Bridging soil biogeochemistry and microbial communities (archaea and bacteria) in tropical seagrass meadows
- 1Department of Soil Science, Federal University of Ceará (UFC), Fortaleza, Ceará, Brazil
- 2Agricultural Production Program, Federal University of Agreste Pernambuco (UFAPE), Garanhuns, Pernambuco, Brazil
- 3Luiz de Queiroz College of Agriculture (ESALQ), University of São Paulo (USP), Department of Soil Science, Piracicaba, São Paulo, Brazil
- 4Institute of Oceanography, Federal University of Rio Grande (FURG), Rio Grande, Rio Grande do Sul, Brazil
- 5Commonwealth Scientific and Industrial Research Organization (CSIRO), Environment, Crawley, WA, Australia
- 6Institute of Eco-environmental Forensics, Shandong University, Qingdao, Shandong, China
- 7School of Environmental Science and Engineering, Shandong University, Qingdao, Shandong, China
- 8National Laboratory for Marine Science and Technology, Qingdao, Shandong, China
- 9Key Laboratory of Marine Ecology and Environmental Science, Institute of Oceanology, Chinese Academy of Sciences, Qingdao, Shandong, China
- 10Cretus, Departamento de Edafoloxía e Química Agrícola, Facultade de Bioloxía, Universidade de Santiago de Compostela (USC), Santiago de Compostela, Spain
Introduction: Seagrass meadows are among the most valuable ecosystems, providing numerous ecosystem services and functions. Despite its importance, there is a lack of knowledge about soil’s biogeochemical process variability, which can control microbiological communities. Thus, this study aimed to evaluate whether seagrass meadows in different geo-environments exhibit varying Fe and sulfate reduction intensities, shaping distinct archaea and bacteria communities.
Methods: Soil samples were collected in seagrass meadows under contrasting climatic, geological, vegetational and hydrological settings along the Brazilian coast (e.g., Semiarid Coast - SC, Southeastern Granitic Coast – GC, and Southern Quaternary Coast - QC). The soils were characterized by particle size, pH, redox potential (Eh), total organic C and total N content, acid-volatile sulfides (AVS), and simultaneously extracted Fe. Furthermore, a solid-phase Fe fractionation was performed to characterize the decomposition pathways in these soils, and the shifts in the microbial community along this spatial variation were analyzed using denaturing gradient gel electrophoresis.
Results: The studied soils presented a sandy texture (values ranging from 74 ± 11.8 to 80.5 ± 6.4%) caused by energetic hydrodynamic conditions. The pH values were circumneutral, while redox conditions presented significant distinction among the studied sites, ranging from anoxic to oxic (values ranging from -63 to +334 mV). The degree of pyritization (DOP) ranged from< 10% to values higher than 80%, highly influenced by rhizospheric oxidation, and higher AVS content was recorded for sites with lower DOP (i.e., GC and QC).
Discussions: Thus, biogeochemical processes in the seagrass soils present a wide variation in response to the geo-environmental settings. Plants influence the soil’s geochemical and microbiological communities, retaining fine particles, promoting rhizosphere oxidation, and inducing anoxic conditions controlling the Fe and S forms. Moreover, the same plant species can result in distinct soil conditions and microbial communities due to geoenvironmental settings.
1 Introduction
Seagrass meadows consist of a worldwide distributed ecosystem, primarily composed of plant species that typically grow fully submerged in shallow coastal areas forming extensive meadows with high productivity and biodiversity (Olsen et al., 2016; Turschwell et al., 2021). The seagrasses are a phylogenetically related plant group (order Alismatales) with low taxonomic diversity (66 known species) assigned to six families (e.g., Cymodoceaceae, Hydrocharitaceae, Posidoniaceae, Zosteraceae; Ruppiaceae and Zannichelliaceae; Zidorn, 2016). These plant species interact with a wide range of megaherbivores (e.g., dugongs, manatees, green turtles) and smaller species (e.g., fishes, molluscs, crustaceans, sponges), supporting complex and diverse communities along the coastal zone of all continents except Antarctica (Nordlund et al., 2016; Unsworth et al., 2019).
This ecosystem can be found along the coast of 163 countries, covering 160,000 to 267,000 km2 (McKenzie et al., 2020) and, thus, widespread over contrasting climatic, geological, vegetational and hydrological settings. However, estimations suggest that it may cover up to 600,000 km2 (Fourqurean et al., 2012), corresponding to 0.2% of the ocean areas (Charette and Smith, 2010), which equals twice the area of mangroves forests (Siikamäki et al., 2013).
Seagrass meadows provide a wide range of ecological services, e.g., support marine food webs (Cui et al., 2021; Valentine and Heck, 2021); supply raw material and food (Connolly et al., 2005; Barbier et al., 2011); provide habitat and nursery (Orth et al., 2006; Liquete et al., 2016); water purification (Fernandes et al., 2009); coastal protection (Ondiviela et al., 2014); but also educational, recreational, spiritual and touristic services (de la Torre-Castro and Rönnbäck, 2004; Nordlund et al., 2016). As a result, seagrass meadows represent one of the most valuable ecosystems on the planet with values higher than U$ 28,000.00 ha−1 yr-1 (2011 values; Barbier et al., 2011; Costanza et al., 2014).
Recently, numerous studies have been conducted to comprehend and highlight the role of seagrass meadows as globally significant carbon (C) sinks, storing significant amounts of C on their soils (Kennedy et al., 2010; Fourqurean et al., 2012; Rozaimi et al., 2016; Serrano et al., 2016; Ricart et al., 2020). The C storage in seagrass meadows results from high productivity (Duarte and Chiscano, 1999; Duarte et al., 2010), refractory plant tissues(Serrano et al., 2015; Kaal et al., 2016; Piñeiro-Juncal et al., 2020a), and the anoxic soil conditions, which may drive microbial communities and the anaerobic organic matter decomposition pathways of iron (Fe) and sulfate reduction (Fourqurean et al., 2012; Ugarelli et al., 2019; Sun et al., 2020).
In this ecosystem, due to the permanent flooding from seawater, with abundant sulfate supply, the organic matter decomposition occurs through Fe oxyhydroxides and sulfate reduction pathways since water column prevent oxygen diffusion through soil profile (Brodersen et al., 2017; Nóbrega et al., 2023). Consequently, Fe2+ and sulfides (including HS- and polysulfides) are produced, which can eventually form iron sulfides such as FeS and FeS2 (Otero and Macias, 2002; Queiroz et al., 2018). In anaerobic conditions, bioauthigenic pyrite (FeS2) emerges as the most stable product of sulfate reduction, holding diverse ecological significance (Otero et al., 2023).
In fact, the dynamics of Fe and sulfate in seagrass soils play an essential role in controlling several other processes that affect the very maintenance of this ecosystem, such as the bioavailability of nutrients (Delgard et al., 2016), trace metals (Tripathi et al., 2014), the fate of phosphorus (Brodersen et al., 2017), the geochemical control over dissolved sulfide (a highly phytotoxic compound for seagrass species (Azzoni et al., 2005; Holmer et al., 2005) and the C dynamics (Yu et al., 2021). Therefore, Fe and sulfate reduction, which are controlled by microbial processes (Brodersen et al., 2018; Piñeiro-Juncal et al., 2020b), play essential roles in the maintenance of different ecosystem services provided by seagrass meadows (e.g., carbon sequestration and pollutants immobilization) (Nordlund et al., 2016; York et al., 2017; Ramírez-Flandes et al., 2019; Ricart et al., 2020; Sun et al., 2020).
However, despite the ecological importance of Fe and sulfate biogeochemistry for seagrass meadows and other coastal wetlands (i.e., controlling pollutant, nutrient and C dynamics), there is still a global knowledge gap on its relationship with the microbial communities and how these groups are shaped in response to the geo-environmental conditions (e.g., distinct climatic, geological conditions and vegetation), which may control the intensity of biogeochemical processes.
Because seagrass meadows colonize highly diverse environments (Gorman et al., 2020; Ricart et al., 2020; Nóbrega et al., 2023), the intensities of these geochemical processes may vary widely and shape different microbial communities. The present study tests the hypothesis that seagrass meadows at contrasting geo-environments (e.g., climate, geology, and plant species and cover) will operate under different Fe and sulfate reduction intensities and, thus, will shape different microbiological communities. This is a novel study bridging the core soil processes of coastal wetlands (i.e., Fe and sulfate reduction) and microbial communities (bacteria and archaea) in diverse tropical geo-environments. Our results provide knowledge for a better understanding of the processes related to organic C accumulation, bioavailability of nutrients, and pollutant cycling in seagrass meadows.
2 Material and methods
2.1 Study site
Soil samples were collected in seagrass meadows located in three contrasting compartments along the 9,200 km of the Brazilian coast (Figure 1), presenting distinct climate (hot semiarid, humid tropical, and humid subtropical climates), geological setting (sedimentary and magmatic rocks), coastal conditions (estuary, beach, and coastal lagoon), and different plant species (Halodule wrightii. and Ruppia maritima) aiming to assess a maximum soil variability. Thus, seagrass soils from the semiarid coast (SC), granitic coast (GC) and quaternary coast (QC) compartments were samples and analyzed (Figure 1).
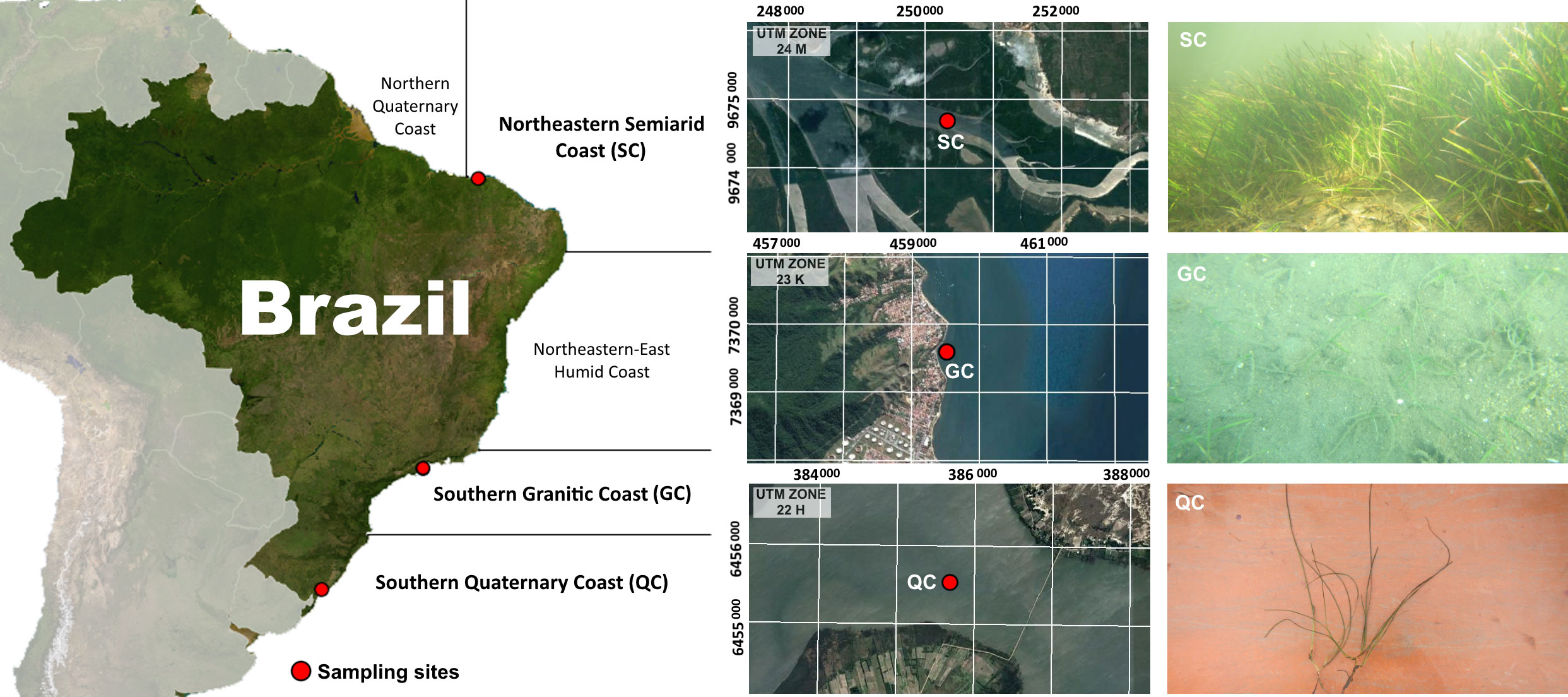
Figure 1 Location of sampling sites along the Brazilian coast. In detail, plant species and canopy characteristics for the three studied sites. The x and y axes in the satellite images indicate the coordinates in UTM.
The semiarid coast (SC) extends from the states of Piauí to Pernambuco (Figure 1), marked by a semiarid climate (mainly classified tropical savanna and hot semi-arid climates; i.e., Aw and BSh climate types, respectively, according to Köppen-Geiger Climate Classification; Peel et al., 2007). The SC presents an annual rainfall (approximately 800 mm) lower than the evapotranspiration; and an average water temperature ranging from 27 to 29°C during the year (average minimum water temperature: 26°C and maximum: 30°C; seatemperature.org). In addition, this compartment is marked by close contact of the “Barreiras” group formation to the coastline, which results in sandy beaches and coral reefs close to the estuaries favouring seagrass beds formation (Copertino et al., 2016). The Barreiras group is a Miocene-Pliocene (Tertiary) transitional-coastal sedimentary deposit whose upper portion is composed dominantly of poorly sorted sand (Vilas Bôas et al., 2001; Arai, 2006). The seagrass meadow soils were collected in an estuary (at Ceará state, NE-Brazil) highly densely vegetated by H. wrightii Asch. (Figure 1); surrounded and close to (< 100 m) mangrove forests under low anthropogenic impacts due to the low occupation of the estuary (Silva and Souza, 2006), and found at water depths ranging from 1.7 to 2.9 m (water depth corrected to the mean water level).
The granitic coast (GC) covers the area from the Guanabara Bay (Rio de Janeiro state) to the Praia dos Sonhos (Santa Catarina state). In this compartment, narrow coastal plains are interrupted by the Serra do Mar’s granitic-gneiss rock with small bays and beaches protected by rocky shores and almost 2,000 islands close to the shore (Schaeffer-Novelli et al., 1990). The humid tropical climate of the region (tropical rainforest climate – Af, according to Köppen-Geiger Climate Classification; Peel et al., 2007) presents an annual rainfall average higher than 1,600 mm during the entire year, with lower monthly precipitation higher than 60 mm, resulting in a hydric surplus, and the average monthly water temperature ranges from 21 and 27°C during the year (average minimum water temperature: 20°C and maximum: 28°C; seatemperature.org; Minuzzi et al., 2007). At this compartment, the representative H. wrightii. meadow was collected in an urbanized beach area (at São Paulo state, Southeastern Brazil), presenting sparse vegetation with low plant density (Figure 1) at a water depth of 1.3 m (corrected to the mean water level).
The south quaternary coastal (QC) compartment extends 600 km from the Granitic coast to the border with Uruguay. This part of the coast presents a humid subtropical climate (Cfa according to Köppen-Geiger Climate Classification; Peel et al., 2007), with low annual temperature mean and a high-temperature oscillation (>10°C), annual precipitation of 1,300 mm, besides the hydric surplus (> 400 mm; Schaeffer-Novelli et al., 1990; Alvares et al., 2013). The average water temperature ranges from 13 to 24°C during the year (average minimum: 12°C and average maximum 26°C; seatemperature.org). The soil samples were collected at the Lagoa dos Patos (Patos Lagoon), at the Rio Grande do Sul State (S-Brazil), a 10,000 km2 water body formed by sandy deposits during transgressive events during the Pleistocene (Toldo Jr. et al., 2000). The ephemeral and euryhaline Ruppia maritima seagrass meadow was collected at an average water depth of 1.5 m (corrected to the mean water level).
Seagrass meadows were observed and visually analyzed in each coast compartment to identify representative meadows (e.g., most frequent plant species, plant density and coverage), avoiding bare areas or seagrass meadows highly colonized with epiphytes or macroalgae.
2.2 Soil sampling procedure and physicochemical (Eh and pH) characterization
Three soil cores were randomly collected inside the representative seagrass meadows in each coast compartment using transparent polycarbonate tubes (60 mm diameter) attached to a submerged soil auger (Uwitec surface corer), which resulted in minimum sample compactation (Erich and Drohan, 2012). Soil samples were carefully extruded from the cores, partitioned into sections (0-3 cm, 3-6 cm, 6-10 cm, 10-13 cm, 13-16 cm, and 16-20 cm, with additional 5 cm increments) and, subsequently, pH and redox potential (Eh) values were promptly recorded to prevent oxidation. Following the characterization of physico-chemical conditions (Eh and pH), the samples were placed in plastic bags and transported to the laboratory under refrigeration (<4°C). In the lab, sub-samples (from the core’s central region) were immediately frozen for subsequent analysis, while other sub-samples were dried (at 45°C until reaching a constant weight) and sieved through a 2 mm mesh.
The pH values were measured using a glass electrode calibrated using pH 4.0 and 7.0 buffer solution during core sectioning. The redox potential (Eh) was determined using a platinum electrode, with the final readings corrected by adding the potential of the calomel reference electrode (+ 244 mV) (Passos et al., 2016). All readings were taken after equilibrating the soil samples and the electrodes for several minutes (~2 min).
2.3 Soil characterization
The soils from each seagrass meadow were characterized by particle size composition, total organic C, and total nitrogen.
The hydrometer method was used for the particle size analysis (Gee and Bauder, 1986) by a combination of physical (overnight shaking) and chemical (1 mol L-1 NaOH + 0.015 mol L-1 (NaPO3)6) dispersion after the organic matter elimination using hydrogen peroxide (30% vol). Total nitrogen (TN) and total organic carbon (TOC) were determined in an elemental analyzer (LECO 628 series). The TOC quantification was the difference between the total C and inorganic C contents (obtained after organic matter combustion in a muffle furnace at 550 °C for two hours; Howard et al., 2014).
2.4 Fractionation of Fe and Sulfur
The partitioning of the solid phase of Fe was performed via a sequential extraction designed by the combination of methods proposed by Tessier et al. (1979); Huerta-Díaz and Morse (1990), and Fortin et al. (1993) using frozen samples, obtaining six operationally distinct fractions as follow: exchangeable Fe (FeEX), extracted with 30 mL of MgCl2 1 mol L-1 for 30 minutes; Fe associated with carbonates (FeCA), extracted with 30 mL of 1 mol L-1 sodium acetate buffer solution (pH = 5.0) for 5 hours; ferrihydrite (FeFR), extracted with 0.04 mol L-1 hydroxilamine + acetic acid 25% (v/v) at 30 °C for 6 hours; lepidocrocite (FeLP), extracted with 0.04 mol L-1 hydroxilamine + acetic acid 25% (v/v) at 96 °C for 6 hours; crystalline Fe oxyhydroxides (FeCR), extracted with 0.25 mol L-1 sodium citrate + 0.11 mol L-1 + 3 g of sodium dithionite for 30 minutes; and Fe associated with pyrite fraction (FePY), extracted with concentrated nitric acid for 2h, after the removal of silicate fraction (10 M HF during 16 hours, then added 5 grams of boric acid and agitated during 8 hours) and organic matter (concentrated H2SO4 during 2 hours). For further details, please see Otero et al. (2009) and Osterrieth et al. (2016).
All solutions used for extraction were pretreatment for removing dissolved oxygen (i.e., purged with N2 for 2 hours) to prevent possible Fe and S oxidation during the extraction steps. The extractants were collected between each step after centrifugation at 6,000 RPM (4 °C) for 30 minutes. The samples were washed with 20 mL of ultrapure water between each step and followed by centrifugation. The concentrations of Fe in each extract were quantified by flame atomic absorption spectrophotometry (Thermo Fisher Scientific, Waltham, MA, USA). Curve calibration solutions were prepared by diluting a certified standard solution (iron standard, TraceCERT®) to determine Fe concentration and a certified reference material (NIST SRM 2709a) was employed for quality control, with triplicate measurements yielding Fe concentration recovery values exceeding 92%. The results of the fractionation are presented as μmol g-1 (micromole per gram) of dried soil, corrected by sample water content.
The degree of Fe pyritization (DOP), which determines the percentage of reactive Fe incorporated into the pyrite fraction, was calculated as follows: DOP (%) = 100*FePY/(FeREACTIVE + FePY) (Huerta-Diaz and Morse, 1992). The reactive fraction (FeREACTIVE) was considered the sum of the fractions exchangeable to crystalline (ΣFeEX → FeCR), whereas the pseudo-total content was determined by the sum of the six fractions (ΣFeEX → FePY).
The acid-volatile sulfides (AVS) fraction was extracted from frozen wet samples by acid distillation with HCl (20 mL, 3 mol L-1) in a gas-tight reaction flask after 40 minutes under continuous N2 flow (Allen et al., 1993; Otero et al., 2009). The evolved H2S was trapped in a Zn acetate solution (3%). The concentration of sulfides was colourimetrically determined by the method proposed by Cline (1969), and the simultaneously extracted Fe (Fe-AVS) was quantified by flame atomic absorption spectrophotometry (Allen et al., 1993).
2.5 Microbial molecular procedures
Frozen soil samples (400 mg) from different coastal compartments and depths (e.g., upper: 0-3 cm; intermediate: 16-20 cm; and deeper: 55-60 cm for SC and QC coast, and 35-40 cm for GC, due to soil depth limitation) were subjected to a total DNA extraction using the Power Soil DNA Isolation kit (MoBio Laboratories, Carlsbad, CA, USA) following the manufactory instructions. First, DNA extraction and integrity were assessed onto a 1.2% agarose gel electrophoresis with 1x TAE buffer (400 mmol L-1 Tris, 20 mmol L-1 acetic acid, and 1 mmol L-1 EDTA). Subsequently, the gels were stained with GelRed™ (0.5 μg mL-1), visualized, and photo-documented under ultraviolet light (DNR–Bio Imaging Systems/MiniBis Pro).
The amplification of the V6 region of ribosomal gene 16S rDNA for the bacterial community was performed with primers F968-GC and R1378, generating fragments of 410 bp (Heuer et al., 1997). The archaeal amplicons from rrs genes were obtained with primers Arch21F and Arch958R in the first reaction and Arch340FGC and Arch519R in the second nested-PCR reaction (Ovreås et al., 1997). The PCR reactions of bacterial communities were conducted in Veriti Thermal Cycler (Applied Biosystems, Waltham, USA), in a final volume of 50 µL, containing 1X Taq Buffer containing 2.50 mmol L-1 MgCl2, 0.25 mmol L-1 of each dNTP, 0.4 mmol L-1 of each primer, 1% formamide and 1U Taq DNA Polymerase (Fermentas, Burlington, Canada). For archaea communities, 1x Taq Buffer contained 3.0 mmol L−1 MgCl2, 0.2 mmol L−1 of each dNTP, 5 pmol L-1 of each primer, and 1U Taq DNA polymerase (Fermentas, Burlington, Canada). The amplification reactions for bacterial DNA were performed in the following conditions: initial denaturation at 94°C and then 35 cycles of denaturation for 1 minute; annealing at 56°C for 1 min and elongation at 72°C for 2 min. At the end of 35 cycles, a final extension step was conducted at 72°C for 10 min. Amplification reactions for archaea communities were performed at 95°C for 5 minutes; and 30 cycles of 1 min at 95°C, 1 min at 55°C, 1 min at 72°C, and final extension for 10 min at 72°C.
The PCR products were loaded onto 6% (16S rRNA genes) and 8% (rrs genes) (w/v) polyacrylamide gels with denaturing gradients of 45 to 65% for bacterial and 30 to 55% for archaea community, using urea 7 mol L-1 and 40% deionized formamide as 100% denaturing solution. The gels were run for 16 h at 100 V at 60°C using the phorU2 systems (Ingeny International, Goes, Netherlands) and stained with SYBR Green I (Invitrogen, Breda, the Netherlands). The DGGE gels were photo-documented with Storm 845 (General Electric) and analyzed using the Image Quant TL unidimensional (Amersham Biosciences, Amersham, UK, v.2003), where band patterns were converted into richness (number of bands) and abundance matrices.
2.6 Statistical analysis
Differences between soil variables were analyzed by the non-parametric Kruskal-Wallis test, followed by the Bonferonni-Dunn method for multiple pairwise comparisons (XLSTAT, Addinsoft, New York, USA, 2014). Kruskal-Wallis text was used since it was not observed a normal distribution and equal variance. Since few assumptions are required for non-parametric analysis, this test is considered more robust for environmental data (Reimann et al., 2008). Pearson’s correlation coefficient (R) established the relationships between different variables. Finally, a Discriminant Analysis (DA) was performed to develop a function that yields optimal discrimination of the study sites (SC, GC, and QC coasts). Through the DA, the relative contribution of the variables could be identified to the separation of the groups, i.e., the most relevant variables for separating the study sites (Reimann et al., 2008).
The bacterial and archaeal community structure changes among sites were obtained through beta-diversity estimations. The beta-diversity analysis compares the groups of microorganisms revealed by the DGGE band patterns among the samples, relying on the microorganisms’ profile structure and relationship with the environmental soil parameters (Lozupone et al., 2007). For the beta-diversity calculation, similarity matrices were produced using the Bray-Curtis algorithm on square-root transformed data, allowing the observation of the distribution of the groups between the samples on a two-dimensional scale. PERMANOVA analyses, based on two factors (i.e., coastal compartment and depth of soil sample), were followed by a Monte Carlo method with fixed effects, sums of squares type III (partial) under a reduced model, and 999 permutations. The ANOSIM test followed a one-way analysis based on one factor allowing the comparison among treatments under 999 permutations based on a p-value<0.05. Finally, we performed a redundancy detrended analysis (RDA) regarding the environmental factors correlated to the bacterial distribution and sampling depth. The statistical tests regarding microbial communities were performed using the software PAST (Hammer et al., 2001), PRIMER 6+PERMANOVA (Clarke and Gorey, 2006), and Canoco (Canoco 4.5, Biometris, Wageningen, The Netherlands).
3 Results
3.1 Characteristics of the physicochemical environments
The particle size of soils from all studied sites presented a predominance of sand, with mean ± standard deviation from 74.0 ± 11.8% at the QC to 80.5 ± 6.4% at the SC (Figure 2; Table 1). In contrast, silt content varied from 6.2 ± 4.9% at SC to 12.2 ± 5.4% at the QC coast, and clay varied from 8.1 ± 4.1% at the GC to 14.1 ± 2.3% at the SC coast. All three particle sizes presented a statistically significant difference (Table 1). Additionally, the soils from the three studied sites presented an erratic sand distribution throughout the soil profiles (Figure 2).
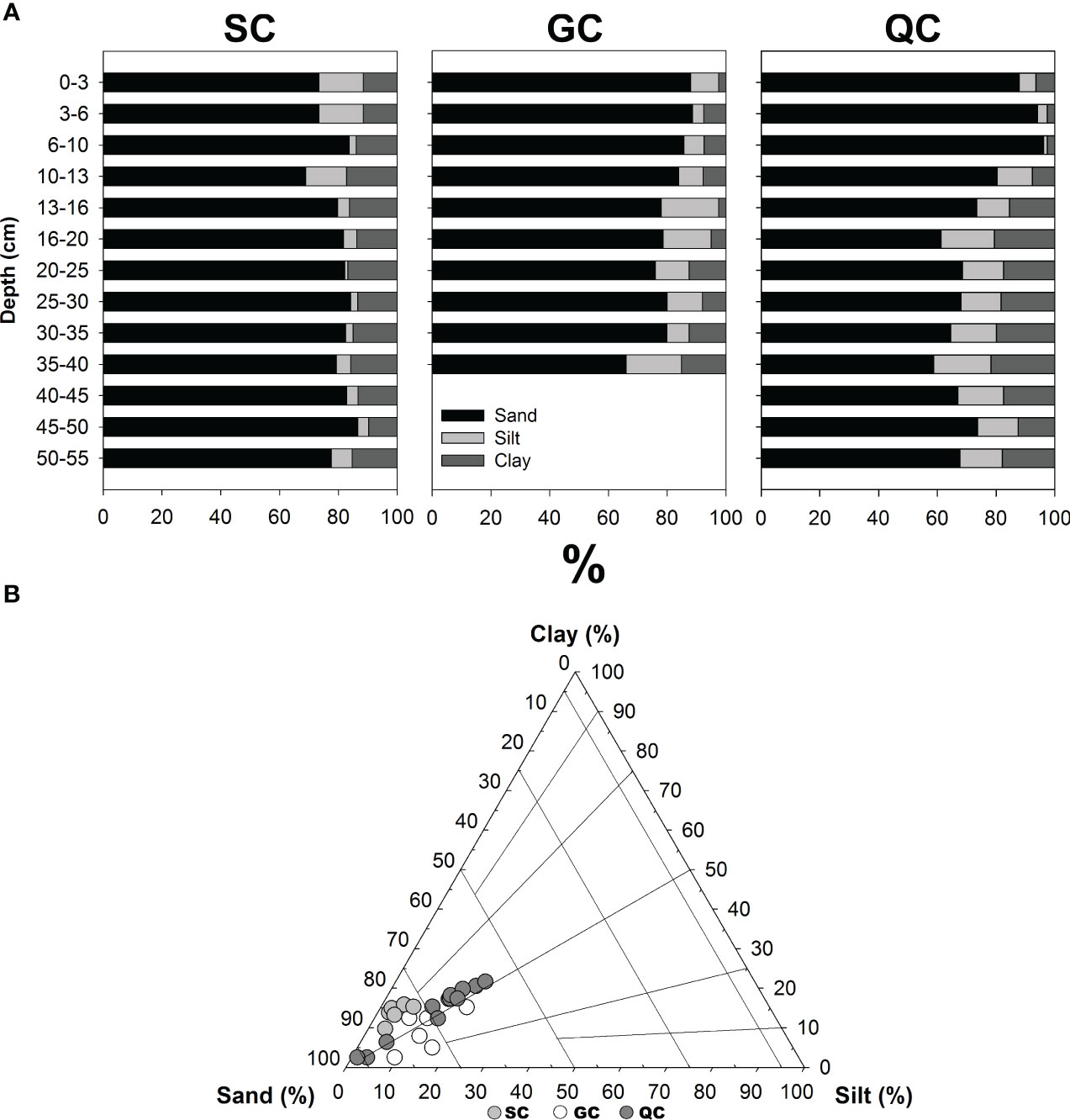
Figure 2 (A) Particle size distribution along seagrass meadows’ soil profiles collected along the Brazilian coast. (B) Natural variability of particle size distribution in the seagrass meadows soils from the Brazilian coast under contrasting geo-environment conditions, according to Flemming (2000).
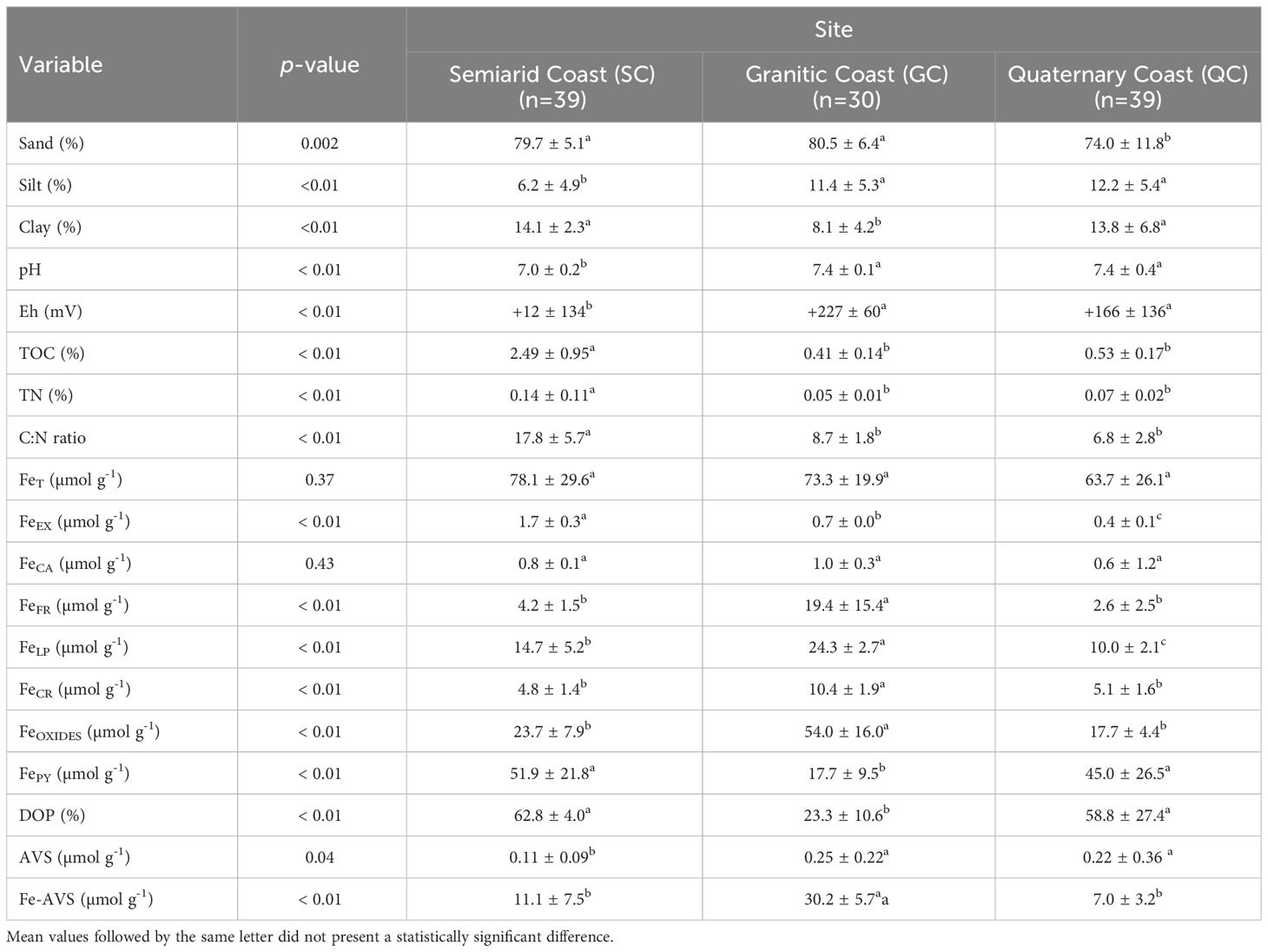
Table 1 Average and Kruskall-Wallis statistical results (p-value) for the variables in the three studied sites.
Regarding the physicochemical conditions, the pH values recorded for the three sites were circumneutral, with lower pH values recorded at the SC (mean value ± standard deviation: 7.0 ± 0.2), whereas GC (7.4 ± 0.1) and QC (7.4 ± 0.4) did not differ statistically (Table 1). Additionally, whereas the pH values of the GC coast soil did not present a significant variation through soil profiles, the soils from the SC coast present higher values at the deeper soil layers (> 10 cm), and the soils from the QC coast presented oscillating values, with a decrease at the intermediate soil layers (10-30 cm) followed by an increase at deeper layers (>30 cm) (Figure 3A).
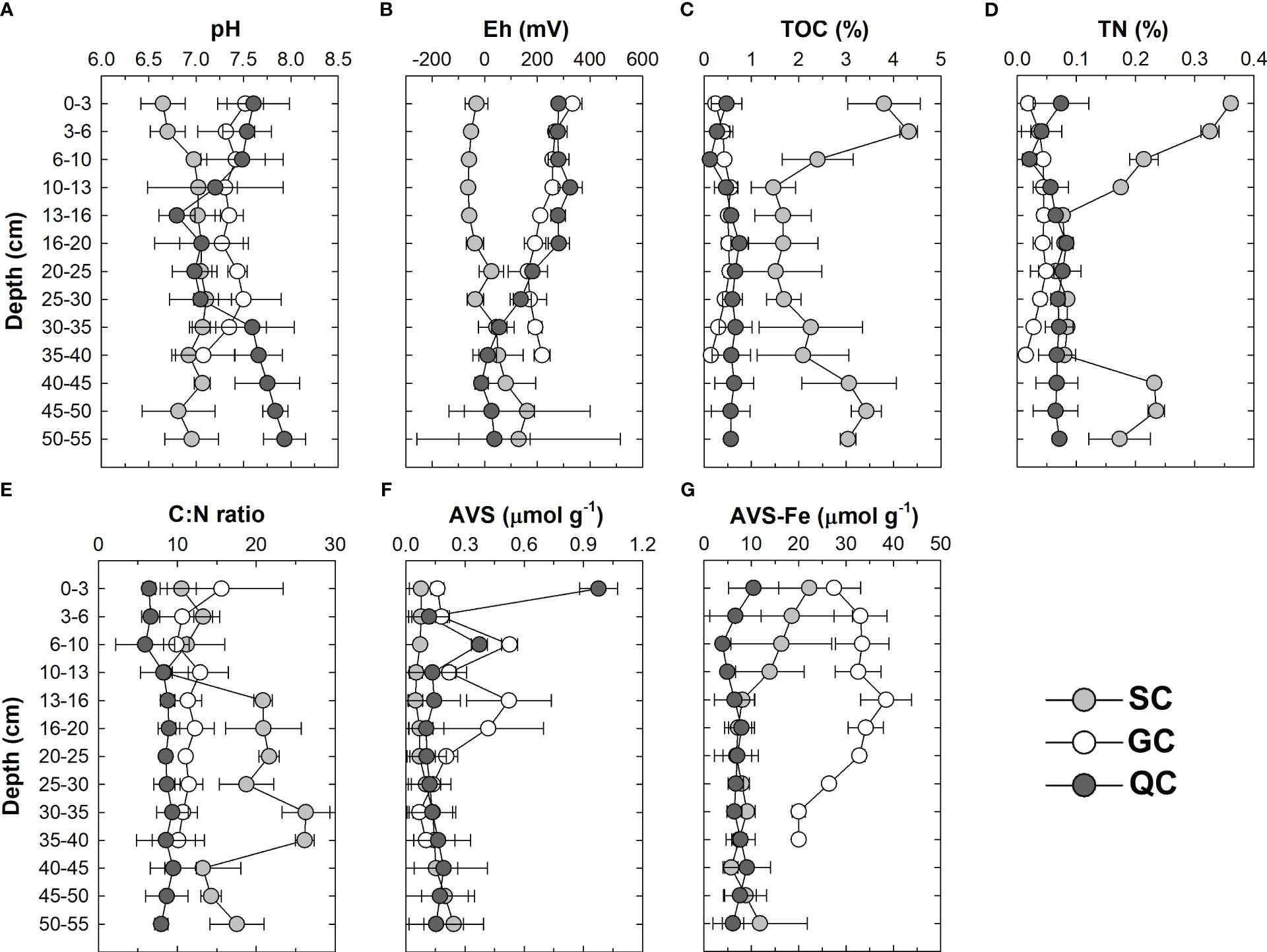
Figure 3 Characterization of the physico-chemical environment of the soil profiles from the three studied seagrass meadows: pH (A), Eh (B), total organic carbon contents, TOC (C), total nitrogen contents, TN (D), C:N ratio (E), concentration of acid volatile sulfides, AVS (F) and Fe associated with AVS fraction, AVS-Fe (G).
The Eh values presented a wide variation, ranging from −63 to +334 mV, evidencing significantly contrasting conditions between the studied sites (Figure 3B; Table 1). Significantly lower Eh values were recorded for the SC (mean ± standard deviation: +12 ± 134 mV), whereas the values recorded for QC (+166 ± 136 mV) and GC (+227 ± 60 mV) did not differ statistically. The redox contrasts were also evidenced in the surface soil horizons (down to 40 cm depth). While the Eh values recorded at GC and QC evidenced an oxic environment (average mean for the surface soil horizons ± standard deviation: +209 ± 113 mV), significantly lower values were recorded at SC (−22 ± 44 mV), thus, evidencing an anoxic environment. As occurred to the pH, the Eh values presented different trends in-depth among the different geoclimatic zones (Figure 3A). At the SC coast, the Eh values increased in-depth, whereas for both GC and QC coast, a decreasing trend in the recorded values (Figure 3B).
Significantly higher TOC contents were found at the SC coast (mean ± standard deviation: 2.49 ± 0.95%; Figure 3C) when compared to QC (0.53 ± 0.17%) and GC (0.41 ± 0.14%; Table 1). Similarly, significantly higher TN contents were recorded at the SC coast (0.14 ± 0.11%; Figure 3D; Table 1), while the values recorded for QC (0.07 ± 0.02%) and GC (0.05 ± 0.01%) coast did not differ significantly (Figure 3D; Table 1). When considering the variation through the soil profile, the TOC and TN values recorded for the GC and QC coast presented a slight variation. On the other hand, for the SC coast, higher values were recorded for the upper (0-10 cm) compared to deeper (>35 cm) soil layers. The C:N ratios were also significantly different among the three sites, with lower values at QC (6.8 ± 2.8), followed by GC (8.7 ± 1.8) and SC (17.8 ± 5.7; Figure 3C).
3.2 Fe fractionation
The values of pseudo-total Fe (FeT) contents were statistically similar among the three sites (means ± standard deviation for the SC:78.1 ± 29.6 μmol g-1; GC: 73.3 ± 19.9 μmol g−1; and QC: 63.7 ± 26.1 μmol g−1; Figure 4; Table 1).
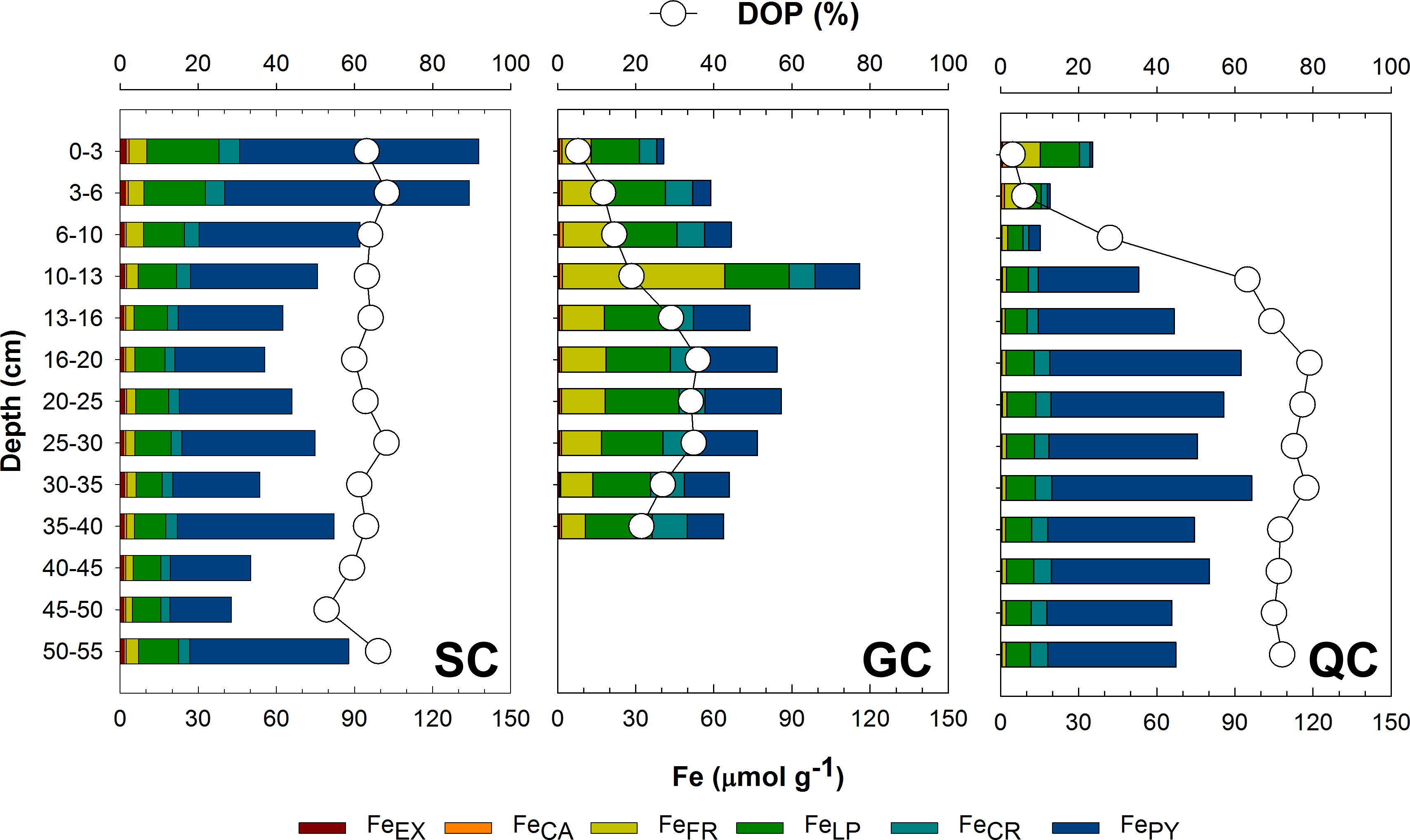
Figure 4 Solid-phase fractionation of Fe for the soils at the SC, GC, and QC coasts. The stacked bars indicate de fractions of soluble and exchangeable Fe (FeEX), Fe bounded to carbonates (FeCA), Fe associated with ferrihydrite (FeFR), Fe associated with lepidocrocite (FeLP), Fe associated with crystalline Fe oxyhydroxides (FeCR), and the Fe associated with pyrite (FePY). The interconnected white circles indicate the degree of Fe pyritization (DOP).
On the other hand, statistically significant differences were recorded for all Fe fractions (Figure 4; Table 1). At QC and SC, FePY was the most important fraction (mean ± standard deviation: 51.9 ± 21.8 μmol g-1 for SC coast and 45.0 ± 26.5 μmol g-1 for QC coast) representing more than 50% of the FeT (DOP > 50%) whereas, for the GC, FePY represent less than 50% of the FeT (DOP< 50%; Figure 4), with significantly lower contents (17.7 ± 9.5 μmol g-1). Additionally, the DOP values presented a significant variation throughout the soil for GC and QC, with a lower DOP at the upper soil layers (0-10 cm; Figure 4). In contrast, the DOP at the SC did not oscillate with soil depth (Figure 4).
Both FeEX and FeCA were the fraction with a lower contribution to FeT, with mean ± standard deviation values of FeEX contents oscillating from 0.4 ± 0.1 to 1.7 ± 0.3 μmol g-1 for QC and SC coast, respectively; and mean values of FeCA varying from 0.6 ± 1.2 and 1.0 ± 0.3 μmol g-1 for the QC and GC coast, respectively (Figure 4).
Regarding the Fe oxides fraction (i.e., FeFR + FeLP + FeCR), significantly higher contents were quantified at GC (mean ± standard deviation: 54.0 ± 16.0 μmol g-1; Table 1), followed by SC (23.7 ± 7.9 μmol g-1), and QC coast (17.7 ± 9.5 μmol g-1). For the three sites, the lepidocrocite (FeLP) was the most dominant oxyhydroxide fraction, with mean ( ± standard deviation) values ranging from 10.0 ± 2.1 to 24.3 ± 2.7 μmol g-1 at the QC and GC coasts (Figure 4; Table 1). Moreover, for the GC, the FeCR fraction was the less relevant oxyhydroxide fraction (10.4 ± 1.9 μmol g-1), whereas the ferrihydrite (FeFR) was the less important Fe oxyhydroxide fraction for the other sites (4.2 ± 1.5 μmol g-1 for NE and 2.6 ± 2.5 μmol g-1 for QC coast; Figure 4).
3.3 Acid volatile sulfides (AVS) and simultaneously extracted Fe (AVS-Fe)
The AVS contents ranged from 0.05 to 2.03 μmol g-1 (Figure 3F) among the studied sites. Significantly, lower AVS contents were recorded at the SC (mean ± standard deviation: 0.11 ± 0.09 μmol g-1), followed by QC (0.22 ± 0.36 μmol g-1) and GC (0.25 ± 0.22 μmol g-1; Figure 3F).
Regarding the AVS-Fe, statistically significant higher values were recorded for the GC (30.2 ± 5.7 μmol g-1), whereas the values recorded for SC (5.6 ± 1.5 μmol g-1) and QC (7.0 ± 3.3 μmol g-1) did not differ statistically (Figure 3G; Table 1).
3.4 Discriminant analysis of soils variables
The discriminant analysis indicated the most relevant variables for the differentiation of the studied sites (Figure 5). For the soils from SC, the vectors position indicates an association with higher TOC, TN, FeEX contents, higher C:N ratio values, and lower pH, Eh, and AVS contents. The GC soils were associated with higher Fe oxyhydroxides (FeFR, FeLP, FeCR, and FeOXIDES) and Fe-AVS as observed by the vectors positioned in its direction and lower DOP, FePY, and clay contents as evidenced by the vectors positioned in the opposite direction (Figure 5). On the other hand, the vector’s position indicates that soils from QC differ from the SC and GC by lower sand and FeEX contents and higher silt and pH (Figure 5). Furthermore, when comparing SC and QC, it can be stated that SC presents higher values of FePY and DOP.
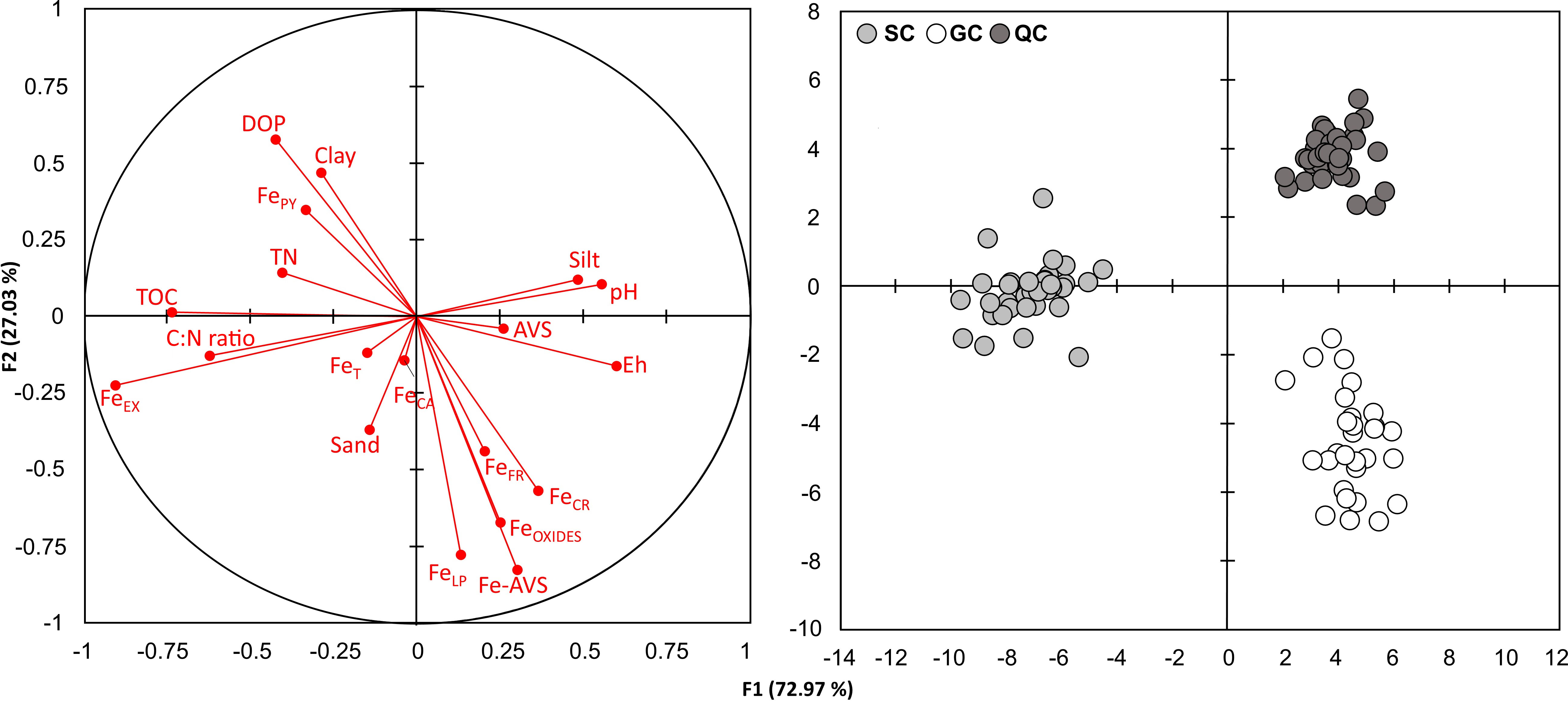
Figure 5 Discriminant analysis results of seagrass meadows’ soils variables in the three studied geo-environment (i.e., SC, GC, and QC) along the Brazilian coast. TOC, Total organic carbon contents; TN, total nitrogen contents; AVS, acid volatile sulfides; AVS-Fe, Fe associated with AVS fraction; FeEX, soluble and exchangeable Fe; FeCA, Fe bounded to carbonates; FeFR, Fe associated with ferrihydrite; FeLP, Fe associated with lepidocrocite; FeCR, Fe associated with crystalline Fe oxyhydroxides; FePY, Fe associated with pyrite; FeOXIDES, the sum of FeFR; FeLP; and FeCR; and DOP, degree of Fe pyritization.
3.5 Bacteria and archaea community characteristics (PCR-DGGE Results)
PCR-DGGE results evidence a clear differentiation in the bacteria and archaea community structure among the coastal compartments and soil depths, shown by the PERMANOVA data analysis (Table 2). In fact, the coastal compartments played a higher influence in the distribution of the archaea (Pseudo-F = 15.657; p = 0.001) and bacteria (Pseudo-F = 8.097; p = 0.001) communities; followed by the influence of soil depth (Pseudo-F = 4.395 and 3.519 for archaea and bacteria communities, respectively; p = 0.001). In addition, the results demonstrated the integration of factors (coastal compartment vs. depth) in the distribution of the bacteria (Pseudo-F = 3.745; p = 0.001) and archaea (Pseudo-F = 6.294; p = 0.001) communities (Table 2).
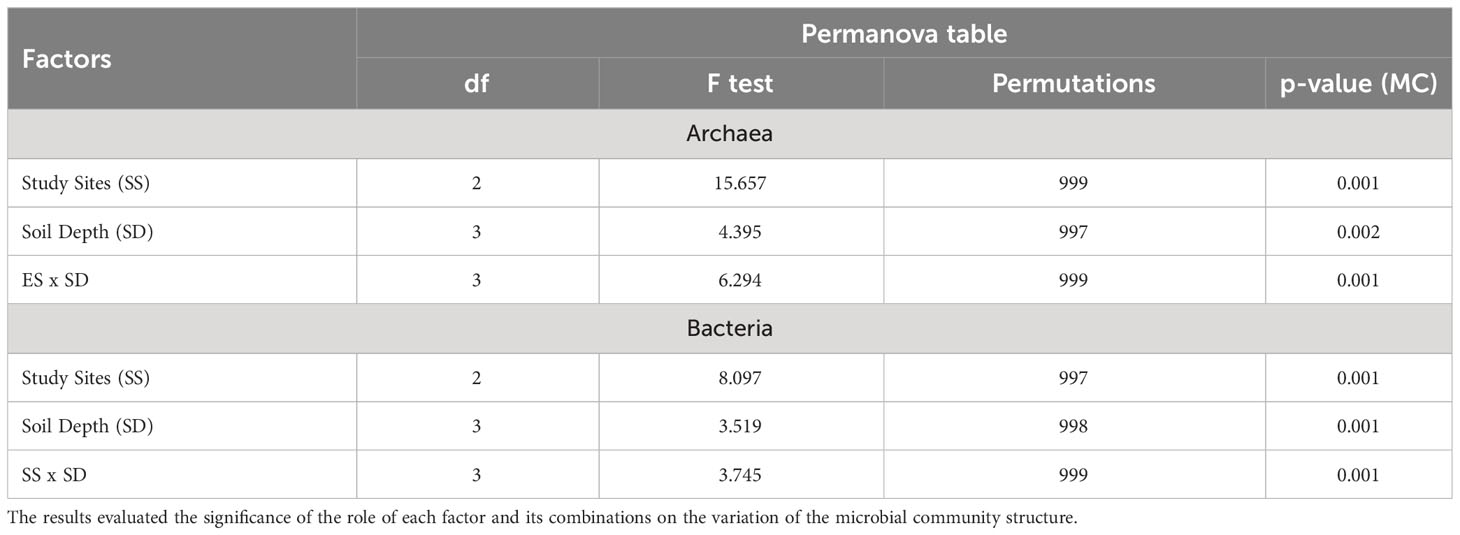
Table 2 Permanova analysis of the in a Monte Carlo test arrangement with 999 permutations, under a p-value MC< 0.05.
Moreover, the separation of the archaea community is also demonstrated by the ANOSIM-test (coastal compartment: Global R = 0.947; p = 0,001 and soil depth: Global R = 0.786; p = 0.001). Regarding the bacterial community, the ANOSIM-test demonstrated a clear separation among the sites (Coastal compartment: Global R = 0.967; p = 0,001). However, some profile overlap was found regarding the soil depth (Global R = 0.58; p = 0.001), indicating some similarity among the bacterial group sampled in distinct soil depths.
According to the redundancy analysis, the soils from SC archaea communities were positively associated with TOC, FeT, TN, DOP, and clay, whereas the GC were associated with FeOXIDES and sand (Figure 6A). The upper horizons of the QC archaea communities were positively associated with higher Eh and pH, whereas the intermediate and deeper soil layers were negatively associated with FeOXIDES (Figure 6A). The intermediate and deeper soil layers from GC were, comparatively, more associated with the deeper layers from SC soils. The GC bacterial communities were positively associated with FeOXIDES, whereas QC communities were negatively associated with FeOXIDES (Figure 6B). The SC communities were positively associated with TOC, TN, FeT, DOP, and clay and negatively associated with Eh and pH (Figure 6B).
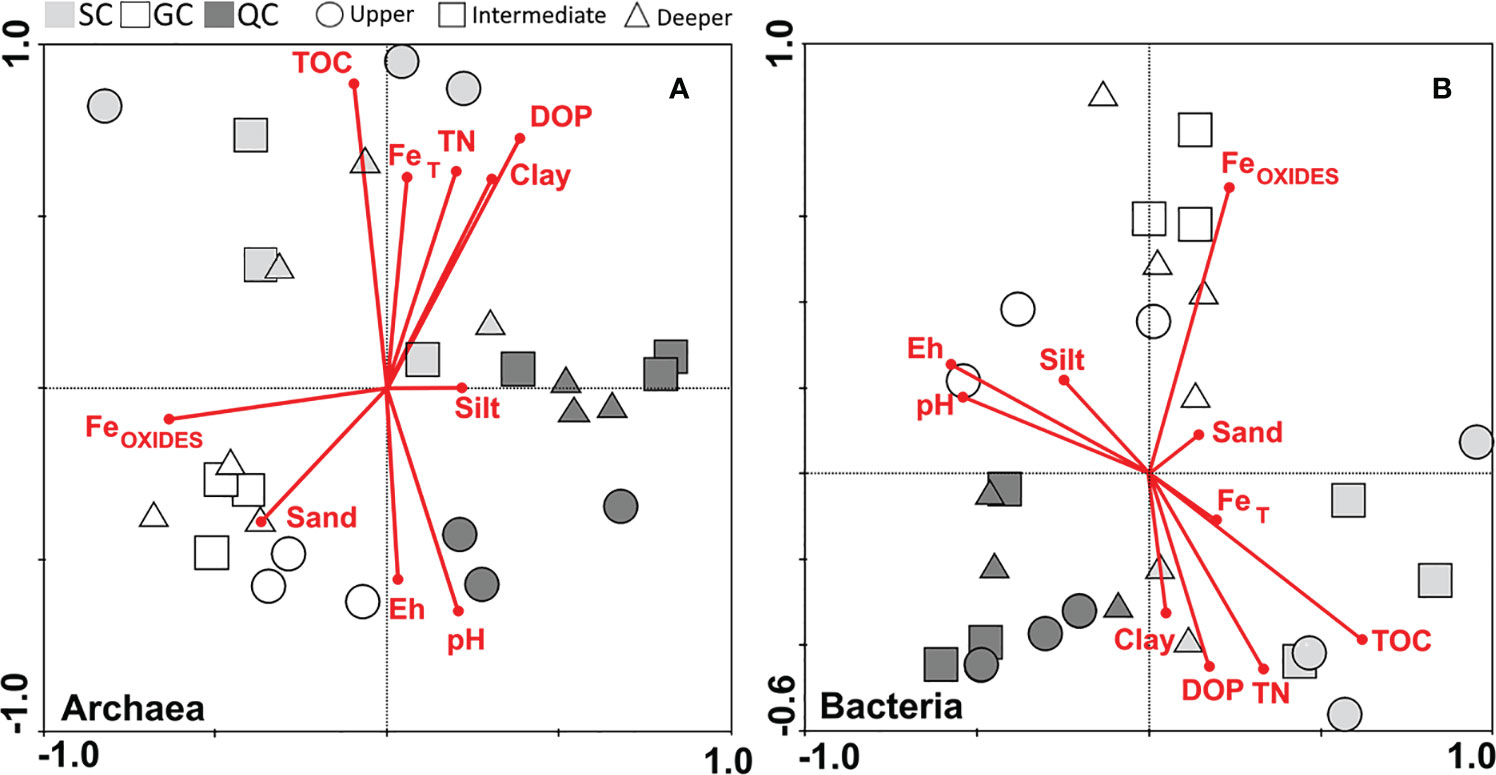
Figure 6 Results of redundancy detrended analysis regarding the environmental factors correlated to (A) archaea and (B) bacteria distribution and depth. TOC: Total organic carbon contents, TN: total nitrogen contents, FeOXIDES: the sum of FeFR, FeLP, and FeCR, FeT: pseudo total Fe, and DOP: degree of Fe pyritization.
4 Discussion
4.1 Physicochemical diversity of seagrass meadows and its effects on soil biogeochemical processes
Diverse studies have shown that soils of coastal wetlands (e.g., mangroves, salt marshes, and hypersaline tidal flats) can be very dynamic and spatially variable, resulting from an interaction between biotic (e.g., plant species and bioturbation; Araújo Júnior et al., 2012; Cabral et al., 2020), and abiotic factors (i.e., physiographic position, flooding frequency, geological bedrocks, and climate; Seybold et al., 2002; Du Laing et al., 2009; Albuquerque et al., 2014; Ferronato et al., 2016; Ferreira et al., 2022).
Our findings indicate that the distinct geo-environments and observed vegetation characteristics (e.g., plant biomass, density, and species) resulted in differentiated soil physico-chemical conditions at the studied sites (Figures 2 and 3; Table 1). Overall, the predominance of sand in the three studied sites reflects a high hydrodynamics energy which influences the particle size distribution in the studied sites. A more energetic hydrodynamic condition favours fine particle transportation and coarse particle accumulation (Flemming, 2000; Pejrup, 1988). Accordingly, it can be stated that a more energetic condition was recorded for soils from GC, where the seagrass meadows were in a beach area with coarse soil textures, and from estuarine soils at SC. Conversely, QC seagrass meadows are located in a closed estuary, i.e., a choked lagoon (Figure 1; Toldo Jr. et al., 2000; Lanari and Copertino, 2017), and thus present characteristics of a slightly lower energetic environment according to Flemming (2000) (i.e., higher contents of fine particles such as silt and clay; Figure 2; Table 1).
On the other hand, SC soils present finer soil texture (mean clay content: 14.1 ± 2.3%; Table 1), mainly at the soil surface, compared to GC soils. These characteristics can be attributed to the increased plant biomass within the meadow in SC, which has developed over coarser soils (Figure 1), which attenuates the effects of turbulence and wave action, promoting sedimentation of finer suspended particles and decreasing its resuspension in the upper soil layers (Gacia et al., 1999; Ondiviela et al., 2014). On the other hand, specifically for the QC samples, the higher sand contents on the upper soil layers (down to 10 cm depth) may result from a recently enhanced erosive event that may have delivered coarse particles to the lagoon (Toldo Jr. et al., 2000).
The contrasting geo-environments reflect significantly distinct redox conditions (Figure 3). For example, at the SC coast, anoxic conditions (Eh< +100 mV; Otero et al., 2009) were predominant, whereas sub-oxic conditions (+100 mV< Eh< +350 mV; Otero et al., 2009) predominate at GC and QC, despite anoxic conditions recorded at deeper layers (deeper than 30 cm) of the QC (Figure 3). Furthermore, despite the SC and GC seagrass meadows presenting similar plant species (H. wrightii; Figure 1), the redox conditions recorded for the GC were closely related to the QC (vegetated by Ruppia maritima; Figure 3). This fact highlights that seagrass plant species do not solely drive redox conditions, since same plant species may present distinct redox conditions. Additionally, compared to seagrass meadows from other sites (Table 3), the redox potentials at GC and QC were consistently higher, indicating the importance of abiotic factors (e.g., particle size distribution, hydrodynamic) for seagrass meadows’ soils characteristics.
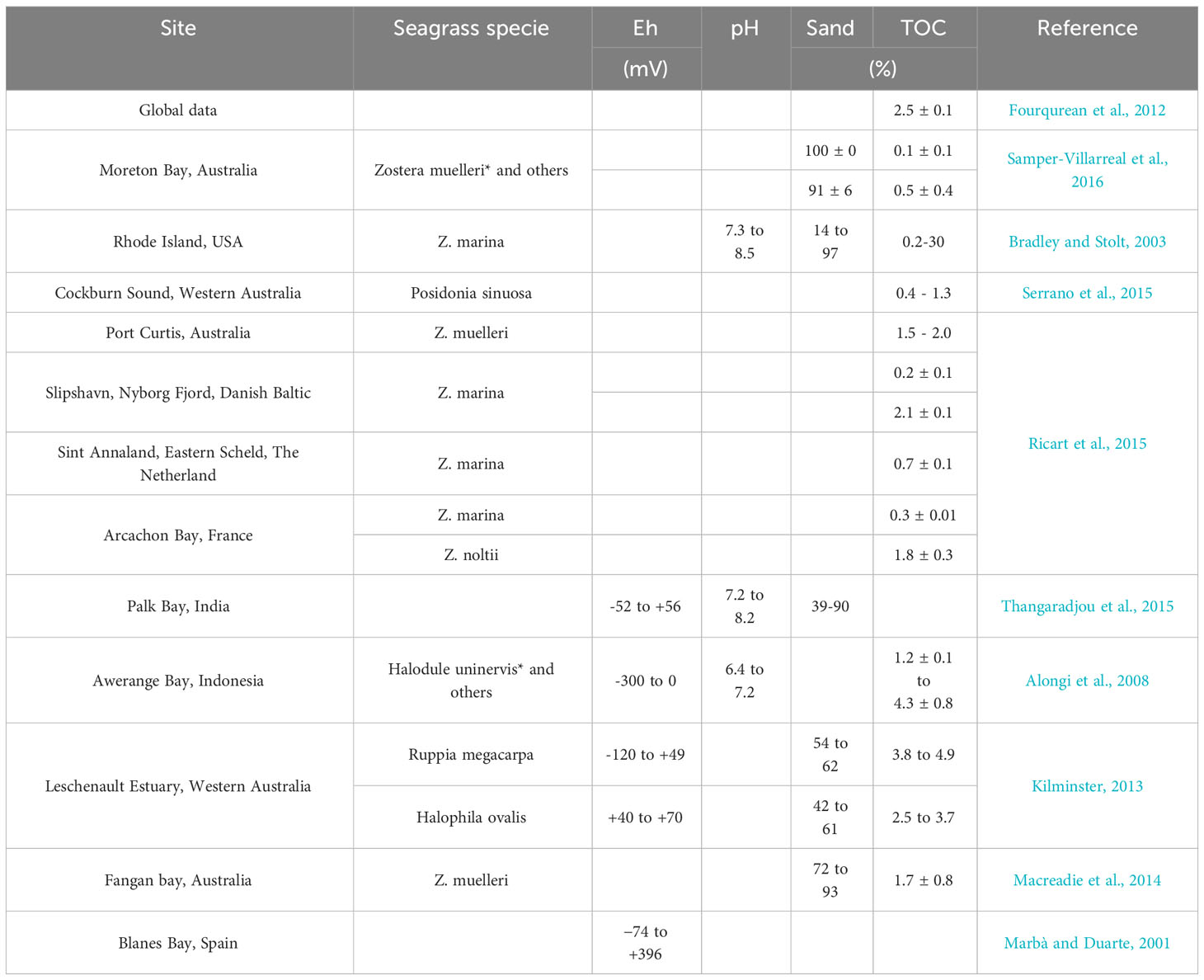
Table 3 Physicochemical (Eh and pH), sand, and total organic carbon (TOC) contents quantified for different seagrass meadows around the globe.
In this sense, the different plant biomass among sites (Figure 1) and the potentially higher input of labile organic matter from the surrounding mangroves and seagrass meadows in the SC soil produced lower Eh values. This explanation corroborates the opposite association observed in the discriminant analysis (Figure 5) and the significant negative correlation between TOC and Eh (Figure 7A). As labile organic matter is produced, exuded, or trapped by seagrasses vegetation, there is an intensification of the microbial respiration processes (Kaldy, 2012; Barrón et al., 2014), depleting the dissolved oxygen concentrations, leading to the consumption of other electron acceptors substituting O2 and resulting in lower Eh values (Canfield et al., 1993; Miller et al., 2007).
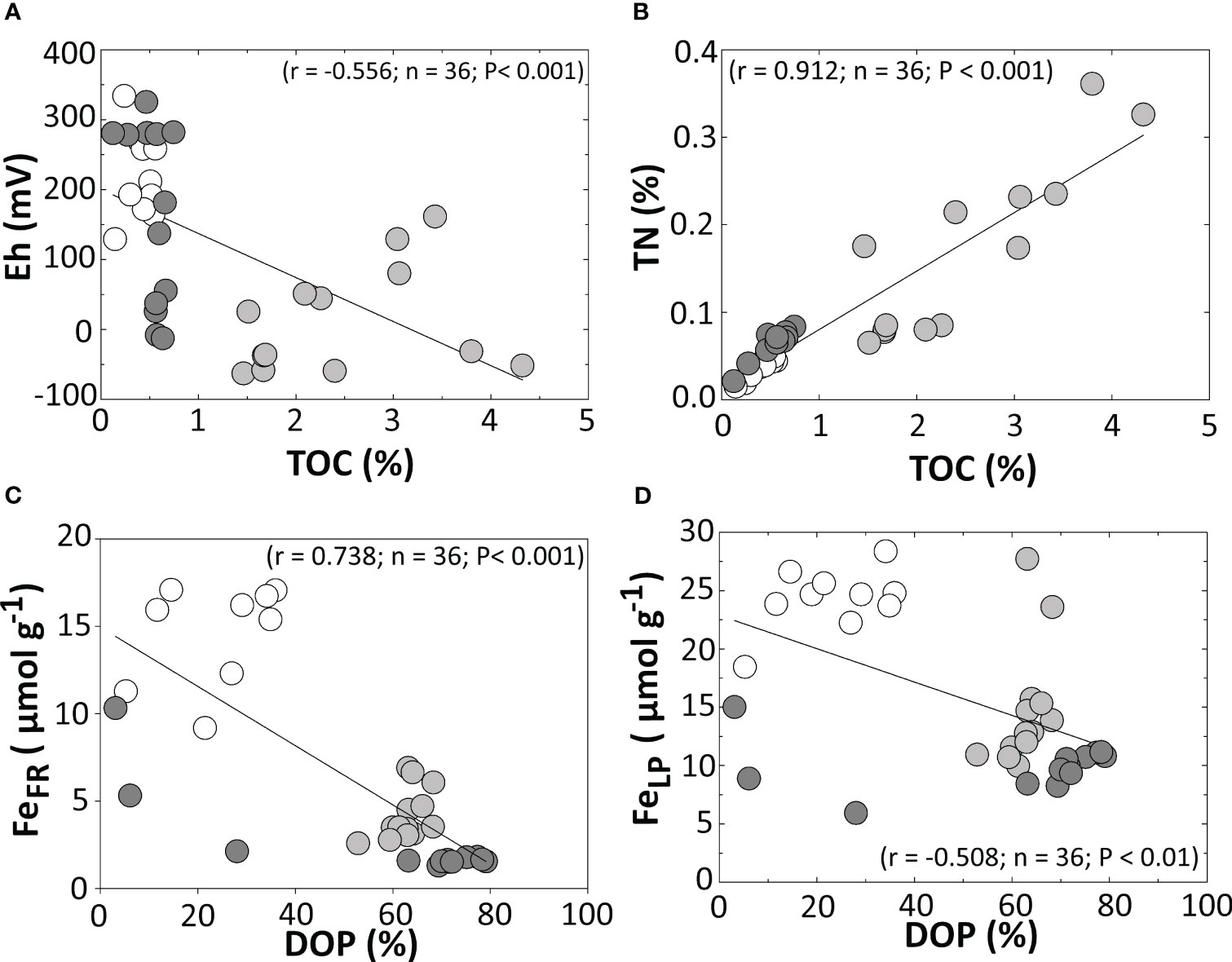
Figure 7 Spearman’s correlations between (A) TOC and Eh, (B) and TN, and between (C) DOP and FeFR, and (D) FeLP.
On the other hand, it must be considered the role of seagrass plants diffusing photosynthetic-generated O2 to protect the basal meristem from the damages arising from sulfides (Brodersen et al., 2015). This fact would explain the higher Eh values found in the uppermost soil layers from the GC and QC (Figure 3B). These roots have an oxidative effect resulting from an effective photosynthetic system (Olsen et al., 2016), and its well-developed aerenchyma enables rapid gas diffusion to roots, rhizomes, basal meristem, and soil (Koren et al., 2015). However, the oxidation of the seagrass rhizosphere can be diminished by an intense soil microbial metabolism, which rapidly consumes the diffused oxygen. As extensive mangrove forest borders the seagrass meadows at the SC sampling site, an intensification of the labile organic matter input (dissolved and particulate; Bouillon et al., 2007; Maher et al., 2013), stimulating microbial respiration, may have prevented the establishment of sub-oxic and oxic conditions at the upper-most soil layers as occurred at GC and QC coasts (Figure 3B).
The circumneutral pH values recorded for the studied sites (Figure 3A) are also related to the reductive processes of Fe and sulfate reduction in these soils. Both geochemical processes consume H+ or produce alkalinity (Equation 1 and 2; Otero et al., 2009). Conversely, the lower pH values at the upper layers of SC (0-10 cm depth) may result from intense rhizospheric activity since the higher roots’ density may result in higher organic acid exudation to increase nutrients bioavailability, especially phosphorus (Long et al., 2008).
The soils’ organic C and N contents were significantly higher on the SC, presumably derived from the higher plant biomass and the input from surrounding mangroves (Figure 1). The higher C:N ratio recorded for the SC coast (Figure 3) supports a hypothesis for C inputs from mangrove forests bordering the seagrass (Kennedy et al., 2010; Garcias-Bonet et al., 2019). Furthermore, there is a significant correlation between TOC and TN for all studied sites (Figure 7B), also represented by the strong association of the TOC and TN by the discriminant analysis results (Figure 5).
This association indicates that the soil organic matter is the source of N in these areas and is possibly attributed to the N fixation and assimilation processes promoted by sulfate-reducing bacteria (Welsh, 2000; Mohr et al., 2021). Additionally, the higher TOC content in the SC results from the less energetic metabolic pathway, corroborated by the lower Eh recorded in this site (Figure 3B). Thus, the intensity of anaerobic microbial metabolism associated with primary productivity may vary and is responsible for the C accumulation in seagrass meadow soils. In this case, the lower metabolic yield (e.g., SC) promotes soil organic matter accumulation, generally with low humification and unsaturation degree but enriched in H, N, and alcoholic and methoxyl groups (Neue et al., 1997; Kristensen et al., 2008).
Compared to seagrass meadows located in other parts of the world, the TOC contents were lower than the global average (average: 2.5%, median 1.8%; Fourqurean et al., 2012; Armitage and Fourqurean, 2016) but comparable to other sites around the globe (Table 3). However, a marked variability was observed between the studied sites, probably associated with the interaction of multiple environmental factors (e.g., seagrass species, landscape configuration, and soil redox conditions; Ricart et al., 2015; Ricart et al., 2020). However, our study evidence that geo-environmental factors controlling microbial metabolisms may be more relevant for C accumulation than plant species since the meadows formed by the same species present significant TOC contents (e.g., SC and GC). Additionally, TOC contents were a significant variable for differentiating microbial communities among studied sites (Figure 7). Thus, more studies must be conducted to improve comprehension of the spatial variability of seagrass meadows, improving the accuracy of the C stored in these ecosystems (Ricart et al., 2020), and how the effects of organic C control microbial communities.
4.2 Fe and S dynamic and metabolic pathways
Despite the differentiated geological setting of the studied sites, with the influence of granitic-gneiss rocks at the GC (Valeriano et al., 2016) and the predominance of quaternary sandy deposits at the SC and QC (Toldo Jr. et al., 2000; Vilas Bôas et al., 2001), the FeT contents did not differ statistically (Table 1). However, the Fe fractionating presented significant differences among the sites. Accordingly, the intensity of Fe and S metabolic pathways contrasted among the studied sites.
The seagrass meadow located at the SC differed from other sites by the significantly higher DOP values, which remained above 60% (Figure 4). Under the anoxic conditions at the SC (Table 1), microbiologically mediated Fe3+ reduction decreased Fe oxyhydroxide contents while increasing the soluble Fe2+ and Fe associated with sulfides (e.g., pyrite). On the other hand, at GC, the higher Eh values indicate an unfavourable environment for Fe reduction (i.e., oxic conditions) and the maintenance of Fe in oxyhydroxide forms (Figure 4). In such environments with redox oscillations between suboxic to oxic and circumneutral pH conditions (e.g., GC; Table 1), soluble Fe2+ is oxidized to Fe3+ and precipitated as oxyhydroxides (Ferreira et al., 2021; Ferreira et al., 2022) For the QC, fairly high DOP values occurred (>60%, especially at soil layers deeper than 10 cm; Figure 4) despite the high redox potentials (suboxic environment). In this case, the presence of pyrite could result from former (or transient) environmental conditions favourable to pyrite formation (Ferreira et al., 2015). Remnant pyrites may be protected by Fe oxyhydroxide coatings, preventing complete pyrite oxidation (Huminicki and Rimstidt, 2009). Furthermore, the presence of poorly crystalline Fe oxyhydroxides, especially FeLP, at the upper layers of GC and QC could be considered a result of the oxidation of sulfides by the rhizosphere (Azzoni et al., 2001; Brodersen et al., 2015) since lepidocrocite is the main product of Fe oxidation in saline environments (Equation 3; Otero et al., 2009).
The higher content of crystalline Fe oxyhydroxides (i.e., FeCR) at the GC could also explain the lower DOP registered at this site since crystalline Fe forms are less prone to reduction due to their mineralogical features such as lower surface area, reactivity, and crystal structure (i.e., rhombohedral and hexagonal or irregular plates) when compared to poorly crystalline Fe oxyhydroxides (Cornell and Schwertmann, 2003). Conversely, the higher susceptibility of poorly crystalline Fe oxyhydroxides to microbial Fe reduction could also explain the negative correlation between DOP vs. FeFR and vs. FeLP (Figures 5, 7C, D).
Moreover, the AVS and AVS-Fe contents also varied among the studied geo-environments (Figures 3F, G), corroborating its effects on Fe and S dynamics. These fractions correspond to a complex mixture of aqueous H2S and its dissociated form, Fe-S complexes, polysulfides, and solid-phase mackinawite and greigite (Rickard et al., 2017), with significant ecological importance once it may control trace metals dynamics and bioavailability (MaChado et al., 2010). Thus, the higher AVS content in the GC compared to SC and QC reinforces the different intensities of Fe and S metabolic pathways among the studied sites. Furthermore, due to its phytotoxicity, high sulfide production has been associated with declining seagrass meadows(Holmer et al., 2005). Accordingly, the higher AVS content at the GC, especially on surface soil layers, may be related to the lower plant density at this site (Figure 1), especially compared to the SC vegetated by the same plant species, since lower plant density and lower organic C content prevents Fe oxyhydroxides reduction (and lower DOP).
Regarding microbial communities, we observed a positive correlation with higher FeOXIDES and sand contents, higher Eh values, and negatively correlated with TOC at GC site (Figure 7). This fact indicates that the low plant density and high Fe inputs from the Fe-rich geological surroundings affected the microbial structure. A recent study has reported that forming distinct Fe-cycling bacterial genera (e.g., Lewinella and Woeseia) communities in seagrass meadows positively correlates with greater Fe contents in its soils (Martin et al., 2022). On the other hand, previous studies have reported that sandy sediments (e.g., GC) with high sulfide production favour the dominance of archaea communities (Llobet-Brossa et al., 2002; Buhring et al., 2005). Specifically, high sulfide production increases genes related to sulfate reduction in a Posidonia spp. ecosystem (Fraser et al., 2023).
The higher plant cover in SC possibly changes microbial activity in the seagrass rhizosphere (Jankowska et al., 2015) since we observed a clear cluster in microbial communities, mainly driven by clay content, TOC, TN, and DOP (Figure 6). Accordingly, previous studies have reported that gross primary production in tropical seagrass bacteria communities increases the amount of C available for sulfate-reducing bacteria metabolism (Pollard and Moriarty, 1991; Williams et al., 2009). Moreover, Zhang et al. (2022) revealed that higher clay contents play an important role in seagrass soil biogeochemistry. For example, these authors reported that seagrass soils with a predominance of fine particles have a higher capacity for root exudate retention, increasing its concentration around the roots and enhancing the abundance of sulfate-reducing bacteria (see DOP values in Table 1). Interestingly, in a Thalassia hemprichii system it was demonstrated that induced carbon addition changed the bacterial community, mainly those functional groups related to N cycle (Zhang et al., 2021). A higher TOC content in SC could increase N uptake and, consequently, boost photosynthetic performance of seagrass and modified rhizosphere microbial communities.
Changes in seagrass microbial communities seem to be context-dependent since microbial groups changed according to geographical location. More importantly, the same plant species (H. wrightii. at SC and GC) shapes different microbial communities in the soil (Figure 6). Thus, probably seagrass microbial communities change according to plant type, but microbial rhizosphere core strongly depends on soil geochemical properties (Cúcio et al., 2016). For example, Vogel et al. (2021) demonstrated that the main drivers of microbial community structure in Thalassia testudinum seagrass were local geo-environmental conditions.
Therefore, our findings reveal that seagrass meadows soils at contrasting geo-environments (e.g., climate, geology, and plant species and cover) present different intensities of Fe and sulfate reduction, ultimately shaping different microbiological communities. More importantly, we provide evidence that bacteria and archaea communities responded similarly to geo-environmental conditions, indicating that both could be equally important to microbial activity and nutrient cycling in seagrass ecosystems. These results shed light on the knowledge gap about the intensities of geochemical processes involving Fe and S dynamic and metabolic pathways in seagrass meadows’ soils.
5 Conclusions
In this study, we identify that seagrass meadows are a heterogeneous ecosystem in which the variability of soil composition affects the intensities of geochemical processes due to distinct geo-environments (e.g., climate, geology, salinity, plant species and coverage, and anthropic influence). For example, in soils under higher plant cover that enhances the C contents and geology that favors higher Fe contents, there are higher contents of fine particles (e.g., clay), an abundance of sulfate-reducing bacteria, and microbial-mediated processes involving Fe and S. This environment favors metabolic pathways with low oxidation rates (e.g., sulfate reduction), Fe sulfides formation, and ultimately, high-capacity C sequestration. On the other hand, in seagrass meadow soils with higher contents of sand and low plant density, a more oxidizing environment is established that favors Fe oxyhydroxides and AVS formation. This contrasting environment presents a lower capacity for organic C accumulation and contaminants immobilization. In addition, the differentiated geo-environmental conditions markedly influenced microbial communities, even when vegetated with the same plant species. Our findings revealed novel insights into the heterogeneity of seagrass meadow soils and a better understanding of the biogeochemical processes inherent to these ecosystems.
Data availability statement
The raw data supporting the conclusions of this article will be made available by the authors, without undue reservation.
Author contributions
GN and TF: Funding acquisition, Investigation, Formal analysis, Conceptualization, Methodology, Validation, Writing original draft, review and editing. PA, HQ, AP, MC, DG, WZ, JS, FA, XO: Investigation, Formal analysis, Data validation, Writing - original draft and editing. All authors contributed to the article and approved the submitted version.
Funding
This work received financial support provided by the National Council for Scientific and Technological Development (CNPq, grants number 430010/2018-4 and 305996/2018-5 to TF), Coordination of Superior Level Staff Improvement (CAPES, Finance Code 001), São Paulo Research Foundation (FAPESP, grants number 2014/11778-5, 2016/21026-6, and 2021/00221-3), and FUNCAP research fellow (Chief Scientist Program). Furthermore, this study is part of the doctoral thesis of the author GN and is in accordance with the copyright terms of use.
Conflict of interest
The authors declare that the research was conducted in the absence of any commercial or financial relationships that could be construed as a potential conflict of interest.
Publisher's note
All claims expressed in this article are solely those of the authors and do not necessarily represent those of their affiliated organizations, or those of the publisher, the editors and the reviewers. Any product that may be evaluated in this article, or claim that may be made by its manufacturer, is not guaranteed or endorsed by the publisher.
References
Albuquerque A. G. B. M., Ferreira T. O. O., Nóbrega G. N. N., Romero R. E. E., Júnior V. S. S., Meireles A. J. A., et al. (2014). Soil genesis on hypersaline tidal flats (apicum ecosystem) in a tropical semi-arid estuary (Ceará, Brazil). Soil Res. 52, 140. doi: 10.1071/SR13179
Allen H. E., Fu G., Deng B. (1993). Analysis of acid-volatile sulfide (AVS) and simultaneously extracted metals (SEM) for the estimation of potential toxicity in aquatic sediments. Environ. Toxicol. Chem. 12, 1441–1453. doi: 10.1002/etc.5620120812
Alongi D. M. (2008). Mangrove forests: Resilience, protection from tsunamis, and responses to global climate change. Estuarine, Coastal and Shelf Science 76, 1–13. doi: 10.1016/j.ecss.2007.08.024.
Alvares C. A., Stape J. L., Sentelhas P. C., de Moraes Gonçalves J. L., Sparovek G. (2013). Köppen’s climate classification map for Brazil. Meteorol. Z. 22, 711–728. doi: 10.1127/0941-2948/2013/0507
Arai M. (2006). A grande elevação eustática do Mioceno e sua influência na origem do Grupo Barreiras. Geol. USP Série Científica 6, 01–06. doi: 10.5327/S1519-874X2006000300002
Araújo Júnior J. M. C., Otero X. L., Marques A. G. B., Nóbrega G. N., Silva J. R. F., Ferreira T. O. (2012). Selective geochemistry of iron in mangrove soils in a semiarid tropical climate: effects of the burrowing activity of the crabs Ucides cordatus and Uca maracoani. Geo-Marine Lett. 32, 289–300. doi: 10.1007/s00367-011-0268-5
Armitage A. R., Fourqurean J. W. (2016). Carbon storage in seagrass soils: long-term nutrient history exceeds the effects of near-term nutrient enrichment. Biogeosciences 13, 313–321. doi: 10.5194/bg-13-313-2016
Azzoni R., Giordani G., Bartoli M., Welsh D. T., Viaroli P. (2001). Iron, sulphur and phosphorus cycling in the rhizosphere sediments of a eutrophic Ruppia cirrhosa meadow (Valle Smarlacca, Italy). J. Sea Res. 45, 15–26. doi: 10.1016/S1385-1101(00)00056-3
Azzoni R., Giordani G., Viaroli P. (2005). Iron–sulphur–phosphorus interactions: implications for sediment buffering capacity in a mediterranean eutrophic lagoon (Sacca di Goro, Italy). Hydrobiologia 550, 131–148. doi: 10.1007/s10750-005-4369-x
Barbier E. B., Hacker S. D., Kennedy C., Koch E. W., Stier A. C., Silliman B. R. (2011). The value of estuarine and coastal ecosystem services. Ecol. Monogr. 81, 169–193. doi: 10.1890/10-1510.1
Barrón C., Apostolaki E. T., Duarte C. M. (2014). Dissolved organic carbon fluxes by seagrass meadows and macroalgal beds. Front. Mar. Sci. 1. doi: 10.3389/fmars.2014.00042. Article 42.
Bouillon S., Dehairs F., Velimirov B., Abril G., Borges A. V. (2007). Dynamics of organic and inorganic carbon across contiguous mangrove and seagrass systems (Gazi Bay, Kenya). J. Geophys. Res. 112, G02018. doi: 10.1029/2006JG000325
Bradley M. P., Stolt M. H. (2003). Subaqueous soil-landscape relationships in a rhode island estuary. Soil Sci. Soc Am. J. 67, 1487–1495. doi: 10.2136/sssaj2003.1487
Brodersen K. E., Koren K., Moßhammer M., Ralph P. J., Kühl M., Santner J. (2017). Seagrass-mediated phosphorus and iron solubilization in tropical sediments. Environ. Sci. Technol. 51, 14155–14163. doi: 10.1021/acs.est.7b03878
Brodersen K. E., Nielsen D. A., Ralph P. J., Kühl M. (2015). Oxic microshield and local pH enhancement protects Zostera muelleri from sediment derived hydrogen sulphide. New Phytol. 205, 1264–1276. doi: 10.1111/nph.13124
Brodersen K. E., Siboni N., Nielsen D. A., Pernice M., Ralph P. J., Seymour J., et al. (2018). Seagrass rhizosphere microenvironment alters plant-associated microbial community composition. Environ. Microbiol. 20, 2854–2864. doi: 10.1111/1462-2920.14245
Buhring S. I., Elvert M., Witte U. (2005). The microbial community structure of different permeable sandy sediments characterized by the investigation of bacterial fatty acids and fluorescence in situ hybridization. Environ. Microbiol. 7, 281–293. doi: 10.1111/j.1462-2920.2004.00710.x
Cabral R. L., Ferreira T. O., Nóbrega G. N., Barcellos D., Roiloa S. R., Zandavalli R. B., et al. (2020). How do plants and climatic conditions control soil properties in hypersaline tidal flats? Appl. Sci. 10, 7624. doi: 10.3390/app10217624
Canfield D. E., Thamdrup B., Hansen J. W. (1993). The anaerobic degradation of organic matter in Danish coastal sediments: Iron reduction, manganese reduction, and sulfate reduction. Geochim. Cosmochim. Acta 57, 3867–3883. doi: 10.1016/0016-7037(93)90340-3
Charette M., Smith W. (2010). The volume of earth’s ocean. Oceanography 23, 112–114. doi: 10.5670/oceanog.2010.51
Cline J. D. (1969). Spectrophotometric determination of hydrogen sulfide in natural waters. Limnol. Oceanogr. 14, 454–458. doi: 10.4319/lo.1969.14.3.0454
Connolly R., Hindell J., Gorman D. (2005). Seagrass and epiphytic algae support nutrition of a fisheries species, Sillago schomburgkii, in adjacent intertidal habitats. Mar. Ecol. Prog. Ser. 286, 69–79. doi: 10.3354/meps286069
Copertino M. S., Creed J. C., Lanari M. O., Magalhães K., Barros K., Lana P. C., et al. (2016). Seagrass and Submerged Aquatic Vegetation (VAS) Habitats off the Coast of Brazil: state of knowledge, conservation and main threats. Braz. J. Oceanogr. 64, 53–80. doi: 10.1590/S1679-875920161036064sp2
Cornell R. M., Schwertmann U. (2003). The Iron Oxides: Structure, Reactions, Occurences and Uses (Weinheim, Germany: Wiley). doi: 10.1002/3527602097
Costanza R., de Groot R., Sutton P., van der Ploeg S., Anderson S. J., Kubiszewski I., et al. (2014). Changes in the global value of ecosystem services. Glob. Environ. Change 26, 152–158. doi: 10.1016/j.gloenvcha.2014.04.002
Cúcio C., Engelen A. H., Costa R., Muyzer G. (2016). Rhizosphere microbiomes of European + seagrasses are selected by the plant, but are not species specific. Front. Microbiol. 7. doi: 10.3389/fmicb.2016.00440
Cui L., Jiang Z., Huang X., Liu S., Wu Y., Fan M. (2021). Decade changes of the food web structure in tropical seagrass meadow: Implication of eutrophication effects. Mar. pollut. Bull. 173, 113122. doi: 10.1016/j.marpolbul.2021.113122
de la Torre-Castro M., Rönnbäck P. (2004). Links between humans and seagrasses—an example from tropical East Africa. Ocean Coast. Manage. 47, 361–387. doi: 10.1016/j.ocecoaman.2004.07.005
Delgard M. L., Deflandre B., Kochoni E., Avaro J., Cesbron F., Bichon S., et al. (2016). Biogeochemistry of dissolved inorganic carbon and nutrients in seagrass (Zostera noltei) sediments at high and low biomass. Estuar. Coast. Shelf Sci. 179, 12–22. doi: 10.1016/j.ecss.2016.01.012
Duarte C. M., Chiscano C. L. (1999). Seagrass biomass and production: a reassessment. Aquat. Bot. 65, 159–174. doi: 10.1016/S0304-3770(99)00038-8
Duarte C. M., Marbà N., Gacia E., Fourqurean J. W., Beggins J., Barrón C., et al. (2010). Seagrass community metabolism: Assessing the carbon sink capacity of seagrass meadows. Global Biogeochem. Cycles 24, n/a–n/a. doi: 10.1029/2010GB003793
Du Laing G., Rinklebe J., Vandecasteele B., Meers E., Tack F. M. G. (2009). Trace metal behaviour in estuarine and riverine floodplain soils and sediments: A review. Sci. Total Environ. 407, 3972–3985. doi: 10.1016/j.scitotenv.2008.07.025
Erich E., Drohan P. J. (2012). Genesis of freshwater subaqueous soils following flooding of a subaerial landscape. Geoderma 179–180, 53–62. doi: 10.1016/j.geoderma.2012.02.004
Fernandes M., Bryars S., Mount G., Miller D. (2009). Seagrasses as a sink for wastewater nitrogen: The case of the Adelaide metropolitan coast. Mar. pollut. Bull. 58, 303–308. doi: 10.1016/j.marpolbul.2008.10.006
Ferreira T. O., Nóbrega G. N., Albuquerque A. G. B. M., Sartor L. R., Gomes I. S., Artur A. G., et al. (2015). Pyrite as a proxy for the identification of former coastal lagoons in semiarid NE Brazil. Geo-Marine Lett. 35, 355–366. doi: 10.1007/s00367-015-0412-8
Ferreira T. O., Nóbrega G. N., Queiroz H. M., de Souza Júnior V. S., Barcellos D., Ferreira A. D., et al. (2021). Windsock behavior: climatic control on iron biogeochemistry in tropical mangroves. Biogeochemistry 156, 437–452. doi: 10.1007/s10533-021-00858-9
Ferreira T. O., Queiroz H. M., Nóbrega G. N., de Souza Júnior V. S., Barcellos D., Ferreira A. D., et al. (2022). Litho-climatic characteristics and its control over mangrove soil geochemistry: A macro-scale approach. Sci. Total Environ. 811, 152152. doi: 10.1016/j.scitotenv.2021.152152
Ferronato C., Falsone G., Natale M., Zannoni D., Buscaroli A., Vianello G., et al. (2016). Chemical and pedological features of subaqueous and hydromorphic soils along a hydrosequence within a coastal system (San Vitale Park, Northern Italy). Geoderma 265, 141–151. doi: 10.1016/j.geoderma.2015.11.018
Flemming B. (2000). A revised textural classification of gravel-free muddy sediments on the basis of ternary diagrams. Cont. Shelf Res. 20, 1125–1137. doi: 10.1016/S0278-4343(00)00015-7
Fortin D., Leppard G. G., Tessier A. (1993). Characteristics of lacustrine diagenetic iron oxyhydroxides. Geochim. Cosmochim. Acta 57, 4391–4404. doi: 10.1016/0016-7037(93)90490-N
Fourqurean J. W., Duarte C. M., Kennedy H., Marbà N., Holmer M., Mateo M. A., et al. (2012). Seagrass ecosystems as a globally significant carbon stock. Nat. Geosci. 5, 505–509. doi: 10.1038/ngeo1477
Fraser M. W., Martin B. C., Wong H. L., Burns B. P., Kendrick G. A. (2023). Sulfide intrusion in a habitat forming seagrass can be predicted from relative abundance of sulfur cycling genes in sediments. Sci. Total Environ. 864, 161144. doi: 10.1016/j.scitotenv.2022.161144
Gacia E., Granata T., Duarte C. (1999). An approach to measurement of particle flux and sediment retention within seagrass (Posidonia oceanica) meadows. Aquat. Bot. 65, 255–268. doi: 10.1016/S0304-3770(99)00044-3
Garcias-Bonet N., Delgado-Huertas A., Carrillo-de-Albornoz P., Anton A., Almahasheer H., Marbà N., et al. (2019). Carbon and nitrogen concentrations, stocks, and isotopic compositions in red sea seagrass and mangrove sediments. Front. Mar. Sci. 6. doi: 10.3389/fmars.2019.00267
Gee G. W., Bauder J. W. (1986). Particle-size analysis. Methods Soil Anal. Part 1—Physical Mineral. Methods 1, 383–411. doi: 10.2136/sssabookser5.1.2ed.c15
Gorman D., Sumida P. Y. G., Figueira R. C. L., Turra A. (2020). Improving soil carbon estimates of mudflats in Araçá Bay using spatial models that consider riverine input, wave exposure and biogeochemistry. Estuar. Coast. Shelf Sci. 238, 106734. doi: 10.1016/j.ecss.2020.106734
Hammer Ø., Harper D. A. T., Ryan P. D. (2001). Past: paleontological statistics software package for education and data analysis. Paleontol. Electron. 4, 9 pp.
Heuer H., Krsek M., Baker P., Smalla K., Wellington E. M. H. (1997). Analysis of actinomycete communities by specific amplification of genes encoding 16S rRNA and gel-electrophoretic separation in denaturing gradients. Appl. Environ. Microbiol. 63, 3233–3241. doi: 10.1128/aem.63.8.3233-3241.1997
Holmer M., Duarte C. M., Marbá N. (2005). Iron additions reduce sulfate reduction rates and improve seagrass growth on organic-enriched carbonate sediments. Ecosystems 8, 721–730. doi: 10.1007/s10021-003-0180-6
Howard J., Hoyt S., Isensee K., Telszewski M., Pidgeon E., Telszewski M. (2014). Coastal blue carbon: methods for assessing carbon stocks and emissions factors in mangroves, tidal salt marshes, and seagrasses (Arlington, VA, USA: Conservation International, Intergovernmental Oceanographic Commission of UNESCO, International Union for Conservation of Nature, Arlington, VA, USA).
Huerta-Díaz M. A., Morse J. W. (1990). A quantitative method for determination of trace metal concentrations in sedimentary pyrite. Mar. Chem. 29, 119–144. doi: 10.1016/0304-4203(90)90009-2
Huerta-Diaz M. A., Morse J. W. (1992). Pyritization of trace metals in anoxic marine sediments. Geochim. Cosmochim. Acta 56, 2681–2702. doi: 10.1016/0016-7037(92)90353-K
Huminicki D. M. C., Rimstidt J. D. (2009). Iron oxyhydroxide coating of pyrite for acid mine drainage control. Appl. Geochemistry 24, 1626–1634. doi: 10.1016/j.apgeochem.2009.04.032
Jankowska E., Jankowska K., Włodarska-Kowalczuk M. (2015). Seagrass vegetation and meiofauna enhance the bacterial abundance in the Baltic Sea sediments (Puck Bay). Environ. Sci. pollut. Res. 22, 14372–14378. doi: 10.1007/s11356-015-5049-7
Kaal J., Serrano O., Nierop K. G. J., Schellekens J., Martínez A., Mateo M.-Á (2016). Molecular composition of plant parts and sediment organic matter in a Mediterranean seagrass (Posidonia oceanica) mat. Aquat. Bot. 133, 50–61. doi: 10.1016/j.aquabot.2016.05.009
Kaldy J. (2012). Influence of light, temperature and salinity on dissolved organic carbon exudation rates in Zostera marina L. Aquat. Biosyst. 8, 19. doi: 10.1186/2046-9063-8-19
Kennedy H., Beggins J., Duarte C. M., Fourqurean J. W., Holmer M., Marbà N., et al. (2010). Seagrass sediments as a global carbon sink: Isotopic constraints. Global Biogeochem. Cycles 24, n/a–n/a. doi: 10.1029/2010GB003848
Kilminster K. (2013). Trace element content of seagrasses in the Leschenault Estuary, Western Australia. Mar. pollut. Bull. 73, 381–388. doi: 10.1016/j.marpolbul.2013.05.030
Koren K., Brodersen K. E., Jakobsen S. L., Kühl M. (2015). Optical sensor nanoparticles in artificial sediments–A new tool to visualize O2 dynamics around the rhizome and roots of seagrasses. Environ. Sci. Technol. 49, 2286–2292. doi: 10.1021/es505734b
Kristensen E., Bouillon S., Dittmar T., Marchand C. (2008). Organic carbon dynamics in mangrove ecosystems: A review. Aquat. Bot. 89, 201–219. doi: 10.1016/j.aquabot.2007.12.005
Lanari M., Copertino M. (2017). Drift macroalgae in the Patos Lagoon Estuary (southern Brazil): effects of climate, hydrology and wind action on the onset and magnitude of blooms. Mar. Biol. Res. 13, 36–47. doi: 10.1080/17451000.2016.1225957
Liquete C., Cid N., Lanzanova D., Grizzetti B., Reynaud A. (2016). Perspectives on the link between ecosystem services and biodiversity: The assessment of the nursery function. Ecol. Indic. 63, 249–257. doi: 10.1016/j.ecolind.2015.11.058
Llobet-Brossa E., Rabus R., Böttcher M., Könneke M., Finke N., Schramm A., et al. (2002). Community structure and activity of sulfate-reducing bacteria in an intertidal surface sediment: a multi-method approach. Aquat. Microb. Ecol. 29, 211–226. doi: 10.3354/ame029211
Long M. H., McGlathery K. J., Zieman J. C., Berg P. (2008). The role of organic acid exudates in liberating phosphorus from seagrass-vegetated carbonate sediments. Limnol. Oceanogr. 53, 2616–2626. doi: 10.4319/lo.2008.53.6.2616
Lozupone C. A., Hamady M., Kelley S. T., Knight R. (2007). Quantitative and qualitative β diversity measures lead to different insights into factors that structure microbial communities. Appl. Environ. Microbiol. 73, 1576–1585. doi: 10.1128/AEM.01996-06
MaChado W., Villar L. S., Monteiro F. F., Viana L. C. A., Santelli R. E. (2010). Relation of acid-volatile sulfides (AVS) with metals in sediments from eutrophicated estuaries: Is it limited by metal-to-AVS ratios? J. Soils Sediments 10, 1606–1610. doi: 10.1007/s11368-010-0297-0
Macreadie P. I., Baird M. E., Trevathan-Tackett S. M., Larkum A. W. D., Ralph P. J. (2014). Quantifying and modelling the carbon sequestration capacity of seagrass meadows – A critical assessment. Mar. pollut. Bull. 83, 430–439. doi: 10.1016/j.marpolbul.2013.07.038
Maher D. T., Santos I. R., Golsby-Smith L., Gleeson J., Eyre B. D. (2013). Groundwater-derived dissolved inorganic and organic carbon exports from a mangrove tidal creek: The missing mangrove carbon sink? Limnol. Oceanogr. 58, 475–488. doi: 10.4319/lo.2013.58.2.0475
Marbà N., Duarte C. (2001). Growth and sediment space occupation by seagrass Cymodocea nodosa roots. Mar. Ecol. Prog. Ser. 224, 291–298. doi: 10.3354/meps224291
Martin B. C., Middleton J. A., Skrzypek G., Kendrick G. A., Cosgrove J., Fraser M. W. (2022). Composition of seagrass root associated bacterial communities are linked to nutrients and heavy metal concentrations in an anthropogenically influenced estuary. Front. Mar. Sci. 8. doi: 10.3389/fmars.2021.768864
McKenzie L. J., Nordlund L. M., Jones B. L., Cullen-Unsworth L. C., Roelfsema C., Unsworth R. K. F. (2020). The global distribution of seagrass meadows. Environ. Res. Lett. 15, 074041. doi: 10.1088/1748-9326/ab7d06
Miller H. L., Meile C., Burd A. B. (2007). A novel 2D model of internal O2 dynamics and H2S intrusion in seagrasses. Ecol. Modell. 205, 365–380. doi: 10.1016/j.ecolmodel.2007.03.004
Minuzzi R. B., Sediyama G. C., Barbosa E., da M., Melo Júnior J.C.F. de (2007). Climatologia do comportamento do período chuvoso da região sudeste do Brasil. Rev. Bras. Meteorol. 22, 338–344. doi: 10.1590/S0102-77862007000300007
Mohr W., Lehnen N., Ahmerkamp S., Marchant H. K., Graf J. S., Tschitschko B., et al. (2021). Terrestrial-type nitrogen-fixing symbiosis between seagrass and a marine bacterium. Nature 600, 105–109. doi: 10.1038/s41586-021-04063-4
Neue H. U., Gaunt J. L., Wang Z. P., Becker-Heidmann P., Quijano C. (1997). Carbon in tropical wetlands. Geoderma 79, 163–185. doi: 10.1016/S0016-7061(97)00041-4
Nóbrega G. N., Otero X. L., Romero D. J., Queiroz H. M., Gorman D., Copertino M., et al. (2023). Masked diversity and contrasting soil processes in tropical seagrass meadows: the control of environmental settings. SOIL 9, 189–208. doi: 10.5194/soil-9-189-2023
Nordlund L. M., Koch E. W., Barbier E. B., Creed J. C. (2016). Seagrass ecosystem services and their variability across genera and geographical regions. PloS One 11, e0163091. doi: 10.1371/journal.pone.0163091
Olsen J. L., Rouzé P., Verhelst B., Lin Y.-C., Bayer T., Collen J., et al. (2016). The genome of the seagrass Zostera marina reveals angiosperm adaptation to the sea. Nature 530, 331–335. doi: 10.1038/nature16548
Ondiviela B., Losada I. J., Lara J. L., Maza M., Galván C., Bouma T. J., et al. (2014). The role of seagrasses in coastal protection in a changing climate. Coast. Eng. 87, 158–168. doi: 10.1016/j.coastaleng.2013.11.005
Orth R. J., Carruthers T. I. M. J. B., Dennison W. C., Carlos M., Fourqurean J. W., Jr K. L. H., et al. (2006). A global crisis for seagrass ecosystems. Bioscience 56, 987–996. doi: 10.1641/0006-3568(2006)56[987:AGCFSE]2.0.CO;2
Osterrieth M., Borrelli N., Alvarez M. F. F., Nóbrega G. N., MaChado W., Ferreira T. O. (2016). Iron biogeochemistry in Holocene palaeo and actual salt marshes in coastal areas of the Pampean Plain, Argentina. Environ. Earth Sci. 75, 672. doi: 10.1007/s12665-016-5506-8
Otero X. L., Ferreira T. O., Huerta-Díaz M. A., Partiti C. S. M., Souza V., Vidal-Torrado P., et al. (2009). Geochemistry of iron and manganese in soils and sediments of a mangrove system, Island of Pai Matos (Cananeia — SP, Brazil). Geoderma 148, 318–335. doi: 10.1016/j.geoderma.2008.10.016
Otero X. L., Guevara P., Sánchez M., López I., Queiroz H. M., Ferreira A., et al. (2023). Pyrites in a salt marsh-ria system: Quantification, morphology, and mobilization. Mar. Geol. 455, 106954. doi: 10.1016/j.margeo.2022.106954
Otero X. L., Macias F. (2002). Variation with depth and season in metal sulfides in salt marsh soils. Biogeochemistry 61, 247–268. doi: 10.1023/A:1020230213864
Ovreås L., Forney L., Daae F. L., Torsvik V. (1997). Distribution of bacterioplankton in meromictic Lake Saelenvannet, as determined by denaturing gradient gel electrophoresis of PCR-amplified gene fragments coding for 16S rRNA. Appl. Environ. Microbiol. 63, 3367–3373. doi: 10.1128/aem.63.9.3367-3373.1997
Passos T. R. G., Artur A. G., Nóbrega G. N., Otero X. L., Ferreira T. O. (2016). Comparison of the quantitative determination of soil organic carbon in coastal wetlands containing reduced forms of Fe and S. Geo-Marine Lett. 36, 223–233. doi: 10.1007/s00367-016-0437-7
Peel M. C., Finlayson B. L., McMahon T. A. (2007). Updated world map of the Köppen-Geiger climate classification. Hydrol. Earth Syst. Sci. 11, 1633–1644. doi: 10.5194/hess-11-1633-2007
Pejrup M. (1988). The Triangular Diagram Used for Classification of Estuarine Sediments: A New Approach. In: Tide-Influenced Sedimentary Environments and Facies (Dordrecht: Springer Netherlands), 289–300. doi: 10.1007/978-94-015-7762-5_21
Piñeiro-Juncal N., Kaal J., Moreira J. C. F., Martínez Cortizas A., Lambais M. R., Otero X. L., et al. (2020a). Cover loss in a seagrass Posidonia oceanica meadow accelerates soil organic matter turnover and alters soil prokaryotic communities. Org. Geochem. 151, 104140. doi: 10.1016/j.orggeochem.2020.104140
Piñeiro-Juncal N., Leiva-Dueñas C., Serrano O., Mateo M. Á., Martínez-Cortízas A. (2020b). Pedogenic processes in a Posidonia oceanica mat. Soil Syst. 4, 18. doi: 10.3390/soilsystems4020018
Pollard P. C., Moriarty D. J. W. (1991). Organic carbon decomposition, primary and bacterial productivity, and sulphate reduction, in tropical seagrass beds of the Gulf of Carpentaria, Australia. Mar. Ecol. Prog. Ser. 69, 149–159. doi: 10.3354/meps069149
Queiroz H. M., Nóbrega G. N., Otero X. L., Ferreira T. O. (2018). Are acid volatile sulfides (AVS) important trace metals sinks in semi-arid mangroves? Mar. pollut. Bull. 126, 318–322. doi: 10.1016/j.marpolbul.2017.11.020
Ramírez-Flandes S., González B., Ulloa O. (2019). Redox traits characterize the organization of global microbial communities. Proc. Natl. Acad. Sci. 116, 3630–3635. doi: 10.1073/pnas.1817554116
Reimann C., Filzmoser P., Garrett R. G., Dutter R. (2008). Statistical Data Analysis Explained (Chichester, UK, UK: Wiley). doi: 10.1002/9780470987605
Ricart A. M., York P. H., Bryant C. V., Rasheed M. A., Ierodiaconou D., Macreadie P. I. (2020). High variability of Blue Carbon storage in seagrass meadows at the estuary scale. Sci. Rep. 10, 1–12. doi: 10.1038/s41598-020-62639-y
Ricart A. M., York P. H., Rasheed M. A., Pérez M., Romero J., Bryant C. V., et al. (2015). Variability of sedimentary organic carbon in patchy seagrass landscapes. Mar. pollut. Bull. 100, 476–482. doi: 10.1016/j.marpolbul.2015.09.032
Rickard D., Mussmann M., Steadman J. A. (2017). Sedimentary sulfides. Elements 13, 117–122. doi: 10.2113/gselements.13.2.117
Rozaimi M., Lavery P. S., Serrano O., Kyrwood D. (2016). Long-term carbon storage and its recent loss in an estuarine Posidonia australis meadow (Albany, Western Australia). Estuar. Coast. Shelf Sci. 171, 58–65. doi: 10.1016/j.ecss.2016.01.001
Samper-Villarreal J., Lovelock C. E., Saunders M. I., Roelfsema C., Mumby P. J. (2016). Organic carbon in seagrass sediments is influenced by seagrass canopy complexity, turbidity, wave height, and water depth. Limnol. Oceanogr. 61, 938–952. doi: 10.1002/lno.10262
Schaeffer-Novelli Y., Cintrón-Molero G., Adaime R. R., de Camargo T. M., Cintron-Molero G., de Camargo T. M. (1990). Variability of mangrove ecosystems along the Brazilian coast. Estuaries 13, 204. doi: 10.2307/1351590
Serrano O., Lavery P. S., Duarte C. M., Kendrick G. A., Calafat A., York P. H., et al. (2016). Can mud (silt and clay) concentration be used to predict soil organic carbon content within seagrass ecosystems? Biogeosciences 13, 4915–4926. doi: 10.5194/bg-13-4915-2016
Serrano O., Ricart A. M., Lavery P. S., Mateo M. A., Arias-Ortiz A., Masque P., et al. (2015). Key biogeochemical factors affecting soil carbon storage in Posidonia meadows. Biogeosci. Discuss. 12, 18913–18944. doi: 10.5194/bgd-12-18913-2015
Seybold C. A., Mersie W., Huang J., Mcnamee C. (2002). Soil redox, pH, temperature, and water-table patterns of a freshwater tidal wetland. Wetlands 22, 149–158. doi: 10.1672/0277-5212(2002)022[0149:SRPTAW]2.0.CO;2
Siikamäki J., Sanchirico J. N., Jardine S., McLaughlin D., Morris D. (2013). Blue carbon: coastal ecosystems, their carbon storage, and potential for reducing emissions. Environ. Sci. Policy Sustain. Dev. 55, 14–29. doi: 10.1080/00139157.2013.843981
Silva E. V., Souza M.M. A. (2006). Main forms of use and occupation of the mangroves of the State of Ceará. Cult. Sci. Period. 1, 12–20.
Sun Y., Song Z., Zhang H., Liu P., Hu X. (2020). Seagrass vegetation affect the vertical organization of microbial communities in sediment. Mar. Environ. Res. 162, 105174. doi: 10.1016/j.marenvres.2020.105174
Thangaradjou T., Bala Krishna Prasad M., Subhashini P., Raja S., Dilipan E., Nobi E. P. (2015). Biogeochemical processes in tropical seagrass beds and their role in determining the productivity of the meadows. Geochemistry International 53, 473–486. doi: 10.1134/S0016702915050055
Tessier A., Campbell P. G. C., Bisson M. (1979). . Biogeochemical processes in tropical seagrass beds and their role in determining the productivity of the meadows. Anal. Chem. 51, 844–851. doi: 10.1021/ac50043a017
Toldo E. E. Jr., Dillenburg S. R., Corrêa I. C. S., Almeida L. E. S. B. (2000). Holocene Sedimentation in Lagoa dos Patos Lagoon, Rio Grande do Sul, Brazil. J. Coast. Res. 16, 816–822. doi: 10.2307/4300091
Tripathi R. D., Tripathi P., Dwivedi S., Kumar A., Mishra A., Chauhan P. S., et al. (2014). Roles for root iron plaque in sequestration and uptake of heavy metals and metalloids in aquatic and wetland plants. Metallomics 6, 1789–1800. doi: 10.1039/C4MT00111G
Turschwell M. P., Connolly R. M., Dunic J. C., Sievers M., Buelow C. A., Pearson R. M., et al. (2021). Anthropogenic pressures and life history predict trajectories of seagrass meadow extent at a global scale. Proc. Natl. Acad. Sci. 118, 1–11. doi: 10.1073/pnas.2110802118
Ugarelli K., Laas P., Stingl U. (2019). The microbial communities of leaves and roots associated with turtle grass (Thalassia testudinum) and manatee grass (syringodium filliforme) are distinct from seawater and sediment communities, but are similar between species and sampling sites. Microorganisms 7, 4. doi: 10.3390/microorganisms7010004
Unsworth R. K. F., Nordlund L. M., Cullen-Unsworth L. C. (2019). Seagrass meadows support global fisheries production. Conserv. Lett. 12, e12566. doi: 10.1111/conl.12566
Valentine J. F., Heck K. L. (2021). Herbivory in seagrass meadows: an evolving paradigm. Estuaries Coasts 44, 491–505. doi: 10.1007/s12237-020-00849-3
Valeriano C., de M., Mendes J. C., Tupinambá M., Bongiolo E., Heilbron M., et al. (2016). Cambro-Ordovician post-collisional granites of the Ribeira belt, SE-Brazil: A case of terminal magmatism of a hot orogen. J. South Am. Earth Sci. 68, 269–281. doi: 10.1016/j.jsames.2015.12.014
Vilas Bôas G. S., Sampaio F. J., Pereira A. M. S. (2001). The Barreiras Group in the Northeastern coast of the State of Bahia, Brazil: depositional mechanisms and processes. An. Acad. Bras. Cienc. 73, 417–427. doi: 10.1590/S0001-37652001000300010
Vogel M. A., Mason O. U., Miller T. E. (2021). Composition of seagrass phyllosphere microbial communities suggests rapid environmental regulation of community structure. FEMS Microbiol. Ecol. 97, 1–13. doi: 10.1093/femsec/fiab013
Welsh D. T. (2000). Nitrogen fixation in seagrass meadows: Regulation, plant-bacteria interactions and significance to primary productivity. Ecol. Lett. 3, 58–71. doi: 10.1046/j.1461-0248.2000.00111.x
Williams C. J., Jaffé R., Anderson W. T., Jochem F. J. (2009). Importance of seagrass as a carbon source for heterotrophic bacteria in a subtropical estuary (Florida Bay). Estuar. Coast. Shelf Sci. 85, 507–514. doi: 10.1016/j.ecss.2009.09.019
York P. H., Smith T. M., Coles R. G., McKenna S. A., Connolly R. M., Irving A. D., et al. (2017). Identifying knowledge gaps in seagrass research and management: An Australian perspective. Mar. Environ. Res. 127, 163–172. doi: 10.1016/j.marenvres.2016.06.006
Yu C., Xie S., Song Z., Xia S., Åström M. E. (2021). Biogeochemical cycling of iron (hydr-)oxides and its impact on organic carbon turnover in coastal wetlands: A global synthesis and perspective. Earth Sci. Rev. 218, 103658. doi: 10.1016/j.earscirev.2021.103658
Zhang J., Ling J., Zhou W., Zhang W., Yang F., Wei Z., et al. (2021). Biochar addition altered bacterial community and improved photosynthetic rate of seagrass: A mesocosm study of seagrass Thalassia hemprichii. Front. Microbiol. 12. doi: 10.3389/fmicb.2021.783334
Zhang X., Liu S., Jiang Z., Wu Y., Huang X. (2022). Gradient of microbial communities around seagrass roots was mediated by sediment grain size. Ecosphere 13, 1–15. doi: 10.1002/ecs2.3942
Keywords: coastal wetlands, Ruppia maritima, Halodule wrightii, Fe fractionation, Metabolic pathways
Citation: Nóbrega GN, de Andrade PAM, Queiroz HM, Pereira APdA, Copertino MdS, Gorman D, Zhuang W, Song J, Andreote FD, Otero XL and Ferreira TO (2023) Bridging soil biogeochemistry and microbial communities (archaea and bacteria) in tropical seagrass meadows. Front. Mar. Sci. 10:1190497. doi: 10.3389/fmars.2023.1190497
Received: 21 March 2023; Accepted: 05 September 2023;
Published: 25 September 2023.
Edited by:
Renato S. Carreira, Pontifical Catholic University of Rio de Janeiro, BrazilReviewed by:
Yunchao Wu, Chinese Academy of Sciences (CAS), ChinaClaudia Hamacher, Rio de Janeiro State University, Brazil
Kun-Seop Lee, Pusan National University, Republic of Korea
Copyright © 2023 Nóbrega, de Andrade, Queiroz, Pereira, Copertino, Gorman, Zhuang, Song, Andreote, Otero and Ferreira. This is an open-access article distributed under the terms of the Creative Commons Attribution License (CC BY). The use, distribution or reproduction in other forums is permitted, provided the original author(s) and the copyright owner(s) are credited and that the original publication in this journal is cited, in accordance with accepted academic practice. No use, distribution or reproduction is permitted which does not comply with these terms.
*Correspondence: Tiago Osório Ferreira, toferreira@usp.br