Changes in growth, morphology, and levels of digestive enzymes and growth-related hormones in early ontogeny of black scraper, Thamnaconus modestus
- 1School of Ocean, Yantai University, Yantai, China
- 2Guangxi Academy of Marine Sciences, Guangxi Academy of Sciences, Nanning, China
- 3Comprehensive Utilization Center og Marine Resource , Institute of Beibu Gulf Marine Industry, Fangchenggang, China
- 4Marine Fish Research Center, Yantai Beizhiyuan Biotechnology Co., Ltd., Yantai, China
- 5Marine Fisheries Department, Jincheng People’s Government of Laizhou, Yantai, China
Introduction: The black scraper, Thamnaconus modestus, is a highly valued marine fish species, but its output has gradually decreased in recent years, which may be due to its low survival rate during early ontogenesis.
Methods: Therefore, in this study, we assessed the changes in growth, morphology, digestive enzymes, and hormone levels in T. modestus from 0–60 days post-hatching (dph) and revealed growth turning points by morphological measurement and determination of digestive enzyme activities and hormone levels. We found that ontogenesis could be divided into the larval (0–20 dph) and juvenile (20–60 dph) stages. Acid and alkaline protease activity significantly increased and decreased, respectively, from 12–25 dph, likely due to the development of stomach and gastric glands. Acid phosphatase levels significantly increased at 0 and 4 dph, which may be related to the regulation of metabolism and immune protection. A sharp increase in alkaline phosphatase levels at 20 and 25 dph was observed and was likely due to the development of the brush border membrane of enterocytes. The amylase level was significantly higher at 25, 30, and 35 dph, possibly due to better digestion and absorption during the transition from consuming Artemia to compound feed. In newly hatched larvae, the level of thyroid hormones triiodothyronine (T3) and thyroxine (T4) gradually increased and peaked at 35 dph, highlighting the importance of these hormones during the development of T. modestus. Growth hormone (GH) levels first increased from 0–8 dph, with a plateau at 8–20 dph, and then increased at 25–30–35 dph. For insulin-like growth factor 1 (IGF-1), a significant increase with a subsequent plateau was observed between 8 and 20 dph, followed by a substantial decrease between 30 and 35 dph. These results suggest that the regulating functions of GH and IGF-1 are synchronised. Digestive enzyme activity and hormone levels of abnormal fry at 30 dph were lower than those of normal fish, highlighting the importance of specific hormones, especially T4 and IGF-1, in the development of T. modestus.
Highlights
● Changes in growth and morphological development were observed.
● Growth turning points were observed at 0–60 days post-hatching.
● Digestive enzymes and growth-related hormone levels were measured.
● T4 and IGF-1 play crucial roles in fish development.
1 Introduction
The black scraper, Thamnaconus modestus (Monacanthidae: Tetraodontiformes), is widely distributed in southern China, the coastal waters of South Korea, and Hokkaido of Japan, and is a highly valued marine fish (Kim, 2007; Mizuno et al., 2012; Kim et al., 2013; Ying et al., 2022). It inhabits a depth range of 50–110 m within a temperature range of 10–28°C and feeds primarily on plankton and benthic organisms (Choi et al., 2002; Kim, 2007). However, recently, the wild-catch output of black scraper has been decreasing, with approximately 122,258 tons caught in 2022 (Wang and Wu, 2023). Furthermore, due to overexploitation, increasing demand, and limited biological information, this amount has gradually decreased, and it has been listed on the International Union for Conservation of Nature Red List of Threatened Species (IUCN, 2023). To increase the output, great efforts have been made to promote the technological development of artificial reproduction and seedling production globally (Arcand-Hoy and Benson, 1998; Kawase, 2002; Mizuno et al., 2012; Kim et al., 2013).
Digestive enzymes are reliable indicators of the nutritional condition of individuals because of their specificity to age and species, sensitivity, and short latency (Lazo et al., 2011). Proteolytic pancreatic enzymes and phosphatase are used as reliable indicators of condition (Martínez-Lagos et al., 2014). Therefore, a detailed knowledge of the changes associated with food ingestion, digestion, and assimilation during development is essential for understanding the nutritional physiology of larval fish (Martinez et al., 1999; Zambonino-Infante et al., 2008). For example, it was reported that feasibility of early weaning of senegal sole, Solea senegalensis and larval co-feeding enhances growth and survival after weaning (Canavate and Fernández-Dıaz, 1999). In Pikeperch, Sander lucioperca, the co-feeding weaning strategy for 7 days from 15 to 22 dph should be followed for successful larviculture (Ljubobratović et al., 2015). Therefore, the use of live preys and weaning is important on the survival rates of larval fish.
Previous studies used the activity and levels of different digestive enzymes as indicators of the development and survival rates of larval fish (Ueberschär, 1993). Fish are poikilothermic and vary considerably in their feeding habits and temperature preferences; thus, their digestive enzymes, such as acid protease (ACP) and alkaline protease (AKP; El-Beltagy et al., 2004), are diverse. Fish proteases have been studied since the 1940s, and an alkaline protease was extracted from the intestine of Nile tilapia, Oreochromis niloticus (Bezerra et al., 2005). Phosphatases are a group of enzymes involved in nutritional processes, mineralisation, intestinal transport and hydrolysis of phosphorylated proteins (Letelier et al., 1985). Acid phosphatase (ACPP) and alkaline phosphatase (AKPP), are present in various animals and play catalytic roles under acidic and alkaline conditions, participating in the transfer and metabolism of phosphate groups. ACPP is an important hydrolytic enzyme, primarily located in the lysosomal and endometrial systems of animals (Pipe, 1990) and is involved in intracellular digestion and immune-related activities. Amylase and lipase are essential enzymes in cellular metabolism in plants, animals, and microorganisms (Champasri et al., 2021). Amylase produced by the intestinal microflora plays a vital role in starch digestion in freshwater fish (Sugita et al., 1997). In milkfish, Chanos chanos, intestinal and pancreatic lipases are physiologically versatile for lipid digestion (Borlongan, 1990). These results indicate that these digestive enzymes are essential for the growth and development of fish.
Thyroid hormones, growth hormone (GH), and insulin-like growth factor 1 (IGF-1) play crucial roles in the development of larval fish (Power et al., 2000; Santos et al., 2002). Thyroid hormones, including triiodothyronine (T3) and thyroxine (T4), produced by the thyroid gland, are essential mediators of vertebrate development, metamorphosis, metabolism, homeostasis, cellular proliferation, and differentiation (Power et al., 2001). GH is a pituitary hormone that regulates vertebrate and somatic growth (Velez and Unniappan, 2021). The liver produces IGF-1 under the control of GH, is structurally related to insulin, and contains three intramolecular disulphide bonds (Moriyama et al., 2000). GH regulates growth by promoting the production and expression of IGF-1, which is widely distributed in various body tissues and a key factor in the biological function of GH. The GH/IGF axis plays a vital role in the growth and development of fish (Yang et al., 1999; Gabillard et al., 2003; Uchida et al., 2003; Revol et al., 2005).
This is the first study to introduce the changes in digestive enzymes and growth-related hormones during early ontogeny in the black scraper. Therefore, our study aimed to investigate changes in growth conditions and individual development, as well as digestive enzymes and growth-related hormones in this species. First, larvae’s morphology and related ontogenetic characteristics were observed and described from 0 to 60 dph. Second, digestive enzymes, including ACP, AKP, ACPP, AKPP, amylase, and lipase, were measured from 0 to 35 dph. Finally, growth-related hormones T3, T4, GH, and IGF-1 levels were measured. The results will provide a scientific basis for the breeding, growth, and nutritional requirements in the early ontogeny of T. modestus.
2 Materials and methods
2.1 Experimental materials
The experiment was conducted at Laizhou Shunchang Fisheries Co., Ltd. in China. Wild adult black scrapers between 2 and 3 years old were collected from the Yellow Sea and reared in incubation ponds (length × width × height, 6.0 × 5.0 × 1.0 m, respectively). The broodstock naturally spawned and fertilised in the pool after implementing reproductive control measures, such as artificial domestication, water temperature regulation, and nutrient enhancement. The fertilised eggs (spherical and viscous) were collected and incubated for 40–44 h under a salinity of 32–33, pH 7.0–8.0, and continuous micro-inflation. The water temperature was 22.0 ± 0.5°C for the rearing of early larvae, after which the temperature was gradually raised to 24.0 ± 0.5°C for the rearing of the juveniles. Seawater was subjected to secondary sand filtration during the larval rearing. The rearing pool was maintained under natural light during the day, and the lights were turned off at night. After hatching, the larvae were fed a series of different feeds including SS-type Brachionus plicatilis (rotifers) (5–11 days post-hatching, [dph]), L-type rotifer (9–22 dph), Artemia nauplii (19–32 dph), A. adults (30–37 dph), and artificial compound feed (32–40 dph) as their growth progressed. The wet weight of the rotifer was used in this experiment.
2.2 Experimental method
2.2.1 Measurement of growth index
Three spawning ponds containing 50–70 thousand larvae were used as the experimental ponds. Specimens were sampled daily from 0–20 dph, every 2 d from 20–40 dph, and every 5 d from 40– 60 dph. Ten individuals were randomly selected from each pond (n = 30 per sampling period). The specimens were anaesthetised with MS-222 and observed under a dissecting microscope (XTL-6745TJ1-720HD, Suzhou Beitejia Optoelectronic Technology Co., Ltd., Jiangsu, China). The total length (TL, mm), body height (BH, mm), head length (HL, mm), body length (BL, mm), pre-anal length (PL, mm), snout length (SL, mm), and eye orbit diameter (ED, mm) were measured using Vimage 2014 software as shown in Figure 1. The body weight (BW, g) was measured using a precision electronic balance (SI-114, Denver Instrument, Bohemia, New York, USA).
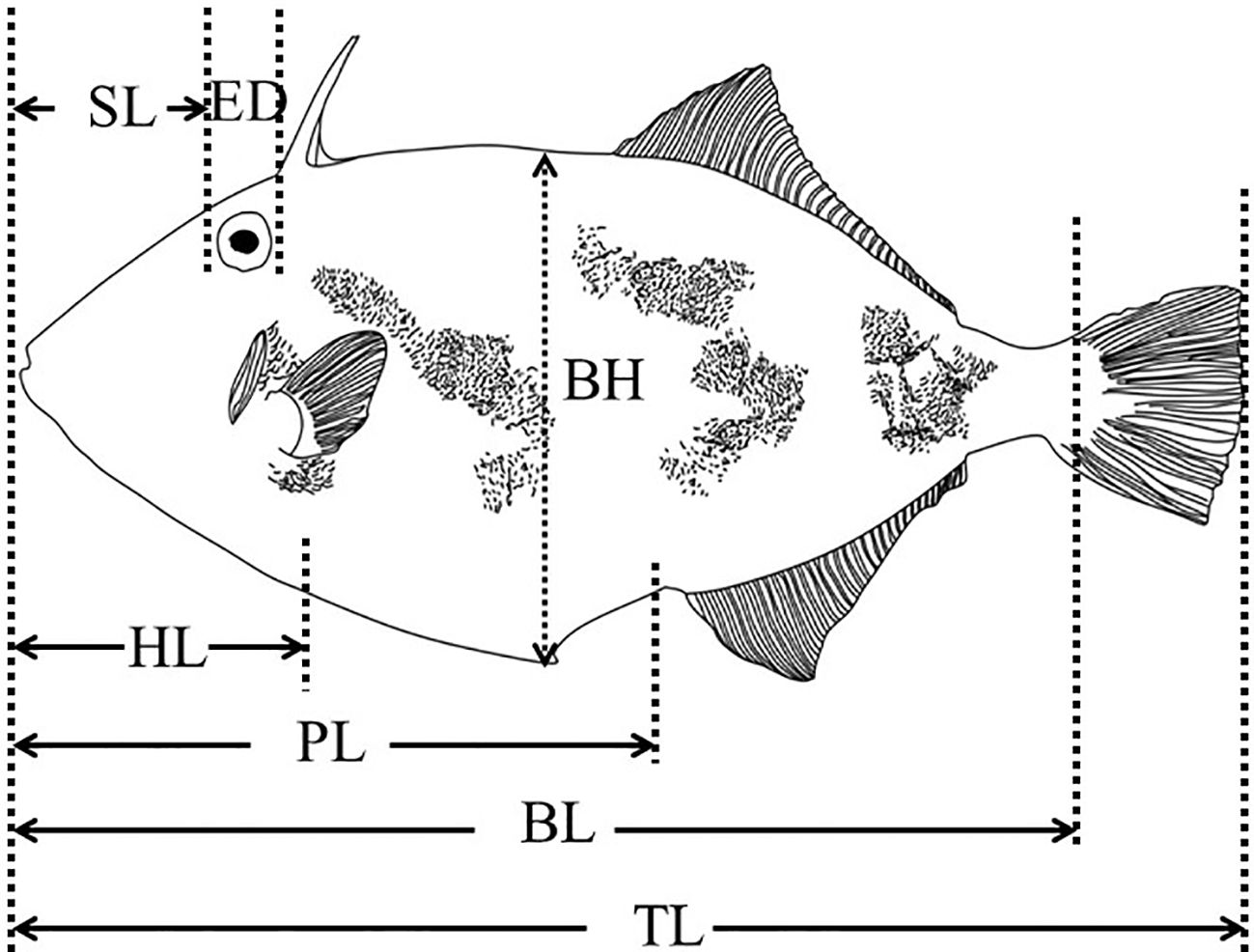
Figure 1 Indicator diagram of growth index measurement of black scraper. TL, total length; BL, body length; PL, pre-anal length; HL, head length; SL, Snout length; ED, Eye orbit diameter; BH, body height.
2.2.2 Sampling procedure
The study was conducted in accordance with the protocols of the Animal Care and Use Committee of Yantai University, China (Permit Number 20170605). At 30 and 35 dph, the abdomen of each specimen was cut, mixed with physiological saline, and homogenised in an ice water bath. After centrifugation at 10,000 × g for 10 min at 4°C, the supernatant was collected and stored at –80°C for later analysis of various digestive enzymes and hormone levels. The entire body of each specimen was sampled and measured at 0–25 dph because the size was too small.
During growth and development, a few fish exhibited abnormal metamorphic characteristics at 30 dph, such as body rollover, loss of balance, and impaired swimming ability. Therefore, experiments were conducted to compare the differences in digestive enzyme activity and hormone levels between the abnormal and normal fish.
2.2.3 Measurement of digestive enzyme activities
At 0–25 dph, the fish were homogenised. At 30 and 35 dph, the abdomens of fish were cut, mixed with physiological saline, and homogenised in an ice water bath. Subsequently, the mixture was centrifugated 2500 × g for 10 min at 4°C, and the supernatant was collected for later analysis of activity measurement of various digestive enzyme activity.
ACP and AKP activities were measured using acidic and alkaline proteinase assay kits (Shanghai Jiwei Biotechnology Co., Ltd., Shanghai, China), respectively. Casein was hydrolysed at 37°C every minute to produce 1 μg tyrosine, the unit of measurement for ACP activity. The method to measure AKP activity is the same as that for ACP; however, the buffer solution is a borax-sodium hydroxide solution with 9.8 pH.
The activities of the other digestive enzymes were measured according to the manufacturer’s instructions (Nanjing Jiancheng Bioengineering Institute). The activity of ACPP and AKPP was measured using acid and alkaline phosphatase assay kits (Shanghai Jiwei Biotechnology Co., Ltd., Shanghai, China), respectively. One gram of tissue protein reacts with the substrate for 30 min or 15 min at 37°C to produce 1 mg phenol, which produces one unit of ACPP or AKPP activity.
Amylase and lipase activities were measured using amylase and lipase assay kits (Shanghai Jiwei Biotechnology Co., Ltd., Shanghai, China), respectively. One milligram of protein in the tissue reacts with the substrate for 30 min at 37°C to hydrolyse 10 mg of starch, which produces one unit of amylase activity. Furthermore, each gram of tissue protein interacts with the substrate for 1 min at 37°C, producing 1 μmol substrate (one King unit of lipase).
2.2.4 Determination of hormone levels
T3, T4, GH, and IGF-1 levels were measured using fish enzyme-linked immunosorbent assay kits (Wuhan Huamei Biological Co., Ltd., Wuhan, China), and absorbance was measured using a microplate reader (Bio-Rad Laboratories, Inc., Hercules, CA, USA) at the Beijing Huaying Biotechnology Institute.
2.3 Statistical analysis
All data were analysed using SPSS 23.0 software (IBM, Armonk, NY, USA). The diagram was plotted using Excel or OriginPro 9.1 software (OriginLab, Northampton, MA, USA). All data are reported as the means ± standard deviation (SD). The normality and homoscedasticity of the continuous data was tested using Shapiro–Wilk tests. Differences between independent samples were assessed using a one-way analysis of variance with Tukey’s Honestly Significant Difference (HSD) test. All results with P < 0.05 were considered statistically significant.
3 Results
3.1 Morphological changes from 0–60 dph
Morphological changes during the development of black scrapers are shown in Figure 2. The characteristics of the larvae, juveniles, and adult fish during each developmental period are described below.
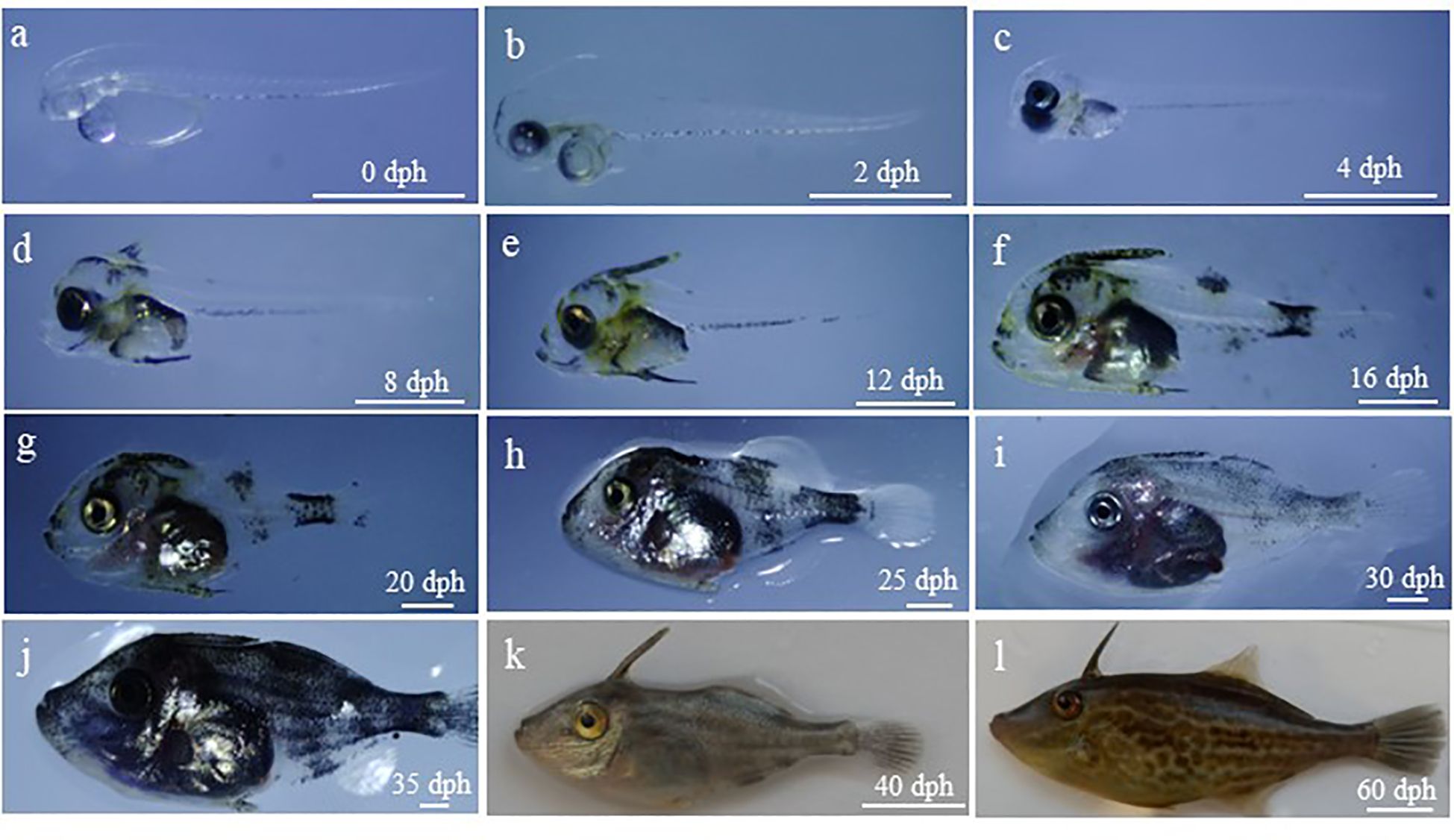
Figure 2 Morphologic changes of larval, juvenile and young black scraper. The age and total length was as follows: (A), 0 dph, 2.10 ± 0.11 mm; (B), 2 dph, 2.50 ± 0.08 mm; (C), 4 dph, 2.55 ± 0.07 mm; (D), 8 dph, 2.96 ± 0.17 mm; (E), 12 dph, 3.71 ± 0.21 mm; (F), 16 dph, 5.29 ± 0.63 mm; (G), 20 dph, 7.43 ± 0.80 mm; (H), 25 dph, 12.85 ± 1.68 mm; (I), 30dph, 19.33 ± 4.60 mm; (J), 35 dph, 24.41 ± 5.90 mm; (K), 40 dph, 38.83 ± 3.28 mm; (L), 60 dph, 63.71 ± 5.89 mm. dph, days post hatching. Scale bar = 1 mm (A-J), 1 cm (K, L).
3.1.1 Larval stage
0 dph: Larvae have a yolk sac and oil globule. Visual sac and crystal are colourless and transparent, and otoliths are clear. The digestive tract, located attached above the yolk sac, is unopened, and the anus is not perforated. The anal fin membrane extends backwards from the anus, and the dorsal fin membrane begins above the middle of the yolk sac. The dorsal and gluteal fins are connected to the caudal fin membrane without pigment distribution. There is a linear array of melanin on the ventral side of each sarcomere. Furthermore, the larvae exhibit the behavioural characteristics of rapidly straightening out and distributing themselves evenly in the pool, and they intermittently move between different water layers (Figure 2A).
2 dph: Yolk sac and oil globules shrink, and the brain protuberates upward. The mouth is not yet properly formed. The pectoral fin membrane is inverted and triangular, and the digestive tract thickens. Their swimming ability improves, and some larvae can consume SS-rotifers (Figure 2B).
4 dph: The colour of visceral mass deepens, and a few star-shaped pigments appear on top of the head. The spine primordium of the dorsal fin appears, and the primordium of the swim bladder appears but is not inflated. The mouth crack enlarges, and most larvae can start ingesting food (Figure 2C).
8 dph: The proportion of head to body increases, and the dorsal fin spine appears at the back of the head. Many melanin and yellow pigments are distributed on the dorsal fin, head, and torso. The swim bladder begins to inflate. Most larvae can now feed on L-rotifers (Figure 2D).
12 dph: The head proportion, pre-anal length and body height increase. Yellow and melanin pigments are widely distributed throughout the body surface, and their colour is deepened. The dorsal fin spines thicken and ride up. The swim bladder is inflated and enlarged (Figure 2E).
16 dph: Larvae have leathery skin and protruding scales on the body surface. Barbs appear on the spine of the dorsal fin, and fin differentiation occurs in the dorsal, gluteal, and pectoral fins. Melanocytes surround the abdomen, almost covering the first half part of the caudal stalk. The growth rate accelerates, and digestion and feeding abilities are enhanced. It can feed on little A. nauplii. The larvae are distributed in the upper layer of the water body and areas with suitable light intensity (Figure 2F).
20 dph: The velvety fine scale is distributed on the surface of larvae. The body height increases, with the end of notochord slightly upturned, and the caudal fin strips differentiate from the lower part of rear. The chrysanthemum-like melanin is concentrated on the top of the skull, dorsal fin spine, abdomen, and caudal peduncle, and dot-like yellow pigments are distributed throughout the body. The larvae could be fully fed on the A. nauplii (Figure 2G).
3.1.2 Juvenile stage
25 dph: The head is round and blunt, and the mouth is covered with leathery skin. The dorsal and gluteal fins are differentiated (Figure 2H).
30 dph: The body surface is covered with coarse and fine scales, and the dorsal, gluteal, caudal, and pectoral fins are well developed. A large amount of melanin is distributed throughout the body. Juveniles are predatory and may consume A. nauplii and little compound feed. Juveniles have negative phototaxis and prefer to gather in dark places (Figure 2I).
35 dph: The juvenile morphology resembles the adult fish, with elongated snout and increased distance between mouth and eyes. There are pigments distributed throughout the body, and the melanin is denser. Juveniles respond and move quickly, consume much food, and have habit of killing each other. The feed is mainly compound feed, and little A. nauplii is eaten (Figure 2J).
40 dph: The juvenile morphology is consistent with the adult fish, with a small mouth and well-developed incisors. Each fin is well developed, with scales all over the body, and adult pigments are dominant. Horizontal dark lines appear in the trunk. Melanin and uranidin are distributed on the body surface (Figure 2K).
60 dph: The fish body is narrower and elongates, and the body height growth is not obvious. The trunk elongates. The total length ratio to body height increases, and the length of dorsal, pectoral, gluteal, and caudal fins increase. Fish swim quickly and may consume much granular compound feed or fresh bait such as Artemia (Figure 2L).
3.2 Changes in growth index from 0–60 dph
The growth rate (assessed as change in body weight [BW] over time) was slow before 20 dph, increased from 20–40 dph, and increased again from 40–60 dph (Figure 3A). Furthermore, the growth rate of body length (BL) pre-anal length (PL), and snout length (SL) was slow before 20 dph, accelerated from 20–36 or 38 dph, and increased again from 36 or 38–60 dph (Figures 3B–D). Similarly, the growth rate of body height (BH) and head length (HL) increased at 18 or 24 dph and increased again from 40–60 dph (Figures 3E, F). The total length (TL) and eye orbit diameter (ED) accelerated at 18 or 20 dph (Figures 3G, H). Therefore, we speculated that there are two growth turning points for BW, BL, PL, SL, BH, and HL, with the first turning point occurring at 18, 20, or 24 dph and the second at 36, 38, or 40 dph. Furthermore, we speculated that the two turning points appeared at 18 or 20 dph for TL and ED.
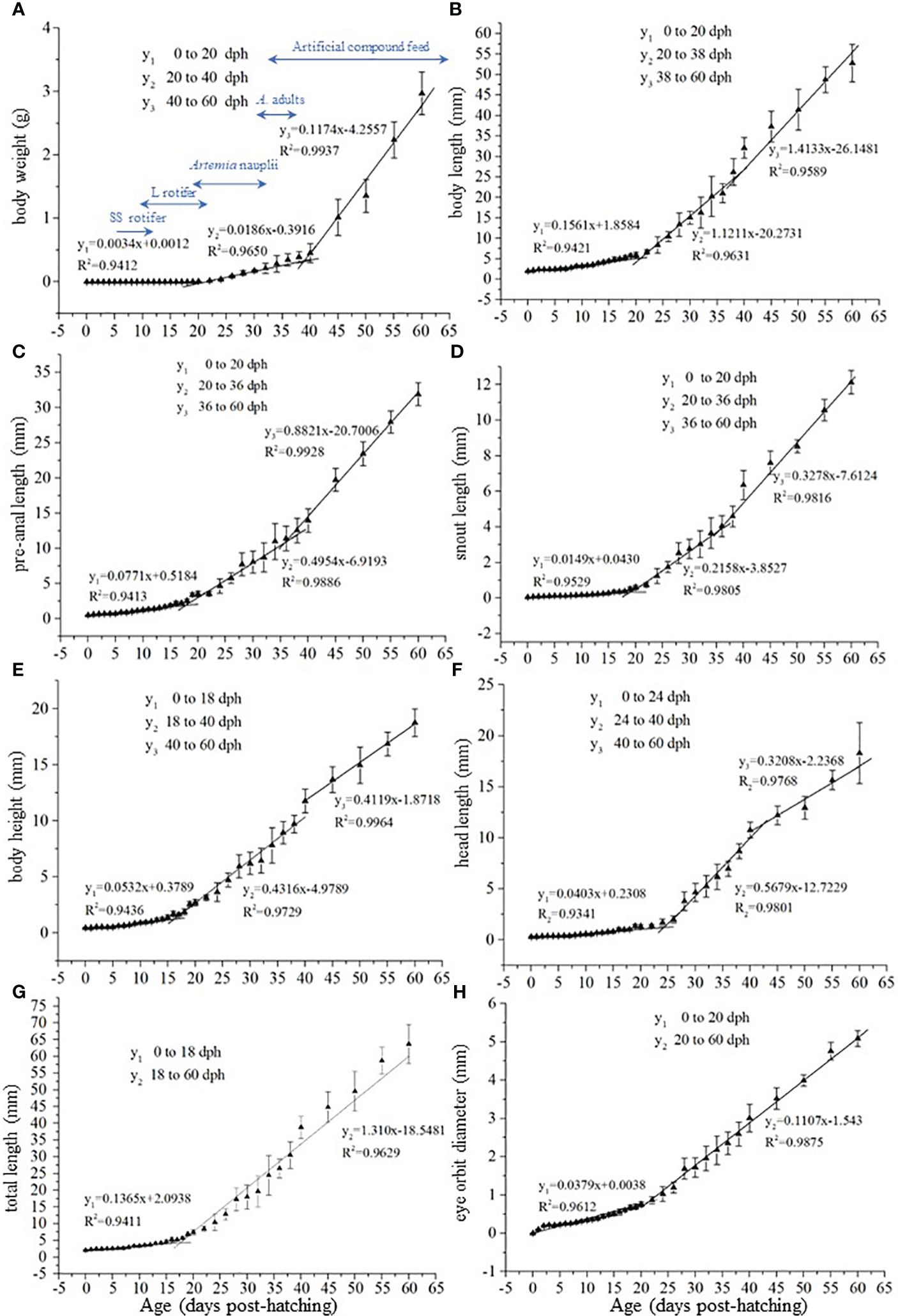
Figure 3 Changes in growth index from 0 to 60 dph in black scraper. In the formula y=kx+b, x represents the age, y represents the growth indicator, b represents the intercept, and k represents the slope.
3.3 Changes in the digestive enzyme activities from 0–35 dph
ACP levels were significantly higher at 4 and 25 dph than at other ages except at 20 dph (P< 0.05) and were significantly higher at 20 dph than at 0, 8, 12, 30, and 35 dph (P < 0.05; Figure 4A). AKP levels were significantly higher at 8, 12, and 16 dph than at the other ages (P < 0.05, Figure 4B). ACPP levels at 0 and 4 dph and AKPP levels at 4, 20, and 25 dph were significantly higher than those at other ages (P < 0.05; Figures 4C, D). Amylase levels were significantly higher at 4, 25, 30, and 35 dph than that at other ages (P < 0.05, Figure 4E). Lipase levels were significantly higher at 4 and 16 dph than at 8, 12, 30, and 35 dph (P < 0.05, Figure 4F).
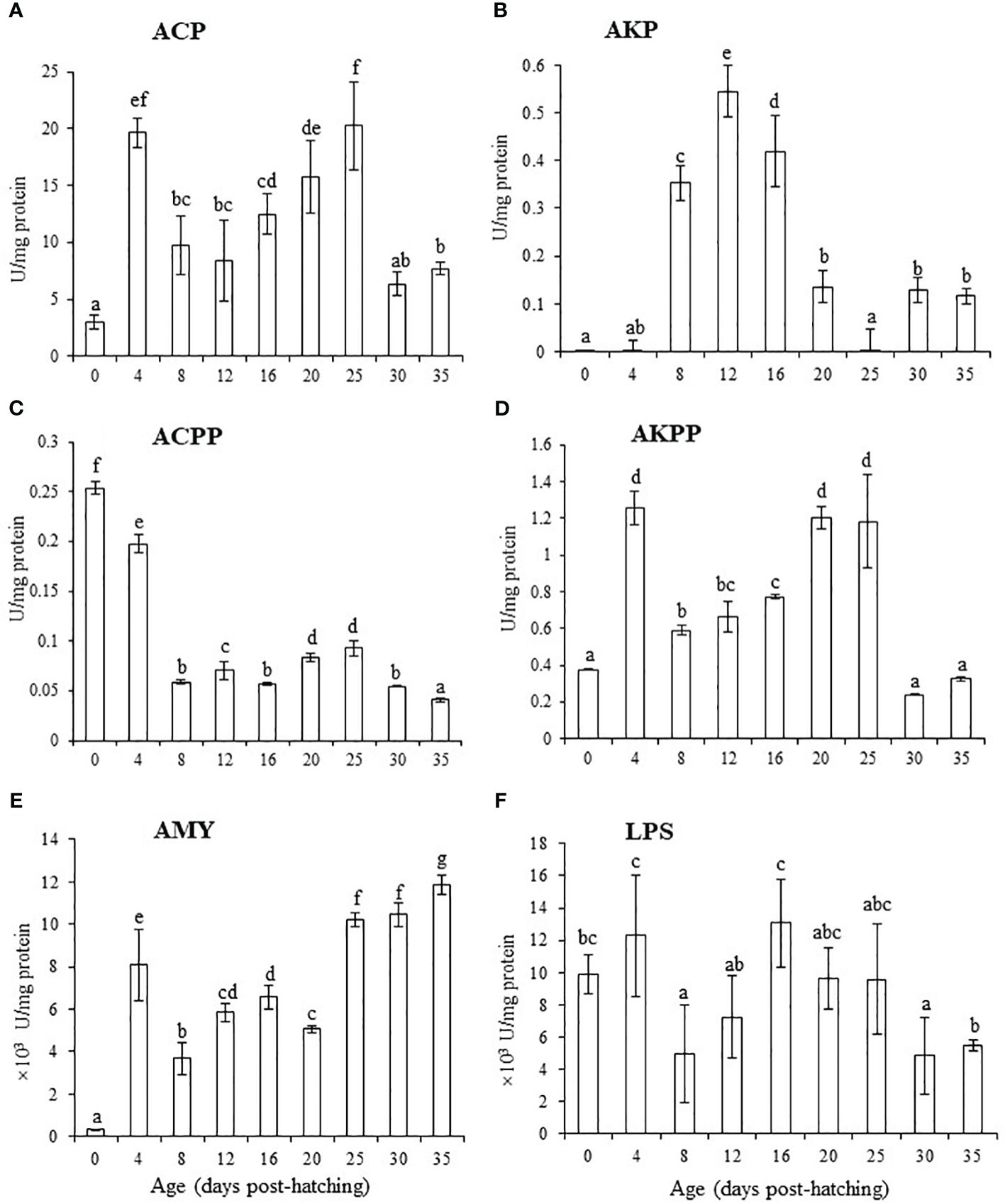
Figure 4 Changes in levels of digestive enzymes from 0 to 35 dph in black scraper. Results are expressed as means ± SD (n = 30). Different lowercase letters indicate significant differences among different ages. ACP, acid protease; AKP, alkaline protease; ACPP, acid phosphatases; AKPP, alkaline phosphatases; AMY, amylase; LPS, lipase.
3.4 Changes in the growth-related hormones from 0–35 dph
The T3 level gradually significantly increased from 0 to 8 dph, with a plateau at 12–30 dph, and then significantly increased at 35 dph (P < 0.05, Figure 5A). T4 levels gradually increased from 0–25 dph and increased significantly at 25, 30, and 35 dph compared with that at other ages (P < 0.05, Figure 5B). GH levels increased from 0–8 dph, with a plateau at 8–20 dph, and then increased again for 25–30–35 dph (Figure 5C). IGF-1 levels increased considerably with a plateau between 8–20 dph and then decreased considerably at 30 and 35 dph (Figure 5D).
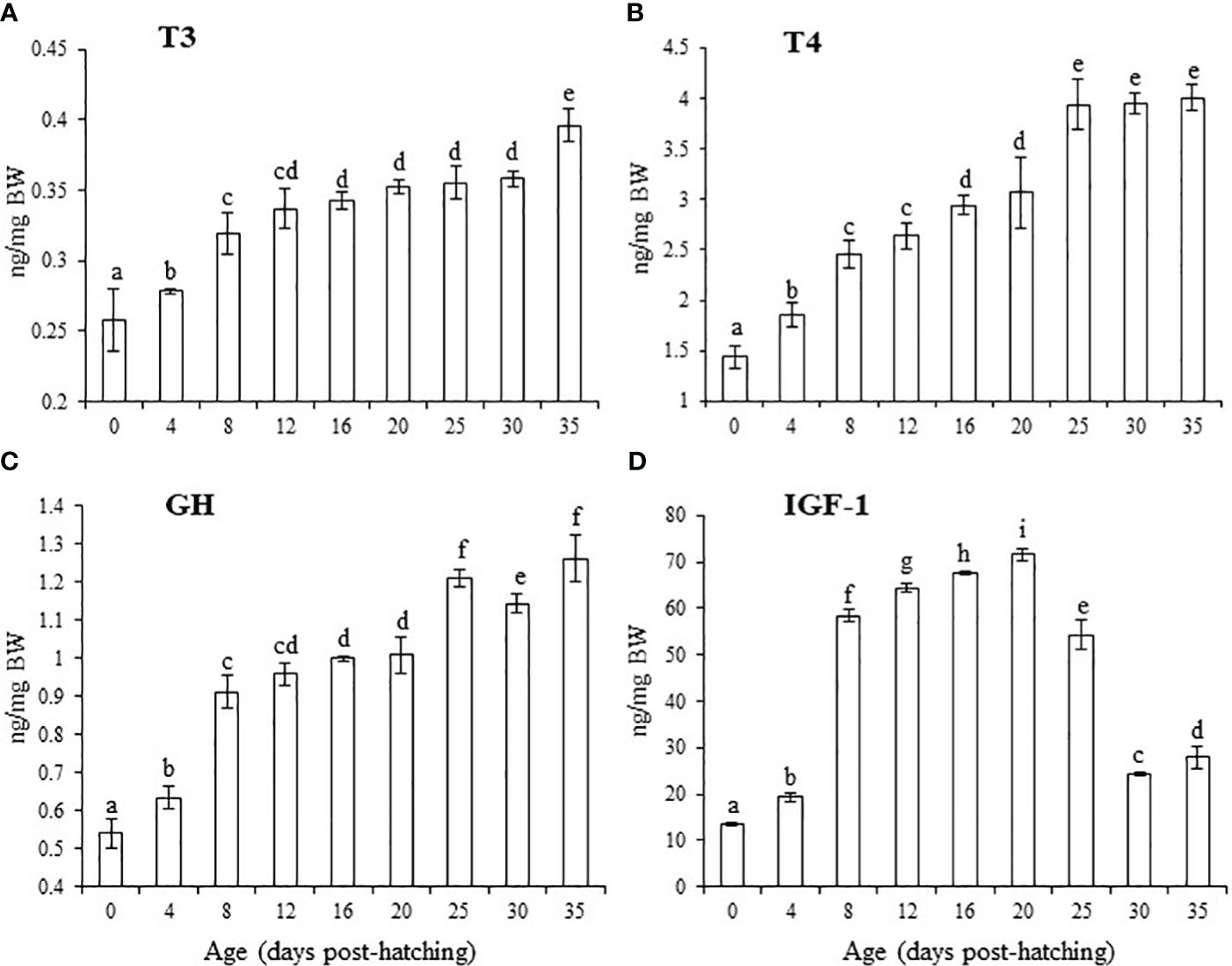
Figure 5 Changes in levels of growth-related hormones from 0 to 35 dph in black scraper. Results are expressed as means ± SD (n = 30). Different lowercase letters indicate significant differences among different ages. T3, triiodothyronine; T4, thyroxine; GH, growth hormone; IGF-1, insulin-like growth factor-1.
3.5 Comparison of digestive enzyme activities and hormone levels between normal and abnormal fish at 30 dph
No differences were observed in the activities of the six digestive enzymes between normal and abnormal fish at 30 dph (Figure 6A). However, T4 and IGF-1 levels in normal fish were significantly higher than those in abnormal fish (P < 0.05, Figure 6B).
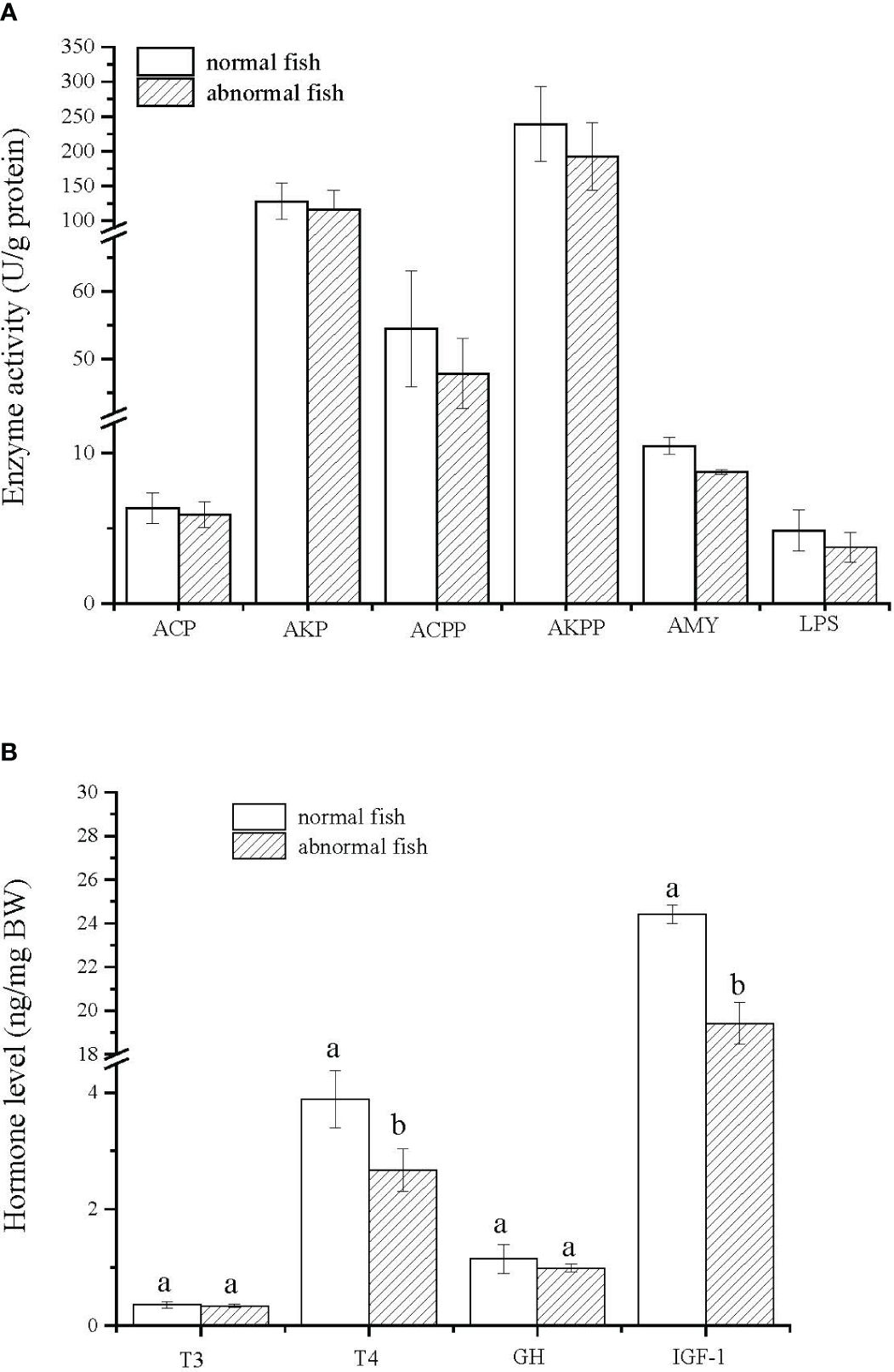
Figure 6 Changes in levels of digestive enzyme and growth-related hormones between normal and metamorphosized abnormal fish at 30 dph. Results are expressed as means ± SD (n = 30). Different lowercase letters indicate significant differences between two groups. ACP, acid protease; AKP, alkaline protease; ACPP, acid phosphatases; AKPP, alkaline phosphatases; AMY, amylase; LPS, lipase; T3, triiodothyronine; T4, thyroxine; GH, growth hormone; IGF-1, insulin-like growth factor-1.
4 Discussion
4.1 Growth characteristics in the early stages of Thamnaconus modestus
Previous studies showed that the growth rates of teleosts vary at different developmental stages, indicating that stages characterise the growth rate and are primarily expressed by growth turning points in the early stages, such as in Limanda yokohamae (Günther, 1877) (Fukuhara, 1988; Hopkins, 1992; Houde, 1997). In the thick-lipped grey mullet, Chelon labrosus, morphometric growth distinguished three distinct developmental stages separated by two morphometric lengths, with the first inflection point at 14 dph and the second at 25 dph (Ben Khemis et al., 2013). In the present study, there were two turning points in growth, as indicated by BW and TL values, from 0–60 dph. The first turning point appeared at 20 dph as showed by BW, BL, PL and SL. Similar results were observed by Guan et al. (2013), who also observed a growth turning point for the TL of T. modestus at 20 dph. This phenomenon may be related to changes in the morphology and diet of larval fish, with a more efficient digestive system formed at this stage (Guan et al., 2013). The fish calibre increased, and the digestive system gradually developed after 20 dph. The fry could swallow and digest the A. nauplii; thus, nutrition increased, and their growth rate accelerated. The second turning point appeared at 40 dph for BW, BH, and HL, and at 36 dph for PL and SL; this may be due to the consumption of compound feed at approximately 36 dph, as it contains more abundant nutrients than A. nauplii and is suitable for rapid growth.
4.2 Changes in activities of the main digestive enzymes of T. modestus
With the formation of digestive organs and glands in the early developmental stage of fish, the digestive enzymes secreted in the body undergo specific changes according various stages of growth (Buddington, 1985; Govoni et al., 1986). Thus, changes in digestive enzyme activity in the body may be caused by individual growth and digestive organ development (Comabella et al., 2006), but also changes in feed types (Morais et al., 2004), and changes in enzyme expression caused by juvenile metabolism (Alvarez-González et al., 2008). In the present study, the two critical periods for quantitative changes in digestive enzyme activity were the transition period from endogenous to exogenous nutrition in fry and the transition period from fry to juveniles (Cousin et al., 1987). Furthermore, the activities of ACP, AKP, ACPP, AKPP, amylase, and lipase were detected in the newly hatched larvae, as reported in the common snook, Centropomus undecimalis, with all digestive enzymatic activities detected during larviculture (Jimenez-Martinez et al., 2012), suggesting that the larvae can digest food when it transitions from endogenous to exogenous nutrition. In the red drum, Sciaenops ocellatus, digestive enzymes for proteins, lipids and amylase were present in the larvae, and their activity subsequently increased with age and length (Lazo et al., 2007). In our study, the larvae start feeding on the third day after hatching; therefore, juvenile digestive enzyme activity may be related to gene expression from endogenous nutrition, not food intake.
In the present study, ACP levels were much lower than those of AKP from 0–20 dph. Similar results were obtained for seabass, Lates calcarifer (Walford and Lam, 1993). For the AKP levels, they significantly increased from 0–12 dph, peaked at 12 dph, and significantly decreased at 16 dph. Similar results have been reported for Eurasian perch, Perca fluviatilis (Cuvier-Péres and Kestemont, 2001), Persian sturgeon, Acipenser persicus (Babaei et al., 2011), and sea bass, Dicentrarchus labrax (Infante and Cahu, 1994). These results suggest that AKP activity first increased and then decreased to finally reach a stable state, a common feature during the individual development of vertebrates and an essential step in the process of metamorphosis. Concerning ACP levels, they significantly increased at 4 dph in T. modestus. This increase may be due to the fact that larvae were fed on rotifers, as it was shown that the ACP activity in the bodies of folded armyworm rotifers was five times higher than that of AKP (Wethmar and Kleinow, 1993); and thus they consumed exogenous enzymes. In the common carp, Cyprinus carpio, increased AKP activity can be related to the adaptation of larvae to digest protein content in the food (Farhoudi et al., 2013). Most studies suggest that as gastric function gradually develops and improves, ACP activity increases, and AKP activity generally decreases (Ribeiro et al., 1999; Comabella et al., 2006; Alvarez-González et al., 2008). These results are similar to those observed in our study. From 12–25 dph, the ACP and AKP levels increased and decreased, respectively, which may be because the stomach gradually develops at approximately 12 dph, the gastric glands may appear and it is known that protein digestion mainly depends on acidic digestion in the stomach. However, the specific time at which gastric glands appear in T. modestus requires further study.
In the present study, ACPP activity significantly increased from hatching. Both acid and alkaline phosphatase activities have been detected during larval development in different marine fishes (Cahu and Infante, 1994; Moyano et al., 1996). In California halibut, Paralichthys californicus, acid phosphatases have been detected in the early stages of development, although ACPP levels were higher at hatching and during yolk sac absorption (Alvarez-González et al., 2005). Once yolk-sac reserves were depleted, acid phosphatase activity decreased below alkaline phosphatase activity levels in this species. In white seabream, Diplodus sargus, ACPP-specific activity increased in this species throughout larval development, peaking at 20 dph (Guerreiro et al., 2010). Similar changes were observed in the senegal sole (Martinez et al., 1999) and bay snook, Petenia splendida (Uscanga-Martínez et al., 2011); this may be because the hatched larvae directly contact the external environment and lose the protection of the egg membrane; thus, higher ACPP activity is required to regulate physiological metabolism and immune protection. Indeed, intestinal AKPP are involved in the absorption of nutrients, such as lipids, glucose, calcium, and inorganic phosphate (Roubaty and Portmann, 1988; Dupuis et al., 1991; Mahmood et al., 1994; Tengjaroenkul et al., 2000). In Senegal sole, AKPP activity decreased from 5–20 dph but subsequently showed a sharp increase, possibly linked to the development of enterocytes (Martinez et al., 1999). In California halibut, AKPP was detected at maximal activity levels at 4 dph, decreasing after a few days and increasing again until the second maximum activity at 15 dph (Alvarez-González et al., 2005). In the present study in T. modestus, AKPP activity significantly increased at 4 dph, and ACPP activity increased significantly at 0 and 4 dph, indicating the significance of intestinal absorption of these two phosphatases. A sharp increase in AKPP activity was detected at 20 and 25 dph, which may reflect the development of the brush border membrane of enterocytes and, hence, an increase in the relevance of the absorptive processes mediated by these enzymes in T. modestus.
In the present study, amylase levels were significantly higher at 4 dph then decreased until 20 dph. A previous study in turbot, Scophthalmus maximus, the authors proposed that this may be due to the consumption of Artemia; therefore, a change in the feeding pattern should result in a significant reduction in amylase activity (Cousin et al., 1987). At 4 dph, the transition period from endogenous to exogenous nutrition suggests that the black scraper could absorb carbohydrates after the full development of the mouth opening; then, it gradually decreased as the larvae developed until 20 dph. However, in seabass larvae, the initial high levels of amylase at 4 dph did not seem to be food induced (enriched rotifers), and may be better explained by programmed gene expression (Infante and Cahu, 2001). Furthermore, we found that amylase levels were significantly higher at 25, 30, and 35 dph, the transition period from Artemia as the food source to compound feed. In the leopard grouper, Mycteroperca rosacea, amylase levels increased significantly between 30 and 40 dph, suggesting that larvae could be successfully weaned from micro-diets during this period (Martínez-Lagos et al., 2014). In the present study, the increase in amylase activity at 30 and 35 dph was likely influenced by the carbohydrate content of the administered compound diet, which contained carbohydrates derived from yeast extract included in the formulation. Similar amylase patterns, reported in other studies, are thought to be influenced by the carbohydrates in compound diets (Cara et al., 2003; Zouiten et al., 2008).
Lipase activity was detected throughout the early developmental stages in our study, and similar results were found for California halibut (Alvarez-González et al., 2005) and common dentex, Dentex dentex (Gisbert et al., 2009). Furthermore, it was previously reported that lipase was actively found in 4 dph T. modestus larvae (Gwak and Lee, 2009). However, lipase activity was detected until 13 dph in the Mayan cichlid, Cichlasoma urophthalmus (López-Ramírez et al., 2011) and until 20 dph in turbot (Cousin et al., 1987). In spotted sand bass, lipase activity was detected immediately after mouth opening, gradually increased until 15 dph, and remained stable until the end of the study period (Alvarez-González et al., 2008). In Japanese flounder, P. olivaceus, neutral lipase activity showed substantial fluctuations in larvae during the first few days (Bolasina et al., 2006). In the present study, lipase activity was significantly higher at 4 and 16 dph. Studies showed that early larvae contain two types of lipases, phosphatase A2 being involved in the digestion of fat in the yolk sac, whereas lipase is involved in fat digestion by the intestinal epithelium (Oozeki and Bailey, 1995; Izquierdo et al., 2000). In the present study, the unstable changes in lipase activity after the first feeding of juveniles may have been caused by the mutual conversion of the two types of lipases, while the decrease in lipase activity in the later stage may have been due to the rich protein and low-fat content in the feed. In the Miiuy croaker (Miichthys miiuy) larvae, amylase and lipase activities can be modulated by feeding modes (Shan et al., 2009). In addition, the pattern of digestive enzyme activity was related to organogenesis and the type of food used at different developmental stages in blackspot seabream, Pagellus bogaraveo (Ribeiro et al., 2008). Therefore, in the present study, the changes in these enzyme activities may be related to the type of bait, the digestive system’s degree of development and the different growth stages. If we understand the changes in digestive enzyme activities during growth and development, it can be used as a reference for improving the weaning process for the larviculture of T. modestus in the future.
4.3 Changes in hormone levels in the early-life stages of T. modestus
The ontogeny of thyroid hormones is related to specific morphological changes such as fins, eyes, and stomach, which characterized of early development in the gilthead seabream, Sparus aurata (Szisch et al., 2005). Significant levels of T3 and T4 were found in the eggs of 26 species of various freshwater, marine, and diadromous teleosts, suggesting that thyroid hormones play crucial roles in egg development (Tagawa et al., 1990b). In the present study, T3 and T4 were detected at relatively low levels in the newly hatched larvae of T. modestus. Similar results have been observed for Japanese flounder (Miwa et al., 1988) and black rockfish, Sebastes schlegelii (Chin et al., 2010). Furthermore, de Jesus et al. (1991) detected T3 and T4 in newly fertilised eggs of the Japanese flounder, suggesting a synergistic action of T3 and T4 during metamorphosis in the flounder. It is reported that the higher concentrations of T3 than T4 and the sharp decrease in T3 concentration immediately after hatching indicates a primary role of T3 in the early development of the flounder embryos and larvae (Tagawa et al., 1990a). In the present study, the low concentration of thyroid hormones in newly hatched larvae may be related to the low level of thyroid development and the consumption or short maintenance time of thyroid hormones (primarily maternal) during embryonic development. Furthermore, the T3 and T4 levels showed an increasing trend with growth and development. Similar results were reported in Spotted halibut, Verasper variegatus, and T4 levels increased gradually during the early metamorphic stage, reaching peak levels at the metamorphosis climax (Hotta, 2001). In silver sea bream Sparus sarba larvae, T3 and T4 were detected at 1 dph, and both increased as development progressed with a distinct increase between 21 and 35 dph (Deane and Woo, 2003b). These results are consistent with those of the present study. In our study, T4 levels were higher than that of T3 at every stage. Similar results have been observed in rabbitfish, Siganus guttatus (Ayson and Lam, 1993), spotted grouper, Epinephelus tauvina (Leatherland et al., 1990), and Atlantic halibut (Einarsdóttir et al., 2006); this may be due to the presence of 5’-monodeiodinase in the body, which converts a portion of T4 into T3 through deiodination in the peripheral tissues. However, there is insufficient evidence to confirm this hypothesis here.
GH and IGF-I play important roles as modulators of development, growth, and reproduction in teleosts (Funes et al., 2006). A study in orange-spotted grouper E. coioides showed that gh mRNA expression was detected in 1 dph larvae, and gh present in eggs and larvae may play a vital role in the early development, especially during the metamorphosis of its larvae (Li et al., 2005). Similarly, transcripts for IGF-I were also detected throughout development in unfertilised eggs, embryos, and larvae in gilthead seabream, suggesting that IGF-I may play a role during the early development of teleosts (Perrot et al., 1999). In the present study, the GH levels gradually increased from 0–8 dph, with a plateau at 8–20 dph, and then a second increase for 25–30–35 dph; and IGF-1 levels showed a large increase with a plateau observed between 8–20 dph and then a large decrease at 30 and 35 dph. Similarly, GH abundance reached a constant and high level in the silver sea bream from 35 dph onward. In contrast, IGF-I level peaked at 35 dph and then significantly decreased (Deane et al., 2003a). The highest amounts of gh mRNA were observed at 25 and 50 dph in Siberian sturgeon, Acipenser baerii larvae (Abdolahnejad et al., 2015). Furthermore, the IGF-1 level was much higher than that of GH during development in the present study. These results suggest that the function of GH in promoting fish growth is closely related to IGF-1, and that the two hormones are synchronised in promoting the growth and development of fish. However, differently, GH and IGF-1 mRNA levels showed approximately inverse expression patterns in the thick-lipped grey mullet (Gilannejad et al., 2020), suggesting that the changes of GH and IGF-1 may be species-specific. In the present study, IGF-1 at 25 dph and GH at 30 dph were significantly decreased, possibly because the juveniles were in a critical metamorphosis stage. Study also shows that fish secrete more thyroid hormones during metamorphosis to adapt to this physiological change and reduce the synthesis and secretion of GH (Shiao and Hwang, 2006; Campinho, 2019).
4.4 Comparison of enzyme activity and hormone content between normal and abnormal fish
Many studies showed that digestive enzyme activity and hormone content change during the transition from larvae to juveniles (metamorphosis) (Blaxter, 1988). In senegal sole, the activities of ACPP, AKPP, and other digestive enzymes decreased during metamorphosis (Martinez et al., 1999). In the present study, digestive enzyme activity in abnormal T. modestus fish was lower than in normal fish, suggesting that most digestive enzymes have low activity during metamorphosis. However, they play an indispensable role in the physiological functions of metamorphosis.
Several studies have shown that the thyroid-growth hormone axis plays an important role in regulating metamorphosis in fish. In orange-spotted grouper, E. coioides, a higher dose of thyroid hormones was appropriate for acceleration of metamorphosis and improvement of survival in 3- and 4-week-old larvae (de Jesus et al., 1998). Furthermore, exogenous thyroid hormone induces premature differentiation of the pectoral fins and accelerates the growth of the pelvic fins in zebrafish (Brown, 1997). In the present study, the levels of T4 and IGF-1 in abnormal fish were significantly lower than those in normal fish. Similar results were observed in Atlantic halibut. Between normally and abnormally metamorphosing larvae, IGF-I content was reduced in the abnormal fish, indicating that IGF-I either has a regulatory role in metamorphosis, or is affected by abnormal metamorphosis (Hildahl et al., 2007). In tongue sole, Cynoglossus semilaevis, IGF-1 plays an important role in the physiological functions related to metamorphosis (Duan, 1998). These results suggest that T4 and IGF-1 play important roles in the normal development of T. modestus.
5 Conclusion
We conclude that (1) growth turning points of T. modestus appeared with changes in growth and development; (2) as growth and development progressed, the levels of various digestive enzymes changed considerably, which may be related to the type of bait, the digestive systems’ degree of development, and different growth stages of T. modestus; and (3) four hormones, especially T4 and IGF-1, play important roles in fish metamorphosis. Digestive enzyme and growth hormone activities are important during growth and development. Since the output and quality of T. modestus are gradually decreasing, further study is essential to understand the relationships between digestive enzymes, growth hormones, weaning, and other factors, such as notochordal or standard lengths, aimed at cultivating the larvae better.
Data availability statement
The raw data supporting the conclusions of this article will be made available by the authors, without undue reservation.
Ethics statement
The animal study was approved by the Animal Care and Use Committee of Yantai University, China (Permit Number 20170605). The study was conducted in accordance with the local legislation and institutional requirements.
Author contributions
LL: Conceptualization, Project administration, Writing – original draft. JZ: Data curation, Funding acquisition, Methodology, Writing – original draft. ZZ: Data curation, Software, Writing – original draft. JW: Investigation, Writing – review & editing. WM: Resources, Visualization, Writing – original draft. CW: Data curation, Validation, Writing – original draft. ZL: Formal analysis, Writing – original draft. WX: Supervision, Writing – original draft, Writing – review & editing.
Funding
The author(s) declare financial support was received for the research, authorship, and/or publication of this article. This work was supported by Shandong Province Modern Agriculture Industry Technology System Fish Industry Innovation Team (SDAIT-12-03), Yantai Science and Technology Innovation Development Plan Project (2022XDRH022), and Lianyungang City Key research and development Plan (Social Development, SF2304) in China.
Acknowledgments
We would like to thank Editage (www.editage.cn) for English language editing.
Conflict of interest
Author CW was employed by the company Yantai Beizhiyuan Biotechnology Co.
The remaining authors declare that the research was conducted in the absence of any commercial or financial relationships that could be construed as a potential conflict of interest.
Publisher’s note
All claims expressed in this article are solely those of the authors and do not necessarily represent those of their affiliated organizations, or those of the publisher, the editors and the reviewers. Any product that may be evaluated in this article, or claim that may be made by its manufacturer, is not guaranteed or endorsed by the publisher.
References
Abdolahnejad Z., Pourkazemi M., Khoshkholgh M. R., Yarmohammadi M. (2015). Expression of growth hormone gene during early development of Siberian sturgeon (Acipenser baerii). Mol. Biol. Res. Commun. 4, 181–188.
Alvarez-González C. A., Cervantes-Trujano M., Tovar-Ramírez D., Conklin D. E., Nolasco H., Gisbert E., et al. (2005). Development of digestive enzymes in California halibut Paralichthys californicus larvae. Fish Physiol. Biochem. 31, 83–93. doi: 10.1007/s10695-006-0003-8
Alvarez-González C. A., Moyano-López F. J., Civera-Cerecedo R., Carrasco-Chávez V., Ortiz-Galindo J. L., Dumas S. (2008). Development of digestive enzyme activity in larvae of spotted sand bass Paralabrax maculatofasciatus. 1. Biochemical analysis. Fish Physiol. Biochem. 34, 373–384. doi: 10.1007/s10695-007-9197-7
Arcand-Hoy L. D., Benson W. H. (1998). Fish reproduction: an ecologically relevant indicator of endocrine disruption. Environ. Toxicol. Chemist.: Int. J. 17, 49–57. doi: 10.1002/etc.5620170108
Ayson F. G., Lam T. J. (1993). Thyroxine injection of female rabbitfish (Siganus guttatus) broodstock: changes in thyroid hormone levels in plasma, eggs, and yolk-sac larvae, and its effect on larval growth and surviva. Aquaculture 109, 83–93. doi: 10.1016/0044-8486(93)90488-K
Babaei S. S., Kenari A. A., Nazari R., Gisbert E. (2011). Developmental changes of digestive enzymes in Persian sturgeon (Acipenser persicus) during larval ontogeny. Aquaculture 318, 138–144. doi: 10.1016/j.aquaculture.2011.04.032
Ben Khemis I., Gisbert E., Alcaraz C., Zouiten D., Besbes R., Zouiten A., et al. (2013). Allometric growth patterns and development in larvae and juveniles of thick-lipped grey mullet Chelon labrosus reared in mesocosm conditions. Aquacult. Res. 44, 1872–1888. doi: 10.1111/are.2013.44.issue-12
Bezerra R. S., Lins E. J., Alencar R. B., Paiva P. M., Chaves M. E., Coelho L. C., et al. (2005). Alkaline proteinase from intestine of Nile tilapia (Oreochromis niloticus). Process Biochem. 40, 1829–1834. doi: 10.1016/j.procbio.2004.06.066
Blaxter J. H. S. (1988). 1 Pattern and variety in development. Fish Physiol. 11, 1–58. doi: 10.1016/S1546-5098(08)60198-3
Bolasina S., Pérez A., Yamashita Y. (2006). Digestive enzymes activity during ontogenetic development and effect of starvation in Japanese flounder, Paralichthys olivaceus. Aquaculture 252, 503–515. doi: 10.1016/j.aquaculture.2005.07.015
Borlongan I. G. (1990). Studies on the digestive lipases of milkfish, Chanos chanos. Aquaculture 89, 315–325. doi: 10.1016/0044-8486(90)90135-A
Brown D. D. (1997). The role of thyroid hormone in zebrafish and axolotl development. Proc. Natl. Acad. Sci. 94, 13011–13016. doi: 10.1073/pnas.94.24.13011
Buddington R. K. (1985). Digestive secretions of lake sturgeon, Acipenser fulvescens, during early development. J. Fish Biol. 26, 715–723. doi: 10.1111/j.1095-8649.1985.tb04311.x
Cahu C. L., Infante J. L. Z. (1994). Early weaning of sea bass (Dicentrarchus labrax) larvae with a compound diet: effect on digestive enzymes. Comp. Biochem. Physiol. Part A: Physiol. 109, 213–222. doi: 10.1016/0300-9629(94)90123-6
Campinho M. A. (2019). Teleost metamorphosis: the role of thyroid hormone. Front. Endocrinol. 10. doi: 10.3389/fendo.2019.00383
Canavate J. P., Fernández-Dıaz C. (1999). Influence of co-feeding larvae with live and inert diets on weaning the sole Solea Senegalensis onto commercial dry feeds. Aquaculture 174, 255–263. doi: 10.1016/S0044-8486(99)00021-6
Cara J. B., Moyano F. J., Cárdenas S., Fernández-Díaz C., Yúfera M. (2003). Assessment of digestive enzyme activities during larval development of white bream. J. Fish Biol. 63, 48–58. doi: 10.1046/j.1095-8649.2003.00120.x
Champasri C., Phetlum S., Pornchoo C. (2021). Diverse activities and biochemical properties of amylase and proteases from six freshwater fish species. Sci. Rep. 11, 5727. doi: 10.1038/s41598-021-85258-7
Chin B. S., Nakagawa M., Tagawa M., Masuda R., Yamashita Y. (2010). Ontogenetic changes of habitat selection and thyroid hormone levels in black rockfish (Sebastes schlegelii) reared in captivity. Ichthyol. Res. 57, 278–285. doi: 10.1007/s10228-010-0165-3
Comabella Y., Mendoza R., Aguilera C., Carrillo O., Hurtado A., García-Galano T. (2006). Digestive enzyme activity during early larval development of the Cuban gar Atractosteus tristoechus. Fish Physiol. Biochem. 32, 147–157. doi: 10.1007/s10695-006-0007-4
Cousin J. C. B., Baudin-Laurencin F., Gabaudan J. (1987). Ontogeny of enzymatic activities in fed and fasting turbot, Scophthalmus maximus L. J. Fish Biol. 30, 15–33. doi: 10.1111/j.1095-8649.1987.tb05728.x
Cuvier-Péres A., Kestemont P. (2001). Development of some digestive enzymes in Eurasian perch larvae Perca Fluviatilis. Fish Physiol. Biochem. 24, 279–285. doi: 10.1023/A:1015033300526
Deane E. E., Kelly S. P., Collins P. M., Woo N. Y. S. (2003a). Larval development of silver sea bream (Sparus sarba): Ontogeny of RNA-DNA ratio, GH, IGF-I, and Na+-K+-ATPase. Mar. Biotechnol. 5, 79–91. doi: 10.1007/s10126-002-0052-7
Deane E. E., Woo N. Y. (2003b). Ontogeny of thyroid hormones, cortisol, hsp70 and hsp90 during silver sea bream larval development. Life Sci. 72, 805–818. doi: 10.1016/S0024-3205(02)02334-2
de Jesus E. G., Hirano T., Inui Y. (1991). Changes in cortisol and thyroid hormone concentrations during early development and metamorphosis in the Japanese flounder, Paralichthys olivaceus. Gen. Comp. Endocrinol. 82, 369–376. doi: 10.1016/0016-6480(91)90312-T
de Jesus E. G. T., Toledo J. D., Simpas M. S. (1998). Thyroid hormones promote early metamorphosis in grouper (Epinephelus coioides) larvae. Gen. Comp. Endocrinol. 112, 10–16. doi: 10.1006/gcen.1998.7103
Duan C. (1998). Nutritional and developmental regulation of insulin-like growth factors in fish. J. Nutr. 128, 306S–314S. doi: 10.1093/jn/128.2.306S
Dupuis Y., Tardivel S., Porembska Z., Fournier P. (1991). Effect of some alkaline phosphatase inhibitors on intestinal calcium transfer. Int. J. Biochem. 23, 175–180. doi: 10.1016/0020-711X(91)90186-Q
Einarsdóttir I. E., Silva N., Power D. M., Smaradottir H., Björnsson B. T. (2006). Thyroid and pituitary gland development from hatching through metamorphosis of a teleost flatfish, the Atlantic halibut. Anat. embryol. 211, 47–60. doi: 10.1007/s00429-005-0055-z
El-Beltagy A. E., El-Adawy T. A., Rahma E. H., El-Bedawey A. A. (2004). Purification and characterization of an acidic protease from the viscera of bolti fish (Tilapia nilotica). Food Chem. 86, 33–39. doi: 10.1016/j.foodchem.2003.08.009
Farhoudi A., Kenari A. A., Nazari R. M., Makhdoomi C. H. (2013). Changes of digestive enzymes activity in common carp (Cyprinus carpio) during larval ontogeny. Iran. J. Fish. Sci. 12, 320–334. doi: 10.22092/IJFS.2018.114280
Fukuhara O. (1988). Morphological and functional development of larval and juvenile Limanda yokohamae (Pisces: Pleuronectidae) reared in the laboratory. Mar. Biol. 99, 271–281. doi: 10.1007/BF00391990
Funes V., Asensio E., Ponce M., Infante C., Cañavate J. P., ManChado M. (2006). Insulin-like growth factors I and II in the sole Solea Senegalensis: cDNA cloning and quantitation of gene expression in tissues and during larval development. Gen. Comp. Endocrinol. 149, 166–172. doi: 10.1016/j.ygcen.2006.05.017
Gabillard J. C., Rescan P. Y., Fauconneau B., Weil C., Le Bail P. Y. (2003). Effect of temperature on gene expression of the Gh/Igf system during embryonic development in rainbow trout (Oncorhynchus mykiss). J. Exp. Zool. Part A: Comp. Exp. Biol. 298, 134–142. doi: 10.1002/jez.a.10280
Gilannejad N., de Las Heras V., Martos-Sitcha J. A., Moyano F. J., Yúfera M., Martínez-Rodríguez G. (2020). Ontogeny of Expression and Activity of Digestive Enzymes and Establishment of gh/igf1 Axis in the Omnivorous Fish Chelon labrosus. Animals 10, 874. doi: 10.3390/ani10050874
Gisbert E., Giménez G., Fernández I., Kotzamanis Y., Estévez A. (2009). Development of digestive enzymes in common dentex Dentex dentex during early ontogeny. Aquaculture 287, 381–387. doi: 10.1016/j.aquaculture.2008.10.039
Govoni J. J., Boehlert G. W., Watanabe Y. (1986). The physiology of digestion in fish larvae. Environ. Biol. Fish. 16, 59–77. doi: 10.1007/BF00005160
Guan J., Ma Z., Zheng Y., Guan S., Li C., Liu H. (2013). Breeding and larval rearing of bluefin leatherjacket, Thamnaconus modestus (Gunther 1877) under commercial scales. Int. J. Aquacult. 3, 55–62. doi: 10.5376/ija.2013.03.0012
Guerreiro I., de Vareilles M., Pousão-Ferreira P., Rodrigues V., Dinis M. T., Ribeiro L. (2010). Effect of age-at-weaning on digestive capacity of white seabream (Diplodus sargus). Aquaculture 300, 194–205. doi: 10.1016/j.aquaculture.2009.11.019
Gwak W. S., Lee S. G. (2009). Developmental changes in digestive organ and digestive enzyme activity of Filefish Thamnaconus modestus. Korean J. Ichthyol. 21, 149–157.
Hildahl J., Sweeney G., Galay-Burgos M., Einarsdóttir I. E., Björnsson B. T. (2007). Cloning of Atlantic halibut growth hormone receptor genes and quantitative gene expression during metamorphosis. Gen. Comp. Endocrinol. 151, 143–152. doi: 10.1016/j.ygcen.2006.10.003
Hopkins K. D. (1992). Reporting fish growth: A review of the basics 1. J. World aquacult. Soc. 23, 173–179. doi: 10.1111/j.1749-7345.1992.tb00766.x
Hotta Y. (2001). Development of the digestive system and metamorphosis relating hormones in spotted halibut larvae and early juveniles. Nippon suisan gakkaishi 67, 40–48. doi: 10.2331/suisan.67.40
Houde E. D. (1997). Patterns and trends in larval-stage growth and mortality of teleost fish. J. Fish Biol. 51, 52–83. doi: 10.1111/j.1095-8649.1997.tb06093.x
Infante J. Z., Cahu C. L. (1994). Influence of diet on pepsin and some pancreatic enzymes in sea bass (Dicentrarchus labrax) larvae. Comp. Biochem. Physiol. Part A: Physiol. 109, 209–212. doi: 10.1016/0300-9629(94)90122-8
Infante J. Z., Cahu C. L. (2001). Ontogeny of the gastrointestinal tract of marine fish larvae. Comp. Biochem. Physiol. Part C: Toxicol. Pharmacol. 130, 477–487. doi: 10.1016/S1532-0456(01)00274-5
IUCN. (2023). The IUCN Red List of Threatened Species. Version 2022-2. Available online at: https://www.iucnredlist.org.
Izquierdo M. S., Socorro J., Arantzamendi L., Hernández-Cruz C. M. (2000). Recent advances in lipid nutrition in fish larvae. Fish Physiol. Biochem. 22, 97–107. doi: 10.1023/A:1007810506259
Jimenez-Martinez L. D., Alvarez-González C. A., Tovar-Ramírez D., Gaxiola G., Sanchez-Zamora A., Moyano F. J., et al. (2012). Digestive enzyme activities during early ontogeny in Common snook (Centropomus undecimalis). Fish Physiol. Biochem. 38, 441–454. doi: 10.1007/s10695-011-9525-9
Kawase K. (2002). Simplicity and diversity in the reproductive ecology of triggerfish (Balistidae) and filefish (Monacanthidae). Fish. Sci. 68, 119–122. doi: 10.2331/fishsci.68.sup1_119
Kim H. R., Choi J. H., Park W. G. (2013). Vertical distribution and feeding ecology of the black scraper, Thamnaconus modestus, in the Southern Sea of Korea. Turkish J. Fish. Aquat. Sci. 13, 249–259. doi: 10.4194/1303-2712-v13_2_07
Lazo J. P., Darias M. J., Gisbert E. (2011). Larval Fish Nutrition, (Edited by G. Joan Holt. ) Section 1, Ontogeny of the digestive tract. Larval Fish Nutrition, 3–46. doi: 10.1002/9780470959862.ch1
Lazo J. P., Mendoza R., Holt G. J., Aguilera C., Arnold C. R. (2007). Characterization of digestive enzymes during larval development of red drum (Sciaenops ocellatus). Aquaculture 265, 194–205. doi: 10.1016/j.aquaculture.2007.01.043
Leatherland J. F., Reddy P. K., Yong A. N., Leatherland A., Lam T. J. (1990). Hepatic 5′-monodeiodinase activity in teleosts in vitro: A survey of thirty-three species. Fish Physiol. Biochem. 8, 1–10. doi: 10.1007/BF00004426
Letelier M. E., Repetto Y., Aldunate Y., Morello A. (1985). Acid and alkaline phosphatase activity in Trypanosoma cruzi epimastigotes. Comp. Biochem. Physiol. Part B: Comp. Biochem. 81, 47–51. doi: 10.1016/0305-0491(85)90159-2
Li W. S., Chen D., Wong A. O., Lin H. R. (2005). Molecular cloning, tissue distribution, and ontogeny of mRNA expression of growth hormone in orange-spotted grouper (Epinephelus coioides). Gen. Comp. Endocrinol. 144, 78–89. doi: 10.1016/j.ygcen.2005.04.018
Ljubobratović U., Kucska B., Feledi T., Poleksić V., Marković Z., Lenhardt M., et al. (2015). Effect of weaning strategies on growth and survival of pikeperch, Sander lucioperca, larvae. Turkish J. Fish. Aquat. Sci. 15, 325–331. doi: 10.4194/1303-2712-v15_2_15
López-Ramírez G., Cuenca-Soria C. A., Alvarez-González C. A., Tovar-Ramírez D., Ortiz-Galindo J. L., Perales-García N., et al. (2011). Development of digestive enzymes in larvae of Mayan cichlid Cichlasoma urophthalmus. Fish Physiol. Biochem. 37, 197–208. doi: 10.1007/s10695-010-9431-6
Mahmood A., Yamagishi F., Eliakim R., DeSchryver-Kecskemeti K., Gramlich T. L., Alpers D. H. (1994). Apossible role for rat intestinal surfactant-like particles in transepithelial triacylglycerol transport. J. Clin. Invest. 93, 70–80. doi: 10.1172/JCI116986
Martinez I., Moyano F. J., Fernández-Díaz C., Yúfera M. (1999). Digestive enzyme activity during larval development of the Senegal sole (Solea Senegalensis). Fish Physiol. Biochem. 21, 317–323. doi: 10.1023/A:1007802708459
Martínez-Lagos R., Tovar-Ramírez D., Gracia-López V., Lazo J. P. (2014). Changes in digestive enzyme activities during larval development of leopard grouper (Mycteroperca rosacea). Fish Physiol. Biochem. 40, 773–785. doi: 10.1007/s10695-013-9884-5
Miwa S., Tagawa M., Inui Y., Hirano T. (1988). Thyroxine surge in metamorphosing flounder larvae. Gen. Comp. Endocrinol. 70, 158–163. doi: 10.1016/0016-6480(88)90105-0
Mizuno K., Shimizu-Yamaguchi S., Miura C., Miura T. (2012). Method for efficiently obtaining fertilized eggs from the black scraper Thamnaconus modestus by natural spawning in captivity. Fish. Sci. 78, 1059–1064. doi: 10.1007/s12562-012-0527-z
Morais S., Cahu C., Zambonino-Infante J. L., Robin J., Rønnestad I., Dinis M. T., et al. (2004). Dietary TAG source and level affect performance and lipase expression in larval sea bass (Dicentrarchus labrax). Lipids 39, 449–458. doi: 10.1007/s11745-004-1250-2
Moriyama S., Ayson F. G., Kawauchi H. (2000). Growth regulation by insulin-like growth factor-I in fish. Biosci. Biotechnol. Biochem. 64, 1553–1562. doi: 10.1271/bbb.64.1553
Moyano F. J., Diaz M., Alarcón F. J., Sarasquete M. C. (1996). Characterization of digestive enzyme activity during larval development of gilthead seabream (Sparus aurata). Fish Physiol. Biochem. 15, 121–130. doi: 10.1007/BF01875591
Oozeki Y., Bailey K. M. (1995). Ontogenetic development of digestive enzyme activities in larval walleye pollock, Theragra chalcogramma. Mar. Biol. 122, 177–186. doi: 10.1007/BF00348930
Perrot V., Moiseeva E. B., Gozes Y., Chan S. J., Ingleton P., Funkenstein B. (1999). Ontogeny of the insulin-like growth factor system (IGF-I, IGF-II, and IGF-1R) in gilthead seabream (Sparus aurata): expression and cellular localization. Gen. Comp. Endocrinol. 116, 445–460. doi: 10.1006/gcen.1999.7337
Pipe R. K. (1990). Hydrolytic enzymes associated with the granular haemocytes of the marine mussel Mytilus edulis. Histochem. J. 22, 595–603. doi: 10.1007/BF01072941
Power D. M., Elias N. P., Richardson S. J., Mendes J., Soares C. M., Santos C. R. A. (2000). Evolution of the thyroid hormone-binding protein, transthyretin. Gen. Comp. Endocrinol. 119, 241–255. doi: 10.1006/gcen.2000.7520
Power D. M., Llewellyn L., Faustino M., Nowell M. A., Björnsson B. T., Einarsdóttir I. E., et al. (2001). Thyroid hormones in growth and development of fish. Comp. Biochem. Physiol. Part C: Toxicol. Pharmacol. 130, 447–459. doi: 10.1016/S1532-0456(01)00271-X
Revol A., Rodríguez M. D. L. G., Montenegro V. H., Aguilera C., Saldaña H. B., Mendoza R. (2005). Cloning of the growth hormone cDNA of alligator gar Atractosteus spatula and its expression through larval development. Comp. Biochem. Physiol. Part A: Mol. Integr. Physiol. 140, 423–429. doi: 10.1016/j.cbpb.2005.02.004
Ribeiro L., Couto A., Olmedo M., Álvarez-Blázquez B., Linares F., Valente L. M. (2008). Digestive enzyme activity at different developmental stages of blackspot seabream, Pagellus bogaraveo (Brunnich 1768). Aquacult. Res. 39, 339–346. doi: 10.1111/j.1365-2109.2007.01684.x
Ribeiro L., Zambonino-Infante J. L., Cahu C., Dinis M. T. (1999). Development of digestive enzymes in larvae of Solea Senegalensis, Kaup 1858. Aquaculture 179, 465–473. doi: 10.1016/S0044-8486(99)00180-5
Roubaty C., Portmann P. (1988). Relation between intestinal alkaline phosphatase activity and brush border membrane transport of inorganic phosphate, D-glucose, and D-glucose-6-phosphate. Pflügers Archiv 412, 482–490. doi: 10.1007/BF00582536
Santos C. R., Anjos L., Power D. M. (2002). Transthyretin in fish: state of the art. Clin. Chem. Lab. Med. 40, 1244–1249. doi: 10.1515/CCLM.2002.215
Shan X. J., Huang W., Cao L., Xiao Z. Z., Dou S. Z. (2009). Ontogenetic development of digestive enzymes and effect of starvation in miiuy croaker Miichthys miiuy larvae. Fish Physiol. Biochem. 35, 385–398. doi: 10.1007/s10695-008-9263-9
Shiao J. C., Hwang P. P. (2006). Thyroid hormones are necessary for the metamorphosis of tarpon Megalops cyprinoides leptocephali. J. Exp. Mar. Biol. Ecol. 331, 121–132. doi: 10.1016/j.jembe.2005.10.014
Sugita H., Kawasaki J., Deguchi Y. (1997). Production of amylase by the intestinal microflora in cultured freshwater fish. Lett. Appl. Microbiol. 24, 105–108. doi: 10.1046/j.1472-765X.1997.00360.x
Szisch V., Papandroulakis N., Fanouraki E., Pavlidis M. (2005). Ontogeny of the thyroid hormones and cortisol in the gilthead sea bream, Sparus aurata. Gen. Comp. Endocrinol. 142, 186–192. doi: 10.1016/j.ygcen.2004.12.013
Tagawa M., Miwa S., Inuii Y., de Jesus E., Hirano T. (1990a). Changes in thyroid hormone concentrations during early development and metamorphosis of the flounder, Paralichthys olivaceus. Zool. Sci. 7, 93–96. doi: 10.34425/zs000700
Tagawa M., Tanaka M., Matsumoto S., Hirano T. (1990b). Thyroid hormones in eggs of various freshwater, marine and diadromous teleosts and their changes during egg development. Fish Physiol. Biochem. 8, 515–520. doi: 10.1007/BF00003409
Tengjaroenkul B., Smith B. J., Caceci T., Smith S. A. (2000). Distribution of intestinal enzyme activities along the intestinal tract of cultured Niletilapia, Oreochromis niloticus L. Aquaculture 182, 317–327. doi: 10.1016/S0044-8486(99)00270-7
Uchida K., Kajimura S., Riley L. G., Hirano T., Aida K., Grau E. G. (2003). Effects of fasting on growth hormone/insulin-like growth factor I axis in the tilapia, Oreochromis mossambicus. Comp. Biochem. Physiol. Part A: Mol. Integr. Physiol. 134, 429–439. doi: 10.1016/S1095-6433(02)00318-5
Ueberschär B. (1993). “Measurement of proteolytic enzyme activity: significance and application in larval fish research,” in Physiological and biochemical aspects of fish development. Eds. Walther B. T., Fyhn H. J. (University of Bergen, Norway), 233–237. Available at: https://oceanrep.geomar.de/id/eprint/12155.
Uscanga-Martínez A., Perales-García N., Álvarez-González C. A., Moyano F. J., Tovar-Ramírez D., Gisbert G. E., et al. (2011). Changes in digestive enzyme activity during initial ontogeny of bay snook Petenia splendida. Fish Physiol. Biochem. 37, 667–680. doi: 10.1007/s10695-011-9467-2
Velez E. J., Unniappan S. (2021). A comparative update on the neuroendocrine regulation of growth hormone in vertebrates. Front. Endocrinol. 11. doi: 10.3389/fendo.2020.614981
Walford J., Lam T. J. (1993). Development of digestive tract and proteolytic enzyme activity in seabass (Lates calcarifer) larvae and juveniles. Aquaculture 109, 187–205. doi: 10.1016/0044-8486(93)90215-K
Wang D., Wu F. X. (2023). China Fishery Statistical Yearbook (Beijing, China: China Agriculture Press).
Wethmar C., Kleinow W. (1993). Characterization of a 27 kDa endopeptidase and detection of a proteinase-inhibitor in homogenates of Brachionus plicatilis (Rotifera). Comp. Biochem. Physiol. Part B: Comp. Biochem. 106, 359–368. doi: 10.1016/0305-0491(93)90313-T
Yang B. Y., Greene M., Chen T. T. (1999). Early embryonic expression of the growth hormone family protein genes in the developing rainbow trout, Oncorhynchus mykiss. Mol. Reprod. Develop.: Incorp. Gamete Res. 53, 127–134. doi: 10.1002/(SICI)1098-2795(199906)53:2<127::AID-MRD1>3.0.CO;2-H
Ying N., Wang Y., Qin B., Chen H., Song X., Yang L., et al. (2022). Report of Amyloodinium ocellatum in farmed black scraper (Thamnaconus modestus) in China. Aquaculture 561, 738722. doi: 10.1016/j.aquaculture.2022.738722
Zambonino-Infante J. L., Gisbert E., Sarasquete C., Navarro I., Gutiérrez J., Cahu C. L. (2008). Ontogeny and physiology of the digestive system of marine fish larvae. Feed. digest. functions fishes, 281–348.
Keywords: growth hormone, IGF-1, phosphatase, thyroid hormones, larval, juvenile
Citation: Liu L, Zeng J, Zhang Z, Wang J, Mei W, Wang C, Liu Z and Xu W (2024) Changes in growth, morphology, and levels of digestive enzymes and growth-related hormones in early ontogeny of black scraper, Thamnaconus modestus. Front. Mar. Sci. 11:1344844. doi: 10.3389/fmars.2024.1344844
Received: 26 November 2023; Accepted: 15 April 2024;
Published: 29 April 2024.
Edited by:
Zhen Ma, Dalian Ocean University, ChinaReviewed by:
Karine Rousseau, Muséum National d’Histoire Naturelle, FranceCarlos Alfonso Alvarez-González, Universidad Juárez Autónoma de Tabasco, Mexico
Copyright © 2024 Liu, Zeng, Zhang, Wang, Mei, Wang, Liu and Xu. This is an open-access article distributed under the terms of the Creative Commons Attribution License (CC BY). The use, distribution or reproduction in other forums is permitted, provided the original author(s) and the copyright owner(s) are credited and that the original publication in this journal is cited, in accordance with accepted academic practice. No use, distribution or reproduction is permitted which does not comply with these terms.
*Correspondence: Wengang Xu, xugang@ytu.edu.cn