Assessing biogeochemical controls on porewater dissolved inorganic carbon cycling in the gas hydrate-bearing sediments of the Makran accretionary wedge, Northeastern Arabian Sea off Pakistan
- 1State Key Laboratory of Marine Geology, Tongji University, Shanghai, China
- 2Key Laboratory of Marine Mineral Resources, Ministry of Natural Resources, Guangzhou Marine Geological Survey, China Geological Survey, Guangzhou, China
Quantitatively assessing the porewater dissolved inorganic carbon (DIC) cycling in methane-enriched marine sediments is crucial to understanding the contributions of different carbon sources to the global marine carbon pool. In this study, Makran accretionary wedge was divided into Zone 1 (high methane flux area) and Zone 2 (background area). Porewater geochemical compositions (Cl–, SO42–, NH4+, Mg2+, Ca2+, Ba2+, DIC and δ13C-DIC) and a reaction-transport model were used to determine the DIC source and calculate the DIC flux through carbonate precipitation and releasing into overlying seawater in sediments. Zone 1 is characterized by the shallower depth of sulfate-methane transition (SMT), where most of porewater sulfate was consumed by anaerobic oxidation of methane (AOM). In contrast, a relatively low flux of methane diffusion in Zone 2 results in a deeper SMT depth and shallow sulfate is predominantly consumed by organoclastic sulfate reduction (OSR). Based on the porewater geochemical profiles and δ13C mass balance, the proportions of porewater DIC originating from methane were calculated as 51% in Zone 1 and nearly 0% in Zone 2. An increase of porewater DIC concentration leads to authigenic carbonate precipitation. Solid total inorganic carbon (TIC), X-ray diffractometry (XRD) and scanning electron microscopy (SEM) analysis display that carbonate content increases with depth and aragonite appears at or below the depths of SMT. Meanwhile, the flux of DIC released from sediments calculated by the reaction-transport model is 51.3 ~ 90.4 mmol/m2·yr in Zone 1, which is significantly higher than that in Zone 2 (22.4 mmol/m2·yr). This study demonstrates that AOM serves as the dominant biogeochemical process regulating the porewater DIC cycle, which has an important impact on the authigenic carbonate burial and the seawater carbonate chemistry.
1 Introduction
Methane (CH4) seepage refers to methane-rich fluid leakage from the seabed, which is widely observed in active and passive continental margin slopes. The fluids show apparent spatial-temporal variations (Haas et al., 2010; Feng et al., 2016; Feng et al., 2020; Liu et al., 2020b; Wei et al., 2021), and often be accompanied by gas hydrate accumulations in areas of gas hydrate stability and where methane concentrations reach saturation (Milkov, 2004; Coffin et al., 2014; Mau et al., 2017; Feng et al., 2018; Zhang et al., 2021a). Under the driving force of geological structure, pressure or temperature changes, the gas hydrate can dissociate and increase the intensity of gas seeps, thereby significantly affecting benthic biogeochemical processes that control the highly spatially heterogeneous seafloor ecosystems (Suess, 2014; Liu et al., 2020a; Liao et al., 2022).
Although the CH4 carbon estimates of hydrate-bound gas in global marine sediments are thought to be highly uncertain, the current estimate is widely believed to range from 1000 to 2500 Gt (Kvenvolden, 1988; Lerche, 2000; Milkov, 2004; Kretschmer et al., 2015). This buried organic carbon reservoir greatly exceeds many other reservoirs such as marine dissolved materials (980 Gt), terrestrial biota (830 Gt), the atmosphere (3.6 Gt) and marine biota (3 Gt) in the global carbon cycle (Kvenvolden, 1988). The dissociation of gas hydrates will result in the consequent liberation of various carbonaceous compounds such as CH4, CO2, and HCO3– (Suess et al., 1999; Phrampus and Hornbach, 2012). Previous studies have shown that more than 90% of the CH4 is consumed through anaerobic oxidation of methane (AOM) in marine sediments (e.g., Boetius et al., 2000; Haese et al., 2003; Chen et al., 2021; Wei et al., 2021; Li et al., 2023), which effectively prevents the diffusion of dissolved CH4 into overlying seawater or even the atmosphere. The AOM is mainly coupled to the sulfate reduction and occurs at a distinct zone which is known as the sulfate-methane transition (SMT) in the sediments (Equation 1) (Reeburgh, 1976; Borowski et al., 1996; D'Hondt et al., 2002; Snyder et al., 2007).
The upward flux of methane is theoretically nearly equal to the downward diffusive sulfate flux within the SMT due to the stoichiometric ratio of 1:1 between SO42- and CH4 in the AOM (Niewöhner et al., 1998; Knittel and Boetius, 2009; Akam et al., 2020). However, the calculated downward diffusive sulfate flux often exceeds that of the upward flux of methane (e.g., Wu et al., 2016; Akam et al., 2020; Zha et al., 2022). A compilation by Egger et al. (2018) from 740 sites covering a wide range of oceanic conditions observed the global mean net flux ratio between SO42− and CH4 of 1.4:1. This observed flux imbalance can be attributed to the organoclastic sulfate reduction (OSR) through the oxidation of buried organic matter (Berner, 1980; Jørgensen et al., 2019; Chen et al., 2020; Zha et al., 2022), and the reaction is represented in very simple form as Equation 2:
Both the microbially-mediated AOM and OSR can generate dissolved inorganic carbon (DIC) and increase porewater alkalinity (Equations 1, 2), thus leading to the promotion of authigenic carbonate precipitation (Equation 3) (Chatterjee et al., 2011; Smith and Coffin, 2014; Chen et al., 2023).
It should be pointed out that other alkaline earth elements such as Mg, Sr, Ba, and Fe can substitute for Ca in varying amounts during the process of carbonate precipitation (Equation 3). In addition to being removed by carbonate precipitation, a portion of the porewater DIC will migrate toward the seafloor and finally enter the overlying seawater (e.g., Smith and Coffin, 2014; Graves et al., 2015; Feng et al., 2020). Therefore, quantitative assessment of the porewater DIC cycle in marine sediments is crucial for better understanding the global authigenic carbonate burial and seawater carbonate chemistry.
The DIC concentration and its isotopic composition (δ13C-DIC) in porewater serve as sensitive indicators of the alteration of carbon species in marine sediments (Chen et al., 2010; Feng et al., 2020; Zha et al., 2022). Previous studies of DIC concentration and its δ13C-DIC have quantitatively tracked various sources, sinks, and transformation terms using the end-member isotopic compositions of various carbon pools (e.g., Aller and Blair, 2004; Formolo et al., 2004; Winde et al., 2014; Wu et al., 2016). Due to the high spatial and temporal heterogeneity of methane turnover above the gas hydrate stability zone (GHSZ), it is necessary to conduct geochemical investigations of the dissolved species found in the porewater of closely associated marine sediments. These resulting geochemical data will help constrain the cycling and fate of DIC related to diagenetic processes in marine sediments with similar continental margin environments, and thus contribute to ascertaining global carbon flux in these settings.
Methane seepage and obvious gas emissions widely occur on the continental slope of the Makran accretionary wedge off Pakistan (von Rad et al., 2000; Delisle and Berner, 2002; Fischer et al., 2012; Wei et al., 2021). Existing works have mainly focused on the use of geophysical methods to identify the distribution and reserves of methane hydrate in this area (von Rad et al., 2000; Römer et al., 2012; Wei et al., 2021; Wu et al., 2021). The conversion of methane carbon to DIC by geochemical processes in the Makran accretionary wedge was rarely reported and in particular, the quantitative assessment of the DIC cycle was not involved. In 2018, China Geological Survey carried out a China - Pakistan joint marine scientific cruise on the Makran continental margin offshore Pakistan (Figure 1A). The hydroacoustic evidence for Bottom Simulating Reflections (BSRs) indicates significant methane-hydrate burial in the study area (e.g., Wei et al., 2021; Wu et al., 2021; Zhang et al., 2021b). In this study, we collected six sediment gravity cores and analyzed the porewater geochemical composition (e.g., Cl–, SO42–, NH4+, Ca2+, Mg2+, Ba2+, DIC and δ13C-DIC), total organic carbon (TOC) and carbonate phase compositions. Our aims are to determine the source of DIC, the relative contributions of different geochemical processes (AOM vs. OSR) to the porewater DIC pool, and quantitatively assess the fate of DIC in the study area using the reaction-transport model.
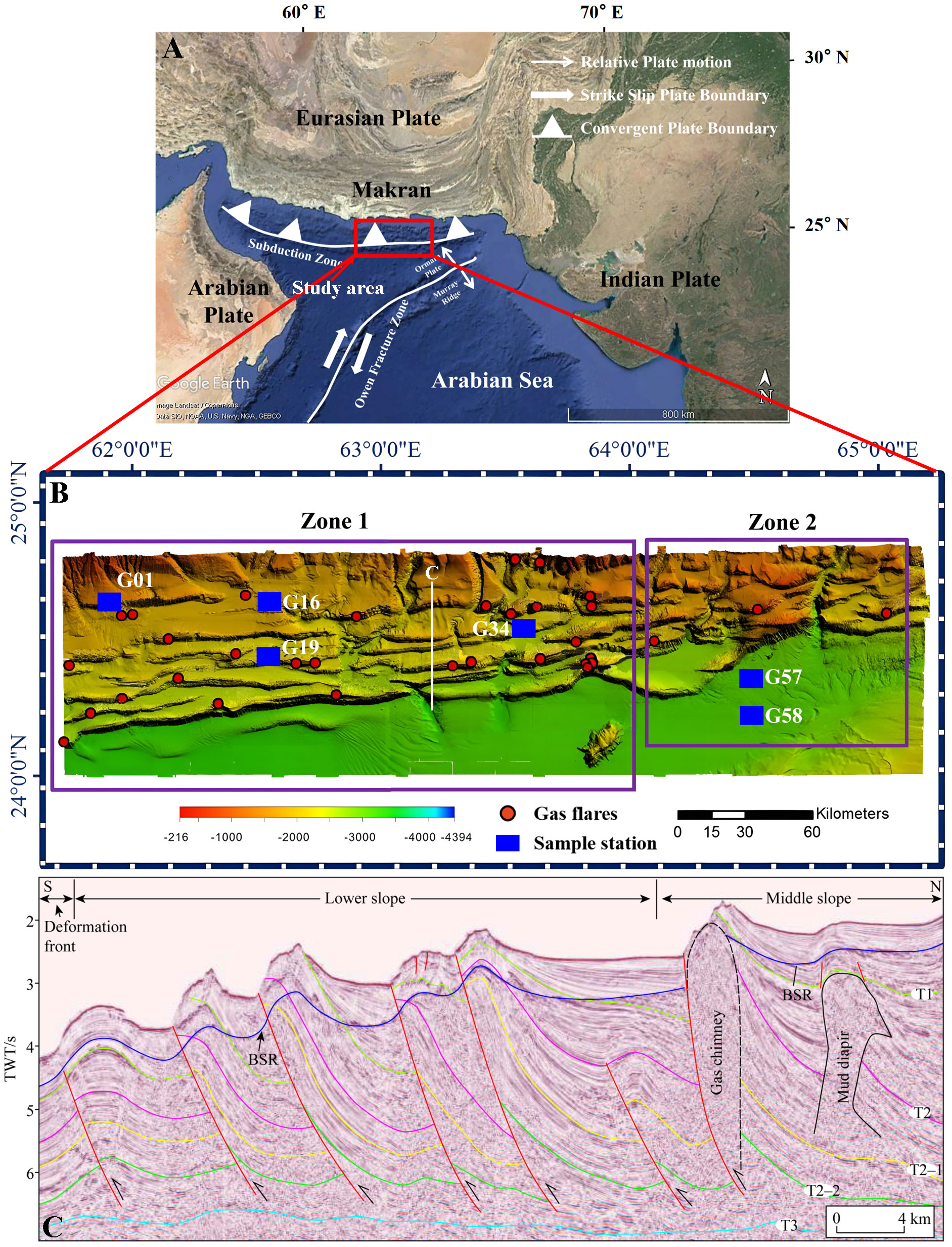
Figure 1 The maps of the study area and sampling sites. (A) Study area and its related tectonic setting; (B) Sampling sites and bottom topography of the investigated area. The stations with gas flares are referred to Wei et al. (2021). White line is the track line of the seismic profiles panel (C); (C) Multi-channel seismic data shows the occurring of bottom simulating with reversed polarity (BSR) in the study area (Zhang et al., 2020).
2 Study location and methodology
2.1 Study area
The study area is located in the Makran accretionary wedge of the northern Indian Ocean, which has a complex thrust structure and the lowest subduction angle worldwide (about 3°) (Kopp et al., 2000; Wu et al., 2021) (Figure 1A). Shallow gas accumulates and moves along normal faults in abundant anticlinal hinges created by the highest subducted sediments, resulting in seepage to the seafloor (Römer et al., 2012; Himmler et al., 2015; Liao et al., 2022). Seismic data revealed widespread distributions of BSRs in the slope area across the Makran continental margin (Figure 1C), indicating the widespread occurrence of gas hydrates in this area (e.g., Grando and McClay, 2007; Zhang et al., 2020; Liao et al., 2022). Various types of faults, mud diapirs, gas chimneys, and associated diapiric structures provide conduits for methane seepage in the Makran (von Rad et al., 2000; Schlüter et al., 2002; Khan et al., 2020; Wei et al., 2021; Wu et al., 2021; Liao et al., 2022). Methane seepage has been discovered ubiquitously (Römer et al., 2012), accompanied by cold seep ecosystems (Fischer et al., 2012) and authigenic carbonates (Himmler et al., 2015).
2.2 Sample collection
From December 2018 to February 2019, a systematic multi-beam sonar survey for gas emissions was conducted with the R/V “Haiyangdizhi 10” during a scientific diving expedition of the Makran accretionary wedge off Pakistan (Wu et al., 2021). Based on active flare density and methane seepage intensity, six sediment gravity cores with lengths ranging from 355 to 835 cm were recovered in two zones: Zone 1 (sites G01, G16, G19, and G34) has a high methane flux and a water depth of 500-2500 m; Zone 2 is the background area near Zone 1, with a water depth greater than 3000 m. In terms of geological structure, Zone 1 is heavily transected by imbricate faults and accretionary structures, while the forearc area is largely intact in deeper Zone 2 (Figure 1B).
After being retrieved, the core was promptly transported to the onboard laboratory for the purpose of collecting methane and extracting porewater. Sediment samples for methane analysis (3 ml) were taken using a 10 ml cut syringe and immediately transferred into 10 ml headspace vials, which contained 3 ml of 1 mol/l NaOH solution. The vials were subsequently capped with a rubber septum and sealed with a crimper, stored at low temperatures for the determination of sediment CH4 concentration. Porewater samples were collected at 60 cm intervals using Rhizon samplers according to the procedure presented by Liu et al. (2020a). The porewater samples were then immediately chemically treated and preserved for a variety of analysis purposes. The solid phase was divided into sub-samples at 10 cm intervals and stored at −20 °C for further analysis.
About 2 ml of porewater subsamples for cations (Ca2+ and Mg2+) and anions (SO42– and Cl–) analysis was acidified with 20 μl 65% HNO3 (suprapure, Merck) to prevent precipitation and sulfur oxidation. Samples for porewater NH4+ analysis were preserved in a 2 ml PE tube and frozen. Subsamples for measurements of DIC concentration and its isotopic composition were stored in 3 ml Labco anaerobic flasks without a headspace and kept at 4 °C in the research vessel’s cold room. Once on land, these samples were immediately placed in a refrigerated case and shipped to the onshore laboratories for further analysis within 4 weeks.
2.3 Analytical methods
2.3.1 Chemical and isotopic analysis of porewater
Dissolved Ca2+, Mg2+ and Ba2+ concentrations were diluted at 1:30 with 18-MΩ water and measured with inductively coupled plasma atomic emission spectroscopy (ICP-AES; IRIS Advantage, Thermo Jarrell Ash). Scandium (0.5 mg/L) was added to both standards and samples for correcting signal drift during instrument operation. Porewater samples were diluted with 2% subboiled HNO3 along with reference materials (CASS-4) from the National Research Council of Canada (NRCC). The accuracy and precision were better than ± 5%.
Porewater samples for SO42− and Cl− concentrations were diluted at 1:250 using 18-MΩ water and measured using a Dionex DX-600 ion chromatograph equipped with a 4 mm AS-9HC column. Reagents from the National Research Center for Certified Reference Materials (Beijing, China) were used as standards. Measurement of duplicate samples indicates the analytical error is less than 2%.
The samples for ammonium (NH4+-N) concentration were diluted at 1:5 and determined using flow injection spectrophotometry in wavelength 543 nm, as described by Hall and Aller (1992). NH4+-N standard solution was prepared using the NH4+-N reference material (BWZ6768-2016). All standard solutions were appropriately diluted to generate a series of standard concentrations. Duplicate analysis after every 10 samples was performed to validate the porewater analysis procedure, and the reproducibility is better than ± 5%.
The CH4 concentration was measured using the method of Jørgensen et al. (2001). The vial containing the sediment sample was shaken thoroughly to allow the porewater methane between the aqueous and gaseous phases to equilibrate. A 0.2-ml headspace gas sample was injected into a gas chromatograph (Agilent 6820). For porewater dissolved CH4 concentration, the calculated CH4 concentration per sediment volume is corrected by assuming a constant sediment porosity of 0.75. The two duplicate samples were measured with a precision of ± 3.0%.
The DIC concentration and its δ13C-DIC were analyzed with the 0.3 ml porewater samples according to the methods described by Liu et al. (2020a). The sealed porewater samples were acidified in precleaned extainers with 0.2 ml of 2 mol/l H3PO4, and the resulting CO2 was purged from the samples. The DIC concentration and the carbon isotopic composition of the CO2 were measured using a Thermo Finnigan MAT 253 isotope ratio mass spectrometer coupled with a Thermo Finnigan Gasbench II. The DIC concentration was measured with a precision of ± 2%. The carbon isotopic ratios are reported in the standard δ notation concerning the Vienna PeeDee Belemnite (VPDB) standard and with both precision and accuracy of better than ± 0.15% (1σ).
2.3.2 Solid sediment analysis
Sediment total organic carbon (TOC) and its stable isotopic values (δ13C-TOC) were analyzed in duplicate, using an elemental analyzer (Vario EL III, Germany) connected to a continuous flow system (Isoprime 100), after removal of carbonate with HCl. The average standard deviations of the measurements were ± 0.2% for TOC and ± 0.2‰ for δ13C-TOC. Weight percentages of total carbon (TC) were determined in duplicate (without acidification) by combustion (at 1000°C) on the elemental analyzer, and total inorganic carbon (TIC) was determined by difference: TIC = TC − TOC.
The X-ray diffraction (XRD) was performed to characterize the mineralogy of the powders of interest. Selected samples (55 cm, 415 cm, and 475 cm at site G16; 55 cm, 415 cm, and 535 cm at site G34; 55 cm, 355 cm and 775 cm at site G58) of 1.5 g were micronized under ethanol with a McCrone micronizing mill and then oven-dried at 60°C. After drying, the micronized samples were thoroughly mixed in an agate mortar and pestle to ensure homogeneity, followed by analyses using an X’pert Pro X-ray powder diffractometer (PANalytical, Netherlands) with Cu Kα radiation at 40 kV and 40 mA. The measurements were carried out in the range of diffraction angles 2θ from 4° to 80° with a scanning step of 0.033° and rotation of the sample at a speed of 15 rpm. Qualitative and quantitative analyses of minerals were performed using the software Profex (version 5.0.2) (Doebelin and Kleeberg, 2015).
Scanning electron microscopy (SEM) (Tescan Mira 3) with energy-dispersive X-ray microanalysis (EDX) (Oxford Ultim Max 40) was used to determine the morphology and elemental composition of the sites G16 and G34 samples because of their high level of carbonate content based on the XRD analysis (Supplementary Table S1). The freeze-dried subsamples were fixed onto aluminum stubs with two-way adherent tabs and allowed to dry overnight. Subsequently, the samples were sputter-coated with gold for 30 s. The SEM was operated at 2 kV with a working distance of 10 mm to facilitate optimum image collection while minimizing charging and sample damage. For EDX analysis, an accelerating voltage of 20 kV was used to generate sufficient X-ray counts.
3 Results
3.1 The depth profiles of porewater geochemistry
Figure 2 shows the depth profiles of the Cl–, SO42–, CH4 and NH4+ concentrations in the porewater of Zone 1 and Zone 2. Porewater Cl– concentration at all sampling sites remains almost constant and close to that in seawater. The SO42– concentration decreases almost linearly with depth in Zone 1, and complete depletion occurs at around the depth of 400 cmbsf (centimeters below sea floor) ~ 500 cmbsf (SMT depth). By contrast, complete depletion of SO42– concentration appears at around 1800 cmbsf at site G57 and 1100 cmbsf at site G58 in Zone 2. A noticeable increase in CH4 concentration was observed around the SMT of the sites G01, G16, G19 and G34 in Zone 1, but CH4 concentration is close to 0 and no change with depth at sites in Zone 2. The concentration of NH4+ ranges from 209.50 μM to 3243.00 μM, and an obvious negative correlation between the NH4+ and SO42– in depth profiles occurs at all sites.
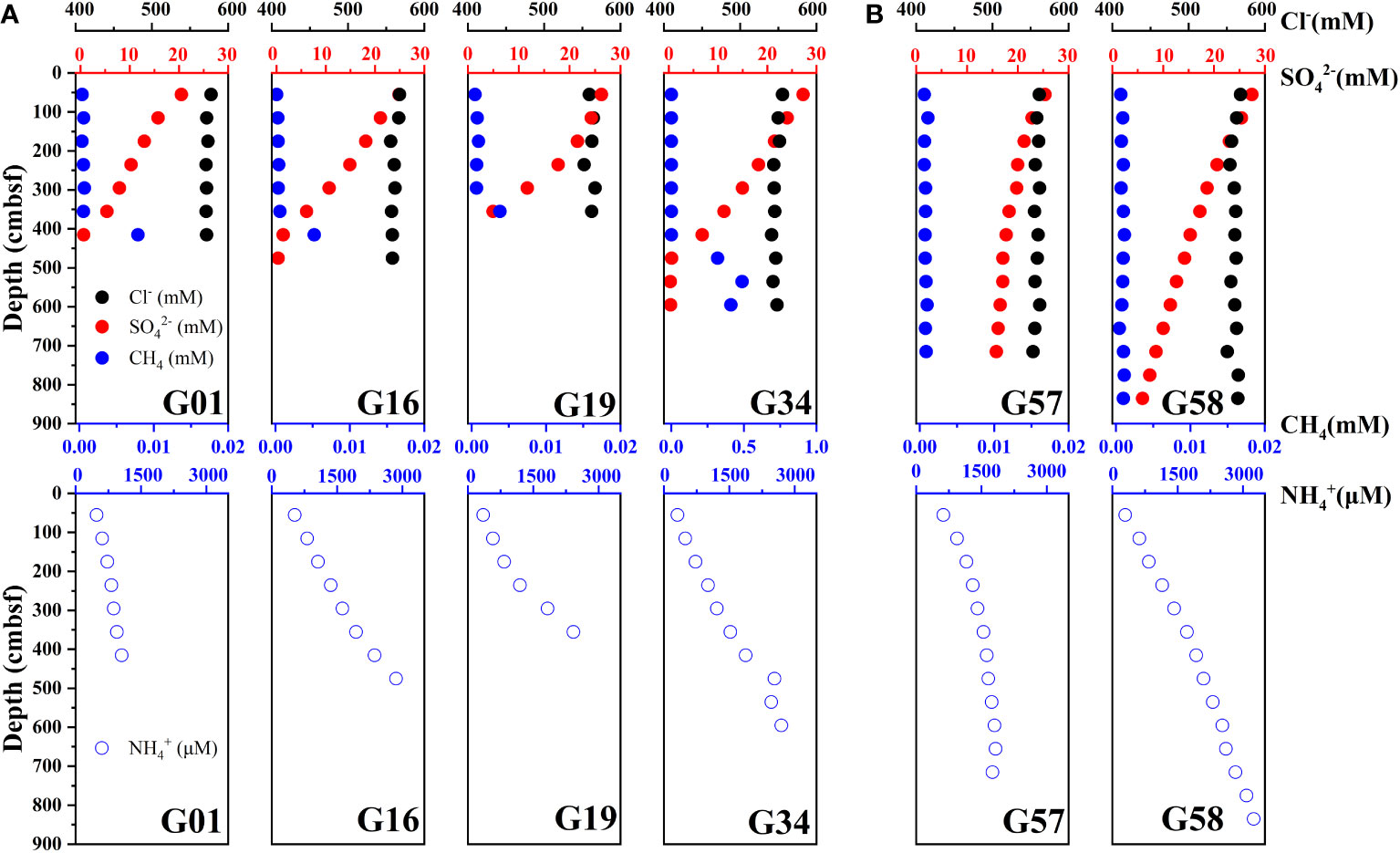
Figure 2 Down-depth profiles of porewater SO42-, Cl-, CH4 and NH4+ in the sediments of the sites (A) G01, G16, G19, and G34 (Zone 1), and (B) G57, G58 (Zone 2).
Depth profiles of the porewater Ca2+, Mg2+ and Ba2+ are shown in Figure 3. The porewater Ca2+ concentrations at sites in Zone 1 and Zone 2 vary from 1.38 – 7.96 mM and 4.40 – 8.50 mM, respectively. The porewater Mg2+ concentrations are in the range of 37.76 – 55.91 mM and 50.64 – 56.33 mM at sites in Zone 1 and Zone 2. The concentrations of Ca2+ and Mg2+ decrease with depth in Zone 1, and their decreasing trends are more pronounced than those in Zone 2. The porewater Ba2+ concentrations at the sites of Zone 1 vary from 0.33 to 4.76 μM, and display a noticeable increasing trend below the depth of complete depletion of porewater sulfate. At the sites of Zone 2, however, the Ba2+ concentrations remain almost constant in the depth profiles, with a range of 0.35 to 0.54 μM.
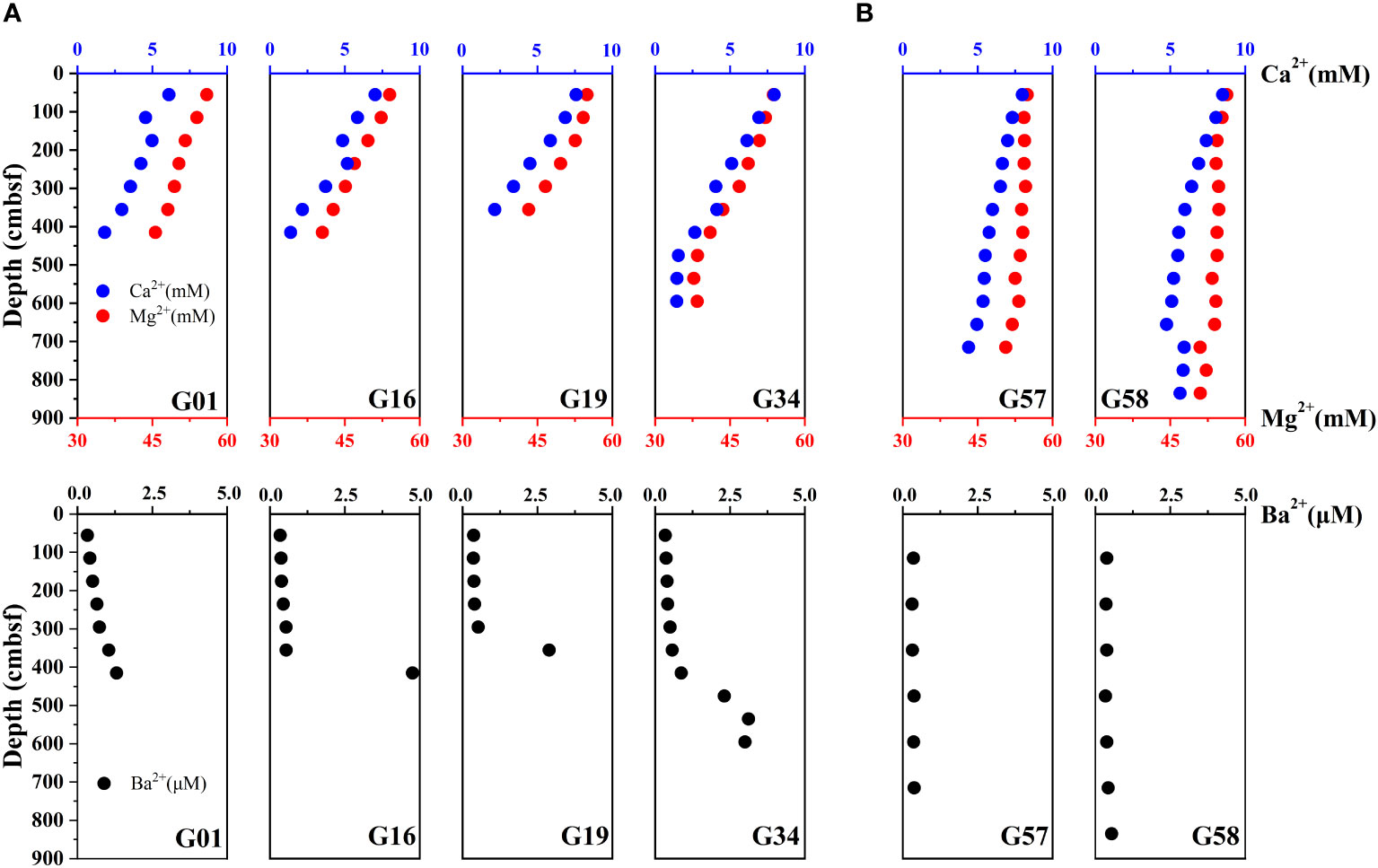
Figure 3 Down-depth profiles of porewater Ca2+, Mg2+ and Ba2+. (A) G01, G16, G19, and G34 (Zone 1), and (B) G57, G58 (Zone 2).
The porewater DIC concentration increases with the depth, and the δ13C-DIC values are negatively correlated with DIC concentration at all sites in Zone 1 (Figure 4A). The porewater DIC concentrations at sites G01, G16, G19 and G34 vary from 11.85 – 24.29 mM, 5.47 – 18.26 mM, 4.24 – 15.45 mM and 3.79 – 18.87 mM, and the δ13C-DIC values range from −29.57 to −15.82 ‰, −35.04 to −15.70 ‰, −39.60 to −12.20 ‰ and −27.39 to −11.27 ‰, respectively. The most negative δ13C-DIC occurs at the depth of maximum sulfate depletion. For site G58 in Zone 2 (Figure 4B), δ13C-DIC values decrease almost linearly from −8.48‰ at the top to a minimum of −15.52‰ at the bottom. For site G57 in Zone 2 (Figure 4B), which has a much deeper SMT, the change in δ13C-DIC values is much more gradual since the site of AOM contributions to DIC is located deeper.
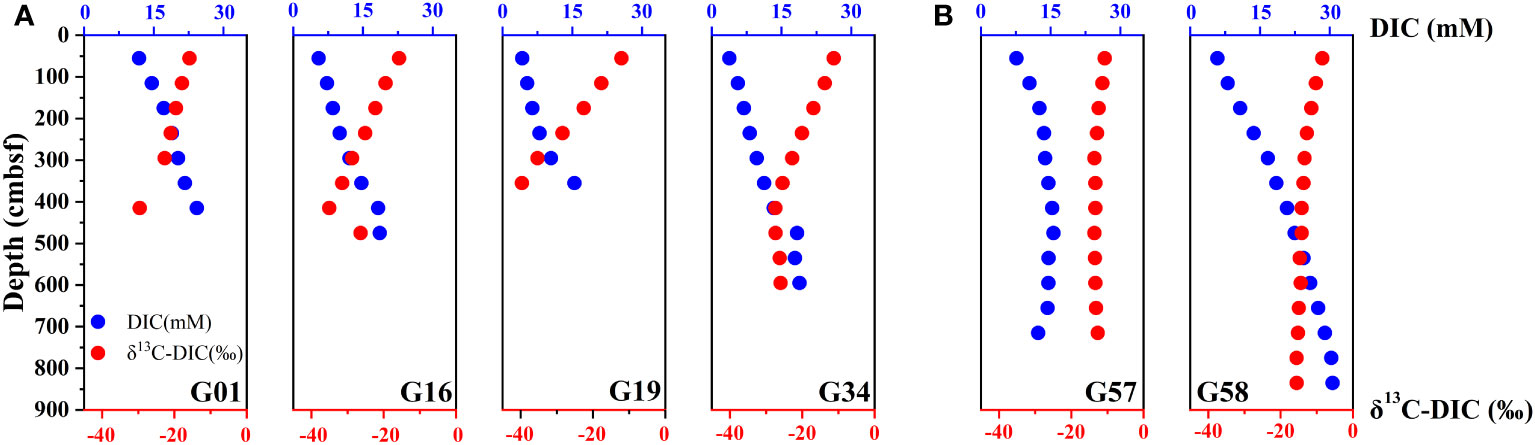
Figure 4 Down-depth profiles of porewater DIC and δ13C-DIC in the sampling sites (A) G01, G16, G19, and G34 (Zone 1), (B) G57, G58 (Zone 2).
3.2 The depth profiles of sediment TOC, TIC and δ13C-TOC
Figure 5 displays the depth profiles of sediment TOC, TIC and δ13C-TOC. At the sites of Zone 1, the TOC shows a decreasing trend as depth increases, varying from 2.02% to 0.28%, while the TIC contents are in the range of 2.03% – 4.21% and exhibit a small increase trend with depth. In contrast, the TOC and TIC at site G58 in Zone 2 show small fluctuations in the depth profile, with ranges of 0.59% – 1.32% for TOC and 2.15% – 2.69% for TIC, respectively. At all sites, there is no clear trend of the δ13C-TOC variation in the depth profile, and their values range from –24.14‰ to –20.59‰.
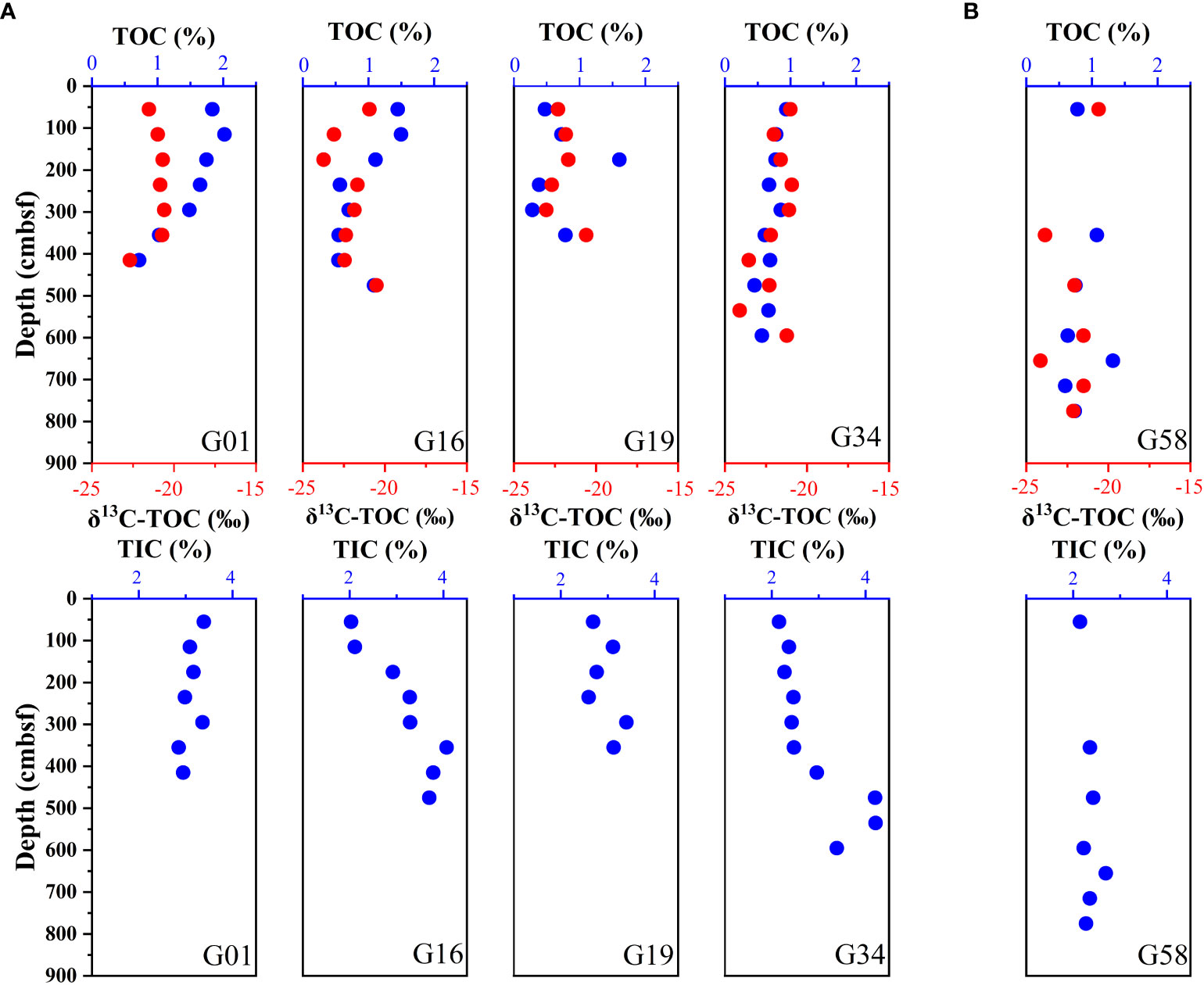
Figure 5 Down-depth profiles of sediment TOC, δ13C-TOC and TIC. (A) G01, G16, G19, and G34 (Zone 1), (B) G58 (Zone 2).
3.3 Morphology and mineralogy of the carbonate phase
Based on the vertical distribution of porewater geochemical profiles (Figures 2, 3), we selected the sediment samples from three different depths of Zone 1 (sites G16 and G34) and Zone 2 (site G58) for XRD analysis, and the results are shown in Figure 6 and Supplementary Table S1. Quantitative analyses of minerals were performed using the default structure repositories in the software Profex (version 5.0.2). Overall, the carbonates at sites G16, G34 and G58 are composed of calcite, aragonite, and dolomite. The percentage of each carbonate mineral within the bulk sediment and the relative abundance of each carbonate type in total carbonates are listed in Supplementary Table S2. By comparison, the ratio of calcite/∑carbonate is the highest in all selected samples (66.48% ~ 85.18% at site G16; 51.56% ~ 81.69% at site G34; 75.59% ~ 82.68% at site G58). Aragonite is almost undetectable in the shallow sediments (55 cmbsf depth) of sites G16 and G34 (aragonite/∑carbonate = 0), and not detected at all selected depths of site G58. The ratio of aragonite/∑carbonate decreases from 20.61% at 415 cmbsf depth to 6.94% at 475 cmbsf depth of site G16, and it increases dramatically from 4.50% at 415 cmbsf depth to 35.26% at 535 cmbsf depth of the site G34. However, the difference in the ratio of dolomite/∑carbonate is very small in all selected samples (12.91% ~ 14.82% at site G16; 13.18% ~ 18.64% at site G34; 17.32% ~ 24.41% at site G58).
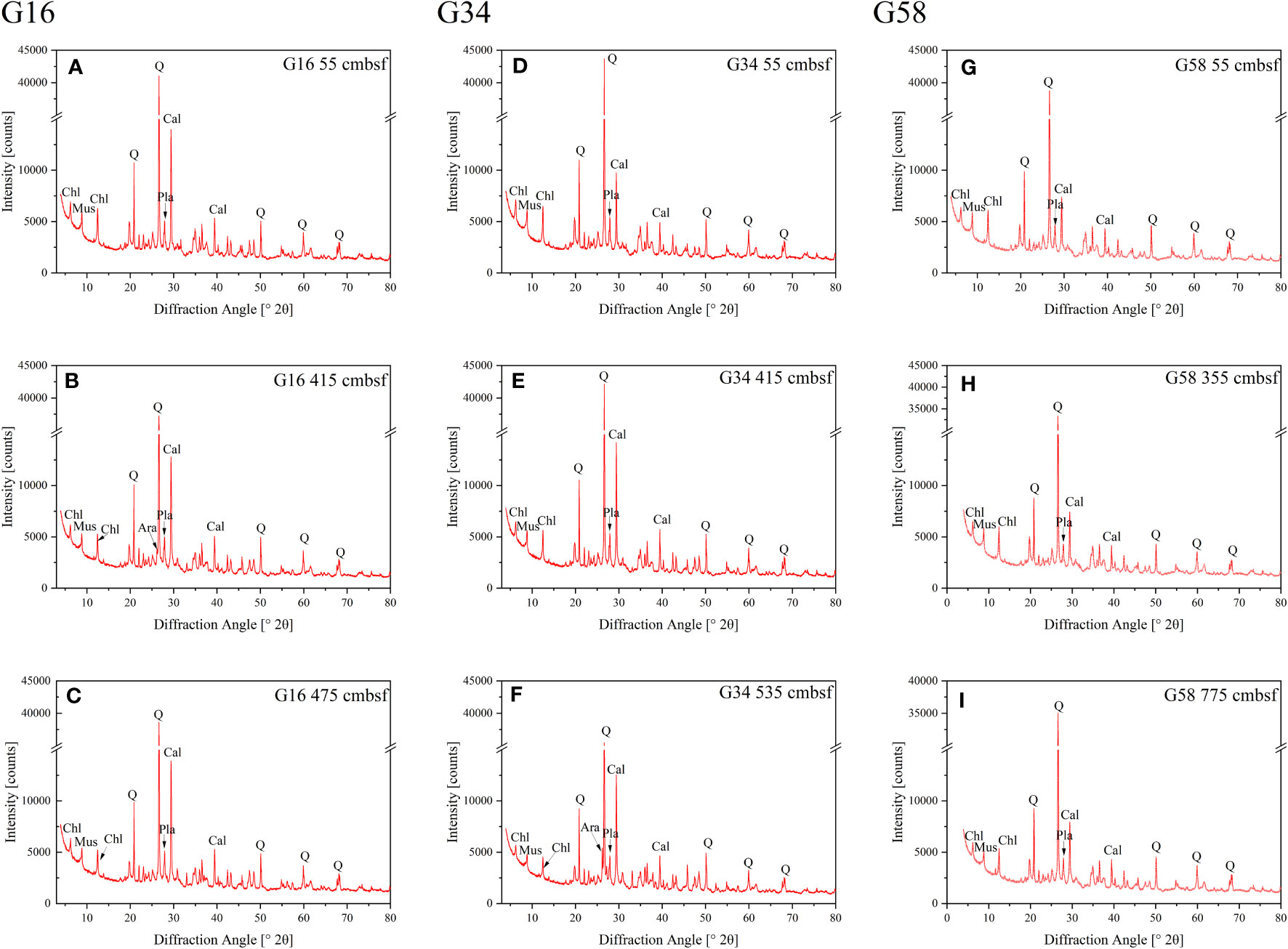
Figure 6 The XRD spectra of the selected sediment samples from three different depths of Zone 1 (G16 and G34) and Zone 2 (G58). (A) G16 55 cmbsf; (B) G16 415 cmbsf; (C) G16 475 cmbsf; (D) G34 55 cmbsf; (E) G34 415 cmbsf; (F) G34 535 cmbsf; (G) G58 55 cmbsf; (H) G58 355 cmbsf and (I) G58 775 cmbsf. Only minerals with a content greater than 5% are shown in the figures (Q, quartz; Cal, calcite; Mus, muscovite; Chl, chlorite; Pla, Plagioclase; Ara, aragonite).
In addition to XRD analysis, the SEM images and EDS signals can also be utilized to identify the various carbonate phases. In this study, most of the carbonate concretions were observed in the deep layer of sites G16 and G34 (Figure 7; Supplementary Table S3). The mineral morphology at the 415 cmbsf depth of site G16 is analogous to that at the 535 cmbsf depth of site G34. Both samples contain aragonite and calcite, where aragonite occurs in the habit of acicular CaCO3 with roughly strip-shaped bodies ranging from 1 to 3 μm in diameter (Figures 7B, G), and calcite appears in a massive form (Figures 7C, H). At the other depths of the sites G16 and G34, no strip-shaped aragonite but only calcite was found (Figures 7A, D–F).
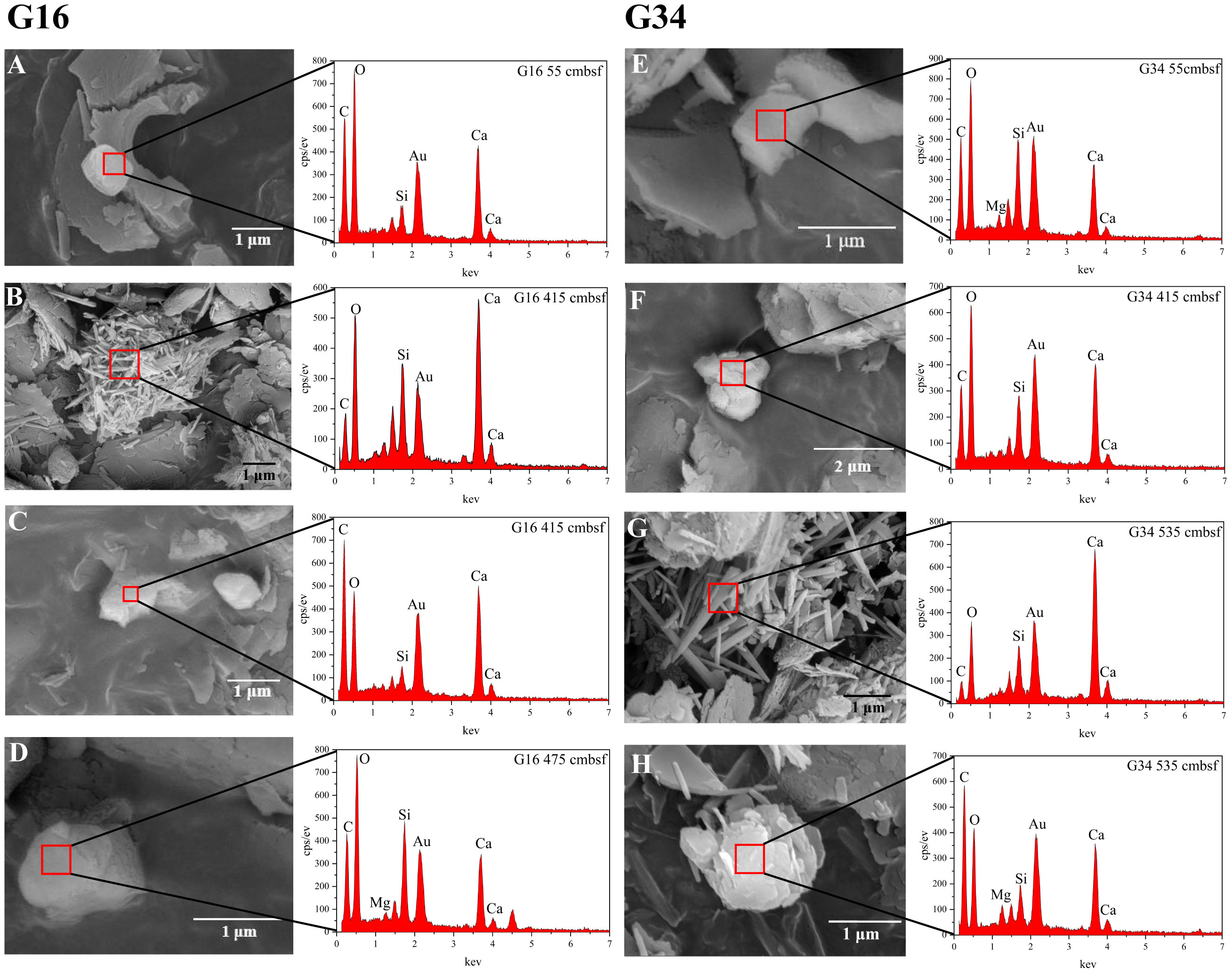
Figure 7 SEM images and EDS signals for sites G16 and G34. (A) Massive calcite at a depth of 55 cmbsf of site G16, (B) small loosely packed aragonite needle cluster with mainly long needles (1 ~ 3 μm) at a depth of 415 cmbsf of site G16, (C) the presence of calcite at a depth of 415 cmbsf of site G16, (D) calcite at a depth of 475 cmbsf of site G16, (E) massive calcite at a depth of 55 cmbsf of site G34, (F) a notable presence of calcite at a depth of 415 cmbsf of site G34, (G) the aragonite at a depth of 535 cmbsf of site G34, which is closely resembled that at a depth of 415 cmbsf of site G16, (H) calcite at a depth of 535 cmbsf of site G34.
4 Modeling results of porewater geochemical profiles
The porewater geochemical profiles of sulfate, methane, calcium, magnesium, and DIC concentration were simulated by a reaction-transport model (RTM) under steady state (e.g., Berner, 1980; Chatterjee et al., 2011; Meister et al., 2019; Xu et al., 2023). The RTM was written using MATLAB R2023a and its code is provided in the Supplementary Material.
The instantaneous reaction rate equation (Equation 4) is as follows:
where C is the concentrations, t is time, ω is the sedimentation rate, φ is the porosity of the marine sediment, Ds is the effective diffusion constant and is corrected by advection and tortuosity (τ2) as τ2 = 1-2·lnφ (Boudreau, 1997). The specific values of simulation parameters are shown in Table 1, and part of the relevant data in the table refers to previous studies in this area (Luff et al., 2000; Hu et al., 2018).
The time of simulation is related to the sedimentation rate and porosity. Here we used an exponential function to describe porosity:
where the value of porosity (φ0) of sediment at the sediment-water interface (SWI) is set to 0.8, λ (-0.01) is the attenuation coefficient and x is the depth. The burial time can be calculated as (Meister et al., 2019):
Sources and sinks of methane, sulfate, and DIC are stoichiometrically coupled to rates of organic matter (OM) degradation via the simplified reactions for sulfate reduction (SR) and methanogenesis (ME).
For OM degradation, the reactive continuum model (RCM) was applied (Boudreau and Ruddick B., 1991):
where the parameter a describes the average lifetime of OM, v defines the shape of the distribution, t is burial time calculated by Equation 6 and G represents the content of OM.
Based on the OM degradation rate, the SR and ME rates can be written as follows:
where fs is an error residual function (Chuang et al., 2019; Xu et al., 2023), as:
where Cs is the sulfate concentration, CS* is the threshold sulfate concentration for methanogenesis (~ 1 mM), and b (~ 0.001) is a parameter controlling the steepness of fS. When OM is degradated by OSR, fs = 1; and when OM is degradated by methanogenesis, fs = 0.
The ratio of DIC production from sulfate consumption via AOM is 1:1, and the rate of AOM can be described as:
where CM is methane concentration, CS is sulfate concentration and kAOM is the first order rate constant for AOM.
The precipitation of carbonate is the sink of calcium and DIC (Equation 3), and its rate was calculated as Equation 12 (Luff et al., 2001):
where Ccalcium and Ccarbonate represent the contents of calcium ions and carbonate, K*SP is the stoichiometric solubility constant of solid carbonate, kCa is the first-order kinetic rate constant for carbonate precipitation. The precipitation rate of magnesium (Mg2+) in porewater is correlated with the precipitation rate of Ca2+ (Fantle and DePaolo, 2006; Fantle and DePaolo, 2007; Zhang and DePaolo, 2020), which can be written as following:
where CMg and CCa are the porewater concentrations of Mg2+ and Ca2+, and kMg is the first-order kinetic rate constant for magnesium precipitation.
The absolute concentrations of [13DIC] and [12DIC] were computed by separate reaction-transport (Equation 14) for each isotope (R = R12 + R13). Minor carbon isotope fractionation occurs during OSR, AOM and carbonate precipitation (Meister et al., 2013; Chuang et al., 2019; Meister et al., 2019). Hence, the sources and sinks are assumed proportional to the isotopic composition of the source pool, and the rate of CH4 can be calculated as follows:
Methanogenesis can occur via two main pathways: the autotrophic pathway using CO2 and H2 and the acetoclastic pathway using acetate. However, independent of the pathway, the sources of methane and DIC are in isotopic proportion with the OM by the fractionation factor α and 2-α, respectively (Meister et al., 2019).
The concentration profiles of diverse species and RAOM from Zone 1 and Zone 2 were simulated in this study. The modeling results are almost consistent with the measured concentrations of SO42−, Ca2+, and Mg2+ in Zone 1 and Zone 2 (Supplementary Table S4; Figure 8). By contrast, DIC concentration and δ13C-DIC have a poor match with the simulated curve (Figure 8) and the possible explanation is that DIC samples undergo degassing during collection and storage, which will cause CaCO3 to precipitate from the solution and lead to changes in physiochemical conditions (Miller et al., 2017).
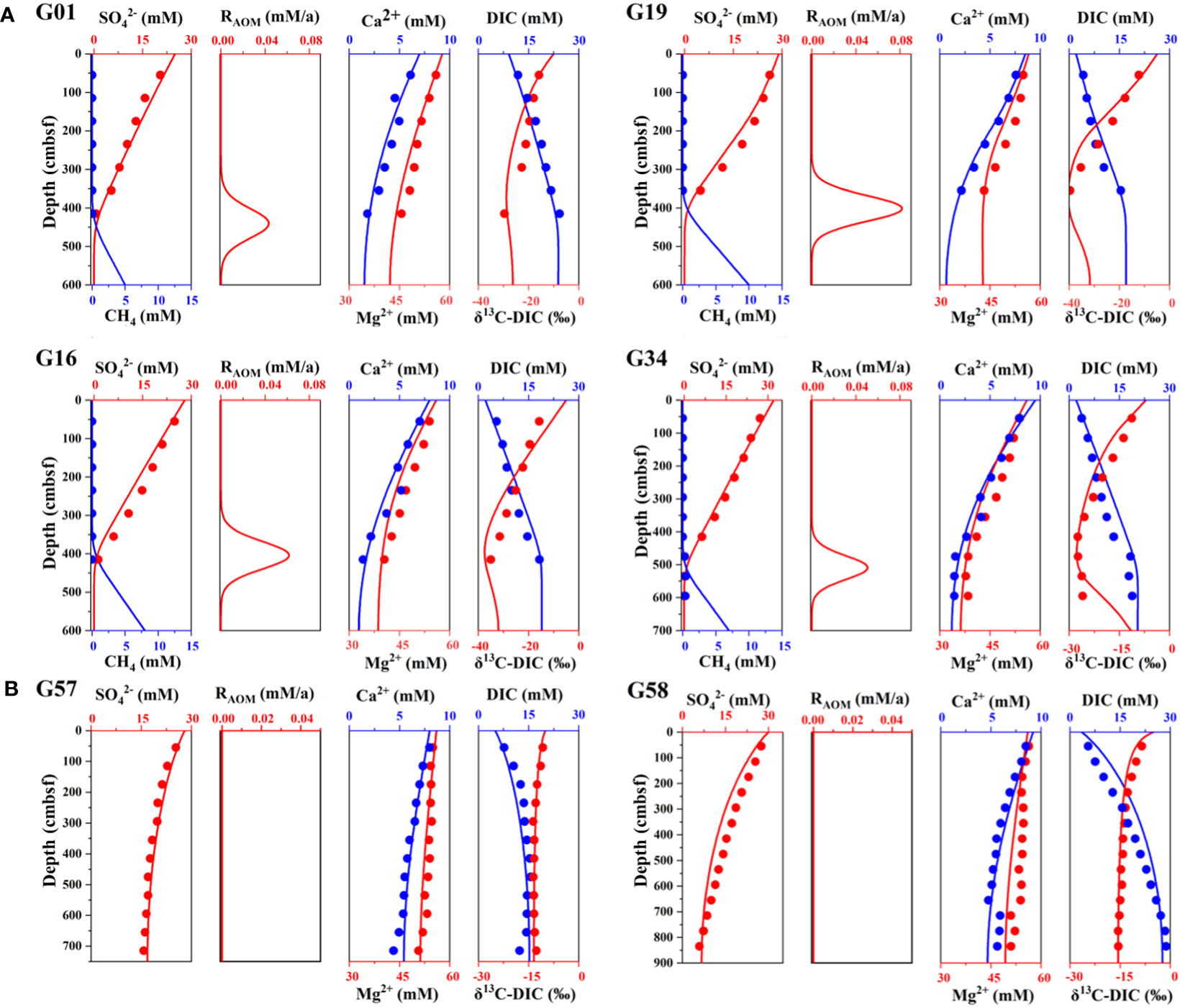
Figure 8 Measured (dots) and simulated (curves) depth profiles of the study sites (A) G01, G16, G19, and G34 (Zone 1) and (B) G57 and G58 (Zone 2). Down-depth profiles of SO42–, CH4, RAOM, Ca2+, Mg2+, DIC and δ13C-DIC. (The depth range of each site in the figure is different).
Considering the uncertainty in methane sampling, the simulation of methane profiles is not only based on methane concentration profiles but also the porewater profiles of SO42- and δ13C-DIC. As shown in Figure 8, the methane concentration at sites G01, G16, G19, and G34 in Zone 1 increases significantly at the bottom of sediments, while there is no methane occurring within the depth profiles of the sites G57 and G58 in Zone 2. In addition, the RAOM of Zone 2 is zero and the decreasing trend of Ca2+ and Mg2+ is not as obvious as that of Zone 1. Based on the calculation of the reaction-transport model, site G19 has the highest value of RAOM with 71.55 mmol/m2·yr. Furthermore, the rates of authigenic carbonate precipitation are simulated as 7.54 mmol/m2·yr at site G01, 8.12 mmol/m2·yr at site G16, 7.20 mmol/m2·yr at site G19, and 10.11 mmol/m2·yr at site G34, respectively. In contrast, the rates of authigenic carbonate precipitation in Zone 2 are much lower than those in Zone 1. According to the OM degradation rate and calculation by the reaction-transport model (Equation 9), the methanogenesis rates at sites G01, G16, G19 and G34 are 1.70 mmol/m2·yr, 2.30 mmol/m2·yr, 1.40 mmol/m2·yr and 0.92 mmol/m2·yr, respectively, however, the rates at sites G57 and G58 are nearly 0 (Supplementary Table S5).
5 Discussion
5.1 The relationship of the fluxes of DIC and SO42- with SMT depth
The SMT, an important biogeochemical zone characterized by the simultaneous consumption of sulfate and methane (AOM, Equation 1), is commonly observed in methane-rich marine sediments at relatively shallow depths below the seafloor (1 ~ 30 mbsf) (Malinverno and Pohlman, 2011). Within or near the SMT zone, the depletion of sulfate can lead to the dissolution of barite, thereby causing an increase in the concentration of porewater Ba2+ (Aloisi et al., 2004; Riedinger et al., 2006; Snyder et al., 2007). In this study, we also observed an increasing trend of Ba2+ concentration at or below the depth of SMT, but its concentration was relatively low, which may be mainly due to the lower content of barite minerals in the sediments (Supplementary Table S1). Here, we define the depth of SO42− becomes near depleted (1 mM) as the SMT depth, which can be derived using the least square method by fitting the SO42– depth profiles of all sampling sites (Borowski et al., 1999; Arndt et al., 2013; Chuang et al., 2016; Feng et al., 2018). When the sediment core sampling does not cross the SMT, the depth of the SMT can be achieved by extrapolating the SO42− concentration gradient to a depth where porewater SO42− concentrations are near depleted (Smith and Coffin, 2014).
It should be noted that a shallow SMT often occurs a few centimeters below the seafloor with significant advection of upward fluids, such as seeps and vents, because the advecting fluids typically have a higher concentration of CH4 than surrounding sediment, and advection often involves multiphase fluid flow (free gas and liquid) that may be episodic (Luff and Wallmann, 2003; Miller et al., 2017). However, the depths of SMT at our sampling sites are greater than 4 m and the porewater SO42- concentrations exhibit a linear decrease in depth profiles, thereby suggesting that the impact of advection of upward fluids is not significant in this study area. Assuming under steady-state conditions, the diffusion rates of diverse species such as SO42–, Ca2+, Mg2+ and DIC, can be calculated from the linear fit to the species concentration (Supplementary Table S4) gradient according to Fick’s first law (Equation 17) (e.g., Berner, 1978; Coffin et al., 2015):
where J is the diffusive flux (mmol/m2 yr); φ is the porosity; Ds is the diffusion coefficient (m2/s) which is related to temperature; c is the concentration of diverse species in pore water (mM), and x is the depth (mbsf). The bottom water temperatures at the study sites are shown in Table 1, and porosity is obtained by a functional relationship with depth (Equation 5). In this study, we set that the downward flux (SO42–) is negative (into the sediments) corresponding to an upward flux (positive) for DIC out of the sediments. Due to the core sampling not crossing the SMT, it is difficult to estimate the flux of DIC upward from below the SMT. Nonetheless, our calculation results of the reaction-transport model show that the methanogenesis rate is much lower than the rates of AOM and OSR, indicating the flux of DIC from below SMT is much smaller than that from above SMT (Equation 9; Supplementary Table S5). Thus, the DICnet flux is approximated as DIC diffusion upward through the sediments above the SMT (JDIC) and the loss of DIC by authigenic carbonate precipitation (Table 2), where the loss flux of DIC can be estimated using the porewater concentration profiles of Ca2+ and Mg2+ (Equation 18) (Smith and Coffin, 2014):
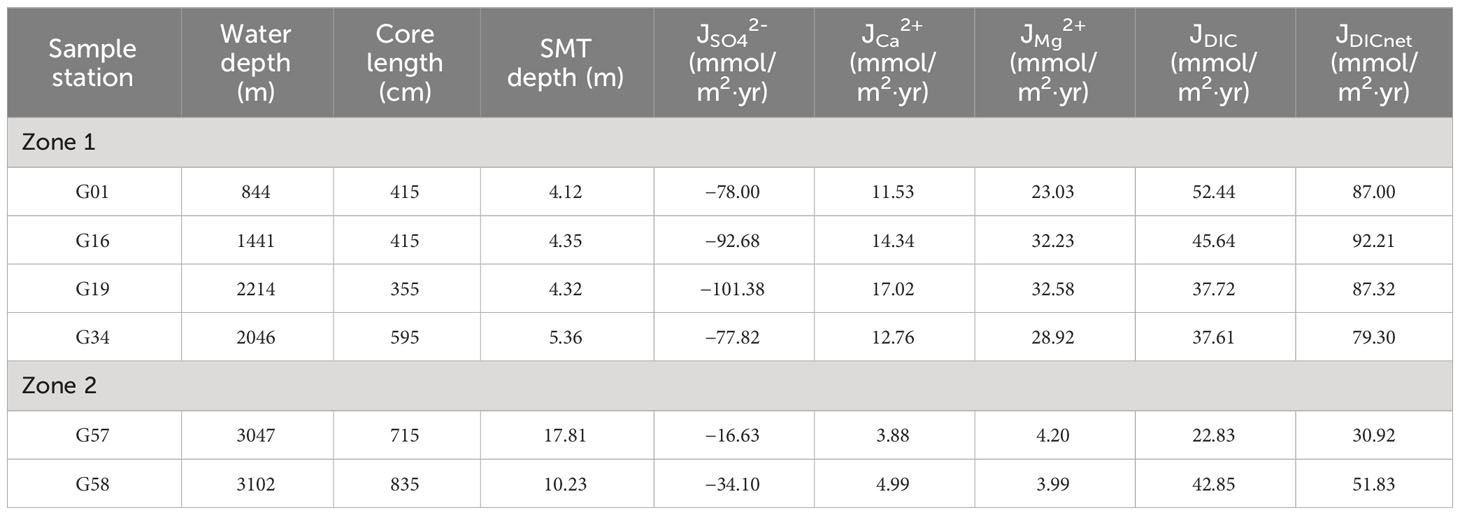
Table 2 SMT depth, sulfate diffusion rates (JSO42-), Ca2+ diffusion rates (JCa2+), Mg2+ diffusion rates (JMg2+) and DIC diffusion rates (JDIC) at all sampling sites of methane-hydrate area in Makran continental margin.
The fluxes of SO42– and DICnet in Zone 2 are significantly lower than those in Zone 1, and the larger fluxes of SO42– and DICnet correspond to the shallower SMT depth (Table 2). We combined the data from this study with the published data (Figure 9), and found that the DICnet and SO42– fluxes of all comparing sites have an exponential relationship with SMT depths (y = 273.63x−1.17 and y = −267.89x−1.37, Figure 9), which is very consistent with the report by Miller et al. (2017). The explanation for this correlation potentially lies in the fact that the fluxes of DIC and SO42– to the SMT depth are mainly controlled by the reactivity and content of sediment OM in depth profiles (Egger et al., 2018; Xu et al., 2023). The diffusion flux of SO42– is approximately equal to that of CH4 within the SMT (Equation 1), so the depth of the SMT can provide a relatively qualitative prediction of upward CH4 flux in the sediments.
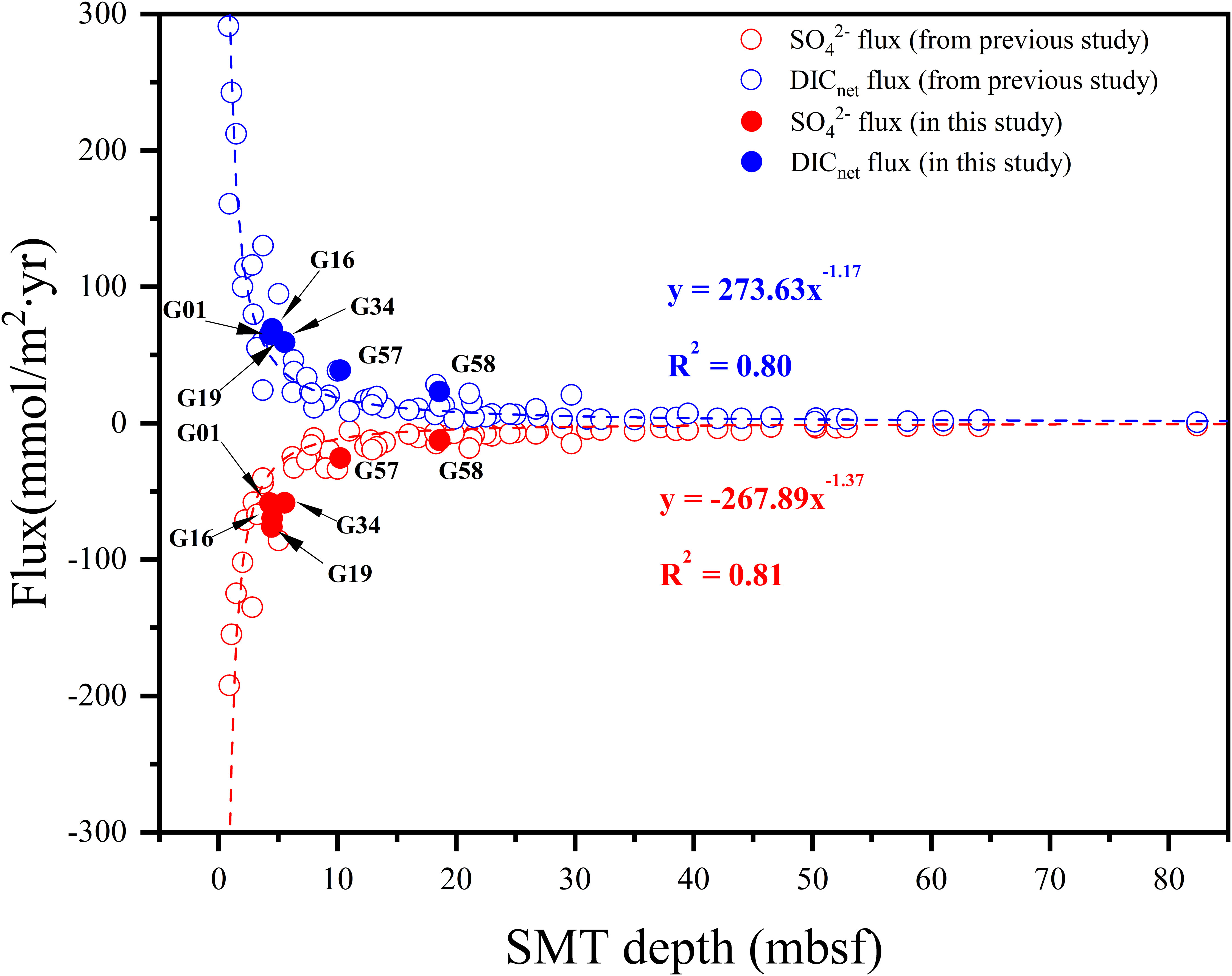
Figure 9 The exponential relationship of SO42− and DICnet flux with SMT depth. The data are soured from the Makran accretionary wedge (Zhang et al., 2021b), Beaufort Sea (Coffin et al., 2013; Miller et al., 2017), East Siberian Slope (Miller et al., 2017), New Jersey Continental Slope (Berg, 2008), Blake Ridge (Paull et al., 1996; Keigwin et al., 1998; Berg, 2008), Gulf of Mexico (Kastner et al., 2008), Amazon Fan (Flood et al., 1995; Burns, 1998; Berg, 2008), Western Africa (Wefer et al., 1998; Berg, 2008), Oman (Berg, 2008), Bering Sea (Keigwin et al., 1998; Takahashi et al., 2011), Cascadia (Claypool et al., 2006; Dickens and Snyder, 2009), Umitaka Spur (Snyder et al., 2007), Japan Sea (D'Hondt et al., 2002), California Margin (Berg, 2008), Nankai Trough (Moore et al., 2001; Berg, 2008), Costa Rica Margin (Kimura et al., 1997; Berg, 2008), New Zealand (Hamdan et al., 2011; Coffin et al., 2014) and Antarctic (Geprägs et al., 2016), where the flux calculation results are cited from Miller et al. (2017).
5.2 Sulfate consumption pathways (OSR vs. AOM)
The depth profiles of porewater δ13C-DIC exhibit the obvious characteristic pattern reflecting the occurrence of AOM. Especially, the minimum values of δ13C-DIC (−27.40 ‰ to −39.60 ‰, Figure 4) at the depth of sulfate depletion are significantly more negative than that of the δ13C of sedimentary OM found in this region (~ −21.0 ‰, von Rad et al., 1996). If only the degradation of organic carbon occurs within this depth range, then the δ13C-DIC value should lie between that of seawater (0 ‰ PDB) and that of organic carbon (~−21.0 ‰). Thus, the isotopic signature of DIC of this study indicates the additional source of light carbon in Zone 1 (Figure 4). The most likely source is AOM, which produces δ13C-DIC that is isotopically lighter than that predicted from the simple mixing of the carbon derived from the OM degradation and seawater. Besides this, the decomposition of OM through bacterial sulfate reduction (OSR) can generate DIC and NH4+ and enter the surrounding porewater in the methane-bearing sediments (e.g., Luo et al., 2013; Zha et al., 2022), so NH4+ is an effective indicator of the extent of OM degradation because NH4+ is mainly produced via anaerobic bacterial decomposition of nitrogen-bearing organic compounds (Wehrmann et al., 2011; Mazumdar et al., 2014). It should be mentioned that the distribution of porewater NH4+ is influenced by many other factors. The first is the dilution of freshwater released by methane-hydrate decomposition. However, no significant fluctuations in Cl− concentration were observed in the depth profiles, indicating that porewater NH4+ concentration is not affected by this effect. Secondly, methanogenesis in the sediments can also increase porewater NH4+ concentration (Mazumdar et al., 2014), but our results from the species concentration profiles and reaction-transport modeling confirm that the effect of deep methanogenesis is very limited (Supplementary Table S5). In addition, the temperature can affect ion diffusion and microbial-mediated OM decomposition, leading to changes in porewater NH4+ concentration in depth profiles (Sivan et al., 2007; Zhang et al., 2021b), but the temperature variation is very small due to a relatively short sediment core (< 8.5 m) in our sampling sites. Thus, the influence of temperature on NH4+ concentration can be ignored.
As shown in Figures 10A and B, the cross-plots of SO42− vs. NH4+ display a significant negative relationship, indicating the OSR process is accompanied by OM decomposition and NH4+ release in the study area. By comparison, the slope of SO42-–NH4+ at site G01 (k = −0.033) is larger than those at all other sites (Figure 10), and the possible reason is due to the adsorption of NH4+ by clay minerals in the sediment at this site (Lukawska-Matuszewska and Kielczewska, 2016). Avinash et al. (2016) showed that the Arabian Sea sediments contain abundant illite with a large capacity for potassium-ammonium cation exchange, which originates from the fluvial or aeolian transport (wind-blown dust from the adjacent deserts). Therefore, we speculate that site G01 is more likely to receive abundant clay minerals like illite to absorb more NH4+ than other sites because it is located in a shallower water depth (844 m, Tabel 2). Although the OSR process occurs, our calculated flux of NH4+ (3.35 ~ 17.78 mmol/m2·yr) is much smaller than that of SO42- (77.82 ~ 101.38 mmol/m2·yr), indicating that OSR does not play a dominant role in porewater sulfate reduction.
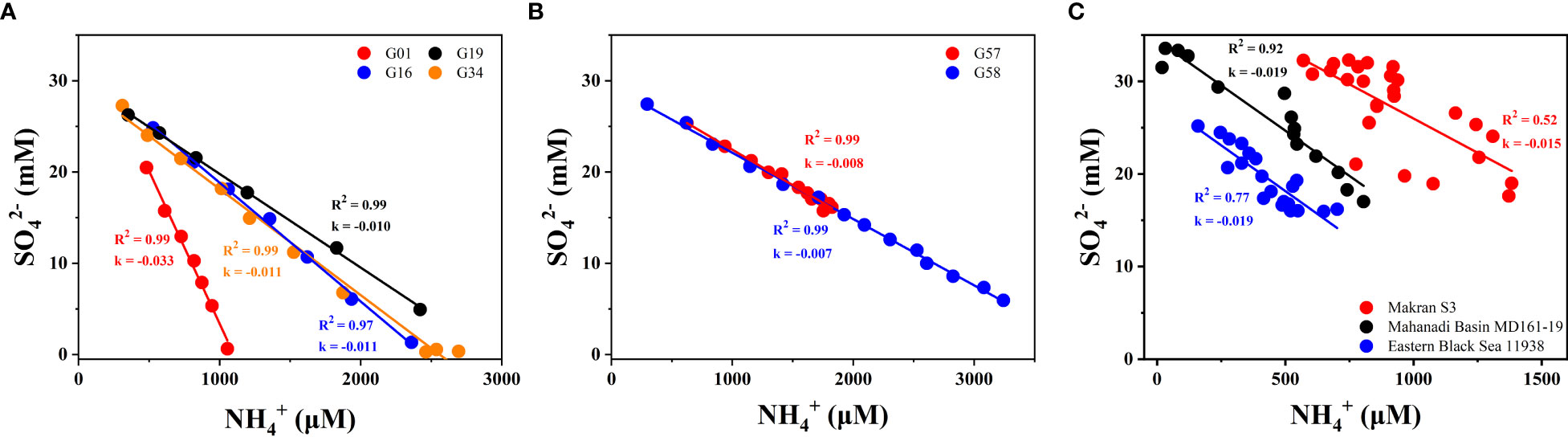
Figure 10 Cross-plot of porewater sulfate versus ammonium at the study sites. (A) sites G01, G16, G19, and G34 (Zone 1); (B) sites G57 and G58 (Zone 2); (C) different seepage sites in previous studies. The k represents the slope of SO42− - NH4+. The correlation coefficients are labeled close to the fitting lines, together with corresponding annotations. Data for Eastern Black Sea 11938 is cited from Reitz et al. (2011); Mahanadi Basin MD161-19 from Mazumdar et al. (2014); and Makran S3 from Zhang et al. (2021b).
Distinguishing the relative contributions of OSR and AOM to sulfate consumption is crucial to accessing methane flux into the SMT and the associated biogeochemical processes occurring in marine sediments (Malinverno and Pohlman, 2011; Luo et al., 2013). In the reaction of AOM, the molecule ratio of the sulfate consumption vs. DIC generation is 1:1 on the basis of the chemical reaction formula (Equation 1), while in the OSR process, the consumption of one molecule of sulfate produces two molecules of DIC (Equation 2). Therefore, the scatter plot of the flux of SO42− vs. DICnet can be used to estimate the relative contribution fractions of AOM and OSR to total porewater SO42− consumption (Smith and Coffin, 2014).
As shown in Figure 11, dots of Zone 1 (G01, G16, G19 and G34) in the methane-hydrate area fall near the AOM line, indicating the AOM reaction is the major process for sulfate reduction (Akam et al., 2020; Zhang et al., 2021b). In comparison, dots of G57 and G58 of Zone 2 in the background area are closer to the OSR line, thus proving that OSR is the dominant process for sulfate consumption. This is further supported by simulation results from the reaction-transport model, as the rates of AOM in Zone 1 are higher than those in Zone 2 (Figure 8; Supplementary Table S5).
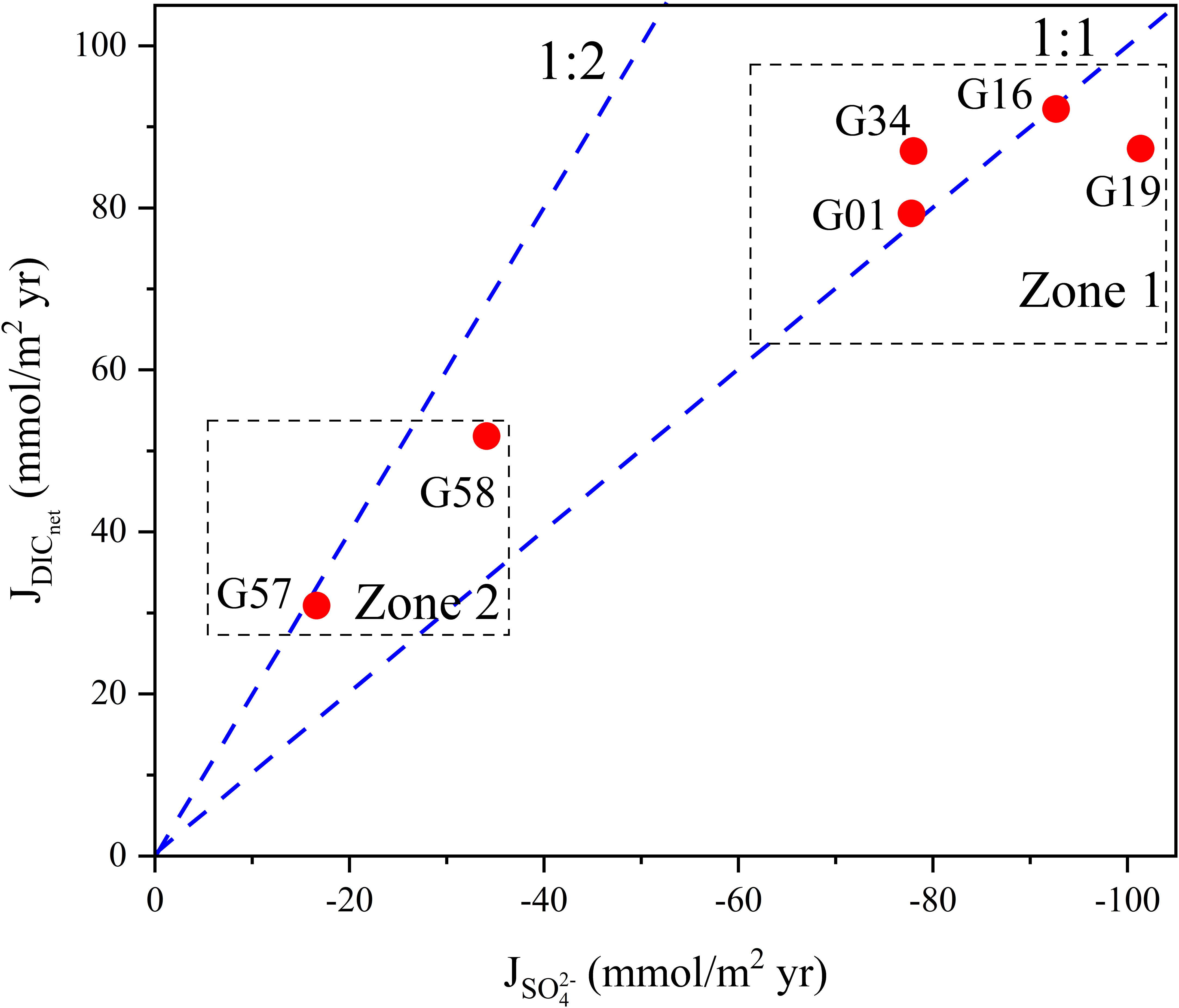
Figure 11 Scatter plot of flux of porewater SO42− vs. DICnet for the sites in Zone 1 and Zone 2 (Table 2). The 1:2 line indicates the molar stoichiometry of SO42− consumption to DIC production for OSR (Equation 2), and the 1:1 line indicates the molar stoichiometry of SO42− consumption to DIC production for AOM (Equation 1).
5.3 Sources of porewater DIC
Porewater DIC mainly originates from AOM, OSR and the seawater that trapped during sediment accumulation (e.g., Borowski et al., 2000; Chatterjee et al., 2011; Feng et al., 2018; Meister et al., 2019; Akam et al., 2020). In some cases, the incorporation of DIC from deep sediments may affect the δ13C-DIC within the SMT (e.g., Chatterjee et al., 2011; Hu et al., 2022; Zha et al., 2022), but based on the modeling results of methane turnovers, the calculating depth-integrated AOM rates at sites G01, G16, G19 and G34 are about 25 to 51 times of the in situ methanogenesis rates (Supplementary Table S6). Therefore, in situ methanogenesis constitutes a minor proportion of the total DIC, whose effect on deep DIC production can thus be ignored in this study.
Here, an isotope mass balance model (Equation 19) was used to distinguish the source of porewater DIC in the closed systems (Borowski et al., 2000; Chen et al., 2010; Zhang et al., 2021b):
where X is the proportion of DIC from different sources, and δ13C is the carbon isotope composition. The subscripts AOM, SW and OM refer to DIC derived from the sources listed above and the value of Xsw is obtained through dividing the DIC concentration of the overlying seawater by the DIC value within the SMT (Borowski et al., 2000). In this study, the average value of δ13C-TOC for each core sediment is taken as the δ13COM. The δ13C value of CH4 (−68.5 ‰) is followed as reported by Römer et al. (2012). The δ13CDIC-added is defined as the isotopic value of the DIC being added to the initial porewater since the burial, which can be obtained from the slope of the linear regression graph of DIC vs. δ13C-DIC*DIC (Ussler and Paull, 2008; Chen et al., 2010; Zhang et al., 2021b).
The δ13CDIC-added at sites G57 (−16.7 ‰) and G58 (−17.2 ‰) are more positive than δ13COM (Figure 12B), indicating that the porewater DIC in Zone 2 is less affected by AOM. In Zone 1, however, the relative contributions of the AOM process to total porewater DIC are 60% at site G01, 52% at site G16, 68% at site G19, and 25% at site G34 (Table 3). By comparison, the δ13C-DICadded value at site G34 (–31.6 ‰) is similar to that at site S3 in Makran from Zhang et al. (2021b) (–33.1 ‰) indicating that the two adjacent sites have the same DIC source (Figure 12A; Table 3). The difference in the percentage of porewater DIC sources at different sites is likely related to the flux of the upward diffusion of CH4 in the methane-hydrate area (Chuang et al., 2013; Wei et al., 2019; Feng et al., 2020).
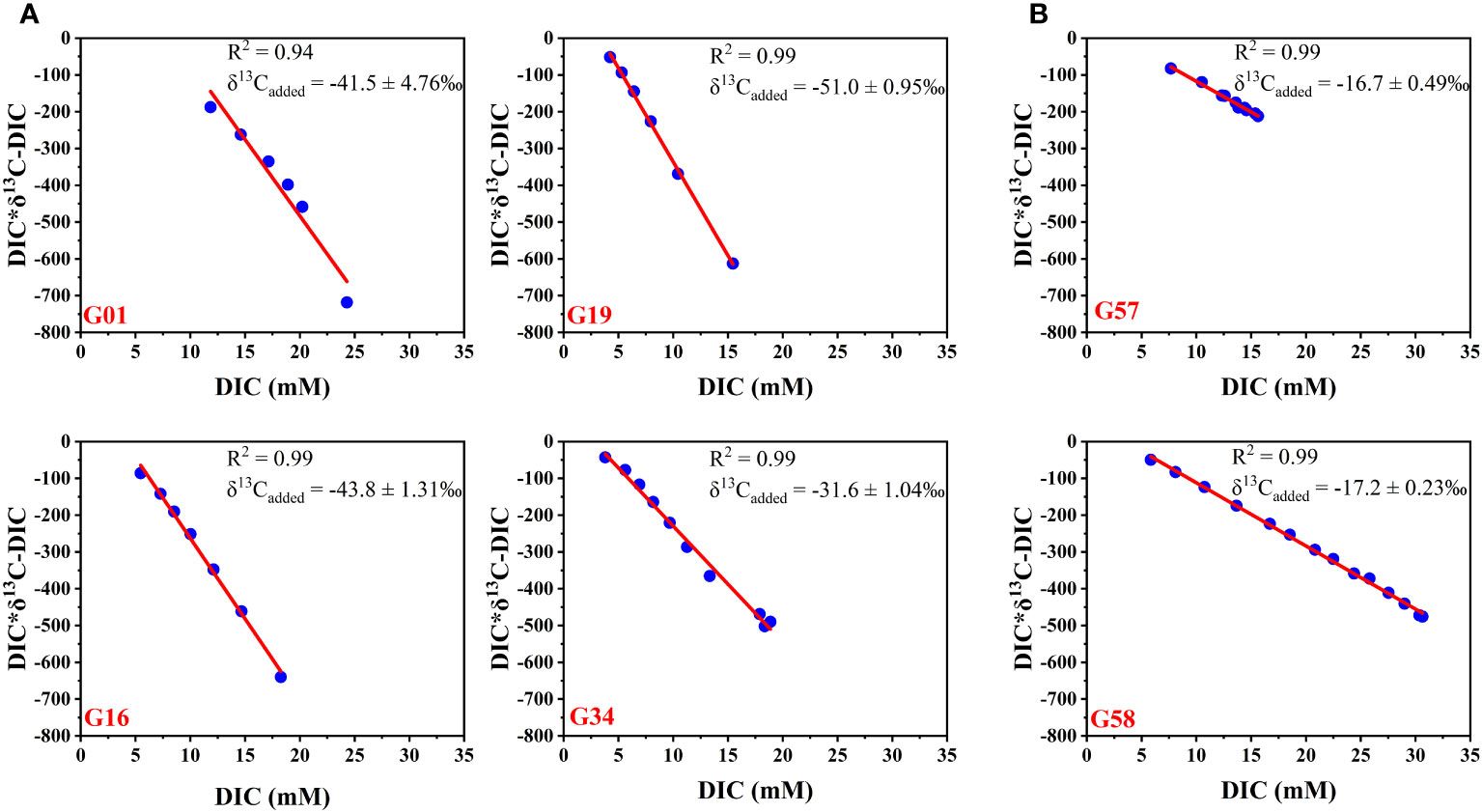
Figure 12 Linear regression diagrams of DIC vs δ13C-DIC*DIC at different sampling sites. (A) sites G01, G16, G19, and G34 (Zone 1); (B) sites G57 and G58 (Zone 2).
5.4 Removal of DIC by authigenic carbonate precipitation
The formation of authigenic carbonate is an important pathway to remove porewater DIC, particularly in continental shelf regions (e.g., Sun and Turchyn, 2014; Bradbury and Turchyn, 2019; Wang et al., 2019; Ruban et al., 2020; Loyd and Smirnoff, 2022). In this study, porewater Ca2+ and Mg2+ concentrations decrease with depth, suggesting the authigenic carbonate precipitation at sites in the study area (Ritger et al., 1987; Aloisi et al., 2002; Snyder et al., 2007; Nöthen and Kasten, 2011). Numerical simulation by the reaction-transport model shows that the rate of authigenic carbonate precipitation is 10 mmol/m2·yr at site G34, slightly higher than that at sites G01 (8 mmol/m2·yr), G16 (8 mmol/m2·yr), and G19 (7 mmol/m2·yr). The rates of authigenic carbonate precipitation at the sites in Zone 1 (average 8.25 mmol/m2·yr) are much higher than the global average (~5 mmol/m2·yr, Sun and Turchyn, 2014), emphasizing the significance of the methane hydrate area in the formation and burial of authigenic carbonate in global marine sediments. Compared with Zone 1, the rate of authigenic carbonates at sites G57 and G58 in the background area (Zone 2) is much lower (average 4 mmol/m2·yr).
The XRD analysis shows the mineral species of authigenic carbonate precipitation at sites G16, G34 and G58, which are mainly composed of calcite, aragonite, and dolomite (Figure 6; Supplementary Table S1). The molar fraction of MgCO3 in calcite can be obtained by lattice parameters of calcite (Titschack et al., 2011; Dos Santos et al., 2017; Fahad and Saeed, 2018). Based on the XRD/Rietveld analysis, we found that the molar fraction of MgCO3 in calcite is less than 10% at all sites in our study, indicating the absence of high-Mg calcite (Supplementary Table S6). In Zone 1, authigenic carbonate phases exhibit notable variations at different depths (Supplementary Table S1; Figure 6) of sites G16 and G34. At the surface sediments (55 cmbsf depth), calcite is the main component of carbonate phases, and aragonite is almost undetectable. However, the highest aragonite content occurs around the SMT with a depth of 415 cmbsf at site G16 (5.27%) and 535 cmbsf at site G34 (12.09%) (Supplementary Table S1), resulting in the highest ratio of aragonite/∑carbonate and the lowest ratio of calcite/∑carbonate appear at the SMT depth (Supplementary Table S2; Supplementary Figure S1). Many factors, such as sulfate inhibition and the presence of extracellular polymeric substances (EPS), can affect the formation of carbonate mineral phases (e.g., Formolo et al., 2004; Himmler et al., 2015; Li et al., 2018; Wang et al., 2019). Based on the results of porewater DIC and XRD analysis, however, we speculate that the solubility of carbonate minerals may play a significant role in the aragonite precipitation. According to carbonate solubility, calcite (K’= 7.98×10–7 at 5°C in 34.5‰ seawater) precipitation takes priority over aragonite (K’= 11.4×10–7 at 5°C in 34.5‰ seawater) (Berner, 1976). Therefore, at surface depth with relatively low DIC concentrations, calcite precipitates first. With the increase of DIC generated by the microbial-mediated AOM occurring around SMT depth (Chafetz et al., 1991; Peckmann et al., 2001; Liu et al., 2016), aragonite starts to precipitate, leading to the highest ratio of aragonite/∑carbonate around the SMT depth compared to other depths. Nevertheless, this ratio remains lower than that of calcite/∑carbonate at all depths. By contrast, aragonite does not appear while calcite is the dominant carbonate phase in Zone 2 (site G58) (Supplementary Tables S1, S2), which is similar to the distribution of carbonate phases in surface sediments of Zone 1, as it is less affected by AOM (Liu et al., 2016; Li et al., 2018; Wei et al., 2020). Although dolomite is one of the carbonate phases in the study area, its content is relatively low (Zone 1: 2.73% ~ 4.47% at site G16; 2.47% ~ 4.52% at site G34; Zone 2: 2.52% ~ 3.10% at site G58) (Supplementary Table S1). Currently, the formation mechanism of dolomite is very complex and remains elusive (Cai et al., 2021). Microbially-mediated dolomite precipitation could possibly occur in modern reductive saline and anoxic environments (Warren, 2000; Cai et al., 2021), such as lagoons and gas hydrate areas (e.g., Casado et al., 2014; Li et al., 2018). Therefore, we infer that it is possible in the past that the accumulation of gas hydrates resulted in saline, sulfate-free porewaters which could host the small amounts of dolomite that we observed in this study.
5.5 Release of DIC to the overlying seawater
Theoretically, DIC that is not precipitated into authigenic carbonates can enter the upper sediment and eventually the overlying seawater through advection or diffusion (Chatterjee et al., 2011; Rassmann et al., 2018; Akam et al., 2020; Zhao et al., 2020). Based on extensive seafloor observations and geochemical analysis, methane hydrate systems contribute considerable proportions of the local carbon budget to the overlying seawater by emitting a large amount of DIC (Aharon et al., 1992; Suess et al., 1999; Garcia-Tigreros and Kessler, 2018; Feng et al., 2020). DIC released from marine sediments has an important influence on the carbonate equilibrium system in seawater (Chen, 2002; Krumins et al., 2013; Akam et al., 2020; Feng et al., 2020). Previous studies have shown that global DIC flux released from sediments to overlying seawater averages 6.5 Tmol/yr (3.2 ~ 9.2 Tmol/yr), of which 98% is released from continental margin sediments with the depth of SMT less than 13 m, equivalent to approximately 20% of the global river DIC (~ 33 Tmol/yr) flowing into the ocean (Meybeck, 1993; Amiotte Suchet et al., 2003; Treude et al., 2005; Aufdenkampe et al., 2011; Egger et al., 2018; Akam et al., 2020).
In this study, we use the reaction-transport model to quantitatively assess the DIC fluxes released from the sediments into the overlying seawater of the Makran continental margin (Table 4). In this calculation, DICOSR, DICAOM and DICcarbonate are simulated by the rate of OM decomposition, the rate of AOM within SMT, and the depth profile of Ca2+ concentration, respectively. As described previously, the situ methanogenesis via shallow OM decomposition is very low (Supplementary Table S5), so the contribution of DIC generated through this process is not considered. The flux of DICburial is constrained by the sedimentation rate and DIC concentration (Zha et al., 2022), thus it can be estimated by the reaction-transport model (Table 4). Furthermore, the DIC released from sediment to overlying seawater can be defined as Equation 20 (Wallmann et al., 2008; Dickens and Snyder, 2009; Chatterjee et al., 2011; Solomon et al., 2014; Akam et al., 2020):
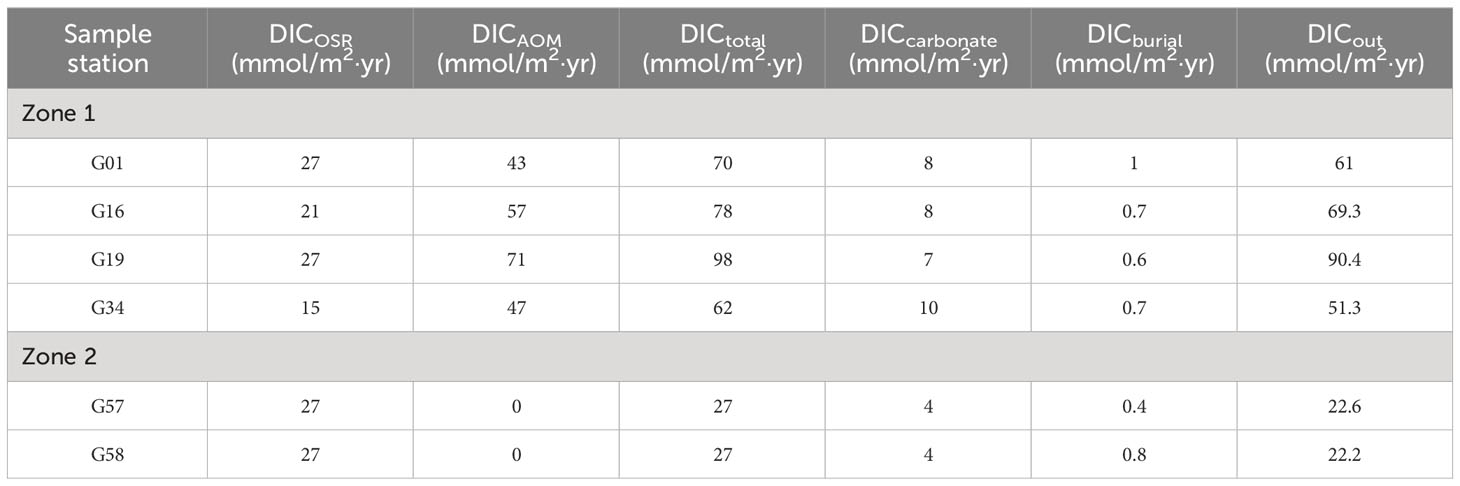
Table 4 The calculated DIC fluxes of the Makran continental margin by simulation using the reaction-transport model.
Based on the Equation 20, we find that DICout differs greatly between the site G19 (90.4 mmol/m2·yr) and G34 (51.3 mmol/m2·yr), although both sites are located in the methane-hydrate area (Figure 1). In addition, we compared the DICout values in Zone 1 (68 ± 14 mmol/m2·yr) and Zone 2 (22.4 mmol/m2·yr), and this difference is likely caused by the different DIC cycling patterns between the two Zones (Table 4; Figure 13). As shown in Table 4 and Figure 13, the DIC fluxes produced by AOM in Zone 1 is 55 ± 11 mmol/m2·yr, which is significantly higher than that in Zone 2 (DICAOM = 0). Although the rate of authigenic carbonate formation is also relatively high in Zone 1, the process of carbonate precipitation is insufficient to prevent the release of large amounts of DIC from the sediment into the overlying seawater. By comparison, the fluxes of DIC released from sediments range from 51.3 to 90.4 mmol/m2·yr in Zone 1, which is higher than those of other methane-hydrate areas, such as the Gulf of Mexico (9 ~ 33 mmol/m2·yr, Smith and Coffin, 2014), Dongsha area of the southern South China Sea (13.1 ~ 26.1 mmol/m2·yr, Chen et al., 2017), Shenhu area of the southern South China Sea (10.1 ~ 31.7 mmol/m2·yr, Wu et al., 2013), and Beikang Basin (32.3 ~ 50.1 mmol/m2·yr, Feng et al., 2018).
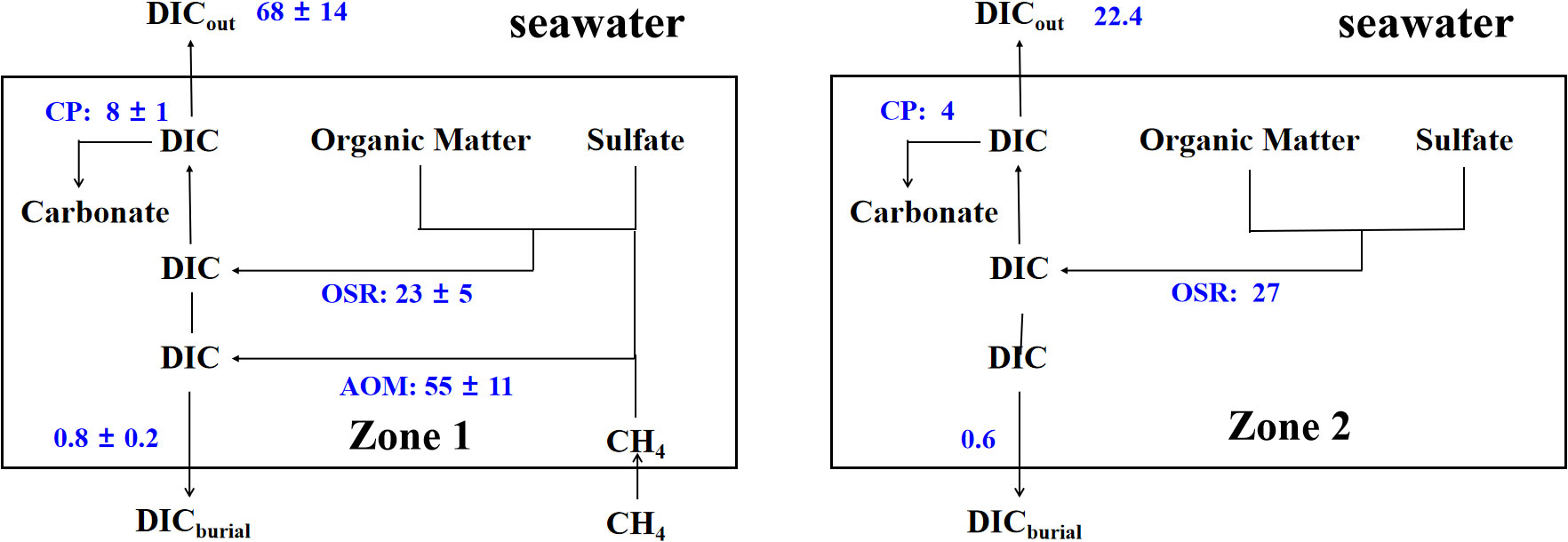
Figure 13 Two simplified schematics of the DIC release to the overlying seawater in Zone 1 and Zone 2. CP is the abbreviation for carbonate precipitation. The numbers represent the average flux and depth-integrated rates of DIC production and consumption in mmol/m2·yr of DIC.
This upward DIC flux across the seafloor accounts for an average of 88% of the total DIC in Zone 1. Importantly, the process of AOM contributed a large proportion of DICout at sites G01, G16, G19, and G34 in Zone 1 (Table 4) due to the upward flux of CH4. High rates of AOM can lead to an increase in DICout flux and total alkalinity (TA) in the overlying seawater (Akam et al., 2020; Zha et al., 2022), which is beneficial to reduce ocean acidification and increases the CO2 absorption capacity of seawater (Chen, 2002; Krumins et al., 2013; Akam et al., 2020; Feng et al., 2020). In future, more related work on carbon cycling in methane-bearing sediments should be conducted to quantitatively assess the DIC and TA released from global marine sediments to the overlying seawater and their impact on carbonate equilibrium in marine systems.
6 Conclusion
Based on the active density of flares at the Makran continental margin, we divided the study area into Zone 1 (an active accretionary environment with high methane flux) and Zone 2 (a farther offshore background area). The differences in geological structures and sampling locations between the two zones have a potential impact on the porewater geochemistry and DIC flux. Our results show that Zone 1 has the larger CH4 flux with the shallower SMT depth, and a noticeable increase in porewater Ba2+ concentration was observed within or near the SMT zone. The flux of SO42− vs. DICnet was used to determine the pathway of sulfate consumption at each site, revealing that almost all porewater SO42− at sites G57 and G58 in Zone 2 is consumed by OSR, the AOM reaction, however, is the main process of sulfate consumption affected by methane diffusion at sites in Zone 1. The relative contributions of AOM to the porewater DIC calculated by the isotope mass balance model are 60% (G01) and 52% (G16), 68% (G19), and 25% (G34) in Zone 1, respectively. The TIC, XRD and SEM analyses show that the total carbonate content increases with depth, and the carbonate phases are composed of calcite, aragonite, and dolomite. By comparison, calcite is the main carbonate phase while the dolomite content is relatively low. Due to high concentration of DIC induced by AOM, aragonite appears at or below the depth of SMT. The reaction-transport model results and calculation reveal that the flux of DIC released from sediments is 51.3 to 90.4 mmol/m2·yr in Zone 1, which is significantly higher than that in Zone 2 (22.4 mmol/m2·yr). Overall, this study highlights that the methane-related carbon pools are highly heterogeneous in the methane-hydrate area, and the contribution of AOM is essential to the authigenic carbonate burial and the bottom seawater chemistry.
Data availability statement
The raw data supporting the conclusions of this article will be made available by the authors, without undue reservation.
Author contributions
YC: Investigation, Formal analysis, Writing - Original Draft. SX: Formal analysis. WL: Investigation. JL: Resources, Investigation. ZZ: Investigation. TY: Investigation. XX: Investigation. XD: Investigation. HY: Resources, Investigation, Conceptualization, Methodology. ZW: Conceptualization, Methodology, Writing - Review & Editing. All authors contributed to the article and approved the submitted version.
Funding
The author(s) declare financial support was received for the research, authorship, and/or publication of this article. This study was funded by the Development Program of China (No. 2022YFC2805400) and National Natural Science Foundation of China (No. 41976057, 42276059).
Acknowledgments
We thank reviewers and Editor Glen T. Snyder for their constructive comments and suggestions, which greatly helped us improve our manuscript. We also thank Dr. Zhe Zhou (Tongji University) for constructive comments on manuscript writing.
Conflict of interest
The authors declare that the research was conducted in the absence of any commercial or financial relationships that could be construed as a potential conflict of interest.
Publisher’s note
All claims expressed in this article are solely those of the authors and do not necessarily represent those of their affiliated organizations, or those of the publisher, the editors and the reviewers. Any product that may be evaluated in this article, or claim that may be made by its manufacturer, is not guaranteed or endorsed by the publisher.
Supplementary material
The Supplementary Material for this article can be found online at: https://www.frontiersin.org/articles/10.3389/fmars.2023.1181921/full#supplementary-material
References
Aharon P., Graber E. R., Roberts H. H. (1992). Dissolved carbon and δ13C anomalies in the water column caused by hydrocarbon seeps on the northwestern Gulf of Mexico slope. Geo-Marine Lett. 12, 33–40. doi: 10.1007/BF02092106
Akam S. A., Coffin R. B., Abdulla H. A. N., Lyons T. W. (2020). Dissolved inorganic carbon pump in methane-charged shallow marine sediments: state of the art and new model perspectives. Front. Mar. Sci. 7. doi: 10.3389/fmars.2020.00206
Aller R. C., Blair N. E. (2004). Early diagenetic remineralization of sedimentary organic C in the Gulf of Papua deltaic complex (Papua New Guinea): Net loss of terrestrial C and diagenetic fractionation of C isotopes. Geochim. Cosmochim. Acta 68, 1815–1825. doi: 10.1016/j.gca.2003.10.028
Aloisi G., Bouloubassi I., Heijs S., Pancost R. D., Pierre C., Sinninghe Damsteé J. S., et al. (2002). CH4-consuming microorganisms and the formation of carbonate crusts at cold seeps. Earth Planet. Sci. Lett. 203, 195–203. doi: 10.1016/S0012-821X(02)00878-6
Aloisi G., Wallmann K., Bollwerk S. M., Derkachev A., Bohrmann G., Suess E. (2004). The effect of dissolved barium on biogeochemical processes at cold seeps. Geochim. Cosmochim. Acta 68 (8), 1735–1748. doi: 10.1016/j.gca.2003.10.010
Amiotte Suchet P., Probst J.-L., Ludwig W. (2003). Worldwide distribution of continental rock lithology: Implications for the atmospheric/soil CO2 uptake by continental weathering and alkalinity river transport to the oceans. Global Biogeochem. Cycles 17 (2), 1038. doi: 10.1029/2002gb001891
Arndt S., Jørgensen B. B., LaRowe D. E., Middelburg J., Pancost R., Regnier P. (2013). Quantifying the degradation of organic matter in marine sediments: a review and synthesis. Earth-sci. Rev. 123, 53–86. doi: 10.1016/j.earscirev.2013.02.008
Aufdenkampe A. K., Mayorga E., Raymond P. A., Melack J. M., Doney S. C., Alin S. R., et al. (2011). Riverine coupling of biogeochemical cycles between land, oceans, and atmosphere. Front. Ecol. Environ. 9, 53–60. doi: 10.1890/100014
Avinash K., Kurian P. J., Warrier A. K., Shankar R., Vineesh T. C., Ravindra R. (2016). Sedimentary sources and processes in the eastern Arabian Sea: Insights from environmental magnetism, geochemistry and clay mineralogy. Geosci. Front. 7, 253–264. doi: 10.1016/j.gsf.2015.05.001
Berg R. D. (2008). Diffusional methane fluxes within continental margin sediments and depositional constraints on formation factor estimates, (University of California, San Diego).
Berner R. A. (1976). The solubility of calcite and aragonite in seawater at atmospheric pressure and 34.5‰ salinity. Am. J. Sci. 276 (6), 713–730. doi: 10.2475/ajs.276.6.713
Berner R. A. (1978). Sulfate reduction and the rate of deposition of marine sediments. Earth Planet. Sci. Lett. 37 (3), 492–498. doi: 10.1016/0012-821x(78)90209-1
Berner R. A. (1980). Early Diagenesis: A Theoretical Approach (No.1). (Princeton University Press). doi: 10.1515/9780691209401
Boetius A., Ravenschlag K., Schubert C. J., Rickert D., Widdel F., Gleeske A., et al. (2000). A marine microbial consortium apparently mediating AOM. Nature 407, 623–626. doi: 10.1038/35036572
Borowski W. S., Hoehler T. M., Alperin M. J., Rodriguez N. M., Paull C. K. (2000). Significance of anaerobic methane oxidation in methane-rich sediments overlying the Blake Ridge gas hydrates. Proc. Ocean Drill. Progr. Sci. Results 164, 87–99. doi: 10.2973/odp.proc.sr.164.214.2000
Borowski W. S., Paull C. K., Ussler W. (1996). Marine pore-water sulfate profiles indicate in situ methane flux from underlying gas hydrate. Geology 24, 655–658. doi: 10.1130/0091-7613(1996)024<0655:MPWSPI>2.3.CO;2
Borowski W. S., Paull C. K., Ussler W. (1999). Global and local variations of interstitial sulfate gradients in deep-water, continental margin sediments: Sensitivity to underlying methane and gas hydrates. Mar. Geol. 159, 131–154. doi: 10.1016/S0025-3227(99)00004-3
Boudreau B. P., Ruddick B. R. (1991). On a reactive continuum representation of organic matter diagenesis. Am. J. Sci. 291, 507–538. doi: 10.2475/ajs.291.5.507
Bradbury H. J., Turchyn A. V. (2019). Reevaluating the carbon sink due to sedimentary carbonate formation in modern marine sediments. Earth Planet. Sci. Lett. 519, 40–49. doi: 10.1016/j.epsl.2019.04.044
Burns S. J. (1998). Carbon isotopic evidence for coupled sulfate reduction-methane oxidation in Amazon Fan sediments. Geochim. Cosmochim. Acta 62, 797–804. doi: 10.1016/S0016-7037(98)00035-0
Cai W. K., Liu J. H., Zhou C. H., Keeling J., Glasmacher U. A. (2021). Structure, genesis and resources efficiency of dolomite: New insights and remaining enigmas. Chem. Geol. 573, 120191. doi: 10.1016/j.chemgeo.2021.120191
Casado A. I., Alonso-Zarza A. M., La Iglesia Á. (2014). Morphology and origin of dolomite in paleosols and lacustrine sequences. Examples from the Miocene of the Madrid Basin. Sediment. Geol. 312, 50–62. doi: 10.1016/j.sedgeo.2014.07.005
Chafetz H., Rush P. F., Utech N. M. (1991). Microenvironmental controls on mineralogy and habit of CaCO3 precipitates: an example from an active travertine system. Sedimentology 38, 107–126. doi: 10.1111/j.1365-3091.1991.tb01857.x
Chatterjee S., Dickens G. R., Bhatnagar G., Chapman W. G., Dugan B., Snyder G. T., et al. (2011). Pore water sulfate, alkalinity, and carbon isotope profiles in shallow sediment above marine gas hydrate systems: A numerical modeling perspective. J. Geophys. Res. Solid Earth 116, 1–25. doi: 10.1029/2011JB008290
Chen C. T. A. (2002). Shelf-vs. dissolution-generated alkalinity above the chemical lysocline. Deep. Res. Part II Top. Stud. Oceanogr. 49 (24-25), 5365–5375. doi: 10.1016/S0967-0645(02)00196-0
Chen Y., Shen L., Huang T., Chu Z., Xie Z. (2020). Transformation of sulfur species in lake sediments at Ardley Island and Fildes Peninsula, King George Island, Antarctic Peninsula. Sci. Total Environ. 703, 135591. doi: 10.1016/j.scitotenv.2019.135591
Chen T., Sun X., Lin Z., Lu Y., Fang Y., Wu Z., et al. (2021). Deciphering the geochemical link between seep carbonates and enclosed pyrite: A case study from the northern South China sea. Mar. Pet. Geol. 128, 105020. doi: 10.1016/j.marpetgeo.2021.105020
Chen Y., Ussler W., Haflidason H., Lepland A., Rise L., Hovland M., et al. (2010). Sources of methane inferred from pore-water δ13C of dissolved inorganic carbon in Pockmark G11, offshore Mid-Norway. Chem. Geol. 275, 127–138. doi: 10.1016/j.chemgeo.2010.04.013
Chen N. C., Yang T. F., Hong W. L., Chen H. W., Chen H. C., Hu C. Y., et al. (2017). Production, consumption, and migration of methane in accretionary prism of southwestern Taiwan. Geochem. Geophys. Geosy. 18, 2970–2989. doi: 10.1002/2017GC006798
Chen N., Yang T. F., Liou Y., Lin H. T., Hong W., Lin S., et al. (2023). Controlling factors on patterns of dissolved organic carbon and volatile fatty acids in a submarine mud volcano offshore southwestern Taiwan. Front. Earth Sci. 11. doi: 10.3389/feart.2023.1210088
Chuang P. C., Dale A. W., Wallmann K., Haeckel M., Yang T. F., Chen N. C., et al. (2013). Relating sulfate and methane dynamics to geology: Accretionary prism offshore SW Taiwan. Geochem. Geophys. Geosy. 14, 2523–2545. doi: 10.1002/ggge.20168
Chuang P. C., Yang T. F., Wallmann K., Matsumoto R., Hu C. Y., Chen H. W., et al. (2019). Carbon isotope exchange during anaerobic oxidation of methane (AOM) in sediments of the northeastern South China Sea. Geochim. Cosmochim. Acta 246, 138–155. doi: 10.1016/j.gca.2018.11.003
Chuang P. C., Young M. B., Dale A. W., Miller L. G., Herrera-Silveira J. A., Paytan A. (2016). Methane and sulfate dynamics in sediments from mangrove-dominated tropical coastal lagoons, Yucatan, Mexico. Biogeosciences 13, 2981–3001. doi: 10.5194/bg-13-2981-2016
Claypool G. E., Milkov A. V., Lee Y. J., Torres M. E., Borowski W. S., Tomaru H. (2006). “Microbial Methane Generation and Gas Transport in Shallow Sediments of an Accretionary Complex, Southern Hydrate Ridge (ODP Leg 204), Offshore Oregon USA,” in Proceedings of the ODP, Scientific Results. Eds. Trehu A. M., Bohrmann G., Torres M. E., Colwell F. S. (College Station, Texas: Ocean Drilling Program).
Coffin R. B., Hamdan L. J., Smith J. P., Rose P. S., Plummer R. E., Yoza B., et al. (2014). Contribution of vertical methane flux to shallow sediment carbon pools across Porangahau Ridge, New Zealand. Energies 7, 5332–5356. doi: 10.3390/en7085332
Coffin R. B., Osburn C. L., Plummer R. E., Smith J. P., Rose P. S., Grabowski K. S. (2015). Deep sediment-sourced methane contribution to shallow sediment organic carbon: Atwater Valley, Texas-Louisiana Shelf, Gulf of Mexico. Energies 8, 1561–1583. doi: 10.3390/en8031561
Coffin R. B., Smith J. P., Plummer R. E., Yoza B., Larsen R. K., Millholland L. C., et al. (2013). Spatial variation in shallow sediment methane sources and cycling on the Alaskan Beaufort Sea Shelf/Slope. Mar. Pet. Geol. 45, 186–197. doi: 10.1016/j.marpetgeo.2013.05.002
D'Hondt S., Rutherford S., Spivack A. J. (2002). Metabolic activity of subsurface life in deep-sea sediments. Science 295 (5562), 2067–2070. doi: 10.1126/science.1064878
Dale A. W., Flury S., Fossing H., Regnier P., Røy H., Scholze C., et al. (2019). Kinetics of organic carbon mineralization and methane formation in marine sediments (Aarhus Bay, Denmark). Geochim. Cosmochim. Acta 252, 159–178. doi: 10.1016/j.gca.2019.02.033
Delisle G., Berner U. (2002). Gas hydrates acting as cap rock to fluid discharge in the Makran accretionary prism? Geol. Soc Spec. Publ. 195, 137–146. doi: 10.1144/GSL.SP.2002.195.01.09
Dickens G. R., Snyder G. T. (2009). Interpreting upward methane flux from marine pore water profiles. Fire Ice 9 (1), 7–10. Available at: https://netl.doe.gov/sites/default/files/publication/MHNewsWinter09.pdf.
Doebelin N., Kleeberg R. (2015). Profex: A graphical user interface for the Rietveld refinement program BGMN. J. Appl. Crystallogr. 48, 1573–1580. doi: 10.1107/S1600576715014685
Dos Santos H. N., Neumann R., Ávila C. A. (2017). Mineral quantification with simultaneous refinement of Ca-Mg carbonates non-stoichiometry by X-Ray diffraction, rietveld method. Minerals 7, 164. doi: 10.3390/min7090164
Egger M., Riedinger N., Mogollón J. M., Jørgensen B. B. (2018). Global diffusive fluxes of methane in marine sediments. Nat. Geosci. 11, 421–425. doi: 10.1038/s41561-018-0122-8
Fahad M., Saeed S. (2018). Determination and estimation of magnesium content in the single phase magnesium-calcite [Ca(1–x)MgxCO3(s)] using electron probe micro-analysis (EPMA) and X-ray diffraction (XRD). Geosci. J. 22, 303–312. doi: 10.1007/s12303-017-0059-8
Fantle M. S., DePaolo D. J. (2006). S.R.X.X.X isotopes and pore fluid chemistry in carbonate sediment of the Ontong Java Plateau: Calcite recrystallization rates and evidence for a rapid rise in seawater Mg over the last 10 million years. Geochim. Cosmochi. Acta 70, 3883–3904. doi: 10.1016/j.gca.2006.06.009
Fantle M. S., DePaolo D. J. (2007). Ca isotopes in carbonate sediment and pore fluid from ODP Site 807A: The Ca2+ (aq)–calcite equilibrium fractionation factor and calcite recrystallization rates in Pleistocene sediments. Geochim. Cosmochi. Acta 71, 2524–2546. doi: 10.1016/j.gca.2007.03.006
Feng J., Li N., Luo M., Liang J., Yang S., Wang H., et al. (2020). A quantitative assessment of methane-derived carbon cycling at the cold seeps in the northwestern south China sea. Minerals 10, 1–23. doi: 10.3390/min10030256
Feng D., Peng Y., Bao H., Peckmann J., Roberts H. H., Chen D. (2016). A carbonate-based proxy for sulfate-driven anaerobic oxidation of methane. Geology 44, 999–1002. doi: 10.1130/G38233.1
Feng J., Yang S., Liang J., Fang Y., He Y., Luo M., et al. (2018). Methane seepage inferred from the porewater geochemistry of shallow sediments in the Beikang Basin of the southern South China Sea. J. Asian Earth Sci. 168, 77–86. doi: 10.1016/j.jseaes.2018.02.005
Fischer D., Sahling H., Nöthen K., Bohrmann G., Zabel M., Kasten S. (2012). Interaction between hydrocarbon seepage, chemosynthetic communities, and bottom water redox at cold seeps of the Makran accretionary prism: Insights from habitat-specific pore water sampling and modeling. Biogeosciences 9, 2013–2031. doi: 10.5194/bg-9-2013-2012
Flood R. D., Piper D. J. W., Klaus A., Scientific Research Party. (1995). Proceedings of the Ocean Drilling Program, Initial Reports. (College Station, TX: Ocean Drilling Program), 155.
Formolo M. J., Lyons T. W., Zhang C., Kelley C., Sassen R., Horita J., et al. (2004). Quantifying carbon sources in the formation of authigenic carbonates at gas hydrate sites in the Gulf of Mexico. Chem. Geol. 205, 253–264. doi: 10.1016/j.chemgeo.2003.12.021
Garcia-Tigreros F., Kessler J. D. (2018). Limited acute influence of aerobic methane oxidation on ocean carbon dioxide and pH in hudson canyon, northern U.S. Atlantic margin. J. Geophys. Res. Biogeosciences 123, 2135–2144. doi: 10.1029/2018JG004384
Geprägs P., Torres M. E., Mau S., Kasten S., Römer M., Bohrmann G. (2016). Carbon cycling fed by methane seepage at the shallow Cumberland Bay, South Georgia, sub-Antarctic. Geochem. Geophys. Geosy. 17, 1401–1418. doi: 10.1002/2016GC006276
Grando G., McClay K. (2007). Morphotectonics domains and structural styles in the Makran accretionary prism, offshore Iran. Sediment. Geol. 196, 157–179. doi: 10.1016/j.sedgeo.2006.05.030
Graves C. A., Steinle L., Rehder G., Niemann H., Connelly D. P., Lowry D., et al. (2015). Fluxes and fate of dissolved methane released at the seafloor at the landward limit of the gas hydrate stability zone offshore western Svalbard. J. Geophys. Res-Oceans 120 (9), 6185–6201. doi: 10.1016/S0016-7037(03)00127-3
Haas A., Peckmann J., Elvert M., Sahling H., Bohrmann G. (2010). Patterns of carbonate authigenesis at the Kouilou pockmarks on the Congo deep-sea fan. Mar. Geol. 268, 129–136. doi: 10.1016/j.margeo.2009.10.027
Haese R. R., Meile C., Van Cappellen P., De Lange G. J. (2003). Carbon geochemistry of cold seeps: Methane fluxes and transformation in sediments from Kazan mud volcano, eastern Mediterranean Sea. Earth Planet. Sci. Lett. 212, 361–375. doi: 10.1016/S0012-821X(03)00226-7
Hall P. O. J., Aller R. C. (1992). Rapid, small-volume, flow injection analysis for ∑CO2, and NH4+ in marine and freshwaters. Limnol. Oceanogr. 37, 1113–1119. doi: 10.4319/lo.1992.37.5.1113
Hamdan L. J., Gillevet P. M., Pohlman J. W., Sikaroodi M., Greinert J., Coffin R. B. (2011). Diversity and biogeochemical structuring of bacterial communities across the Porangahau ridge accretionary prism, New Zealand. FEMS Microbiol. Ecol. 77, 518–532. doi: 10.1111/j.1574-6941.2011.01133.x
Himmler T., Birgel D., Bayon G., Pape T., Ge L., Bohrmann G., et al. (2015). Formation of seep carbonates along the Makran convergent margin, northern Arabian Sea and a molecular and isotopic approach to constrain the carbon isotopic composition of parent methane. Chem. Geol. 415, 102–117. doi: 10.1016/j.chemgeo.2015.09.016
Hu Y., Luo M., Chen L., Liang Q., Feng D., Tao J., et al. (2018). Methane source linked to gas hydrate system at hydrate drilling areas of the South China Sea: Porewater geochemistry and numerical model constraints. J. Asian Earth Sci. 168, 87–95. doi: 10.1016/j.jseaes.2018.04.028
Hu Y., Zhang X., Feng D., Peckmann J., Feng J., Wang H., et al. (2022). Enhanced sulfate consumption fueled by deep-sourced methane in a hydrate-bearing area. Sci. Bull. 67, 122–124. doi: 10.1016/j.scib.2021.09.006
Jørgensen B. B., Beulig F., Egger M., Petro C., Scholze C., Røy H. (2019). Organoclastic sulfate reduction in the sulfate-methane transition of marine sediments. Geochim. Cosmochim. Acta 254, 231–245. doi: 10.1016/j.gca.2019.03.016
Jørgensen B. B., Weber A., Zopfi J. (2001). Sulphate reduction and anaerobic oxidation in Black Sea sediments. Deep. Res. I 48, 2097–2120. doi: 10.1016/s0967-0637(01)00007-3
Kastner M., Claypool G., Robertson G. (2008). Geochemical constraints on the origin of the pore fluids and gas hydrate distribution at Atwater Valley and Keathley Canyon, northern Gulf of Mexico. Mar. Pet. Geol. 25, 860–872. doi: 10.1016/j.marpetgeo.2008.01.022
Keigwin L. D., Rio D., Acton G. D., Shipboard Scientific Party. (1998). Proceedings of the Ocean Drilling Program, Initial Reports. (College Station, TX: Ocean Drilling Program), 172.
Khan M. J., Ali M., Xu M., Khan M. (2020). Seismicity analysis of selected faults in Makran Southern Pakistan. Acta Geophys. 68, 965–978. doi: 10.1007/s11600-020-00447-8
Kimura G., Silver E. A., Blum P., Shipboard Scientific Party. (1997). Proceedings of the Ocean Drilling Program, Initial Reports, vol. 170.
Knittel K., Boetius A. (2009). Anaerobic oxidation of methane: Progress with an unknown process. Annu. Rev. Microbiol. 63, 311–334. doi: 10.1146/annurev.micro.61.080706.093130
Kopp C., Fruehn J., Flueh E. R., Reichert C., Kukowski N., Bialas J., et al. (2000). Structure of the makran subduction zone from wide-angle and reflection seismic data. Tectonophysics 329, 171–191. doi: 10.1016/S0040-1951(00)00195-5
Kretschmer K., Biastoch A., Rüpke L., Burwicz E. (2015). Modeling the fate of methane hydrates under global warming. Global Biogeochem. Cycles 29, 610–625. doi: 10.1002/2014GB005011
Krumins V., Gehlen M., Arndt S., Van Cappellen P., Regnier P. (2013). Dissolved inorganic carbon and alkalinity fluxes from coastal marine sediments: Model estimates for different shelf environments and sensitivity to global change. Biogeosciences 10, 371–398. doi: 10.5194/bg-10-371-2013
Kvenvolden K. A. (1988). Methane hydrate - A major reservoir of carbon in the shallow geosphere? Chem. Geol. 71, 41–51. doi: 10.1016/0009-2541(88)90104-0
Lerche I. (2000). Estimates of worldwide gas hydrate resources. Energy Explor. Exploit. 18, 329–337. doi: 10.1260/0144598001492157
Li N., Jin M., Peckmann J., Chen D., Feng D. (2023). Quantification of the sources of sedimentary organic carbon at methane seeps: A case study from the South China Sea. Chem. Geol. 627, 121463. doi: 10.1016/j.chemgeo.2023.121463
Li J., Peng X., Bai S., Chen Z., Van Nostrand J. D. (2018). Biogeochemical processes controlling authigenic carbonate formation within the sediment column from the Okinawa Trough. Geochim. Cosmochim. Acta 222, 363–382. doi: 10.1016/j.gca.2017.10.029
Liao J., Liu X., Zhao Q., Gong J., Yin W., Li S., et al. (2022). Characteristics of high saturation hydrate reservoirs in the low-angle subduction area of the makran accretionary prism. Front. Earth Sci. 10. doi: 10.3389/feart.2022.861162
Liu S., Feng X., Feng Z., Xiao X., Feng L. (2020b). Geochemical evidence of methane seepage in the sediments of the Qiongdongnan Basin, South China Sea. Chem. Geol. 543, 119588. doi: 10.1016/j.chemgeo.2020.119588
Liu W., Wu Z., Xu S., Wei J., Peng X., Li J., et al. (2020a). Pore-water dissolved inorganic carbon sources and cycling in the shallow sediments of the Haima cold seeps, South China Sea. J. Asian Earth Sci. 201, 104495. doi: 10.1016/j.jseaes.2020.104495
Liu X., Xu T., Tian H., Wei M., Jin G., Liu N. (2016). Numerical modeling study of mineralization induced by methane cold seep at the sea bottom. Mar. Pet. Geol. 75, 14–28. doi: 10.1016/j.marpetgeo.2016.04.010
Loyd S. J., Smirnoff M. N. (2022). Progressive formation of authigenic carbonate with depth in siliciclastic marine sediments including substantial formation in sediments experiencing methanogenesis. Chem. Geol. 594, 120775. doi: 10.1016/j.chemgeo.2022.120775
Luff R., Haeckel M., Wallmann K. (2001). Robust and fast FORTRAN and MATLAB® libraries to calculate pH distributions in marine systems. Comput. Geosci-UK 27, 157–169. doi: 10.1016/S0098-3004(00)00097-2
Luff R., Wallmann K. (2003). Fluid flow, methane fluxes, carbonate precipitation and biogeochemical turnover in gas hydrate-bearing sediments at Hydrate Ridge, Cascadia Margin: Numerical modeling and mass balances. Geochim. Cosmochim. Acta 67, 3403–3421. doi: 10.1016/S0016-7037(03)00127-3
Luff R., Wallmann K., Grandel S., Schlüter M. (2000). Numerical modeling of benthic processes in the deep Arabian Sea. Deep. Res. Part II Top. Stud. Oceanogr. 47, 3039–3072. doi: 10.1016/S0967-0645(00)00058-8
Lukawska-Matuszewska K., Kielczewska J. (2016). Effects of near-bottom water oxygen concentration on biogeochemical cycling of C, N and S in sediments of the Gulf of Gdansk (southern Baltic). Cont. Shelf Res. 117, 30–42. doi: 10.1016/j.csr.2016.02.001
Luo M., Chen L., Wang S., Yan W., Wang H., Chen D. (2013). Pockmark activity inferred from pore water geochemistry in shallow sediments of the pockmark field in southwestern Xisha Uplift, northwestern South China Sea. Mar. Pet. Geol. 48, 247–259. doi: 10.1016/j.marpetgeo.2013.08.018
Malinverno A., Pohlman J. W. (2011). Modeling sulfate reduction in methane hydrate-bearing continental margin sediments: Does a sulfate-methane transition require anaerobic oxidation of methane? Geochem. Geophy. Geosy. 12, Q07006. doi: 10.1029/2011GC003501
Mau S., Römer M., Torres M. E., Bussmann I., Pape T., Damm E., et al. (2017). Widespread methane seepage along the continental margin off Svalbard-from Bjørnøya to Kongsfjorden. Sci. Rep. 7, 1–13. doi: 10.1038/srep42997
Mazumdar A., Peketi A., Joao H. M., Dewangan P., Ramprasad T. (2014). Pore-water chemistry of sediment cores off Mahanadi Basin, Bay of Bengal: Possible link to deep seated methane hydrate deposit. Mar. Pet. Geol. 49, 162–175. doi: 10.1016/j.marpetgeo.2013.10.011
Meister P., Liu B., Ferdelman T. G., Jørgensen B. B., Khalili A. (2013). Control of sulphate and methane distributions in marine sediments by organic matter reactivity. Geochim. Cosmochi. Acta 104, 183–193. doi: 10.1016/j.gca.2012.11.011
Meister P., Liu B., Khalili A., Böttcher M. E., Jørgensen B. B. (2019). Factors controlling the carbon isotope composition of dissolved inorganic carbon and methane in marine porewater: An evaluation by reaction-transport modelling. J. Mar. Syst. 200, 103227. doi: 10.1016/j.jmarsys.2019.103227
Meybeck M. (1993). Riverine transport of atmospheric carbon: sources, global typology and budget. Water Air Soil pollut. 70, 443–463. doi: 10.1007/BF01105015
Milkov A. V. (2004). Global estimates of hydrate-bound gas in marine sediments: How much is really out there? Earth-Science Rev. 66, 183–197. doi: 10.1016/j.earscirev.2003.11.002
Miller C. M., Dickens G. R., Jakobsson M., Johansson C., Koshurnikov A., O’Regan M., et al. (2017). Pore water geochemistry along continental slopes north of the East Siberian Sea: Inference of low methane concentrations. Biogeosciences 14, 2929–2953. doi: 10.5194/bg-14-2929-2017
Moore G. F., Taira A., Klaus A., Shipboard Scientific Party. (2001). Proceedings of the Ocean Drilling Program, Initial Reports. Volume 190.
Niewöhner C., Hensen C., Kasten S., Zabel M., Schulz H. D. (1998). Deep sulfate reduction completely mediated by anaerobic methane oxidation in sediments of the upwelling area off Namibia. Geochim. Cosmochim. Acta 62, 455–464. doi: 10.1016/S0016-7037(98)00055-6
Nöthen K., Kasten S. (2011). Reconstructing changes in seep activity by means of pore water and solid phase Sr/Ca and Mg/Ca ratios in pockmark sediments of the Northern Congo Fan. Mar. Geol. 287, 1–13. doi: 10.1016/j.margeo.2011.06.008
Paull C. K., Matsumoto R., Wallace P. J., Shipboard Scientific Party. (1996). Proceedings of the IODP, Initial Reports. (College Station, TX, USA), Volume 164.
Peckmann J., Reimer A., Luth U., Luth C., Hansen B. T., Heinicke C., et al. (2001). Methane-derived carbonates and authigenic pyrite from the northwestern Black Sea. Mar. Geol. 177, 129–150. doi: 10.1016/S0025-3227(01)00128-1
Phrampus B. J., Hornbach M. J. (2012). Recent changes to the Gulf Stream causing widespread gas hydrate destabilization. Nature 490, 527–530. doi: 10.1038/nature11528
Rassmann J., Lansard B., Gazeau F., Guidi-Guilvard L., Pozzato L., Alliouane S., et al. (2018). Impact of ocean acidification on the biogeochemistry and meiofaunal assemblage of carbonate-rich sediments: Results from core incubations (Bay of Villefranche, NW Mediterranean Sea). Mar. Chem. 203, 102–119. doi: 10.1016/j.marchem.2018.05.006
Reeburgh W. S. (1976). Methane consumption in Cariaco Trench waters and sediments. Earth Planet. Sci. Lett. 28, 337–344. doi: 10.1016/0012-821X(76)90195-3
Reitz A., Pape T., Haeckel M., Schmidt M., Berner U., Scholz F., et al. (2011). Sources of fluids and gases expelled at cold seeps offshore Georgia, eastern Black Sea. Geochim. Cosmochim. Acta 75, 3250–3268. doi: 10.1016/j.gca.2011.03.018
Riedinger N., Kasten S., Gröger J., Franke C., Pfeifer K. (2006). Active and buried authigenic barite fronts in sediments from the Eastern Cape Basin. Earth Planet. Sci. Lett. 241, 876–887. doi: 10.1016/j.epsl.2005.10.032
Ritger S., Carson B., Suess E. (1987). Methane-derived authigenic carbonates formed by subduction-induced pore-water expulsion along the oregon/washington margin. Bull. Geol. Soc Am. 98, 147–156. doi: 10.1130/0016-7606(1987)98<147:MACFBS>2.0.CO;2
Römer M., Sahling H., Pape T., Bohrmann G., Spieß V. (2012). Quantification of gas bubble emissions from submarine hydrocarbon seeps at the Makran continental margin (offshore Pakistan). J. Geophys. Res-Oceans 117, C10015. doi: 10.1029/2011JC007424
Ruban A., Rudmin M., Dudarev O., Mazurov A. (2020). The formation of authigenic carbonates at a methane seep site in the northern part of the laptev sea. Minerals 10, 1–14. doi: 10.3390/min10110948
Schlüter H. U., Prexl A., Gaedicke C., Roeser H., Reichert C., Meyer H., et al. (2002). The Makran accretionary wedge: Sediment thicknesses and ages and the origin of mud volcanoes. Mar. Geol. 185, 219–232. doi: 10.1016/S0025-3227(02)00192-5
Sivan O., Schrag D. P., Murray R. W. (2007). Rates of methanogenesis and methanotrophy in deep-sea sediments. Geobiology 5, 141–151. doi: 10.1111/j.1472-4669.2007.00098.x
Smith J. P., Coffin R. B. (2014). Methane flux and authigenic carbonate in shallow sediments overlying methane hydrate bearing strata in Alaminos Canyon, Gulf of Mexico. Energies 7, 6118–6141. doi: 10.3390/en7096118
Snyder G. T., Hiruta A., Matsumoto R., Dickens G. R., Tomaru H., Takeuchi R., et al. (2007). Pore water profiles and authigenic mineralization in shallow marine sediments above the methane-charged system on Umitaka Spur, Japan Sea. Deep. Res. Part II Top. Stud. Oceanogr. 54, 1216–1239. doi: 10.1016/j.dsr2.2007.04.001
Solomon E. A., Spivack A. J., Kastner M., Torres M. E., Robertson G. (2014). Gas hydrate distribution and carbon sequestration through coupled microbial methanogenesis and silicate weathering in the Krishna-Godavari Basin, offshore India. Mar. Pet. Geol. 58, 233–253. doi: 10.1016/j.marpetgeo.2014.08.020
Suess E. (2014). Marine cold seeps and their manifestations: geological control, biogeochemical criteria and environmental conditions. Int. J. Earth Sci. 103, 1889–1916. doi: 10.1007/s00531-014-1010-0
Suess E., Torres M. E., Bohrmann G., Collier R. W., Greinert J., Linke P., et al. (1999). Gas hydrate destabilization: Enhanced dewatering, benthic material turnover and large methane plumes at the Cascadia convergent margin. Earth Planet. Sci. Lett. 170, 1–15. doi: 10.1016/S0012-821X(99)00092-8
Sun X., Turchyn A. V. (2014). Significant contribution of authigenic carbonate to marine carbon burial. Nat. Geosci. 7, 201–204. doi: 10.1038/ngeo2070
Takahashi K., Ravelo A. C., Alvarez Zarikian C. A., Expedition 323 Scientists. (2011). Proceedings of the Integrated Ocean Drilling Program, Volume 323.
Titschack J., Goetz-Neunhoeffer F., Neubauer J. (2011). Magnesium quantifcation in calcites [(Ca, Mg)CO3] by rietveld-based XRD analysis: Revisiting a well-established method. Am. Mineral. 96, 1028–1038. doi: 10.2138/am.2011.3665
Treude T., Niggemann J., Kallmeyer J., Wintersteller P., Schubert C. J., Boetius A., et al. (2005). Anaerobic oxidation of methane and sulfate reduction along the Chilean continental margin. Geochim. Cosmochim. Acta 69, 2767–2779. doi: 10.1016/j.gca.2005.01.002
Ussler W., Paull C. K. (2008). Rates of anaerobic oxidation of methane and authigenic carbonate mineralization in methane-rich deep-sea sediments inferred from models and geochemical profiles. Earth Planet. Sci. Lett. 266, 271–287. doi: 10.1016/j.epsl.2007.10.056
von Rad U., Berner U., Delisle G., Doose-Rolinski H., Fechner N., Linke P., et al. (2000). Gas and fluid venting at the Makran accretionary wedge off Pakistan. Geo-Marine Lett. 20, 10–19. doi: 10.1007/s003670000033
von Rad U., Rösch H., Berner U., Geyh M., Marchig V., Schulz H. (1996). Authigenic carbonates derived from oxidized methane vented from the Makran accretionary prism off Pakistan. Mar. Geol. 136, 55–77. doi: 10.1016/S0025-3227(96)00017-5
Wallmann K., Aloisi G., Haeckel M., Tishchenko P., Pavlova G., Greinert J., et al. (2008). Silicate weathering in anoxic marine sediments. Geochim. Cosmochim. Acta 72, 2895–2918. doi: 10.1016/j.gca.2008.03.026
Wang M., Li Q., Cai F., Liang J., Yan G., Wang Z., et al. (2019). Formation of authigenic carbonates at a methane seep site in the middle Okinawa Trough, East China Sea. J. Asian Earth Sci. 185, 104028. doi: 10.1016/j.jseaes.2019.104028
Warren J. (2000). Dolomite: occurrence, evolution and economically important associations. Earth Sci. Rev. 52, 1–81. doi: 10.1016/S0012-8252(00)00022-2
Wefer G., Berger W. H., Richter C., Shipboard Scientific Party. (1998). Proceedings of the Ocean Drilling Program, Initial Reports. (College Station, TX: Ocean Drilling Program), 175, 487–504.
Wehrmann L. M., Risgaard-Petersen N., Schrum H. N., Walsh E. A., Huh Y., Ikehara M., et al. (2011). Coupled organic and inorganic carbon cycling in the deep subseafloor sediment of the northeastern Bering Sea Slope (IODP Exp.323). Chem. Geol. 284, 251–261. doi: 10.1016/j.chemgeo.2011.03.002
Wei J., Liang J., Lu J., Zhang W., He Y. (2019). Characteristics and dynamics of gas hydrate systems in the northwestern South China Sea - Results of the fifth gas hydrate drilling expedition. Mar. Pet. Geol. 110, 287–298. doi: 10.1016/j.marpetgeo.2019.07.028
Wei J., Wu T., Deng X., Haider S. W., Kahkashan S., Yang S. (2021). Seafloor methane emission on the Makran continental margin. Sci. Total Environ. 801, 149772. doi: 10.1016/j.scitotenv.2021.149772
Wei J., Wu T., Zhang W., Deng Y., Xie R., Feng J., et al. (2020). Deeply buried authigenic carbonates in the qiongdongnan basin, South China sea: Implications for ancient cold seep activities. Minerals 10, 1–19. doi: 10.3390/min10121135
Winde V., Böttcher M. E., Escher P., Böning P., Beck M., Liebezeit G., et al. (2014). Tidal and spatial variations of DI13C and aquatic chemistry in a temperate tidal basin during winter time. J. Mar. Syst. 129, 396–404. doi: 10.1016/j.jmarsys.2013.08.005
Wu T., Deng X., Yao H., Liu B., Ma J., Haider S. W., et al. (2021). Distribution and development of submarine mud volcanoes on the Makran Continental Margin, offshore Pakistan. J. Asian Earth Sci. 207, 104653. doi: 10.1016/j.jseaes.2020.104653
Wu L., Yang S., Liang J., Su X., Fu S., Sha Z., et al. (2013). Variations of pore water sulfate gradients in sediments as indicator for underlying gas hydrate in Shenhu Area, the South China Sea. Sci. China-Earth Sci. 56, 530–540. doi: 10.1007/s11430-012-4545-6
Wu Z., Zhou H., Ren D., Gao H., Li J. (2016). Quantifying the sources of dissolved inorganic carbon within the sulfate-methane transition zone in nearshore sediments of Qi’ao Island, Pearl River Estuary, Southern China. Sci. China-Earth Sci. 59, 1959–1970. doi: 10.1007/s11430-016-0057-0
Xu S., Liu B., Wu Z., Kowalski N., Böttcher M. E. (2023). The role of anaerobic methane oxidation on the carbonate authigenesis in sediments of the subtropical Beibu Gulf, South China Sea: A reactive–transport modelling approach. Chem. Geol. 619, 121319. doi: 10.1016/j.chemgeo.2023.121319
Zha R., Yang T., Shi X., Su P., Fang Y. (2022). Quantitative assessment of dissolved inorganic carbon cycling in marine sediments from gas hydrate-bearing areas in the South China Sea. Mar. Petrol. Geol. 145, 105881. doi: 10.1016/j.marpetgeo.2022.105881
Zhang S., DePaolo D. J. (2020). Equilibrium calcite-fluid Sr/Ca partition coefficient from marine sediment and pore fluids. Geochim. Cosmochi. Acta 289, 33–46. doi: 10.1016/j.gca.2020.08.017
Zhang X., Gong J., Sun Z., Liao J., Zhai B., Wang L., et al. (2021b). Pore-water geochemistry in methane-seep sediments of the Makran accretionary wedge off Pakistan: Possible link to subsurface methane hydrate. Acta Oceanol. Sin. 40, 23–32. doi: 10.1007/s13131-021-1899-7
Zhang Z., He G., Yao H.-q., Deng X., Yu M., Huang W., et al. (2020). Diapir structure and its constraint on gas hydrate accumulation in the Makran accretionary prism, offshore Pakistan. China Geol. 3, 611–622. doi: 10.31035/cg2020049
Zhang W., Liang J., Liang Q., Wei J., Wan Z., Feng J., et al. (2021a). Gas hydrate accumulation and occurrence associated with cold seep systems in the northern south China sea: an overview. Geofluids 2021, 1–24. doi: 10.1155/2021/5571150
Keywords: Makran accretionary wedge, anaerobic oxidation of methane (AOM), sulfate reduction, dissolved inorganic carbon (DIC), authigenic carbonate precipitation
Citation: Chen Y, Xu S, Liu W, Zhang Z, Yang T, Xiao X, Deng X, Li J, Yao H and Wu Z (2024) Assessing biogeochemical controls on porewater dissolved inorganic carbon cycling in the gas hydrate-bearing sediments of the Makran accretionary wedge, Northeastern Arabian Sea off Pakistan. Front. Mar. Sci. 10:1181921. doi: 10.3389/fmars.2023.1181921
Received: 08 March 2023; Accepted: 13 December 2023;
Published: 08 January 2024.
Edited by:
Glen T. Snyder, The University of Tokyo, JapanReviewed by:
Dong Feng, Shanghai Ocean University, ChinaFiroz Badesab, Council of Scientific and Industrial Research (CSIR), India
Copyright © 2024 Chen, Xu, Liu, Zhang, Yang, Xiao, Deng, Li, Yao and Wu. This is an open-access article distributed under the terms of the Creative Commons Attribution License (CC BY). The use, distribution or reproduction in other forums is permitted, provided the original author(s) and the copyright owner(s) are credited and that the original publication in this journal is cited, in accordance with accepted academic practice. No use, distribution or reproduction is permitted which does not comply with these terms.
*Correspondence: Huiqiang Yao, hqyao@163.com; Zijun Wu, wuzj@tongji.edu.cn