Hard clam resilience to marine heatwaves in the face of climate change
- 1Fisheries College, Guangdong Ocean University, Zhanjiang, China
- 2Tianjin Key Laboratory of Aqua-ecology and Aquaculture, College of Fisheries, Tianjin Agricultural University, Tianjin, China
- 3Guangdong Science and Technology Innovation Center of Marine Invertebrate, Guangdong Ocean University, Zhanjiang, China
- 4Guangdong Provincial Key Laboratory of Aquatic Animal Disease Control and Healthy Culture, Guangdong Ocean University, Zhanjiang, China
The frequency and severity of marine heatwaves (MHWs) have reached new heights in the last two decades. Intensifying MHWs can affect intertidal bivalves, but the extent of their impacts remains largely underestimated. Here, we tested how persistent (P-MHW) and repeated (R-MHW) MHWs events affected the physiological energetics of ecologically and economically clams Mercenaria mercenaria inhabiting intertidal habitats. Compared to individuals maintained under ambient conditions, the clams exposed to both two scenarios of MHWs exhibited significant increases in their clearance rate, absorption efficiency, respiration rate, excretion rate, and scope for growth, showing compensatory energetic mechanisms to cope with MHWs. Especially, physiological energetics of M. mercenaria were more sensitive to repeated than persistent scenarios of MHWs. Given that the physiological response can act as an early and sensitive indicator of the fitness of intertidal bivalves, our results indicated that M. merceneria can likely hold the ability to readily recover from repeated to persistent exposure MHWs, enabling its continued resilience in a rapidly changing marine environment.
1 Introduction
Marine heatwaves (MHWs) have become more common in the past decade as a result of the continuous rise in Earth’s air surface temperatures which is mostly caused by rapidly increasing concentrations of atmospheric carbon dioxide (Horton et al., 2016). MHWs are defined as anomalies in seawater temperatures which are considerably greater than any previous levels that have been used in the past (Marin et al., 2021). The frequency, severity, and duration of MHWs are projected to rise dramatically in a rapidly changing marine environment, causing profoundly ecological and economic repercussions (Wernberg et al., 2023).
Throughout the past 20 years, MHWs have become more severe, recurrent, and longer in coastal ecosystems, especially attacking intertidal zones that support a very productive coastal environment (Masanja et al., 2023; Whalen et al., 2023). The thermal conditions in intertidal regions, specifically in subtidal zones, undergo substantial changes that surpass the fluctuations observed in ocean water temperatures, both in terms of amplitude and variability (Dong et al., 2022). Therefore, when MHWs occur, intertidal species are susceptible to abrupt changes in the environment and exceptionally high temperatures. This can result in a series of interconnected ecological consequence (He et al., 2021; Smith et al., 2023). There has been also a surge in the occurrence, duration, and severity of MHWs in China’s coastal areas (Yao et al., 2020; Li et al., 2023). The Bohai Bay, which is known for its abundant fishing opportunities, has been severely affected by intensifying MHWs events during summertime (Yao et al., 2020). MHWs can potentially have catastrophic effects, such as the bleaching of corals in tropical and subtropical environments, as well as the mass mortality events of marine bivalves on a wide scale (Holbrook et al., 2020; Smith et al., 2023).
Thus, projecting marine organism and ecosystem responses to MHWs is a global ecological problem (Smith et al., 2023). Despite the increasing prevalence of MHWs at an unprecedented rate, there remains significant uncertainty surrounding the response of economically and ecologically relevant organisms to the intensification of MHWs (Garrabou et al., 2022; Smith et al., 2023). MHWs may cause profound impacts at various levels of biological organization on marine bivalves, including hindering metabolism, reducing energy reserves, impairing reproduction, and triggering dysbiosis at the molecular level (Masanja et al., 2023). Encompassing population feedback down to the molecular level can hence provide a more accurate assessment of the effects of MHWs on marine bivalves and their ecosystems. In particular, these observations can be broadly expressed in terms of energy, as energy balance is crucial in determining the ability to tolerate and respond to stress (Sokolova et al., 2012). Indeed, thermal stress can affect various aspects of the allocation of available resources for energy consumption in bivalves, including obtaining energy by consuming food and breaking it down through digestion, as well as energy expenditure through respiration and excretion (Dong et al., 2018; Talevi et al., 2023). During periods of prolonged MHWs events, the intense thermal stress can significantly impact the distribution of energy between crucial physiological processes and functions associated with fitness, as demonstrated in the case of the hard clam Mercenaria mercenaria (Hu et al., 2022) and many other bivalve species (Liu et al., 2023; Shen et al., 2023; Talevi et al., 2023). When marine bivalves are exposed to temperatures that exceed their thermal tolerance limits, they may experience either internal hypoxia or hyperoxia (Lattos et al., 2023; Xu et al., 2023). An important metric for the total energy budget, the scope for growth (SFG), might fall sharply as a consequence of this thermal stress. It is possible to swiftly identify the energy state using the SFG approach, which provides a significant insight into the thermal stress response as a result (Ezgeta-Balić et al., 2011; Filgueira et al., 2011). As far as we know, however, there has been limited research on the physiological energy requirements of marine bivalves in relation to increasing MHWs in intertidal habitats.
The present study aimed at gaining a better understanding of the response of the ecologically and economically hard clam M. mercenaria to intensifying MHWs in the Bohai Sea. We exposed clams to simulated continuous and recurrent scenarios of MHWs. Physiological energetics were then assessed by encompassing the essential energetic processes such as feeding, digesting, respiration, excretion, and the energy budget exemplified by the SFG. Given that many previous studies have shown that intertidal bivalve species can survive in very tough conditions (He et al., 2021; Milan et al., 2023), we hypothesize that M. mercenaria might be able to cope with MHWs that nowadays frequently attack their habitats in the Bohai Sea.
2 Materials and methods
2.1 Bivalve collection and maintenance
The Bohai Sea has experienced significant impacts from climate change, especially suffering rapid increases in the frequency, intensity, and duration of MHWs (Yao et al., 2020; Mo et al., 2022). M. mercenaria is widely distribute in the intertidal zone and serve as a significant species for aquaculture worldwide (Hargrove et al., 2015). In the Bohai Sea, they are highly plentiful and serve as crucial fishing clam. Our goal was to study the multi-level reactions of intertidal bivalves to MHWs using adult hard clams as a model species. We hand-picked clams at low tide in the intertidal culture zone of Tianjin City, located in the Bohai Sea, China. The average length of the collected specimens was 31.40 ± 2.11 mm, the width 13.21 ± 1.25 mm, the height 21.30 ± 1.87 mm, and the wet weight 5.95 ± 1.05 g. At the sampling site and on the day of collection (June 18, 2022), the seawater temperature reached 24°C. We cleaned the clams within one hour of their arrival at the laboratory to eliminate any pollutants from their shells. While in the lab, the clams were subjected to identical circumstances to those on the day of collection for two weeks to help them acclimate at a constant temperature of 24°C, salinity of 32, pH of 8.0, dissolved oxygen above 7.0 mg/L. They were provided daily with about 20,000 Chlorella spp. cells/mL. Over the acclimation, no mortality was recorded.
2.2 Experimental setup
To examine the influence of MHWs on hard clams, three seawater recirculating systems were established. The structure of these systems was the same as in our previous study (Liang et al., 2022). In brief, each system consisted of three 60 L tanks (housing 45 clams per tank), a 120 L filtering tank (setting to minimize any interference from the metabolic waste products of the clams), and an 80 L temperature control tank (containing heating bod and pump of chiller). The experiment was set up with a group of ambient control, a group of persistent MHW (P-MHW), and a group of repeated MHW (R-MHW). According to the study reported by Yao et al (2020), seawater temperature thresholds setting at 25°C and 30°C, respectively (Figure 1). Seawater temperature, pH, and dissolved oxygen were measured twice daily for a 42-day experimental period. For 42 days, the control group was cultivated at a temperature of 25°C. The P-MHW group was maintained temperatures at 25°C for 14 days. On day 14, the temperatures were uniformly raised to 30°C at a rate of 1°C/h. The group was then kept at 30°C from day 14 to day 34. On day 35, the temperatures were uniformly lowered to 25°C at a constant pace of 1°C/h kept for 7 d. The R-MHW group simulated two occurrences of MHWs. In the first scenario, the temperature was controlled at 25°C on days 0-14, and, on days 14 the degree of heating was elevated to 30°C at a uniform rate of 1°C/h. The temperature was maintained for 7 days. On day 21, the temperature was uniformly reduced to 25°C at 1°C/h for 7 days. During the second scenario, the operation was repeated, on day 28, the temperature was warmed up to 30°C for 7 days, and on day 35, the temperature was uniformly reduced to 25°C and maintained for 7 days. In addition, all the measurements of physiological energetics were performed before the seawater temperature change.
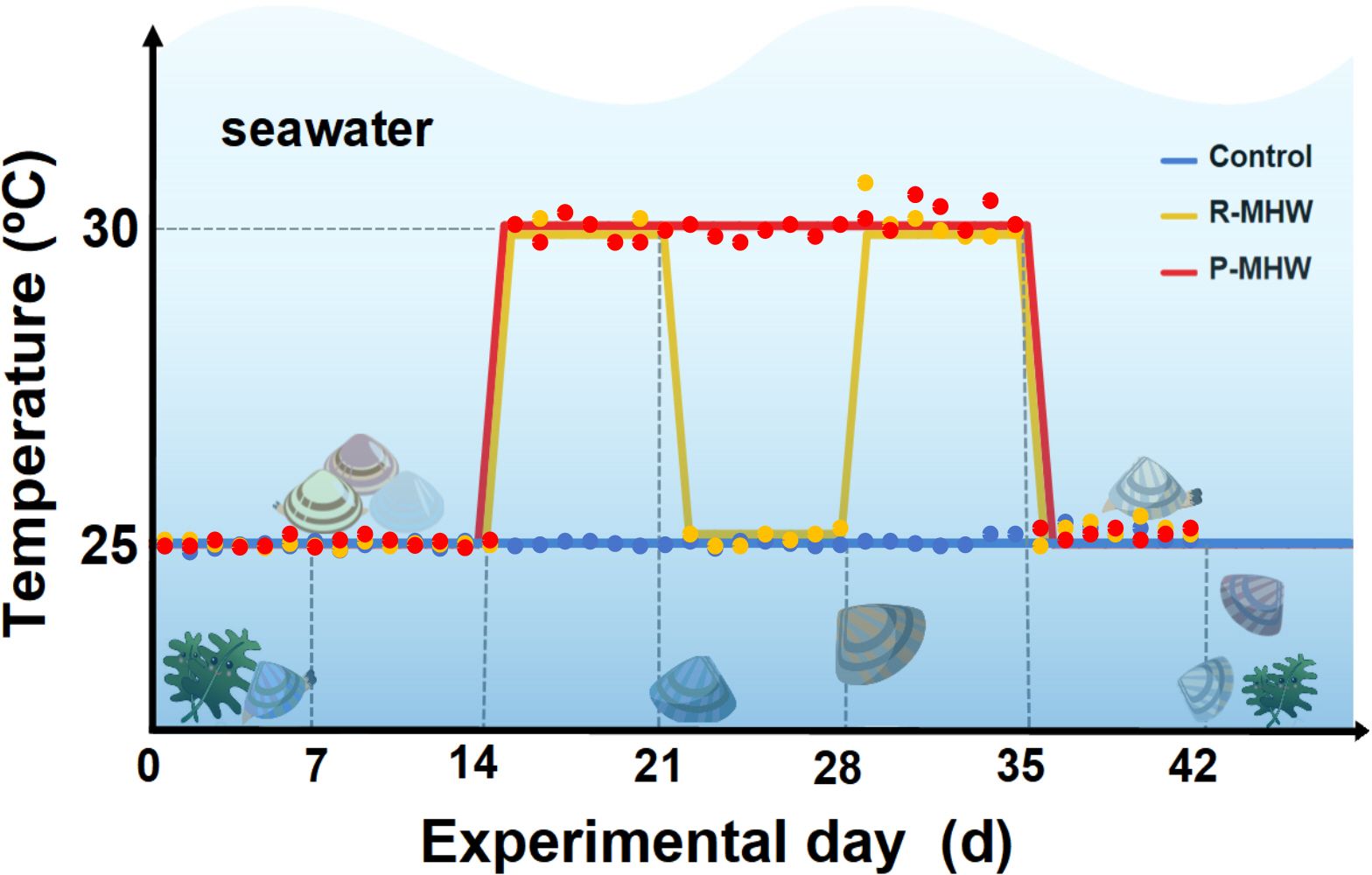
Figure 1 Summary of the experimental methodology and layout. For additional information, please see the text.
2.3 Physiological energetic measurements
2.3.1 Clearance rate
Clearance rate (CR) was measured individually in a beaker containing 2 L of seawater. Before measuring the CR, the calm was starved for 12 h to empty their guts. Based on a preliminary analysis, we determined that the optimal quantity for preventing pseudo-feces was a starting concentration of about 25,000 Chlorella spp. cells/mL. To measure the extent of algal buildup, we used two similar beakers as control samples, without any clam present. We quantified algal concentrations using a hemocytometer after a duration of 2 hours to ensure that CR determinations were not affected by large decline of cell concentration (less than 30%). CR was calculated by the following formula of Coughlan (1969):
where, CR represents clearance rate (L g− 1 h− 1); V is the volume of seawater (i.e., 2L); C1 represents the algal concentration at the start of the experiment, and C2 is the algal concentration after 2 hours of filtering; Δ t represents time elapsed (2 hours); dtw is the dry soft tissue mass (g) of each clam.
2.3.2 Absorption efficiency
The absorption efficiency (AE) quantifies the degree to which clams are capable of assimilating organic materials derived from algae. After conducting CR measurements, we meticulously gathered the clam feces from each beaker using 40-mm Whatman® GF/C glass fiber filters which were pre-combusted and pre-weighed the filters at a temperature of 450°C for 8 hours. Afterward, the filters were dried in an oven at a temperature of 110 °CC for 24 hours. After weighing, the filters were combusted in a muffle furnace at a temperature of 450°C for a duration of 6 hours. Finally, we weighed the filters again to determine their dry weight and ash-free weight, respectively. AE was computed according to Conover (1966):
Where, AE represents the absorption efficiency (%); F is the ratio of ash-free weight to dry weight in the algae and E represents the ratio of ash-free weight to dry weight in the feces.
2.3.3 Respiration rate
The respiration rate (RR) was measured individually from corresponding treatment tank in a sealed beaker containing 2 L of air saturated seawater. The experiment started with the clams engaging in active filtration to ensure the intake of oxygen in the beaker, and then continued for a duration of 2 hours. Oxygen concentration in each beaker was not allowed to drop below 30% of saturation throughout the experiment. In addition, two beakers served as control samples without clams. An oxygen meter (HACH HQ30d) was used in each beaker to determine the initial and final dissolved oxygen at the beginning and end of the experiment. The calculation of RR was performed using the following equation:
Where, RR represents respiration rate (mg O2 g−1 h−1); V is the seawater volume (2 L); ΔDO indicates the change in oxygen concentration in the seawater over the period of the experiment (mg O2 L−1); Δ t is time elapsed (2 hours); dtw is the dry soft tissue mass (g) of each clam.
2.3.4 Ammonia excretion rate
The ammonia excretion rate (ER) was monitored directly after the RR measurements. We employed the phenol-hypochlorite technique to evaluate the levels of ammonia nitrogen (NH4-N) in seawater at the beginning and conclusion of the experiment. The experiment included two beakers that were devoid of clams. We used the equation to determine the ER:
where, ER represents ammonia excretion rate (mg NH4-N g− 1 h− 1); V is seawater volume (2 L); C1 represents the concentration of NH4-N at the start of the experiment (mg L-1), and C2 is the concentrations of NH4-N by the end of the experiment (mg L-1); Δ t is time elapsed (2 hours); dtw is the dry soft tissue mass (g) of each clam.
2.3.5 Oxygen to nitrogen ratio
The quantification of oxygen consumption for nitrogen excretion may be determined by calculating the oxygen to nitrogen ratio (O:N). The ratio may be calculated using the following equation suggested by Widdows and Johnson (1988).
2.3.6 Scope for growth
Once essential physiological data has been converted into energy equivalents, researchers can determine the scope for growth (SFG) using the equation proposed by Bayne and Newell (1983):
Where, SFG represents scope for growth (J g− 1 h− 1); A is the total energy received from algae (J g− 1 h− 1); R is the energy used for respiration (J g− 1 h− 1); U is the energy used for excretion (J g− 1 h− 1). The conversion coefficient of energy referred to Wang et al. (2015).
2.4 Statistical analysis
The experimental data were analyzed using the SPSS Statistics 26.0 software. The data’s homogeneity was assessed using Levene’s F-test, while their normality was examined using the Shapiro-Wilks test. The analysis included a two-way analysis of variance (ANOVA), followed by a Fisher’s Least Significant Difference posthoc test. Data with a p-value< 0.05 were considered statistically significant differences among treatments in this investigation.
3 Results
The following is evidence of the physiological energetics of heatwave responses in hard clams. Figure 2 demonstrates a statistically significant CR (p< 0.05). A notable interaction effect exists between temperature and time. This suggests variations in the temporal patterns between the two MHWs scenarios. CR ranged from 0.54 to 1.13 L h- 1 g- 1. The P-MHW group showed a downward trend during the 30°C stress, especially on the 35 day of the experiment, which was significantly lower than that the other two experimental groups. Even when the temperature returned to 25°C for one week, it was still significantly lower than that of the control group (p< 0.05). The clearance rate of the R-MHWs group showed a fluctuating trend during the experiment that increased with the onset of heat waves but decreased with the recovery of temperature.
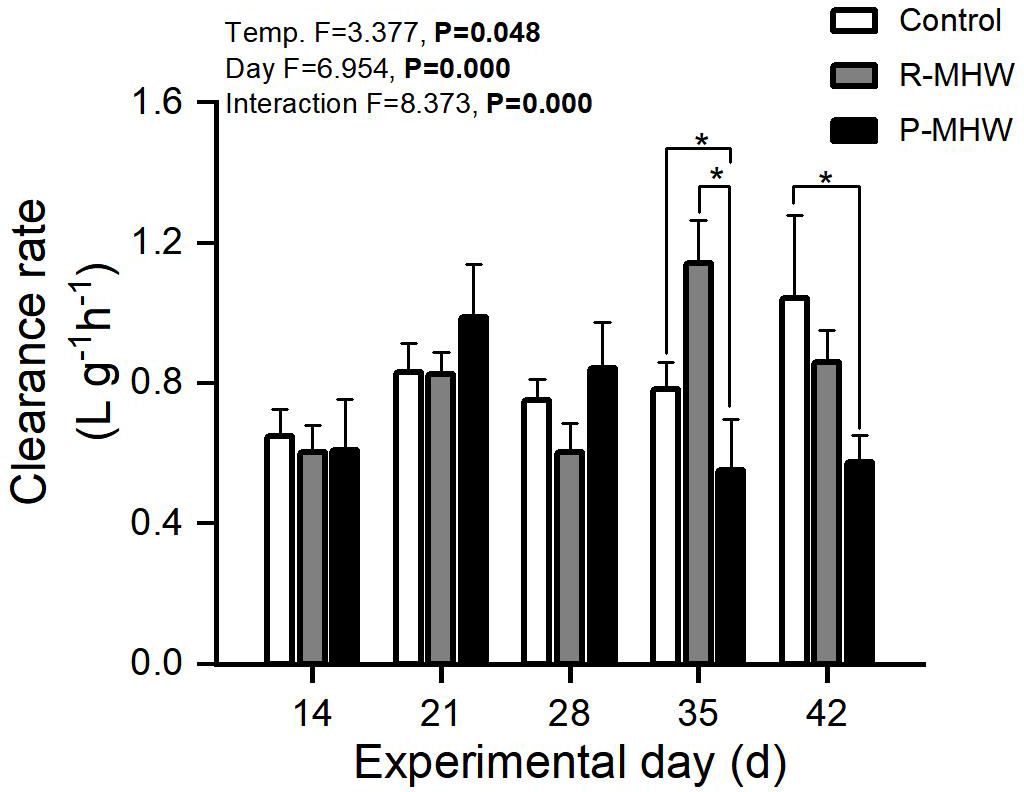
Figure 2 Clearance rate of M. mercenaria when subjected to simulated marine heatwaves (MHWs). The impacts of heatwaves’ severity (temperature) and duration (day) were analyzed using a two-way ANOVA, and the statistical results were displayed in each panel. "*" indicates significant differences between experimental groups with broken line connections (p<0.05).
The severity and length of the heatwave and the interaction between these two factors significantly influenced the AE (p< 0.05), as shown in Figure 3. Absorption efficiency varied over a wide range (39.3-67.0%). Over time, there was an increased first and then decreased under prolonged MHW stress in the P-MHW group, especially on day 35, which was significantly lower than that of other experimental groups (p< 0.05). However, when the temperature was returned to 25°C for one week, it also returned to normal levels. AE of R-MHW group increased during the first thermal stress and decreased after the MHWs disappeared.
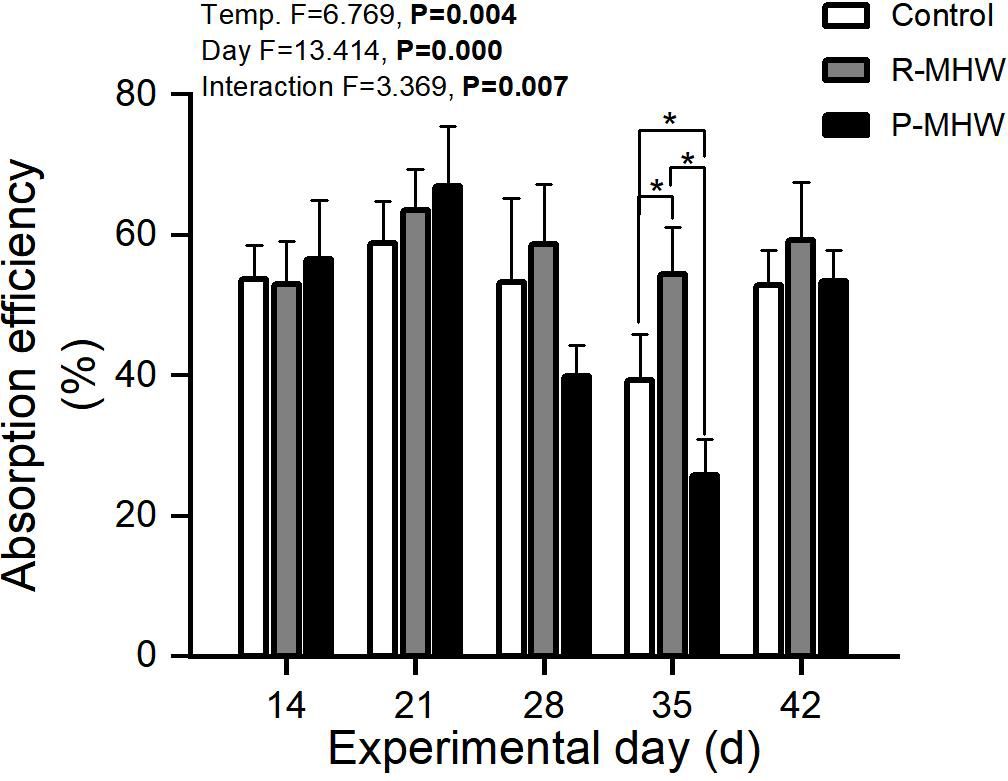
Figure 3 Absorption efficiency of M. mercenaria exposed to simulated heatwaves. Statistical results showing impacts of the intensity (temperature) and duration (day) of heatwaves were generated by two-way ANOVA and exhibited in each panel. "*" indicates significant differences between experimental groups with broken line connections (p<0.05).
Statistically, there is a significant effect of time on RR and a significant interaction effect between temperature and time (p< 0.05; Figure 4); Under heat stress, RR of the P-MHW group showed a similar pattern to that of AE, a significant difference was observed between P-MHW and control at day 35. The RR of R-MHW group increased strongly when experiencing the second heat wave stress, which was significantly higher than that of the control group (p< 0.05).
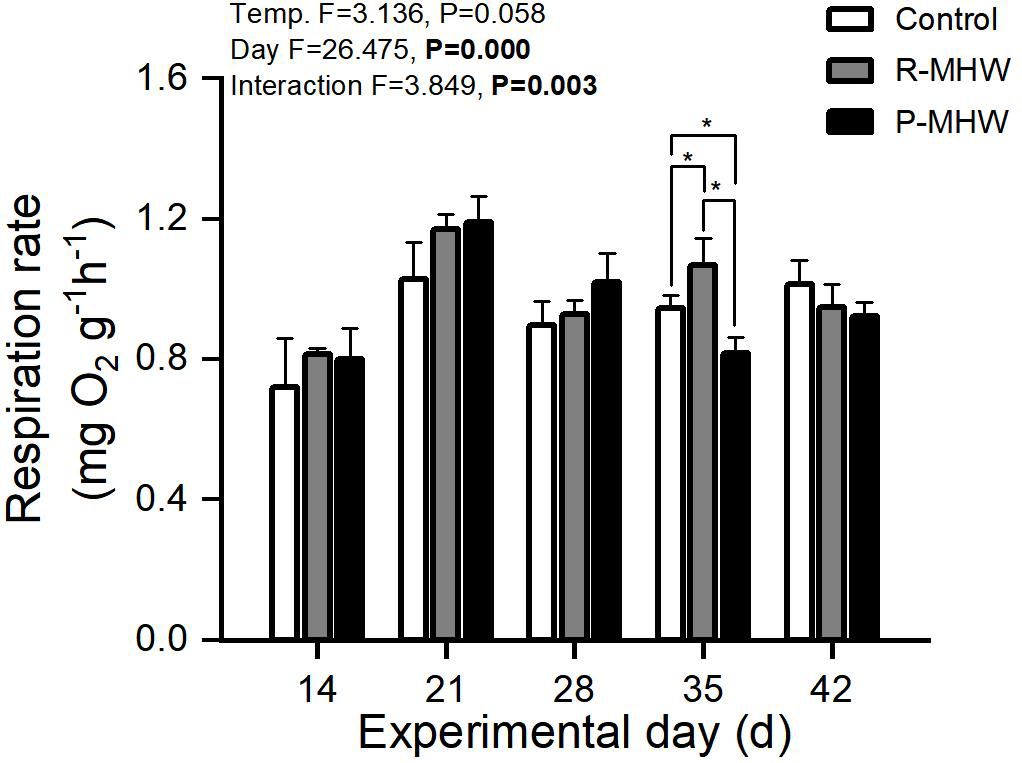
Figure 4 Respiration rate of M. mercenaria exposed to simulated heatwaves. Statistical results showing impacts of the intensity (temperature) and duration (day) of heatwaves were generated by two-way ANOVA and exhibited in each panel. "*" indicates significant differences between experimental groups with broken line connections (p<0.05).
There has been an overall increasing trend in excretion rates across both temperature conditions over time. Statistically, there is a significant main effect of time (p< 0.05; Figure 5). However, there were no significant effects of temperature or interaction.
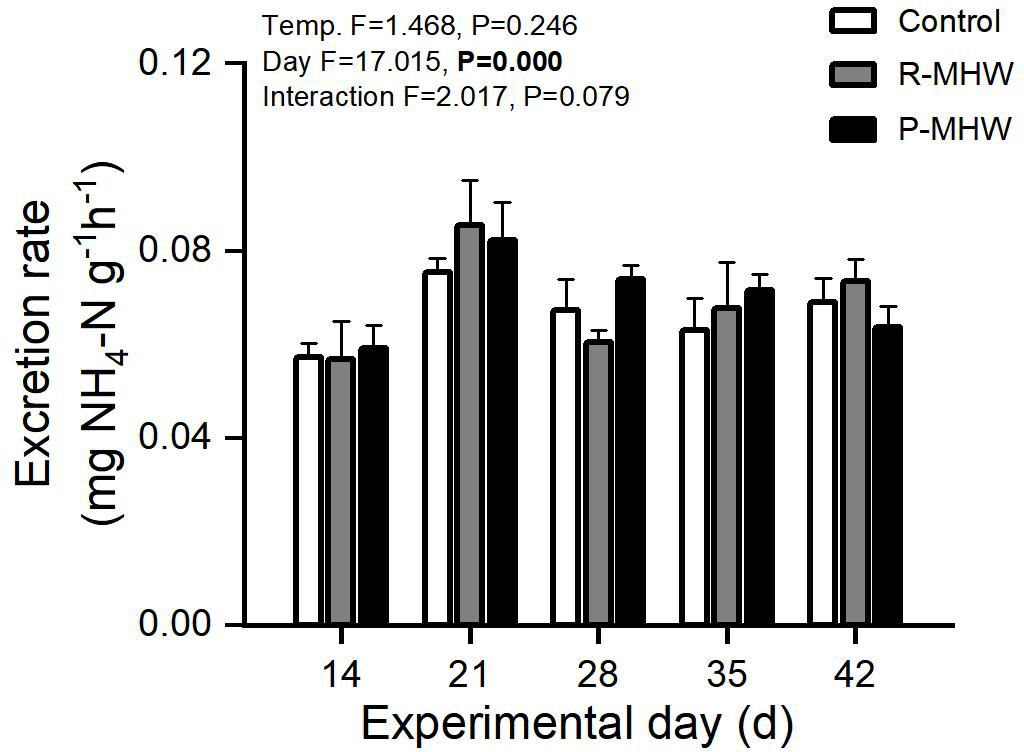
Figure 5 Excretion rate of M. mercenaria exposed to simulated heatwaves. Statistical results showing impacts of the intensity (temperature) and duration (day) of heatwaves were generated by two-way ANOVA and exhibited in each panel.
The temporal pattern shows excretion rates increase relatively consistently over time under both persistent and repeated heatwave conditions. The lack of a statistically significant temperature or interaction effect implies that the impact of MHWs on excretion rates is minimal.
There is no clear trend in the O:N ratio over time under either temperature condition. The O:N ratio fluctuates but remains relatively stable. Statistically, no significant main effect of time, temperature, or their interaction exists on the O:N ratio. The lack of a temporal pattern or temperature effect indicates that prolonged exposure to elevated temperatures from marine heatwaves does not impact the O:N ratio. The O:N ratio varies between 10.0 and 14.1 over time in the control and both experimental groups, with no clear directionality. The lack of significant effects implies that hard clams maintain stable oxygen-to-nitrogen waste excretion ratios despite heatwave conditions and over long periods (p > 0.05; Figure 6).
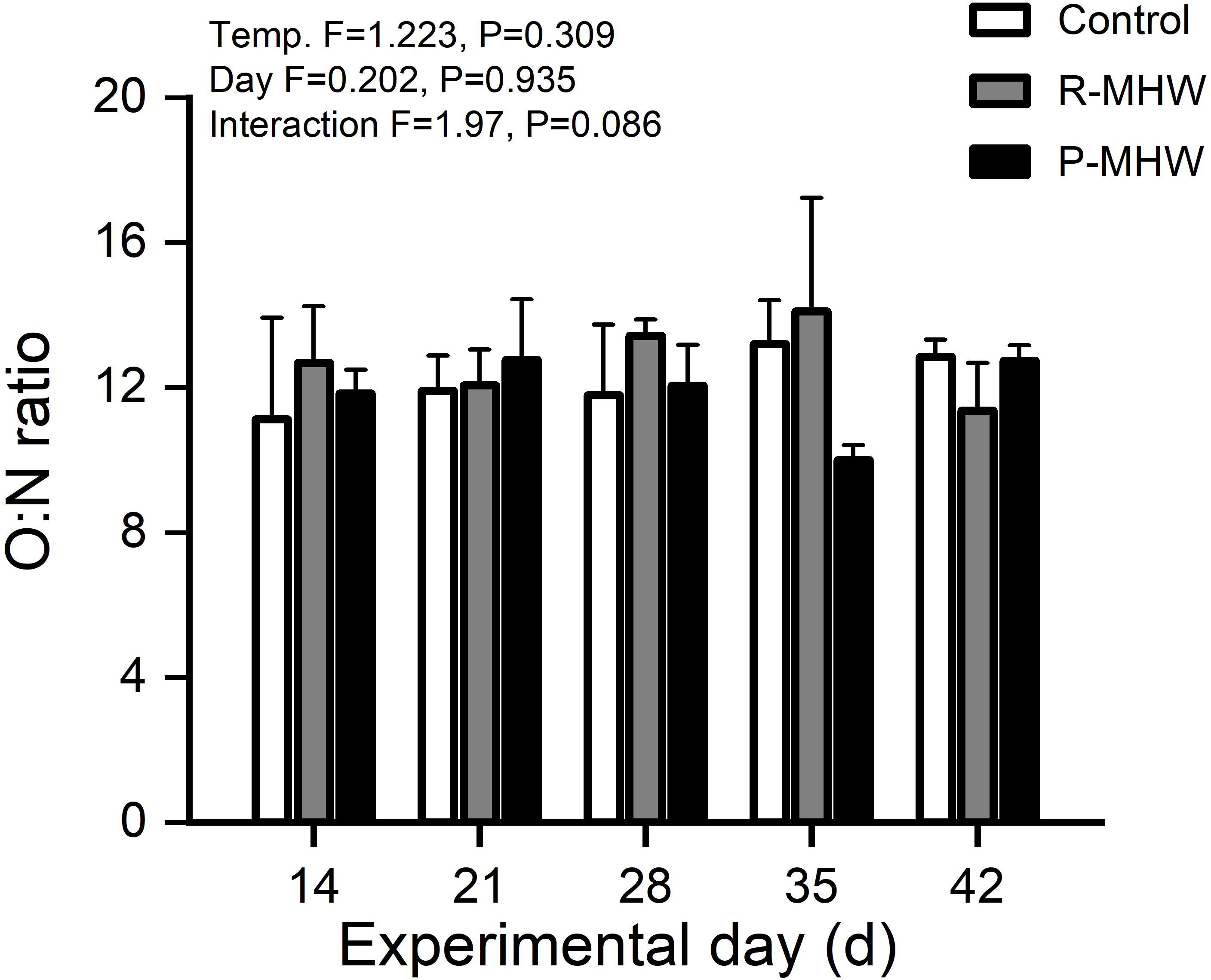
Figure 6 O:N ratio of M. mercenaria exposed to simulated heatwaves. Statistical results showing impacts of the intensity (temperature) and duration (day) of heatwaves were generated by two-way ANOVA and exhibited in each panel.
According to Figure 7, there were notable impacts on the potential for growth from the length and interaction with heatwave intensity (p< 0.05). Persistent heatwave exposure (on day 35) resulted in a significant decrease (p< 0.05; Figure 7). The SFG values of the P-MHW group were still significantly lower than those of the control and repeated heatwave groups (p<0.05), even after a week of temperature recovery (day 35 and 42).
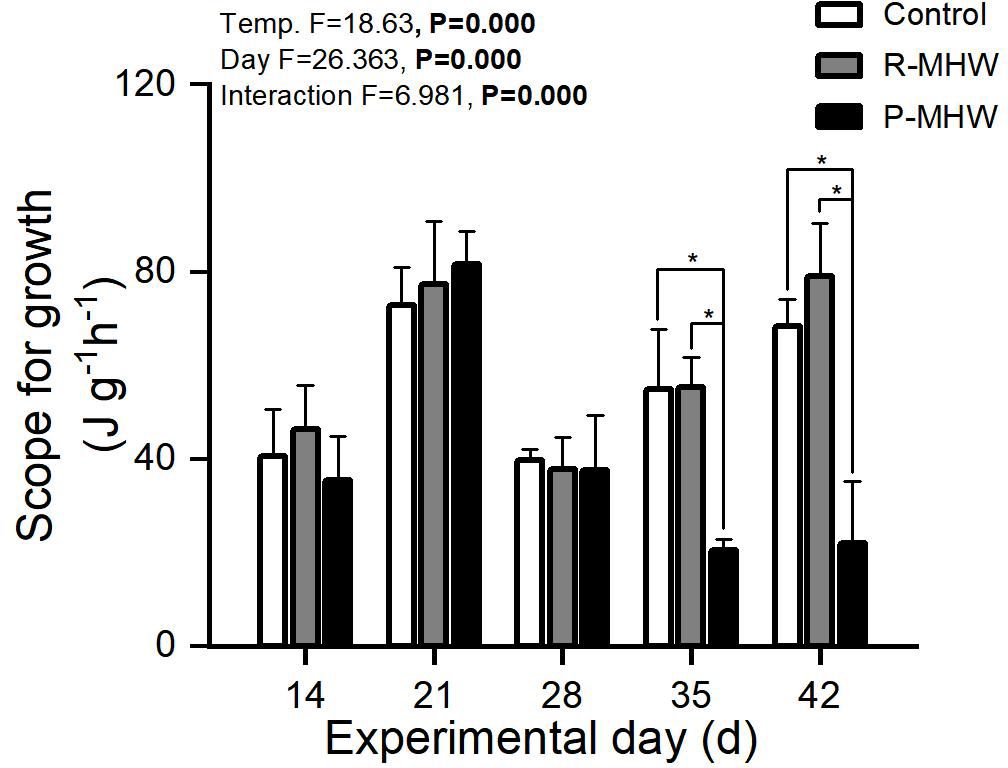
Figure 7 Scope for growth of M. mercenaria exposed to simulated heatwaves. Statistical results showing impacts of the intensity (temperature) and duration (day) of heatwaves were generated by two-way ANOVA and exhibited in each panel. "*" indicates significant differences between experimental groups with broken line connections (p<0.05).
4 Discussion
Given the imminent rise in the occurrence of MHWs, it is crucial to understand the mechanisms by which marine bivalves can recover from MHWs. This understanding is essential in determining their overall response to MHWs. In this study, we examined the physiological energy responses of hard clams as they adapted to continuous and repeated MHWs. Our research indicates that intertidal clams have the ability to withstand and endure marine heatwaves MHWs.
Previous studies on marine bivalves have observed a statistically significant and consistent adaptive ability in the clam’s clearance rate in response to the interaction between temperature and time (Kittner and Riisgård, 2005; Pernet et al., 2007). Riisgård (2001) also noted a similar temporal pattern in the clearance rate at which the clam Ruditapes philippinarum, with an initial decrease followed by a return to normal levels following prolonged exposure to a heat stress. These findings suggest that metabolic adaptation may enable the clearance rate to recover after an initial decrease caused by sudden exposure to high temperatures. Our results align with those of Kittner and Riisgård (2005), who observed a similar adaptation trend in the clearance rates of Mytilus edulis under heat stress. The consistent reaction of different clam species offers compelling evidence of the temporal metabolic adaptation that allows the clearance rate to settle following the initial shock of a heatwave.
Further, the significant decline in absorption efficiency under continuous heatwaves mirrors the patterns reported for other bivalves under thermal stress. For example, Talevi et al. (2023) noted decreasing absorption efficiency in the Manila clam R. philippinarum as heatwave durations increased. They attributed this to potential damage to the clams’ digestive system, hampering food absorption. The current study’s findings match those of Domínguez et al. (2021), who reported impaired absorption efficiency in the Cerastoderma edule after prolonged exposure to elevated temperatures. Comparatively, M. mercenaria appears similarly vulnerable to digestive disruption from metabolic stress during extended heatwaves. However, the absorption efficiency exhibited recovery tendencies once heatwave conditions ended. This suggests the digestive impairment may be reversible once temperatures return to normal ranges.
The marked increase in respiration rate of M. mercenaria aligns with previous evidence of bivalves’ metabolic responses to thermal stress. For instance, Trigos et al. (2015) noted significantly elevated respiration in Pinna nobilis under thermal stress. They attributed this to increased energetic costs for ventilation and circulation to manage elevated oxygen demand and respiratory acidosis. Furthermore, the current study’s findings converge with those of Bosch-Belmar et al. (2023), who reported increased respiration rates in the Mediterranean Sponge Chondrilla nucula under experimentally induced heat stress. The comparable responses of varying marine organisms add robustness to the concept of increased respiration as a metabolic adaptation to fulfill elevated energy requirements under thermal stress.
There has been an overall increasing trend in excretion rates across both temperature conditions over time. However, there were no significant effects of temperature or interaction. The temporal pattern shows excretion rates increase relatively consistently over time under both control and heatwave conditions. The lack of a statistically significant temperature or interaction effect implies that the impact of MHWs on excretion rates is minimal. M. mercenaria’s lack of significant change in excretion rate due to thermal stress parallels prior observations in M. edulis (Barrento et al., 2013). This contrasts with studies like those that noted increased excretion in M. edulis (Barrento et al., 2013) and Corbicula fluminea (Xiao et al., 2014) under warming conditions. The variance suggests potential species-specific differences in metabolic responses. However, M. mercenaria’s stable excretion rates align with the findings of Scapharca subcrenata (Jiang et al., 2020) under simulated heat stress. Metabolically, this may indicate the capacity of M. mercenaria to maintain waste excretion rates despite thermal stress without the need for physiological adjustment. Comparatively, this area requires further research across bivalve species to determine adaptation patterns.
The O:N ratio can be a useful indicator for determining the relative contribution of carbohydrate metabolism versus protein catabolism in bivalves. During aerobic respiration, when there is sufficient oxygen available, carbohydrates such as glycogen break down to produce carbon dioxide (CO2), water (H2O), and energy. When oxygen levels are low or carbohydrate stores are depleted, bivalves resort to anaerobic respiration or protein breakdown to meet their energy needs. A study on Haliotis discus hannai (Kang et al., 2019) exemplifies this. Because of this, the link between the amount of oxygen to nitrogen and the rise in MHWs suggests that M. mercenaria may be able to use its own energy to deal with physical problems. According to Hu et al. (2022), if the claim that energy supply increases during heatwaves is true, it seems likely that M. mercenaria can easily distribute energy among its different body systems in a way that follows the rules of energy management in stress adaptation and resilience.
The energy balance is crucial in determining the extent to which marine bivalves can withstand, adjust to, and ultimately adapt to heatwaves. Considering the assimilation of energy from food and native nutrients, as well as the energy used in respiration and excretion, the SFG can provide a thorough understanding of M. mercenaria’s probable response to heatwaves. Heatwave exposure to the clams led to a significant reduction in SFG, suggesting that clams under heatwave stress likely redirected their energy resources towards vital physiological functions. This discovery aligns with previous studies conducted on clams subjected to thermal stress Paphia undulata (Han et al., 2008) and R philippinarum (Zhang et al., 2018). These studies indicate that the fast rise in metabolic requirements is the probable cause of the susceptibility of SFG to high temperatures.
This finding suggests that components of the energy budget that are energetically efficient may display versatility. The findings suggest that M. mercenaria may possess the capacity to mitigate physiological effects during heatwaves. Hence, SFG serves as a measure of both the development and reproduction capabilities of organisms, as well as their ability to cope with impending stressors.
5 Conclusion
Overall, this study shows that hard clams effectively counteract the physiological effects caused by frequent heatwaves. Based on the observation that physiological energies exist, it is feasible to obtain a glance into mechanisms supporting such abilities. Processes that are of utmost relevance in determining the fate of M. mercenaria in a sea that is quickly warming require additional exploration. These processes are particularly important at the most fundamental levels of biological structure, such as the levels of biochemistry and molecular structure. This information will provide insight into the combined impact of biological incursions and global change on intertidal ecosystems.
Data availability statement
The original contributions presented in the study are included in the article/Supplementary Material. Further inquiries can be directed to the corresponding authors.
Ethics statement
The manuscript presents research on animals that do not require ethical approval for their study.
Author contributions
JL: Writing – original draft. FM: Writing – review & editing. YL: Writing – review & editing. YG: Writing – review & editing. YD: Writing – review & editing. LZ: Writing – review & editing.
Funding
The author(s) declare financial support was received for the research, authorship, and/or publication of this article. The current investigation has been facilitated by funding from various sources including the Guangdong Zhujiang Talents Program (2021QN02H665), the National Science Foundation of China (42076121, M-0163, 42211530423), the Department of Education of Guangdong Province (2020KTSCX050 and 2022ZDZX4012), the earmarked fund for Modern Agro-industry Technology Research System (CARS-49), and the program for Scientific Research Start-up Funds of Guangdong Ocean University.
Conflict of interest
The authors declare that the research was conducted in the absence of any commercial or financial relationships that could be construed as a potential conflict of interest.
Publisher’s note
All claims expressed in this article are solely those of the authors and do not necessarily represent those of their affiliated organizations, or those of the publisher, the editors and the reviewers. Any product that may be evaluated in this article, or claim that may be made by its manufacturer, is not guaranteed or endorsed by the publisher.
Supplementary material
The Supplementary Material for this article can be found online at: https://www.frontiersin.org/articles/10.3389/fmars.2024.1382825/full#supplementary-material
References
Barrento S., Lupatsch I., Keay A., Christophersen G. (2013). Metabolic rate of blue mussels (Mytilus edulis) under varying post-harvest holding conditions. Aquat. Living Resour. 26, 241–247. doi: 10.1051/alr/2013050
Bayne B. L., Newell R. C. (1983). Physiological energetics of marine molluscs. Mollusca. Eds. A. S. M. Saleuddin and K. M. Wilbur (New York: Academic Press), 407–515. doi: 10.1016/B978-0-12-751404-8.50017-7
Bosch-Belmar M., Milanese M., Sarà A., Mobilia V., Sarà G. (2023). Effect of acute thermal stress exposure on ecophysiological traits of the Mediterranean Sponge Chondrilla nucula: implications for climate change. Biology 13, 1. doi: 10.3390/biology13010009
Conover R. J. (1966). Assimilation of organic matter by zooplankton. Limnol. Oceanogr. 11, 338–354. doi: 10.4319/lo.1966.11.3.0338
Coughlan J. (1969). The estimation of filtering rate from the clearance of suspensions. Mar. Biol. 2, 356–358. doi: 10.1007/BF00355716
Domínguez R., Olabarria C., Woodin S. A., Wethey D. S., Peteiro L. G., Macho G., et al. (2021). Contrasting responsiveness of four ecologically and economically important bivalves to simulated heat waves. Mar. Environ. Res. 164, 105229. doi: 10.1016/j.marenvres.2020.105229
Dong Y. W., Liao M. L., Han G. D., Somero G. N. (2022). An integrated, multi-level analysis of thermal effects on intertidal molluscs for understanding species distribution patterns. Biol. Rev. 97, 554–581. doi: 10.1111/brv.12811
Dong Z. G., Chen Y. H., Ge H. X., Li X. Y., Wu H. L., Wang C. H., et al. (2018). Response of growth and development of the Pacific oyster (Crassostrea gigas) to thermal discharge from a nuclear power plant. BMC Ecol. 18, 31. doi: 10.1186/s12898-018-0191-y
Ezgeta-Balić D., Rinaldi A., Peharda M., Prusina I., Montalto V., Niceta N., et al. (2011). An energy budget for the subtidal bivalve Modiolus barbatus (Mollusca) at different temperatures. Mar. Environ. Res. 71, 79–85. doi: 10.1016/j.marenvres.2010.10.005
Filgueira R., Rosland R., Grant J. (2011). A comparison of scope for growth (SFG) and dynamic energy budget (DEB) models applied to the blue mussel (Mytilus edulis). J. Sea Res. 66, 403–410. doi: 10.1016/j.seares.2011.04.006
Garrabou J., Gómez-Gras D., Medrano A., Cerrano C., Ponti M., Schlegel R., et al. (2022). Marine heatwaves drive recurrent mass mortalities in the Mediterranean Sea. Global Change Biol. 28, 5708–5725. doi: 10.1111/gcb.16301
Han K. N., Lee S. W., Wang S. Y. (2008). The effect of temperature on the energy budget of the Manila clam, Ruditapes philippinarum. Aquacult. Int. 16, 143–152. doi: 10.1007/s10499-007-9133-y
Hargrove J. S., Sturmer L., Scarpa J., Austin J. D. (2015). Assessment of genetic diversity in wild and aquaculture stocks of Mercenaria mercenaria in Florida. J. Shellfish Res. 34, 355–365. doi: 10.2983/035.034.0218
He G. X., Liu X. L., Xu Y., Liang J., Deng Y. W., Zhang Y. H., et al. (2021). Repeated exposure to simulated marine heatwaves enhances the thermal tolerance in pearl oysters. Aquat. Toxicol. 239, 105959. doi: 10.1016/j.aquatox.2021.105959
Holbrook N. J., Sen Gupta A., Oliver E. C., Hobday A. J., Benthuysen J. A., Scannell H. A., et al. (2020). Keeping pace with marine heatwaves. Nat. Rev. Earth Environ. 1, 482–493. doi: 10.1038/s43017-020-0068-4
Horton R. M., Mankin J. S., Lesk C., Coffel E., Raymond C. (2016). A review of recent advances in research on extreme heat events. Curr. Climate Change Rep. 2, 242–259. doi: 10.1007/s40641-016-0042-x
Hu Z., Feng J., Song H., Zhou C., Yang M. J., Shi P., et al. (2022). Metabolic response of Mercenaria mercenaria under heat and hypoxia stress by widely targeted metabolomic approach. Sci. Total Environ. 809, 151172. doi: 10.1016/j.scitotenv.2021.151172
Jiang Y. Z., Jiao H. F., Sun P., Yin F., Tang B. J. (2020). Metabolic response of Scapharca subcrenata to heat stress using GC/MS-based metabolomics. PeerJ 8, e8445. doi: 10.7717/peerj.8445
Kang H. Y., Lee Y. J., Song W. Y., Kim T. I., Lee W. C., Kim T. Y., et al. (2019). Physiological responses of the abalone Haliotis discus hannai to daily and seasonal temperature variations. Sci. Rep. 9, 8019. doi: 10.1038/s41598-019-44526-3
Kittner C., Riisgård H. U. (2005). Effect of temperature on filtration rate in the mussel Mytilus edulis:: no evidence for temperature compensation. Mar. Ecol. Prog. Ser. 305, 147–152. doi: 10.3354/meps305147
Lattos A., Papadopoulos D. K., Giantsis I. A., Feidantsis K., Georgoulis I., Karagiannis D., et al. (2023). Investigation of the highly endangered Pinna nobilis' mass mortalities: Seasonal and temperature patterns of health status, antioxidant and heat stress responses. Mar. Environ. Res. 188, 105977. doi: 10.1016/j.marenvres.2023.105977
Li Z. J., Wan L. Y., Liu Y., Wang Z. Y., Wu L. Y. (2023). Analysis of Marine Heatwaves in China’s coastal seas and adjacent offshore waters. Atmosphere 14, 1738. doi: 10.3390/atmos14121738
Liang J., Liu Y. H., Zhu F. X., Li Y. R., Liang S., Guo Y. (2022). Impact of ocean acidification on the physiology of digestive gland of razor clams Sinonovacula constricta. Front. Mar. Sci. 9, 1010350. doi: 10.3389/fmars.2022.1010350
Liu X. L., Peng Y. L., Xu Y., He G. X., Liang J., Masanja F., et al. (2023). Responses of digestive metabolism to marine heatwaves in pearl oysters. Mar. pollut. Bull. 186, 114395. doi: 10.1016/j.marpolbul.2022.114395
Marin M., Feng M., Phillips H. E., Bindoff N. L. (2021). A global, multiproduct analysis of coastal marine heatwaves: Distribution, characteristics, and long-term trends. J. Geophysical Research-Oceans 126, e2020JC016708. doi: 10.1029/2020JC016708
Masanja F., Yang K., Xu Y., He G. X., Liu X. L., Xu X., et al. (2023). Impacts of marine heat extremes on bivalves. Front. Mar. Sci. 10, 1159261. doi: 10.3389/fmars.2023.1159261
Milan M., Bernardini I., Bertolini C., Dalla Rovere G., Manuzzi A., Pastres R., et al. (2023). Multidisciplinary long-term survey of Manila clam grown in farming sites subjected to different environmental conditions. Sci. Total Environ. 863, 160796. doi: 10.1016/j.scitotenv.2022.160796
Mo S. H., Chen T. R., Chen Z. S., Zhang W. J., Li S. (2022). Marine heatwaves impair the thermal refugia potential of marginal reefs in the northern South China Sea. Sci. Total Environ. 825, 154100. doi: 10.1016/j.scitotenv.2022.154100
Pernet F., Tremblay R., Comeau L., Guderley H. (2007). Temperature adaptation in two bivalve species from different thermal habitats: energetics and remodelling of membrane lipids. J. Exp. Biol. 210, 2999–3014. doi: 10.1242/jeb.006007
Riisgård H. U. (2001). On measurement of filtration rates in bivalves—The stony road to reliable data:: review and interpretation. Mar. Ecol. Prog. Ser. 211, 275–291. doi: 10.3354/meps211275
Shen Y. W., You W. W., Luo X., Lu Y., Huang M. Q., Ke C. (2023). An overview of the mechanisms underlying hypoxia tolerance differences in aquatic animals and their inspirations for aquaculture. Rev. Fish Biol. Fisheries 33, 1223–1236. doi: 10.1007/s11160-023-09793-4
Smith K. E., Burrows M. T., Hobday A. J., King N. G., Moore P. J., Gupta A. S., et al. (2023). Biological impacts of marine heatwaves. Annu. Rev. Mar. Sci. 15, 119–145. doi: 10.1146/annurev-marine-032122-121437
Sokolova I. M., Frederich M., Bagwe R., Lannig G., Sukhotin A. A. (2012). Energy homeostasis as an integrative tool for assessing limits of environmental stress tolerance in aquatic invertebrates. Mar. Environ. Res. 79, 1–15. doi: 10.1016/j.marenvres.2012.04.003
Talevi J., Steeves L., Coffin M., Guyondet T., Sakamaki T., Comeau L., et al. (2023). The physiological state of four commercially important bivalve species during a naturally occurring heatwave. Can. J. Zool. 101, 913–929. doi: 10.1139/cjz-2022-0215
Trigos S., Rafael J., Vicente N., Tena J., Torres J. (2015). Respiration rates of the fan mussel Pinna nobilis at different temperatures. J. Molluscan Stud. 81, 217–222. doi: 10.1093/mollus/eyu075
Wang Y. J., Li L. S., Hu M. H., Lu W. Q. (2015). Physiological energetics of the thick shell mussel Mytilus coruscus exposed to seawater acidification and thermal stress. Sci. Total Environ. 514, 261–272. doi: 10.1016/j.scitotenv.2015.01.092
Wernberg T., Thomsen M. S., Baum J. K., Bishop M. J., Bruno J. F., Coleman M. A., et al. (2023). Impacts of climate change on marine foundation species. Annu. Rev. Mar. Sci. 16, 247–282. doi: 10.1146/annurev-marine-042023-093037
Whalen M. A., Starko S., Lindstrom S. C., Martone P. T. (2023). Heatwave restructures marine intertidal communities across a stress gradient. Ecology 104, e4027. doi: 10.1002/ecy.4027
Widdows J., Johnson D. (1988). Physiological energetics of Mytilus edulis: scope for growth. Mar. Ecol. 46, 113–121. doi: 10.3354/meps046113
Xiao B. C., Li E. C., Du Z. Y., Jiang R. L., Chen L. Q., Yu N. (2014). Effects of temperature and salinity on metabolic rate of the Asiatic clam Corbicula fluminea (Mülle). SpringerPlus 3, 1–9. doi: 10.1186/2193-1801-3-455
Xu X., Zhang X. Z., Peng J. X., Deng Y. W., Liu Y., Jiang L. Y., et al. (2023). Survival and physiological energetics of highly invasive mussels exposed to heatwaves. Mar. Environ. Res. 187, 105948. doi: 10.1016/j.marenvres.2023.105948
Yao Y. L., Wang J. J., Yin J. J., Zou X. Q. (2020). Marine heatwaves in China's marginal seas and adjacent offshore waters: past, present, and future. J. Geophys. Res.: Oceans 125, e2019JC015801.
Keywords: global warming, extreme weather events, physiological energetics, marine bivalves, Mercenaria mercenaria
Citation: Liang J, Masanja F, Li Y, Guo Y, Deng Y and Zhao L (2024) Hard clam resilience to marine heatwaves in the face of climate change. Front. Mar. Sci. 11:1382825. doi: 10.3389/fmars.2024.1382825
Received: 06 February 2024; Accepted: 11 March 2024;
Published: 22 March 2024.
Edited by:
Youji Wang, Shanghai Ocean University, ChinaReviewed by:
Zhongming Huo, Dalian Ocean University, ChinaZhiguo Dong, Jiangsu Ocean University, China
Copyright © 2024 Liang, Masanja, Li, Guo, Deng and Zhao. This is an open-access article distributed under the terms of the Creative Commons Attribution License (CC BY). The use, distribution or reproduction in other forums is permitted, provided the original author(s) and the copyright owner(s) are credited and that the original publication in this journal is cited, in accordance with accepted academic practice. No use, distribution or reproduction is permitted which does not comply with these terms.
*Correspondence: Yuewen Deng, dengyw@gdou.edu.cn; Liqiang Zhao, lzhao@gdou.edu.cn