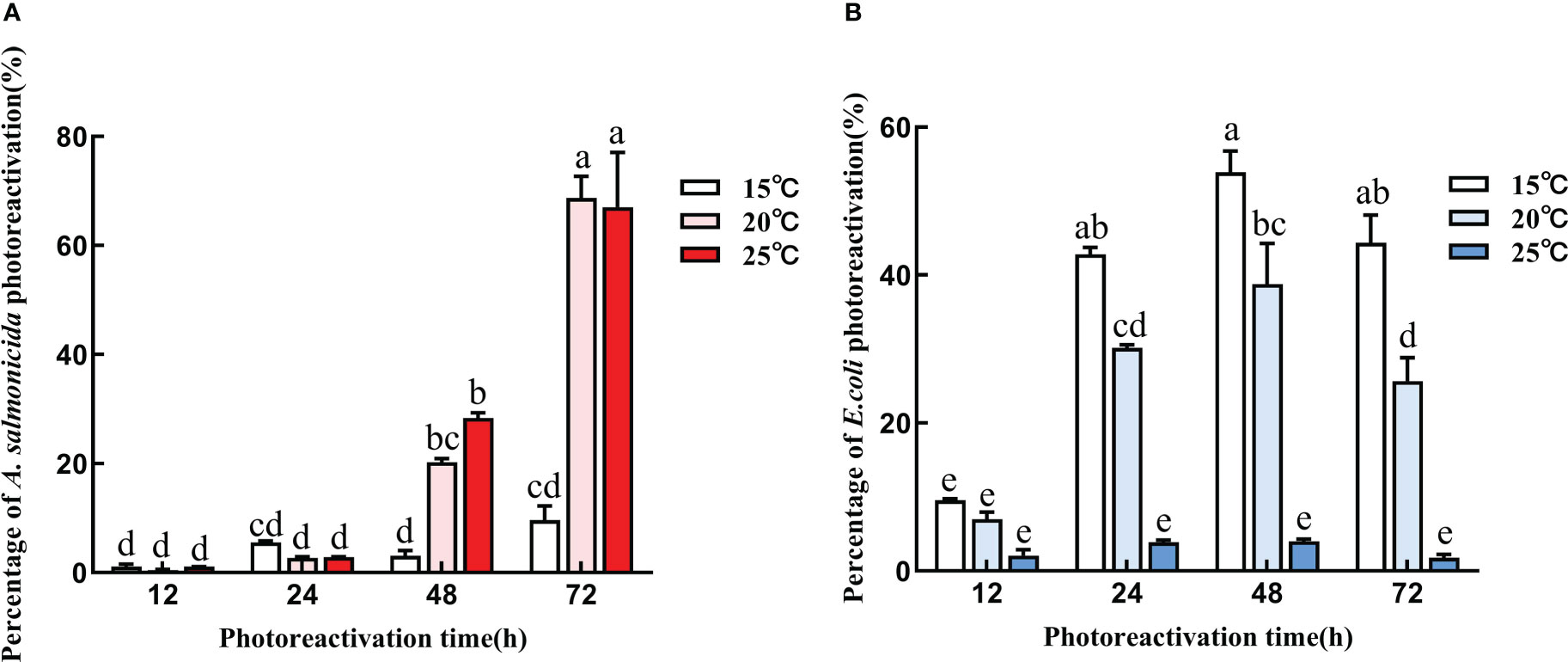
Figure 6 Photoreactivation with different temperature conditions (A) A salmonicida; (B) E coli). Note: Different lowercase letters in the figure indicate that bacterial reactivation is significantly different under different conditions. (p< 0.05).
3.5 Correlations
To reveal the critical factors affecting bacterial inactivation and reactivation, correlation analyses of UVC-LED fluence, repair mode, repair time, temperature and reactivation rate were performed. Reactivation rates of the two bacteria were negatively correlated with UVC-LED fluence, and positively correlated with repair time (Figure 7). Inactivations of A. salmonicida and E. coli gradually increased with increasing UVC-LED fluence, and both photoreactivation as well as dark repair rates significantly increased with increasing repair time. Temperature was negatively correlated with repair methods, therefore, more attention should be paid to temperature and light after UVC-LED treatment in aquaculture. The repair rate of A. salmonicida was significantly correlated with repair methods and temperature (Figure 7A). The photoreactivation rate of A. salmonicida increased with increasing temperature, and the reactivation rate at 25°C was significantly higher than that at 15°C. The repair rate of E. coli showed opposite correlations with repair methods and temperature. Under dark repair, E. coli was easier to repair, compared with A. salmonicida (Figure 7B). Therefore, reactivation varied with bacterial species and various reactivated conditions.
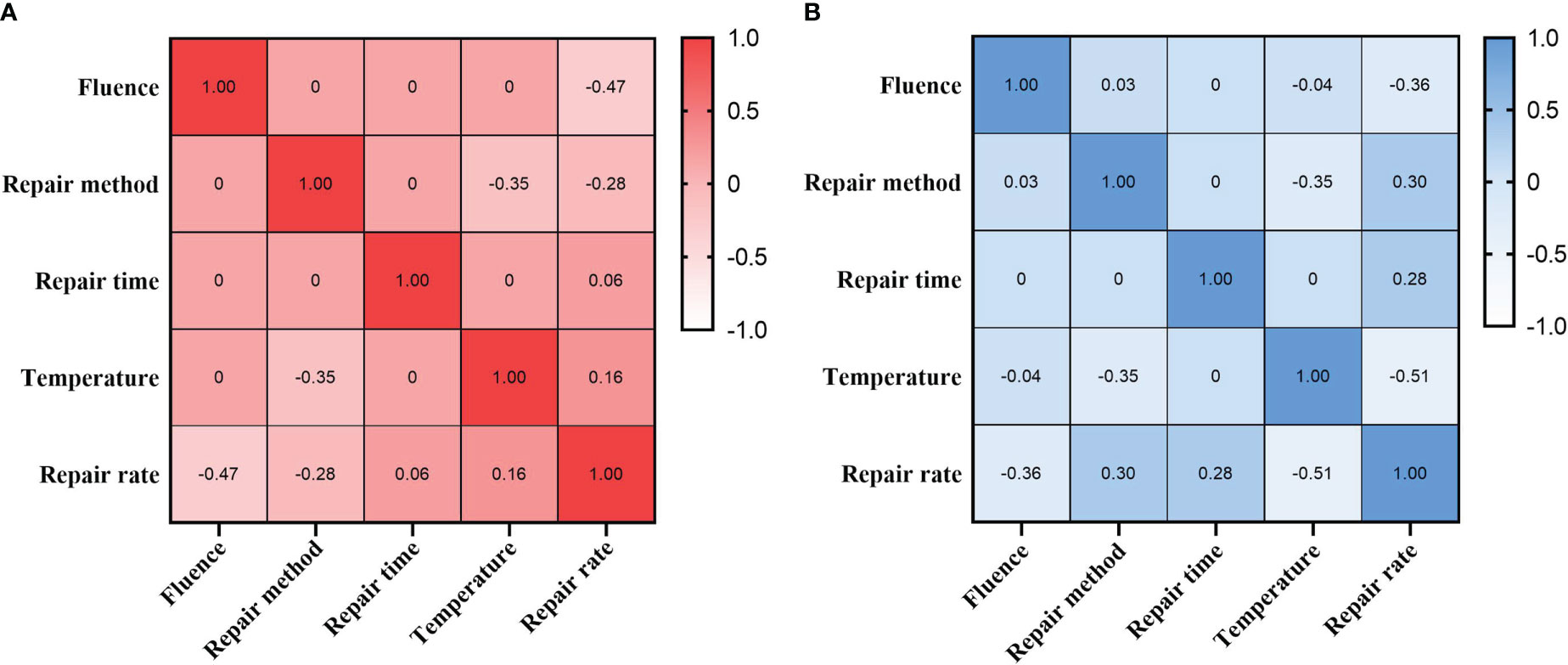
Figure 7 Correlations between UV fluence, repair method, repair time, temperature and repair rate (A) A salmonicida; (B) E coli).
4 Discussion
4.1 Factors affecting bacterial inactivation
Ultraviolet, which can directly act on DNA, has significant inactivation effects on bacteria. In this study, similar disinfection trends for the two bacteria were observed after UVC-LED irradiation, and the inactivation rate increased with increasing UVC-LED fluence. Compared with A. salmonicida, the inactivation curve of E. coli had a shoulder effect and a tailing stage. Deficient E. coli inactivation was achieved at low UVC irradiations, consistent with a previous study (Hijnen et al., 2006). Bowker et al. also found that when the fluence of 255 nm UVC-LED less than 6.3 mJ/cm2, the inactivation of E.coli was less than 1 log (Bowker et al., 2011; Masjoudi et al., 2021). The shoulder effects that occurred in light-induced inactivation can be explained by biological processes rather than photochemical reactions in cells that are attributed to photoreactivation and dark reactivation (Serna-Galvis et al., 2019; Song et al., 2019). The tailing stage refers to self-aggregation of bacteria when UVC irradiation reachs a certain dose (Kollu and Ormeci, 2015). The UVC-LED wavelength also played a vital role in sterilization (Jeong and Ha, 2019). UVC-LED at 254 nm resulted in 5-log inactivation of E. coli at about 27 mJ/cm2 (Zhou et al., 2017), and inactivation of E. coli reached 6-log at 0.7 mJ/cm2 by 266 nm UVC-LED (Kim et al., 2016), inactivation of E. coli reached 5-log at about 18 mJ/cm2 by 280 nm UVC-LED (Li et al., 2017a). Therefore, 265 nm UV wavelength has the strongest sterilization efficiency. Even under the same wavelength of UV radiation, there were significant differences in inactivation efficiencies. In the study by Song et al. (2019), 5-log inactivation of E. coli required 7 mJ/cm2 irradiation by 265 nm UVC-LED, while 36 mJ/cm2 was required to achieve the same effect in this study, which may be associated with initial bacterial concentration. In this study, the initial concentration of E. coli was 1.69×108 CFU/mL, while the initial concentration in the study by Song et al. was 106 CFU/mL (2019). These results imply that the number of bacteria directly affects the irradiation of UVC absorbed by bacteria, which leads to different sterilization effects. At 265 nm, UVC-LED exerted excellent inactivation effects on the two bacteria. Due to the lack of a thick peptidoglycan layer, the gram-negative bacteria (A. salmonicida and E. coli) were highly sensitive to UV. The gram-negative bacteria has been shown to be more sensitive to UVC irradiation than gram-positive bacteria, which has a thicker peptidoglycan layer (Rohde, 2019). In the study, 4.6 mJ/cm2 was required for E. faecalis to reach 5-log inactivation under irradiation of 222 nm KrCl excimer lamp while only 2.4 mJ/cm2 was required for E. coli (Tsenter et al., 2022). Therefore, there are wide variations in UVC resistance of different bacteria (Huang et al., 2016). Under the same UVC-LED irradiation conditions, the inactivation rate of A. salmonicida was higher than that of E. coli. A. salmonicida showed a high sensitivity and poor tolerance to UVC-LED. In standard protocols, only 2.7 mJ/cm2 was required for A. salmonicida to reach 2-log inactivation under irradiation of 254 nm LP lamp while 6.4 mJ/cm2 and 5.6 mJ/cm2 was required for E. coli by 275 nm and 265 nm UV-LED (Masjoudi et al., 2021). Differences in UVC sterilization effects were associated with UVC wavelength, microorganisms, initial bacterial concentration and other conditions. UVC radiation directly damaged to bacterial DNA. However, the commonly used evaluation method, gel electrophoresis, which can only detect the extent of double-strand breaks in bacterial DNA, and it cannot be well detected other types of DNA damage such as single-strand break. UVC irradiation is usually used in the disinfection process of source water, wastewater or recirculating water in aquaculture systems, while reactive oxygen species residues may be present in the water and could potentially cause oxidative damage to aquatic animals (Lushchak, 2011). Especially, if UVC irradiation is used for breeding disinfection, it is necessary to evaluate its safety.
4.2 Comparisons of photoreactivation and dark repair
When bacteria are not completely inactivated by UVC irradiation, some of them remain in a sublethal state of “viable-but-nonculturable”, which may be restored to the culturable state under certain conditions (Arvaniti et al., 2021). Although the inactivation rate of low-dose UVC treatment on bacteria reached 99% and their growth activities were significantly inhibited, they were still in a culturable state. Therefore, in this study, photoreactivation rates of A. salmonicida and E. coli at low-dose UVC-LED treatment were significantly higher than those at high-dose UVC treatments. In a previous study, the photoreactivation rates of E. coli were 28.73% after 5 mJ/cm2 UVC irradiation and 0.042% after 20 mJ/cm2 UVC irradiation using 254 nm low-pressure mercury lamp (Guo et al., 2009a). Besides, high doses of UVC irradiation may aggravate the DNA damage of bacteria and require a longer time to repair, thereby reducing the bacterial photoreactivation rate (Guo et al., 2011; Shafaei et al., 2017). Photoreactivation delay was observed in the high-dose UVC-LED treated group, the highest photoreactivation rate of A. salmonicida appeared at 48 h after 12 mJ/cm2 UVC-LED irradiation, and appeared at 72 h after 24 mJ/cm2 UVC-LED irradiation. Similarly, the highest photoreactivation rates of E. coli treated with 20 mJ/cm2 and 36 mJ/cm2 UVC-LED irradiation were established at 48 h and 72 h, respectively. The photoreactivation rate was also associated with light intensity. Increasing the reactivation intensity improved the photoreactivation rate of E. coli to a certain extent. The recovered E. coli concentration under light intensity of 2920 lux was higher than that of 296 lux (Lamont et al., 2004).
Compared with photoreactivation, the efficiency of dark reactivation of both bacteria was significantly lower, suggesting that light can provide energy for A. salmonicida and E. coli reactivations. Photolyase can convert this energy into chemical energy and generate free radicals to act on cyclobutane pyrimidine dimers (CPDs), promoting bacterial reactivation (Cadet and Davies, 2017). Moreover, photoreactivation, which is light-dependent, requires less energy and is more efficient. Photoreactivation of E. coli was significantly higher than that of dark repair, and increased with prolongation of time (Chen et al., 1994). After UVC exposure of 5 mJ/cm2, photoreactivation rate of E. coli reached 10.5%, while the dark repair rate was only 1.98% after reactivation for 24 h (Xu et al., 2015). Li et al. (Li et al., 2017a) also confirmed the lower reactivation rate of E. coli under dark conditions. Therefore, a sufficient high dose of UVC irradiation should be used to inhibit bacterial reactivation during aquaculture wastewater treatment. Moreover, bacterial reactivation can be inhibited to a certain extent by reducing the time and intensity of visible light exposure after sterilization.
4.3 Effects of temperature on photoreactivation
The activity of bacteria may be inhibited by various factors, including temperature, which may directly affect the rate of the intracellular enzymatic reaction or affects the mobility of the cell membrane (Wan et al., 2022). Considering the practical applications of UVC inactivation in aquaculture, it is important to investigate bacterial photoreactivations at different temperatures. In this study, the two gram-negative bacteria exhibited different reactivation effects under the same temperature and reactivation durations. The optimal culture temperatures for A. salmonicida and E. coli were 28°C and 37°C, respectively. The photoreactivation rates for bacteria were not consistent with optimal culture temperatures, indicating that bacterial photoactivation did not depend on their optimal culture temperatures. In a previous study, differences in photoreactivation kinetics of E. coli cultured at 15-30°C were insignificant while photoreactivation efficiencies of bacteria significantly decreased when the temperature exceeded 37°C (Xu et al., 2015). Besides the significant reduction in photoactivation efficiencies of E. coli, the intracellular photolysis protein levels also decreased when E. coli was cultured in this temperature range. Photolyase of E. coli may be a cryogenic enzyme that exerts better photoreactivation abilities at lower temperatures. Differences in structure, metabolism, and photolyase numbers result in differences in bacterial photoreactivation responses (Quek and Hu, 2008; Cadet and Davies, 2017). Temperature directly affects the rate of the intracellular enzymatic reaction, which leads to differences in the growth and metabolic behavior of bacteria under different temperature conditions. It influences the contents of unsaturated fatty acids in microbial cells, thus affecting the mobility of the cell membrane, the absorption of nutrients and the secretion of metabolites (Wan et al., 2022). Maximum photoreactivations of A. salmonicida and E. coli appeared at 72 h and 48 h, which might be associated with bacterial reproduction rates. A. salmonicida grows slowly and should be cultured at 28°C for 48 h, while E. coli is cultured at 37°C for 24 h. Studies on reactivation of A. salmonicida are very few and photoactivation as well as its influencing factors should be investigated further. In summary, elucidation of inactivation and reactivation of A. salmonicida by UVC-LED will inform on application potential of UVC-LED in aquaculture.
5 Conclusions
UVC-LED effectively disinfects the common pathogenic bacteria (A. salmonicida and E. coli) in aquaculture. However, there were variations in bacterial resistance to UVC-LED, and A. salmonicida being more sensitive to UVC-LED than E. coli. Compared to dark repair, photoreactivation was the best reactivation mechanism for irradiated bacteria, as light provides energy to promote the reactivation of bacteria. With the increase of UVC-LED irradiation dose, it may increase the damage degree of bacteria and reduce the regeneration potential, leading to delayed reactivation. The intensity of reactivation light also affected bacterial reactivation. In addition, temperature affected the bacterial photoreactivation rate. Photoreactivation rates of A. salmonicida and E. coli exhibited different variation trends with temperature elevations, and their optimal reactivation temperatures were 25°C and 15°C, respectively. Therefore, photoreactivation mechanisms of the two bacteria differed. In present study, we did not focus on the sterilization in the breeding process. When UVC irradiation applied during breeding, more attention needs to be given to the potential harm on the cultured organisms. This study shows the feasibility of UVC-LED to effectively inactivate bacteria in aquaculture wastewater and elucidates on the reactivation mechanisms of A. salmonicida and E. coli after inactivation by UVC-LED. Our findings provide a reference point for optimal design and operations of ultraviolet sterilization devices in aquaculture. The results of present study are important reference for large-scale aquaculture wastewater treatment and provide insights into the treatment of aquaculture source water.
Data availability statement
The raw data supporting the conclusions of this article will be made available by the authors, without undue reservation.
Author contributions
WZ conducted the sample collection, data curation and draft writing. RH performed the molecular work. TZ, BW, NL and YS performed the data visualization. HM and JZ contributed to the concept and design of the study. YL and QZ conducted the writing-review and editing. All authors contributed to the article and approved the submitted version.
Funding
This study was funded by Modern Agro-industry Technology Research System (CARS-49), Innovation Support Program for High-level Talents of Dalian City (2019RD12), Basic scientific research project of Education Department of Liaoning province (LJKMZ20221107), The Overseas Training Program for Innovation Team of Liaoning Province (201818), Innovation and Entrepreneurship Project for High-Level Talents of Dalian (2020RQ109).
Acknowledgments
We would like to thank Foshan Electrical and Lighting Co., Ltd and Shenzhen Fluence Technology PLC for their assistance with light source design in our experiments.
Conflict of interest
The authors declare that the research was conducted in the absence of any commercial or financial relationships that could be construed as a potential conflict of interest.
Publisher’s note
All claims expressed in this article are solely those of the authors and do not necessarily represent those of their affiliated organizations, or those of the publisher, the editors and the reviewers. Any product that may be evaluated in this article, or claim that may be made by its manufacturer, is not guaranteed or endorsed by the publisher.
References
Arvaniti M., Tsakanikas P., Papadopoulou V., Giannakopoulou A., Skandamis P. (2021). Listeria monocytogenes sublethal injury and viable-but-Nonculturable state induced by acidic conditions and disinfectants. Microbiol. Spectrum. 9 (3), e01377–e01321. doi: 10.1128/Spectrum.01377-21
Beck S. E., Ryu H., Boczek L. A., Cashdollar J. L., Jeanis K. M., Rosenblum J. S., et al. (2017). Evaluating UV-c LED disinfection performance and investigating potential dual-wavelength synergy. Water Res. 109, 207–216. doi: 10.1016/j.watres.2016.11.024
Bowker C., Sain A., Shatalov M., Ducoste J. (2011). Microbial UV fluence-response assessment using a novel UV-LED collimated beam system. Water Res. 45 (5), 2011–2019. doi: 10.1016/j.watres.2010.12.005
Cadet J., Davies K. J. A. (2017). Oxidative DNA damage & repair: an introduction. Free Radical Biol. Med. 107, 2–12. doi: 10.1016/j.freeradbiomed.2017.03.030
Chatterley C., Linden K. (2010). Demonstration and evaluation of germicidal UV-LEDs for point-of-use water disinfection. J. Water Health 8 (3), 479–486. doi: 10.2166/wh.2010.124
Chen J., Mitchell D. L., Britt A. B. (1994). A light-dependent pathway for the elimination of UV-induced pyrimidine (6-4) pyrimidinone photoproducts in arabidopsis. Plant Cell. 6 (9), 1311–1317. doi: 10.1105/tpc.6.9.1311
Coscelli G. A., Bermúdez R., Losada A. P., Santos Y., Quiroga M. I. (2015). Vaccination against Aeromonas salmonicida in turbot (Scophthalmus maximus l.): study of the efficacy, morphological changes and antigen distribution. Aquacult. 445, 22–32. doi: 10.1016/j.aquaculture.2015.04.011
Deborde M., Von Gunten U. (2008). Reactions of chlorine with inorganic and organic compounds during water treatment–kinetics and mechanisms: a critical review. Water Res. 42 (1-2), 13–51. doi: 10.1016/j.watres.2007.07.025
Green A., Popović V., Pierscianowski J., Biancaniello M., Warriner K., Koutchma T. (2018). Inactivation of Escherichia coli, Listeria and Salmonella by single and multiple wavelength ultraviolet-light emitting diodes. Innovative Food Sci. Emerging Technologies. 47, 353–361. doi: 10.1016/j.ifset.2018.03.019
Guo M., Hu H., Bolton J. R., El-Din M. G. (2009a). Comparison of low- and medium-pressure ultraviolet lamps: photoreactivation of Escherichia coli and total coliforms in secondary effluents of municipal wastewater treatment plants. Water Res. 43 (3), 815–821. doi: 10.1016/j.watres.2008.11.028
Guo M., Hu H., Liu W. (2009b). Preliminary investigation on safety of post-UV disinfection of wastewater: bio-stability in laboratory-scale simulated reuse water pipelines. Desalination. 239 (1-3), 22–28. doi: 10.1016/j.desal.2008.03.003
Guo M., Huang J., Hu H., Liu W. (2011). Growth and repair potential of three species of bacteria in reclaimed wastewater after UV disinfection. Biomed. Environ. Sci. 24 (4), 400–407. doi: 10.3967/0895-3988.2011.04.011
Hijnen W. A. M., Beerendonk E. F., Medema G. J. (2006). Inactivation credit of UV radiation for viruses, bacteria and protozoan (oo)cysts in water: a review. Water Res. 40 (1), 3–22. doi: 10.1016/j.watres.2005.10.030
Hu X., Cao Y., Lu M., Xu Y., Su H., Xu W., et al. (2019). Research progress of microalgae in treatment of nitrogen and phosphorus in tail water from aquaculture. Open J. Fisheries Res. 6 (4), 172–178. doi: 10.12677/OJFR.2019.64023
Huang J., Xi J., Hu H., Li Y., Lu S., Tang F., et al. (2016). UV Light tolerance and reactivation potential of tetracycline-resistant bacteria from secondary effluents of a wastewater treatment plant. J. Environ. Sci. 41, 146–153. doi: 10.1016/j.jes.2015.04.034
Jeong Y., Ha J. (2019). Simultaneous effects of UV-a and UV-b irradiation on the survival of Escherichia coli O157:H7, Salmonella typhimurium, and Listeria monocytogenes in buffer solution and apple juice. J. Food Protection. 82 (12), 2065–2070. doi: 10.4315/0362-028x.Jfp-19-131
Kashimada K., Kamiko N., Yamamoto K., Ohgaki S. (1996). Assessment of photoreactivation following ultraviolet light disinfection. Water Sci. Technol. 33(10), 261–269. doi: 10.1016/0273-1223(96)00428-3
Kelner A. (1949). Effect of visible light on the recovery of streptomyces griseus conidia from ultraviolet irradiation injury. Proc. Natl. Acad. Sci. 35(2), 73–79. doi: 10.1073/pnas.35.2.73
Kim S., Kim D., Kang D. (2016). Using UVC light-emitting diodes at wavelengths of 266 to 279 nanometers to inactivate foodborne pathogens and pasteurize sliced cheese. Appl. Environ. Microbiol. 82(1), 11–17. doi: 10.1128/AEM.02092-15
Kollu K., Ormeci B. (2015). UV-Induced self-aggregation of E. coli after low and medium pressure ultraviolet irradiation. J. Photochem. Photobiol. B: Biol. 148, 310–321. doi: 10.1016/j.jphotobiol.2015.04.013
Lamont Y., MacGregor S., Anderson J., Fouracre R. (2004). “Effect of visible light exposure on E. coli treated with pulsed UV-rich light,” in Conference Record of the Twenty-Sixth International Power Modulator Symposium, 2004 and 2004 High-Voltage Workshop. San Francisco, CA, USA pp. 619–622. doi: 10.1109/MODSYM.2004.1433653
Lee Y., Von Gunten U. (2016). Advances in predicting organic contaminant abatement during ozonation of municipal wastewater effluent: reaction kinetics, transformation products, and changes of biological effects. Environ. Science: Water Res. Technol. 2 (3), 421–442. doi: 10.1039/C6EW00025H
Li H., Osman H. B., Kang C., Ba T. (2017b). Numerical and experimental investigation of UV disinfection for water treatment. Appl. Thermal Engineering. 111, 280–291. doi: 10.1016/j.applthermaleng.2016.09.106
Li G., Wang W., Huo Z., Lu Y., Hu H. (2017a). Comparison of UV-LED and low pressure UV for water disinfection: photoreactivation and dark repair of Escherichia coli. Water Res. 126, 134–143. doi: 10.1016/j.watres.2017.09.030
Lindenauer K. G., Darby J. L. (1994). Ultraviolet disinfection of wastewater: effect of dose on subsequent photoreactivation. Water Res. 28 (4), 805–817. doi: 10.1016/0043-1354(94)90087-6
Locas A., Demers J., Payment P. (2008). Evaluation of photoreactivation of escherichia coli and enterococci after UV disinfection of municipal wastewater. Can. J. Microbiol. 54 (11), 971–975. doi: 10.1139/w08-088%m18997854
Lu Y., Yang B., Zhang H., Lai A. C.-K. (2021). Inactivation of foodborne pathogenic and spoilage bacteria by single and dual wavelength UV-LEDs: synergistic effect and pulsed operation. Food Control. 125, 107999. doi: 10.1016/j.foodcont.2021.107999
Lushchak V. I. (2011). Environmentally induced oxidative stress in aquatic animals. Aquat. Toxicol. 101 (1), 13–30. doi: 10.1016/j.aquatox.2010.10.006
Mamane-Gravetz H., Linden K. G. (2005). Relationship between physiochemical properties, aggregation and uv inactivation of isolated indigenous spores in water. J. Appl. Microbiol. 98 (2), 351–363. doi: 10.1111/j.1365-2672.2004.02455.x
Masjoudi M., Mohseni M., Bolton J. R. (2021). Sensitivity of bacteria, protozoa, viruses, and other microorganisms to ultraviolet radiation. J. Res. Natl. Inst Standards Technol. 126, 1–77. doi: 10.6028/jres.126.021
Moreno-Andrés J., Rueda-Márquez J. J., Homola T., Vielma J., Moríñigo M.Á., Mikola A., et al. (2020). A comparison of photolytic, photochemical and photocatalytic processes for disinfection of recirculation aquaculture systems (RAS) streams. Water Res. 181, 115928. doi: 10.1016/j.watres.2020.115928
Nebot Sanz E., Salcedo Dávila I., Andrade Balao J. A., Quiroga Alonso J. M. (2007). Modelling of reactivation after UV disinfection: effect of UV-c dose on subsequent photoreactivation and dark repair. Water Res. 41 (14), 3141–3151. doi: 10.1016/j.watres.2007.04.008
Nyangaresi P. O., Qin Y., Chen G., Zhang B., Lu Y., Shen L. (2018). Effects of single and combined UV-LEDs on inactivation and subsequent reactivation of E. coli in water disinfection. Water Res. 147, 331–341. doi: 10.1016/j.watres.2018.10.014
Nyhan L., Przyjalgowski M., Lewis L., Begley M., Callanan M. (2021). Investigating the use of ultraviolet light emitting diodes (UV-LEDs) for the inactivation of bacteria in powdered food ingredients. Foods. 10 (4), 797. doi: 10.3390/foods10040797
Oguma K., Katayama H., Ohgaki S. (2004). Photoreactivation of Legionella pneumophila after inactivation by low- or medium-pressure ultraviolet lamp. Water Res. 38 (11), 2757–2763. doi: 10.1016/j.watres.2004.03.024
Okeke B. C., Thomson M. S., Moss E. M. (2011). Occurrence, molecular characterization and antibiogram of water quality indicator bacteria in river water serving a water treatment plant. Sci. Total Environment. 409 (23), 4979–4985. doi: 10.1016/j.scitotenv.2011.07.029
Quek P. H., Hu J. (2008). Influence of photoreactivating light intensity and incubation temperature on photoreactivation of Escherichia coli following LP and MP UV disinfection. J. Appl. Microbiol. 105 (1), 124–133. doi: 10.1111/j.1365-2672.2008.03723.x
Ramírez N., Serey M., Illanes A., Piumetti M., Ottone C. (2021). Immobilization strategies of photolyases: challenges and perspectives for DNA repairing application. J. Photochem. Photobiol. B: Biol. 215, 112113. doi: 10.1016/j.jphotobiol.2020.112113
Rohde M. (2019). The gram-positive bacterial cell wall. Microbiol. Spectrum. 7 (3), 7.3.10. doi: 10.1128/microbiolspec.GPP3-0044-2018
Serna-Galvis E. A., Troyon J. A., Giannakis S., Torres-Palma R. A., Carena L., Vione D., et al. (2019). Kinetic modeling of lag times during photo-induced inactivation of E. coli in sunlit surface waters: unraveling the pathways of exogenous action. Water Res. 163, 114894. doi: 10.1016/j.watres.2019.114894
Shafaei S., Klamerth N., Zhang Y., McPhedran K., Bolton J. R., El-Din M. G. (2017). Impact of environmental conditions on bacterial photoreactivation in wastewater effluents. Environ. Science: Processes Impacts. 19 (1), 31–37. doi: 10.1039/C6EM00501B
Shen L., Griffith T. M., Nyangaresi P. O., Qin Y., Pang X., Chen G., et al. (2020). Efficacy of UVC-LED in water disinfection on bacillus species with consideration of antibiotic resistance issue. J. Hazardous Materials. 386, 121968. doi: 10.1016/j.jhazmat.2019.121968
Shu H., Sun H., Huang W., Zhao Y., Ma Y., Chen W., et al. (2022). Nitrogen removal characteristics and potential application of the heterotrophic nitrifying-aerobic denitrifying bacteria Pseudomonas mendocina S16 and Enterobacter cloacae DS’5 isolated from aquaculture wastewater ponds. Bioresource Technol. 345, 126541. doi: 10.1016/j.biortech.2021.126541
Song K., Mohseni M., Taghipour F. (2019). Mechanisms investigation on bacterial inactivation through combinations of UV wavelengths. Water Res. 163, 114875. doi: 10.1016/j.watres.2019.114875
Tsenter I., Garkusheva N., Matafonova G., Batoev V. (2022). A novel water disinfection method based on dual-wavelength UV radiation of KrCl (222 nm) and XeBr (282 nm) excilamps. J. Environ. Chem. Engineering. 10 (3), 107537. doi: 10.1016/j.jece.2022.107537
Wan C., Fu L., Li Z., Liu X., Lin L., Wu C. (2022). Formation, application, and storage-reactivation of aerobic granular sludge: a review. J. Environ. Management. 323, 116302. doi: 10.1016/j.jenvman.2022.116302
Wang Y., Zheng Y., Qian X., Yang X., Chen J., Wu W. (2022). Aquaculture wastewater pollution and purification technology in China: research progress. J. Agricult. 12 (3), 65–70. doi: 10.11923/j.issn.2095-4050.cjas20200300088
Xu L., Tian C., Lu X., Ling L., Lv J., Wu M., et al. (2015). Photoreactivation of Escherichia coli is impaired at high growth temperatures. J. Photochem. Photobiol. B: Biol. 147, 37–46. doi: 10.1016/j.jphotobiol.2015.03.012
Zheng R., Xiang K., Zhu S. (2011). Bacterial inactivation effect of UV in aquaculture recirculating water. Trans. Chin. Soc. Agric. Engineering. 27 (11), 257–262. doi: 10.3969/j.issn.1002-6819.2011.11.049
Keywords: UVC-LED, aquaculture, bacteria disinfection, photoreactivation, dark repair
Citation: Zhang W, Huang R, Zhang T, Wang B, Li N, Sun Y, Ma H, Zhang Q, Zhang J and Liu Y (2023) Study on the inactivation and reactivation mechanism of pathogenic bacteria in aquaculture by UVC-LED. Front. Mar. Sci. 10:1139713. doi: 10.3389/fmars.2023.1139713
Received: 07 January 2023; Accepted: 28 April 2023;
Published: 12 May 2023.
Edited by:
Yiqin Deng, Chinese Academy of Fishery Sciences (CAFS), ChinaReviewed by:
Ravi Raghavbhai Sonani, University of Virginia, United StatesBiao Jiang, Zhongkai University of Agriculture and Engineering, China
Copyright © 2023 Zhang, Huang, Zhang, Wang, Li, Sun, Ma, Zhang, Zhang and Liu. This is an open-access article distributed under the terms of the Creative Commons Attribution License (CC BY). The use, distribution or reproduction in other forums is permitted, provided the original author(s) and the copyright owner(s) are credited and that the original publication in this journal is cited, in accordance with accepted academic practice. No use, distribution or reproduction is permitted which does not comply with these terms.
*Correspondence: Qian Zhang, qianzhang@dlou.edu.cn; Junxin Zhang, junxin_zhang@dlou.edu.cn