Interactive effects of temperature and nitrogen on the physiology of kelps (Nereocystis luetkeana and Saccharina latissima)
- 1University of Washington, Department of Biology, Seattle, WA, United States
- 2University of Washington, Friday Harbor Laboratories, Friday Harbor, WA, United States
- 3Washington State Department of Natural Resources, Olympia, WA, United States
Kelp forest declines have been linked to warming ocean temperatures worldwide. Ocean warming rarely occurs in isolation, so multiple stressor studies are necessary to understand the physiological responses of kelp to climate change. The canopy-forming bull kelp, Nereocystis luetkeana, is going locally extinct in areas of the Salish Sea that are seasonally warm and nutrient poor, while the understory kelp, Saccharina latissima, persists at those sites. Further, nitrogen availability can alter physiological responses of kelps to temperature stress, including alleviating warming stress. We compared the physiological responses of kelp sporophytes to high temperature stress and nitrogen limitation between two populations of N. luetkeana with different environmental histories (warm and nutrient poor vs. cold and nutrient rich) and between two species, N. luetkeana and S. latissima. Using laboratory mesocosms, we tested the interactive effects of short term (8-9 day) exposure of kelp blades to different temperatures: low (9, 13°C), moderate (15, 16°C), and warm (21°C) at two different nitrogen concentrations: low (1-3 μM) vs. high (>10 μM). We examined a wide array of physiological responses: blade growth, photosynthesis, respiration, photosynthetic yield, nutrient uptake, and tissue C:N. Both kelp species responded negatively to elevated temperatures, but not to low nitrogen levels. Blades of both species showed signs of metabolic stress and reduced growth in the warmest temperature treatment (21°C), at both high and low nitrogen levels, suggesting that N. luetkeana and S. latissima are susceptible to thermal stress over short time periods. Populations of N. luetkeana from warm, nutrient poor and cool, nutrient rich areas were equally susceptible to the effects of ocean warming. Our results suggest that nutrient additions may actually reduce kelp performance at supra-optimal temperatures, and a thorough understanding of kelp responses to coastal temperature and nutrient dynamics is needed to guide conservation and restoration actions.
1 Introduction
The ocean is warming rapidly due to the thermal impacts of greenhouse gases, as it has absorbed more than 90% of the global excess heat generated since 1971 (Zanna et al., 2019). Global mean sea surface temperatures have increased by 0.88°C over the last century, and marine heatwaves are twice as frequent (IPCC, 2022). Some coastal regions are warming more rapidly – for example, the Salish Sea in the Northeast Pacific is projected to warm by 1.5-3°C by 2100 (Riche et al., 2014; Khangaonkar et al., 2019). Temperature exerts a major influence on organismal physiology due to the temperature dependence of biochemical reactions, including metabolic rates (Brown et al., 2004; Dillon et al., 2010). Ectothermic organisms, whose metabolism is controlled by environmental temperatures, are especially susceptible to exceeding their thermal tolerance limits. Marine ectotherms experience temperatures closer to their upper limits than terrestrial ectotherms, leading to greater vulnerability in the face of global climate change (Pinsky et al., 2019).
To persist in the face of climate change, species can acclimate, adapt, or migrate to less stressful conditions. Immobile organisms such as primary producers may be more susceptible to climate change, but they also experience high selection pressure in response to changing environmental conditions (Bennett et al., 2019). Populations can differ in their thermal tolerance limits due to acclimation to local environmental conditions or adaptive genetic differentiation (King et al., 2017). Previous thermal exposure, which tends to differ across populations in heterogeneous environments, can modify physiological responses to warming (Becheler et al., 2022; Gauci et al., 2022). For example, macroalgae sampled across latitudinal gradients that spanned a range of thermal environments displayed variable responses to heat stress (Wernberg et al., 2018; Liesner et al., 2020; Vranken et al., 2021). Knowledge of population-level variability in thermal tolerance is critical to determine how a species will respond to global climate change, as certain populations may go locally extinct.
Kelp, marine macroalgae in the order Laminariales, are foundation species that create underwater forests in temperate and subpolar regions. Kelp forests have been declining rapidly in response to warming ocean temperatures and marine heatwaves (Arafeh-Dalmau et al., 2019; Smale, 2020), with more than one-third of global kelp forests in decline over the past 50 years (Krumhansl et al., 2016). Supra-optimal temperatures are physiologically stressful, leading to higher respiration rates and greater metabolic demand in kelp sporophytes (Gerard, 1997; Staehr and Wernberg, 2009; Biskup et al., 2014; Umanzor et al., 2021). Photosynthesis rates usually decline above optimum temperatures while respiration rates continue to increase, leading to decreased growth rates (Umanzor et al., 2021). These responses to elevated temperatures can lead to decreased size and survival of sporophytes, and thus kelp forest losses. In addition to supra-optimal temperatures, low dissolved inorganic nitrogen concentrations can be stressful for marine primary producers, as they require nitrogen to sustain rapid growth rates (Gerard, 1997; Fernández et al., 2020; Umanzor et al., 2021). Nitrogen-containing chlorophyll pigments decline with nitrogen deficiency, which can reduce photosynthesis and growth rates (Korb and Gerard, 2000; Roleda and Hurd, 2019).
In temperate marine ecosystems, sea surface temperatures and dissolved inorganic nitrogen levels are often negatively correlated due to the effects of upwelling and stratification (Palacios et al., 2013). Upwelling brings cool, nutrient rich water to the surface, while thermal stratification of the water column can lead to nutrient depletion by phytoplankton in the warmer surface waters. In estuarine systems such as the Salish Sea, temperature and nutrients are driven partly by mixing associated with tidal currents, but thermal stratification and nutrient reduction still result from sustained high temperatures (Khangaonkar et al., 2021). As sea surface temperatures continue to rise and stratification increases, surface nitrate concentrations are predicted to decline (IPCC, 2022), thus there is an urgent need to understand the interactive effects of these correlated stressors on kelp physiology.
Prior experimental studies have found positive or neutral effects of nitrogen availability on kelp performance and thermal tolerance. In the macroscopic sporophyte phase, high nitrogen concentrations improved the growth and photosynthetic rates of Macrocystis pyrifera blades at elevated temperatures (Fernández et al., 2020). Gerard (1997) found that the photosynthetic performance of juvenile S. latissima improved at high temperatures only when N reserves were maintained. Survival, photosynthesis, and growth of juvenile sporophytes of Ecklonia cava improved with additional nitrogen across all temperatures (Gao et al., 2016). Photosynthetic responses to simulated marine heatwaves improved with higher nitrate availability, but pigment content decreased at high temperatures despite high nitrate levels (Umanzor et al., 2021). Franco et al. (2018) found that nitrogen had no effect on the survival of Laminaria ochroleuca at elevated temperatures, but additional nitrogen increased growth under optimal temperatures. Studies on the interactive effects of temperature and nitrogen on early life history stages of kelp (zoospores, gametophytes, and microscopic sporophytes) found that elevated temperatures were much more limiting than low nitrogen concentrations (Mabin et al., 2013; Martins et al., 2017; Muth et al., 2019; Weigel et al., 2023). These studies highlight the complexity of kelp responses to interactive stressors, including the potential for nitrogen availability to reduce negative impacts of high temperatures, and the importance of assessing nitrogen and temperature interactions across multiple species and populations.
The annual bull kelp, Nereocystis luetkeana, is one of the largest canopy-forming kelp species in the Northeast Pacific. Bull kelp forests have been declining rapidly in some areas, including warmer, wave-sheltered coastlines in British Columbia (Starko et al., 2019; Starko et al., 2022), warm and nutrient-poor regions of South Puget Sound (Berry et al., 2021), and in northern California following the 2013 marine heatwave and sea urchin population increases (Rogers-Bennett and Catton, 2019). Additionally, a decade-long temperature increase of 3.5°C induced by the thermal outflow from a power plant led to a 97% decline in the abundance of N. luetkeana in California (Schiel et al., 2004). Despite the correlation of bull kelp losses with elevated summer water temperatures and low nutrient concentrations, no studies to date have examined the interactive effects of these two stressors on the physiology of sporophytes of N. luetkeana.
Kelp forests often contain diverse assemblages of understory species that inhabit deeper subtidal environments and make important year-round contributions to kelp forest productivity (Bell and Kroeker, 2022). These forests also provide many important ecosystem services, including habitat for rockfish (Williams et al., 2010). In Washington state (USA), the understory kelp S. latissima has persisted in areas where the N. luetkeana canopy has disappeared (Berry et al., 2021). As an understory kelp, S. latissima greatly differs from N. luetkeana in morphology and habitat, and may compete with N. luetkeana for space and light. Both species grow in the shallow subtidal; S. latissima grows at depths up to 20 m (Lamb and Hanby, 2005), while N. luetkeana can be found deeper (Abbott & Hollenberg, 1976). Buoyant pneumatocysts keep the majority of N. luetkeana blade biomass at the surface, while blades of S. latissima remain prostrate near the benthos. As nitrogen concentrations are generally higher and temperatures are cooler in deeper waters beneath the thermocline due to stratification (Khangaonkar et al., 2019), it is possible that S. latissima has a refuge from the stressful surface conditions that N. luetkeana experiences. However, thermal tolerances and nitrogen requirements often differ among kelp species (Lüning and Freshwater, 1988; Roleda and Hurd, 2019), and it is not known the extent to which the persistence of S. latissima at warm, nutrient poor sites is due to higher thermotolerance or nutrient acquisition abilities or stress avoidance due to its benthic habitat.
We conducted two controlled mesocosm experiments to determine the interactive effects of temperature and nitrogen on the physiology of N. luetkeana and S. latissima sporophytes. We first compared the physiological responses of N. luetkeana from two populations with different environmental histories (warm and nutrient poor vs. cold and nutrient rich) to test the hypothesis that a population with a history of stressful environmental conditions will perform better when challenged with thermal and/or nutrient stress, due to local adaptation or acclimation. We then compared the physiological responses of N. luetkeana and S. latissima collected from the same location to test the hypothesis that the persistence of the understory species is due to superior performance under temperature stress and nitrogen limitation. By measuring diverse response variables including growth, photosynthesis and respiration rates, photosynthetic physiology via pulse amplitude modulated (PAM) fluorometry, blade tissue C:N, and nitrogen (NO3, NH4) uptake rates, we aimed to gain a comprehensive mechanistic understanding of the effects of these stressors on kelp physiology. We hypothesized that photophysiology and growth would be negatively impacted by warmer temperatures, but greater nitrogen availability could mitigate the effects of high temperature stress. Additionally, we expected blade tissue nitrogen to increase with nitrogen availability, but if nutrient uptake rates are temperature-dependent, then blade tissue nitrogen may be affected by temperature as well.
2 Methods
2.1 Kelp blade collection
We conducted two separate experiments to examine the interactive effects of temperature and nutrients on 1) sporophyte blades of Nereocystis luetkeana collected from two populations with different thermal histories (hereafter referred to as “population exp.”), and 2) sporophyte blades of N. luetkeana and Saccharina latissima collected from the same location (“species exp.”). For the population exp., we collected non-reproductive blades of N. luetkeana on July 06, 2022 from two different spatially separated populations: 1) Cherry Point, Strait of Georgia, USA (48.855194, -122.730944), a warm and nutrient poor site, and 2) Turn Rock, San Juan Islands, USA (48.535333, -122.964778), a cool and nutrient rich site (Weigel et al., 2023). We collected blades of N. luetkeana (n = 48 per population from distinct individuals) from the surface by carefully detaching the blade from the pneumatocyst at the narrowest point. Individuals were randomly chosen, and multiple areas within the kelp bed were sampled to achieve a representative sample. For the species exp., we collected non-reproductive blades of N. luetkeana from the surface and whole sporophytes of S. latissima (n = 42 per species) via scuba diving at Deadman Island, San Juan Islands, USA (48.457833, -122.942111) on August 04, 2022. We collected sporophytes of S. latissima (30-40 cm in length) from a depth of 5-9 m (relative to mean lower low water) by carefully detaching the holdfast from the substrate. Individuals were randomly collected as they were encountered during a scuba dive. We also collected extra kelp blade tissue samples from each population and species to compare the C:N content with that of experimental kelp (n = 8 for population exp., n = 7 for species exp.).
We transported kelp immediately back to Friday Harbor Laboratories (FHL) in coolers with seawater and ice packs, and placed samples into flow-through seawater tanks at 11-12°C. On the day of collection, we removed visible epiphytes, cut the blades to a standard length, and tagged each replicate by sewing fishing line with small numbered beads into the thick narrow tissue at the proximal end of the blade (N. luetkeana) or the stipe (S. latissima). We cut the blades of N. luetkeana to a length of 40 cm from the blade base in the population exp., and 30 cm from the blade base in the species exp. to better match the size of S. latissima. In addition, we removed the holdfasts from S. latissima and cut blades to 30 cm so both species had equivalent tissue types and initial wounding levels.
2.2 Experimental design
We manipulated seawater temperatures and nutrient concentrations using 100 L (91 x 34 x 30 cm; L x W x H) recirculating tanks in the FHL Ocean Acidification Environmental Laboratory (O’Donnell et al., 2013; Guenther et al., 2022). Natural seawater was UV-sterilized to deter phytoplankton and diatom growth prior to starting experiments. For both experiments, treatments included 3 static temperatures crossed with 2 nitrogen levels (n = 7-8 blades per treatment). Our biological replicates were individual blades that were mixed between both populations or species within tanks; replication for each treatment and response variable can be found in Table S1. To establish low N levels (<3 μM), kelp blades drew down dissolved nitrogen from starting mean ( ± SD) NO3 levels of 23.40 ± 0.22 μM (population exp.) and 18.14 ± 0.01 μM (species exp.) over 2 days. Temperatures were 13, 16 and 21°C in the population exp. and 9, 15 and 21°C in the species exp. The two lower temperatures span the normal range of cooler areas in the Salish Sea, while temperatures of 15-16°C are often experienced during the summer and fall in warmer areas, and 21°C represents a marine heatwave or extreme event (Weigel et al., 2023). Temperatures in the species exp. were slightly lower to mimic relatively cooler conditions likely experienced by S. latissima in the understory of a stratified water column. We manipulated temperatures in each tank following the methods in O’Donnell et al. (2013) and Guenther et al. (2022) and measured temperature ( ± 0.1°C) with HOBO Pendant Temperature/Light Data Loggers (Onset model #MX2202, Bourne, MA) every 5 min. We slowly changed temperatures (by 2°C per day) over 5 days until final treatment temperatures were reached (Figure S1). LED lights (5000K daylight) provided 100 μmol m−2 s−1 of photosynthetically active radiation to the water surface on a 16:8 light-dark cycle. Additionally, we added two submersible micro-pumps (Aquaneat BP-50, CLL Pet Supplies, Madison, WI) in each tank to move blades around and reduce boundary layers. Finally, we exposed kelp blades to temperature and nutrient treatments (described below) for 8 days (population exp.) and 9 days (species exp.), after which we measured response variables (Figure S1).
After a 2 day period of initial nutrient drawdown, which occurred at the start of the 5-day acclimation period (Figure S1), we manipulated nutrient concentrations in recirculating tanks with daily nutrient additions of sodium nitrate (NaNO3), ammonium sulfate ((NH4)2SO4), and sodium phosphate (NaH2PO4). We calculated targeted nutrient additions based on tank volume, expected starting concentrations of dissolved inorganic nutrients, and established nutrient uptake rates for N. luetkeana (Weigel and Pfister, 2021) and S. latissima (Forbord et al., 2021). We aimed to manipulate nitrogen levels, so we added phosphate in a 16:1 N:P ratio to ensure that phosphorus did not become limiting. For the population exp., we aimed to maintain dissolved nutrient concentrations (μM) of 35 NO3, 2 NH4 and 2.2 PO4 (high N treatment), which are reflective of oceanic seawater entering the Salish Sea (Pfister et al., 2007), vs. 5 NO3, 0.5 NH4 and 0.3 PO4 (low N treatment), which are reflective of nutrient poor conditions in Puget Sound in summer (Berry et al., 2021). Starting seawater N availability (Table S2) was high (23 µmol NO3) for the San Juan Islands (Murray et al., 2015), and nitrogen uptake rates were lower than expected in the population exp., causing a slow increase in N availability over the experiment that led to higher than targeted N levels (Figure S2). Therefore, in the species exp. we targeted lower dissolved nutrient concentrations (μM) of 15 NO3, 2 NH4 and 1.9 PO4 (high N treatment) vs. 1 NO3, 0.1 NH4 and 0.06 PO4 (low N treatment).
To monitor whether nutrients were at targeted levels, we collected seawater samples from each tank 2 hours after daily nutrient addition. Samples were filtered through 0.7 μm glass-fiber filters and frozen until analysis. The University of Washington Marine Chemistry Lab quantified seawater inorganic nutrient concentrations (NO3, NH4, PO4) using standard methods (Intergovernmental Oceanography Commission, 1994).
2.3 Response variables
We blotted and weighed field-collected blades to determine the initial wet biomass, photographed each with a reference scale at 1 mm resolution to determine the initial surface area and length, and measured initial dark adapted photosystem II yield with a PAM fluorometer (described below). We measured growth (wet weight, length, surface area) and PAM response variables after the acclimation period and at the conclusion of the treatment period (Figure S1). We measured length and surface area from photographs with a reference scale at 1 mm resolution by outlining each blade in ImageJ (Schneider et al., 2012). The blade area of S. latissima was underestimated by this method because of the bullate morphology, compared to the smooth blades of N. luetkeana. We calculated change in wet mass (hereafter biomass) as percent change from beginning to end of the treatment period (Figure S1). Finally, we calculated growth of blade length and area as the difference between final and initial measurements and divided by the number of days in treatment. We considered blades to be dead if they were bleached in color and the tissue was too fragmented to be manipulated as a whole blade.
We measured dark adapted yield of photosystem II (Fv/Fm) with a Pocket PEA Chlorophyll Fluorimeter (Hansatech Instruments, Norfolk, England) following 15 min of dark adaptation under leaf clips. We collected measurements 10 cm from the base of the blade at the same time each day. We collected Fv/Fm measurements daily in the population exp., and only on days 1, 6, and 14 in the species exp. As with the growth rates, we calculated percent change in Fv/Fm from the beginning to the end of the treatment period (Figure S1).
After 6 to 8 days of exposure to experimental treatments (Figure S1), we quantified mass-specific metabolic rates (net photosynthesis and dark respiration) from changes in dissolved oxygen (DO) over time. We measured DO (in mg/L) optically with a FireSting-GO2 pocket oxygen meter equipped with a robust oxygen probe and a temperature sensor (Pyroscience, Aachen, Germany). We incubated individual blades in 1.6 L clear Nalgene bottles filled with seawater from the corresponding tank and maintained treatment temperatures by submerging the bottles. Bottles were sealed water-tight with an 8 mm rubber stopper to allow for quick access with minimal disturbance during DO measurements. A large (1,000 GPH) aquarium pump shook the bottles around in the tanks to ensure mixing.
We measured dark respiration from 75 min incubations under dark tarps with lights off, then photosynthesis from 45 min incubations in 100 μmol m-2s-1 of light. Prior to the measurements for respiration, we acclimated blades to dark conditions for 15 mins to allow oxygen to equilibrate in the bottles. During each run, we had a seawater control to account for potential plankton metabolism. To accommodate all replicate blades in the experiment, we split measurements into multiple runs over 2 days (Figure S1). We measured the biomass of the blades immediately after metabolic rates, and converted to dry mass based on the relationship between wet mass and dry mass from blades sampled at the end of the experiment. We calculated photosynthesis and respiration rates (mg O2 g-1 hr-1) as:
Where ΔO2is the change in DO in mg L-1 between the start and end of the incubation, C is the seawater correction factor due to metabolism by plankton, t is the incubation time in hours, V is the volume of the bottle in liters, and m is the dry mass of the blade in grams.
On the final day of each experiment (Figure S1), we quantified kelp blade nutrient uptake rates on a random subset of blades (n = 4 per treatment). Each kelp blade was placed into a sealed 1.6 L clear Nalgene bottle filled with seawater from the corresponding tank and maintained at the treatment temperature. Therefore, low N treatments had lower nutrient availability at the start of the nutrient uptake assay. We collected initial seawater nutrient samples from the well-mixed tank prior to filling bottles and final seawater nutrient samples from each individual chamber after an incubation period of 100 mins. We included seawater control bottles to account for possible nutrient uptake by plankton in the seawater. We filtered seawater samples through 0.7 μm glass-fiber filters and froze the samples until analysis of NO3, NH4, and PO4 concentrations. We calculated nutrient uptake rates (μmol g-1hr-1) as:
Where ΔNis the change in NO3 or NH4 concentration (μmol L-1) between the start and end of the incubation, C is the seawater correction factor due to uptake by microalgae, V is the volume of the chamber in liters, m is the dry mass of the blades in grams, and t is incubation period in hours.
At the end of each experiment, we dried kelp blade tissues to constant mass in a drying oven. We pulverized dried kelp blade tissues (field-collected and experimental) with a Retsch Mixer Mill 400 tissue grinder. The Washington State University Stable Isotope Core Laboratory analyzed these samples for percent carbon and nitrogen on an elemental analyzer.
2.4 Data analyses
We used R version 4.1.2 for all data analyses and visualizations (R Core Team, 2021). Using the “car” package in R, we ran multi-factor ANOVAs with temperature, nutrients, and population (population exp.) or species (species exp.), and their interactions, as fixed effects (Fox and Weisberg, 2019). We ran separate models on each response variable for each experiment. We used Type III Sum of Squares ANOVAs to account for our unbalanced design (in the species exp., as some kelp died at the highest temperature, Table S1) as well as interactions among factors. Following significant ANOVA outcomes, we performed Tukey post hoc tests with the package “emmeans” (Lenth et al., 2023).
3 Results
3.1 Site differences and efficacy of treatments
On the day of the kelp collections, seawater dissolved inorganic nutrient levels were high at Turn Rock (NO3: 23.4, NH4: 0.2, PO4: 1.8 μM) and low at Cherry Point (NO3: 0.8, NH4: 0.4, PO4: 0.8 μM; Table S2). From July 07 to Sept. 27, 2022, Cherry Point experienced elevated water temperatures (mean ± SD: 13.3 ± 1.8°C, min: 10.3°C, max: 20.5°C), while Turn Rock (mean ± SD: 11.3 ± 0.8°C, min: 10.0°C, max: 15.2°C) remained cool (Figure S3; Weigel et al., 2023). Deadman Island temperatures are generally cool, and likely similar to Turn Rock due to their close proximity (8 km). Nutrient levels at Deadman Island were high on the day of collection (NO3: 19.1, NH4:0.7, PO4: 1.7 μM; Table S2).
Temperature treatments in the population and species experiments were stable and within 1°C of the target. In the population exp., temperatures as mean ± SD and ranges as (min, max) were 12.7 ± 0.2°C (12.1, 13.4), 16.2 ± 0.1°C (16.0, 16.9), and 20.6 ± 0.1°C (20.2, 20.8) during the full treatment period (Figure S1A). In the species exp., temperatures were 9.0 ± 0.3°C (8.3, 9.2), 14.6 ± 0.1°C (14.4, 15.4), and 20.7 ± 0.2°C (20.1, 21.6) during the full treatment period (Figure S1B). Meanwhile, nutrient treatments were within targeted range for the species exp. but were higher than expected in the population exp. Following nutrient draw down, mean NO3 levels in the low N treatments remained around 1.0 μM (species exp.) and below 3 μM (population exp.; Table S2; Figure S2). In the population exp., NO3 levels in the high N treatments increased gradually over time from 25 up to 100 μM, with a mean of ~80 μM (Table S2; Figure S2). We reduced daily nutrient additions for the species exp., so mean NO3 levels in the high N treatments were between 10 and 20 μM (Table S2; Figure S2). Despite the difference in NO3 concentrations in the high N treatments between the two experiments, there were clear differences between low and high N treatments in each experiment (Figure S2).
3.2 Survival and condition
In both experiments, we observed mortality or visual tissue damage and decay only at the highest temperature of 21°C (Figure S4). In the population exp., survival was 100% across all treatments, but signs of damage to kelp blades were visible in both high and low nutrient treatments at 21°C. In the species exp., survival was 100% across nutrient treatments in the 9 and 15°C treatments, but not in the 21°C treatments. At the conclusion of the species exp., the 21°C high N treatment had high mortality (N. luetkeana: 71%, S. latissima: 57%) while the 21°C low N treatment had low mortality (N. luetkeana: 0%, S. latissima: 14%). Mortality prevented measurement of some response variables at the highest temperature.
3.3 Growth rates
In both experiments, blade growth rates responded to temperature and nutrient treatments, and were also influenced by source population or species (Table 1). All aspects of blade growth, whether measured as change in biomass, length, or blade area, were positive at the two cooler temperatures (population exp: 13 and 16°C, species exp: 9 and 15°C) in each experiment (Figures 1, 2) and significantly lower at 21°C (P< 0.05). At 21°C, change in biomass was negligible in the population exp. and negative in the species exp., indicating a net loss of tissue (Figure 1). Further, for all metrics of growth, there were no differences between growth rates at the cool and moderate temperatures (P > 0.05), indicating that kelp grew equally well at 13 vs. 16°C and at 9°C vs. 15°C (Figures 1, 2). The only exception was that in the species exp., N. luetkeana displayed a 0.5 cm/day higher elongation rate at 15°C compared to 9°C (P< 0.001, Figure 2B). At these cooler temperatures, blades in both experiments grew ~15-30% in overall biomass (Figure 1), 1.0-1.5 cm in length per day, and 5-10 cm2 in area per day (Figure 2), demonstrating that laboratory conditions were suitable to both species.
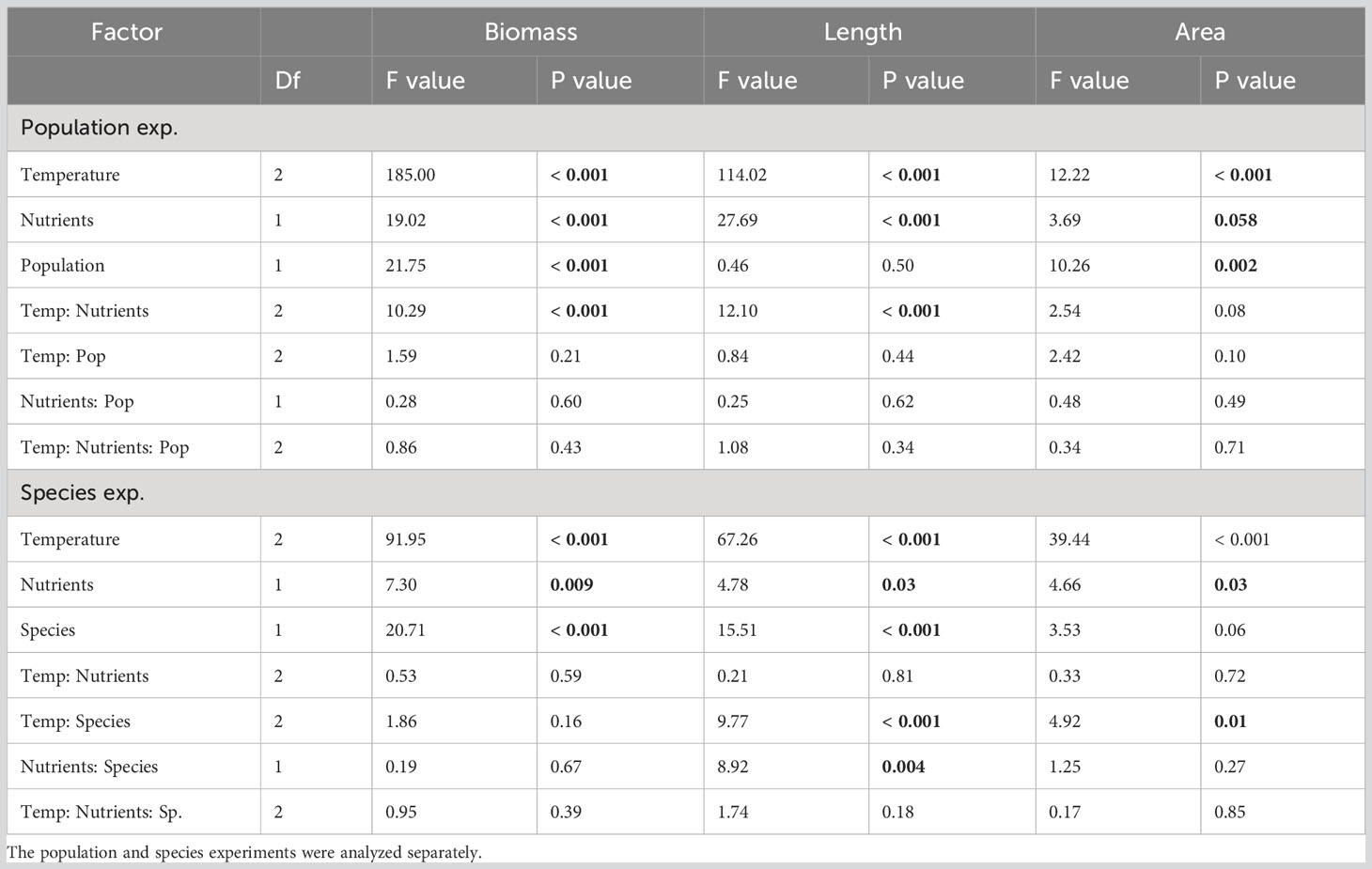
Table 1 Summary of two-factor ANOVAs of the fixed and interactive effects of temperature, nutrients and population or species on three kelp growth metrics: biomass as wet weight (percent change), change in length per day, and change in area per day.
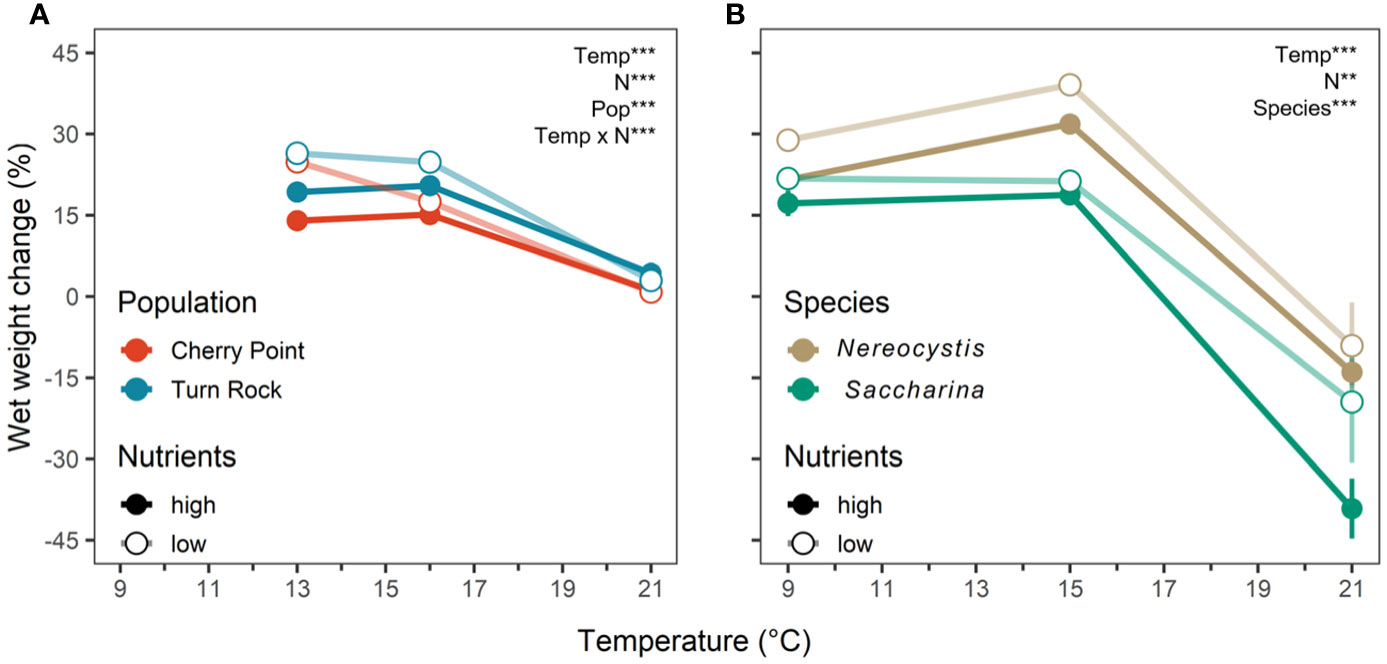
Figure 1 Change in wet weight (%, mean ± SE) during the experimental treatment period across temperature and nutrient levels as either high N (closed circle) or low N (open circle). (A) (left) is the Nereocystis population exp. from Cherry Point (red) and Turn Rock (blue). (B) (right) is the species exp. with Nereocystis (brown) and Saccharina (green) from Deadman Island. Significant effects are indicated by text with asterisks, see Table 1 for details.
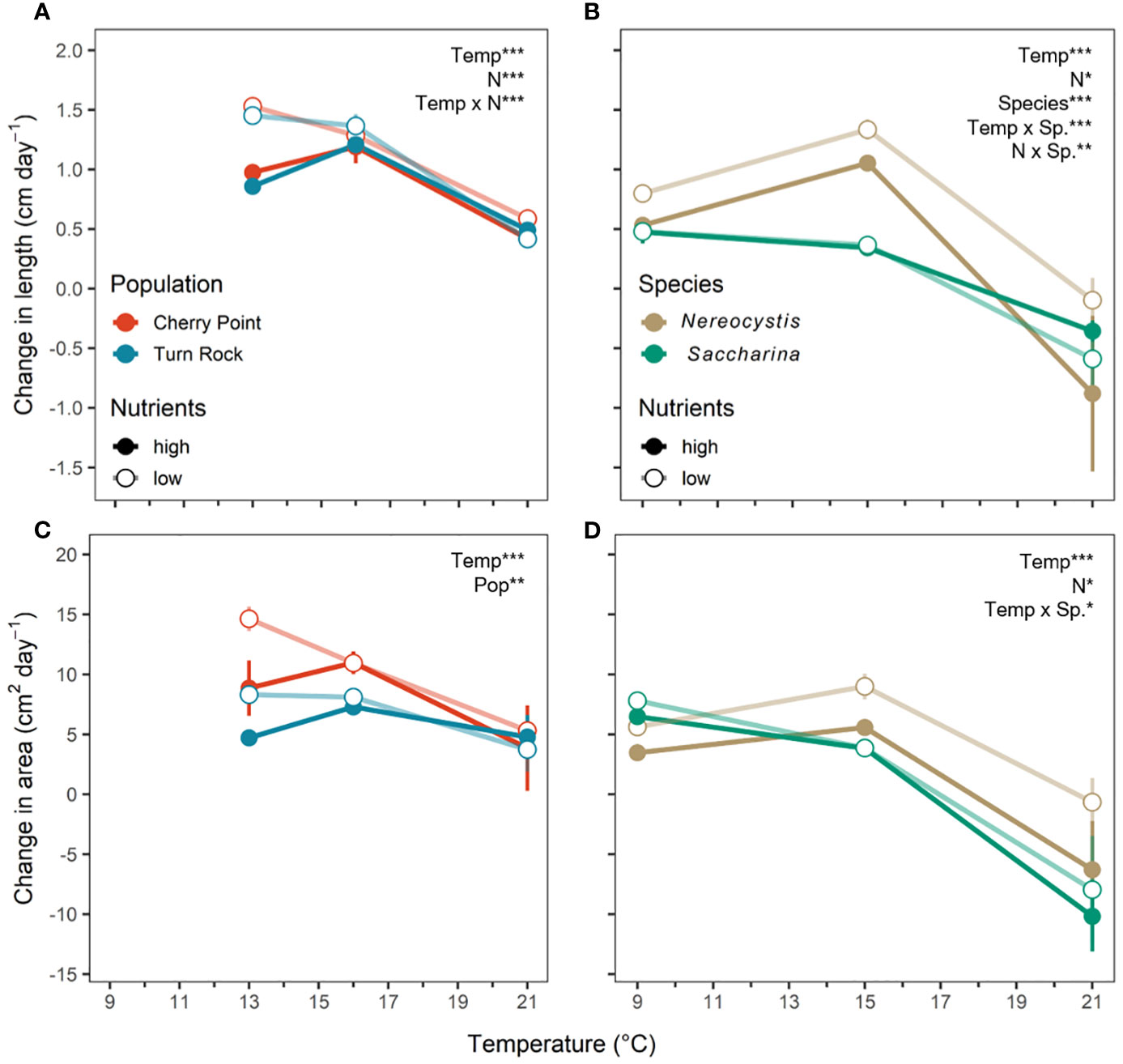
Figure 2 Change in (A, B) blade length in cm/day and (C, D) area in cm2/day as mean ± SE per day during the experimental treatment period across temperature and nutrient levels as either high N (closed circle) or low N (open circle). (A, C) (left) are the population exp. with Nereocystis from Cherry Point (red) and Turn Rock (blue). (B, D) (right) are the species exp. with Nereocystis (brown) and Saccharina (green) from Deadman Island. Significant effects are indicated by text with asterisks, see Table 1 for details.
Kelp blade growth metrics responded to nitrogen manipulations in both experiments (Table 1). In the population exp., change in biomass and length responded significantly to the interaction between temperature and nitrogen (P< 0.001, Table 1). Change in biomass in the low N relative to the high N treatment was 11% higher at 13°C, and 2% higher at 16°C (Figure 1A). Additionally, kelp from the low N treatment elongated by 0.5 cm more per day at 13°C (P > 0.05, Figure 2A). In the species exp., there were no interactions between temperature and nitrogen, but there were significant effects of nitrogen on all growth metrics (P< 0.05, Table 1); kelp from the low N treatments displayed higher growth rates compared to kelp from high N treatments. On average, N. luetkeana blades in low N treatments grew 7% more in biomass, 0.4 cm/day in length, and 4 cm2/day in area compared to blades in high N treatments. Likewise, S. latissima grew 9% more in biomass, 0.1 cm/day in length, and 1.2 cm2/day in area in low N compared to high N treatments (Figures 1B, 2B, D). However, change in biomass was low to negative at 21°C in both experiments at both high and low nitrogen levels, with an average loss of 9% biomass at 21°C (Figure 1).
Finally, there were significant effects of source population and species on kelp growth metrics (Table 1). In the population exp., blades of N. luetkeana from Turn Rock grew significantly more in biomass compared to those from Cherry Point by 4% (Figure 1A), while N. luetkeana from Cherry Point grew significantly more in total blade area than those from Turn Rock by 3 cm2 per day, averaged across treatments (Figure 2C), yet the two populations had equivalent changes in length (Figure 2A). In the species exp., N. luetkeana increased in biomass by 13% more than S. latissima across all temperatures (Figure 1B), while N. luetkeana elongated more only at 15°C (Figure 2B) and N. luetkeana grew more in blade area compared to S. latissima at 15°C and 21°C (Figure 2D).
3.4 Photosynthesis and respiration rates
In both experiments, net photosynthesis responded to temperature and nutrients, as well as their interaction (Table 2). Net photosynthesis rates increased with temperature in the population exp., but were highest at the moderate temperature in the species exp. (Figures 3A, B); however, these temperature effects interacted with nutrients. In the population exp., net photosynthesis increased significantly at each temperature under low N conditions, with the greatest net photosynthesis at 21°C (P< 0.05, Figure 3A). At high N, net photosynthesis was 0.7 mg O2 g-1 hr-1 lower at the coldest temperature (P< 0.0001, Figure 3A), but did not differ between 15 and 21°C (P = 0.4, Figure 3A). Additionally, net photosynthesis was 0.1 mg O2 g-1 hr-1 higher at low N than high N at 13 and 21°C than at 16°C (P< 0.05, Figure 3A), but nutrient treatments did not differ at 16°C (P > 0.05, Figure 3A). In the species exp., net photosynthesis did not differ with temperature in the low N treatments (P > 0.05), but was 0.9 mg O2 g-1 hr-1 higher at 15°C compared to 9 and 21°C in the high N treatments (P< 0.05, Figure 3B). Finally, net photosynthesis of both species only responded to nutrients at 21°C, where low N kelp had 0.8 mg O2 g-1 hr-1 greater net photosynthesis compared to high N kelp (P< 0.001, Figure 3B).
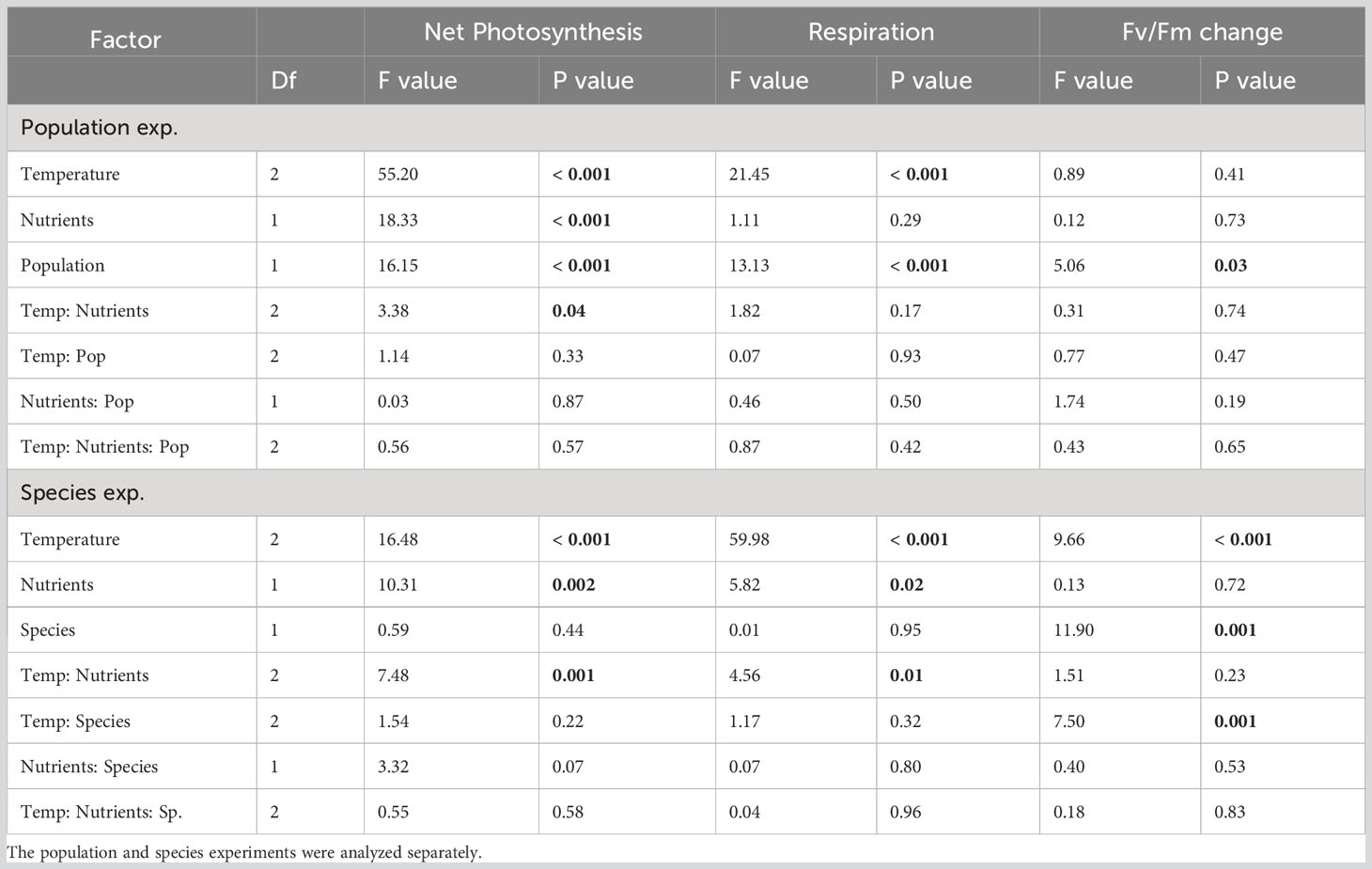
Table 2 Summary of two-factor ANOVAs of the fixed and interactive effects of temperature, nutrients and population or species on kelp photophysiology (net photosynthesis, dark respiration, and change in Fv/Fm).
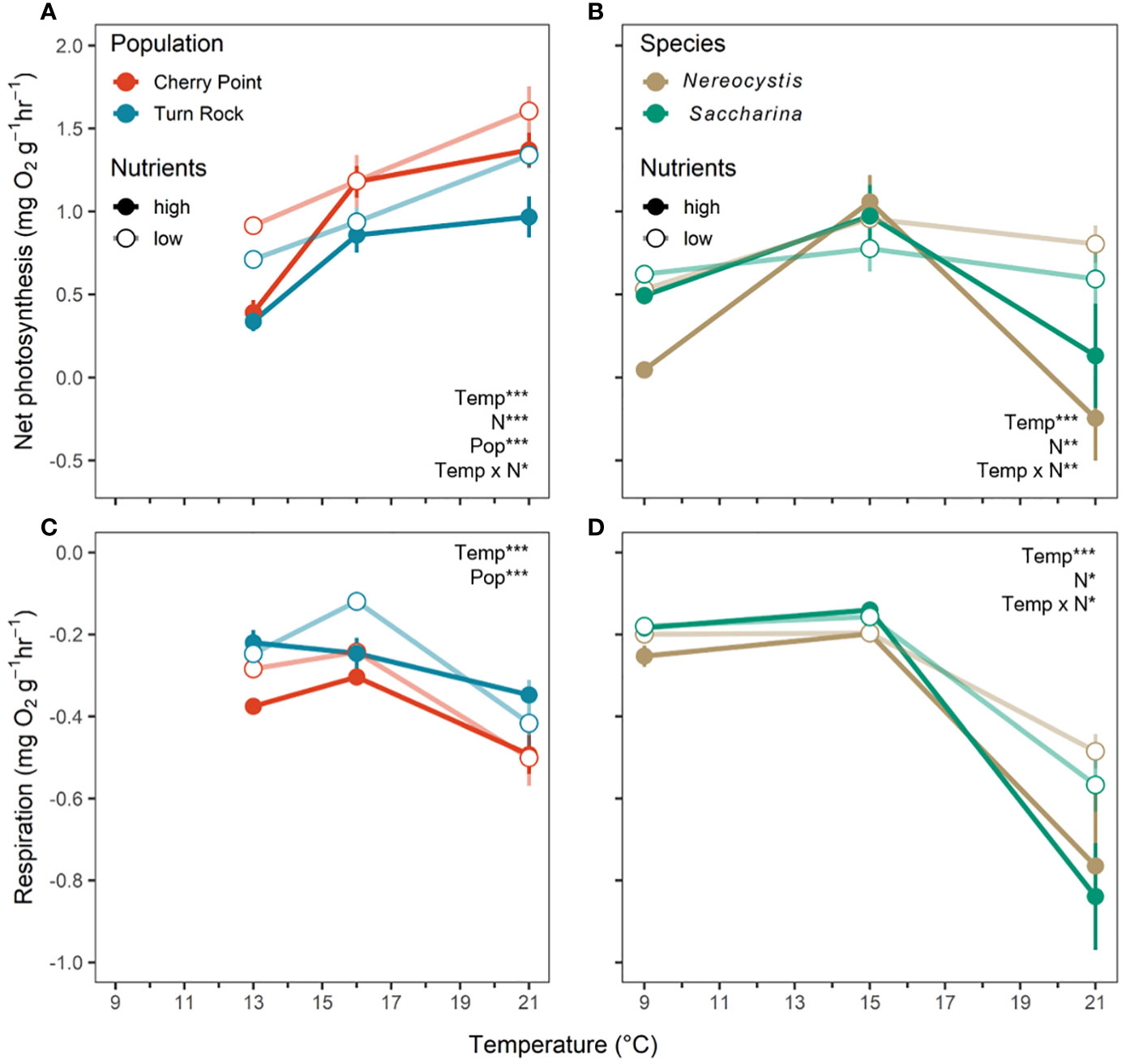
Figure 3 Metabolism measured as change in dissolved oxygen (mean ± SE) for (A, B) net photosynthesis and (C, D) dark respiration at the end of the experiments across temperature and nutrient levels as either high N (closed circle) or low N (open circle). Note that the respiration y-axis is negative, indicating oxygen consumption, with higher respiration rates at more negative values. (A, C) (left) are the population exp. with Nereocystis from Cherry Point (red) and Turn Rock (blue). (B, D) (right) are the species exp. with Nereocystis (brown) and Saccharina (green) from Deadman Island. Significant effects are indicated by text with asterisks, see Table 2 for details.
In both experiments, respiration increased with temperature and was highest at 21°C (Table 2; Figures 3C, D, P< 0.05). Respiration was not affected by nutrients in the population exp, but responded significantly to the interaction between temperature and nutrients in the species exp. (Table 2). In the species exp., high N kelp had 0.3 mg O2 g-1 hr-1 greater respiration rates at 21°C than low N kelp (P< 0.001, Figure 3D). Finally, we found a significant effect of population but not species on net photosynthesis and respiration rates (Table 2). Kelp from Cherry Point had higher net photosynthesis and respiration rates compared to Turn Rock by 0.25 and 0.1 mg O2 g-1 hr-1, respectively (Table 2; Figures 3A, C).
3.5 Dark-adapted Yield of PSII (Fv/Fm)
Change in Fv/Fm responded to temperature in the species exp. (Figure 4B), but not in the population exp. (Table 2), where change in Fv/Fm during the treatment period was close to 0% (Figure 4A; S4). Nutrients did not affect photosynthetic yield in either experiment (Table 2). In the population exp., photosynthetic yield at high temperatures was 3.1% lower in kelp from Turn Rock than Cherry Point (Table 2; Figures 4A, S5). In the species exp., Fv/Fm responded significantly to the interaction between temperature and species, with 14.5% greater declines in Fv/Fm for N. luetkeana than S. latissima at 21°C (P< 0.0001, Figure 4B).
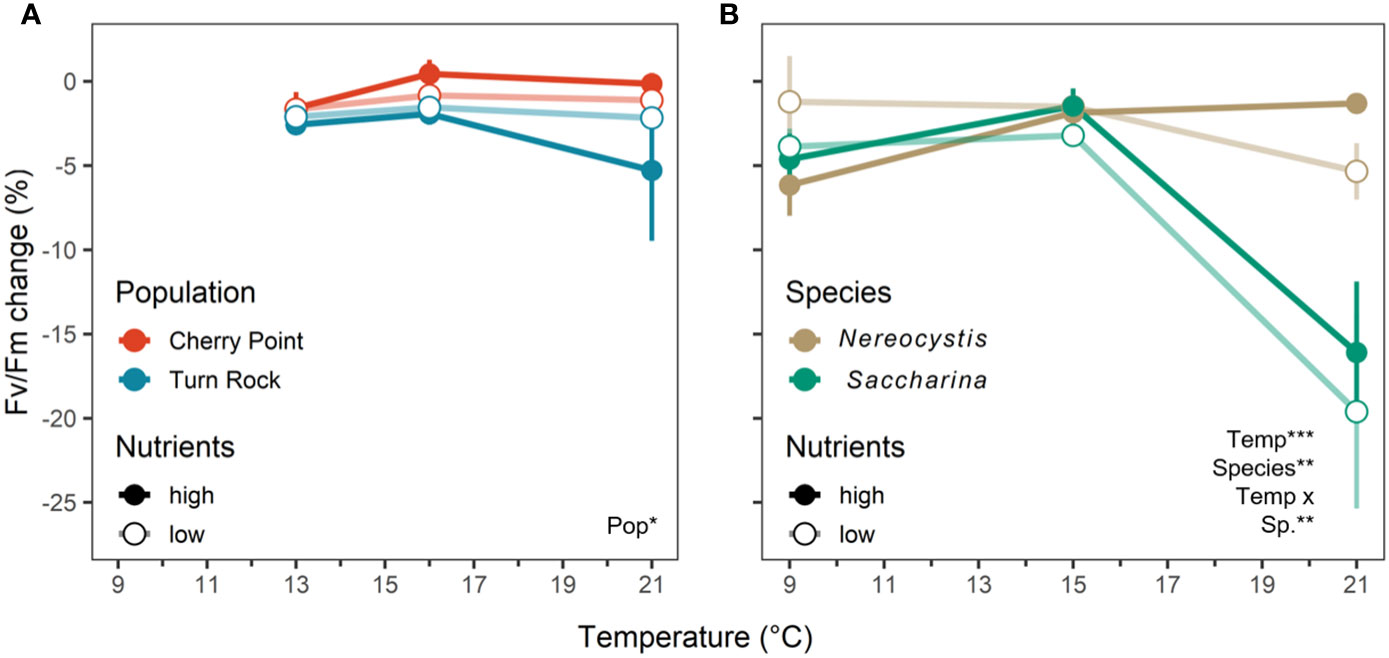
Figure 4 Change of dark-adapted yield of photosystem II (Fv/Fm) (%, mean ± SE) during the experimental treatment period across temperature and nutrient levels as either high N (closed circle) or low N (open circle). (A) (left) is the population exp. with Nereocystis from Cherry Point (red) and Turn Rock (blue). (B) (right) is the species exp. with Nereocystis (brown) and Saccharina (green) from Deadman Island. In the species exp., replication for the 21°C high N treatment was low (n = 2) due to mortality (Table S1). Significant effects are indicated by text with asterisks, see Table 2 for details.
3.6 Nitrate and ammonium uptake rates
As expected, uptake rates of nitrate (NO3) and ammonium (NH4) were concentration dependent. NO3 and NH4 uptake rates were significantly higher in the high N treatments compared to the low N treatments across both experiments, reflecting the difference in nitrogen availability (Table 3; Figures 5A–D). However, in the population exp., NO3 and NH4 uptake rates were higher in the high N treatment only at 16 and 21°C by 1.6 µmol NO3 g-1hr-1 and 0.1 µmol NH4 g-1hr-1 (P< 0.05, Figures 5A, C). In the species exp., NO3 and NH4 uptake rates increased significantly at high N by 0.3 – 1.4 µmol NO3 g-1hr-1 and 0.4 – 0.6 µmol NH4 g-1hr-1 (Table 3; Figures 5B, D). However, we note that nutrient uptake rates were not measured for the warmest high N treatment (21°C high N) in the species exp. due to blade mortality in that treatment, making it difficult to evaluate interactive temperature effects.
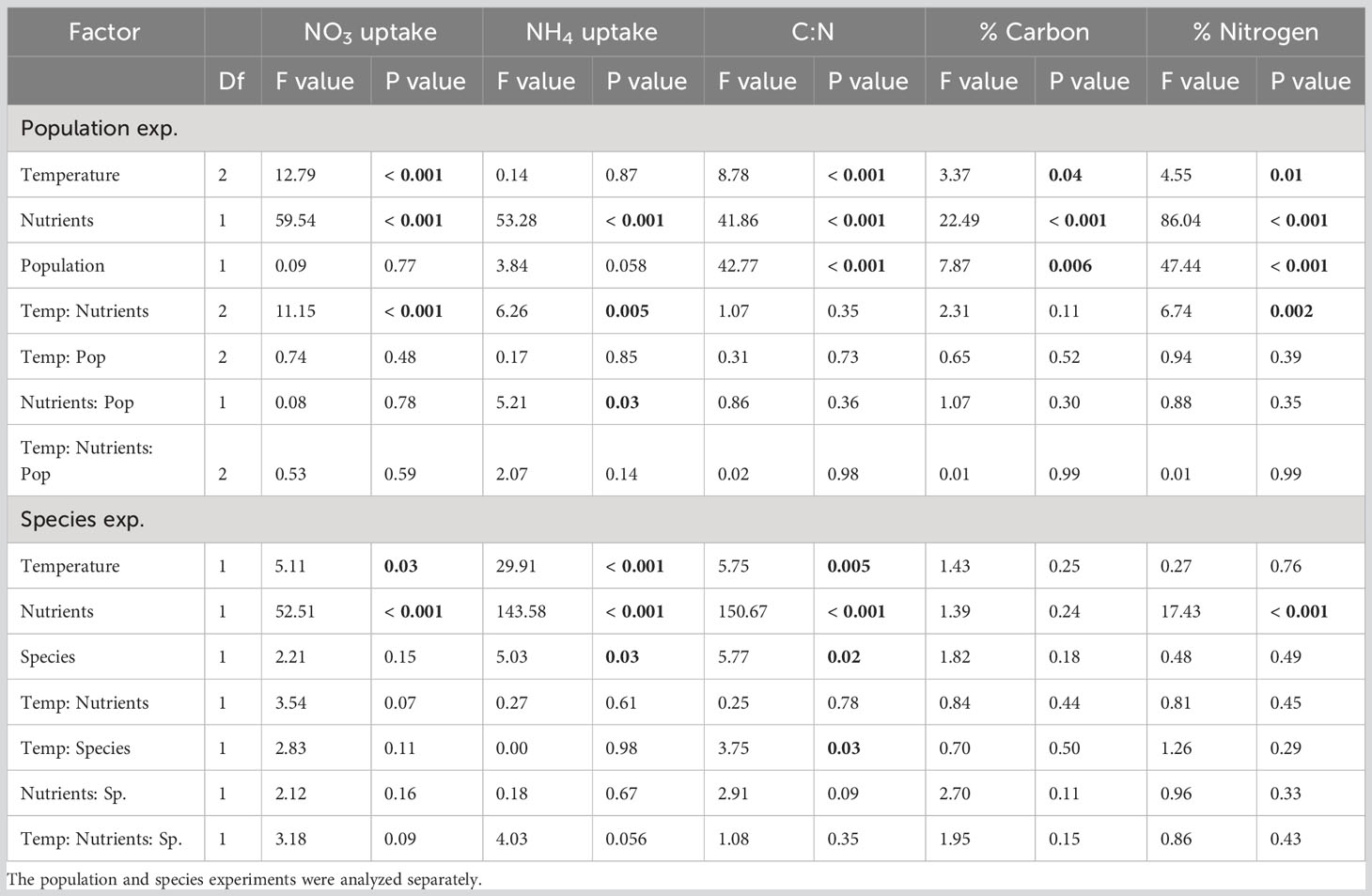
Table 3 Summary of two-factor ANOVAs of the fixed and interactive effects of temperature, nutrients and population or species on kelp for five nutrient assays: nitrate uptake rates, ammonium uptake rates, blade C:N, blade percent carbon, and blade percent nitrogen.
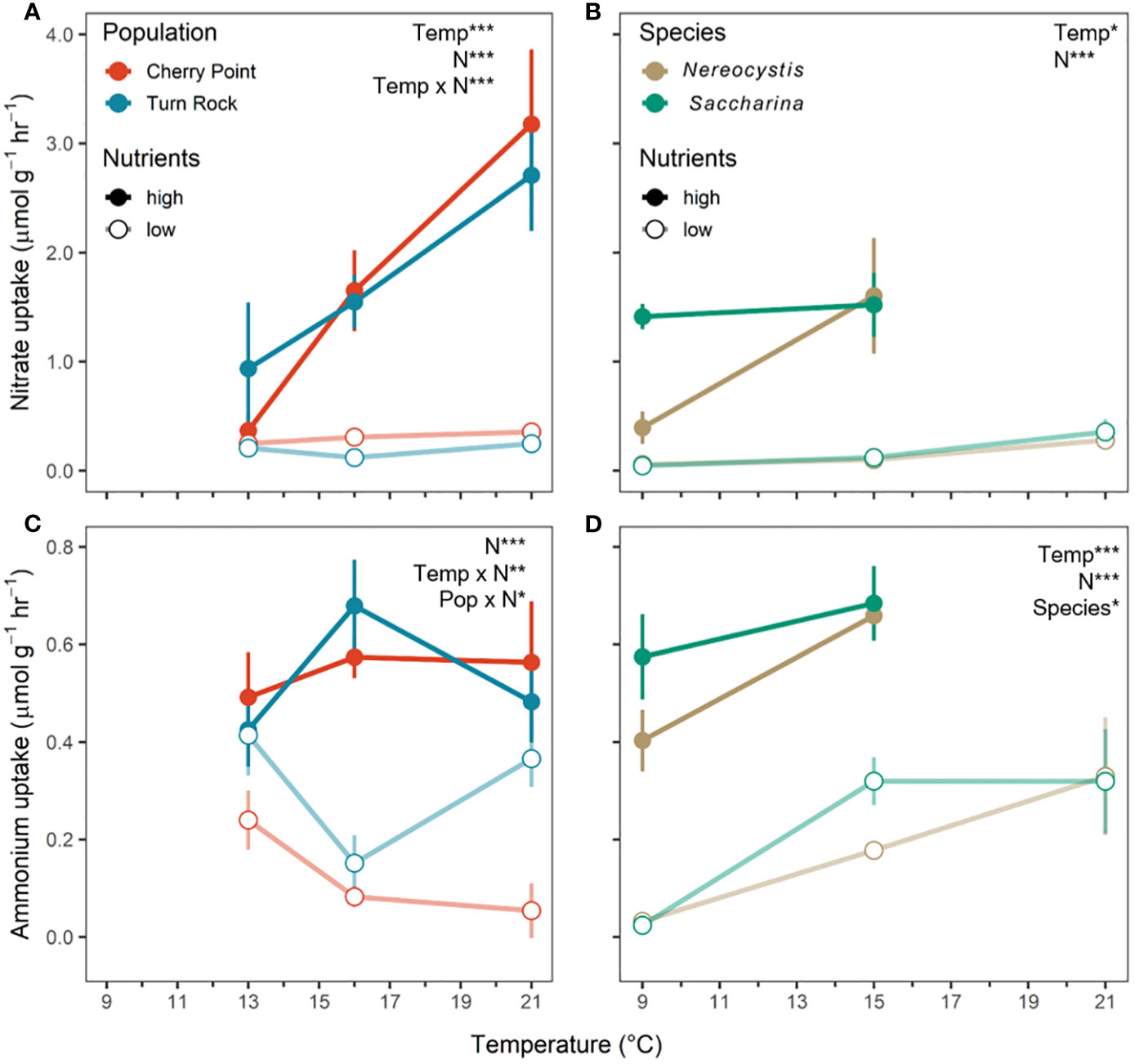
Figure 5 Nutrient uptake rates of (A, B) nitrate (NO3) and (C, D) ammonium (NH4) as mean ± SE across temperature and nutrient levels as either high N (closed circle) or low N (open circle). (A, C) (left) are the population exp. with Nereocystis from Cherry Point (red) and Turn Rock (blue). (B, D) (right) are the species exp. with Nereocystis (brown) and Saccharina (green) from Deadman Island. In the species exp., nutrient uptake was not measured for the 21°C high N treatment due to low replication associated with kelp mortality. Significant effects are indicated by text with asterisks, see Table 3 for details.
Nitrate uptake rates generally increased with temperature across experiments (Figures 5A, B), but there were significant interactive effects of temperature and nitrogen in the population exp. (Table 3). In the species exp., both NO3 and NH4 uptake rates were greater at higher temperatures and nutrient levels, and there were no significant interactions (Table 3; Figures 5B, D). In the population exp., there was an interactive effect of temperature and nutrients (Table 3); NO3 uptake rates increased with temperature only in the high N treatment (P< 0.001), and did not differ across temperatures at low N (P > 0.05). In contrast, NH4 uptake rates in the population exp. only differed across temperatures at low N, where NH4 uptake rates were greater at 13°C compared to 16°C by 0.2 µmol NH4 g-1hr-1 (P< 0.05, Figure 5C). Finally, NH4 uptake rates responded significantly to the interaction between nutrients and population (Table 3); in the low N treatment, kelp from Turn Rock had higher NH4 uptake rates (by 0.2 µmol NH4 g-1hr-1) than those from Cherry Point (P< 0.01, Figure 5C). In the species exp., S. latissima had higher NH4 uptake rates than N. luetkeana at 9 and 15°C by 0.1 µmol NH4 g-1hr-1 (Table 3; Figure 5D).
3.7 Blade tissue percent carbon and nitrogen
At the end of each experiment, blade tissue % nitrogen was significantly higher in the high N treatment compared to the low N treatment (Table 3; Figures 6E, F), demonstrating that kelp blade nitrogen content was successfully manipulated in both experiments. Compared to field-collected blade tissue samples, the nitrogen content of the high N kelp in the population exp. was 0.7-1.0% higher (P< 0.05), while the nitrogen content of the low N kelp did not differ from field-collected samples (P > 0.05, Figure 6E). In the species exp., neither treatment differed significantly from the nitrogen content of field-collected samples (Figure 6F).
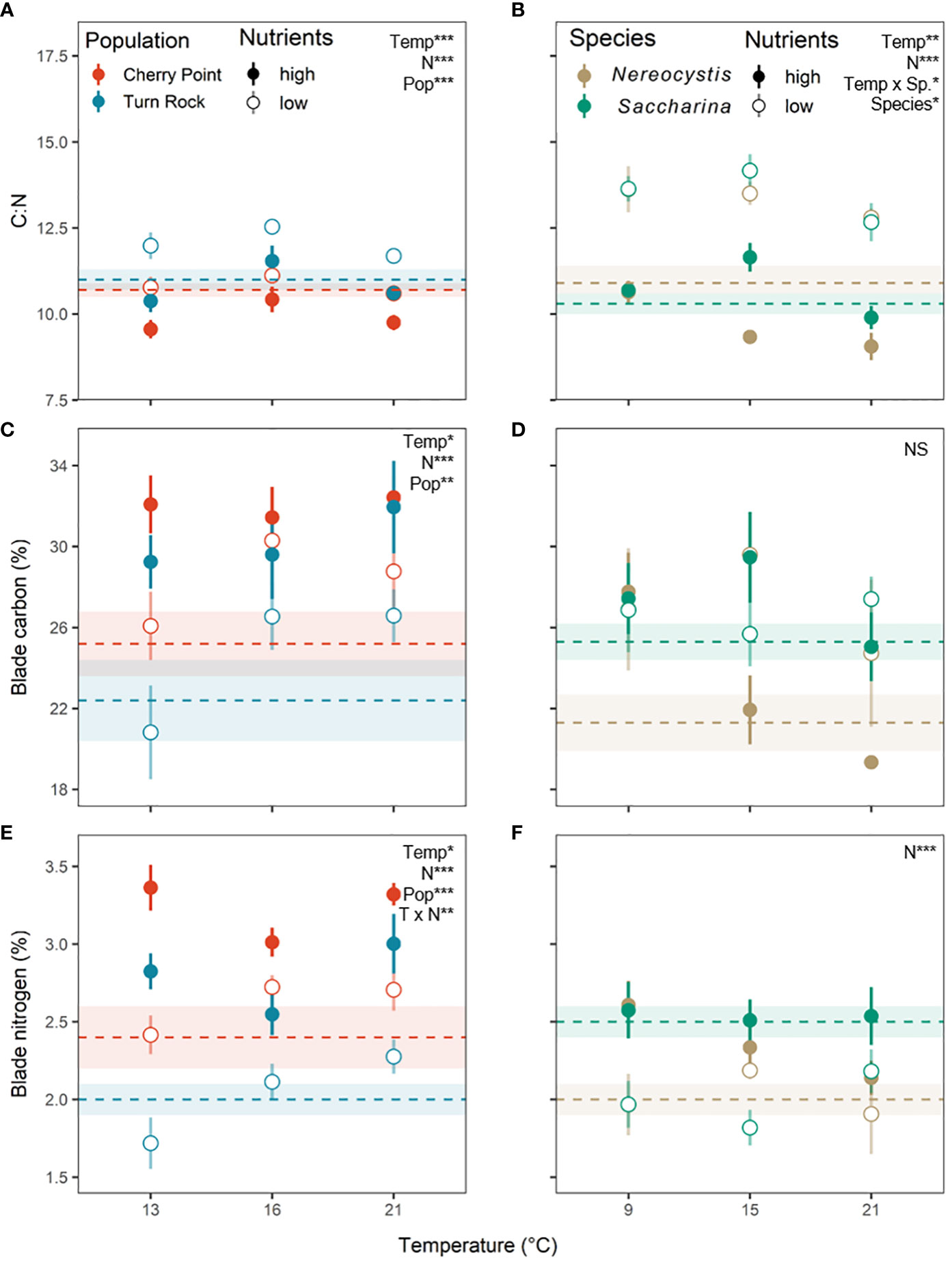
Figure 6 Kelp blade tissue (A, B) carbon to nitrogen ratios, (C, D) percent carbon, and (E, F) percent nitrogen at the conclusion of the experiments across temperature and nutrient levels as either high N (closed circle) or low N (open circle). Circles are mean ± SE of experimental blades. Horizontal dashed lines with shading are mean ± SE of field collected blades representing natural conditions. (A, C, E) (left) are the population exp. with Nereocystis from Cherry Point (red) and Turn Rock (blue). (B, D, F) (right) are the species exp. with Nereocystis (brown) and Saccharina (green) from Deadman Island. Significant effects are indicated by text with asterisks for both high and low nutrient treatments, see Table 3 for details.
Kelp blade nitrogen content also changed significantly within the experimental treatments. In the species exp., nutrient treatment was the only factor that affected kelp nitrogen content (Table 3), while in the population exp., blade tissue % nitrogen also responded to temperature, population, and the interaction between temperature and nitrogen (Table 3). Surprisingly, despite lower seawater NO3 levels at Cherry Point (Table S2), the blade tissue nitrogen content of N. luetkeana from Cherry Point was significantly higher than the nitrogen content of blades from Turn Rock at the time of collection (Table 3; Figure 6E). At high nutrient levels in the population exp., percent nitrogen at 16°C was lower by 0.3% compared to 13 and 21°C (P< 0.05, Figure 6E), while at low nutrient levels, % nitrogen was 0.4% lower at 13°C (P< 0.05, Figure 6E).
In the population exp., the carbon content of kelp blades differed significantly with temperature, nitrogen, and by population (Table 3; Figure 6C). The carbon content of kelp in the species exp. did not differ with any experimental factor (Table 3; Figure 6D). In the population exp., N. luetkeana from Cherry Point had 2.7% higher carbon content than those from Turn Rock (Table 3; Figure 6E). In addition, kelp from the high N treatment had 4.6% greater percent carbon compared to kelp from the low N treatment (Table 3; Figure 6E). Finally, kelp blade carbon content responded significantly to temperature; percent carbon was 2.9% higher at 21°C compared to 13°C (P=0.046), but neither treatment differed from 16°C (Figure 6E).
Correspondingly, there were also differences in the C:N ratio of kelp blade tissues in both experiments with temperature, nitrogen, and species or population (Table 3; Figures 6A, B). In both experiments, kelp from the low N treatment had a higher C:N compared to kelp from the high N treatment, by 1.1 for the population exp. and 3.1 for the species exp. (Figures 6A, B), reflecting the different nitrogen treatments. In the population exp., N. luetkeana from Turn Rock had higher blade tissue C:N by 1.1 compared to those from Cherry Point (Table 3; Figure 6A). There also was a significant effect of temperature on blade tissue C:N in the population exp. (Table 3), where kelp from 16°C had a 0.7 higher C:N compared to kelp from 13 and 21°C (P< 0.05, Figure 6A). In the species exp., blade tissue C:N responded significantly to the interaction between temperature and species (Table 3), where the C:N of N. luetkeana was 1.2 higher at 9 than 21°C (P = 0.046), while the C:N of S. latissima was 1.6 higher at 15 than 21°C (P = 0.0024, Figure 6B). Finally, the two species only differed in blade tissue C:N at 15°C (P = 0.0003), where S. latissima had a 1.5 higher C:N than N. luetkeana (Figure 6B).
4 Discussion
Despite their different morphologies and habitats, the canopy-forming kelp Nereocystis luetkeana and the understory kelp Saccharina latissima responded strongly and similarly to elevated temperatures, showing signs of metabolic stress and low to negative growth rates at 21°C. Surprisingly, we did not find any negative impacts of low NO3 concentrations (1-3 μM) on the growth, metabolism, or photophysiology of either species. Our findings suggest that physiological damage from exposure to supra-optimal temperatures (21°C) can be greater than stress from short-term nutrient limitation. Additionally, we found few differences in the physiological responses of N. luetkeana from two populations with different environmental histories, suggesting that kelp from warm and cool areas are equally susceptible to the effects of ocean warming. Finally, we did not find evidence that S. latissima could outperform N. luetkeana under temperature stress and nitrogen limitation.
Kelps require nitrogen to sustain rapid growth rates, as both amino acids (the building blocks of proteins) and chlorophyll pigments are nitrogen rich (Korb and Gerard, 2000). In some cases, nitrogen-replete conditions can alleviate the effects of high temperature stress (Fernández et al., 2020). Most studies report higher physiological stress under warm temperatures combined with low nitrogen levels (Gerard, 1997; Gao et al., 2016; Endo et al., 2020; Umanzor et al., 2021). In contrast, we found that low nitrogen levels were not physiologically stressful for N. luetkeana or S. latissima sporophytes and high nitrogen levels did not improve survival, growth, or photophysiology at high temperatures over short time periods (8-9 days). Surprisingly, in the species exp., mortality at 21°C was higher for both species in the high N treatment compared to the low N treatment. We found that nitrate levels as low as 1- 3 μM supported high growth rates at cold to moderate temperatures of 9 to 16°C for up to 9 days. These low nitrogen concentrations are similar to the nutrient-poor conditions experienced during the summer by declining kelp populations in southern Puget Sound (Berry et al., 2021). While they were not limiting to kelp growth at any temperature in our experiments, the time span of the experiment (8-9 days) was shorter than the duration of low nutrient levels in South Puget Sound, which can last for months (Berry et al., 2021).
While our study indicated strong physiological effects of elevated temperatures and no deleterious effects of low nitrogen or rescue from thermal stress with the availability of nitrogen, the experimental duration was short. Other studies testing the interactive effects of temperature and nitrogen stress were also conducted over even shorter periods (3 to 5 days: (Fernández et al., 2020; Umanzor et al., 2021). However, after only 8-9 days of exposure to 21°C, kelp in both experiments experienced tissue damage and decay. In the Salish Sea, stressful conditions can span hours, days, weeks or even months in response to tidal cycles, mixing, wind, bathymetry, and other physical factors. For example, subtidal daily maximum seawater temperatures exceeded 16°C for 50 consecutive days in a kelp forest in southern Puget Sound, but warm temperatures occurred at other sites for just a few days (Weigel et al., 2023), and daily maximum temperatures at Cherry Point exceeded 18°C on multiple days in the summer (Figure S3). We hypothesize that longer periods of elevated temperatures would exacerbate blade tissue damage, which was already present after 8-9 days of exposure to 21°C (Figure S4). In contrast, the effects of longer duration nutrient limitation are harder to predict. Blade tissue nitrogen declined significantly after 8-9 days in low N treatments, suggesting that nitrogen stores would become depleted with persistent nutrient limitation. Gerard (1997) found that holding juvenile S. latissima for 1.5 to 6 weeks with no nutrient enrichment depleted internal N reserves to growth-limiting levels of 1.8% nitrogen. In our experiments, high vs. low N treatments had a mean of 3.0 vs. 2.3% and 2.5 vs. 2.0% nitrogen in the population and species exp., respectively. It is possible that the blades could have drawn from internal reserves during the limited duration of our study, as kelps are known to store nitrogen for later use (Gerard, 1982; Korb and Gerard, 2000; Pueschel and Korb, 2001). Our experiments, as well as others, show that nutrient uptake rates can increase with temperature (Harlin and Craigie, 1978; Nishihara et al., 2005; Roleda and Hurd, 2019). Both NO3 and NH4 uptake rates increased with temperature, which could increase blade tissue nitrogen, but only if there is sufficient dissolved inorganic nitrogen available in the environment. Over longer time periods, nutrient limitation likely plays an increasingly important role in kelp physiological performance as reserves are depleted. The magnitude and duration of elevated temperatures and limited nutrients will ultimately drive kelp responses. A more nuanced understanding of stress thresholds related to magnitude and duration may explain, in part, observed patterns of kelp loss and persistence in the Salish Sea and other regions.
Counterintuitively, we found that some aspects of kelp performance were higher in the low N treatment in both experiments. The nutrient concentrations in the species exp. were on target, representing high (10-20 µM) and low (1 µM) NO3 levels in the Salish Sea (Murray et al., 2015; Berry et al., 2021). We note that the population exp. had higher than targeted NO3 levels in the high N treatments (~80 µM), which elevated blade percent nitrogen above field conditions, but both experiments successfully manipulated kelp blade nitrogen content. There are many other kelp studies using excess nitrogen availability that do not report deleterious effects (e.g. 240 µM NO3 in Pueschel and Korb, 2001, 80 µM NO3 Fernández et al., 2020). Because we found similar counterintuitive effects of low N in both experiments, it is unlikely that the excessive nitrogen levels in the population exp. altered our conclusions. Both species grew significantly more biomass in low N treatments in the species exp., and N. luetkeana in the population exp. that were exposed to cool and moderate temperatures grew more in low N treatments. One possible explanation for this counterintuitive growth trend is that kelp grew more to increase blade area for nutrient uptake under nitrogen limited conditions, as they did in the species exp. Analogously, terrestrial plants can increase their root-to-shoot ratio in nitrogen-poor soil (Lopez et al., 2023). In response to nitrate enhancement in the field, blades of M. pyrifera increased in thickness and tissue percent N, but did not elongate faster (Stephens and Hepburn, 2016). It is clear that further physiological work is needed to understand the negative impacts of high N on both kelp species at elevated temperatures, but our experiments strongly suggest that low N levels in the Salish Sea are not a cause of kelp declines.
Saccharina latissima (sugar kelp) is a cosmopolitan species with one of the widest distributions of the Laminariales (Bartsch et al., 2008), found across multiple oceans and a wide range of temperatures. This kelp is also an important food source that is prominent in seaweed aquaculture (Forbord et al., 2012; Visch et al., 2020). There are few studies on S. latissima from the Northeast Pacific, but studies in other locations also found negative physiological responses to warming above 20°C. For example, pigment concentrations and photosynthetic capacity decreased and respiration increased under high temperatures (Andersen et al., 2013; Nepper-Davidsen et al., 2019). Similar to our observations, S. latissima tissue damage and weakness increased at 21°C compared to cooler temperatures (Simonson et al., 2015). We hypothesize that the understory stature of S. latissima, relative to canopy-forming N. luetkeana, may play a role in its ability to persist where N. luetkeana has been lost in areas of the southern Salish Sea (Berry et al., 2021), since it inhabits cooler and deeper water. Additionally, S. latissima had higher nitrogen uptake rates and blade tissue percent nitrogen compared to N. luetkeana, which could be beneficial in nutrient poor waters.
Nereocystis luetkeana is a monotypic genus endemic to the west coast of North America (Abbott and Hollenberg, 1976). Optimal growth of N. luetkeana sporophyte blades occurred at 12°C in British Columbia, Canada (Supratya et al., 2020), while in our study, the highest growth rates occurred at 13 to 16°C. Supratya et al. (2020) also found that N. luetkeana blades were able to elongate at temperatures as high as 20 and 22°C after exposure for 7 days. We found that temperatures up to 16°C for 8-9 days had no negative impacts on the physiology of N. luetkeana, but at 21°C, respiration was significantly elevated, and growth was low to negative. Increased metabolic demand, via elevated respiration, may have prevented kelp from allocating carbon into new biomass. However, while N. luetkeana in the species exp. had low or even negative net photosynthesis rates at 21°C, both populations of N. luetkeana still had positive net photosynthesis at 21°C in the population exp. N. luetkeana is an annual species, with sporophyte growth peaking in late spring to early summer. It is possible that kelp in the species exp. experienced more stressful field conditions prior to the experiment, as the species exp. was conducted later in the growing season (early August).
Other physiological responses to high temperatures, such as lipid degeneration and oxidative damage (Schmid et al., 2020; Umanzor et al., 2021), likely contributed to the low growth rates despite positive net photosynthesis. We also saw signs of tissue degradation at 21°C in both species (Figure S4). Interestingly, we observed blade tissue degradation at 21°C in both nitrogen treatments, and in the species exp., we observed kelp mortality in the 21°C high N treatment, suggesting that tissue damage is a result of high temperature, and not low nutrient levels. In the Salish Sea, other studies report N. luetkeana blade tissue loss, degradation, and negative growth rates at warm, nutrient poor sites during the summer (Berry et al., 2021; Starko et al., 2022). Portions of the Salish Sea experience extremely warm bottom temperatures (up to 20°C, Weigel et al., 2023) relative to other areas within the range of N. luetkeana, such as coastal Pacific Ocean populations (Hamilton et al., 2020). Given our results, recent declines N. luetkeana within the Salish Sea are likely due to elevated temperatures, and prolonged sea surface temperatures > 21°C are likely to lead to further kelp forest declines.
Strong negative effects of ocean warming have been well established for many taxa, including other foundation species (Anthony, 2016; Newcomb et al., 2019; Smale, 2020). Warming rarely occurs in isolation, therefore studies of multiple interacting stressors are necessary to understand rapidly changing environments (Crain et al., 2008; Gunderson et al., 2016; Newcomb et al., 2019). It is important to gain an understanding of the physiological mechanisms for kelp forest declines and responses to multiple stressors, which will help determine priorities for management and restoration actions. Hollarsmith et al. (2022) identified multiple stressor pathways that may be contributing to kelp declines in the Salish Sea, including temperature, nutrients, salinity, water clarity, benthic sedimentation, and algal competition, among others. While we focused on temperature and nutrients, it is likely that other factors such as decreased light availability to benthic life cycle stages are also important in urbanized environments. For example, poor water clarity combined with elevated temperatures exacerbated the loss of Macrocystis pyrifera in New Zealand (Tait et al., 2021). Yet studies on Ecklonia radiata found that nutrient enrichment may alleviate the stress of low light availability due to turbidity (Blain and Shears, 2020), highlighting the complexities of multiple stressors. Future studies in the Salish Sea should examine the interactions among temperature, nutrients, light, and duration of stressors which together may affect competitive outcomes between benthic and canopy species.
We found that sporophytes of S. latissima and N. luetkeana responded similarly and strongly to high temperatures, which were much more stressful than low nitrogen levels. Our results support the prioritization of management actions that limit temperature increases, especially in the coastal oceans and inland seas where temperatures are rising more rapidly (Riche et al., 2014; Khangaonkar et al., 2019; IPCC, 2022). In addition to curtailing emissions, management actions to maintain and restore water circulation (Khangaonkar and Yang, 2011; Khangaonkar and Wang, 2013) could support cooler water temperatures in portions of the Salish Sea. Further, our results demonstrate that management actions involving nutrient additions are unlikely to enhance kelp performance at elevated temperatures. In fact, anthropogenic nutrient additions can exacerbate other kelp stressors, including light limitation and competition with other primary producers (Filbee-Dexter and Wernberg, 2018; Tait et al., 2021). Therefore, important policies to limit eutrophication should remain in place.
Our results highlight the importance of considering elevated temperatures in kelp forest conservation. The deleterious effects of warming to 21°C were consistent across populations of N. luetkeana and between species, suggesting that kelp from different thermal environments are equally susceptible to the effects of ocean warming. Other studies on multiple populations of N. luetkeana in Salish Sea indicated a lack of local adaptation or acclimation with critical temperatures of 16 - 18°C (microscopic sporophytes and gametophytes, respectively) (Weigel et al., 2023). The lack of local adaptation to rising ocean temperatures highlights the need to understand more about genetic connectivity and differences across populations when selecting populations for restoration efforts. Furthering our understanding of the interactive effects of temperature and nutrient stressors on kelp physiology is critical to guide stressor identification and target conservation and restoration.
Data availability statement
The datasets presented in this study can be found in online repositories. The names of the repository/repositories and accession number(s) can be found below: https://doi.org/10.5061/dryad.9p8cz8wpn.
Author contributions
RF: Conceptualization, Data curation, Formal analysis, Funding acquisition, Investigation, Methodology, Project administration, Visualization, Writing – original draft, Writing – review & editing. BW: Conceptualization, Data curation, Formal analysis, Funding acquisition, Investigation, Methodology, Project administration, Visualization, Writing – original draft, Writing – review & editing. EC: Conceptualization, Funding acquisition, Resources, Supervision, Writing – review & editing. HB: Conceptualization, Funding acquisition, Resources, Writing – review & editing. MD: Conceptualization, Funding acquisition, Resources, Writing – review & editing.
Funding
The author(s) declare financial support was received for the research, authorship, and/or publication of this article. This research was funded by the Washington State Legislature 2021-23 proviso for kelp conservation awarded to MD and BW, and a U.S. Geological Survey Northwest Climate Adaptation Science Center award G17AC000218 to RF.
Acknowledgments
We thank Sadie Small, Naomi Hiʻiaka Vliet, Eleanor Rollins, Aaron Ninokawa, Yiyi Li, Nichos Molnar, Leta Dawson, Grace Leuchtenberger, and Josephine Grell for assistance in the laboratory, and Carla Narvaez for assistance with scuba diving collections. We also thank the staff at Friday Harbor Labs for logistical support and Jennifer Ruesink for helpful comments on the manuscript.
Conflict of interest
The authors declare that the research was conducted in the absence of any commercial or financial relationships that could be construed as a potential conflict of interest.
Publisher’s note
All claims expressed in this article are solely those of the authors and do not necessarily represent those of their affiliated organizations, or those of the publisher, the editors and the reviewers. Any product that may be evaluated in this article, or claim that may be made by its manufacturer, is not guaranteed or endorsed by the publisher.
Supplementary material
The Supplementary Material for this article can be found online at: https://www.frontiersin.org/articles/10.3389/fmars.2023.1281104/full#supplementary-material
References
Abbott I. A., Hollenberg G. J. (1976). Marine algae of California (Stanford, CA, United States: Stanford University Press).
Andersen G. S., Pedersen M. F., Nielsen S. L. (2013). Temperature acclimation and heat tolerance of photosynthesis in Norwegian Saccharina latissima (Laminariales, Phaeophyceae). J. Phycol. 49, 689–700. doi: 10.1111/jpy.12077
Anthony K. R. N. (2016). Coral reefs under climate change and ocean acidification: Challenges and opportunities for management and policy. Annu. Rev. Environ. Resour. 41, 59–81. doi: 10.1146/annurev-environ-110615-085610
Arafeh-Dalmau N., Montaño-Moctezuma G., Martínez J. A., Beas-Luna R., Schoeman D. S., Torres-Moye G. (2019). Extreme marine heatwaves alter kelp forest community near its equatorward distribution limit. Front. Mar. Sci. 6. doi: 10.3389/fmars.2019.00499
Bartsch I., Wiencke C., Bischof K., Buchholz C. M., Buck B. H., Eggert A., et al. (2008). The genus Laminaria sensu lato: recent insights and developments. Eur. J. Phycol. 43, 1–86. doi: 10.1080/09670260701711376
Becheler R., Haverbeck D., Clerc C., Montecinos G., Valero M., Mansilla A., et al. (2022). Variation in thermal tolerance of the giant kelp’s gametophytes: Suitability of habitat, population quality or local adaptation? Front. Mar. Sci. 9. doi: 10.3389/fmars.2022.802535
Bell L. E., Kroeker K. J. (2022). Standing crop, turnover, and production dynamics of Macrocystis pyrifera and understory species Hedophyllum nigripes and Neoagarum fimbriatum in high latitude giant kelp forests. J. Phycol. 58, 773–788. doi: 10.1111/jpy.13291
Bennett S., Duarte C. M., Marbà N., Wernberg T. (2019). Integrating within-species variation in thermal physiology into climate change ecology. Philos. Trans. R. Soc B Biol. Sci. 374, 20180550. doi: 10.1098/rstb.2018.0550
Berry H. D., Mumford T. F., Christiaen B., Dowty P., Calloway M., Ferrier L., et al. (2021). Long-term changes in kelp forests in an inner basin of the Salish Sea. PloS One 16, e0229703. doi: 10.1371/journal.pone.0229703
Biskup S., Bertocci I., Arenas F., Tuya F. (2014). Functional responses of juvenile kelps, Laminaria ochroleuca and Saccorhiza polyschides, to increasing temperatures. Aquat. Bot. 113, 117–122. doi: 10.1016/j.aquabot.2013.10.003
Blain C. O., Shears N. T. (2020). Nutrient enrichment offsets the effects of low light on growth of the kelp Ecklonia radiata. Limnol. Oceanogr. 65, 2220–2235. doi: 10.1002/lno.11449
Brown J. H., Gillooly J. F., Allen A. P., Savage V. M., West G. B. (2004). Toward a metabolic theory of ecology. Ecology 85, 1771–1789. doi: 10.1890/03-9000
Crain C. M., Kroeker K., Halpern B. S. (2008). Interactive and cumulative effects of multiple human stressors in marine systems. Ecol. Lett. 11, 1304–1315. doi: 10.1111/j.1461-0248.2008.01253.x
Dillon M. E., Wang G., Huey R. B. (2010). Global metabolic impacts of recent climate warming. Nature 467, 704–706. doi: 10.1038/nature09407
Endo H., Inomata E., Gao X., Kinoshita J., Sato Y., Agatsuma Y. (2020). Heat stress promotes nitrogen accumulation in meristems via apical blade erosion in a brown macroalga with intercalary growth. Front. Mar. Sci. 7. doi: 10.3389/fmars.2020.575721
Fernández P. A., Gaitán-Espitia J. D., Leal P. P., Schmid M., Revill A. T., Hurd C. L. (2020). Nitrogen sufficiency enhances thermal tolerance in habitat-forming kelp: implications for acclimation under thermal stress. Sci. Rep. 10, 3186. doi: 10.1038/s41598-020-60104-4
Filbee-Dexter K., Wernberg T. (2018). Rise of turfs: A new battlefront for globally declining kelp forests. BioScience 68, 64–76. doi: 10.1093/biosci/bix147
Forbord S., Etter S. A., Broch O. J., Dahlen V. R., Olsen Y. (2021). Initial short-term nitrate uptake in juvenile, cultivated Saccharina latissima (Phaeophyceae) of variable nutritional state. Aquat. Bot. 168, 103306. doi: 10.1016/j.aquabot.2020.103306
Forbord S., Skjermo J., Arff J., Handå A., Reitan K. I., Bjerregaard R., et al. (2012). Development of Saccharina latissima (Phaeophyceae) kelp hatcheries with year-round production of zoospores and juvenile sporophytes on culture ropes for kelp aquaculture. J. Appl. Phycol. 24, 393–399. doi: 10.1007/s10811-011-9784-y
Fox J., Weisberg S. (2019) An {R} Companion to Applied Regression. Available at: https://socialsciences.mcmaster.ca/jfox/Books/Companion/.
Franco J. N., Tuya F., Bertocci I., Rodríguez L., Martínez B., Sousa-Pinto I., et al. (2018). The ‘golden kelp’ Laminaria ochroleuca under global change: Integrating multiple eco-physiological responses with species distribution models. J. Ecol. 106, 47–58. doi: 10.1111/1365-2745.12810
Gao X., Endo H., Nagaki M., Agatsuma Y. (2016). Growth and survival of juvenile sporophytes of the kelp Ecklonia cava in response to different nitrogen and temperature regimes. Fish. Sci. 82, 623–629. doi: 10.1007/s12562-016-0998-4
Gauci C., Bartsch I., Martins N., Liesner D. (2022). Cold thermal priming of Laminaria digitata (Laminariales, Phaeophyceae) gametophytes enhances gametogenesis and thermal performance of sporophytes. Front. Mar. Sci. 9. doi: 10.3389/fmars.2022.862923
Gerard V. A. (1982). Growth and utilization of internal nitrogen reserves by the giant kelp Macrocystis pyrifera in a low-nitrogen environment. Mar. Biol. 66, 27–35. doi: 10.1007/BF00397251
Gerard V. A. (1997). The role of nitrogen nutrition in high-temperature tolerance of the kelp, Laminaria saccharina (Chromophyta). J. Phycol. 33, 800–810. doi: 10.1111/j.0022-3646.1997.00800.x
Guenther R., Porcher E., Carrington E., Martone P. (2022). Effects of temperature and pH on the growth, calcification, and biomechanics of two species of articulated coralline algae. Mar. Ecol. Prog. Ser. 700, 79–93. doi: 10.3354/meps14166
Gunderson A. R., Armstrong E. J., Stillman J. H. (2016). Multiple stressors in a changing world: The need for an improved perspective on physiological responses to the dynamic marine environment. Annu. Rev. Mar. Sci. 8, 357–378. doi: 10.1146/annurev-marine-122414-033953
Hamilton S. L., Bell T. W., Watson J. R., Grorud-Colvert K. A., Menge B. A. (2020). Remote sensing: generation of long-term kelp bed data sets for evaluation of impacts of climatic variation. Ecology 101, e03031. doi: 10.1002/ecy.3031
Harlin M. M., Craigie J. S. (1978). Nitrate uptake by Laminaria longicruris (Phaeophyceae). J. Phycol. 14, 464–467. doi: 10.1111/j.1529-8817.1978.tb02470.x
Hollarsmith J. A., Andrews K., Naar N., Starko S., Calloway M., Obaza A., et al. (2022). Toward a conceptual framework for managing and conserving marine habitats: A case study of kelp forests in the Salish Sea. Ecol. Evol. 12, e8510. doi: 10.1002/ece3.8510
IPCC (2022). Climate Change 2022: Impacts, Adaptation and Vulnerability Contribution of Working Group II to the Sixth Assessment Report of the Intergovernmental Panel on Climate Change. Available at: https://www.ipcc.ch/report/ar6/wg2/downloads/report/IPCC_AR6_WGII_Citation.pdf (Accessed May 14, 2023).
Intergovernmental Oceanography Commission (1994). Protocols for the joint global ocean flux study (JGOFS) core measurements. (Paris, France: IOC Manuals and Guides 29, UNESCO).
Khangaonkar T., Nugraha A., Xu W., Balaguru K. (2019). Salish Sea response to global climate change, sea level rise, and future nutrient loads. J. Geophys. Res. Oceans 124, 3876–3904. doi: 10.1029/2018JC014670
Khangaonkar T., Nugraha A., Yun S. K., Premathilake L., Keister J. E., Bos J. (2021). Propagation of the 2014–2016 Northeast Pacific marine heatwave through the Salish Sea. Front. Mar. Sci. 8. doi: 10.3389/fmars.2021.787604
Khangaonkar T., Wang T. (2013). Potential alteration of fjordal circulation due to a large floating structure—Numerical investigation with application to Hood Canal basin in Puget Sound. Appl. Ocean Res. 39, 146–157. doi: 10.1016/j.apor.2012.11.003
Khangaonkar T., Yang Z. (2011). A high-resolution hydrodynamic model of Puget Sound to support nearshore restoration feasibility analysis and design. Ecol. Restor. 29, 173–184. doi: 10.3368/er.29.1-2.173
King N. G., McKeown N. J., Smale D. A., Moore P. J. (2018) The importance of phenotypic plasticity and local adaptation in driving intraspecific variability in thermal niches of marine macrophytes Ecography 41, 1469–1484. doi: 10.1111/ecog.03186
Korb R., Gerard V. (2000). Nitrogen assimilation characteristics of polar seaweeds from differing nutrient environments. Mar. Ecol. Prog. Ser. 198, 83–92. doi: 10.3354/meps198083
Krumhansl K. A., Okamoto D. K., Rassweiler A., Novak M., Bolton J. J., Cavanaugh K. C., et al. (2016). Global patterns of kelp forest change over the past half-century. Proc. Natl. Acad. Sci. 113, 13785–13790. doi: 10.1073/pnas.1606102113
Lamb A., Hanby B. P. (2005). ““Brown algae phylum Ochrophyta,”,” in Marine Life of the Pacific Northwest: A Photographic Encyclopedia of Invertebrates, Seaweeds and Selected Fishes (Madeira Park, British Columbia, Canada: Harbour Publishing), 29–36.
Lenth R. V., Bolker B., Buerkner P., Giné-Vázquez I., Herve M., Jung M., et al. (2023) emmeans: Estimated Marginal Means, aka Least-Squares Means. Available at: https://cran.r-project.org/web/packages/emmeans/index.html (Accessed May 14, 2023).
Liesner D., Shama L. N. S., Diehl N., Valentin K., Bartsch I. (2020). Thermal plasticity of the kelp Laminaria digitata (Phaeophyceae) across life cycle stages reveals the importance of cold seasons for marine forests. Front. Mar. Sci. 7. doi: 10.3389/fmars.2020.00456
Lopez G., Ahmadi S. H., Amelung W., Athmann M., Ewert F., Gaiser T., et al. (2023). Nutrient deficiency effects on root architecture and root-to-shoot ratio in arable crops. Front. Plant Sci. 13. doi: 10.3389/fpls.2022.1067498
Lüning K., Freshwater W. (1988). Temperature tolerance of Northeast Pacific marine algae. J. Phycol. 24, 310–315. doi: 10.1111/j.1529-8817.1988.tb04471.x
Mabin C., Gribben P., Fischer A., Wright J. (2013). Variation in the morphology, reproduction and development of the habitat-forming kelp Ecklonia radiata with changing temperature and nutrients. Mar. Ecol. Prog. Ser. 483, 117–131. doi: 10.3354/meps10261
Martins N., Tanttu H., Pearson G. A., Serrão E. A., Bartsch I. (2017). Interactions of daylength, temperature and nutrients affect thresholds for life stage transitions in the kelp Laminaria digitata (Phaeophyceae). Bot. Mar. 60, 109–121. doi: 10.1515/bot-2016-0094
Murray J. W., Roberts E., Howard E., O’Donnell M., Bantam C., Carrington E., et al. (2015). An inland sea high nitrate-low chlorophyll (HNLC) region with naturally high pCO2. Limnol. Oceanogr. 60, 957–966. doi: 10.1002/lno.10062
Muth A. F., Graham M. H., Lane C. E., Harley C. D. G. (2019). Recruitment tolerance to increased temperature present across multiple kelp clades. Ecology 100, e02594. doi: 10.1002/ecy.2594
Nepper-Davidsen J., Andersen D., Pedersen M. (2019). Exposure to simulated heatwave scenarios causes long-term reductions in performance in Saccharina latissima. Mar. Ecol. Prog. Ser. 630, 25–39. doi: 10.3354/meps13133
Newcomb L. A., George M. N., O’Donnell M. J., Carrington E. (2019). Only as strong as the weakest link: structural analysis of the combined effects of elevated temperature and pCO2 on mussel attachment. Conserv. Physiol. 7, coz068. doi: 10.1093/conphys/coz068
Nishihara G. N., Terada R., Noro T. (2005). Effect of temperature and irradiance on the uptake of ammonium and nitrate by Laurencia brongniartii (Rhodophyta, Ceramiales). J. Appl. Phycol. 17, 371–377. doi: 10.1007/s10811-005-5519-2
O’Donnell M. J., George M. N., Carrington E. (2013). Mussel byssus attachment weakened by ocean acidification. Nat. Clim. Change 3, 587–590. doi: 10.1038/nclimate1846
Palacios D. M., Hazen E. L., Schroeder I. D., Bograd S. J. (2013). Modeling the temperature-nitrate relationship in the coastal upwelling domain of the California Current. J. Geophys. Res. Oceans 118, 3223–3239. doi: 10.1002/jgrc.20216
Pfister C. A., Wootton J. T., Neufeld C. J. (2007). The relative roles of coastal and oceanic processes in determining physical and chemical characteristics of an intensively sampled nearshore system. Limnol. Oceanogr. 52, 1767–1775. doi: 10.4319/lo.2007.52.5.1767
Pinsky M. L., Eikeset A. M., McCauley D. J., Payne J. L., Sunday J. M. (2019). Greater vulnerability to warming of marine versus terrestrial ectotherms. Nature 569, 108–111. doi: 10.1038/s41586-019-1132-4
Pueschel C. M., Korb R. E. (2001). Storage of nitrogen in the form of protein bodies in the kelp Laminaria solidungula. Mar. Ecol. Prog. Ser. 218, 107–114. doi: 10.3354/meps218107
Riche O., Johannessen S. C., Macdonald R. W. (2014). Why timing matters in a coastal sea: Trends, variability and tipping points in the Strait of Georgia, Canada. J. Mar. Syst. 131, 36–53. doi: 10.1016/j.jmarsys.2013.11.003
Rogers-Bennett L., Catton C. A. (2019). Marine heat wave and multiple stressors tip bull kelp forest to sea urchin barrens. Sci. Rep. 9, 15050. doi: 10.1038/s41598-019-51114-y
Roleda M. Y., Hurd C. L. (2019). Seaweed nutrient physiology: application of concepts to aquaculture and bioremediation. Phycologia 58, 552–562. doi: 10.1080/00318884.2019.1622920
R Core Team (2021) R: A language and environment for statistical computing. R Foundation for Statistical Computing. (Vienna, Austria. Available at: https://www.R-project.org/
Schiel D. R., Steinbeck J. R., Foster M. S. (2004). Ten years of induced ocean warming causes comprehensive changes in marine benthic communities. Ecology 85, 1833–1839. doi: 10.1890/03-3107
Schmid M., Fernández P. A., Gaitán-Espitia J. D., Virtue P., Leal P. P., Revill A. T., et al. (2020). Stress due to low nitrate availability reduces the biochemical acclimation potential of the giant kelp Macrocystis pyrifera to high temperature. Algal Res. 47, 101895. doi: 10.1016/j.algal.2020.101895
Schneider C. A., Rasband W. S., Eliceiri K. W. (2012). NIH Image to ImageJ: 25 years of image analysis. Nat. Methods 9, 671–675. doi: 10.1038/nmeth.2089
Simonson E. J., Scheibling R. E., Metaxas A. (2015). Kelp in hot water: I. Warming seawater temperature induces weakening and loss of kelp tissue. Mar. Ecol. Prog. Ser. 537, 89–104. doi: 10.3354/meps11438
Smale D. A. (2020). Impacts of ocean warming on kelp forest ecosystems. New Phytol. 225, 1447–1454. doi: 10.1111/nph.16107
Staehr P. A., Wernberg T. (2009). Physiological responses of Ecklonia radiata (Laminariales) to a latitudinal gradient in ocean temperature. J. Phycol. 45, 91–99. doi: 10.1111/j.1529-8817.2008.00635.x
Starko S., Bailey L. A., Creviston E., James K. A., Warren A., Brophy M. K., et al. (2019). Environmental heterogeneity mediates scale-dependent declines in kelp diversity on intertidal rocky shores. PloS One 14, e0213191. doi: 10.1371/journal.pone.0213191
Starko S., Neufeld C. J., Gendall L., Timmer B., Campbell L., Yakimishyn J., et al. (2022). Microclimate predicts kelp forest extinction in the face of direct and indirect marine heatwave effects. Ecol. Appl. 32, e2673. doi: 10.1002/eap.2673
Stephens T. A., Hepburn C. D. (2016). A kelp with integrity: Macrocystis pyrifera prioritises tissue maintenance in response to nitrogen fertilisation. Oecologia 182, 71–84. doi: 10.1007/s00442-016-3641-2
Supratya V. P., Coleman L. J. M., Martone P. T. (2020). Elevated temperature affects phenotypic plasticity in the bull kelp (Nereocystis luetkeana, Phaeophyceae). J. Phycol. 56, 1534–1541. doi: 10.1111/jpy.13049
Tait L. W., Thoral F., Pinkerton M. H., Thomsen M. S., Schiel D. R. (2021). Loss of giant kelp, Macrocystis pyrifera, driven by marine heatwaves and exacerbated by poor water clarity in New Zealan. Front. Mar. Sci. 8. doi: 10.3389/fmars.2021.721087
Umanzor S., Sandoval-Gil J. M., Sánchez-Barredo M., Ladah L. B., Ramírez-García M.-M., Zertuche-González J. A. (2021) Short-term stress responses and recovery of giant kelp (Macrocystis pyrifera, Laminariales, Phaeophyceae) juvenile sporophytes to a simulated marine heatwave and nitrate scarcity J. Phycol. 57, 1604-1618. doi: 10.1111/jpy.13189
Visch W., Kononets M., Hall P. O. J., Nylund G. M., Pavia H. (2020). Environmental impact of kelp (Saccharina latissima) aquaculture. Mar. pollut. Bull. 155, 110962. doi: 10.1016/j.marpolbul.2020.110962
Vranken S., Wernberg T., Scheben A., Severn-Ellis A. A., Batley J., Bayer P. E., et al. (2021). Genotype–Environment mismatch of kelp forests under climate change. Mol. Ecol. 30, 3730–3746. doi: 10.1111/mec.15993
Weigel B. L., Pfister C. A. (2021). The dynamics and stoichiometry of dissolved organic carbon release by kelp. Ecology 102, e03221. doi: 10.1002/ecy.3221
Weigel B. L., Small S. L., Berry H. D., Dethier M. N. (2023) Effects of temperature and nutrients on microscopic stages of the bull kelp (Nereocystis luetkeana, Phaeophyceae) J. Phycol. 59, 893-907. doi: 10.1111/jpy.13366
Wernberg T., Coleman M. A., Bennett S., Thomsen M. S., Tuya F., Kelaher B. P. (2018). Genetic diversity and kelp forest vulnerability to climatic stress. Sci. Rep. 8, 1851. doi: 10.1038/s41598-018-20009-9
Williams G. D., Levin P. S., Palsson W. A. (2010). Rockfish in Puget Sound: An ecological history of exploitation. Mar. Policy 34, 1010–1020. doi: 10.1016/j.marpol.2010.02.008
Keywords: thermal stress, foundation species, Laminariales, physiology, metabolism, photosynthesis, respiration, nutrient uptake
Citation: Fales RJ, Weigel BL, Carrington E, Berry HD and Dethier MN (2023) Interactive effects of temperature and nitrogen on the physiology of kelps (Nereocystis luetkeana and Saccharina latissima). Front. Mar. Sci. 10:1281104. doi: 10.3389/fmars.2023.1281104
Received: 24 August 2023; Accepted: 06 November 2023;
Published: 28 November 2023.
Edited by:
Thanos Dailianis, Hellenic Centre for Marine Research (HCMR), GreeceReviewed by:
Caitlin Blain, The University of Auckland, New ZealandSara Gonzalez, Woods Hole Oceanographic Institution, United States
Copyright © 2023 Fales, Weigel, Carrington, Berry and Dethier. This is an open-access article distributed under the terms of the Creative Commons Attribution License (CC BY). The use, distribution or reproduction in other forums is permitted, provided the original author(s) and the copyright owner(s) are credited and that the original publication in this journal is cited, in accordance with accepted academic practice. No use, distribution or reproduction is permitted which does not comply with these terms.
*Correspondence: Robin J. Fales, robinfales.eco@gmail.com
†These authors have contributed equally to this work and share first authorship