Short-term and long-term exposure to combined elevated temperature and CO2 leads to differential growth, toxicity, and fatty acid profiles in the harmful dinoflagellate Karlodinium veneficum
- 1College of Earth, Ocean and Environment, School of Marine Science and Policy, University of Delaware, Lewes, DE, United States
- 2Horn Point Laboratory, University of Maryland Center for Environmental Science, Cambridge, MD, United States
- 3Department of Microbiology, The University of Tennessee, Knoxville, TN, United States
- 4Department of Chemistry, Henson School of Science and Technology, Salisbury University, Salisbury, MD, United States
Ocean warming and acidification may significantly alter the distribution and intensity of harmful algal blooms as well as their effects on marine food webs. Estimating such effects rely, in part, on understanding the physiological response of individual algal species to controlled laboratory simulations of climate change conditions. Here we report the physiological response of the harmful dinoflagellate Karlodinium veneficum to the combined effects of elevated temperature and CO2 (29°C/1000 ppm CO2). We first examined these effects by comparing ambient control (25°C/441 ppm CO2) and elevated conditions under short-term (~20 generations) growth. Next, we compared the short-term elevated condition to a longer-term (~200 generations) growth scenario under the same elevated temperature and CO2. Under the short-term elevated conditions, K. veneficum growth declined, cell toxicity increased, and saturated and mono-unsaturated fatty acid (FA) composition varied significantly from ambient conditions. Meanwhile, after ~ 200 generations of growth under elevated temperature and CO2, K. veneficum carbon assimilation, growth, and cell toxicity were significantly higher than the short-term elevated treatment. Further, while total saturated FA declined, essential fatty acids increased and likely represented an adaptive temporal response to long-term exposure to high temperature and CO2. Such shifts in FA profiles and cell toxicity may possibly alter K. veneficum nutritional quality as prey and its mixotrophic behavior, thereby affecting the energy and mass transfer through the marine food webs as well as bloom dynamics.
1 Introduction
With the continued release of greenhouse gases, the upper ocean has warmed by 0.11°C per decade from 1971–2010, and the surface ocean pH has decreased by 0.1 since the beginning of the industrial era (IPCC, 2014, 2021). It is virtually certain that ocean warming and acidification will continue beyond 2100 (IPCC, 2014, 2021). These changes directly and indirectly affect phytoplankton physiology and their role in several marine ecosystems. How climate change could affect phytoplankton that cause harmful algal blooms (HABs) is particularly concerning because of their negative effects on aquatic ecosystems, and their impacts to socio-economics, as well as human health and wellbeing.
Over the past several decades, there is mounting evidence that climate change has increased HAB intensity, frequency, and range (Hallegraeff, 2010; Wells et al., 2015; Gobler et al., 2017; Trainer et al., 2019; Glibert, 2020; Gobler, 2020), and toxic dinoflagellate blooms are often projected to increase under future climate change scenarios (Tatters et al., 2013a; Glibert et al., 2014; Hattenrath-Lehmann et al., 2015; Gobler et al., 2017; Brandenburg et al., 2019; Glibert, 2020). However, dinoflagellate growth and cell toxicity do not always increase in response to ocean warming and/or acidification. For example, elevated CO2 and temperature (750-1000 ppm and +4-5°C), led to increased growth and saxitoxin content in Alexandrium catenella (Tatters et al., 2013a), but did not affect the growth and/or the amount of brevetoxin in Karenia brevis (Errera et al., 2014). In addition to species-specific differences, there may be considerable variation to warming and acidification among individual genotypes within a single species. For example, elevated CO2 and temperature exposure led to strain-specific differences in growth in eight isolates of the dinoflagellate Alexandrium ostenfeldii, while total toxin production remained unchanged in most strains (Kremp et al., 2012). Furthermore, toxicity responses to elevated CO2 and temperature across dinoflagellate taxa may vary due to underlying metabolic pathways (Brandenburg et al., 2019).
Ocean warming and acidification may significantly alter phytoplankton cell biochemistry, and in particular lipid composition. Algal fatty acids, especially omega-3 long chain polyunsaturated fatty acids (n-3 LC-PUFA) such as eicosapentaenoic acid (EPA; 20:5n-3) and docosahexaenoic acid (DHA; 22:6n-3) are germane since they are exclusively synthesized by phytoplankton and are predicted to decline in response to ocean warming (Hixson and Arts, 2016) and acidification (Meyers et al., 2019). Lipid profile adjustments are also taxon-specific (Hixson and Arts, 2016) and do not always change under elevated CO2 (Wang et al., 2017). Mesozooplankton (e.g., copepod) growth and life history, such as fecundity, egg hatching, and naupliar survival, are critically dependent on algal-derived fatty acids, and n-3 fatty acids in particular. In addition, shifts in phytoplankton fatty acid content strongly affect not only organismal fitness, but also trophic energy transfer efficiency among phytoplankton and zooplankton (Jónasdóttir et al., 2009; Cripps et al., 2016; McLaskey et al., 2019; Meyers et al., 2019).
Many experiments designed to test the effects of climate change to phytoplankton are short-term, typically lasting less than 15 algal generations (e.g. Fu et al., 2010; Errera et al., 2014; Hennon et al., 2017; Bercel and Kranz, 2019; Seto et al., 2019). However, some longer experiments have provided nuanced and unique insights into phytoplankton physiological acclimation and/or adaptation to future climate change that deviate from shorter duration experiments (e.g. Collins and Bell, 2004; Flores-Moya et al., 2012; Tatters et al., 2013b). For example, after growing diatoms for 2 years under elevated temperature (30°C), essential fatty acids that significantly declined when shifted from 26°C to 30°C for only seven days partially recovered after the longer growth period (Jin et al., 2020).
Karlodinium veneficum is a common coastal estuarine toxic dinoflagellate with a near-global distribution that produces a suite of toxic compounds called karlotoxins (KmTx) that have cytotoxic, ichthyotoxic, hemolytic, and grazer deterrent properties (Deeds et al., 2002; Adolf et al., 2007; Waggett et al., 2008; Fu et al., 2010; Place et al., 2012). Karlodinium veneficum blooms often cause massive fish kills and are also toxic to oyster embryos, larvae, and juveniles (Adolf et al., 2007; Glibert et al., 2007; Brownlee et al., 2008; Stoecker et al., 2008; Waggett et al., 2008). As a mixotroph, K. veneficum bloom initiation is affected not only by the availability of nutrients but also by prey (Adolf et al., 2008; Li et al., 2015; Lin et al., 2018). Temperature, light intensity, and dissolved inorganic carbon availability also affect growth (Adolf et al., 2007, 2009; Fu et al., 2010). Karlodinium veneficum growth is often greater at an intermediate temperature (e.g., 25°C) than at slightly warmer temperatures (e.g., 28–30°C; Lin et al., 2018; Vidyarathna et al., 2020, 2023), and is also resilient to low pH (7.8–6.0; Nielsen et al., 2010), and increases at high pCO2 (e.g., 745 ppm) (Fu et al., 2010). In addition, high temperature (i.e. ≥ 28°C) or high pCO2 can increase K. veneficum cell toxicity (Fu et al., 2010; Vidyarathna et al., 2020), but these factors were treated in isolation and under short-term exposure. In this regard, it is unclear how the combined effects of elevated temperature and CO2 may affect K. veneficum physiology and long-term acclimation or adaptation.
Here, we tested the physiological response of a mid-Atlantic strain of Karlodinium veneficum to short- and long-term growth under combined warming and acidification. Our central objective was to compare growth, photosynthesis, cellular biochemistry, and toxicity after short-term (~20 generations, 40 days) and long-term exposure (~200 generations, 380 days) to projected future high temperature and CO2 conditions. Long-term treatments resulted in greater growth, toxicity and essential fatty acids than in short-term treatments thereby providing potential implications for both Karlodinium prey and its predators.
2 Materials and methods
2.1 General experimental design
Short-term experiments were conducted at two temperatures and CO2 concentrations: ‘ambient’ conditions were set as 25°C and 400 ppm pCO2, while the ‘treatment’ conditions were designed to simulate warming and acidification conditions projected for the end of the 21st century (baseline RCP scenarios, IPCC, 2014) and set to 29°C and 1000 ppm pCO2. Cultures were acclimated to short-term ambient and treatment conditions for at least 20 generations in steady state before examining the short-term response (abbreviated as ST hereafter) to elevated temperature and CO2. The treatment cultures were then further acclimated for ~200 generations to measure if the long-term (abbreviated as LT hereafter) response to elevated temperature and CO2 differed from the ST response to these same conditions.
2.2 Phytoplankton cultures and acclimation to experimental conditions
Karlodinium veneficum, originally collected from the Delaware Inland Bays, Delaware, USA (CCMP2936; National Center for Marine Algae and Microbiota, Bigelow, USA), was initially grown in replicate (n=4) non-axenic batch cultures in modified f/2 media (320 µM NO3- and 20 µM PO43-; Guillard and Ryther, 1962) at 25°C under a light intensity of 100 µmol photons m-2 s-1 provided by cool white fluorescent bulbs set to a 12:12 hour light:dark cycle. Media seawater was collected from the Indian River Inlet, DE, diluted with laboratory-grade fresh water to a salinity of 20, and the total alkalinity was adjusted to ~2200 µmol kg-1 by adding NaHCO3. This TA adjustment was done to avoid disrupting the carbonate chemistry of the stock cultures and to bring the carbonate chemistry parameters back to the baseline level since the dilution reduced the DIC and TA significantly (~1000 and ~800 umol kg-1 for TA and DIC respectively). Water was then filtered (0.2µm, Whatman Polycap 75 TC) and autoclaved. Algal cultures were slowly acclimated to aeration by bubbling filtered (0.2 µm) air through fine glass frits (Prism Research Glass Inc., USA), and were kept in log-phase growth by weekly inoculations into fresh f/2 media.
Cultures were then transferred to 2L Pyrex bottles (Corning) and maintained in temperature- and pH-controlled cyclostats, containing filter-sterilized media (n=4, 25°C, 400 ppm pCO2) under the same light conditions as described above (see MacIntyre and Cullen (2005) for detailed description of continuous algal culturing). Cultures were sampled every two days to monitor cell growth by cell number and in vivo chlorophyll a (chl a) fluorescence. The media inflow rate was adjusted to match the intrinsic growth rate of acclimated cultures (based on cell number) until cultures reached steady state (~3 weeks) and was held constant through the rest of the experimental period. Steady-state conditions were confirmed when cell density and in vivo chl a fluorescence no longer changed during the acclimation phase and for several days after inflow rate adjustments stopped. Once cultures were fully acclimated to the ambient conditions, they were grown for a further ~18 generations (40 days) in pH-controlled continuous culture.
Treatment batch cultures were initially acclimated to 29°C by ramping the temperature at a rate of 0.5°C day-1 in temperature-controlled incubators (Percival Scientific), and were held for ~6 generations prior to transferring to pH-controlled cyclostats. For elevated temperature conditions, cyclostat bottles were kept in a clear acrylic water jacket connected to a recirculating water bath set to 29°C. The pCO2 in each bottle was then slowly raised to ~1000 ppm at a rate of 100 ppm day-1. Cell growth was regularly monitored after the pCO2 ramping period, and the media inflow rate was adjusted to match the intrinsic growth rate as explained above for ambient cultures. To limit biofouling, cultures were transferred to new sterile bottles every three weeks. Short-term (ST) treatment conditions lasted for ~22 generations (58 days) after which cultures were sampled for physiological analyses. The remaining cultures were further acclimated to treatment conditions for an additional ~180 generations. After ~200 generations at steady state (381 days total), treatment cultures were considered acclimated to long-term (LT) conditions and were sampled again for physiological analyses. All routine samples (i.e., prior to terminating an experiment) for biomass estimation and carbonate chemistry parameter analyses were withdrawn directly from the cultures using a sample tube attached to the culture vessel.
2.3 Carbonate chemistry control and measurement
CO2 levels (~400 and 1000 ppm) were controlled with a pH-stat system (Qubit Systems Inc, Canada), with pH levels set to 8.2 and 7.9 for ambient and treatment conditions respectively. The pH in each culture bottle was continually recorded and logged in computer-controlled software (Logger Pro) that controlled a 3-way solenoid delivering either CO2 (certified 2%, Tech Gas Inc, USA) or CO2-free air passed through a soda lime column. pH electrodes were cleaned and re-calibrated with NBS buffer standards (pH 4.01 and 9.18, Orion™) every 7-14 days. The pH stability and accuracy of the system was confirmed by independent measurements of culture outflow with a laboratory grade pH system (Fisher Scientific A815 Plus pH meter equipped with Fisherbrand™ accumet™ pH sensor).
The total dissolved inorganic carbon (DIC) of each container was analyzed using an in-house custom DIC analyzer (Friedrich et al., 2002). Water samples were acidified, followed by gas stripping and delivery to a non-dispersive infrared gas analyzer (LI-Cor) for total CO2 measurement. Media and sample total alkalinity (TA) were measured using a bromocresol purple colorimetric assay (Yao and Byrne, 1998) with a spectrometer (Ocean Optics, USB4000-ES) and a titrator (Metrohm 876 Dosimat plus, Switzerland). All DIC and TA measurements were compared to certified reference materials (Dickson laboratory, San Diego, USA), and seawater carbonate chemistry was calculated with the CO2SYS software (Pierrot et al., 2006) based on DIC and pH (NBS) (Table 1).
2.4 Cell growth and chlorophyll a
Cell growth was monitored every two days by measuring in vivo chl a fluorescence (10 AU; Turner Designs, USA) and active chl a fluorescence (fast repetition rate fluorometry, Chelsea Instruments, UK), and periodic cell counts confirmed estimates by in vivo fluorescence. Cell density was calculated by counting glutaraldehyde-preserved (final concentration 1% v/v) sub samples (1 mL) with a Neubauer hemocytometer and a light microscope (100x magnification). Chl a concentration was determined according to Welschmeyer (1994), with acetone extracts measured on a fluorometer (10 AU; Turner Designs, USA). Once cultures reached steady-state, cell biomass was monitored several times each week by in vivo chl a fluorescence. Cell specific growth rate (µ, day-1) was calculated according to Brading et al. (2011), using the following formula:
where = time (days), t = time interval between sampling, = in vivo chl a fluorescence, = culture volume in the vessel at the time of sampling, = outflow volume (mL) generated during the time interval between sampling and = the maximum volume of medium (mL) in the vessel.
2.5 Cell photochemistry
Cells were dark acclimated for 15 min and algal photochemistry was monitored by active chl a fluorescence by fast repetition rate fluorometry (FRRf) with a FASTtracka II fluorometer with a FASTact light and temperature control assembly (Chelsea Instruments, UK) connected to a constant temperature water bath set to the growth temperature. Fluorescence induction was recorded by applying a single-turnover fluorescence protocol that consisted of 100 1µs flashlets at ∼1-μs intervals provided by a bank of blue LEDs (peak excitation 450 nm, 200 µs total induction time), followed by 50 flashlets spaced at increasing intervals to allow for PSII reaction center re-oxidation. Measurements were recorded from the average of 15–30 acquisitions at 100 ms intervals. Photochemical activity in the light activated state was then assessed by the same fluorescence induction protocol after exposing cells to white light for 3 min that was provided by a bank of LEDs set to 104 µmol photons m-2 s-1. Fluorescence data were fit in FASTpro software (v3.0, Chelsea Instruments) and used to calculate the maximum quantum yield of photosystem II (Fv/Fm) and the quantum yield of photosystem II in the light activated state (Fq’/Fm’) (Cosgrove and Borowitzka, 2010).
2.6 Photosynthetic carbon assimilation
Subsamples were removed from each replicate culture (5 mL) and combined with 12.5 µL of 14C sodium bicarbonate (specific activity 0.1 µCi mL-1 MP Biomedicals) and incubated in a temperature-controlled aluminum block set to 25°C or 29°C for 30 min. Light in each well was set to 200 µmol photons m-2 s-1 and was provided by a cool white LED under each sample well. Preliminary measurements confirmed that photosynthesis in all samples was saturated at this light intensity (average Ek ~ 160 µmol photons m-2 s-1). Dark controls were run in duplicate. Incubations were stopped by adding 1% glutaraldehyde (final concentration) to each vial, and residual inorganic C was removed by adding hydrochloric acid (1.2 M final concentration), followed by shaking samples overnight. After adding 5 mL scintillation cocktail (Ultima Gold, Perkin Elmer), radioactivity was measured in a liquid scintillation counter (Beckman LS-6500). Carbon assimilation in the light was corrected for any dark incorporation and expressed as the assimilation rate normalized to cell number (cell h-1). As samples were not filtered after 14C assimilation, presented values include both particulate and dissolved production.
2.7 Particulate organic carbon, nitrogen and phosphorus
Samples (25-50 mL) for particulate organic carbon (POC), nitrogen (PON) and phosphorus (POP) were filtered onto 25 mm pre-combusted glass-fiber filters (2h at 450°C, GF/C, Whatman), dried at 60°C for 24 h, and then stored in a desiccator prior to analyses. Cellular C and N were quantified with a CHN elemental analyzer (ECS 4010 Elemental combustion system; Costech Instruments, USA), with phenylalanine and EDTA used as standards. POP was measured following the method of Solórzano and Sharp (1980) using Phosphate standard (RICCA, 1000 ppm PO43-).
2.8 Carbohydrates and soluble proteins
Twenty-five to fifty milliliter samples were centrifuged (4,800 x g) and cell pellets were stored at -20°C until further analyses. Carbohydrate content was determined by the phenol-sulphuric acid method using D-(+)-glucose as a standard (DuBois et al., 1951) after acid hydrolyzing the cell pellets in 1M H2SO4 at 100°C for 1 h. Proteins were extracted by bead beating (BioSpec) cell pellets with 0.5 mm glass beads for 60 s in 500 µL of filtered sea water (FSW, nominal pore 0.2 µm) followed by centrifugation (3,380 x g, 5 min). Soluble protein was determined with a linearized Bradford assay (Ernst and Zor, 2010). All absorbance measurements were recorded using a plate reader (FLUOstar Omega, BMG Labtech).
2.9 Fatty acids
Fifty milliliter samples were centrifuged (4,800 x g) and the pellets were freeze-dried (Virtis, 12SL) and stored in a desiccator. Prior to lipid extraction, samples were spiked with 20 µL of C13:0ME internal standard (10 mg mL-1, Sigma Aldrich, St. Louis, MO). Acid-catalyzed transesterification of lipids to fatty acid methyl esters (FAMEs) and the extraction of FAMEs into hexane was performed as in Van Wychen et al. (2013). FAME extracts were stored at -20°C until analysis. Supelco 37 Component FAME mix (Sigma Aldrich, St. Louis, MO) was spiked with the 5 µL of the same C13:0ME internal standard as above and were used as standards (total fatty acid concentration ranging from 0.01 to 0.5 mg mL-1). FAMEs were analyzed by gas chromatography (GC) on a Hewlett Packard HP 7890B Series (Palo Alto, CA) equipped with a flame ionization detection and a Zebron ZB-1 wax column (30m x 250µm x 25µm, Phenomenex, Torrance, CA). FAMEs were separated using splitless injection (injection volume =3 µL, inlet temperature = 250°C). An initial column heating to 100°C was maintained for 1 min followed by ramping at 25°C min-1 up to 170°C, then 2°C min-1 up to 200°C, which was held for 1 min prior to ramping at 18°C min-1 up to 250°C, which was held for 8 min. FAMEs were identified based on their retention times, and peaks were compared to the standard curve in Open LAB CDS GC software (Agilent Technologies). Fatty acid recovery was corrected for the recovery of the internal standard, and FAME concentration normalized to cell number was then converted to the corresponding fatty acid concentration using individual Sheppard factors (Sheppard, 1992).
2.10 Cellular toxicity
Fifty milliliter samples were centrifuged (4,800 x g) at 4°C (Sorval RC-5B, USA), and cell pellets were extracted in 1–2 mL of methanol for 24 h in the dark at -20°C. Cell toxicity was assessed by the hemolytic assay according to Eschbach et al. (2001) and with a Rainbow Trout (RT) fish gill cell (FGC) assay according to Dayeh et al. (2005) with modifications (Vidyarathna et al., 2020) and was normalized to total cellular carbon.
2.11 Data analyses
Statistical analyses were performed using R (v 4.1.1; R Core Team 2021). The data were checked for normality and homogeneity of the variance by Shapiro-Wilk and Bartlett tests respectively. Treatment effects (increased temperature and CO2) were tested between ambient and ST cultures and the effect of acclimation time to increased temperature and CO2 (short term and long term) between ST and LT cultures were analyzed separately using one-way analysis of variance (ANOVA). Results were considered significant at α = 0.05.
3 Results
3.1 Cell growth, carbon assimilation, photophysiology and chlorophyll a
The ambient cell growth rate was 0.32 ± 0.02 day-1, while the treatment growth significantly decreased in the short-term (ST) cultures to 0.27 ± 0.01 (ANOVA, p=0.006, on day 50); however, after ~200 generations, growth in the long-term (LT) treatment increased to 0.36 ± 0.01 day-1 (ANOVA, p=0.0004 compared to ST cultures, Figure 1). Carbon assimilation on the other hand increased in response to both treatment effect (ANOVA, p= 0.03 between ambient and ST cultures) and to acclimation time (ANOVA, p= 0.03 between ST and LT cultures) (Figure 2). In contrast, ambient K. veneficum cultures had a higher PSII maximum quantum yield (Fv/Fm), than the ST cultures (ANOVA, p= 0.0004, Figure 3A). Of the two treatments, Fv/Fm was significantly higher in the LT cultures than in the ST cultures (ANOVA, p=0.01). There was less variability in the effective quantum yield of PSII (Fq’/Fm’) in the light activated state (Figure 3B), as Fq’/Fm’ was significantly lower in the ST cultures than in the ambient and LT cultures (ANOVA, p=0.02 and 0.005, respectively). Overall, Fv/Fm and Fq’/Fm’ ranged between 0.44-0.48 and 0.39-0.41 respectively, and no warming or acidification induced stress on K. veneficum photochemistry was evident. Cellular chl a remained unchanged between ambient and ST cultures (ANOVA, p=0.16) and increased significantly in LT cultures compared to ST cultures (ANOVA, p<0.001, Figure 3C).
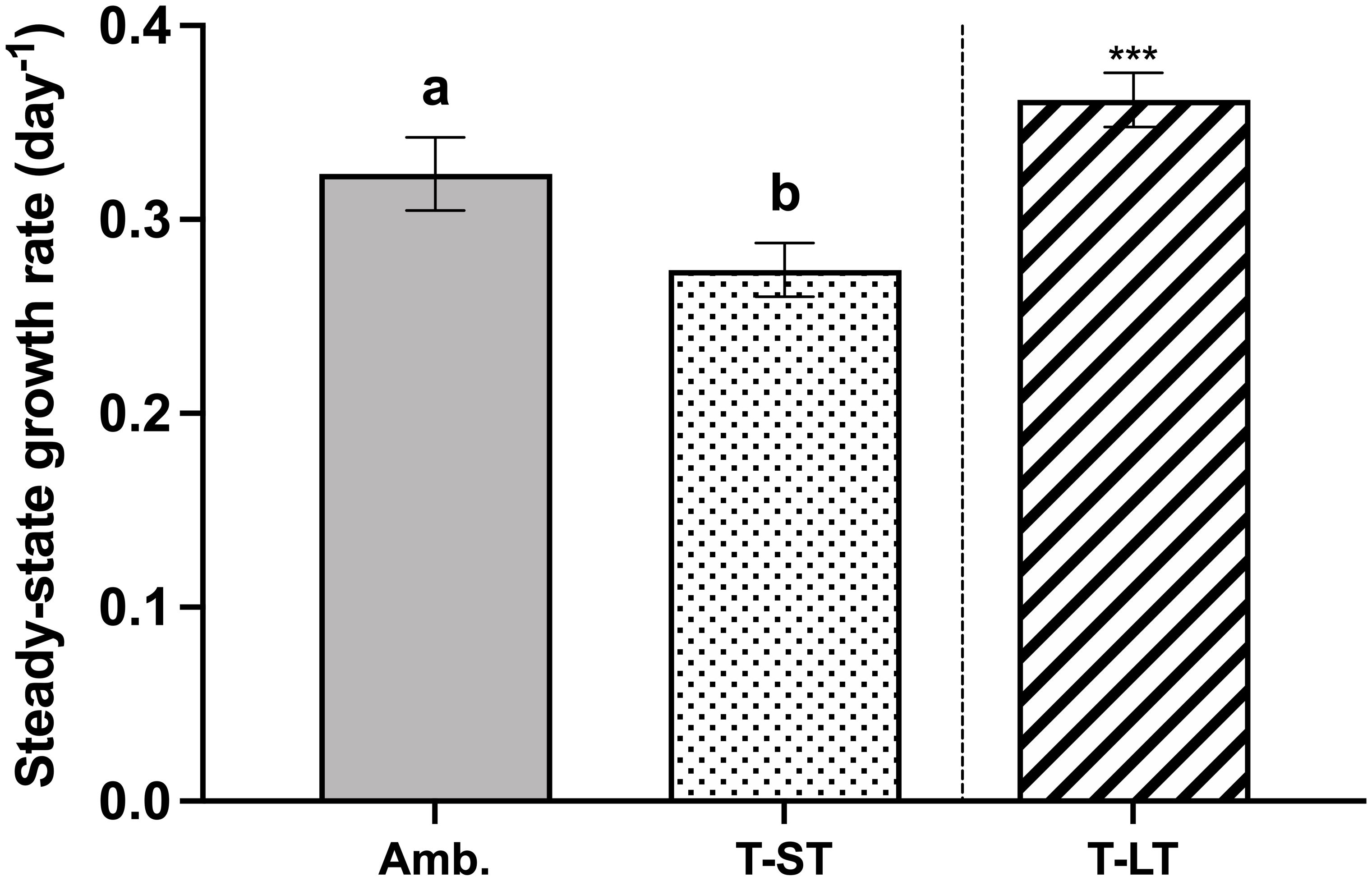
Figure 1 Steady-state growth rates (µ, day-1) of K. veneficum acclimated to Ambient (Amb.) and treatment conditions (short term: ST and long term: LT). Error bars denote the standard deviation of 40, 76 and 84 replicates for Amb., ST and LT cultures, respectively. Letters above the bars indicate significant differences between ambient and ST cultures (treatment effects) while asterisks indicate significant differences between ST and LT cultures (acclimation time effect) (ANOVA, p< 0.05).
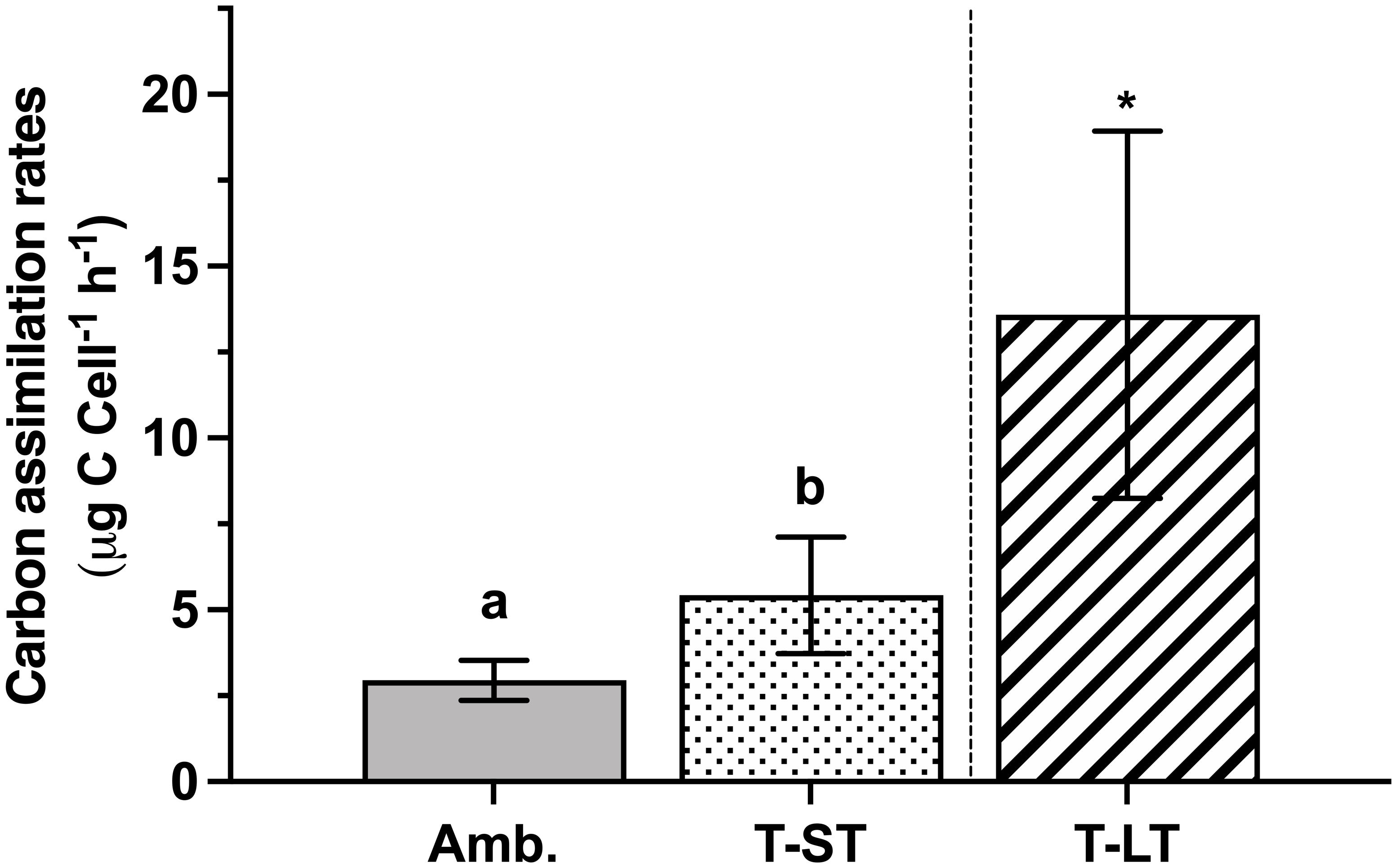
Figure 2 Carbon assimilation rates of K. veneficum (µg C Cell-1, h-1) acclimated to ambient (Amb.) and treatment conditions (short term: ST and long term: LT). Error bars denote the standard deviation of 4 replicates (n=4) for Amb and ST cultures and 3 replicates (n=3) for LT cultures. Letters above the bars indicate significant differences between ambient and ST cultures (treatment effects) while asterisks indicate significant differences between ST and LT cultures (acclimation time effect) (ANOVA, p< 0.05). Carbon assimilation rates per chlorophyll a (µg C Chl a-1, h-1) and cellular carbon (µg C C-1, h-1) yielded the same results (data not shown).
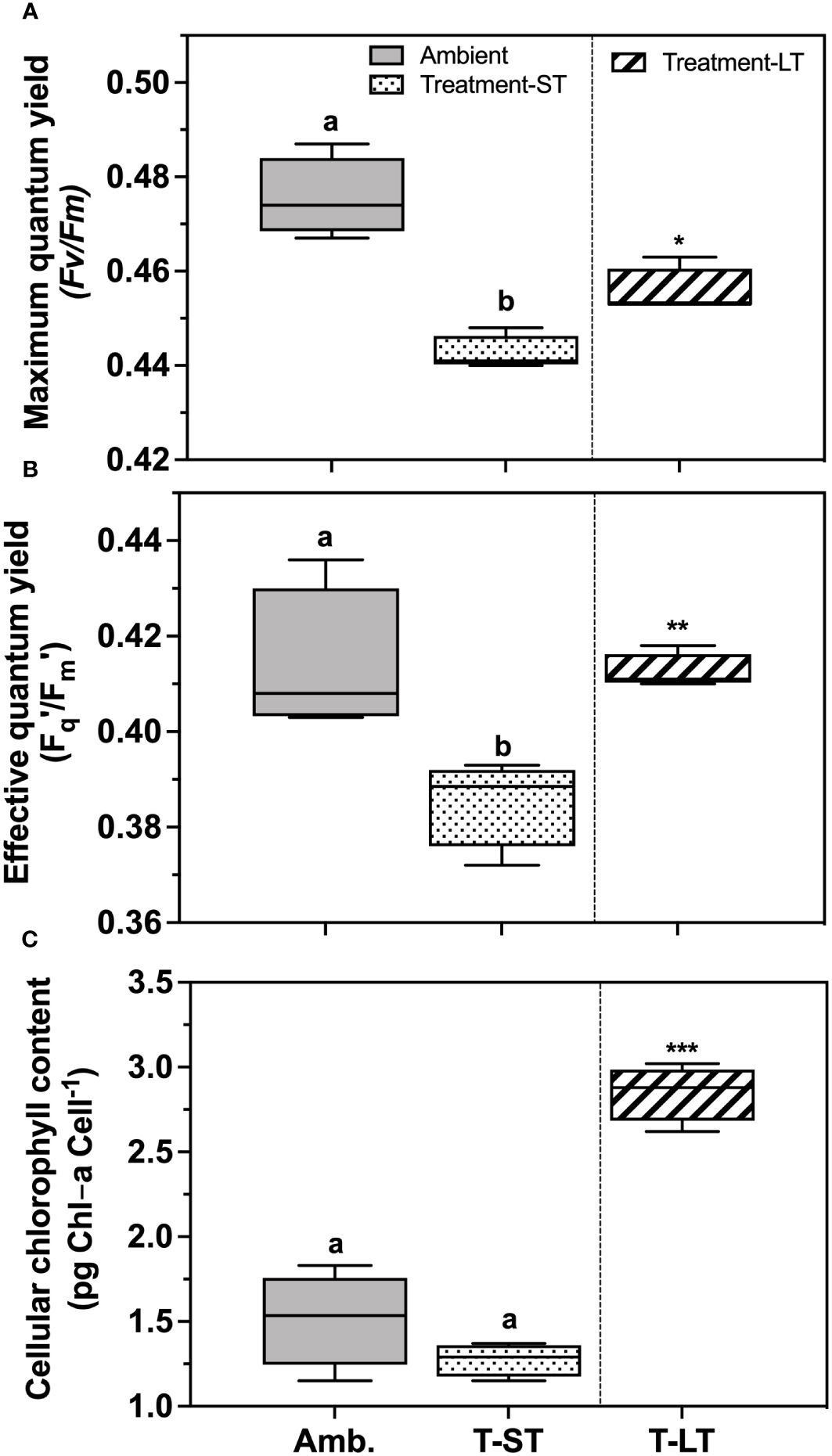
Figure 3 Maximum photochemical efficiency of photosystem II (Fv/Fm) (A), effective light acclimated photochemical efficiency of PSII (Fq′/Fm′) (B) and cellular chlorophyll-a content (pg Chl-a cell-1), (C) of K. veneficum acclimated to ambient (Amb) and treatment conditions (short term: ST and long term: LT). Error bars denote the standard deviations: Amb, n=4; ST n=36 and LT, n=21 panels (A, B) and Amb, n=4; ST, n=4; LT, n=3 (panel (C). Letters above the bars indicate significant differences between ambient and ST cultures (treatment effects) while asterisks indicate significant differences between ST and LT cultures (acclimation time effect) (ANOVA, p< 0.05).
3.2 Elemental composition and cellular carbohydrates and proteins
Cell quotas for C and P (POC and POP) were not affected by treatment (ANOVA, p= 0.08 and 0.73 for POC and POP respectively) or acclimation time (ANOVA, p= 0.40 and 0.81 for POC and POP respectively) and remained unchanged across ambient, ST, and LT cultures (Table 2). In contrast, N cell quota (PON), was significantly higher in ST cultures than in ambient cultures (ANOVA, p= 0.01), but remained unchanged between ST and LT cultures (ANOVA, p= 0.49). Meanwhile, the atomic N:P and C:P ratios remained similar across ambient and ST cultures (ANOVA, p=0.57 and 0.81 for N:P and C:P ratios respectively) and ST and LT cultures (ANOVA, p=0.94 and 0.15 for N:P and C:P ratios respectively, Table 2), while the C:N ratio was significantly lower in ST cultures than the ambient and LT cultures (ANOVA, p=0.004 and p=0.002, respectively, Table 2).
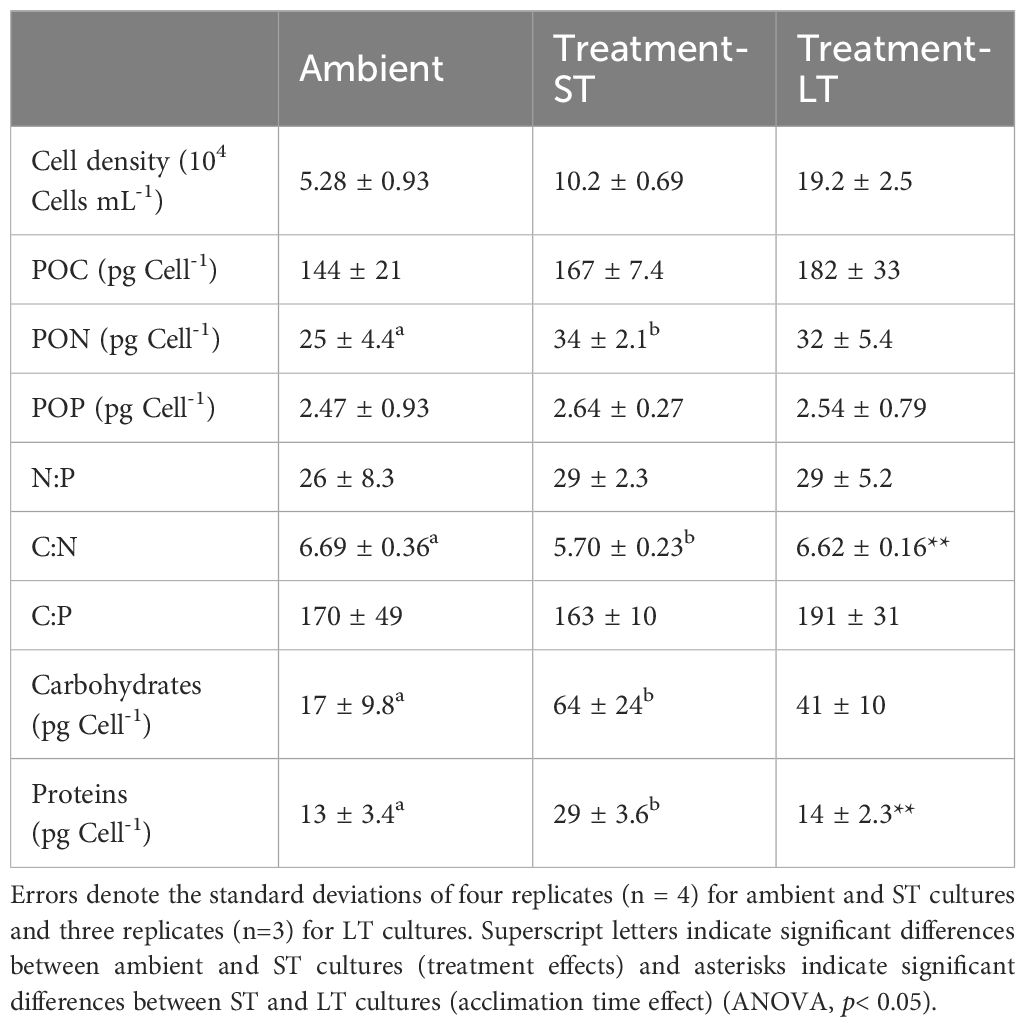
Table 2 Cell densities, cellular carbon, nitrogen, phosphorus, elemental ratios, and cellular carbohydrate and soluble protein of K. veneficum acclimated to ambient, treatment-ST and treatment-LT conditions.
Cellular carbohydrates were significantly higher in ST cultures than in ambient cultures (ANOVA, p=0.01, Table 2), but decreased slightly in the LT cultures in comparison to the ST cultures (ANOVA, p=0.2). ST culture cellular protein was also significantly higher than both ambient (ANOVA, p=0.0007) and LT cultures (ANOVA, p=0.002, Table 2).
3.3 Cellular fatty acid composition
Total fatty acid content remained similar across each culture condition and ranged between 21-33 pg cell-1 (ANOVA, p=0.09 and 0.99 for ambient vs. ST and ST vs. LT comparisons respectively, Figure 4A). In contrast, fatty acid composition changed considerably in response to (short-term treatment and time (Figures 4B, C). The percentage of saturated fatty acids (SFA) was higher in the ST cultures than the ambient cultures (ANOVA, p=0.0007), but this increase was not sustained through time under the treatment conditions, as SFAs decreased significantly in the LT cultures (ANOVA, p<0.0001, Figure 4B). In contrast, the percentage of monounsaturated fatty acids (MUFA) in the ST cultures was significantly lower than the ambient cultures (ANOVA, p<0.0001), while the LT culture MUFAs were significantly higher than the ST cultures (ANOVA, p<0.0001, Figure 4B).
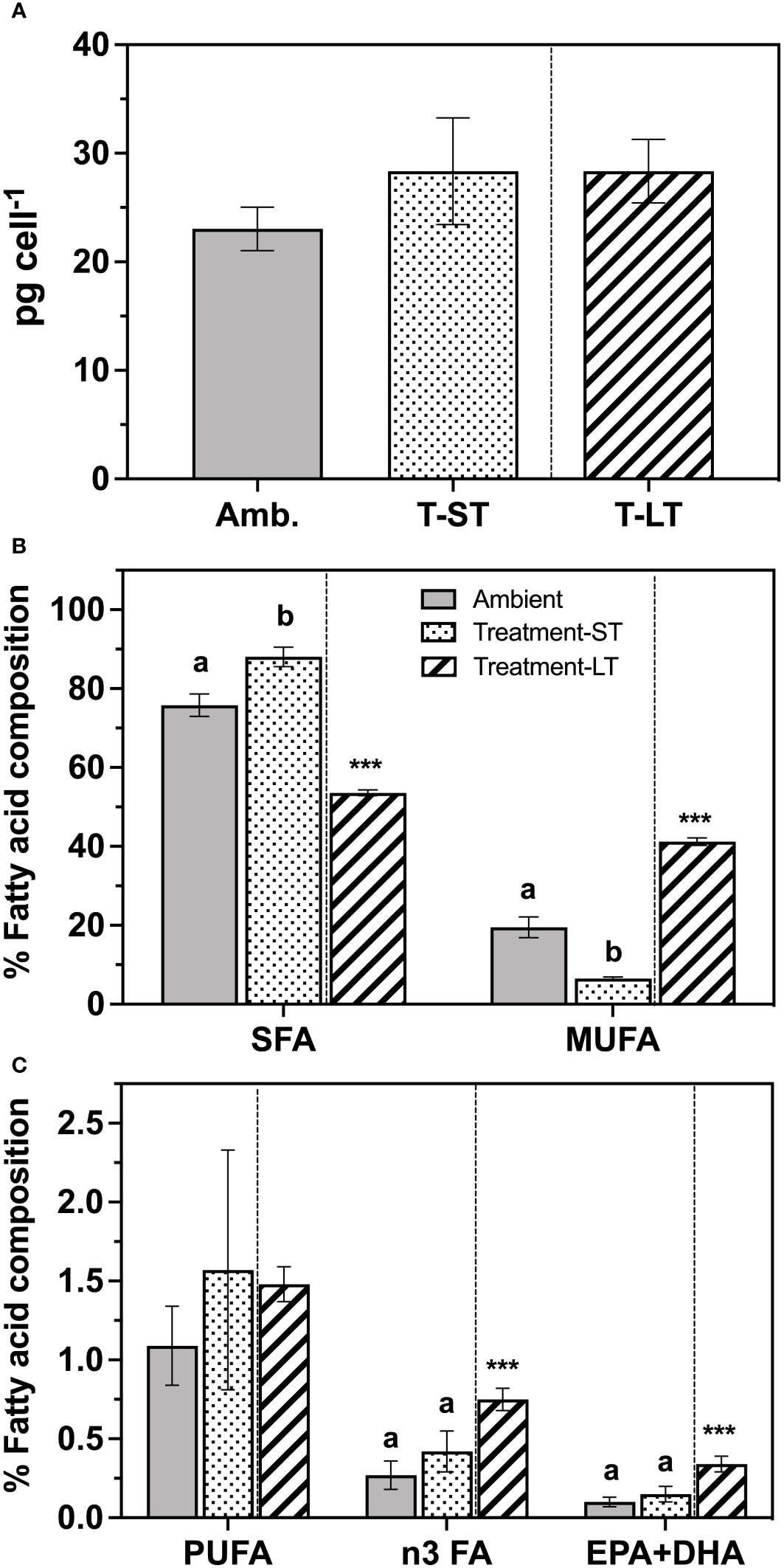
Figure 4 Total fatty acid contents (pg cell-1) (A), and percent composition of saturated fatty acids (SFA), mono-unsaturated fatty acids (MUFA) (B), and polyunsaturated fatty acids (PUFA) (C) of K. veneficum acclimated to ambient (Amb) and treatment conditions (short term: ST and long term: LT). Error bars denote the standard deviation of 4 replicates (n=4) for Amb and ST cultures and 3 replicates (n=3) for LT cultures. Letters above the bars indicate significant differences between ambient and ST cultures (treatment effects) while asterisks indicate significant differences between ST and LT cultures (acclimation time effect) (ANOVA, p< 0.05). n3 FA, omega-3 fatty acids; EPA, Eicosapentaenoic acid; DHA, Docosahexaenoic acid.
Polyunsaturated fatty acids (PUFA) accounted for only 2.4–8.6% of total FA content and were similar between ambient and ST (ANOVA, p=0.61), and ST and LT culture conditions (ANOVA, p=0.89, Figure 4C). However, the PUFA composition changed substantially in the LT cultures. In particular, the percentage of n3 fatty acids (n3 FA), a subgroup of PUFA, was significantly greater than those recorded in the ST cultures (ANOVA, p=0.0003, Figure 4C). Notably, both major essential fatty acids EPA (eicosapentaenoic acid) and DHA (docosahexaenoic acid) were significantly greater in LT cultures than in the ST cultures (ANOVA, p<0.0001, Figure 4C). We also observed an increase in the percentage of very long chain fatty acids over time (VLCFA, i.e. FA with carbon chains >20) from ~6% in ST cultures to approximately 15% in the LT cultures (Supplementary Table 1).
Twenty-seven fatty acids were identified (Supplementary Table 1), and the two most abundant SFA in K. veneficum were palmitic acid (16:0) and myristic acid (14:0), which together represented 66%, 76% and 45% of the total FA content at ambient, ST and LT cultures, respectively. Oleic and elaidic acids (18:1 cis+trans) together represented the major MUFA in ambient cultures, while eicosenoic acid (20:1) was the dominant MUFA in the treatment cultures. Karlodinium veneficum n3 PUFA included alpha-linolenic acid (ALA, 18:3n-3), eicosapentaenoic acid (EPA, 20:5n-3), and docosahexaenoic acid (DHA, 22:6n-3). In addition, eicosatrienoic acid (ETE, 20:3n-3) was also detected in the LT cultures.
3.4 Cell toxicity
Hemolytic activity normalized to either cell number or carbon remained unchanged between the ambient and ST treatment and when comparing ST vs LT conditions (ANOVA, p>0.5, Figure 5); however, toxicity against the fish gill cell (FGC) line increased (i.e., EC50 decreased) significantly in both ST and LT cultures (Figure 5). Specifically, the FGC toxicity in the ST cultures was 2.5 times greater than the ambient cultures (ANOVA, p=0.001), while FGC toxicity was 2.6 times greater in the LT cultures than in the ST cultures (ANOVA, p=0.005). Likewise, when normalized to cellular carbon (EC50: µg C mL-1), FGC toxicity followed a similar pattern and increased significantly from ambient to ST (ANOVA, p=0.0001) and ST to LT (ANOVA, p= 0.01) culture conditions.
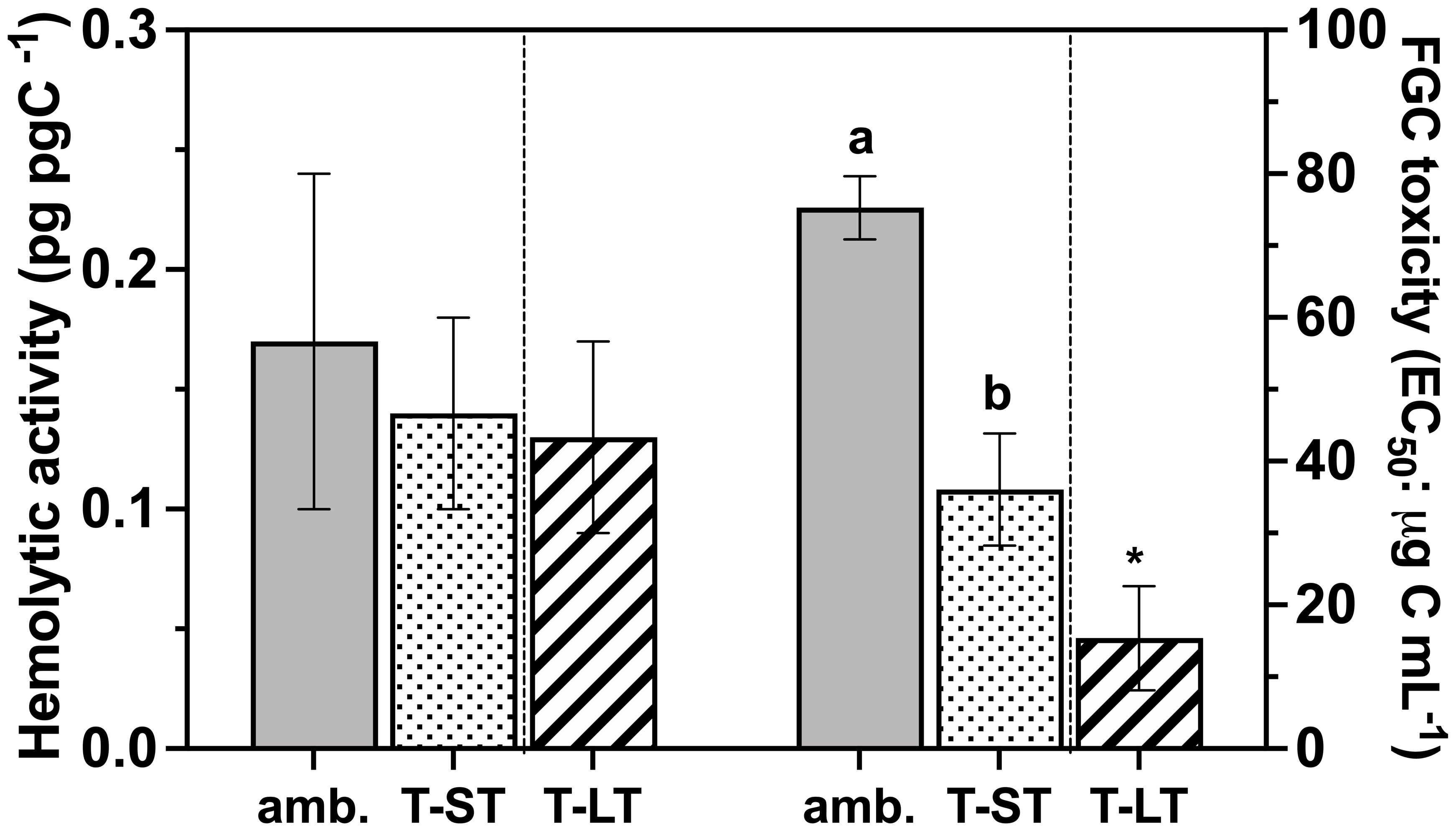
Figure 5 Hemolytic activity (pg pg C-1 - Left Y axes) and the rainbow trout fish gill cell toxicity (FGC; EC50: µg Carbon mL-1 - Right Y axes) of K. veneficum acclimated to ambient (Amb) and treatment (short term: ST and long term: LT) conditions. Error bars denote the standard deviation of four replicates (n=4) for Amb and ST cultures and three replicates (n=3) for LT cultures. Letters above the bars indicate significant differences between ambient and ST cultures (treatment effects) while asterisks indicate significant differences between ST and LT cultures (acclimation time effect) (ANOVA, p< 0.05).
4 Discussion
Acclimating K. veneficum to long term (LT, ~200 generations) combined elevated temperature and CO2 increased carbon assimilation, growth, and FGC toxicity. The long-term treatment also resulted in major changes in key fatty acid classes, as the percent of monounsaturated (MUFA) and essential fatty acids (n3 FA) increased, while saturated fatty acids (SFA) decreased. However, some of these differences were not evident or changed in opposite ways when K. veneficum was acclimated to short-term (ST, ~20 generations) elevated temperature and CO2. For example, ST growth and % MUFA were lower and % SFA were higher than the ambient cultures, while carbon fixation remained similar in both conditions. In contrast, other cellular parameters, such as FGC toxicity, were consistently higher in ST cultures than in ambient conditions. These different results between the ST and LT experiments suggest that complete acclimation to elevated temperature and pCO2 required >20 generations.
4.1 The effects of combined elevated temperature and CO2 on K. veneficum growth and toxicity
While our experimental design did not allow us to test the independent effects of elevated temperature and CO2, K. veneficum and some other dinoflagellates often grow faster under elevated CO2 (Fu et al., 2010; Errera et al., 2014) but grow slower under elevated temperature (Seto et al., 2019; Vidyarathna et al., 2020, 2023). However, pre-acclimation to heating prior to changing CO2 can lead to different outcomes, as Coyne et al. (2021) noted higher growth after acclimating K. veneficum to 30°C for over one year prior to applying short-term high CO2 (24 days/750 ppm CO2). Excess CO2 may provide this K veneficum isolate some relief from heating, whereby elevated CO2 reduces the energetic demand for acquiring and fixing carbon at higher temperatures. We hypothesize that lower growth rates in the ST cultures were likely caused by a stronger negative effect of temperature than high CO2. This may be common in many short-term experiments (typically < ~20 generations) and explains similar growth responses in this and other dinoflagellates when comparing ‘combined’ and ‘warming only’ treatments in similar experimental designs (Feng et al., 2008; Fu et al., 2008; Seto et al., 2019). Likewise, our previous work has noted that the thermal optima for growth in this strain of K. veneficum is closer to 25°C (Vidyarathna et al., 2020). While carbon fixation remained similar between the ambient and ST treatment, the lower photochemical performance in the ST treatment (Figures 3A, B) that was not apparent in the LT treatment suggests that some aspect of photosynthetic electron transport in the light reactions may have been the rate limiting step in the ST acclimation. Although not evaluated in our study, the possible shifts in bacterial consortia [e.g. Alteromonas (Deng et al., 2022)] associated with K. veneficum cultures may also have contributed to promoting algal growth under elevated temperature and CO2 as has been reported for other photosynthetic organisms such as Prochlorococcus (Hennon et al., 2018).
While growth was higher in the LT treatment, carbon assimilation still exceeded the growth rate while elemental composition remained unchanged. Such decoupling between photosynthesis and growth may signal a mechanism to remove excess photosynthate as dissolved organic carbon, perhaps as transparent exopolymeric particles (Engel et al., 2014; Passow and Laws, 2015), when electron transport and carbon fixation are high (Claquin et al., 2008). In contrast, when exposed to long term high CO2 (~900 generations), the diatom Phaeodactylum tricornutum reduced photosynthesis and respiration, thereby allocating a similar amount of carbon for growth as the low CO2 selected population with no change in growth rates (Jin et al., 2022). Similar to our LT treatments, phytoplankton elemental composition, including in other dinoflagellates, does not necessarily change in response to elevated CO2 (Burkhardt et al., 1999; Clark et al., 2014; Wynn-Edwards et al., 2014; Sugie and Yoshimura, 2016; Bercel and Kranz, 2019; Xu et al., 2023), but intracellular distributions can still change, as Chl a content approximately doubled in our LT treatments. In contrast, nitrogen, protein, and carbohydrate quotas increased in the ST cultures and this excess stored carbon may reflect a more immediate physiological response to elevated temperature and CO2.
The greater K. veneficum FGC toxicity under elevated temperature and CO2 noted between ambient and ST cultures as well as ST and LT cultures is similar (~ 52%) to increased FGC toxicity in this same isolate in response to elevated temperature alone, which peaked at 25°C–28°C (Vidyarathna et al., 2020). Hence, the increased FGC toxicity in our ST treatment was possibly due more to elevated temperature than CO2. Meanwhile, the even greater increase in FGC toxicity in the LT cultures (57% higher than the ST cultures) perhaps represented further cellular adjustments to carbon allocation in response to the combined effects of elevated temperature and CO2.
In contrast to FGC toxicity, hemolytic toxicity remained similar across ambient and the ST treatment cultures and also did not change in the LT treatment. Notably, elevated temperature alone led to increased hemolytic toxicity in late-exponential phase batch cultures of K. veneficum, and batch grown K. veneficum hemolytic toxicity cell-1 at 25°C was 4x higher (Vidyarathna et al., 2020) than in the continuous cultures reported here. Further, although K. veneficum hemolytic activity increased with elevated CO2 and P-limitation (Fu et al., 2010), the absolute toxicity cell-1 was lower than data from batch cultures [> ~50 ng cell-1 vs. 95 ng cell-1 in Vidyarathna et al. (2020)]. While we cannot discount possible synergestic effects of elevated temperature and CO2, K. veneficum hemolytic activity may be affected more by nutrient limitation and starvation.
4.2 Temporal changes in K veneficum lipids under elevated temperature and CO2 and the possible implications for trophic transfer and mixotrophy
Phytoplankton often change their fatty acid (FA) composition to maintain membrane homeoviscocity in response to changing temperature (Sinensky, 1974). Increasing saturated fatty acid content at higher temperature helps to maintain membrane rigidity (Fuschino et al., 2011; Baker et al., 2018; Hixson and Arts, 2016), which we also noted in our ST treatment. However, not all shifts in FA content in our ST treatment were similar to previous studies where the % PUFA often declines at higher temperature (Fuschino et al., 2011; Hixson and Arts, 2016; Torstensson et al., 2013; Hyun et al., 2016) but remained unchanged here. Further, while FA composition (% SFA and MUFA) did not change in phytoplankton within mesocoms exposed to elevated temperature and CO2 for 20 days (Meyers et al., 2022), we found a significant decrease in the % MUFA in our ST treatment. Hence, K. veneficum FA reaction norms may change rapidly in response to both elevated temperature and CO2 but are likely species- and strain- dependent (Jin et al., 2020) and not necessarily reflective of the larger phytoplankton community.
In contrast to the ST cultures, the significant increase in growth and decline in total saturated FA in the LT cultures may have been an adaptive response; however, our experimental design did not have a long-term ambient control to directly compare against the LT treatment. Furthermore, this also prevented us from explicitly testing for trait adaptation by performing reciprocal shifts between ambient and treatment conditions (i.e., comparing high temperature and CO2 treated algae against the ambient control samples after shifting them into the high temperature and CO2 and vice versa) (Schlüter et al., 2014). Nevertheless, the decline in saturated FA is similar to that noted in the diatom Thalassiosira pseudonana, where saturated FA were lower after 500 generations at 31°C, providing evidence for some thermal adaptation (O’Donnell et al., 2019). Importantly, the measured response traits in long-term experiments can return to pre-exposure levels or fall below those of the original non-adapted population (Schlüter et al., 2016; Collins et al., 2020). For example, initial FA and lipids declined in three diatom species and then fully recovered after two years of long-term growth at 30°C (Jin et al., 2020). However, the FA profile shift in our LT cultures was a significant re-arrangement in lipid composition that included a larger proportion of essential FA and very long chain FA (VLCFA) not seen in the ST cultures. Furthermore, n-3 PUFA and n-6 PUFA increased in LT cultures (Figure 4C; Supplementary Table 1) and contrasts with the suggestion that global heating alone could favor phytoplankton n-6 PUFA over n-3 PUFA (Hixson and Arts, 2016). Hence, the increased FA elongation and desaturation in K. veneficum in response to long-term exposure to elevated temperature and CO2 may represent a unique interactive effect of these combined climate stressors.
Altered algal fatty acid (FA) profiles due to climate change has important ecological implications for marine food webs, as primary consumers acquire most FA through phytoplankton grazing. While total FA content remained unchanged, elevated temperature and CO2 led to major changes in key K. veneficum FA classes. Studies with other algal species have reported a broad range of FA variability from little to no change (e.g. Fitzer et al., 2019; O’Donnell et al., 2019; Meyers et al., 2022) to a significant decline in response to elevated temperature and/or CO2 (Rossoll et al., 2012; Hyun et al., 2016; Jacob et al., 2017). Increased essential fatty acids, including EPA, DHA, in the LT treatment holds particular ecological relevance as they are exclusively synthesized by phytoplankton and affect both consumer growth and fitness. Declining phytoplankton n3 FA in response to elevated temperature potentially weakens energy and mass transfer through the marine food web (Fuschino et al., 2011; Rossoll et al., 2012; Fitzer et al., 2019; Meyers et al., 2019) where a temperature increase of 2.5°C may reduce phytoplankton EPA by 8.2% and DHA by 27.8% (Hixson and Arts, 2016). In contrast, we found a consistent increase in the percentage of n3-PUFA when moving from ambient to ST and ST to LT treatments. Additionally, since the total FA cell-1 did not change, the increased n3-PUFA may have resulted from desaturating pre-existing fatty acids–a process that requires reducing power from NADPH or NADH (Sato et al., 2003). Here, n3-PUFA increased proportionally with photosynthetic C fixation across ambient and treatment cultures (Figure 4), hence excess energy not used for growth may have been used to support desaturation of SFA to n3-FA.
Altered biochemical composition of algal prey can affect the growth, reproduction, survival, and biochemical stoichiometry of grazers such as copepods and bivalves (Rossoll et al., 2012; Cripps et al., 2016; Fields et al., 2022; Pan et al., 2023), and leads to cascading effects to fitness at higher trophic levels. For example, Cripps et al. (2016) estimated that at elevated CO2, carbon trophic transfer efficiency from phytoplankton to copepods declined to <50% of the control population. However, other studies have reported greater fitness in the copepod, Calanus finmarchicus when raised at elevated CO2 (Fields et al., 2022) and copepod biomass (particularly in smaller size classes) can increase at elevated CO2, likely due to indirect effects through increased food availability (e.g. Taucher et al., 2017). In contrast, others have reported that ocean acidification alone or, in combination with elevated temperature, did not affect prey or zooplankton FA composition (Meyers et al., 2022). While these responses are results of a complex interplay of trophic interactions, short-term acclimation vs long-term adaptation as well as differences in sensitivities to elevated temperature and/or CO2 among different taxa may explain some of this variability (Lohbeck et al., 2012; Tatters et al., 2013b; Bermúdez et al., 2015; Jin et al., 2020; Xu et al., 2023). Among the order Gymnodiniales, Karlodinium species are globally prevalent (Le Bescot et al., 2016), and K. veneficum is a common constituent in coastal mid-Atlantic waters such as the Chesapeake Bay (Zhang et al., 2008). Further, given that K. veneficum is consumed by micrograzers, including other heterotrophic dinoflagellates in this region (Johnson et al., 2003) as well as the copepod Acartia tonsa (Hong et al., 2012), possible shifts in algal biochemical composition under future warming and acidification as shown here could affect such trophic interactions.
In addition to autotrophy, mixotrophic feeding by K. veneficum can significantly increase bloom formation (Li A. et al., 2001; Place et al., 2012; Li et al., 2015). While not included in our experimental design, mixotrophy could play an important role in some climate change scenarios, especially in regard to elevated temperature, which can significantly increase prey ingestion and digestion (Li A. et al., 2001; Wilken et al., 2013; Li et al, 2022). Other factors related to bloom progression, such as nutrient starvation, can negatively affect algal response to pH-induced stress, and are important to consider in future studies, as mixotrophic phytoplankton may be favored under some ocean acidification scenarios (Flynn et al., 2015). Taken together our results suggest that elevated CO2 and temperature can enhance carbon assimilation and increase K. veneficum autotrophic growth and toxicity, which could further influence bloom formation and severity. However, long-term exposure to these conditions also resulted in notable shifts in greater proportions of essential fatty acids that might counteract the negative effects of elevated cell toxicity to grazers. Such interactions, as well as possible shifts in mixotrophic behavior and subsequent changes to algal toxicity warrant further investigation in more complex future climate change simulations.
Data availability statement
The raw data supporting the conclusions of this article will be made available by the authors, without undue reservation.
Ethics statement
Ethical approval was not required for the studies on animals in accordance with the local legislation and institutional requirements because only commercially available established cell lines were used.
Author contributions
NV: Conceptualization, Data curation, Formal analysis, Investigation, Methodology, Visualization, Writing – original draft, Writing – review & editing. LS: Investigation, Writing – review & editing. KM: Investigation, Resources, Writing – review & editing. KC: Conceptualization, Funding acquisition, Resources, Writing – review & editing. JC: Conceptualization, Funding acquisition, Resources, Supervision, Writing – review & editing. MW: Conceptualization, Funding acquisition, Methodology, Project administration, Supervision, Writing – original draft, Writing – review & editing.
Funding
The author(s) declare financial support was received for the research, authorship, and/or publication of this article. This study was supported by the National Oceanic and Atmospheric Administration (NOAA) through grant NA15NOS4780182.
Acknowledgments
We thank the reviewers for their valuable comments. We thank Dr. Patricia Glibert (Horn point laboratory-UMCES) for valuable comments on the manuscript. This is contribution number ECO1091 from the NOAA ECOHAB program.
Conflict of interest
The authors declare that the research was conducted in the absence of any commercial or financial relationships that could be construed as a potential conflict of interest.
The author(s) declared that they were an editorial board member of Frontiers, at the time of submission. This had no impact on the peer review process and the final decision.
Publisher’s note
All claims expressed in this article are solely those of the authors and do not necessarily represent those of their affiliated organizations, or those of the publisher, the editors and the reviewers. Any product that may be evaluated in this article, or claim that may be made by its manufacturer, is not guaranteed or endorsed by the publisher.
Supplementary material
The Supplementary Material for this article can be found online at: https://www.frontiersin.org/articles/10.3389/fmars.2024.1305495/full#supplementary-material
References
Adolf J. E., Bachvaroff T. R., Place A. R. (2009). Environmental modulation of karlotoxin levels in strains of the cosmopolitan dinoflagellate, Karlodinium veneficum (Dinophyceae). J. Phycol. 45, 176–192. doi: 10.1111/j.1529-8817.2008.00641.x
Adolf J. E., Krupatkina D., Bachvaroff T., Place A. R. (2007). Karlotoxin mediates grazing by Oxyrrhis marina on strains of Karlodinium veneficum. Harmful Algae 6, 400–412. doi: 10.1016/j.hal.2006.12.003
Adolf J. E., Bachvaroff T., Place A. R. (2008). Can cryptophyte abundance trigger toxic karlodinium veneficum blooms in eutrophic estuaries? Harmful Algae 8, 119–128. doi: 10.1016/j.hal.2008.08.003
Baker K. G., Radford D. T., Evenhuis C., Kuzhiumparam U., Ralph P. J., Doblin M. A. (2018). Thermal niche evolution of functional traits in a tropical marine phototroph. J. Phycol. 54, 799–810. doi: 10.1111/jpy.12759
Bercel T. L., Kranz S. A. (2019). Insights into carbon acquisition and photosynthesis in Karenia brevis under a range of CO2 concentrations. Prog. Oceanogr. 172, 65–76. doi: 10.1016/j.pocean.2019.01.011
Bermúdez R., Feng Y., Roleda M. Y., Tatters A. O., Hutchins D. A., Larsen T., et al. (2015). Long-term conditioning to elevated pCO2 and warming influences the fatty and amino acid composition of the diatom Cylindrotheca fusiformis. PloS One 10, 1–15. doi: 10.1371/journal.pone.0123945
Brading P., Warner M. E., Davey P., Smith D. J., Achterberg E. P., Suggett D. J. (2011). Differential effects of ocean acidification on growth and photosynthesis among phylotypes of Symbiodinium (Dinophyceae). Limnol. Oceanogr. 56, 927–938. doi: 10.4319/lo.2011.56.3.0927
Brandenburg K. M., Velthuis M., Van de Waal D. B. (2019). Meta-analysis reveals enhanced growth of marine harmful algae from temperate regions with warming and elevated CO2 levels. Glob Change Biol. 25, 2607–2618. doi: 10.1111/gcb.14678
Brownlee E. F., Sellner S. G., Sellner K. G., Nonogaki H., Adolf J. E., Bachvaroff T. R., et al. (2008). Responses of Crassostrea virginica (Gmelin) and C. ariakensis (Fujita) to bloom-forming phytoplankton including ichthyotoxic Karlodinium veneficum (Ballantine). J. Shellfish Res. 27, 581–591. doi: 10.2983/0730-8000(2008)27[581:ROCVGA]2.0.CO;2
Burkhardt S., Zondervan I., Riebesell U. (1999). Effect of CO2 concentration on C:N:P ratio in marine phytoplankton: A species comparison. Limnol. Oceanogr. 44, 683–690. doi: 10.4319/lo.1999.44.3.0683
Claquin P., Probert I., Lefebvre S., Veron B. (2008). Effects of temperature on photosynthetic parameters and TEP production in eight species of marine microalgae. Aquat. Microb. Ecol. 51, 1–11. doi: 10.3354/ame01187
Clark D. R., Flynn K. J., Fabian H. (2014). Variation in elemental stoichiometry of the marine diatom Thalassiosira weissflogii (Bacillariophyceae) in response to combined nutrient stress and changes in carbonate chemistry. J. Phycol. 50, 640–651. doi: 10.1111/jpy.12208
Collins S., Bell G. (2004). Phenotypic consequences of 1000 generations of selection at elevated CO2 in a green alga. Nature 431, 566–569. doi: 10.1038/nature02945
Collins S., Boyd P., Doblin M. A. (2020). Evolution, microbes, and changing ocean conditions. Annu. Rev. Mar. Sci. 12, 181–208. doi: 10.1146/annurev-marine-010318-095311
Cosgrove J., Borowitzka M. A. (2010). ““Chlorophyll fluorescence terminology: an introduction,”,” in Chlorophyll a Fluorescence in Aquatic Sciences: Methods and Applications. Eds. Suggett D. J., Prášil O., Borowitzka M. A. (Springer Netherlands, Dordrecht), 1–17. doi: 10.1007/978-90-481-9268-7_1
Coyne K. J., Salvitti L. R., Mangum A. M., Ozbay G., Main C. R., Kouhanestani Z. M., et al. (2021). Interactive effects of light, CO2 and temperature on growth and resource partitioning by the mixotrophic dinoflagellate, Karlodinium veneficum. PloS One 16, e0259161. doi: 10.1371/journal.pone.0259161
Cripps G., Flynn K. J., Lindeque P. K. (2016). Ocean acidification affects the phyto-zoo plankton trophic transfer efficiency. PloS One 11, 1–15. doi: 10.1371/journal.pone.0151739
Dayeh V. R., Schirmer K., Lee L. E. J., Bols N. C. (2005). ““Rainbow trout gill cell line microplate cytotoxicity test,”,” in Small-scale Freshwater Toxicity Investigations: Toxicity Test Methods. Eds. Blaise C., Férard J.-F. (Springer Netherlands, Dordrecht), 473–503. doi: 10.1007/1-4020-3120-3_16
Deeds J. R., Terlizzi D. E., Adolf J. E., Stoecker D. K., Place A. R. (2002). Toxic activity from cultures of Karlodinium micrum (=Gyrodinium galatheanum) (Dinophyceae) - A dinoflagellate associated with fish mortalities in an estuarine aquaculture facility. Harmful Algae 1, 169–189. doi: 10.1016/S1568-9883(02)00027-6
Deng Y., Wang K., Hu Z., Hu Q., Tang Y. Z. (2022). Identification and implications of a core bacterial microbiome in 19 clonal cultures laboratory-reared for months to years of the cosmopolitan dinoflagellate Karlodinium veneficum. Front. Microbiol. 13. doi: 10.3389/fmicb.2022.967610
DuBois M., Gilles K., Hamilton J. K., Rebers P. A., Smith F. (1951). A colorimetric method for the determination of sugars. Nature 168, 167. doi: 10.1038/168167a0
Engel A., Piontek J., Grossart H.-P., Riebesell U., Schulz K. G., Sperling M. (2014). Impact of CO2 enrichment on organic matter dynamics during nutrient induced coastal phytoplankton blooms. J. Plankton Res. 36, 641–657. doi: 10.1093/plankt/fbt125
Ernst O., Zor T. (2010). Linearization of the bradford protein assay. J. Vis. Exp. 38, 1918. doi: 10.3791/1918
Errera R. M., Yvon-Lewis S., Kessler J. D., Campbell L. (2014). Reponses of the dinoflagellate Karenia brevis to climate change: pCO2 and sea surface temperatures. Harmful Algae 37, 110–116. doi: 10.1016/j.hal.2014.05.012
Eschbach E., Scharsack J. P., John U., Medlin L. K. (2001). Improved erythrocyte lysis assay in microtiter plates for sensitive detection and efficient measurement of hemolytic compounds from ichthyotoxic algae. J. Appl. Toxicol. 21, 513–519. doi: 10.1002/jat.797
Feng Y., Warner M. E., Zhang Y., Sun J., Fu F., Rose J. M., et al. (2008). Interactive effects of increased pCO2, temperature and irradiance on the marine coccolithophore Emiliania huxleyi (Prymnesiophyceae). Eur. J. Phycology 43, 87–98. doi: 10.1080/09670260701664674
Fields D. M., Runge J. A., Thompson C. R. S., Durif C. M. F., Shema S. D., Bjelland R. M., et al. (2022). A positive temperature-dependent effect of elevated CO2 on growth and lipid accumulation in the planktonic copepod, Calanus finmarchicus. Limnol. Oceanogr. 68, S87–S100. doi: 10.1002/lno.12261
Fitzer S. C., Plancq J., Floyd C. J., Kemp F. M., Toney J. L. (2019). Increased pCO2 changes the lipid production in important aquacultural feedstock algae Isochrysis galbana, but not in Tetraselmis suecica. Aquac. Fish. 4, 142–148. doi: 10.1016/j.aaf.2019.02.008
Flores-Moya A., Rouco M., García-Sánchez M. J., García-Balboa C., González R., Costas E., et al. (2012). Effects of adaptation, chance, and history on the evolution of the toxic dinoflagellate Alexandrium minutum under selection of increased temperature and acidification. Ecol. Evol. 2, 1251–1259. doi: 10.1002/ece3.198
Flynn K. J., Clark D. R., Mitra A., Fabian H., Hansen P. J., Glibert P. M., et al. (2015). Ocean acidification with (de)eutrophication will alter future phytoplankton growth and succession. Proc. R. Soc B Biol. Sci. 282, 20142604. doi: 10.1098/rspb.2014.2604
Friedrich G. E., Walz P. M., Burczynski M. G., Chavez F. P. (2002). Inorganic carbon in the central California upwelling system during the 1997-1999 El Ninão-La Ninãa event. Prog. Oceanogr. 54, 185–203. doi: 10.1016/S0079-6611(02)00049-6
Fu F. X., Place A. R., Garcia N. S., Hutchins D. A. (2010). CO2 and phosphate availability control the toxicity of the harmful bloom dinoflagellate Karlodinium veneficum. Aquat. Microb. Ecol. 59, 55–65. doi: 10.3354/ame01396
Fu F. X., Zhang Y., Warner M. E., Feng Y., Sun J., Hutchins D. A. (2008). A comparison of future increased CO2 and temperature effects on sympatric Heterosigma akashiwo and Prorocentrum minimum. Harmful Algae 7, 76–90. doi: 10.1016/j.hal.2007.05.006
Fuschino J. R., GusChina I. A., Dobson G., Yan N. D., Harwood J. L., Arts M. T. (2011). Rising water temperatures alter lipid dynamics and reduce n-3 essential fatty acid concentrations in Scenedesmus obliquus (chlorophyta). J. Phycol. 47, 763–774. doi: 10.1111/jpy.2011.47.issue-4
Glibert P. M. (2020). Harmful algae at the complex nexus of eutrophication and climate change. Harmful Algae 91, 101583. doi: 10.1016/j.hal.2019.03.001
Glibert P. M., Alexander J., Meritt D. W., North E. W., Stoecker D. K. (2007). Harmful algae pose additional challenges for oyster restoration: impacts of the harmful algae Karlodinium veneficum and Prorocentrum minimum on early life stages of the oysters Crassostrea virginica and Crassostrea ariakensis. J. Shellfish Res. 26, 919–925. doi: 10.2983/0730-8000(2007)26[919:HAPACF]2.0.CO;2
Glibert P. M., Icarus Allen J., Artioli Y., Beusen A., Bouwman L., Harle J., et al. (2014). Vulnerability of coastal ecosystems to changes in harmful algal bloom distribution in response to climate change: projections based on model analysis. Glob. Change Biol. 20, 3845–3858. doi: 10.1111/gcb.12662
Gobler C. J., Doherty O. M., Hattenrath-Lehmann T. K., Griffith A. W., Kang Y., Litaker R. W. (2017). Ocean warming since 1982 has expanded the niche of toxic algal blooms in the North Atlantic and North Pacific oceans. Proc. Natl. Acad. Sci. 114, 4975–4980. doi: 10.1073/pnas.1619575114
Gobler C. J. (2020). Climate change and harmful algal blooms: Insights and perspective. Harmful Algae. 91, 101731. doi: 10.1016/j.hal.2019.101731
Guillard R. R. L., Ryther J. H. (1962). Studies of Marine Planktonic Diatoms: I. Cyclotella nana Hustedt, and Detonula confervacea (Cleve) Gran. Can. J. Microbiol. 8, 229–239. doi: 10.1139/m62-029
Hallegraeff G. M. (2010). Ocean climate change, phytoplankton community responses, and harmful algal blooms: A formidable predictive challenge. J. Phycol. 46, 220–235. doi: 10.1111/jpy.2010.46.issue-2
Hattenrath-Lehmann T. K., Smith J. L., Wallace R. B., Merlo L. R., Koch F., Mittelsdorf H., et al. (2015). The effects of elevated CO2 on the growth and toxicity of field populations and cultures of the saxitoxin-producing dinoflagellate, Alexandrium fundyense. Limnol. Oceanogr. 60, 198–214. doi: 10.1002/lno.10012
Hennon G. M. M., Limón M. D. H., Haley S. T., Juhl A. R., Dyhrman S. T., Scott K. (2017). Diverse CO2 -induced responses in physiology and gene expression among eukaryotic phytoplankton. Front. Microbiol. 8. doi: 10.3389/fmicb.2017.02547
Hennon G. M. M., Morris J. J., Haley S. T., Zinser E. R., Durrant A. R., Entwistle E., et al. (2018). The impact of elevated CO2 on Prochlorococcus and microbial interactions with ‘helper’ bacterium Alteromonas. ISME J. 12, 520–531. doi: 10.1038/ismej.2017.189
Hixson S. M., Arts M. T. (2016). Climate warming is predicted to reduce omega-3, long-chain, polyunsaturated fatty acid production in phytoplankton. Glob Chang Biol. 22 (8), 2744–2755. doi: 10.1111/gcb.13295
Hong H., Talapatra S., Katz J., Tester P. A., Waggett R. J., Place A. R. (2012). Algal toxins alter copepod feeding behavior. PloS One 7, e36845. doi: 10.1371/journal.pone.0036845
Hyun B., Ju S. J., Ko A. R., Choi K. H., Jung S. W., Jang P. G., et al. (2016). Thermal effects on the growth and fatty acid composition of four harmful algal bloom species: Possible implications for ichthyotoxicity. Ocean Sci. J. 51, 333–342. doi: 10.1007/s12601-016-0029-5
IPCC (2014). “Climate change 2014: synthesis report,” in Contribution of Working Groups I, II and III to the Fifth Assessment Report of the Intergovernmental Panel on Climate Change. Eds. Pachauri R. K., Meyer L. A. (IPCC, Geneva, Switzerland), 151. Core Writing Team.
IPCC (2021). “Summary for policymakers,” in Climate Change 2021: The Physical Science Basis. Contribution of Working Group I to the Sixth Assessment Report of the Intergovernmental Panel on Climate Change. Eds. Masson-Delmotte V., Zhai P., Pirani A., Connors S. L., Péan C., Berger S., et al. (Cambridge University Press, Cambridge, United Kingdom and New York, NY, USA), 3–32. doi: 10.1017/9781009157896.001
Jacob B. G., Dassow P. V. O. N., Salisbury J. E., Navarro J. M., Vargas C. A. (2017). Impact of low pH/high pCO2 on the physiological response and fatty acid content in diatom Skeletonema pseudocostatum. J. Mar. Biol. Assoc. 97, 225–233. doi: 10.1017/S0025315416001570
Jin P., Gonzàlez G., Agustí S. (2020). Long-term exposure to increasing temperature can offset predicted losses in marine food quality (fatty acids) caused by ocean warming. Evol. Appl. 13, 2497–2506. doi: 10.1111/eva.13059
Jin P., Ji Y., Huang Q., Li P., Pan J., Lu H., et al. (2022). A reduction in metabolism explains the tradeoffs associated with the long-term adaptation of phytoplankton to high CO2 concentrations. New Phytol. 233, 2155–2167. doi: 10.1111/nph.17917
Johnson M. D., Rome M., Stoecker D. K. (2003). Microzooplankton grazing on Prorocentrum minimum and Karlodinium micrum in Chesapeake Bay. Limnol. Oceanogr. 48, 238–248. doi: 10.4319/lo.2003.48.1.0238
Jónasdóttir S. H., Visser A. W., Jespersen C. (2009). Assessing the role of food quality in the production and hatching of Temora longicornis eggs. Mar. Ecol. Prog. Ser. 382, 139–150. doi: 10.3354/meps07985
Kremp A., Godhe A., Egardt J., Dupont S., Suikkanen S., Casabianca S., et al. (2012). Intraspecific variability in the response of bloom-forming marine microalgae to changed climate conditions. Ecol. Evol. 2, 1195–1207. doi: 10.1002/ece3.245
Le Bescot N., Mahé F., Audic S., Dimier C., Garet M.-J., Poulain J., et al. (2016). Global patterns of pelagic dinoflagellate diversity across protist size classes unveiled by metabarcoding. Environ. Microbiol. 18, 609–626. doi: 10.1111/1462-2920.13039
Li A., Stoecker D. K., Coats D. W. (2001). Use of the ‘Food vacuole content’ method to estimate grazing by the mixotrophic dinoflagellate gyrodinium galatheanum on cryptophytes. J. Plankton Res. 23, 303–318. doi: 10.1093/plankt/23.3.303
Li J., Glibert P. M., Gao Y. (2015). Temporal and spatial changes in Chesapeake Bay water quality and relationships to Prorocentrum minimum, Karlodinium veneficum, and CyanoHAB events 1991-2008. Harmful Algae 42, 1–14. doi: 10.1016/j.hal.2014.11.003
Li M., Chen Y., Zhang F., Song Y., Glibert P. M., Stoecker D. K. (2022). A three-dimensional mixotrophic model of Karlodinium veneficum blooms for a eutrophic estuary. Harmful Algae 113, 102203. doi: 10.1016/j.hal.2022.102203
Lin C.-H., Flynn K. J., Mitra A., Glibert P. M. (2018). Simulating effects of variable stoichiometry and temperature on mixotrophy in the harmful dinoflagellate karlodinium veneficum. Front. Mar. Sci. 5. doi: 10.3389/fmars.2018.00320
Lohbeck K. T., Riebesell U., Reusch T. B. H. (2012). Adaptive evolution of a key phytoplankton species to ocean acidification. Nat. Geosci. 5, 346–351. doi: 10.1038/ngeo1441
MacIntyre H. L., Cullen J. J. (2005). “Using cultures to investigate the physiological ecology of microalgae,” in Algal Culturing Techniques. Ed. Andersen R. (Elsevier Academic Press), 287–326.
McLaskey A. K., Keister J. E., Schoo K. L., Olson M. B., Love B. A. (2019). Direct and indirect effects of elevated CO2 are revealed through shifts in phytoplankton, copepod development, and fatty acid accumulation. PloS One 14, e0213931. doi: 10.1371/journal.pone.0213931
Meyers M. T., Cochlan W. P., Carpenter E. J., Kimmerer W. J. (2019). Effect of ocean acidification on the nutritional quality of marine phytoplankton for copepod reproduction. PloS One 14. doi: 10.1371/journal.pone.0217047
Meyers M., Décima M., Law C. S., Gall M., Barr N., Miller M. R., et al. (2022). No evidence of altered relationship between diet and consumer fatty acid composition in a natural plankton community under combined climate drivers. J. Exp. Mar. Bio. Ecol. 551. doi: 10.1016/j.jembe.2022.151734
Nielsen L. T., Jakobsen H. H., Hansen P. J. (2010). High resilience of two coastal plankton communities to twenty-first century seawater acidification: Evidence from microcosm studies. Mar. Biol. Res. 6, 542–555. doi: 10.1080/17451000903476941
O’Donnell D. R., Du Z., Litchman E. (2019). Experimental evolution of phytoplankton fatty acid thermal reaction norms. Evol. Appl. 12, 1201–1211. doi: 10.1111/eva.12798
Pan Y., Meng R., Li Y., Yang L., Mei L., Wu Y., et al. (2023). Changes in biochemical metabolites in manila clam after a temporary culture with high-quality microalgal feed mixed with the dinoflagellate species Karlodinium veneficum and K. zhouanum. Harmful Algae 125, 102422. doi: 10.1016/j.hal.2023.102422
Passow U., Laws E. A. (2015). Ocean acidification as one of multiple stressors: growth response of Thalassiosira weissflogii (diatom) under temperature and light stress. Mar. Ecol. Prog. Ser. 541, 75–90. doi: 10.3354/meps11541
Pierrot D., Lewis E., Wallace D. (2006). MS Excel program developed for CO2 system calculations (Oak Ridge Oak Ridge Natl. Lab. Dioxide Inf. Anal. Cent). Available at: http://cdiac.ornl.gov/oceans/co2rprt.html.
Place A. R., Bowers H. A., Bachvaroff T. R., Adolf J. E., Deeds J. R., Sheng J. (2012). Karlodinium veneficum-The little dinoflagellate with a big bite. Harmful Algae 14, 179–195. doi: 10.1016/j.hal.2011.10.021
R Core Team (2021). R: A language and environment for statistical computing (Vienna, Austria: R Foundation for Statistical Computing). Available at: https://www.R-project.org/.
Rossoll D., Bermúdez R., Hauss H., Schulz K. G., Riebesell U., Sommer U., et al. (2012). Ocean acidification-induced food quality deterioration constrains trophic transfer. PloS One 7, e34737. doi: 10.1371/journal.pone.0034737
Sato N., Tsuzuki M., Kawaguchi A. (2003). Glycerolipid synthesis in Chlorella kessleri 11h - II. 1633 (1), 35–42. doi: 10.1016/S1388-1981(03)00070-2
Schlüter L., Lohbeck K. T., Gröger J. P., Riebesell U., Reusch T. B. (2016). Long-term dynamics of adaptive evolution in a globally important phytoplankton species to ocean acidification. Sci. Adv. 2, e1501660. doi: 10.1126/sciadv.1501660
Schlüter L., Lohbeck K. T., Gutowska M. A., Gröger J. P., Riebesell U., Reusch T. B. H. (2014). Adaptation of a globally important coccolithophore to ocean warming and acidification. Nat. Clim. Change 4, 1024–1030. doi: 10.1038/nclimate2379
Seto D. S., Karp-Boss L., Wells M. L. (2019). Effects of increasing temperature and acidification on the growth and competitive success of Alexandrium catenella from the Gulf of Maine. Harmful Algae 89, 101670. doi: 10.1016/j.hal.2019.101670
Sheppard A. J. (1992). Lipid manual: Methodology suitable for fatty acid-cholesterol analysis (Dubuque, Iowa: Wm. C. Brown Publishers).
Sinensky M. (1974). Homeoviscous adaptation–a homeostatic process that regulates the viscosity of membrane lipids in Escherichia coli. Proc. Natl. Acad. Sci. 71, 522–525. doi: 10.1073/pnas.71.2.522
Solórzano L., Sharp J. H. (1980). Determination of total dissolved phosphorus and particulate phosphorus in natural waters1. Limnol. Oceanogr. 25, 754–758. doi: 10.4319/lo.1980.25.4.0754
Stoecker D. K., Adolf J. E., Place A. R., Glibert P. M., Meritt D. W. (2008). Effects of the dinoflagellates Karlodinium veneficum and Prorocentrum minimum on early life history stages of the eastern oyster (Crassostrea virginica). Mar. Biol. 154, 81–90. doi: 10.1007/s00227-007-0901-z
Sugie K., Yoshimura T. (2016). Effects of high CO2 levels on the ecophysiology of the diatom Thalassiosira weissflogii differ depending on the iron nutritional status. ICES J. Mar. Sci. 73, 680–692. doi: 10.1093/icesjms/fsv259
Tatters A. O., Flewelling L. J., Fu F., Granholm A. A., Hutchins D. A. (2013a). High CO2 promotes the production of paralytic shellfish poisoning toxins by Alexandrium catenella from Southern California waters. Harmful Algae 30, 37–43. doi: 10.1016/j.hal.2013.08.007
Tatters A. O., Roleda M. Y., Schnetzer A., Fu F., Hurd C. L., Boyd P. W., et al. (2013b). Short- and long-term conditioning of a temperate marine diatom community to acidification and warming. Philos. Trans. R. Soc B Biol. Sci. 368, 20120437. doi: 10.1098/rstb.2012.0437
Taucher J., Haunost M., Boxhammer T., Bach L. T., Algueró-Muñiz M., Riebesell U. (2017). Influence of ocean acidification on plankton community structure during a winter-to summer succession: An imaging approach indicates that copepods can benefit from elevated CO2 via indirect food web effects. PloS One 12, e0169737. doi: 10.1371/journal.pone.0169737
Torstensson A., Hedblom M., Andersson J., Andersson M. X., Wulff A. (2013). Synergism between elevated pCO2 and temperature on the Antarctic sea ice diatom Nitzschia lecointei. Biogeosciences 10, 6391–6401. doi: 10.5194/bg-10-6391-2013
Trainer V. L., Moore S. K., Hallegraeff G., Kudela R. M., Clement A., Mardones J. I., et al. (2019). Pelagic harmful algal blooms and climate change: Lessons from nature’s experiments with extremes. Harmful Algae 91, 101591. doi: 10.1016/j.hal.2019.03.009
Van Wychen S., Ramirez K., Laurens L. M. L. (2013). Determination of Total Lipids as Fatty Acid Methyl Esters (FAME) by in situ Transesterification: Laboratory Analytical Procedure (LAP) (Golden, CO: National Renewable Energy Laboratory). NREL/TP-2700-87501
Vidyarathna N. K., Ahn S.H.(., Glibert P. M. (2023). Thermal niche of the dinoflagellate Karlodinium veneficum across different salinity and light levels. J. Plankton Res. 45 (4), 604–613, fbad019. doi: 10.1093/plankt/fbad019
Vidyarathna N. K., Papke E., Coyne K. J., Cohen J. H., Warner M. E. (2020). Functional trait thermal acclimation differs across three species of mid-Atlantic harmful algae. Harmful Algae 94, 101804. doi: 10.1016/j.hal.2020.101804
Waggett R. J., Tester P. A., Place A. R. (2008). Anti-grazing properties of the toxic dinoflagellate Karlodinium veneficum during predator-prey interactions with the copepod Acartia tonsa. Mar. Ecol. Prog. Ser. 366, 31–42. doi: 10.3354/meps07518
Wang T., Tong S., Liu N., Li F., Wells M. L., Gao K. (2017). The fatty acid content of plankton is changing in subtropical coastal waters as a result of OA: Results from a mesocosm study. Mar. Environ. Res. 132, 51–62. doi: 10.1016/j.marenvres.2017.10.010
Wells M. L., Trainer V. L., Smayda T. J., Karlson B. S. O., Trick C. G., Kudela R. M., et al. (2015). Harmful algal blooms and climate change: Learning from the past and present to forecast the future. Harmful Algae 49, 68–93. doi: 10.1016/j.hal.2015.07.009
Welschmeyer N. A. (1994). Fluorometric analysis of chlorophyll a in the presence of chlorophyll b and pheopigments. Limnol. Oceanogr. 39, 1985–1992. doi: 10.4319/lo.1994.39.8.1985
Wilken S., Huisman J., Naus-Wiezer S., Van Donk E. (2013). Mixotrophic organisms become more heterotrophic with rising temperature. Ecol. Lett. 16, 225–233. doi: 10.1111/ele.12033
Wynn-Edwards C., King R., Davidson A., Wright S., Nichols P. D., Wotherspoon S., et al. (2014). Species-specific variations in the nutritional quality of Southern Ocean phytoplankton in response to elevated pCO2. Water 6, 1840–1859. doi: 10.3390/w6061840
Xu D., Zheng G., Brennan G., Wang Z., Jiang T., Sun K., et al. (2023). Plastic responses lead to increased neurotoxin production in the diatom Pseudo-nitzschia under ocean warming and acidification. ISME J. 17, 525–536. doi: 10.1038/s41396-023-01370-8
Yao W., Byrne R. H. (1998). Simplified seawater alkalinity analysis: Use of linear array spectrometers. Deep Sea Res. Part I Oceanogr. Res. Pap. 45, 1383–1392. doi: 10.1016/S0967-0637(98)00018-1
Keywords: ocean acidification, climate change, harmful algae, Karlodinium veneficum, algal toxicity, fatty acids, trophic transfer
Citation: Vidyarathna NK, Smith LE, Miller KR, Coyne KJ, Cohen JH and Warner ME (2024) Short-term and long-term exposure to combined elevated temperature and CO2 leads to differential growth, toxicity, and fatty acid profiles in the harmful dinoflagellate Karlodinium veneficum. Front. Mar. Sci. 11:1305495. doi: 10.3389/fmars.2024.1305495
Received: 01 October 2023; Accepted: 26 March 2024;
Published: 09 April 2024.
Edited by:
Haruko Kurihara, University of the Ryukyus, JapanReviewed by:
Matthew John Harke, Gloucester Marine Genomics Institute (GMGI), United StatesMichael William Lomas, Bigelow Laboratory For Ocean Sciences, United States
Hisashi Endo, Kyoto University, Japan
Copyright © 2024 Vidyarathna, Smith, Miller, Coyne, Cohen and Warner. This is an open-access article distributed under the terms of the Creative Commons Attribution License (CC BY). The use, distribution or reproduction in other forums is permitted, provided the original author(s) and the copyright owner(s) are credited and that the original publication in this journal is cited, in accordance with accepted academic practice. No use, distribution or reproduction is permitted which does not comply with these terms.
*Correspondence: Mark E. Warner, mwarner@udel.edu