Bulk and amino acid isotope evidence of supplementary food sources besides euphotic production for a deep-sea coral community in the South China Sea
- 1State Key Laboratory of Marine Geology, Tongji University, Shanghai, China
- 2Southern Marine Science and Engineering Guangdong Laboratory (Zhuhai), Zhuhai, China
Deep-sea coral communities, rich in various zoobenthos, have been discovered in the South China Sea (SCS) in recent years. Yet little is known about the trophic structure of these communities. In this study, we applied bulk isotope and compound-specific isotope analysis of amino acids (CSIA-AAs) to explore feeding strategies and estimate the trophic positions (TPs) and isotopic baseline for 6 deep-sea gorgonians and 7 other zoobenthos collected from a deep-sea coral community in the SCS. Bulk carbon and nitrogen isotope values (δ13C and δ15N) suggested that the zoobenthos in the community have a variety of food sources. Amino acids δ15N results indicated that the TP is 2.3 ± 0.2 (mean ± 1σ) for the deep-sea gorgonians and varies from 2.0 ± 0.3 (sponge) to 3.5 ± 0.5 (starfish) for other zoobenthos. The δ15N values of phenylalanine revealed variable isotopic baselines ranging from +3.0 ± 0.9‰ to +11.7 ± 0.5‰, reflecting the incorporation of nitrogen from sources not limited to surface primary producers. Taken together, our data suggest that zoobenthos in the deep-sea coral community are mostly omnivorous, and their diet does not come solely from export production from the sea surface, with symbiotic bacteria as a potential important source.
1 Introduction
Deep-sea corals are found on hard seafloor substrates in dark and cold environments. Because of their extraordinary longevity and ability to record dietary information in accretionary growth bands that are well preserved through time, they are unique bio-archives capable of providing centennial- to millennial-scale records of past ocean conditions at sub-decadal resolution (Robinson et al., 2014; Sherwood et al., 2014; McMahon et al., 2015; Glynn et al., 2022). Furthermore, deep-sea coral communities provide a complex habitat that supports high biodiversity and serves as a critical gathering point for fish and invertebrates (Husebo et al., 2002). Despite their seclusion, they face threats from climate change and anthropogenic perturbations, including direct (e.g., deep-sea fishing practices) and indirect (e.g., changes in marine primary productivity, global warming, or ocean acidification) ones (Roark et al., 2009). The longevity and slow growth rate of deep-sea organisms make their regeneration extremely difficult, and damage to these communities has far-reaching implications for biodiversity, ecosystem structure, and functional extinctions in the deep sea (Roark et al., 2009). This excites recent interest in the conservation and protection of deep-sea coral communities, which requires a better understanding of deep-sea coral ecology, trophic connectivity, and the coupling between deep-sea communities and surface ocean primary production. Among related investigations, carbon and nitrogen isotope analysis are important research methods.
Analysis of carbon and nitrogen stable isotope values (δ13C and δ15N) in organisms has emerged as one of the most powerful tools for investigating consumer food source and trophic position (TP) in the food web over the last few decades (e.g., McClelland and Montoya, 2002; Chikaraishi et al., 2009; Larsen et al., 2013; Arthur et al., 2014; Sherwood et al., 2014; Shen et al., 2021). The bulk stable isotopic value of an organism’s tissue is generally related to its food source (Mincks et al., 2008). During feeding, the smaller fractionation of carbon isotopes (~1‰) makes δ13Cbulk useful for distinguishing food sources with distinctly different δ13Cbulk values, whereas the larger fractionation of nitrogen isotopes (3‰–5‰) makes δ15Nbulk suitable for delineating trophic positions (Hobson and Welch, 1992; Michener and Schell, 1994). The later developed technique of compound-specific amino acids (AAs) analyses is a complementary approach to bulk tissue isotope analysis and offers insights into trophic dynamics that are challenging to ascertain from bulk analysis alone. The principle of this method is the variation in isotopic fractionation among various AAs throughout metabolic processing (McClelland and Montoya, 2002). The application of δ15NAA is based on different degrees of nitrogen isotope fractionation that occur during trophic transfer between ‘‘source” and ‘‘trophic” AAs, with little or low 15N enrichment in the source AAs and high 15N enrichment in the trophic AAs (Popp et al., 2007). As a result, the information about source nitrogen δ15N baseline and trophic position changes can be obtained from paired analyses of δ15N values for source (δ15NSAA) and trophic AAs (δ15NTAA) (Chikaraishi et al., 2009; McMahon and McCarthy, 2016; Ohkouchi et al., 2017). The δ13CAA has been found to have the potential to fingerprint food sources (Larsen et al., 2009, 2013; Arthur et al., 2014; Glynn et al., 2019), but is less applied than the δ15NAA, perhaps due to, e.g., the classification diversity of essential amino acids in different organisms (Wang, 1999; Larsen et al., 2009) and the occurrence of kinetic carbon isotope effects during derivatization before δ13CAA values are measured (Corr et al., 2007a).
Globally, bulk and AAs isotope analyses have been increasingly applied in deep-sea coral studies. Most of these investigations and pure culture experiments point to sinking particulate organic matter (POM) as the dominant food for deep-sea corals and their low tropical level (Duineveld et al., 2004; Sherwood et al., 2005; Roark et al., 2009; Van Oevelen et al., 2018; Murray et al., 2019; Shen et al., 2021). This conclusion has been the theoretical basis for using deep-sea corals to reconstruct past nutrient and production dynamics in the surface ocean (Sherwood et al., 2011, 2014; Williams et al., 2017; Glynn et al., 2019). There are, however, a few studies suggesting the deep-sea coral system is also connected to submarine methane seeps (Mortensen et al., 2001; Hovland and Risk, 2003; Judd and Hovland, 2007) and symbiotic microbes that carry out complex element cycling (Grover et al., 2014; Kellogg et al., 2016; Lawler et al., 2016; Röthig et al., 2017a; Röthig et al., 2017b).
In recent years, the manned submersible has been increasingly exploited in deep-sea investigations in the South China Sea (SCS) and is uncovering the dark ocean ecosystem. One of the fascinating findings from these investigations is the existence of “coral forest” in the depths of 1200–1400 m, which is characterized by tall, whip-shaped bamboo corals and an understory shrub of “sea fan” corals and supra-benthos faunas (Li and Wang, 2019). Till now, little is known about the feeding strategies and trophic connections of the deep-sea communities. In the deep SCS, previous investigations showed that the deep microbial carbon demand substantially exceeded the available particulate organic carbon exported from the euphotic zone (Shen et al., 2020). We therefore postulate that the zoobenthos of the deep-sea coral community in the SCS may also be starved if they rely solely on the export particulate matter from the euphotic layer and hence require supplementation from other food sources, perhaps symbiotic microbes. In this study, we address this issue by analyzing the δ13Cbulk, δ15Nbulk and δ15NAA of the collected animals in a deep-sea coral community.
2 Materials and methods
2.1 Samples collection and treatment
In this study, various fauna in a deep-sea coral community were collected by the “Shenhaiyongshi” manned submersible during an SCS voyage in August 2019, at 1200–1400 m water depth (16.57°N, 110.72°E, Figure 1). They consisted of 6 deep-sea gorgonians (genus of Alcyonacea) and 7 zoobenthos species, including Holothuroidea (sea cucumber), Ophiuroidea (brittle star), Asteroidea (starfish), Hexactinellida (sponge), Siphonostomatoida (sea louse), Actiniaria (sea anemone), and Eumalacostraca (deep-sea shrimp). After boarding, the soft tissues of gorgonians and other zoobenthos were immediately separated from their skeletons and stored at –20°C. Seawaters at 30 m, 300 m, 600 m, and 1500 m depths above the benthic community were lifted along with a CTD instrument for suspended POM collection, which were filtered from ~100-liter waters on pre-combusted (450°C) glass fiber filters (GF/F, 0.7 μm pore size, 142 mm diameter). All suspended POM samples were stored at –20°C and brought back to the laboratory for further processing.
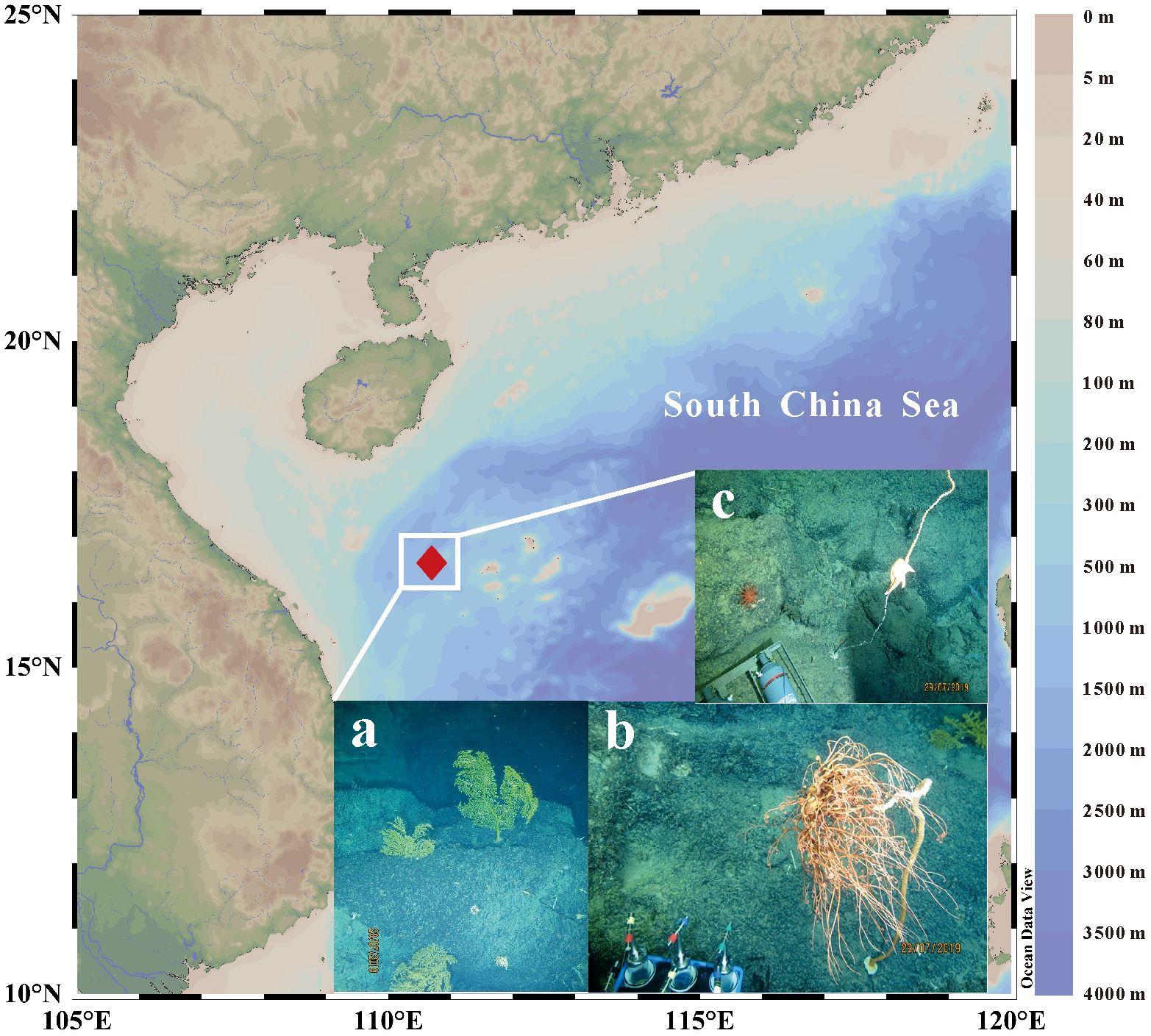
Figure 1 Site of the deep-sea coral community in the South China Sea and several deep-sea coral photos taken in the manned submersible. (A) Sea fan-shaped gorgonians, a suborder of soft corals; (B) Tree-shaped gorgonians; (C) A starfish is climbing on and eating a bamboo coral.
In the laboratory, POM samples were lyophilized at –30°C, and the soft tissues of gorgonians and other zoobenthos were thawed, then rinsed three times with Milli-Q water, and finally dried at 40°C for 20 h. After drying, these soft tissues were finely powdered in an agate mortar.
2.2 Stable isotope analysis
Before bulk isotope analysis, the powdered zoobenthos’ soft tissues were treated with 1 N HCl to remove any inorganic carbon. Bulk δ13C and δ15N values of soft tissues were measured on an isotope ratio mass spectrometer (Finnigan DELTAplus XP) interfaced with an elemental analyzer (Carlo Erba Instruments Flash 1112). The δ13C and δ15N values were reported using the standard notation relative to the Vienna Pee Dee Belemnite (VPDB) standard and air N2, respectively. Each zoobenthos sample was analyzed in duplicate, and three external isotope standards (Acetanilide, +1.18 ± 0.02‰ for δ15N, –29.53 ± 0.01‰ for δ13C) were introduced between every eight samples. The average standard deviations (SD) of external isotope standards were ±0.02‰ for δ13C and ±0.41‰ for δ15N. In order to allow sufficient sample amounts for AAs isotope analysis, POM was not subjected to bulk isotope analysis.
About 50 mg of soft tissue powder from each species were taken for AAs extraction. Briefly, proteins in the samples were acid hydrolyzed in the 6 N HCl solution at 110°C for 20 h to release the AA monomers. After evaporation to dryness with a rotary evaporator in a water bath at 70°C, AAs were dissolved in 0.01 N HCl and purified by passing them through a cation exchange column (Dowex 50WX × 400 ion exchange resin) with 2 N ammonia hydroxide as the eluent. After being dried once more, AAs were derivatized to N-acetyl methyl (NACME) esters, according to (Corr et al. 2007a, b). After the derivatization reaction, the solution was gently blown dry under ice-bath conditions with a stream of ultra-high purity N2 and then re-dissolved in dichloromethane for compound-specific AAs isotope analysis.
Isotope analysis of the individual AAs was performed on the gas chromatography-isotope ratio mass spectrometer (GC-IRMS, Thermo Scientific). Compounds were separated on the GC equipped with a TG-5MS column (60 m × 0.32 mm inner diameter, 0.25 μm film thickness) for δ15N analysis. The external isotope standards (USGS 65, glycine, 20.68 ± 0.06‰ for δ15N) were inserted in triples between every five samples to monitor the instrumental performance during the analyses. The δ15N standard deviations of the external standard were ±1.0‰ throughout the experiments. All samples were analyzed 3 times, and the isotope values were reported as mean ± SD. The standard deviation values were different for different AAs, overall ranging from ±0.0‰ to ±2.2‰.
The suspended POM samples were measured for δ15NAAs using the same procedures as above without duplicate analysis because of limited sample amounts. Therefore, the standard deviations of suspended POM isotope data were constrained by the inserted external standards.
2.3 Calculation of trophic position and isotope baseline
We note that through the acid hydrolysis procedure, glutamine (Gln) is converted to glutamic acid (Glu), and asparagine (Asn) is converted into aspartic acid (Asp). As a result, the final measurements combine Gln + Glu (referred to as Glx) and Asn + Asp (Asx). When classified in terms of nitrogen isotopes, Glx, Asx, Leu, Ile, and Val are trophic AAs (TAAs), while Phe and Tyr are source AAs (SAAs) (McMahon and McCarthy, 2016; O’Connell, 2017). Gly, Ser, and Thr are kept as separate groups given the lack of consensus on the degree of trophic fractionation between diet and consumer (McMahon and McCarthy, 2016). Theoretically, any SAA and TAA can be used to determine TP, but Phe and Glx are most commonly utilized (e.g., McClelland and Montoya, 2002; Chikaraishi et al., 2009; McMahon et al., 2018), likely because of the relatively higher precision due to better chromatographic separation. Indeed, the peaks for the two AAs in the chromatogram of our instrument showed extremely high precision and clarity. Therefore, the value of TP was based on the δ15N of the two AAs (TPGlx/Phe) and calculated as follows:
where δ15NGlx and δ15NPhe are the measured δ15N values of Glx and Phe, respectively; βGlx/Phe is the isotopic difference between Glx and Phe in the primary producers at the base of a food web; and ΔGlx/Phe is the assumed 15N enrichment in Glx relative to Phe with each trophic transfer from food source to consumer (Chikaraishi et al., 2009, 2010). Based on the latest meta-analysis, βGlx/Phe is 3.3‰ in the food chain of non-vascular primary producers (Ramirez et al., 2021), while ΔGlx/Phe has a value of +7.6‰ (Chikaraishi et al., 2009; Nielsen et al., 2015; McMahon and McCarthy, 2016). Uncertainties of TP using Equation 1 were calculated by the propagation of errors as described by Ohkouchi et al. (2017). In the calculation, the reproducibility of Glx and Phe in this work served as a representation of their analytical errors, and the uncertainties of βGlx/Phe and ΔGlx/Phe were set as 1.8‰ and 1.2‰, respectively (Chikaraishi et al., 2009; Ramirez et al., 2021).
The nitrogen isotope baseline (δ15Nbaseline) of a community was reconstructed based on δ15NPhe (δ15Nbaseline_Phe) as follows:
In the equation, 0.4‰ is the small trophic discrimination factor in Phe for each trophic position with a plausible range of ±0.5‰ (1σ) (Chikaraishi et al., 2009). In our calculation, the standard deviation of the δ15Nbaseline_Phe was estimated by taking into account the errors of δ15N and TP as stated above.
3 Results
3.1 Bulk isotopic composition
The detailed results of bulk isotopic composition for all zoobenthos are given in Supplementary Table S1 and shown in Figure 2A. The δ13Cbulk values of the six deep-sea gorgonians ranged from –20.9 ± 0.0‰ to –18.2 ± 0.6‰, with a mean value of –19.7 ± 1.0‰. Among coral-associated zoobenthos, sponge had the most negative δ13Cbulk values (–24.2 ± 0.2‰), and starfish had the most positive values (–16.0 ± 0.3‰), with an overall average value of –19.1 ± 2.4‰. The δ15Nbulk values of the six deep-sea gorgonians ranged from +11.1 ± 0.1‰ to +13.9 ± 0.0‰, with a mean value of +12.2 ± 0.9‰. The δ15Nbulk values of coral-associated zoobenthos ranged from +9.7 ± 0.1‰ to +18.0 ± 0.0‰, with the minimum value for sea anemone, the maximum value for starfish, and the overall average value being +13.9 ± 2.6‰.
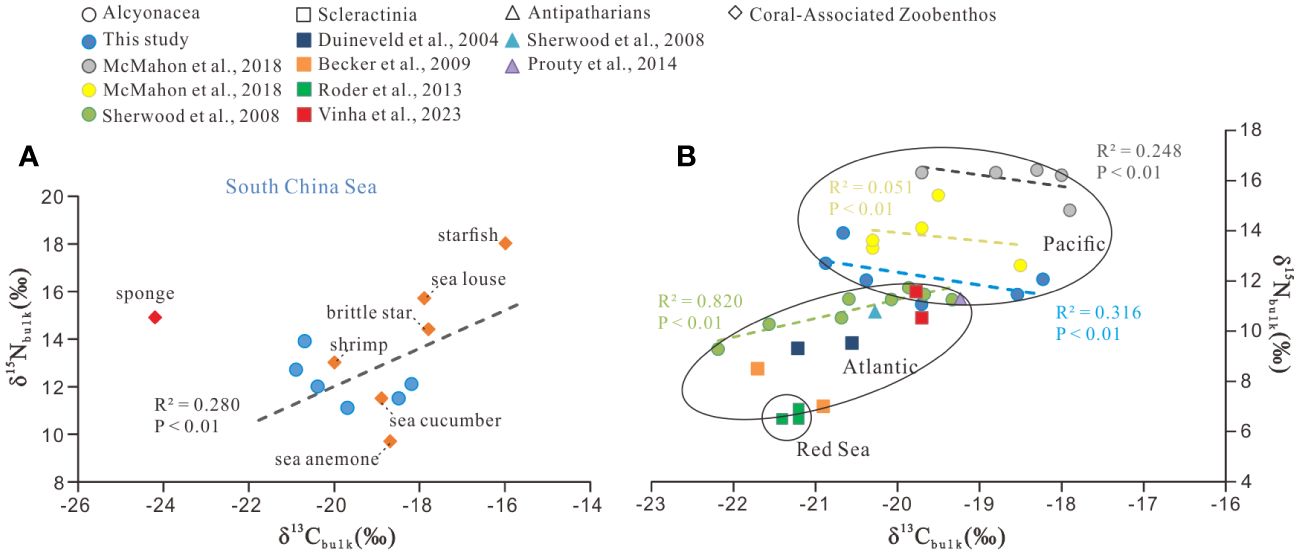
Figure 2 Correlations between the bulk carbon (δ13Cbulk) and nitrogen (δ15Nbulk) stable isotopes. (A) Data of zoobenthos in the SCS (this study) and (B) data comparison of deep-sea corals from the SCS with those from other regions. The different colors in B represent different studies or various genera of corals.
3.2 Amino acids nitrogen isotopes
The δ15N values were only available for 10 AAs in our analysis, as shown in Supplementary Table S2 and Figure 3. Individual AA δ15N values differed significantly among the zoobenthos, with starfish generally having the highest δ15NAAs values (from −10.3‰ to +34.3‰) and brittle star having the lowest δ15NAAs values (from −3.9‰ to +23.8‰), followed by gorgonians. The average δ15NAAs values of the six gorgonians ranged from −5.8 ± 2.7 ‰ (Thr) to +24.2 ± 1.2 ‰ (Ile). For suspended POM, the sample with the highest δ15NAAs values was at 600 m of the site CTD04, ranging from +4.4‰ (Asx) to +13.1‰ (Glx), and that with the lowest values was at 300 m of the CTD04, ranging from −2.6‰ (Thr) to +8.5‰ (Glx). In general, the δ15NAAs values in suspended POM were significantly more negative than those of zoobenthos, except Thr. The δ15N values for some AAs, e.g., Gly, Tyr, and Val, were not measurable due to their low abundances and limited suspended POM sample amounts.
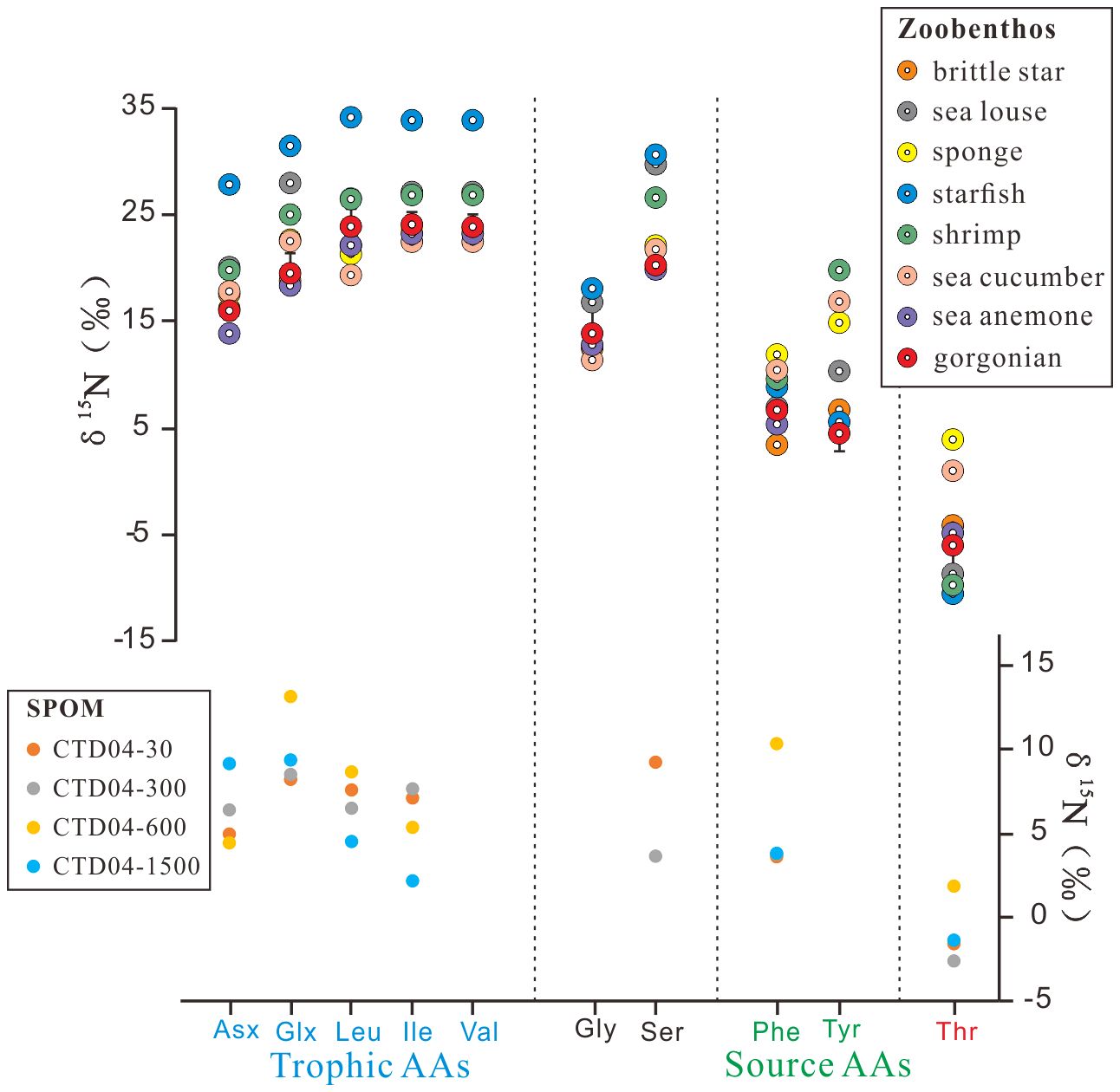
Figure 3 Measured δ15N of individual amino acids (AAs) in zoobenthos and suspended particulate organic material (SPOM). The gorgonian data points represent the average δ15N values of the six gorgonians. Trophic-AA and source-AA refer to the two amino acid categories based on N isotope behaviors during trophic transfer.
Applying Equation 1 to our sample data, the TP values of the six gorgonians ranged between 2.0 ± 0.3 and 2.5 ± 0.4, and those of the other zoobenthos ranged from 2.0 ± 0.3 (sponge) to 3.5 ± 0.5 (starfish). In contrast, the TP values of suspended POM ranged from 0.8 ± 0.3 to 1.7 ± 0.3, with lower values (0.8 ± 0.3 to 1.2 ± 0.3) occurring in upper water layers (30−300 m) (Figure 4). Overall, the uncertainties in TPs were smaller (1σ = 0.2 to 0.4) than the TP values for all samples analyzed in this study and hence did not influence comparisons between species based on TP values.
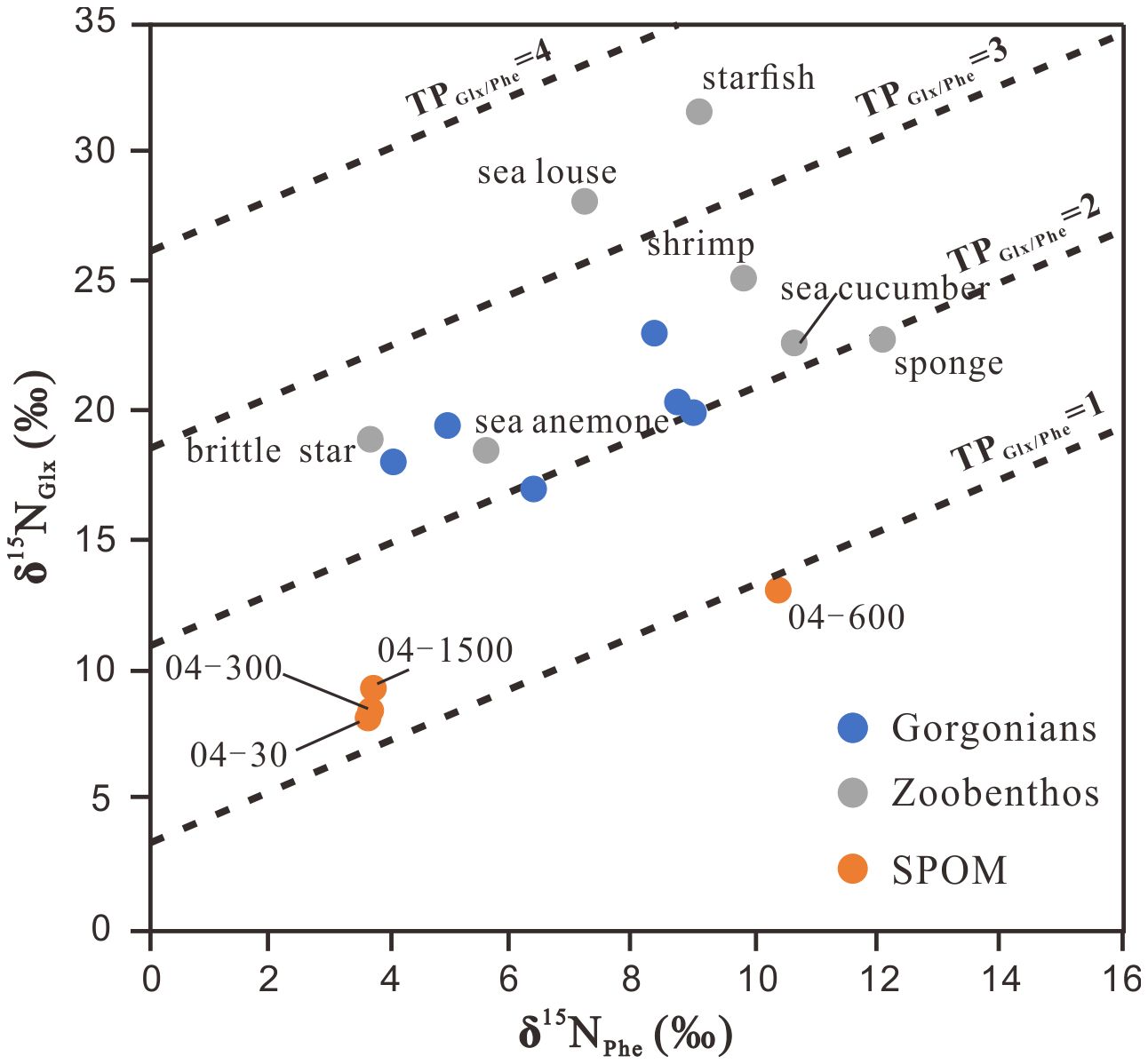
Figure 4 The trophic position (TP) of deep-sea corals, coral-associated zoobenthos and suspended particulate organic matter (SPOM) calculated by δ15N values of glutamate (Glx) and phenylalanine (Phe).
Applying Equation 2 to our zoobenthic δ15NPhe data, the δ15Nbaseline_Phe values ranged from +3.0 ± 0.9‰ (brittle star) to +11.7 ± 0.5‰ (sponge), and the average δ15Nbaseline_Phe value of the six gorgonians was +6.4 ± 2.0‰, varying between +3.4 ± 0.7‰ and +8.5 ± 1.0‰ (Figure 5). δ15Nbaseline_Phe values of suspended POM were about equal to their δ15NPhe values, ranging from +3.5 ± 0.9‰ (CTD04–30m) to +10.4 ± 0.9‰ (CTD04–600m), with an average of +5.3 ± 3.0‰ (Figure 5).
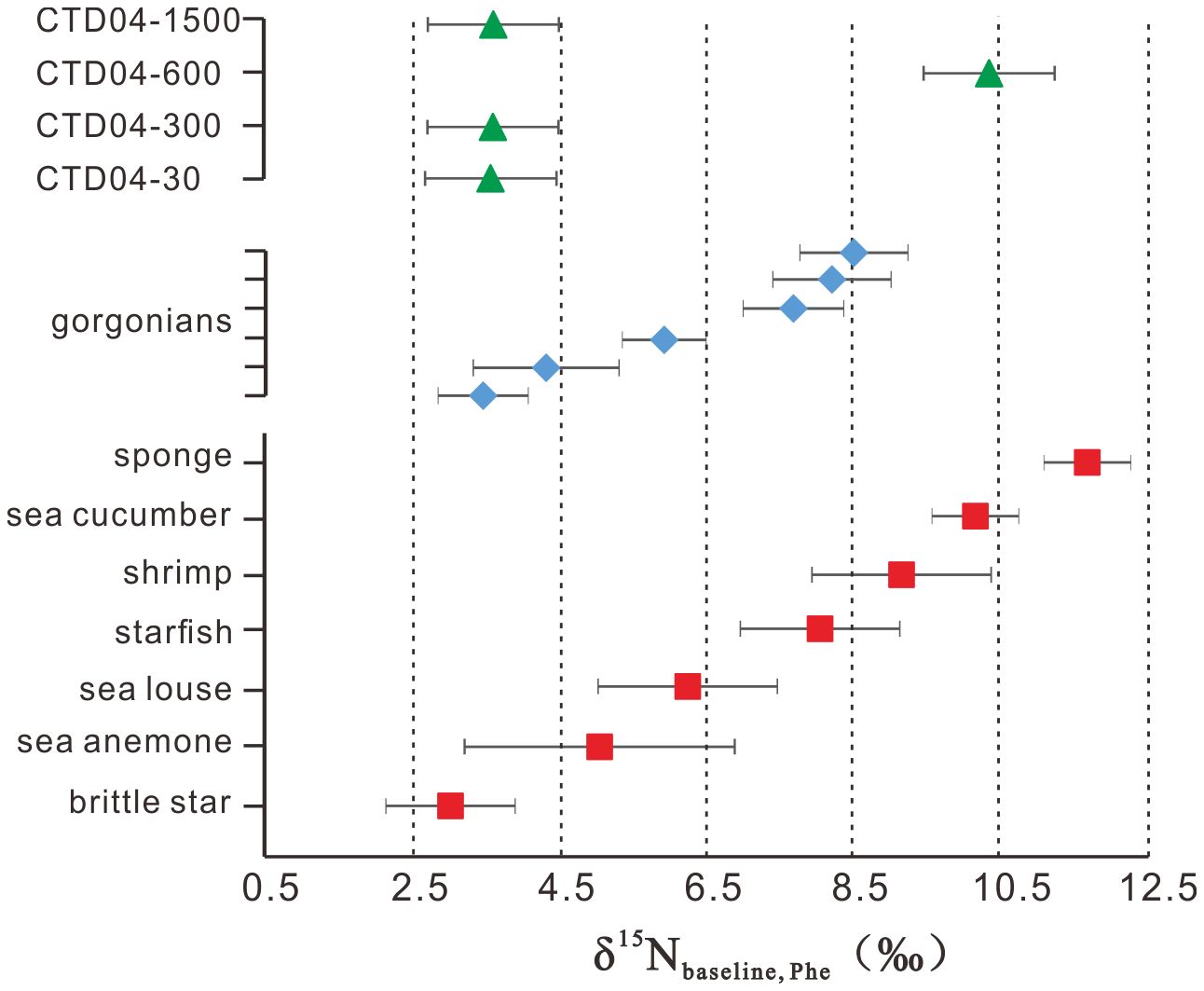
Figure 5 The δ15Nbaseline_Phe value of suspended particulate organic matter (triangle), deep-sea gorgonians (rhombus) and associated zoobenthos (square).
4 Discussion
4.1 Feeding strategies of zoobenthos indicated by bulk isotope characteristics
In a deep-sea community, the relationship between δ13Cbulk and δ15Nbulk may provide clues for food sources for the community, with a strong correlation suggestive of a simple food source (Cartes et al., 2007) and a weak correlation suggestive of a wide array of sources for production (Polunin et al., 2001; Fanelli et al., 2011). A number of δ15Nbulk and δ13Cbulk data have been published for deep-sea corals in the world’s oceans, facilitating a comparison of the data in the SCS with other regions. As can be seen in Figure 2B, δ15Nbulk and δ13Cbulk values in deep-sea corals exhibited clear geographic trends, with generally higher values in the Pacific (δ15Nbulk: +11.1‰ to +16.4‰; δ13Cbulk: –17.9‰ to –20.9‰; McMahon et al., 2018 and this study), lower values in the north Atlantic (δ15Nbulk: +7.0‰ to +11.7‰; δ13Cbulk: –19.2‰ to –22.2‰; Sherwood et al., 2008; Becker et al., 2009; Prouty et al., 2014; Vinha et al., 2023), and the lowest isotopic values in the Red Sea (δ15Nbulk: +6.5‰ to +6.9‰; δ13Cbulk: –21.2‰ to –21.4‰; Roder et al., 2013). The data points from the SCS fall between those from the Northeastern Pacific and the Atlantic (Figure 2B). In the Pacific, the deep-sea soft corals of Primnoa pacifica and Isidella sp. have been indicated to rely on multiple foods, including export production by eukaryotic microalgae, cyanobacteria, and heterotrophic bacteria (McMahon et al., 2018). This is likely the reason why the correlations between δ13Cbulk and δ15Nbulk are negative and weak for P. pacifica (R2 = 0.051) and Isidella sp. (R2 = 0.247) (Figure 2B). In the SCS, six coral data points show a similar picture (R2 = 0.316). On the contrary, the deep-sea soft corals in the northwestern Atlantic exhibit a significant positive δ13Cbulk–δ15Nbulk correlation (R2 = 0.820), suggesting a relatively simple source for them (Sherwood et al., 2008; Figure 2B).
The scattered distribution of the six deep-sea gorgonians isotope values (Figure 2A) is somewhat surprising, as they have been considered to favor fresh, rapidly sinking POM (Sherwood et al., 2005; Van Oevelen et al., 2018; Murray et al., 2019). In this study, the δ13Cbulk values of deep-sea gorgonians ranging from –20.9‰ to –18.2‰ cannot be fully explained by the δ13C value of previously reported sinking POM (–25‰ to –20.8‰; Liu et al., 2007; Zhang et al., 2022) in the SCS, considering the small enrichment of ~0.5–1.0‰ during consumption (Hobson and Welch, 1992; Michener and Schell, 1994). Moreover, the large δ15N offset between these deep-sea gorgonians (from +11.1 to +13.9‰) and sinking POM (< +4‰; Yang et al., 2017) also does not support sinking POM as the sole and direct source of food for deep-sea corals. As a matter of fact, although often considered deposit feeders, deep-sea corals have a variety of feeding strategies, with feeding types ranging from preying on algae-derived POM and zooplankton (Duineveld et al., 2004; Sherwood et al., 2005; Roark et al., 2009; Van Oevelen et al., 2018; Murray et al., 2019; Shen et al., 2021) to relying on symbiotic bacteria in vivo or in vitro (Grover et al., 2014; Kellogg et al., 2016; Lawler et al., 2016; Röthig et al., 2017a; Rincon-Tomas et al., 2019).
In the δ13Cbulk–δ15Nbulk plot (Figure 2A), sponges are distinguished by their most negative δ13Cbulk (–24.2‰) and relatively high δ15Nbulk (+14.9‰). The δ13Cbulk values of sponge are similar to the reported δ13C values of dissolved organic matter (DOM) (–23.5‰ to –20.5‰; Ding et al., 2020) and sinking POM (–25‰ to –20.8‰; Liu et al., 2007; Zhang et al., 2022). Suspended POM have similar concentration weighted mean values of –22.1‰ to –24.0‰ in the top 200 m waters (Liu et al., 2007), but no data are available for deeper waters. According to Yang et al. (2017), suspended and sinking particles in the SCS contain very different δ15N values, showing lower δ15N in sinking POM (+3.2‰ at 3000–4000 m) but higher δ15N in suspended POM (+8.2‰) below 500 m. The δ15N value of DOM in the deep SCS also lacks data and was hypothesized to be as high as that of suspended POM (Yang et al., 2017). Therefore, DOM is likely a major food source for sponges, which is consistent with their feeding strategies. Moreover, they can transfer DOM to POM as food for corals and associated fauna through a sponge loop (de Goeij et al., 2013; Rix et al., 2016, 2018; Bart et al., 2021). Excluding sponge, the δ13Cbulk–δ15Nbulk data of other zoobenthos exhibit a weak correlation (R2 = 0.280) (Figure 2A), suggesting that in addition to possible common food sources, there are also diverse sources of their own. Nevertheless, starfish and sea louse exhibited the most positive values in δ13Cbulk and δ15Nbulk among these zoobenthos, suggesting the two are at the top of the trophic hierarchy of this deep-sea community. Indeed, starfish are carnivorous and can consume coral’s detrital waste or directly feed on coral tissue (Larkum, 1988; Caballes et al., 2016), and the sea louse is parasitic (Leonardi et al., 2020) and is usually found to live on other animals. Other zoobenthos showing intermediate δ13Cbulk values and relatively lower δ15Nbulk values are generally consistent with their feeding strategies. For example, the deep-sea anemone is mixotrophic chemosynthesis (Goffredi et al., 2021), the deep-sea shrimp is a plankton predator (Cartes et al., 2014), the brittle star is omnivorous (Bart et al., 2021), and the sea cucumber is filter feeding (Billett, 1991). Similarly, Iken et al. (2001) studied trophic food webs in the deep sea and observed large overlaps in isotopic values between the different food web members, which were thought to be a result of competition for and adaptation to limited food availability in the deep-sea environments.
Fundamentally, the ultimate food sources are autotrophs, including photosynthetic phytoplankton that are the main source of organic matter exported from the euphotic layer and various chemoautotrophs in the water column. In addition to the classic, well-known Calvin–Benson–Bassham (CBB) cycle, several minor autotrophic carbon fixation mechanisms have also been identified, all of which have different carbon isotope fractionation during the carbon fixation process (Berg et al., 2010; Ward and Shih, 2019). Although the production by these minor autotrophic carbon fixation mechanisms in the SCS is unknown, we tentatively assess their contribution as food sources for gorgonians and other zoobenthos in this study. A Bayesian stable isotope mixing model with five sources additional to the CBB machanism, including the reductive citric acid cycle (rTCA), Wood–Ljungdahl pathway (or the reductive Acetyl-CoA, rAcCoA), 3-Hydroxypropionate bicycle (3HP), 3-Hydroxypropionate–4-hydroxybutyrate cycle (3HP/4HB) and Dicarboxylate–4-hydroxybutyrate cycle (DC/4HB), was run using the R software (R 4.3.3) package MixSIAR (Ferreira de Moraes and Henry-Silva, 2018). Carbon isotope fractionations of these mechanisms against DIC (–0.61‰ to +0.05‰ in the SCS; Ding et al., 2020) are set as follows except for 3HP/4HB according to Berg et al. (2010): –25 ± 5‰ for CBB, –7 ± 5‰ for rTCA, –30‰ for rAcCoA, –13.1 ± 0.6‰ for 3HP and –2 ± 2‰ for DC/4HB. In deep sea environment the ubiquitous Thaumarchaea likely dominate the 3HP/4HB pathway with carbon isotope fractionation ~ –19 ± 1‰ (Pearson et al., 2019). The MixSIAR model results show that the food sources of deep-sea gorgonians mainly originate from the CBB (56.8% ± 14.7%), with the 3HP/4HB (15.8% ± 15.4%), the 3HP (9.0% ± 9.2%), the rAcCoA (8.2% ± 8.4%), the rTCA (5.4% ± 5.5%) and the DC/4HB (4.9% ± 5.0%) as secondary sources (Figure 6). Other zoobenthos also have similar food source contributions (Figure 6, detail see in Supplementary Table S3). Because photoautotrophs, dominated by phytoplankton in the marine euphoic zone, mainly use the CBB cycle, these results demonstrate that the deep-sea coral community in this study relies mainly on the export production of surface photoautotrophs, with other chemoautotrophs as supplementary.
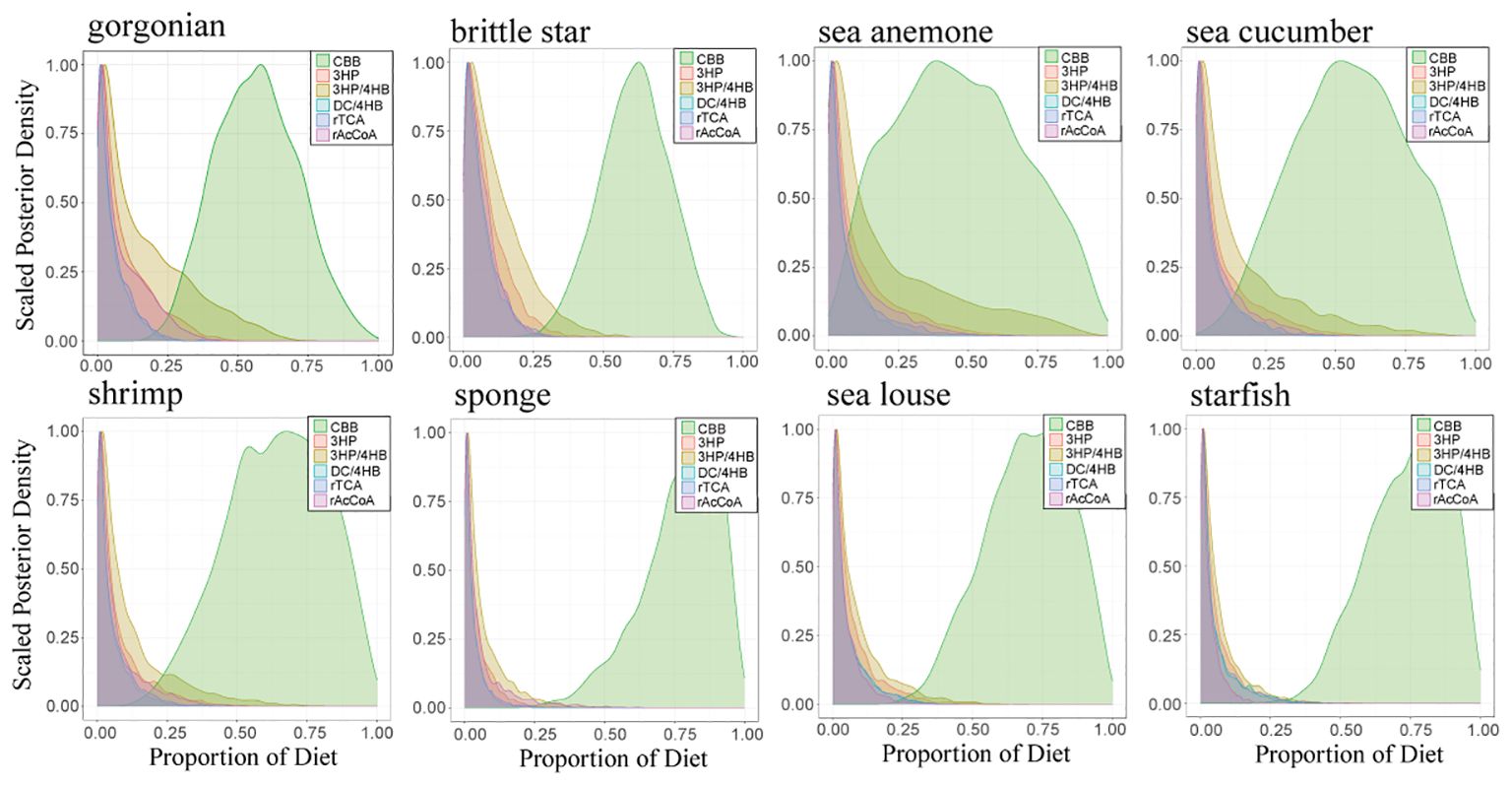
Figure 6 Probability distribution density diagram of contributions from the six pathways of autotrophic carbon fixation: the Calvin–Benson–Bassham cycle (CBB), the reductive Acetyl-CoA or Wood-Ljungdahl pathway (rAcCoA), the reductive tricarboxylic acid cycle (rTCA), the 3-hydroxypropionate bicycle (3HP), the 3-hydroxypropionate/4-hydroxybutyrate cycle (3HP/4HB), and the dicarboxylate/4-hydroxybutyrate cycle (DC/4HB), based on the MixSIAR model.
4.2 δ15N baseline of the deep-sea coral community
Organisms draw their nitrogen from the base of the food web, the δ15N value of which, i.e., δ15Nbaseline, is essential to determine if variation in the δ15N of an organism reflects changes in food web structure, or just a variation in the δ15Nbaseline (Post, 2002). However, δ15Nbaseline may vary due to differences in the isotopic ratio of nitrogen available for uptake at the base of the food web and through variable expression of fractionation during uptake (Post, 2002; Woodcock et al., 2012). Compound-specific δ15N analysis is preferred for the δ15Nbaseline determination because the negligible 15N enrichment in SAAs, like Phe, allows the δ15Nbaseline to be obtained despite the general trophic enrichment by heterotrophic organisms (McCarthy et al., 2007; McMahon and McCarthy, 2016; Ohkouchi et al., 2017). If primary producers at the sea surface are the only basal source of nitrogen, then δ15Nbaseline_Phe should roughly correspond to the mean δ15N value of inorganic nitrogen in oligotrophic tropical and temperate regions (e.g., Sherwood et al., 2011, 2014; Xing et al., 2020).
In the SCS, the δ15N values of thermocline nitrate and the surface primary producers are steady at about +5.0‰, and those for sinking POM keep in a narrow range of +3.2‰ to +3.6‰ below 1000 m in the SCS (Yang et al., 2017, 2018). These data, however, cannot explain the overall δ15Nbaseline_Phe values of the deep-sea coral community, which range from +3.0 ± 0.9‰ to +11.7 ± 0.5‰, hence reflecting various N sources in addition to phytoplankton. For the suspended POM, the large changes in δ15Nbaseline_Phe values with different water depths (Figure 5) indicate that they have undergone complex processes, including the degradation and addition of new AAs produced from chemoautotrophs when they remain in the water column for a long residence time (Liu et al., 2007; Hong et al., 2021). Microbial degradation may hydrolyze the labile proteins in the POM, resulting in 15N-enriched residual AAs, regardless of SAAs and TAAs (Hannides et al., 2013). Chemoautotrophs may have distinct δ15NPhe values caused by the diversity of Phe metabolic pathways among microbes (Macko et al., 1987; Maki et al., 2014; Yamaguchi et al., 2017). For the zoobenthos, the wide δ15Nbaseline_Phe value range is consistent with their diverse feeding strategies discussed above. These additional bases for the food web, although not clearly known, could be related to microbes that reside in POM or are symbiotic with zoobenthos. Indeed, previous studies have suggested that deep-sea gorgonians in a deep-sea environment with limited food supply may rely on symbiotic bacteria to have a complete nitrogen cycle that ensures adequate nitrogen sources (Kellogg et al., 2016; Lawler et al., 2016; Röthig et al., 2017a). These symbiotic bacteria can parasitize on the surface mucosa of gorgonians and possibly outside the gorgonians, where the gorgonians feed by ingesting the bacteria or the metabolites they produce. We speculate that these symbiotic microbes may be characterized by unique δ15NPhe values, though not well constrained, representing diversity of the δ15N baseline at the species or individual level, despite being collected from a single study site. Thus, together with the δ13Cbulk–δ15Nbulk results, we believe that these symbiotic microbes may indeed be an important food candidate for the deep-sea coral community.
4.3 Trophic position of deep-sea zoobenthos
The trophic discrimination factor (TDF), which is also frequently used to estimate the TPs, varies in a typical range from 3‰ to 4‰ in terms of δ15Nbulk between predator and prey in marine environments (McCutchan et al., 2003; del Rio et al., 2009). However, due to the large uncertainty in the estimated TDF, there are some limitations to the application of this method (Ohkouchi et al., 2017). The differences in δ15Nbulk values between deep-sea gogornias (+12.2 ± 0.9‰) and other zoobenthos in this study are less than 3‰, except for starfish (+18.0 ± 0.0‰) and sea louse (+15.7 ± 0.1‰). A comparison of these differences would seem to point to a similar TP, with the exception of the sea louse and starfish, which are at higher TPs. However, the potentially various N sources due to the aforementioned multiple food sources make it difficult to use δ15Nbulk values to estimate TPs for individual organisms in this study. Assuming that the N provided by sinking POM (δ15N = +3.5‰; Yang et al., 2017) is the only initial N source in the SCS, the deep-sea gorgonians would be at TP of ~4, sea louse at ~5, and starfish at ~6. Such higher TPs for animals in an oligotrophic deep-sea environment are unrealistic, because even in a common food web, apex predators’ TPs are no more than 5 (Bonhommeau et al., 2013), and in a deep-sea food web, they would be less than 3 (Kercher and Shugart, 1975).
The above difficulties in estimating TPs with δ15Nbulk can be partly solved by compound-specific δ15N of AAs. The TP defined by the Glx and Phe δ15N values has been widely used to understand food web structure (Xing et al., 2020) because it can constrain both trophic changes in the δ15N value and baseline variation within a single organism (McCarthy et al., 2007; Popp et al., 2007; Ohkouchi et al., 2017). Although not strictly comparable to the TP of a single organism, the apparent TP values of POM may reflect the relative balance of autotrophic and heterotrophic sources in a detrital pool (Yamaguchi and McCarthy, 2018). In this study, the resultant lower TPs (0.9 ± 0.3 to 1.2 ± 0.3) of upper water (30−600 m) suspended POM indicate their main phytoplankton origin, whereas elevated TPs (1.3 ± 0.3) of suspended POM at the deep (1500 m) are consistent with previous results for suspended particles in mesopelagic waters (McCarthy et al., 2007; Yamaguchi and McCarthy, 2018) and suggest additional inputs other than microalgae during downward movement (Figure 4). Among the zoobenthos, sponge has the highest δ15NPhe values (12.1 ± 0.2‰) and the lowest TP (2.0 ± 0.3), suggesting its food, mainly deep-sea DOM as discussed above, has a complex source of N, not just from euphotic phytoplankton. The δ15NPhe values show some overlap between the gorgonians and suspended POM, and the TPs of the gorgonians range from 2.0 ± 0.3 to 2.5 ± 0.4 (Figure 4), indicating the suspended POM is a likely N source. Sponge-derived POM (Rix et al., 2016, 2018; Bart et al., 2021) and nitrogen-processing symbiotic microbes (Kellogg et al., 2016; Lawler et al., 2016; Röthig et al., 2017a) could be additional sources that may elevate the TPs of gorgonians. Similar phenomena could also occur for brittle star, sea anemone, shrimp, and sea cucumber, which have similar ranges of δ15NPhe and TP values between 2.2 ± 0.3 and 2.6 ± 0.4 (Figures 3, 4). The sea louse and starfish, which are the same as in the bulk isotope analysis, have the highest TPs, 3.3 ± 0.4 and 3.5 ± 0.5, respectively, indicating they are secondary consumers feeding on primary consumers. Sea lice are parasitic organisms that derive nutrients from their hosts, indicating a likely association with primary consumers exhibiting TP values ranging between 2 and 3. Starfish are carnivores, and in the surface ocean, they are often seen in coral reef areas feeding on corals (Buhl-Mortensen and Mortensen, 2004). Coral tissue is often found in the stomach contents of deep-sea starfish (Gale et al., 2013; Birk et al., 2018), which likely also happens at the sea floor in the deep SCS.
5 Conclusion
In the present study, the δ13Cbulk and δ15Nbulk and compound-specific amino acids δ15N are applied to trace the trophic structure of a deep-sea coral community in the SCS. We illustrate the trophic trends of various zoobenthos species occupying trophic positions between 2.0 ± 0.3 and 3.5 ± 0.5, as well as a wide range of isotopically diverse primary producers with δ15NPhe-derived baseline values ranging from +3.0 ± 0.9‰ to +11.7 ± 0.5‰. The δ13Cbulk–δ15Nbulk correlation of gorgonians and MixSIAR model analysis suggest a variety of food sources, probably microbes in the water column and/or symbiotic with zoobenthos, in addition to phytoplankton-derived POM. These results suggest that the food sources of the deep-sea coral community in the oligotrophic SCS are more diverse than just the primary producers in the surface ocean, and the deep-sea coral likely is an omnivorous or opportunistic feeder (Dodds et al., 2009; Mueller et al., 2014). Further work is needed to refine carbon and nitrogen sources and cycling in the community, which is critical to understanding and protecting such a fragile, dark ecosystem.
Data availability statement
The original contributions presented in the study are included in the article/Supplementary Material. Further inquiries can be directed to the corresponding author.
Ethics statement
Ethical approval was not required for the study involving animals in accordance with the local legislation and institutional requirements because the deep-sea corals and other benthic organisms collected in this study do not raise local ethical concerns.
Author contributions
ZL: Conceptualization, Formal Analysis, Methodology, Visualization, Writing – original draft. LC: Data curation, Investigation, Writing – review & editing. GJ: Conceptualization, Funding acquisition, Methodology, Writing – review & editing.
Funding
The author(s) declare financial support was received for the research, authorship, and/or publication of this article. This work was supported by Natural Science Foundation of China (Grant No. 42030504).
Acknowledgments
The authors thank the scientific group and the captain and crew aboard the R/V ShenhaiYongshi for organizing for the faunal sampling in this study. Two reviewers are appreciated for their helpful comments.
Conflict of interest
The authors declare that the research was conducted in the absence of any commercial or financial relationships that could be construed as a potential conflict of interest.
Publisher’s note
All claims expressed in this article are solely those of the authors and do not necessarily represent those of their affiliated organizations, or those of the publisher, the editors and the reviewers. Any product that may be evaluated in this article, or claim that may be made by its manufacturer, is not guaranteed or endorsed by the publisher.
Supplementary material
The Supplementary Material for this article can be found online at: https://www.frontiersin.org/articles/10.3389/fmars.2024.1399814/full#supplementary-material
References
Arthur K. E., Kelez S., Larsen T., Choy C. A., Popp B. N. (2014). Tracing the biosynthetic source of essential amino acids in marine turtles using delta C-13 fingerprints. Ecology 95, 1285–1293. doi: 10.1890/13–0263.1
Bart M. C., Hudspith M., Rapp H. T., Verdonschot P. F. M., De Goeij J. M. (2021). A deep-sea sponge loop? Sponges transfer dissolved and particulate organic carbon and nitrogen to associated fauna. Front. Mar. Sci. 8. doi: 10.3389/fmars.2021.604879
Becker E. L., Cordes E. E., Macko S. A., Fisher C. R. (2009). Importance of seep primary production to Lophelia pertusa and associated fauna in the Gulf of Mexico. Deep-Sea Res. Part I-Oceanogr. Res. Pap. 56, 786–800. doi: 10.1016/j.dsr.2008.12.006
Berg I. A., Kockelkorn D., Ramos-Vera W. H., Say R. F., Zarzycki J., Huegler M., et al. (2010). Autotrophic carbon fixation in archaea. Nat. Rev. Microbiol. 8, 447–460. doi: 10.1038/nrmicro2365
Birk M. H., Blicher M. E., Garm A. (2018). Deep-sea starfish from the Arctic have well-developed eyes in the dark. P. R. Soc B-Biol. Sci. 285, 20172743. doi: 10.1098/rspb.2017.2743
Bonhommeau S., Dubroca L., Le Pape O., Barde J., Kaplan D. M., Chassot E., et al. (2013). Eating up the world’s food web and the human trophic level. Proc. Natl. Acad. Sci. U. S. A. 110, 20617–20620. doi: 10.1073/pnas.1305827110
Caballes C. F., Pratchett M. S., Kerr A. M., Rivera-Posada J. A. (2016). The role of maternal nutrition on oocyte size and quality, with respect to early larval development in the coral-eating starfish, Acanthaster planci. PloS One 11 (6), e0158007. doi: 10.1371/journal.pone.0158007
Cartes J. E., Fanelli E., Kapiris K., Bayhan Y. K., Ligas A., López-Pérez C., et al. (2014). Spatial variability in the trophic ecology and biology of the deep-sea shrimp Aristaeomorpha foliacea in the Mediterranean Sea. Deep-Sea Res. Part I-Oceanogr. Res. Pap 87, 1–13. doi: 10.1016/j.dsr.2014.01.006
Cartes J. E., Huguet C., Parra S., Sanchez F. (2007). Trophic relationships in deep-water decapods of Le Danois bank (Cantabrian Sea, NE Atlantic): Trends related with depth and seasonal changes in food quality and availability. Deep-Sea Res. Part I-Oceanogr. Res. Pap. 54, 1091–1110. doi: 10.1016/j.dsr.2007.04.012
Chikaraishi Y., Ogawa N. O., Kashiyama Y., Takano Y., Suga H., Tomitani A., et al. (2009). Determination of aquatic food-web structure based on compound-specific nitrogen isotopic composition of amino acids. Limnol. Oceanogr. Meth. 7, 740–750. doi: 10.4319/lom.2009.7.740
Chikaraishi Y., Ogawa N., Ohkouchi N. (2010). Further evaluation of the trophic level estimation based on nitrogen isotopic composition of amino acids. Earth Life Isotopes 3, 37–51.
Corr L. T., Berstan R., Evershed R. P. (2007a). Optimisation of derivatisation procedures for the determination of delta C-13 values of amino acids by gas chromatography/combustion/isotope ratio mass spectrometry. Rapid Commun. Mass Spectrom. 21, 3759–3771. doi: 10.1002/rcm.3252
Corr L. T., Berstan R., Evershed R. P. (2007b). Development of N-acetyl methyl ester derivatives for the determination of delta(13)C values of amino acids using gas chromatography-combustion-isotope ratio mass spectrometry. Anal. Chem. 79, 9082–9090. doi: 10.1021/ac071223b
de Goeij J. M., van Oevelen D., Vermeij M. J. A., Osinga R., Middelburg J. J., de Goeij A. F. P. M., et al. (2013). Surviving in a marine desert: The sponge loop retains resources within coral reefs. Science 342, 108–110. doi: 10.1126/science.1241981
del Rio C. M., Wolf N., Carleton S. A., Gannes L. Z. (2009). Isotopic ecology ten years after a call for more laboratory experiments. Biol. Rev. 84, 91–111. doi: 10.1111/j.1469–185X.2008.00064.x
Ding L., Qi Y. Z., Shan S., Ge T. T., Luo C. L., Wang X. C. (2020). Radiocarbon in dissolved organic and inorganic carbon of the South China Sea. J. Geophys. Res.-Oceans 125. doi: 10.1029/2020jc016073
Dodds L. A., Black K. D., Orr H., Roberts J. M. (2009). Lipid biomarkers reveal geographical differences in food supply to the cold-water coral Lophelia pertusa (Scleractinia). Mar. Ecol.-Prog. Ser. 397, 113–124. doi: 10.3354/meps08143
Duineveld G. C. A., Lavaleye M. S. S., Berghuis E. M. (2004). Particle flux and food supply to a seamount cold-water coral community (Galicia Bank, NW Spain). Mar. Ecol.-Prog. Ser. 277, 13–23. doi: 10.3354/meps277013
Fanelli E., Papiol V., Cartes J. E., Rumolo P., Brunet C., Sprovieri M. (2011). Food web structure of the epibenthic and infaunal invertebrates on the Catalan slope (NW Mediterranean): Evidence from delta C-13 and delta N-15 analysis. Deep-Sea Res. Part I-Oceanogr. Res. Pap. 58, 98–109. doi: 10.1016/j.dsr.2010.12.005
Ferreira de Moraes C. R., Henry-Silva G. G. (2018). Mixing models and stable isotopes as tools for research on feeding aquatic organisms. Cienc. Rural 48 (7), e20160101. doi: 10.1590/0103–8478cr20160101
Gale K. S. P., Hamel J. F., Mercier A. (2013). Trophic ecology of deep-sea Asteroidea (Echinodermata) from eastern Canada. Deep-Sea Res. Pt. I. 80, 25–36. doi: 10.1016/j.dsr.2013.05.016
Glynn D. S., McMahon K. W., Guilderson T. P., McCarthy M. D. (2019). Major shifts in nutrient and phytoplankton dynamics in the North Pacific Subtropical Gyre over the last 5000 years revealed by high-resolution proteinaceous deep-sea coral delta N-15 and delta C-13 records. Earth Planet. Sci. Lett. 515, 145–153. doi: 10.1016/j.epsl.2019.03.014
Glynn D. S., McMahon K. W., Sherwood O. A., Guilderson T. P., McCarthy M. D. (2022). Investigating preservation of stable isotope ratios in subfossil deep-sea proteinaceous coral skeletons as paleo-recorders of biogeochemical information over multimillennial timescales. Geochim. Cosmochim. Ac. 338, 264–277. doi: 10.1016/j.gca.2022.09.023
Goffredi S. K., Motooka C., Fike D. A., Gusmao L. C., Tilic E., Rouse G. W., et al. (2021). Mixotrophic chemosynthesis in a deep-sea anemone from hydrothermal vents in the Pescadero Basin, Gulf of California. BMC Biol. 19 , 8. doi: 10.1186/s12915–020-00921–1
Grover R., Ferrier-Pages C., Maguer J.-F., Ezzat L., Fine M. (2014). Nitrogen fixation in the mucus of Red Sea corals. J. Exp. Biol. 217, 3962–3963. doi: 10.1242/jeb.111591
Hannides C. C. S., Popp B. N., Choy C. A., Drazen J. C. (2013). Midwater zooplankton and suspended particle dynamics in the North Pacific Subtropical Gyre: A stable isotope perspective. Limnol. Oceanogr. 58, 1931–1946. doi: 10.4319/lo.2013.58.6.1931
Hobson K. A., Welch H. E. (1992). Determination of trophic relationships within a high Arctic marine food web using delta C-13 and delta N-15 analysis. Mar. Ecol.-Prog. Ser. 84, 9–18. doi: 10.3354/meps084009
Hong Q., Peng S., Zhao D., Cai P. (2021). Cross-shelf export of particulate organic carbon in the northern South China Sea: Insights from a 234Th mass balance. Prog. Oceanogr. 193, 102532. doi: 10.1016/j.pocean.2021.102532
Hovland M., Risk M. (2003). Do Norwegian deep-water coral reefs rely on seeping fluids? Mar. Geol. 198, 83–96. doi: 10.1016/s0025–3227(03)00096–3
Husebo A., Nottestad L., Fossa J. H., Furevik D. M., Jorgensen S. B. (2002). Distribution and abundance of fish in deep-sea coral habitats. Hydrobiologia 471, 91–99. doi: 10.1023/a:1016549203368
Iken K., Brey T., Wand U., Voigt J., Junghans P. (2001). Food web structure of the benthic community at the Porcupine Abyssal Plain (NE Atlantic): a stable isotope analysis. Prog. Oceanogr. 50, 383–405. doi: 10.1016/s0079–6611(01)00062–3
Judd A., Hovland M. (2007). Seabed fluid flow: The impact on geology, biology and the marine environment. Cambridge University Press. doi: 10.1017/CBO9780511535918
Kellogg C. A., Ross S. W., Brooke S. D. (2016). Bacterial community diversity of the deep-sea octocoral Paramuricea placomus. Peerj 4, 25. doi: 10.7717/peerj.2529
Kercher J. R., Shugart H. H. (1975). Trophic structure, effective trophic position, and connectivity in food webs. Am. Nat. 109, 191–206. doi: 10.1086/282986
Larkum A. W. D. (1988). High-rates of nitrogen-fixation on coral skeletons after predation by the crown of thorns starfish Acanthaster planci. Mar. Biol. 97, 503–506. doi: 10.1007/bf00391046
Larsen T., Taylor D. L., Leigh M. B., O’Brien D. M. (2009). Stable isotope fingerprinting: a novel method for identifying plant, fungal, or bacterial origins of amino acids. Ecology 90, 3526–3535. doi: 10.1890/08–1695.1
Larsen T., Ventura M., Andersen N., O’Brien D. M., Piatkowski U., McCarthy M. D. (2013). Tracing carbon sources through aquatic and terrestrial food webs using amino acid stable isotope fingerprinting. PloS One 8, 9. doi: 10.1371/journal.pone.0073441
Lawler S. N., Kellogg C. A., France S. C., Clostio R. W., Brooke S. D., Ross S. W. (2016). Coral-associated bacterial diversity is conserved across two deep-sea Anthothela species. Front. Microbiol. 7. doi: 10.3389/fmicb.2016.00458
Leonardi M. S., Crespo J. E., Soto F. A., Vera R. B., Rua J. C., Lazzari C. R. (2020). Under pressure: the extraordinary survival of seal lice in the deep sea. J. Exp. Biol. 223 (17), jeb226811. doi: 10.1242/jeb.226811
Li J. R., Wang P. X. (2019). Discovery of deep-water bamboo coral forest in the South China Sea. Sci. Rep. 9, 5. doi: 10.1038/s41598–019-51797–3
Liu K. K., Kao S. J., Hu H. C., Chou W. C., Hung G. W., Tseng C. M. (2007). Carbon isotopic composition of suspended and sinking particulate organic matter in the northern South China Sea - From production to deposition. Deep-Sea Res. Part II-Top. Stud. Oceanogr. 54, 1504–1527. doi: 10.1016/j.dsr2.2007.05.010
Macko S. A., Fogel Estep M. L., Hare P. E., Hoering T. C. (1987). Isotopic fractionation of nitrogen and carbon in the synthesis of amino acids by microorganisms. Chem. Geol. 65, 79–92. doi: 10.1016/0168–9622(87)90064–9
Maki K., Ohkouchi N., Chikaraishi Y., Fukuda H., Miyajima T., Nagata T. (2014). Influence of nitrogen substrates and substrate C:N ratios on the nitrogen isotopic composition of amino acids from the marine bacterium Vibrio harveyi. Geochim. Cosmochim. Acta 140, 521–530. doi: 10.1016/j.gca.2014.05.052
McCarthy M. D., Benner R., Lee C., Fogel M. L. (2007). Amino acid nitrogen isotopic fractionation patterns as indicators of heterotrophy in plankton, particulate, and dissolved organic matter. Geochim. Cosmochim. Ac. 71, 4727–4744. doi: 10.1016/j.gca.2007.06.061
McClelland J. W., Montoya J. P. (2002). Trophic relationships and the nitrogen isotopic composition of amino acids in plankton. Ecology 83, 2173–2180. doi: 10.1890/0012–9658(2002)083[2173:Tratni]2.0.Co;2
McCutchan J. H., Lewis W. M., Kendall C., McGrath C. C. (2003). Variation in trophic shift for stable isotope ratios of carbon, nitrogen, and sulfur. Oikos 102, 378–390. doi: 10.1034/j.1600–0706.2003.12098.x
McMahon K. W., McCarthy M. D. (2016). Embracing variability in amino acid delta N-15 fractionation: mechanisms, implications, and applications for trophic ecology. Ecosphere 7 (12), e01511. doi: 10.1002/ecs2.1511
McMahon K. W., McCarthy M. D., Sherwood O. A., Larsen T., Guilderson T. P. (2015). Millennial- scale plankton regime shifts in the subtropical North Pacific Ocean. Science 350, 1530–1533. doi: 10.1126/science.aaa9942
McMahon K. W., Williams B., Guilderson T. P., Glynn D. S., McCarthy M. D. (2018). Calibrating amino acid delta C-13 and delta N-15 offsets between polyp and protein skeleton to develop proteinaceous deep-sea corals as paleoceanographic archives. Geochim. Cosmochim. Ac. 220, 261–275. doi: 10.1016/j.gca.2017.09.048
Michener R. H., Schell D. M. (1994). Stable isotope ratios as tracers in marine aquatic food webs. Stable Isotopes Ecol. Environ. ence. 138–158.
Mincks S. L., Smith C. R., Jeffreys R. M., Sumida P. Y. G. (2008). Trophic structure on the West Antarctic Peninsula shelf: Detritivory and benthic inertia revealed by delta C-13 and delta N-15 analysis. Deep-Sea Res. Part II-Top. Stud. Oceanogr. 55, 2502–2514. doi: 10.1016/j.dsr2.2008.06.009
Mortensen P. B., Hovland M. T., Fossa J. H., Furevik D. M. (2001). Distribution, abundance and size of Lophelia pertusa coral reefs in mid-Norway in relation to seabed characteristics. J. Mar. Biol. Assoc. U.K. 81, 581–597. doi: 10.1017/s002531540100426x
Mueller C. E., Larsson A. I., Veuger B., Middelburg J. J., van Oevelen D. (2014). Opportunistic feeding on various organic food sources by the cold-water coral Lophelia pertusa. Biogeosciences 11, 123–133. doi: 10.5194/bg-11–123-2014
Murray F., De Clippele L. H., Hiley A., Wicks L., Roberts J. M., Hennige S. (2019). Multiple feeding strategies observed in the cold-water coral Lophelia pertusa. J. Mar. Biol. Assoc. U.K. 99, 1281–1283. doi: 10.1017/s0025315419000298
Nielsen J. M., Popp B. N., Winder M. (2015). Meta-analysis of amino acid stable nitrogen isotope ratios for estimating trophic position in marine organisms. Oecologia 178, 631–642. doi: 10.1007/s00442–015-3305–7
O’Connell T. C. (2017). ‘Trophic’ and ‘source’ amino acids in trophic estimation: a likely metabolic explanation. Oecologia 184, 317–326. doi: 10.1007/s00442–017-3881–9
Ohkouchi N., Chikaraishi Y., Close H. G., Fry B., Larsen T., Madigan D. J., et al. (2017). Advances in the application of amino acid nitrogen isotopic analysis in ecological and biogeochemical studies. Org. Geochem. 113, 150–174. doi: 10.1016/j.orggeochem.2017.07.009
Pearson A., Hurley S. J., Elling F. J., Wilkes E. B. (2019). CO2-dependent carbon isotope fractionation in Archaea, Part I: Modeling the 3HP/4HB pathway. Geochim. Cosmochim. Ac. 261, 368–382. doi: 10.1016/j.gca.2019.06.042
Polunin N. V. C., Morales-Nin B., Pawsey W. E., Cartes J. E., Pinnegar J. K., Moranta J. (2001). Feeding relationships in Mediterranean bathyal assemblages elucidated by stable nitrogen and carbon isotope data. Mar. Ecol. Prog. Ser. 220, 13–23.
Popp B. N., Graham B. S., Olson R. J., Hannides C. C. S., Lott M. J., Lopez-Ibarra G. A., et al. (2007). Insight into the trophic ecology of yellowfin tuna, Thunnus albacares, from compound-specific nitrogen isotope analysis of proteinaceous amino acids. Stable Isotopes as Indic. Ecol. Change 1, 173–190. doi: 10.1016/S1936–7961(07)01012–3
Post D. M. (2002). Using stable isotopes to estimate trophic position: Models, methods, and assumptions. Ecology 83, 703–718. doi: 10.2307/3071875
Prouty N. G., Roark E. B., Koenig A. E., Demopoulos A. W. J., Batista F. C., Kocar B. D., et al. (2014). Deep-sea coral record of humanimpact on watershed quality in the Mississippi River Basin. Global Biogeochem. Cy. 28, 29–43. doi: 10.1002/2013gb004754
Ramirez M. D., Besser A. C., Newsome S. D., McMahon K. W. (2021). Meta-analysis of primary producer amino acid delta N-15 values and their influence on trophic position estimation. Methods Ecol. Evol. 12, 1750–1767. doi: 10.1111/2041–210x.13678
Rincon-Tomas B., Duda J. P., Somoza L., Gonzalez F. J., Schneider D., Medialdea T., et al. (2019). Cold-water corals and hydrocarbon-rich seepage in Pompeia Province (Gulf of Cadiz) - living on the edge. Biogeosciences 16, 1607–1627. doi: 10.5194/bg-16–1607-2019
Rix L., de Goeij J. M., Mueller C. E., Struck U., Middelburg J. J., van Duyl F. C., et al. (2016). Coral mucus fuels the sponge loop in warm- and cold-water coral reef ecosystems. Sci. Rep. 6, 18715. doi: 10.1038/srep18715
Rix L., de Goeij J. M., van Oevelen D., Struck U., Al-Horani F. A., Wild C., et al. (2018). Reef sponges facilitate the transfer of coral-derived organic matter to their associated fauna via the sponge loop. Mar. Ecol. Prog. Ser. 589, 85–96. doi: 10.3354/meps12443
Roark E. B., Guilderson T. P., Dunbar R. B., Fallon S. J., Mucciarone D. A. (2009). Extreme longevity in proteinaceous deep-sea corals. P. Nati. Acad. Sci. U.S.A. 106, 5204–5208. doi: 10.1073/pnas.0810875106
Robinson L. F., Adkins J. F., Frank N., Gagnon A. C., Prouty N. G., Roark E. B., et al. (2014). The geochemistry of deep-sea coral skeletons: A review of vital effects and applications for palaeoceanography. Deep-Sea Res. Part II-Top. Stud. Oceanogr. 99, 184–198. doi: 10.1016/j.dsr2.2013.06.005
Roder C., Berumen M. L., Bouwmeester J., Papathanassiou E., Al-Suwailem A., Voolstra C. R. (2013). First biological measurements of deep-sea corals from the Red Sea. Sci. Rep. 32802. doi: 10.1038/srep02802
Röthig T., Yum L. K., Kremb S. G., Roik A., Voolstra C. R. (2017a). Microbial community composition of deep-sea corals from the Red Sea provides insight into functional adaption to a unique environment. Sci. Rep. 7, 44714. doi: 10.1038/srep44714
Röthig T., Roik A., Yum L. K., Voolstra C. R. (2017b). Distinct bacterial microbiomes associate with the deep-sea coral Eguchipsammia fistula from the Red Sea and from aquaria settings. Front. Mar. Sci. 4. doi: 10.3389/fmars.2017.00259
Shen J., Jiao N., Dai M., Wang H., Qiu G., Chen J., et al. (2020). Laterally transported particles from margins serve as a major carbon and energy source for dark ocean ecosystems. Geophys. Res. Lett. 47, e2020GL088971. doi: 10.1029/2020GL088971
Shen Y., Guilderson T. P., Sherwood O. A., Castro C. G., Chavez F. P., McCarthy M. D. (2021). Amino acid delta C-13 and delta N-15 patterns from sediment trap time series and deep-sea corals: Implications for biogeochemical and ecological reconstructions in paleoarchives. Geochem. Cosmachim. Ac. 297, 288–307. doi: 10.1016/j.gca.2020.12.012
Sherwood O. A., Guilderson T. P., Batista F. C., Schiff J. T., McCarthy M. D. (2014). Increasing subtropical North Pacific Ocean nitrogen fixation since the Little Ice Age. Nature 505, 78–7+. doi: 10.1038/nature12784
Sherwood O. A., Heikoop J. M., Scott D. B., Risk M. J., Guilderson T. P., McKinney R. A. (2005). Stable isotopic composition of deep-sea gorgonian corals Primnoa spp.: A new archive of surface processes. Mar. Ecol. Prog. Ser. 301, 135–148. doi: 10.3354/meps301135
Sherwood O. A., Jamieson R. E., Edinger E. N., Wareham V. E. (2008). Stable C and N isotopic composition of cold-water corals from the Newfoundland and Labrador continental slope: Examination of trophic, depth and spatial effects. Deep-Sea Res. Part I-Oceanogr. Res. Pap. 55, 1392–1402. doi: 10.1016/j.dsr.2008.05.013
Sherwood O. A., Lehmann M. F., Schubert C. J., Scott D. B., McCarthy M. D. (2011). Nutrient regime shift in the western North Atlantic indicated by compound-specific delta N-15 of deep-sea gorgonian corals. Proc. Natl. Acad. Sci. U.S.A. 108, 1011–1015. doi: 10.1073/pnas.1004904108
Van Oevelen D., Duineveld G. C. A., Lavaleye M. S. S., Kutti T., Soetaert K. (2018). Trophic structure of cold-water coral communities revealed from the analysis of tissue isotopes and fatty acid composition. Mar. Biol. Res. 14, 287–306. doi: 10.1080/17451000.2017.1398404
Vinha B., Rossi S., Gori A., Hanz U., Pennetta A., De Benedetto G. E., et al. (2023). Trophic ecology of Angolan cold-water coral reefs (SE Atlantic) based on stable isotope analyses. Sci. Rep. 13, 9933. doi: 10.1038/s41598–023-37035-x
Wang P. X. (1999). Response of western Pacific marginal seas to glacial cycles: Paleoceanographic and sedimentological features. Mar. Geol. 156, 5–39. doi: 10.1016/s0025–3227(98)00172–8
Ward L. M., Shih P. M. (2019). The evolution and productivity of carbon fixation pathways in response to changes in oxygen concentration over geological time. Free Radical Bio. Med. 140, 188–199. doi: 10.1016/j.freeradbiomed.2019.01.049
Williams B., Thibodeau B., Chikaraishi Y., Ohkouchi N., Walnum A., Grottoli A. G., et al. (2017). Consistency in coral skeletal amino acid composition offshore of Palau in the western Pacific warm pool indicates no impact of decadal variability in nitricline depth on primary productivity. Limnol. Oceanogr. 62, 399–407. doi: 10.1002/lno.10364
Woodcock P., Edwards D. P., Newton R. J., Edwards F. A., Khen C. V., Bottrell S. H., et al. (2012). Assessing trophic position from nitrogen isotope ratios: effective calibration against spatially varying baselines. Naturwissenschaften 99, 275–283. doi: 10.1007/s00114–012-0896–2
Xing D., Choi B., Takizawa Y., Fan R., Sugaya S., Tuchiya M., et al. (2020). Trophic hierarchy of coastal marine fish communities viewed via compound-specific isotope analysis of amino acids. Mar. Ecol. Prog. Ser. 652, 137–144. doi: 10.3354/meps13475
Yamaguchi Y. T., Chikaraishi Y., Takano Y., Ogawa N. O., Imachi H., Yokoyama Y., et al. (2017). Fractionation of nitrogen isotopes during amino acid metabolism in heterotrophic and chemolithoautotrophic microbes across Eukarya, Bacteria, and Archaea: Effects of nitrogen sources and metabolic pathways. Org. geochem. 111, 101–112. doi: 10.1016/j.orggeochem.2017.04.004
Yamaguchi Y. T., McCarthy M. D. (2018). Sources and transformation of dissolved and particulate organic nitrogen in the North Pacific Subtropical Gyre indicated by compound-specific delta N-15 analysis of amino acids. Geochim. Cosmochim. Ac. 220, 329–347. doi: 10.1016/j.gca.2017.07.036
Yang J. Y. T., Kao S. J., Dai M. H., Yan X. L., Lin H. L. (2017). Examining N cycling in the northern South China Sea from N isotopic signals in nitrate and particulate phases. J. Geophys. Res.-Biogeosci. 122, 2118–2136. doi: 10.1002/2016jg003618
Yang Z., Chen J. F., Chen M., Ran L. H., Li H. L., Lin P., et al. (2018). Sources and transformations of nitrogen in the South China Sea: Insights from nitrogen isotopes. J. Oceanogr. 74, 101–113. doi: 10.1007/s10872–017-0443-z
Keywords: deep-sea coral community, gorgonian, tropic position, amino acids, carbon and nitrogen isotopes, South China Sea
Citation: Luo Z, Chen L and Jia G (2024) Bulk and amino acid isotope evidence of supplementary food sources besides euphotic production for a deep-sea coral community in the South China Sea. Front. Mar. Sci. 11:1399814. doi: 10.3389/fmars.2024.1399814
Received: 12 March 2024; Accepted: 08 May 2024;
Published: 22 May 2024.
Edited by:
Santiago Herrera, Lehigh University, United StatesReviewed by:
Kelton W. McMahon, University of Rhode Island, United StatesAlberto Sánchez-González, National Polytechnic Institute (IPN), Mexico
Copyright © 2024 Luo, Chen and Jia. This is an open-access article distributed under the terms of the Creative Commons Attribution License (CC BY). The use, distribution or reproduction in other forums is permitted, provided the original author(s) and the copyright owner(s) are credited and that the original publication in this journal is cited, in accordance with accepted academic practice. No use, distribution or reproduction is permitted which does not comply with these terms.
*Correspondence: Guodong Jia, jiagd@tongji.edu.cn