Analysis of reproductive traits reveals complex population dynamics on a small geographical scale in Atlantic herring
- 1Department of Biology, University of Bergen, Bergen, Norway
- 2GEOMAR Helmholtz Centre for Ocean Research Kiel, Evolutionary Ecology of Marine Fishes, Kiel, Germany
- 3Institute of Marine Research, Nordnes, Bergen, Norway
- 4Marine and Freshwater Research Institute, Hafnarfjörður, Iceland
Atlantic herring (Clupea harengus) has a complex population structure and displays a variety of reproductive strategies. Differences in reproductive strategies among herring populations are linked to their time of spawning, as well as to their reproductive investment which can be an indicator for migratory vs. stationary behavior. These differences are reflected in the number of oocytes (fecundity) and the size of the oocytes prior spawning. We studied potential mixing of herring with different reproductive strategies during the spring spawning season on a coastal spawning ground. It has been hypothesized that both spring and autumn spawning herring co-occur on this specific spawning ground. Therefore, we investigated the reproductive traits oocyte size, fecundity, fertilization success as well as length of the hatching larvae during the spring spawning season from February to April. We used a set of 11 single nucleotide polymorphism markers (SNPs), which are associated with spawning season, to genetically identify autumn and spring spawning herring. Reproductive traits were investigated separately within these genetically distinct spawning types. Furthermore, we used multivariate analyses to identify groups with potentially different reproductive strategies within the genetic spring spawners. Our results indicate that mixing between ripe spring and autumn spawners occurs on the spawning ground during spring, with ripe autumn spawners being generally smaller but having larger oocytes than spring spawners. Within spring spawners, we found large variability in reproductive traits. A following multivariate cluster analysis indicated two groups with different reproductive investment. Comparisons with other herring populations along the Norwegian coastline suggest that the high variability can be explained by the co-occurrence of groups with different reproductive investments potentially resulting from stationary or migratory behavior. Fertilization success and the length of the hatching larvae decreased with progression of the spawning season, with strong inter-individual variation, supporting our findings. Incorporating such complex population dynamics into management strategies of this species will be essential to build its future population resilience.
Introduction
Knowledge of population origin and dynamics of marine fishes is of prior importance for the successful management of natural populations. Although many marine fish populations are considered connected and weakly structured, complex population structure in the marine environment is more common than previously thought (Ruzzante et al., 2006; Ciannelli et al., 2013). Neglecting the population structure and dynamics of harvested species may cause local overexploitation and depletion (Reiss et al., 2009). This can in turn reduce population diversity and the scope to respond to natural and anthropogenically induced changes (Smith et al., 1991; Smedbol and Stephenson, 2001). Separation of exploited populations as well as assignment of individuals to populations is thus necessary in a management context but requires variability in a trait between populations. Such variability within a trait may be caused by the direct effects of the environment on the phenotype, known as phenotypic plasticity, or by the underlying genotype (Via et al., 1995). To date, fish populations are mainly separated by morphology, life-history traits or their degree of genetic differentiation (Cadrin et al., 2013).
Life-history traits can vary substantially between species as well as populations of marine fishes. There are a variety of reproductive strategies, reflected in different spawning times, the frequency of spawning within one reproductive season (batch versus total spawners) and the degree of reproductive investment (Murua and Saborido-Rey, 2003; McBride et al., 2015). Among populations of the same species, spawning time is an important driver of population differentiation (Tallman, 1986; Quinn et al., 2000; Martinez Barrio et al., 2016; Balazik et al., 2017). At the same time, it may be used to assign individuals to populations spawning in different seasons (Ntiba and Jaccarini, 1990; Brophy et al., 2006). Traditionally, this has been done on a phenotypic level by maturity staging (Berg et al., 2021). However, the fast development of genetic methods including whole genome sequencing has resulted in the discovery of single nucleotide polymorphism markers (SNPs) identifying the specific spawning season (Kerr et al., 2019; O’Donnell and Sullivan, 2021). Investigating the reproductive investment in terms of oocyte size and fecundity on the other hand can give deeper insights into the ecology of a species and may be used to estimate the recruitment potential of a population (Hughes et al., 2000; Armstrong and Witthames, 2012). Several factors influence the reproductive investment of marine fish and these different strategies investing more or less energy in reproduction are often linked to the migration behavior. Migration behavior is a metabolic cost that influences how much energy can be allocated to reproduction (Stearns, 1989; Kinnison et al., 2003; Christie et al., 2018). Reproductive investment hence is larger in stationary populations than in migratory ones (Leggett and Carscadden, 1978; Silva et al., 2013) and could be a factor limiting effective migration and thus gene flow among populations.
A typical example with both migratory and stationary populations is the Atlantic herring (Clupea harengus), an ecologically and economically important species in the North-East Atlantic. This species is known for its complex population structure (Martinez Barrio et al., 2016; Feng et al., 2017; Han et al., 2020). Herring populations differ largely in their degree of reproductive investment which is reflected in the size and number of oocytes (fecundity) just prior to spawning (Hempel and Blaxter, 1967; Silva et al., 2013; dos Santos Schmidt et al., 2021). Furthermore, population specific spawning times exist in this species, with most populations spawning in either spring or autumn. Recent studies identified SNPs in herring that are highly associated with the spawning season (Bekkevold et al., 2016; Lamichhaney et al., 2017), and they are successfully used to discriminate herring with different spawning seasons (Kerr et al., 2019; Bekkevold et al., 2023). Early works on the reproductive strategies of Atlantic herring by Hempel and Blaxter (1967) as well as more recent studies (Damme et al., 2009; dos Santos Schmidt et al., 2021) confirm a clear difference in oocyte size and fecundity among herring populations spawning in these opposite seasons. Herring spawning in spring produce less but larger eggs matching the prevailing environmental conditions at the time of hatching when critical time windows before the onset of the spring bloom need to be bridged (Cushing, 1990). In contrast, autumn spawners show higher fecundities and smaller oocytes (dos Santos Schmidt et al., 2021).
Herring with different spawning seasons and migratory behaviors are being recognized along the Norwegian west coast. Spring spawning herring in these waters can be divided into highly migratory Norwegian spring spawning herring (NSSH) and stationary spring spawning populations. NSSH undergo extensive seasonal migrations between their feeding grounds in the Norwegian Sea (Dragesund et al., 1980; Slotte, 1999; Varpe et al., 2005) and their spawning grounds along the Norwegian coast (Dragesund et al., 1997). Migration patterns and the utilization of spawning grounds have changed in response to population size over time and are considered highly plastic (Dragesund et al., 1997; Huse et al., 2010). In contrast, stationary spring spawning herring populations are geographically restricted populations which are adapted to local conditions, completing their entire life cycle within fjords (Aasen, 1952; Mikkelsen et al., 2018), semi-enclosed basins (Johannessen et al., 2009) or brackish lakes (Eggers et al., 2014). Differences in reproductive strategies in terms of reproductive investment can be linked to divergent life-history strategies of migratory and stationary herring populations. Stationary herring populations show higher reproductive investment displayed by smaller oocytes and higher fecundity, while their oceanic migrating counterparts invest less in reproduction having larger oocytes but lower fecundity (Silva et al., 2013; dos Santos Schmidt et al., 2021). Further growth of herring is reported to be different between stationary and migratory populations, where stationary herring were generally found to be smaller than migratory (Silva et al., 2013; Johannessen et al., 2014). In addition to spring spawners, autumn spawners are found along the Norwegian coast which show contrasting reproductive strategies (Husebø et al., 2005). A particular spawning ground of interest can be found at the south west coast of Norway where ripe autumn and spring spawning herring have been observed, mixing both during autumn and spring (Berg et al., 2021). Spawning herring can be found from February to June at this spawning ground clearly extending the typical spawning period of NSSH. During autumn, ripe spring spawning herring are also present, but in much lower numbers compared to the spring season (Berg et al., 2021). Therefore, it could be hypothesized that spring spawners being present in autumn are stationary, while others show migratory behavior and only return for spawning. Furthermore, earlier findings showed a large difference in egg size (Berg et al., 2019) suggesting different reproductive strategies even though this was based on less than 10 spring spawners. Therefore, we investigate several reproductive traits over the spring season to identify groups with different reproductive strategies.
We hypothesized that herring with different reproductive strategies are present at the local spawning ground during the spring spawning season. In the first step, we used genetic methods to identify autumn and spring spawners based on recently identified SNPs (Lamichhaney et al., 2017; Han et al., 2020). This allows us to investigate reproductive traits (e.g. oocyte size) separately among these two reproductive strategies. Furthermore, we use a multivariate approach based on the reproductive traits to identify clusters within the spring spawners which could potentially by linked to different reproductive strategies. Lastly, we compared the variability in reproductive traits observed on the coastal spawning ground with other populations found along the Norwegian coastline. To answer the formulated hypothesis, we sampled herring on the spawning ground from February to April and analyzed the reproductive traits oocyte size, fecundity, fertilization success as well as length of the hatching larvae.
Materials and methods
Study area and sampling
Atlantic herring from different sampling locations were included in this study. The main sampling location was along the Norwegian south-west coast inside Herdlefjorden approximately 26 km northwest of Bergen (60°34’11.2”N, 5°0’18.9”E, Figure S1) where herring were caught by gillnets on a coastal spawning ground (6 – 20 m), hereafter referred to as Askøy. A total number of 448 herring were caught and analyzed during the spring spawning season in March 2018 and from February until April 2019 (Table 1). Gillnets of three different mesh sizes (29, 31, 34 mm) were used at each sampling date ensuring fish of different length classes, as well as maturity stages were caught. In addition to herring from Askøy, samples from two well described herring populations were collected as reference material for comparison of reproductive traits; 1. Norwegian spring spawning herring (NSSH), and 2. North Sea autumn spawning herring (NSASH). Pre-spawning NSSH samples were caught during a research cruise in February 2014 (62°40’N, 5°29’E, Figure S1) and pre-spawning NSASH samples originated from commercial landings in the North Sea (59°15’N, 1°30’E, Figure S1) in September 2013 (dos Santos Schmidt et al., 2017). Furthermore, samples of NSSH collected near its main spawning ground (62°40’N, 5°29’E) in 2018 and 2019 during the annual NSSH spawning survey were used for a length-class comparison between Askøy samples and NSSH.
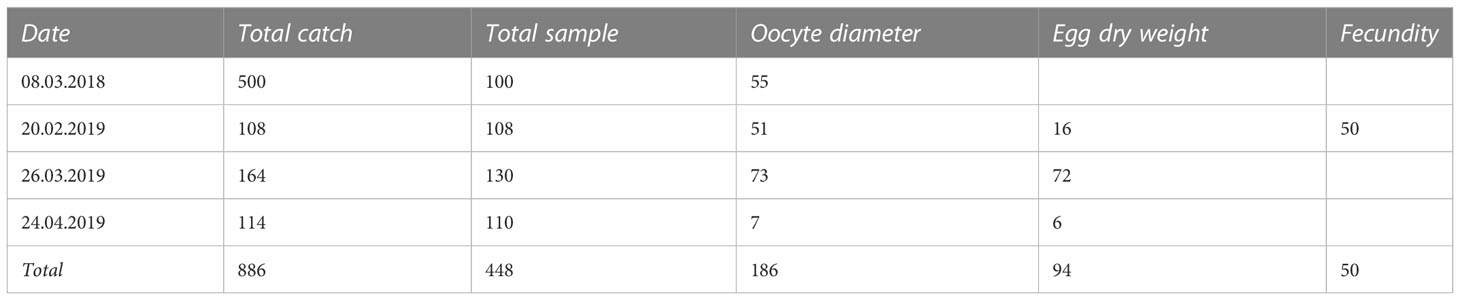
Table 1 Number of individuals sampled at the coastal spawning ground Askøy and analyzed for different morphometric and life-history traits per sampling date.
Genetic analysis
A total of 448 herring were used for genotyping. DNA was extracted from fin clips using the DNeasy 96 Blood & Tissue Kit (Qiagen, Germany). All samples were genotyped for 11 SNPs with specifically designed primers, and amplification were organized in multiplex reactions. Genotype calling was performed on a MassARRAY® platform using an iPLEX® reaction (Agena Bioscience, n.d.), as described by Gabriel et al. (2009). The selected 11 SNPs are all associated with spawning time and can consequently identify different spawning seasons, mainly spring vs. autumn. Markers were selected based on sequence data and inference from Han et al. (2020) and Lamichhaney et al. (2017). The chromosomal location of SNPs and their power to discriminate spawning season (Lamichhaney et al., 2017; Kerr et al., 2019; Han et al., 2020) as well as the segregation of genotypes (e.g., Hardy-Weinberg and linkage dis-equilibrium; Bekkevold et al., 2023) are well documented. SNPs sequences are given in Supplementary Table S1. In addition to Askøy samples, we have genotyped NSASH, western Baltic Skagerrak spring spawning herring (WBSS-SK) and NSSH as references sampled which have recently been described in a genetic stock identification study (Bekkevold et al., 2023). Sampling location and dates of the reference samples are summarized in Table S2 and in more detail in Bekkevold et al. (2023). The reference samples of NSASH and NSSH represent the same populations as used for the reproductive traits. Prior to any statistical analysis, extensive data quality checks were performed on the SNPs to ensure good clustering and low levels of missing data. Individuals missing ≥25% of genotype calls were excluded from the analysis (N = 27) which resulted in the removal of 6 females from the oocyte diameter data.
Morphometric measurements
All following described methods were identically applied for all samples collected from Askøy as well as for the two reference samples of NSSH and NSASH. Samples were processed using exactly the same methodology at the same laboratory, deviations are clearly marked. For each fish individual total length (TL) to the nearest 0.1 cm (0.5 cm for reference samples) and whole-body wet weight (W) to the nearest 0.1 g (nearest g for reference samples) was recorded. The gonads were carefully dissected, and fresh gonad weight (GW) was recorded to the nearest 0.1 g. A subsample of the ovary was collected from the center of the right lobe to be used in the fecundity and egg dry weight analysis. Gonad samples were immediately fixed using a 3.6% phosphate-buffered formaldehyde solution. Eight different maturity stages were discriminated based on visual inspection of the gonads: 1-2, immature; 3-5, maturing and/or pre-spawning; 6, spawning/ripe; 7, spent; and 8, resting stage (Mjanger et al., 2007). Since many of the fish were in spawning condition (stage 6 or above, Figure S2) we used somatic weight (Ws), somatic condition factor (Ks) and, somatic gonadosomatic index (GSIS). Only for fecundity data we used Fulton`s condition (K). Indices were calculated by the following equations:
Oocyte diameter and fecundity analysis
Female herring were either in vitellogenesis or hydration stage, classified macroscopically during the wholemount analysis. Oocytes in vitellogenic stage are still surrounded by the follicle, whereas hydrated oocytes are released from the follicle in the ovarian cavity. The following analyses were restricted to hydrated oocytes, hereafter only referred to as oocytes. Only for the fecundity analysis we used both vitellogenic and hydrated oocytes. It should be noted that on the early and late sampling dates (February and April 2019) most fish were either in vitellogenic stage (maturity stage 4/5) or spent/regenerating (maturity stage 7/8) and thus not included in the analyses (Figure S2). The term egg only refers to oocytes that would be released to the external environment (spawned; Heins and Brown-Peterson, 2022) and is only used in the context of the fertilization experiments. However, an exception is the term egg dry weight which in this study refers to the dry weight of hydrated oocytes.
The mean oocyte diameter (OD) per sample was determined through image analysis (auto diametric method; Thorsen and Kjesbu, 2001). Any oocyte that showed signs of atresia such as an enlarged or irregular shape (Corriero et al., 2021) was excluded from the analysis. Potential fecundity (FP) was determined by the equation (dos Santos Schmidt et al., 2017):
Subsequently, relative somatic fecundity (RFP,S) was calculated as:
The power function to calculate FP was established for NSSH, however, it has been found to be consistent across different herring populations (dos Santos Schmidt et al., 2017) and was considered appropriate for the herring samples in this study (see Figure S3). Only fecundity estimates for Askøy from February 2019 were used later during the analysis, since for the other dates fecundity is likely underestimated due to egg losses before and during sampling. We nevertheless investigated relative somatic fecundity estimates across the different sampling dates (Figure S4) to reveal overall variability in fecundity among fish from the coastal spawning ground, well knowing that actual fecundity estimates for these dates might be higher.
Egg dry weight
The egg dry weight (EDW) was determined from formalin-fixed hydrated oocytes (Hempel & Blaxter, 1967). A total of 50 hydrated oocytes per sample were rinsed three times with distilled water and transferred onto aluminum cups. Hydrated oocytes were oven-dried for 72 h at 60°C and the dry weight was taken immediately after drying was finished.
Fertilization and larval length
At each sampling in 2019 four fully mature (stage 6: spawning) females were crossed with four fully mature males. Fertilization of herring took place approximately 2 h post-mortem. Eggs were gently striped onto prepared glass plates in triplicates and activated sperm was poured over the settled eggs. Egg trays were gently moved to ensure proper fertilization. To ensure the highest fertilization rates, sperm activation and fertilization were performed in 16 psu at 10°C (Berg et al., 2019). After 30 min the egg plates were removed from the trays, flushed off with running water, and transferred into prepared flow-through incubation trays. The salinity was set to 35 psu and water temperatures were 8.5 ± 0.15°C during the experiments. Three randomly chosen sections of each plate were photographed 24 h post fertilization. All clearly identifiable fertilized and non-fertilized eggs were counted and subsequently fertilization rates were estimated as following:
where Nf represents the number of fertilized eggs and Nt the total number of eggs on each plate. At least 20 fertilized and unfertilized eggs per cross were measured in ImageJ to calculate the increase in size 24 h after fertilization.
Two days prior to the estimated day of hatching, egg plates were individually transferred into plastic buckets, which were placed in flow-through incubation trays at 8.5°C. Once hatching was completed, the plates were removed from the buckets. Day of hatching was defined when ~ 50 % of the larvae had hatched. Digital pictures of individual larvae were taken 14 days post hatching (dph; n = 20). Larvae were not fed after hatching. The fertilization experiment was reviewed and approved by the FOTS Norwegian Food Safety Authority (ID-8459).
Data analysis
All statistical analysis and visualizations were conducted using the software R (R Core Team, 2014). For all statistical tests, the level of significance was set to α = 0.05.
To infer the genetic structuring of the sampled herring Bayesian clustering analysis was carried out in STRUCTURE v.2.3.4 (Pritchard et al., 2000) using an admixture model and correlated allele frequency without population information. Ten runs with a burn-in of 1,000 and 10,000 Markov chain Monte Carlo (MCMC) iterations were performed for K = 1–4. STRUCTURE output was analyzed by calculating the ad hoc summary statistic ΔK and mean LnP(K) (Evanno et al., 2005), in addition, four alternative statistics (MedMed, MedMean, MaxMed, and MaxMean) were estimated using StructureSelector (Li and Liu, 2018). All suggest that the most likely number of genetic clusters is two. These two clusters could be as expected identified as spring vs. autumn spawning herring based on the three reference samples. Finally, the ten iterations of K=2 were averaged with CLUMPP v.1.1.1 (Jakobsson and Rosenberg, 2007) using the FullSearch algorithm and the G’ pairwise matrix similarity statistic and graphically displayed using bar plots.
Parametric and non-parametric tests were used during analysis. When assessing the assumptions for parametric statistical test, normality was inspected graphically and numerically by performing the Shapiro-Wilk test. Homogeneity of variances was inspected graphically and tested using Levene’s test. Independence of errors in regression analysis was tested using the Durbin-Watson test. In case the assumptions were accepted, a t-test was performed to test for differences between two groups, while analysis of variance (ANOVA) was used to test for differences in biological characteristics among several groups (e.g., sampling dates). In case of a significant test outcome, post-hoc testing was performed using Tukey HSD. The relationship among a continuous predictor variable and continuous response variable was modelled by linear regression. When plotting the results of a linear regression, only significant regression lines are shown. Analysis of covariance (ANCOVA) was performed to test for differences in the slope and intercept between linear regression models. In case of multiple regression analysis, all potential predictors were incorporated in the starting model and subsequently non-significant variables were removed from the model (backward selection). Due to collinearity of the predicting variables total weight and total length in the regression model for potential fecundity, only total length was kept in the model. When the assumptions for parametric tests were rejected, non-parametric alternatives such as Wilcoxon-Mann-Whitney-U-test and Kruskal-Wallis-test were used. Variances in oocyte diameter as well as egg dry weight were compared among Askøy samples and the two reference samples using an F-test, which tests the null hypothesis of equal variances.
To evaluate sub structuring among the Askøy samples a cluster analysis was conducted. For the cluster analysis we only considered genetically identified spring spawners. Prior to k-means clustering, extensive data exploration with the aim of finding the most likely number of clusters underlying the analyzed data were conducted. Predictor variables were chosen based on biological meaningfulness and on cluster compactness (within sums of squares/total sums of squares), comparing different predictors. The final cluster analysis was done with total length, KS and hydrated oocyte diameter as predictor variables. None of these traits were correlated with each other and could therefore be used without any further transformation or allometric correction in the cluster analysis. Since the number of clusters in the data where a priori not known, we conducted agglomerative hierarchical clustering to infer structuring among the analyzed fish (Figure S5). In addition we used the majority rule implemented in the NbClust package (Charrad et al., 2014) and the partial R² computed for a cluster number of 1 to 15 clusters to find the suitable number of clusters present in the data. K-means cluster analysis was validated through linear discriminant analysis which revealed an overall model accuracy of 97%.
Results
Genetic assignment of spring and autumn spawners
421 herring were successfully genotyped and classified as either genetic spring (N = 382) or autumn (N = 39) spawner (Figure 1A). Most fish were classified as genetic spring spawners (~ 90%) which were significantly larger (t-test, p < 0.001; Figure 1B) than autumn spawners but had a reduced somatic condition (t-test, p < 0.01; Figure 1C). Maturity proportions were not different among genetic spring and autumn spawners and were dominated by actively spawning fish in stage 6 (Figure S6). Genetic autumn spawners had mainly the largest oocytes among the sampled fish, both within vitellogenic as well as hydrated oocytes (Figure 1D). However, few autumn spawners had a relatively large total length, but small hydrated oocyte diameter near the maximum diameter observed for NSASH (Figure 1D, Table 2).
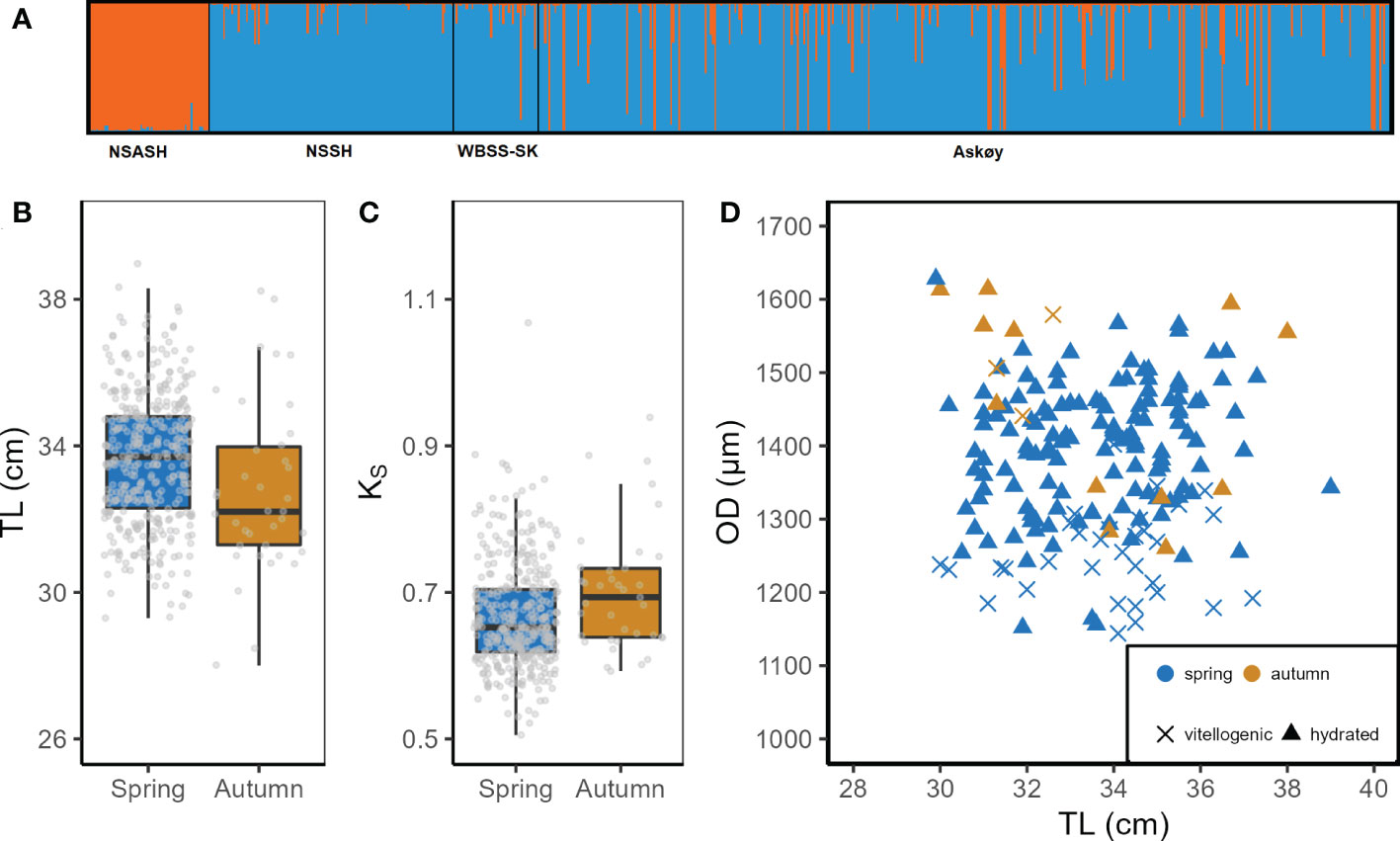
Figure 1 (A) STRUCUTRE plot results for K=2 presenting autumn spawning herring in orange and spring spawning herring in blue. North Sea autumn spawning herring, Norwegian spring spawning herring and Western Baltic spring spawning herring were used as reference populations in the analysis. (B) Total length (TL) and (C) somatic condition factor (KS) in relation to genetic assignment, and (D) oocyte diameter (OD) in relation to TL. Genetic spawning season as well as oocyte stage are represented in different color and symbols, respectively.
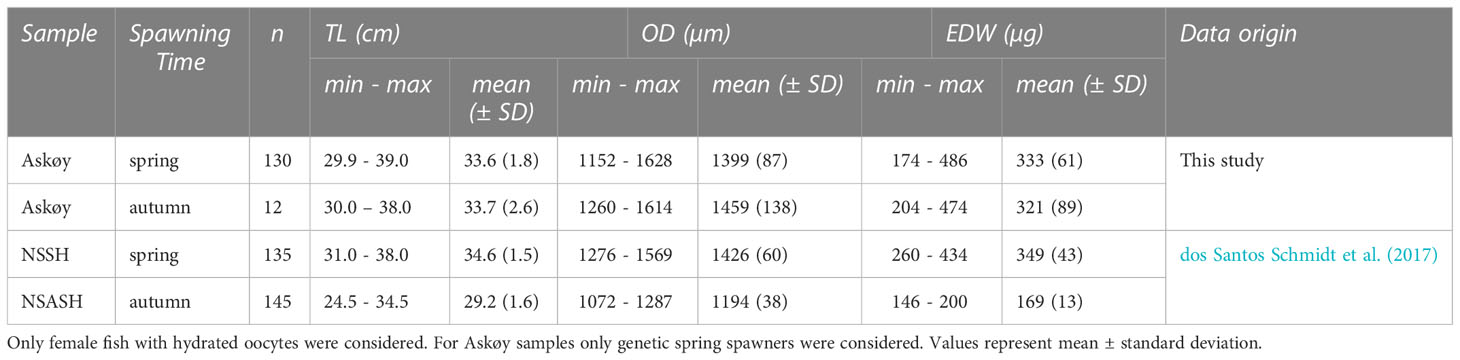
Table 2 Morphometric data including total length (TL) and life-history data including oocyte diameter (OD) and egg dry weight (EDW) of herring collected on the coastal spawning ground Askøy and two reference populations for comparison, namely Norwegian spring spawning herring (NSSH), and North Sea autumn spawning herring (NSASH).
Reproductive traits along spring autumn spawner continuum
In the following results we refer only to genetic spring spawners from Askøy therefore genetic autumn spawners were subsequently removed from any further analyses. This was done because genetic autumn spawners are an additional source of variance in e.g. hydrated oocyte diameter (Figure 1D) and could thereby confound results and interpretations on the variability of traits of genetic spring spawners. Hydrated oocyte diameter varied in size from 1152 µm to 1628 µm (Table 2) and ranged by a total factor of 1.4 among Askøy samples. No temporal trends were detected across sampling dates (ANOVA; p = 0.18; Figure S7). Neither maternal length (ANOVA, p = 0.41, Figure S7) nor somatic weight (ANOVA, p = 0.44) explained the variation in hydrated oocyte diameter, but there was a slight but significant effect of somatic condition (ANOVA, R2 = 0.04, p = 0.009). Hydrated oocyte diameter was found to be similar for Askøy samples and NSSH, while NSASH had smaller oocytes (Kruskal-Wallis; p < 0.001; Table 2). However, variance of hydrated oocyte diameter was significantly larger in Askøy samples than in NSSH (F-test; F = 2.52, p < 0.001) as well as NSASH (F = 5.45, p <0.001) and exceeded those observed in other herring populations (Figure S8) but was similar to those populations where potential mixing with migratory herring (NSSH) occurs. The largest variability in hydrated oocyte diameter was found in length classes from 30 – 34 cm in Askøy samples (Figure 2A). Some individuals from Askøy had hydrated oocyte diameters similar to NSASH, while many fish had similar or larger hydrated oocyte diameters than NSSH (Figure 2A). Egg dry weight ranged from 174 µg to 486 µg and the mean egg dry weight of Askøy samples was close to NSSH, but much lower in NSASH (Kruskal-Wallis; p < 0.001; Table 3). Variance in egg dry weight was larger in Askøy than in NSSH (F = 2.49, p = 0.005) and NSASH (F = 18.25, p < 0.001), respectively (Figure 2B). Askøy and NSSH showed the same linear relationship between egg dry weight and hydrated oocyte diameter (ANCOVA; slope & intercept p > 0.05; Figure 2B). NSASH showed narrow egg dry weight variation, and a more stable relationship with oocyte diameter, therefore it was not included in the regression (Figure 2B).
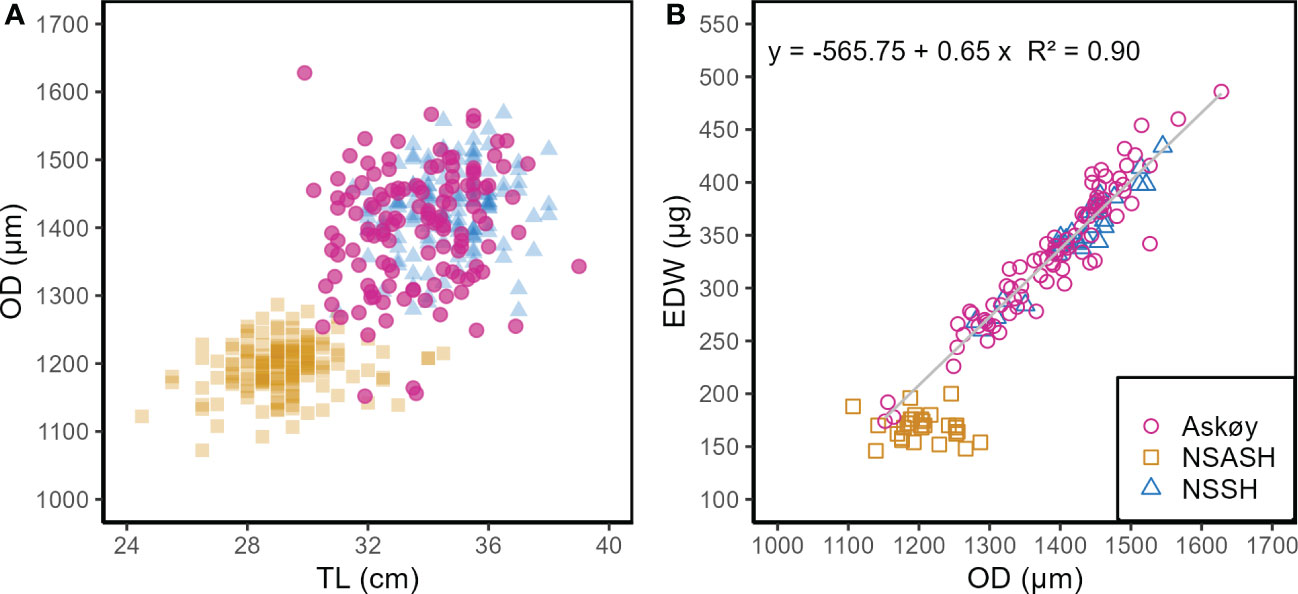
Figure 2 (A) Oocyte diameter (OD) in relation to total length (TL) and (B) egg dry weight (EDW) in relation to oocyte diameter for Askøy samples, Norwegian spring spawning herring (NSSH), and North Sea autumn spawning herring (NSASH). Regression line and formula refers to a common regression of Askøy and NSSH data. Within Askøy samples only hydrated oocytes of genetic spring spawners were included.
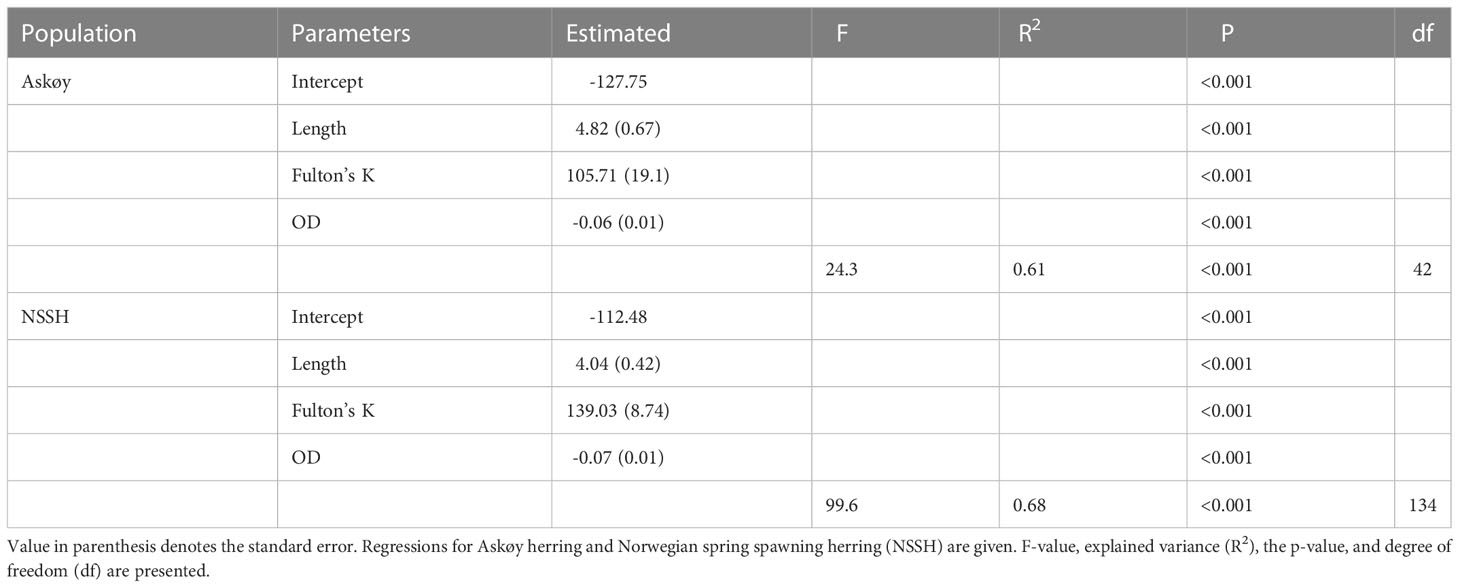
Table 3 Multiple regression output for potential fecundity (FP) as a function of length, Fulton’s K and oocyte diameter (OD).
Reproductive traits within spring spawners
Potential fecundity (FP; Figure 3A) and relative somatic fecundity (RFP,S; Figure 3B) was higher in Askøy samples than in NSSH (Mann–Whitney U test, p < 0.001 respectively). FP increased with total length for both groups (Figure 3A). RFP,S seemed to lack relationship with total length in both groups (Figure 3B). A multiple linear regression model with total length, Fulton’s K and oocyte diameter as predictor variables was able to explain 61% and 68% of the observed variability in FP for Askøy samples (ANOVA; p < 0.001) and for NSSH samples (ANOVA; p < 0.001), respectively (Table 3). Herring on the coastal spawning ground Askøy were significantly smaller than NSSH from their main spawning ground in both sampling years 2018 and 2019 (Mann–Whitney U test p < 0.001; Figure 4).
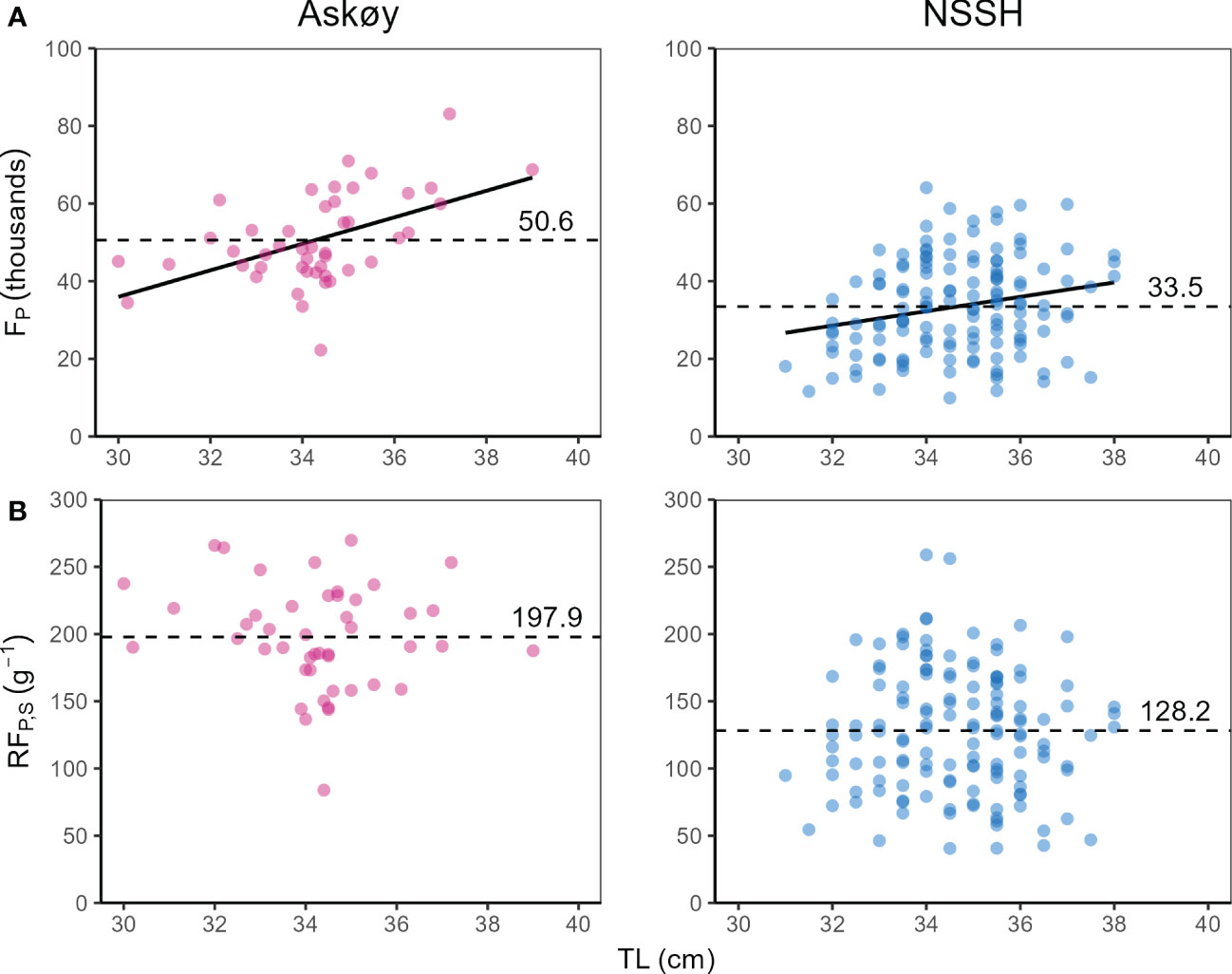
Figure 3 (A) Potential fecundity (FP) and (B) relative somatic fecundity (RFP,S) in relation to total length (TL) for Askøy samples (genetic spring spawners collected in February 2019) and Norwegian spring spawning herring (NSSH). Solid line represents significant regression line, while dotted line represents the grand mean.
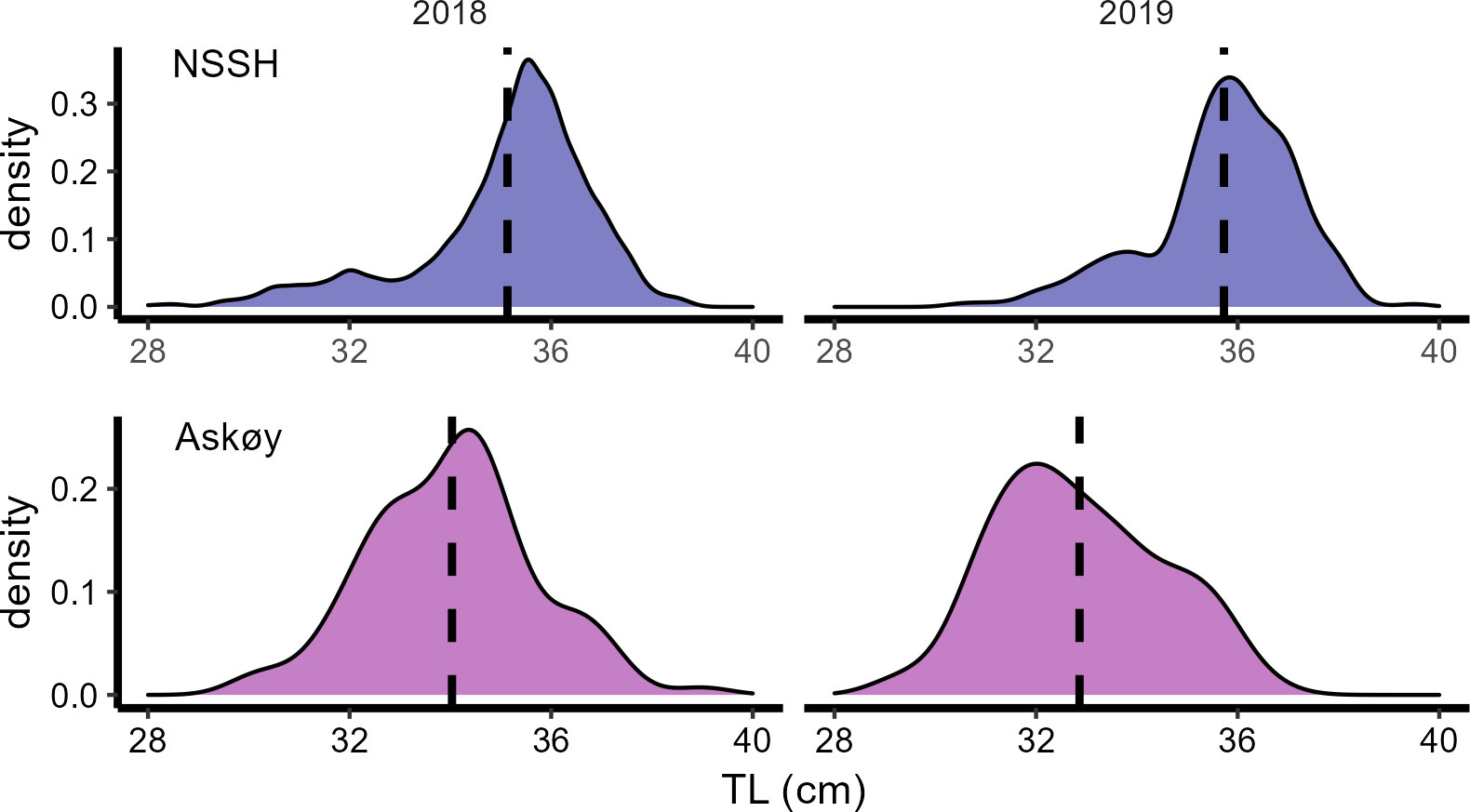
Figure 4 Total length (TL) frequency distributions among Askøy and Norwegian spring spawning herring (NSSH). Horizontal dashed line indicates the mean value. NSSH were sampled near their main spawning ground in 2018 and 2019. For Askøy only genetic spring spawners caught in March 2018 and February 2019 are shown.
Hierarchical cluster analysis indicated structuring into two clusters among the genetic spring spawners within Askøy samples (Figure S5). Furthermore, the majority rule (NbClust) proposed 2 as the most likely number of clusters underlying the analyzed data with total length, KS and hydrated oocyte diameter as predictor variables. The adjusted R2 was 0.30, hence the overall structuring of the data cannot be considered strong. Subsequent k-means clustering (k = 2) resulted in two clusters where the first cluster was characterized by generally smaller fish with smaller hydrated oocytes (Figure 5; Table 4). Fish in the second cluster were generally larger and had the largest hydrated oocytes in their length class (Figure 5; Table 4). However, fish in cluster 2 had a lower somatic condition compared to cluster 1 (Table 4). The variability in reproductive traits has been reduced, at least for cluster 2, which is now at similar levels compared with other populations along the Norwegian coast (Figure S8).
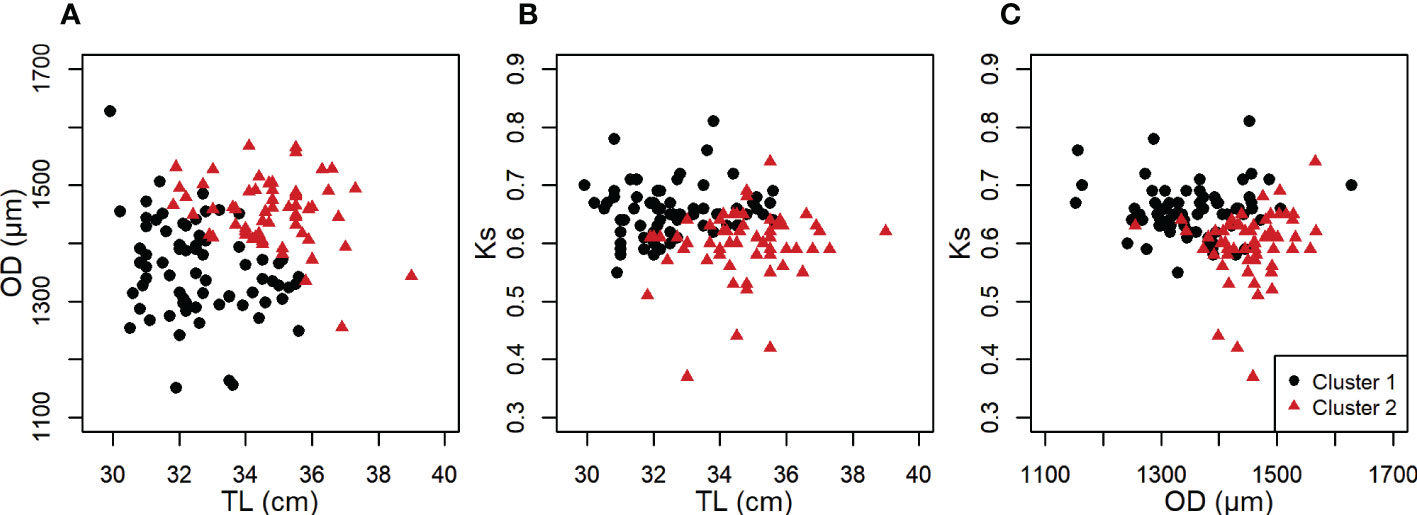
Figure 5 (A) Oocyte diameter (OD) as a function of total length, (B) somatic condition (Ks) as a function of total length and (C) somatic condition as a function of oocyte diameter for the two identified clusters within genetic spring spawners based on k-means cluster analysis using female fish in hydration stage (n = 130).

Table 4 Summary of morphometric (total length) and life-history traits [somatic weight (Ws), somatic condition (Ks) and oocyte diameter (OD)] per cluster for spring spawning female fish in hydration stage.
Cross fertilizations and hatching larvae
Fertilization success varied over the spawning season as well within a given date (Figure 6A). Fertilization rates were higher for all crosses in February, slightly lower in March and the lowest in April. Individual variability in fertilization success increased over the spawning season (Figure 6A). Overall, fertilized eggs were larger than unfertilized eggs (t-test; p < 0.001). The increase in size from unfertilized to fertilized eggs varied over time (Figure 6B), being lower in April compared to the previous dates (Kruskal-Wallis; p = 0.02).
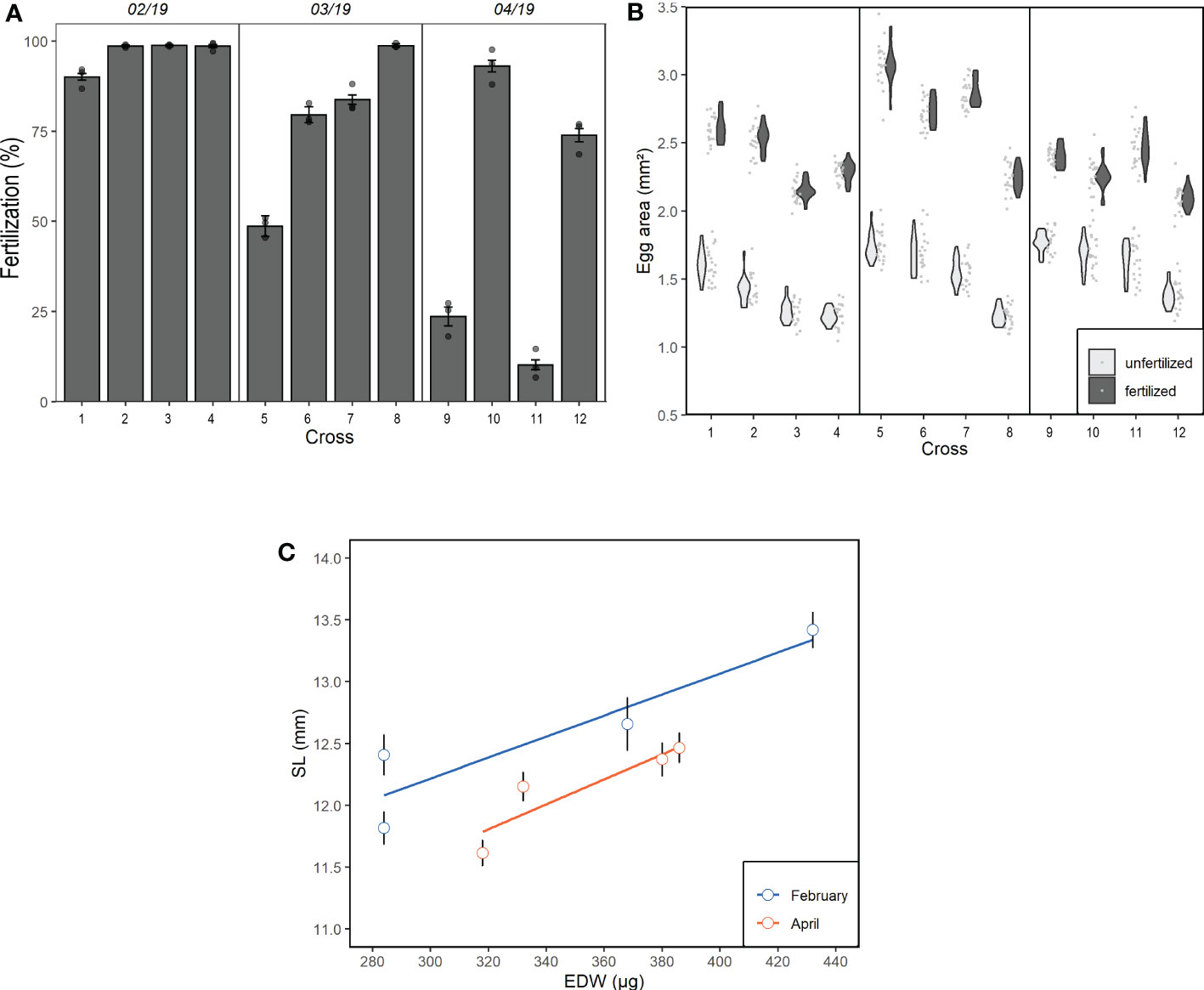
Figure 6 (A) Fertilization rates and (B) egg size (both fertilized and unfertilized) for individual crossings conducted during three experiments in February (1-4), March (5-8) and April (9-12) 2019. (C) Larval standard length (SL) as a function of the egg dry weight (EDW) 14 days post hatching for February and April. Values present mean ± standard deviation.
Egg dry weight and larval length were positively related at both dates (R2 = 0.675; ANOVA; p < 0.001; Figure 6C). Larvae hatching from the experiment in February were on average longer at 14 dph compared to those from April indicated by a significant difference in the intercept (ANCOVA; p < 0.001, Figure 6C). The parental fish from the two experiments showed no difference in length (t-test; p = 0.69).
Discussion
In this study we investigated reproductive traits of Atlantic herring collected on the coastal spawning ground Askøy in western Norway during the spring spawning season to evaluate potential co-occurrence of herring with different reproductive strategies. Based on genetic analysis we identified mainly spring spawning herring, but also some autumn spawning herring which further differed in their reproductive traits. We conducted a multivariate cluster analysis on the genetic spring spawners to identify groups with potentially different reproductive strategies because their variability in both hydrated oocyte diameter and egg dry weight exceeded those observed within other populations of Atlantic herring. The identified clusters indicate two groups with different reproductive investment. Those with lower investment had generally larger oocytes but lower body condition and fecundity, while it is the opposite for herring with high investment. This difference in reproductive investment can be typically linked to different migratory behavior: low reproductive investment = migratory vs. high investment = stationary. Furthermore, highly variable results from cross-fertilization experiments as well as the hatching larvae within a single date and between dates strengthen our interpretation that spring spawners are represented by groups with different reproductive strategies. Also, comparisons to other herring populations occurring along the Norwegian coastline suggest that the high variability within spring spawners can be linked to potential mixing of groups with different reproductive strategies.
Our genetic analysis revealed the co-occurrence of both genetic spring spawners and autumn spawners on the spawning ground. We confirm the potential mixing of herring with opposite spawning seasons, as most spring and autumn spawners were caught in spawning condition (stage 6). It has been previously demonstrated that crossbred individuals between genetic spring and autumn spawners exist on this spawning ground (Berg et al., 2021), but the low number of genetic autumn spawners spawning during spring seems not sufficient to erode genetic differences among genetic spring and autumn spawners. The overall smaller size and higher somatic condition of autumn spawners (see Figure 1C) compared to spring spawners indicates a stationary life-style of these fish, in line with Berg et al. (2021) who found autumn spawners on the same spawning ground throughout the year. The reproductive traits of genetic autumn spawners differed from the North Sea autumn spawning herring (NSASH) used as reference population. In contrast to NSASH, ripe genetic autumn spawners from the coastal spawning ground showed exceptionally large oocytes exceeding those of spring spawners. This seems contradictory; however, one must keep in mind that these fish ended up spawning in the opposite season. These individuals could, however, be recruit spawners which delayed spawning time resulting in an extended oocyte growth period and finally larger eggs at spawning. Delayed spawning time of recruit spawners is a phenomenon that has been already described for Baltic cod (Wieland et al., 2000). We also noticed a higher prevalence of atresia in ripe genetic autumn spawning females (25% of the females showed signs of atresia within autumn spawners and 5% within spring spawners). Since we find overall a very good agreement between hydrated oocyte diameter and egg dry weight and have removed any atretic oocytes during the analysis of oocyte diameter, increased oocyte diameter in those females is not driven by the prevalence of atresia. However, this finding indicates that physiological constrains with respect to delayed or switched spawning could exist, which may have severe consequences under accelerating global change.
We found 10-times higher abundance of spring spawners than autumn spawners on the spawning ground during spring, while during autumn less spring spawners tend to be found (Berg et al., 2021). Many spring spawners hence migrate to the spawning ground during spring. Within spring spawning herring populations, stationary populations show in general a smaller size and slower growth compared to migratory herring populations (Silva et al., 2013; dos Santos Schmidt et al., 2021). This life-history pattern is especially relevant in a situation where migration allows for the exploitation of high food abundance, while stationary lifestyles are restricted to local resources. Migratory NSSH are the dominating population found in the Norwegian Sea (Husebø et al., 2005) and their fast growth as well as larger size compared to stationary populations seems to be linked to their extensive feeding migrations (Dragesund et al., 1980). Spawning migrations of NSSH are state dependent with larger fish migrating further south, where conditions for offspring survival are likely to be more favorable (Slotte, 1999). Thus, we expect the largest fish among the NSSH to reach the spawning grounds around the study area. However, many herring on the coastal spawning ground Askøy were smaller compared to NSSH collected on their main spawning ground, which was consistent across years. Hence not all fish from the coastal spawning ground seem to display this migratory behavior. The co-occurrence of herring with divergent life-history traits has also been described for the semi-enclosed basin Lindåspollene, an area geographically close to Askøy (Johannessen et al., 2014), where NSSH could be distinguished from a stationary herring component based on a different growth pattern and vertebrae count.
Variability in reproductive traits such as oocyte diameter and egg dry weight within a population is to be expected and at least partly explained by maternal factors (Blaxter and Hempel, 1963; Óskarsson et al., 2019). Despite the large variability observed in oocyte diameter and egg dry weight of Askøy samples in our study (EDW varied by a factor of 2.8), we did not find any relationship with maternal size. However, the variability in these traits might be explained by other maternal effects such as condition. Several studies question maternal effects on oocyte diameter when oocytes of similar developmental stage are being compared (Óskarsson et al., 2002; Kennedy et al., 2011). Therefore, other factors likely drive the observed variability in these traits. Spawning time explains in part the variation in oocyte diameter among herring populations (Hempel and Blaxter, 1967; dos Santos Schmidt et al., 2017) which is seen as an adaptation to the prevailing environmental conditions at the time of hatching (Cushing, 1990). Within spring spawning herring populations, stationary populations produce smaller oocytes compared to migratory oceanic populations (dos Santos Schmidt et al., 2021). The cluster analysis indicated structuring into two groups among the genetic spring spawners from Askøy, potentially representing different reproductive investment. Fish of smaller size with smaller oocytes identified as a separate group seem to invest more energy into reproduction which is often the case for stationary herring, being in line with findings from other herring populations (dos Santos Schmidt et al., 2021). In fact, we found three fish with exceptionally small oocytes in March 2019 (OD 1199 – 1216 µm), which had a similar size to those previously identified as originating from stationary spring spawners (Lamichhaney et al., 2017; Berg et al., 2019); Figure S9). The other cluster which was characterized by large oocytes of fish with generally larger size seems to represent herring with low reproductive investment, most likely migratory NSSH or nonstationary coastal herring. Reduced condition in this group resulted from long-distance migrations to the spawning ground (Slotte, 1999).
Besides considering the size of the oocytes, the number of potentially spawned oocytes (potential fecundity) is an important measure for understanding the reproductive strategies and success of a population (Kjesbu, 2009). Life-history theory predicts that the available energetic resources are allocated between metabolic activities and reproduction (Stearns, 1989; Christie et al., 2018). Reproductive investment hence differs between populations with a stationary lifestyle from migratory ones. Stationary spring spawning herring populations tend to have higher fecundity compared to oceanic migratory populations (Silva et al., 2013) although this pattern does not seem to apply universally to all herring populations (dos Santos Schmidt et al., 2021). In line with our findings of divergent oocyte diameters within Askøy samples, fecundity estimates indicate the presence of both stationary and migratory herring, as they exceed those of NSSH. Although fecundity shows inter-annual variability within populations (dos Santos Schmidt et al., 2020) the maximum fecundities observed among Askøy samples exceed this range. In general, the variability of reproductive traits of herring caught at the coastal spawning ground is exceptional but comparison with findings from other studies support our interpretation of two spring spawning groups with different reproductive strategies. Furthermore, the variability of the identified clusters had been reduced, especially for cluster 2 which is now at levels of known populations (Figure S8). For those populations with similar high variability (Figure S8), it has been shown that they mix with migratory NSSH during spawning or at least parts of the local population display some sort of migratory behavior (Johannessen et al., 2009; Sørensen, 2012; Eggers et al., 2014; Eggers et al., 2015).
While fecundity and oocyte diameter can only theoretically describe the reproductive success of spawning herring, cross fertilizations under realistic conditions provide further important insights. The fertilization experiments conducted at the three sampling dates revealed a trend of decreasing fertilization success as the spawning season proceeded. It is important to highlight that individual fertilization success became more variable over the course of time, in some crosses showing a very low fertilization rate while in others, the fertilization was fully successful at the end of the spawning season. This may be linked to group specific optimal spawning times driven by different foraging strategies/migration behaviors of the parental fish. A modelling study on Pacific herring indicated that variability in spawning time might be a result from different parental feeding grounds and their condition (Ljungström et al., 2018), which in turn can effect paternal influences on the fertilization success (Ottesen and Babiak, 2007; Berg et al., 2019) such as a reduction in sperm quality (Evans et al., 2017). This idea seems to be further supported by the differences in size found in the hatching larvae. Larvae hatched from the fertilization experiment in February were significantly longer than larvae from April, which may be an adaptation towards the experienced environmental conditions at the time of hatching and first feeding (Cushing, 1990). Differences in larval length are thought to be driven by qualitative egg traits (e.g. amino acid content), which can explain the difference in hydration and osmotic swelling (Kristoffersen and Finn, 2008) between eggs fertilized in February to those in April. Temperature (Viader-Guerrero et al., 2021) and salinity may be additional factors influencing osmotic swelling and the development of the hatching larvae, but since the experiments were conducted under controlled environmental conditions these did not differ between the experiments. Ultimately two different foraging areas of the parental fish could have led to the observed differences in qualitative egg traits
The complexity observed in the present study is representative for many locations along the Norwegian coast where herring with different reproductive strategies as well as migration behaviors are thought to co-occur during spawning. The diversity of reproductive strategies uncovered herein reflects a valuable resource. Preserving this diversity is considered key to promote population resilience to future stressors as well as human exploitation (Harma et al., 2012). This will also be essential for sustainable harvesting and management of Atlantic herring. Currently herring local and stationary populations are neglected by managers and included in the quota provided for the large oceanic stocks. Thus, smaller local populations could be depleted by a few catches of industry trawlers. Having the opportunity to identify areas with the occurrence of stationary populations will help us to protect them. Furthermore, the identification of genetically spring and autumn spawners is of high value for the management of herring stocks in the North Sea where these have to be separated for the assessment (Bekkevold et al., 2023). However, future studies such as tagging experiments are needed to resolve this complexity in more detail. Even though our findings in variability among reproductive traits in combination with previous studies support our hypothesis that herring with different reproductive strategies are present on the spawning ground (besides autumn and spring spawners), future studies are needed to validate our findings.
Conclusion
Our study has revealed co-occurrence of herring with different reproductive strategies on a coastal spawning ground in western Norway. Analysis of reproductive traits suggests the presence of both ripe genetic autumn and spring spawners during the spring spawning season. Furthermore, spring spawners showed potential differences in reproductive investment which can be linked to migratory and stationary behavior of herring. Future studies employing an extensive set of genetic markers or utilizing whole genome sequencing techniques should address the potential for population differentiation in the herein identified groups in more detail. Tracking studies employing acoustic telemetry will finally be needed to pinpoint the migration behavior of individual herring in the area.
Data availability statement
The data presented in the study are deposited at the Norwegian Marine Data Centre (NMDC), https://doi.org/10.21335/NMDC-827566645.
Ethics statement
The animal study was reviewed and approved by FOTS Norwegian Food Safety Authority (ID-8459).
Author contributions
FB conceptualized the study. FB and JM designed and conducted the sampling. JM and TCdSS performed the oocyte laboratory analysis. JM performed the statistical analysis on reproductive traits, supervised by FB, TCdSS, JG, and CC. GS performed the genetic laboratory analysis. GS and FB conducted the statistical genetic analysis. JM wrote the first draft of the manuscript. All authors contributed to manuscript revision, read, and approved the submitted version.
Funding
This work was funded by the Research Council Norway 254774 (GENSINC).
Acknowledgments
We are grateful to Christel Krossøy, Frank Midtøy, Heikki Savolainen, and Julie Skadal from the UiB for their help with sampling the biological material. We thank Arild Folkvord for project administration and his suggestions on the manuscript.
Conflict of interest
The authors declare that the research was conducted in the absence of any commercial or financial relationships that could be construed as a potential conflict of interest.
Publisher’s note
All claims expressed in this article are solely those of the authors and do not necessarily represent those of their affiliated organizations, or those of the publisher, the editors and the reviewers. Any product that may be evaluated in this article, or claim that may be made by its manufacturer, is not guaranteed or endorsed by the publisher.
Supplementary material
The Supplementary Material for this article can be found online at: https://www.frontiersin.org/articles/10.3389/fmars.2023.978694/full#supplementary-material
References
Aasen O. (1952). The lusterfjord herring and its environment. Fiskeridirektoratets Skrifter Serie Havundersøkelser 10 (2), 1–67.
Armstrong M. J., Witthames P. R. (2012). Developments in understanding of fecundity of fish stocks in relation to egg production methods for estimating spawning stock biomass. Fisheries Res. 117-118, 35–47. doi: 10.1016/j.fishres.2010.12.028
Balazik M. T., Farrae D. J., Darden T. L., Garman G. C. (2017). Genetic differentiation of spring-spawning and fall-spawning male Atlantic sturgeon in the James river, Virginia. PloS One 12 (7), e0179661. doi: 10.1371/journal.pone.0179661
Bekkevold D., Berg F., Polte P., Bartolino V., Ojaveer H., Mosegaard H., et al. (2023). Mixed-stock analysis of Atlantic herring (Clupea harengus): a tool for identifying management units and complex migration dynamics. ICES J. Mar. Sci., 1–12. doi: 10.1093/icesjms/fsac223
Bekkevold D., Gross R., Arula T., Helyar S. J., Ojaveer H. (2016). Outlier loci detect intraspecific biodiversity amongst spring and autumn spawning herring across local scales. PloS One 11 (4), e0148499. doi: 10.1371/journal.pone.0148499
Berg F., Østgaard H. D., Slotte A., Andersson L., Folkvord A. (2021). A combination of genetic and phenotypic characterization of spring- and autumn-spawning herring suggests gene flow between populations. ICES J. Mar. Sci. 78 (2), 694–703. doi: 10.1093/icesjms/fsaa046
Berg F., Slotte A., Andersson L., Folkvord A. (2019). Genetic origin and salinity history influence the reproductive success of Atlantic herring. Mar. Ecol. Prog. Ser. 617-618, 81–94. doi: 10.3354/meps12680
Blaxter J. H. S., Hempel G. (1963). The influence of egg size on herring larvae (Clupea harengus L.). ICES J. Mar. Sci. 28 (2), 211–240. doi: 10.1093/icesjms/28.2.211
Brophy D., Danilowicz B. S., King P. A. (2006). Spawning season fidelity in sympatric populations of Atlantic herring (Clupea harengus). Can. J. Fisheries Aquat. Sci. 63 (3), 607–616. doi: 10.1139/f05-235
Cadrin S., Kerr L., Mariani S. (2013). Stock identification methods: Applications in fishery science, 2nd ed (Cadrin: Academic Press).
Charrad M., Ghazzali N., Boiteau V., Niknafs A. (2014). NbClust: An r package for determining the relevant number of clusters in a data set. J. Stat. Software 61 (6), 36. doi: 10.18637/jss.v061.i06
Christie M. R., McNickle G. G., French R. A., Blouin M. S. (2018). Life history variation is maintained by fitness trade-offs and negative frequency-dependent selection. Proc. Natl. Acad. Sci. 115 (17), 4441–4446. doi: 10.1073/pnas.1801779115
Ciannelli L., Fisher J. A. D., Skern-Mauritzen M., Hunsicker M. E., Hidalgo M., Frank K. T., et al. (2013). Theory, consequences and evidence of eroding population spatial structure in harvested marine fishes: A review. Mar. Ecol. Prog. Ser. 480, 227–243. doi: 10.3354/meps10067
Corriero A., Zupa R., Mylonas C. C., Passantino L. (2021). Atresia of ovarian follicles in fishes, and implications and uses in aquaculture and fisheries. J. Fish Dis. 44 (9), 1271–1291. doi: 10.1111/jfd.13469
Cushing D. H. (1990). Plankton production and year-class strength in fish populations: An update of the match/mismatch hypothesis. Adv. Mar. Biol. 26, 249–293. doi: 10.1016/S0065-2881(08)60202-3
Damme C., Dickey-Collas M., Rijnsdorp A. D., Kjesbu O. S. (2009). Fecundity, atresia, and spawning strategies of Atlantic herring (Clupea harengus). Can. J. Fisheries Aquat. Sci. 66 (12), 2130–2141. doi: 10.1139/F09-153
dos Santos Schmidt T. C., Devine J. A., Slotte A., Claireaux M., Johannessen A., Enberg K., et al. (2020). Environmental stressors may cause unpredicted, notably lagged life-history responses in adults of the planktivorous Atlantic herring. Prog. Oceanography 181, 102257. doi: 10.1016/j.pocean.2019.102257
dos Santos Schmidt T. C., Hay D. E., Sundby S., Devine J. A., Óskarsson G. J., Slotte A., et al. (2021). Adult body growth and reproductive investment vary markedly within and across Atlantic and pacific herring: A meta-analysis and review of 26 stocks. Rev. Fish Biol. Fisheries 31 (3), 685–708. doi: 10.1007/s11160-021-09665-9
dos Santos Schmidt T. C., Slotte A., Kennedy J., Sundby S., Johannessen A., Óskarsson G. J., et al. (2017). Oogenesis and reproductive investment of Atlantic herring are functions of not only present but long-ago environmental influences as well. Proc. Natl. Acad. Sci. 114 (10), 2634–2639. doi: 10.1073/pnas.1700349114
Dragesund O., Hamre J., Ulltang Ø. (1980). Biology and population dynamics of the Norwegian spring-spawning herring. Rapports procès-verbaux Des. réunions / Conseil Permanent Int. pour l'Exploration la Mer 177, 43–71.
Dragesund O., Johannessen A., Ulltang Ø. (1997). Variation in migration and abundance of norwegian spring spawning herring (Clupea harengus L.). Sarsia 82 (2), 97–105. doi: 10.1080/00364827.1997.10413643
Eggers F., Olsen E. M., Moland E., Slotte A. (2015). Individual habitat transitions of Atlantic herring Clupea harengus in a human-modified coastal system. Mar. Ecol. Prog. Ser. 520, 245–256. doi: 10.3354/meps11103
Eggers F., Slotte A., Libungan L. A., Johannessen A., Kvamme C., Moland E., et al. (2014). Seasonal dynamics of Atlantic herring (Clupea harengus L.) populations spawning in the vicinity of marginal habitats. PloS One 9 (11), e111985. doi: 10.1371/journal.pone.0111985
Evanno G., S. R., Goudet J. (2005). Detecting the number of clusters of individuals using the software structure: A simulation study. Mol. Ecol. 14 (8), 2611–2620. doi: 10.1111/j.1365-294X.2005.02553.x
Evans J. P., Lymbery R. A., Wiid K. S., Rahman M. M., Gasparini C. (2017). Sperm as moderators of environmentally induced paternal effects in a livebearing fish. Biol. Lett. 13 (4), 20170087. doi: 10.1098/rsbl.2017.0087
Feng C., Pettersson M., Lamichhaney S., Rubin C.-J., Rafati N., Casini M., et al. (2017). Moderate nucleotide diversity in the Atlantic herring is associated with a low mutation rate. eLife 6, e23907. doi: 10.7554/eLife.23907
Gabriel S., Ziaugra L., Tabbaa D. (2009). SNP genotyping using the sequenom MassARRAY iPLEX platform. Curr. Protoc. Hum. Genet. 60 (1), 2.12.1–2.12.18. doi: 10.1002/0471142905.hg0212s60
Han F., Jamsandekar M., Pettersson M. E., Su L., Fuentes-Pardo A. P., Davis B. W., et al. (2020). Ecological adaptation in Atlantic herring is associated with large shifts in allele frequencies at hundreds of loci. eLife 9, e61076. doi: 10.7554/eLife.61076
Harma C., Brophy D., Minto C., Clarke M. (2012). The rise and fall of autumn-spawning herring (Clupea harengus L.) in the celtic Sea between 1959 and 2009: Temporal trends in spawning component diversity. Fisheries Res. 121-122, 31–42. doi: 10.1016/j.fishres.2012.01.005
Heins D. C., Brown-Peterson N. J. (2022). The reproductive biology of small fishes and the clutch concept: Combining macroscopic and histological approaches. Aquaculture Fish Fisheries 00 (00), 1–12. doi: 10.1002/aff2.49
Hempel G., Blaxter J. H. S. (1967). Egg weight in Atlantic herring (Clupea harengus L.). ICES J. Mar. Sci. 31 (2), 170–195. doi: 10.1093/icesjms/31.2.170
Hughes T. P., Baird A. H., Dinsdale E. A., Moltschaniwskyj N. A., Pratchett M. S., Tanner J. E., et al. (2000). Supply-side ecology works both ways: The link between benthic adults, fecundity, and larval recruits. Ecology 81 (8), 2241–2249. doi: 10.1890/0012-9658(2000)081[2241:SSEWBW]2.0.CO;2
Husebø Å., Slotte A., Clausen L. A. W., Mosegaard H. (2005). Mixing of populations or year class twinning in Norwegian spring spawning herring? Mar. Freshw. Res. 56 (5), 763–772. doi: 10.1071/mf04170
Huse G., Fernö A., Holst J. C. (2010). Establishment of new wintering areas in herring co-occurs with peaks in the ‘first time/repeat spawner’ ratio. Mar. Ecol. Prog. Ser. 409, 189–198. doi: 10.3354/meps08620
Jakobsson M., Rosenberg N. A. (2007). CLUMPP: A cluster matching and permutation program for dealing with label switching and multimodality in analysis of population structure. Bioinformatics 23 (14), 1801–1806. doi: 10.1093/bioinformatics/btm233
Johannessen A., Nøttestad L., Fernö A., Langård L., Skaret G. (2009). Two components of northeast Atlantic herring within the same school during spawning: support for the existence of a metapopulation? ICES J. Mar. Sci. 66 (8), 1740–1748. doi: 10.1093/icesjms/fsp183
Johannessen A., Skaret G., Langard L., Slotte A., Husebo A., Ferno A. (2014). The dynamics of a metapopulation: changes in life-history traits in resident herring that co-occur with oceanic herring during spawning. PloS One 9 (7), e102462. doi: 10.1371/journal.pone.0102462
Kennedy J., Nash R. D. M., Slotte A., Kjesbu O. S. (2011). The role of fecundity regulation and abortive maturation in the reproductive strategy of Norwegian spring-spawning herring (Clupea harengus). Mar. Biol. 158 (6), 1287–1299. doi: 10.1007/s00227-011-1648-0
Kerr Q., Fuentes-Pardo A. P., Kho J., McDermid J. L., Ruzzante D. E. (2019). Temporal stability and assignment power of adaptively divergent genomic regions between herring (Clupea harengus) seasonal spawning aggregations. Ecol. Evol. 9 (1), 500–510. doi: 10.1002/ece3.4768
Kinnison M. T., Unwin M. J., Quinn T. P. (2003). Migratory costs and contemporary evolution of reproductive allocation in male chinook salmon. J. Evolutionary Biol. 16 (6), 1257–1269. doi: 10.1046/j.1420-9101.2003.00631.x
Kjesbu O. S. (2009). “Applied fish reproductive biology: Contribution of individual reproductive potential to recruitment and fisheries management,” in Fish reproductive biology (Kjesbu: Wiley-Blackwell), 293–332.
Kristoffersen B. A., Finn R. N. (2008). Major osmolyte changes during oocyte hydration of a clupeocephalan marine benthophil: Atlantic herring (Clupea harengus). Mar. Biol. 154 (4), 683–692. doi: 10.1007/s00227-008-0961-8
Lamichhaney S., Fuentes-Pardo A. P., Rafati N., Ryman N., McCracken G. R., Bourne C., et al. (2017). Parallel adaptive evolution of geographically distant herring populations on both sides of the north Atlantic ocean. Proc. Natl. Acad. Sci. 114 (17), E3452–E3461. doi: 10.1073/pnas.1617728114
Leggett W. C., Carscadden J. E. (1978). Latitudinal variation in reproductive characteristics of American shad (Alosa sapidissima): Evidence for population specific life history strategies in fish. J. Fisheries Res. Board Canada 35 (11), 1469–1478. doi: 10.1139/f78-230
Li Y.-L., Liu J.-X. (2018). StructureSelector: A web-based software to select and visualize the optimal number of clusters using multiple methods. Mol. Ecol. Resour. 18 (1), 176–177. doi: 10.1111/1755-0998.12719
Ljungström G., Francis T. B., Mangel M., Jørgensen C. (2018). Parent-offspring conflict over reproductive timing: Ecological dynamics far away and at other times may explain spawning variability in pacific herring. ICES J. Mar. Sci. 76 (2), 559–572. doi: 10.1093/icesjms/fsy106
Martinez Barrio A.M., Lamichhaney S., Fan G., Rafati N., Pettersson M., Zhang H., et al. (2016). The genetic basis for ecological adaptation of the Atlantic herring revealed by genome sequencing. eLife 5 (MAY2016), 1–32. doi: 10.7554/eLife.12081
McBride R. S., Somarakis S., Fitzhugh G. R., Albert A., Yaragina N. A., Wuenschel M. J., et al. (2015). Energy acquisition and allocation to egg production in relation to fish reproductive strategies. Fish Fisheries 16 (1), 23–57. doi: 10.1111/faf.12043
Mikkelsen N., Pedersen T., dos Santos Schmidt T. C., Falk-Petersen I.-B., Slotte A. (2018). Are life histories of Norwegian fjord herring populations of pacific ancestry similar to those of Atlantic or pacific herring? J. Mar. Syst. 180, 237–245. doi: 10.1016/j.jmarsys.2016.12.004
Mjanger H., Hestenes K., Oisen E. B. S., Wenneck T. D. L., Aanes S. (2007). Håndbok for prøvetaking av fisk og krepsdyr versjon 3.16 (Bergen: Institute of Marine Research).
Murua H., Saborido-Rey F. (2003). Female reproductive strategies of marine fish species of the north Atlantic. J. Northwest Atlantic Fishery Sci. 33, 23–31. doi: 10.2960/J.v33.a2
Ntiba M. J., Jaccarini V. (1990). Gonad maturation and spawning times of Siganus sutor off the keny a coast: Evidence for definite spawning seasons in atropical fish. J. Fish Biol. 37 (2), 315–325. doi: 10.1111/j.1095-8649.1990.tb05862.x
O’Donnell T. P., Sullivan T. J. (2021). Low-coverage whole-genome sequencing reveals molecular markers for spawning season and sex identification in gulf of Maine Atlantic cod (Gadus morhua, Linnaeus 1758). Ecol. Evol. 11 (15), 10659–10671. doi: 10.1002/ece3.7878
Óskarsson G. J., Kjesbu O. S. S., Slotte A. (2002). Predictions of realised fecundity and spawning time in Norwegian spring-spawning herring (Clupea harengus). J. Sea Res. 48 (1), 59–79. doi: 10.1016/S1385-1101(02)00135-1
Óskarsson G. J., Taggart C. T., Stephenson R. L. (2019). Variation in egg mass within two Atlantic herring Clupea harengus stocks. J. Fish Biol. 95, 367–378. doi: 10.1111/jfb.13956
Ottesen O. H., Babiak I. (2007). Parental effects on fertilization and hatching success and development of Atlantic halibut (Hippoglossus hippoglossus L.) embryos and larvae. Theriogenology 68 (9), 1219–1227. doi: 10.1016/j.theriogenology.2007.08.015
Pritchard J. K., Stephens M., Donnelly P. (2000). Inference of population structure using multilocus genotype data. Genetics 155 (2), 945–959. doi: 10.1093/genetics/155.2.945
Quinn T. P., Unwin M. J., Kinnison M. T. (2000). Evolution of temporal variation in the wild: Genetic divergence in timing of migration and breeding by introduced chinook salmon populations. Evolution 54 (4), 1372–1385. doi: 10.1111/j.0014-3820.2000.tb00569.x
Reiss H., Hoarau G., Dickey-Collas M., Wolff W. J. (2009). Genetic population structure of marine fish: mismatch between biological and fisheries management units. Fish Fisheries 10 (4), 361–395. doi: 10.1111/j.1467-2979.2008.00324.x
Ruzzante D. E., Mariani S., Bekkevold D., Andre C., Mosegaard H., Clausen L. A., et al. (2006). Biocomplexity in a highly migratory pelagic marine fish, Atlantic herring. Proc. R. Soc. B: Biol. Sci. 273 (1593), 1459–1464. doi: 10.1098/rspb.2005.3463
Sørensen Ø.B. (2012). Comparative biology and population dynamics between trondheimsfjord herring and Norwegian spring spawning herring, implications for management. Master's thesis Univ. Bergen Norway. 1–58.
Silva F. F. G., Slotte A., Johannessen A., Kennedy J., Kjesbu O. S. (2013). Strategies for partition between body growth and reproductive investment in migratory and stationary populations of spring-spawning Atlantic herring (Clupea harengus L.). Fisheries Res. 138, 71–79. doi: 10.1016/j.fishres.2012.07.013
Slotte A. (1999). Effects of fish length and condition on spawning migration in Norwegian spring spawning herring (Clupea harengus L.). Sarsia 84 (2), 111–127. doi: 10.1080/00364827.1999.10420439
Smedbol R. K., Stephenson R. (2001). The importance of managing within-species diversity in cod and herring fisheries of the north-western Atlantic. J. Fish Biol. 59 (sa), 109–128. doi: 10.1111/j.1095-8649.2001.tb01382.x
Smith P. J., Francis R. I. C. C., McVeagh M. (1991). Loss of genetic diversity due to fishing pressure. Fisheries Res. 10 (3), 309–316. doi: 10.1016/0165-7836(91)90082-Q
Stearns S. C. (1989). Trade-offs in life-history evolution. Funct. Ecol. 3 (3), 259–268. doi: 10.2307/2389364
Tallman R. F. (1986). Genetic differentiation among seasonally distinct spawning populations of chum salmon, oncorhynchus keta. Aquaculture 57 (1-4), 211–217. doi: 10.1016/0044-8486(86)90199-7
Thorsen A., Kjesbu O. S. (2001). A rapid method for estimation of oocyte size and potential fecundity in Atlantic cod using a computer-aided particle analysis system. J. Sea Res. 46 (3-4), 295–308. doi: 10.1016/S1385-1101(01)00090-9
Varpe Ø., Fiksen Ø., Slotte A. (2005). Meta-ecosystems and biological energy transport from ocean to coast: The ecological importance of herring migration. Oecologia 146 (3), 443. doi: 10.1007/s00442-005-0219-9
Viader-Guerrero M., Guzmán-Villanueva L. T., Spanopoulos-Zarco M., Estrada-Godínez J. A., Maldonado-García D., Gracia-López V., et al. (2021). Effects of temperature on hatching rate and early larval development of longfin yellowtail seriola rivoliana. Aquaculture Rep. 21, 100843. doi: 10.1016/j.aqrep.2021.100843
Via S., Gomulkiewicz R., De Jong G., Scheiner S. M., Schlichting C. D., Van Tienderen P. H. (1995). Adaptive phenotypic plasticity: consensus and controversy. Trends Ecol. Evol. 10 (5), 212–217. doi: 10.1016/S0169-5347(00)89061-8
Keywords: herring, life-history, population structure, fecundity, reproduction, spawning
Citation: Mueller J, dos Santos Schmidt TC, Seljestad GW, Clemmesen C, Gröger JP and Berg F (2023) Analysis of reproductive traits reveals complex population dynamics on a small geographical scale in Atlantic herring. Front. Mar. Sci. 10:978694. doi: 10.3389/fmars.2023.978694
Received: 26 June 2022; Accepted: 20 January 2023;
Published: 01 February 2023.
Edited by:
Jawahar Patil, University of Tasmania, AustraliaReviewed by:
Mbaye Tine, Gaston Berger University, SenegalIoannis A. Giantsis, University of Western Macedonia, Greece
Copyright © 2023 Mueller, dos Santos Schmidt, Seljestad, Clemmesen, Gröger and Berg. This is an open-access article distributed under the terms of the Creative Commons Attribution License (CC BY). The use, distribution or reproduction in other forums is permitted, provided the original author(s) and the copyright owner(s) are credited and that the original publication in this journal is cited, in accordance with accepted academic practice. No use, distribution or reproduction is permitted which does not comply with these terms.
*Correspondence: Jonas Mueller, jonas@domain-mueller.de; Florian Berg, florian.berg@hi.no
†Present address: Jonas Mueller, Institute of Animal Breeding and Husbandry, Department for Marine Aquaculture, Christian-Albrechts University, Kiel, Germany