Gas Sensing by Microwave Transduction: Review of Progress and Challenges
- Faculty of Electrical Engineering and Computer Science, Ningbo University, Ningbo, China
Microwave transduction is a novel research field in gas sensing owing to its simplicity, low cost, passive, and non-contact operations that indicate a huge potential in applications for gas sensing. At present, the study of microwave gas sensors is limited to testing the macroscopic performance of materials. Although the permittivity change of materials is the reason for microwave transduction, the knowledge of the fundamental mechanism is an urgent requirement for boosting the development of microwave gas sensors. In this review, we summarized the presented progresses of microwave gas sensors, including the performance of the different materials and propagative structures, as well as their sensitive signal. We have attempted to explain the sensitive mechanism of these materials, discuss the function of the propagative structure, and analyze the sensitive signal extracted from the parameters of electromagnetic waves. Finally, the challenges in the study of sensitive materials and propagative structures used in microwave gas sensors are analyzed. It is clear from the published literatures that microwave transduction provide a new route for gas detection, and it is expected that commercial manifestation will occur. The future research on microwave gas sensors will continue to exploit their sensitive materials and propagative structure for different applications. The understanding of the microscopic polarization interactions with gases will assist in and guide the fabrication of high-performance microwave gas sensors in the future.
Introduction
In the recent decade, increasing interest in detecting gases and their composition have resulted in intensive studies applied to various fields, such as industrial emission control, household security, medical diagnostics, and environmental monitoring (Korotcenkov, 2012). Generally, gas sensing is the process of converting gas sensitive interactions to a measurable signal, which involves two different processes (Yamazoe and Shimanoe, 2009). The first process is to sense gas via physical or chemical interactions with sensitive materials, which is called the sensitive process; the second process is to transduce the interactions to signals, which is called transduction. Various transduction technologies have been employed in the gas sensors, e.g., conductometric transduction converts redox reactions of gas molecules into electrical signals (Xing et al., 2015; Wang et al., 2019); optical transduction utilizes infrared spectral characteristics of gas molecules (Babeva et al., 2017); mass transduction measures weight of gas molecules using a microbalance, and converts it to a vibration frequency signal (Tu et al., 2015; Lv et al., 2018); electrochemical transduction is based on electron transfer between electrolyte and gas molecules (Zheng et al., 2015).
Although the performance parameters (sensitivity, selectivity, and stability) of gas sensors based on conventional transduction were constantly improved (Moos et al., 2009; Kim et al., 2013), the use of the electromagnetic waves for sensing purposes is an actively researched approach with considerable potential for commercialization (Alshamma'a et al., 2014). In particular, microwave is an important frequency band for communication and remote sensing applications; it is a fast-growing technology and has been successfully applied in industrial and medical applications (Barochi et al., 2011).
The microwave transduction is based on the dielectric response of sensitive materials incorporated with propagative structures in the presence of gas that is subjected to a microwave signal (Fonseca et al., 2015b). Batteryless and wireless operations are suggested to be the major advantages of microwave gas sensors; however, the lack of fundamental understanding of the sensitive mechanism hinders the development of microwave gas sensors. Several challenges were presented in the study of microwave transduction. There is the need of a review to focus on the presented progress and challenges of microwave gas sensors.
Table 1 summaries the reported microwave gas sensor. This review article will start by describing the working principles of the microwave gas sensor. Then, the current progress will be discussed corresponding to the sensitive material, propagative structure, and sensitive signal. Subsequently, the difficulties and challenges will be discussed. Furthermore, an outlook on the future development of microwave gas sensor will be presented.
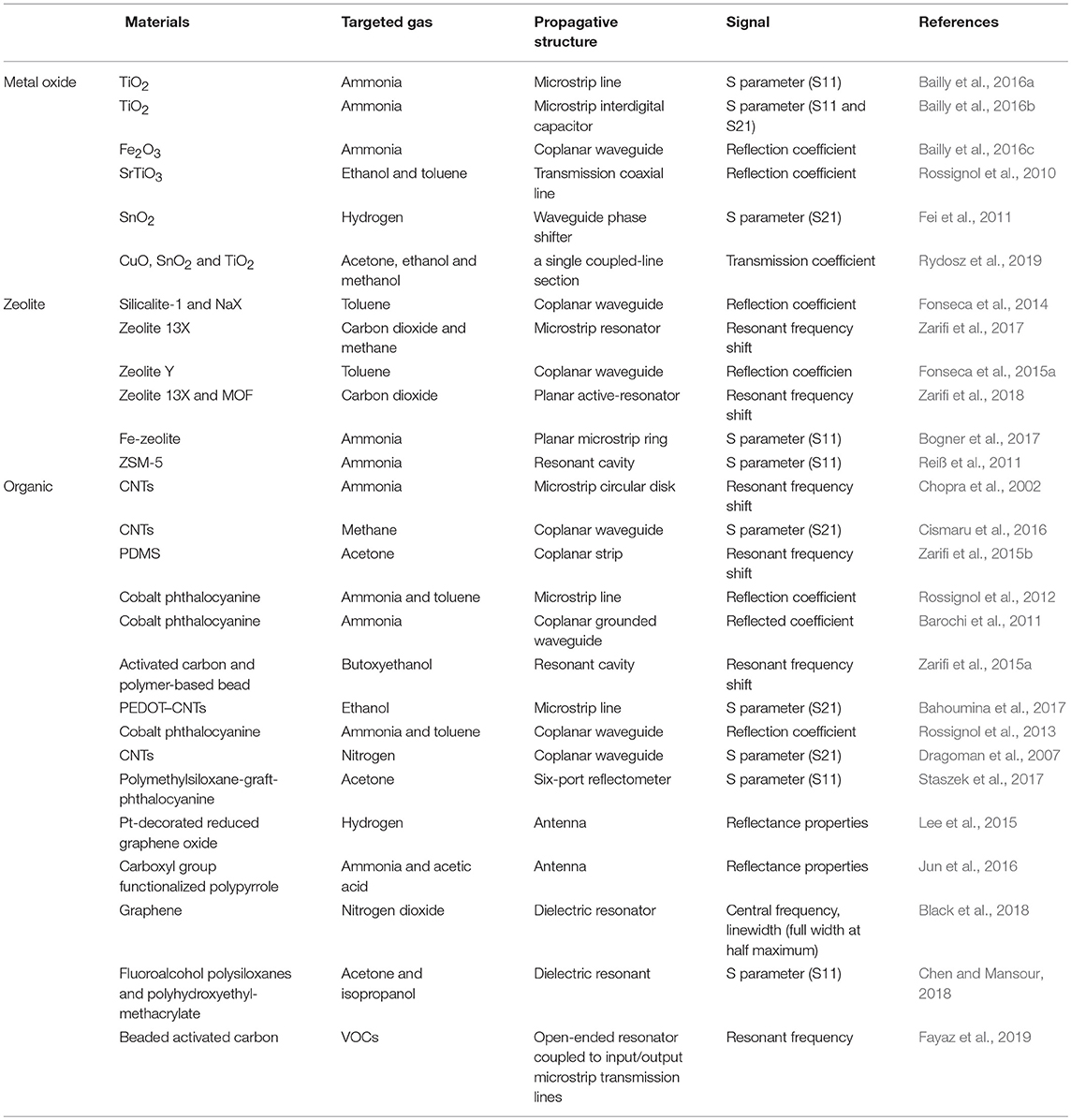
Table 1. Summary of microwave gas sensors on their materials, targeted gas, propagative structure and signal.
Working Principle of Microwave Transduction
The evolving research on microwave transduction at frequencies ranging from 300 MHz to 300 GHz demonstrates that gas sensing is based on the phenomena of propagation and reflection of an incident electromagnetic wave in sensitive material. As the characteristics (modulus and phase) of the reflected and transmitted electromagnetic waves depend on the physical and chemical properties of the materials, the interactions with gas molecules should modify their permittivity and change the velocity of the electromagnetic waves (El Bouazzaoui et al., 2011). By measuring the variations in the transmitted and reflected microwaves at discrete frequency intervals, the frequency change or attenuation of the incident electromagnetic waves can be linked to the gas sensitive interactions (Huang et al., 2018).
The permittivity ε of a material is a complex quantity; it describes the dielectric polarization of the materials to an applied electric field (Xie et al., 2015). Because there is a lag between the changes in polarization of the materials and the alternating electric field, the permittivity depends on the frequency of the field. According to the Maxwell–Garnett equation, the permittivity of materials is described as follow (Auerbach et al., 2003):
εs is the static permittivity, ε∞ is the permittivity at the high frequency limit, ω is the angular frequency, τ is the characteristic relaxation time, and σ is the conductivity of the material. The real part of the permittivity (ε′) represents the energy stored in the material. The imaginary part of the permittivity (ε′′) is linked to the conductivity of the material.
The evolution of permittivity with the frequency of electric field indicates the change of dominant polarization process, which is shown in the Figure 1. If a polarization process follows the oscillation frequency of the field, its permittivity become dominant; otherwise, it decreases. The averaged time for a polarization process is characteristic relaxation time. Thus, according to the relaxation time from slow to fast, the polarization processes are ionic conduction, dipolar polarization, ionic polarization, atomic polarization, and electronic polarization. Further, there is no clear boundary for different polarizations. When the frequency is above the ultraviolet range, the permittivity approaches the constant ε0, which is the permittivity of free space.
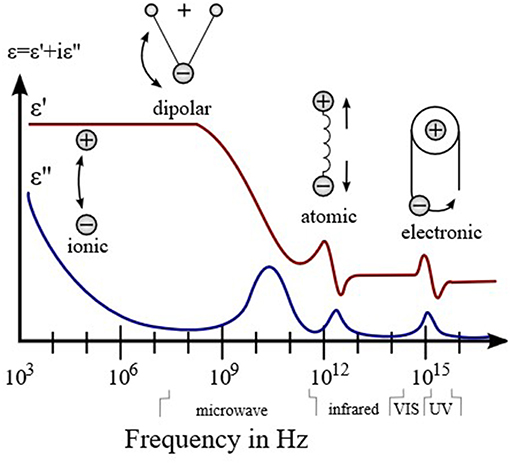
Figure 1. Dielectric permittivity spectrum over a wide range of frequencies. Various processes are labeled in the image.
The permittivity is influenced by the dipolar polarization in the low microwave frequency range and ionic polarization in the high microwave frequency range. The dipolar polarization is orientation of permanent dipoles in materials responsed to electric field, and the ionic polarization is the polarization caused by relative displacements between the positive and negative ions (Mitroy et al., 2010). For microwave gas sensing, the adsorption of polar gas molecules is expected to induce dipole-dipole interactions with the permanent dipole of the materials. These dipole interactions will vary the timescale or strength of the orientation of the permanent dipole and the displacement of ions; consequently, a change in the polarization as well as the permittivity of the materials is demonstrated.
Besides the sensitive materials, the microwave gas sensor requires propagative structures to transmit the microwave (Fonseca et al., 2014). The propagative structure can be classified as non-resonator and resonator depend on the propagation of electromagnetic waves; standing wave transmits in resonator and traveling wave transmits in non-resonator. The non-resonator has simple structure and wide bandwidth; the structure of resonator is designed in a narrow bandwidth, its resonant frequency is stable during measurement, so higher resolution can be achieved for the resonator. There are many geometric structures that are used for microwave gas sensing, e.g., microstrip line, coplanar waveguide, coaxial line, and resonant cavity (Figure 2). By designing their geometry, the sensors are operated in different microwave ranges.
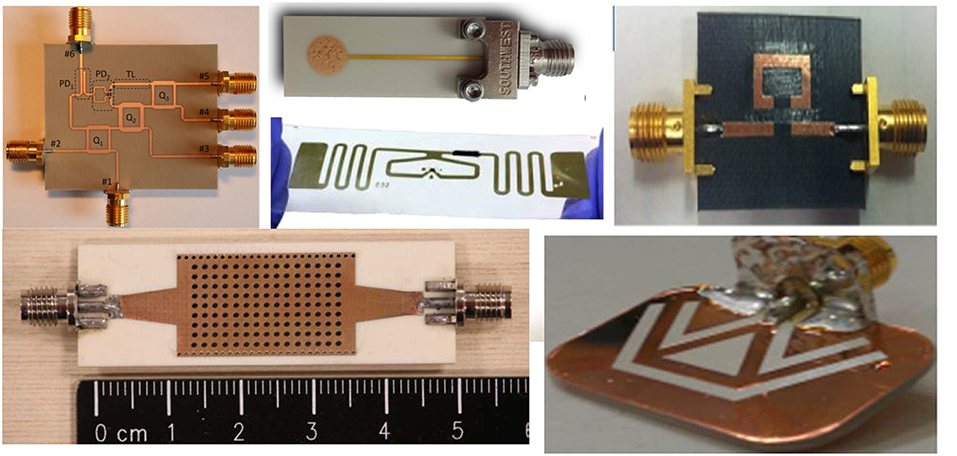
Figure 2. Various propagative structures applied in microwave gas sensors. Reproduced with permissions from Fei et al. (2011), Fonseca et al. (2014), Zarifi et al. (2016), Bogner et al. (2017), and Staszek et al. (2017). Copyright 2014, 2016, 2017 Elsevier; copyright 2016 American Chemical Society; copyright 2016 IEEE.
Sensitive materials are deposited in various forms on the propagative structures. In general, there are two forms, which are surface film and volumic bulk. The surface film is formed by depositing sensitive materials on the propagative structure, and volumic bulk uses sensitive materials to replace the substrate of the propagative structure. Although gas diffusion in the volumic bulk requires a longer time than the surface film, the volumic bulk has a larger cross-sectional area to interact with the microwave. Various procedures using sensors are implemented in accordance with the chosen sensitive materials, propagative structures, and their integrated forms (surface film or volumic bulk).
Sensitive Materials
So far, metal oxide, zeolite, and organic, which are the conventional sensing materials, were applied in the microwave gas sensors to detect gaseous compounds such as volatile organic compound, NH3, CO2, CO, O2, or H2. Although the gas sensitive reactions of these materials under conductometric transduction were extensively studied, the link between the dipole reaction and microwave signals is still unknown.
Metal Oxides
Since the gas sensing of oxides is based on surface interactions with gas molecules, nano-oxides with high surface area present advantage on enhancing sensitivity. TiO2 and α-Fe2O3 nanoparticles in the form of a surface film on the waveguide substrate were applied for NH3 sensing (Bailly et al., 2016a,b,c); the principle of measurement is shown in Figure 3A. The permittivity of oxides changed when exposed to NH3 at room temperature, which caused the variation of the reflected coefficient (S11) and the transmitted coefficient (S21). Although an increase in the conductivity of TiO2 and α-Fe2O3 with ammonia exposure were reported in Moseley and Williams (1990), the authors assumed that the formation of NH species on the surface of the oxides was the source for the permittivity change; however, the relation of NH to the decrease in permittivity is unclear. Furthermore, the effect of the shape of oxides involved in microwave transduction was studied. It was observed that different morphologies presented a significant difference in behavior upon ammonia adsorption; the sensitive responses of different morphologies are shown in Figure 3B. The crystal planes with high surface energy and abundant unsaturated active sites usually serve as a promising platform to interact with gas molecules (Gao and Zhang, 2018). Controlled morphology with exposed high energy facets are still valid for the metal oxides in microwave transduction (Bailly et al., 2016c).
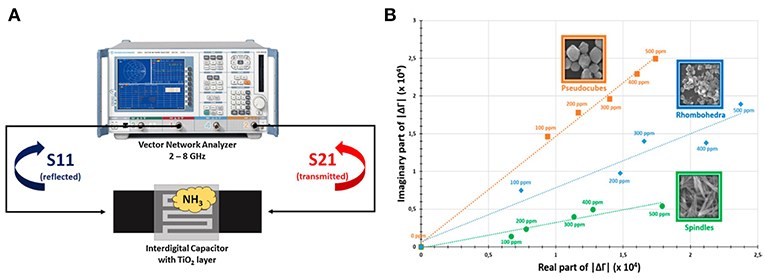
Figure 3. (A) Principle of microwave transduction applied to reflection/transmission measurements, (B) response and recovery times for a microwave sensor exposed to ammonia. Reproduced with permissions from Bailly et al. (2016b,c). Copyright 2016 Elsevier; copyright 2016 American Chemical Society.
Other metal oxides such as SnO2 and SrTiO3 presented decreasing permittivity to hydrogen, ethanol, and toluene in microwave transduction (Rossignol et al., 2010; Fei et al., 2011). In the literatures, SnO2 and SrTiO3 employed volumic bulk form; a small concentration of hydrogen was detected by filling SnO2 in the substrate of a waveguide phase shifter. Increasing the cross-sectional area of sensitive material in electromagnetic wave could be a way for enhancing the sensitivity of the microwave sensor.
Zeolites
Bulk adsorption is expected to increase the influence of gas molecule on the overall permittivity of sensitive materials; thus, microporous and mesoporous materials were applied in the microwave gas sensors.
Zeolite is crystalline aluminosilicates with pores and channels of molecular dimensions. Approximately 190 zeolites with distinct framework topologies have been reported (Zheng et al., 2012). Four commonly used zeolites are shown in Figure 4. The aluminosilicate framework of zeolites is negative owing the Al3+ presented in the network of [SiO4]4− tetrahedra; thus, a cation is introduced to balance the negative charge around Al3+ sites; the extra-framework cations are mobile in the channel of zeolites as the zeolites are ionic conductors (Xu et al., 2006; Sahner et al., 2008).
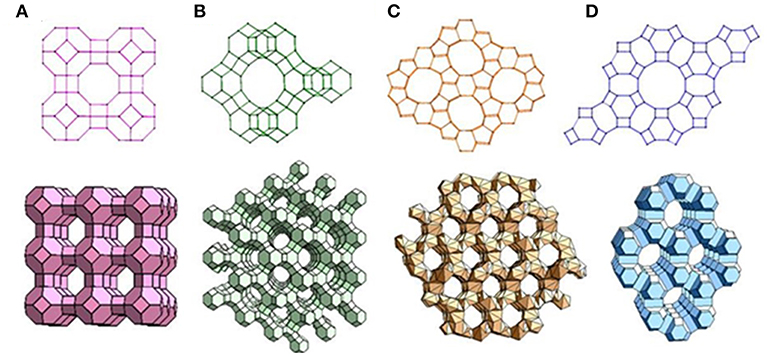
Figure 4. Representative zeolite frameworks (with pore openings). (A) Zeolite A (3D, 4.2 Å); (B) zeolite Y (3D, 7.4 Å); (C) Zeolite L (1D, 7.1 Å); (D) ZSM-5 (2D, 5.3 × 5.6 Å, 5.1 × 5.5 Å). D indicates the dimensions of the channel system. Reproduced from Zheng et al. (2012).
Because of the large volume of pores in the zeolites, the accessible volume for gas molecules is from 5 to 30%. The overall permittivity of zeolite is contributed by the aluminosilicate framework (εframe) and pores (εpore), so the filling of gas molecules into the pores can alter the permittivity of the zeolites. The change of the permittivity of the pores based on bulk gas adsorption is described as follow:
where εn is the initial permittivity of the pores, which usually is 1 (N2, εN2 = 1), εg is the permittivity of the gas molecules, f is the filling factor (f = Vg/Vpore),Vg is the volume of adsorbed gas molecules, and Vpore is the volume of the pores. Thus, increasing the permittivity of zeolite can be achieved by adsorbed polar gas. Several studies have observed this performance (Zarifi et al., 2017). The evolution of gas adsorption in zeolite and the permittivity change are shown in Figure 5.
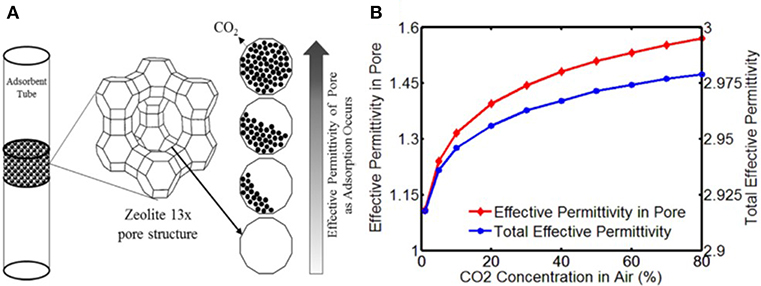
Figure 5. (A) Evolution of CO2 adsorption in Zeolite X and the corresponding permittivity change, (B) simulation results of concentration effect on permittivity of pores and adsorbents. Reproduced with permission from Zarifi et al. (2017). Copyright 2017 Elsevier.
Besides the physical adsorption, the coordinatively unsaturated metal sites in the zeolite are also sites for gas adsorption by the electrostatic process (Zarifi et al., 2018). Therefore, more unsaturated metal sites in the sensitive material induce higher adsorption capacity, and this demonstrated a larger sensitive response.
The amplitude variations at resonant frequencies were observed for zeolite Y on coplanar waveguides for toluene exposure in the range of 2–10 GHz (Fonseca et al., 2015a). The variable orientations (increasing or decreasing orientations) of the signals differed at different resonant frequencies. Although the amplitude variation of resonant frequency originated from the permittivity change of zeolite, different variable orientations revealed complicated interactions of zeolite with gas molecules.
For the dipole interaction of zeolites with gas molecules, scarce characterizations were presented. Based on the ionic model of zeolite, the mobile cation was localized in the center of a neighboring tetrahedron [AlO4]−, and their dipole (μ1) was parallel to the electric field vector. When gas molecules enter into the cavity, they will perturb the original electric field, the cation will jump a distance and orient itself to a new dipole moment (μ2) (Figure 6) (Wei and Hillhouse, 2006; Nicolas et al., 2008). This dipole change could modify the permittivity of zeolite, and the cations at different sites in zeolitic framework are expected to introduce dipole interactions with different strength. Detailed characterizations are needed to verify the dipole interaction.
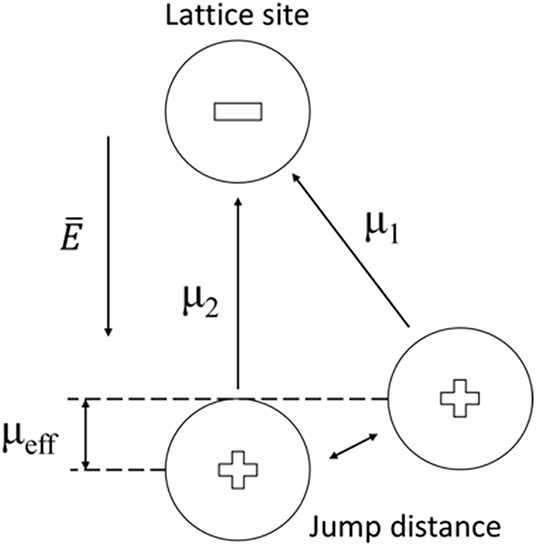
Figure 6. Schematic for dipole moment between cation and lattice site by a change of place of the cation in a zeolite channel.
Organics
Carbon materials and polymers were widely applied as gas sensitive materials. Carbon nanotubes (CNTs) and graphene have high surface areas, and their properties can be modified by simple chemical processes (Kauffman and Star, 2008; Yuan and Shi, 2013).
It is well-known that the conductivity of carbon nanotubes decreases when exposed to NH3 and CH4 (Kauffman and Star, 2008). However, frequency shifts were observed for a resonator based on carbon nanotubes under NH3 or CH4 environment (Chopra et al., 2002; Occhiuzzi et al., 2011; Cismaru et al., 2016). The conductivity change, which is the imaginary part of permittivity, can vary the amplitude of resonant frequency, but the frequency shift indicates a change of the real part of permittivity. A possible explanation is that gas molecules interact with carbon nanotubes to create bound charges resulting in changes in the real part of permittivity of carbon nanotubes.
Noble metal loading on carbon materials can effectively enhance the sensitivity of a sensor. Pt loaded graphene can detect H2 in concentrations as minimal as 1 ppm in the frequency range from 880 to 940 MHz (Lee et al., 2015), which is shown in the Figure 7. The large surface area of graphene and uniform Pt decoration provide an excellent platform for gas adsorption and dielectric response. However, the mechanism of permittivity change for the nano-composition of Pt/graphene under H2 is rarely discussed.
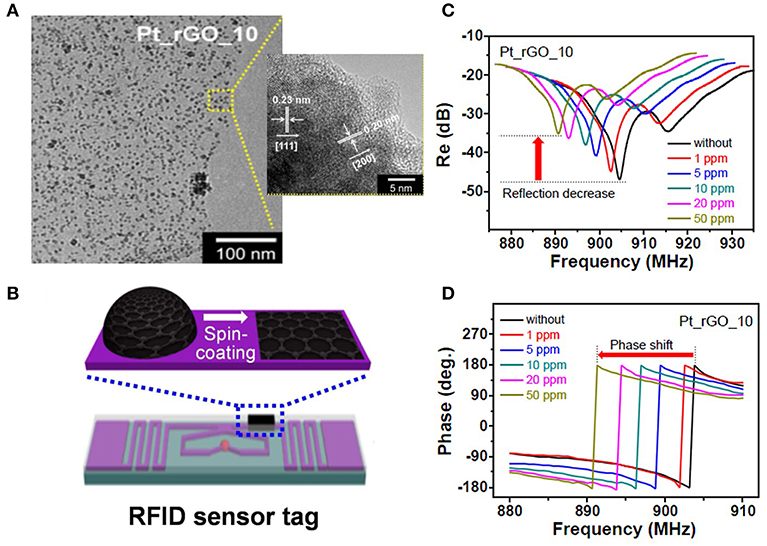
Figure 7. (A) Transmission electron microscopy images of Pt-decorated reduced graphene oxide (Pt_rGO), (B) Pt_rGO-immobilized radio-frequency identification sensor tag, (C,D) change in the reflectance properties and measured reflection phase of different Pt_rGO-based wireless sensors as a function of the hydrogen gas concentration. Reproduced with permission from Lee et al. (2015). Copyright 2015 American Chemical Society.
Many advantages lead to polymers being a good candidate for gas sensing, such as low cost, high sensitivity at low temperature, good gas permeability, flexibility, simple synthesis, and environmental stability (Kubersky et al., 2015; Wang et al., 2017). In microwave transduction, a physically adsorbed gas molecule can diffuse into polymer and swell its volume, which contribute to permittivity change (Zarifi et al., 2015a,b). With the support of a six-port reflectometer, the limit of detection of copolymer Pc film to acetone was identified to be 0.5 ppm (Staszek et al., 2017). The dynamic change of S11 for different acetone concentrations are shown in the Figure 8. Except for the physical adsorption of gas molecules, the conductivity of polymer also results in a permittivity change. The composition of conducting polymer and carbon nanotubes were explored for ethanol detection, and insertion losses and frequency shifts on the resonant frequency were observed (Bahoumina et al., 2017). A conducting polymer with a functional group exhibited high sensitivity to ammonia and acetic acid. The authors observed that the increase in density of carboxylic functional groups enhanced the sensitivity (Jun et al., 2016), which indicate that the dipole of functional groups are involved in the dielectric response.
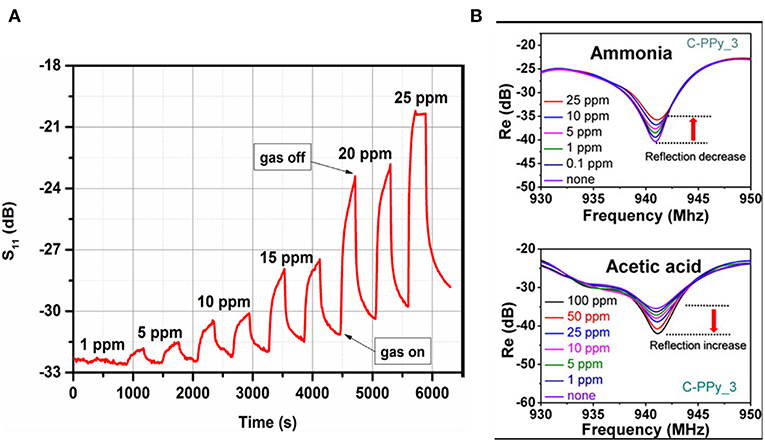
Figure 8. (A) Magnitude of reflection coefficient S11 measured at the resonant frequency under exposure to acetone in the 0–25 ppm concentration range, (B) change in the reflectance properties of different concentrations of ammonia and acetic acid. Reproduced with permissions from Jun et al. (2016) and Staszek et al. (2017). Copyright 2017 Elsevier; copyright 2016 American Chemical Society.
Metal phthalocyanines are sensitive to various gases owing to their catalyst. The redox with gas molecules can not only modify their density of charge carriers and conductivity, but also the permittivity of material (Bai and Shi, 2007). Cobalt phthalocyanine (CoPc) was applied for toluene and ammonia detection on various substrate (Barochi et al., 2011; Rossignol et al., 2012, 2013). The real and imaginary part of the reflected coefficient changed when exposed to NH3, except for the conductivity change. The surface dipoles or electronic structure of CoPc is supposed to vary through the interactions with gas molecules; however, this is merely a hypothesis.
In summary, the mechanism of sensitive materials in microwave gas sensor is rather complicated. Only conductivity change is not sufficient to explain the variation of complex permittivity. So far, lack of experimental evidences for dipoles and ionic polarization in microwave range prevents further discussion. However, there are two strategies are suggested for increasing the sensitivity of materials. Increasing adsorption capacity of materials is a valid method to detect polar gas molecules, higher adsorption capacity could leads to larger dielectric response of materials. Thus, microporous and mesoporous materials will be effective materials for microwave gas sensor. The other aspect is the polarized species in materials which can interact with targeted gas molecules. For example, hydroxide radicals could bind NH3 to form dipoles (NH), which modify the polarization of material; lewis acids can interact with reducing gases that form intermediate dipoles or annihilate Lewis sites, which change the permittivity of materials. Well-designed polar species in materials devoted for gas sensing are required. At the same time, increasing the density of polarized species can greatly improve the sensitivity. Moreover, the morphology of oxides is observed to have effect on dielectric response, different density of defects and dangle groups are supposed to the reason.
Propagative Structures
A propagative structure is used to transmit electromagnetic waves and supports the sensitive materials for adapting in different environments. Its quality factor determines the resolution of the sensor. The quality factor is a ratio of energy loss related to the stored energy of the propagative structures; a high quality factor indicates a resonance profile with narrow resonator's bandwidth relative to its center frequency (Zarifi et al., 2015c).
The microwave resonant cavity has an significantly high quality factor, which increases the resolution of the sensor, but it only works at a single frequency (Tooski, 2010, 2011). The planar microstrip line, coplanar waveguide, and interdigital capacitor are the common structures employed in the experiments on microwave sensors because of their merits, such as simple structure, compact size, low-cost manufacturing, large operation frequency range, and simplicity in integrating in an antenna for remote sensing. For precise measurement, an active microstrip line and six-port reflectometer were applied to increase the quality factor of the sensors. Their resonance peaks were sharp and a small frequency shift could be easily detected as shown in Figure 9.
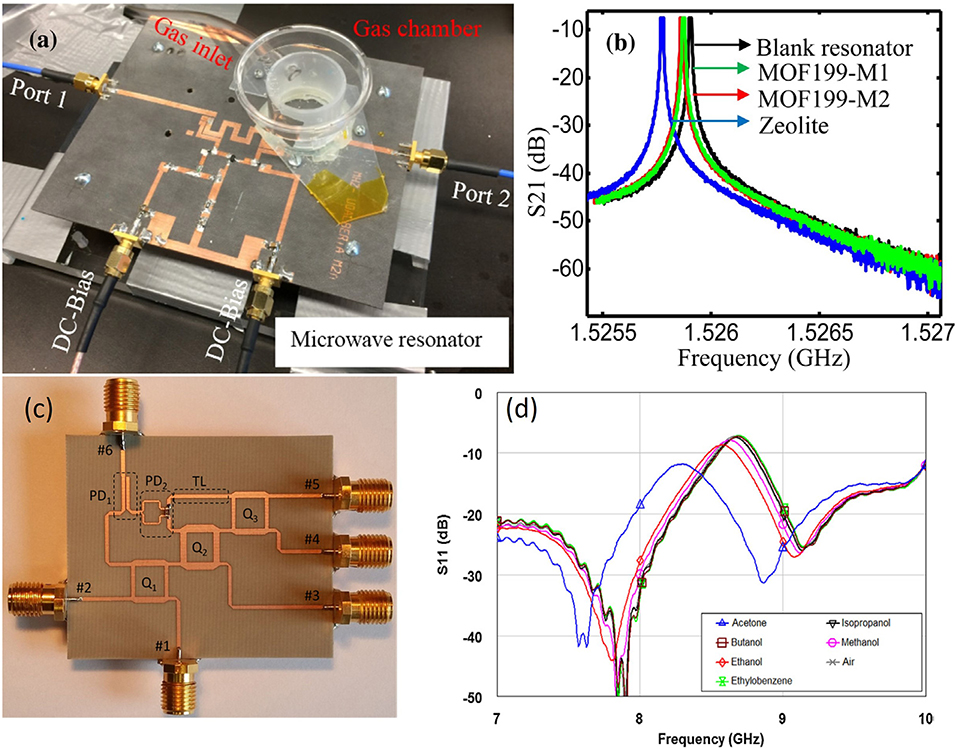
Figure 9. (a) Experimental setup with the microwave active resonator sensor, (b) resonant profile (S21) of the sensor based on the active resonator, (c) photograph of the fabricated six-port reflectometer, (d) resonant profile S11 of the sensor based on the six-port reflectometer. Reproduced with permissions from Staszek et al. (2017) and Zarifi et al. (2018). Copyright 2017, 2018 Elsevier.
To cancel the influence of temperature or humidity on the electromagnetic behavior, the differential method was proposed by using a reference resonator (Bahoumina et al., 2017; Huang et al., 2018). The response is the difference between the sensitive resonator and the reference resonator with a blank or with materials with known permittivity. The configuration of differential resonators is shown in Figure 10. The differential method ensures a low variance signal with good repeatability.
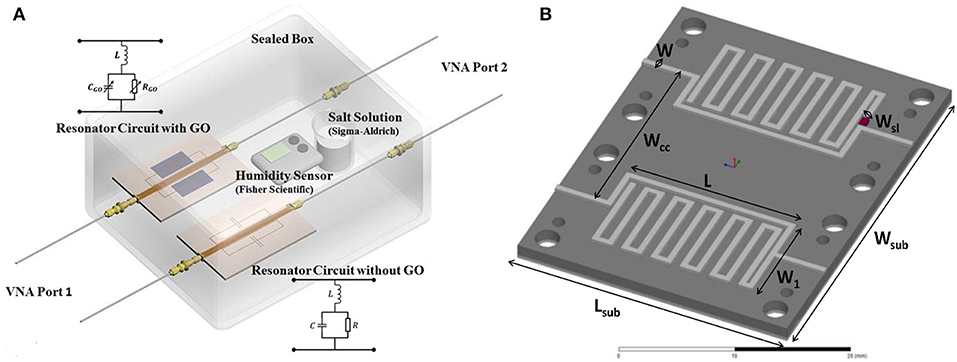
Figure 10. (A) Separated sensitive resonator and reference resonator, (B) dimensions and geometry of differential sensor based on two stub resonators. Reproduced with permissions from Bahoumina et al. (2017) and Huang et al. (2018). Copyright 2017 Elsevier.
The microwave cavity waveguide provided a powerful technique for measuring NH3 loading in the catalyst of a diesel engine (Dietrich et al., 2017). The catalyst itself was the sensitive material, and the catalyst canning was the propagative structure. A planar propagative structure was also applied in non-contact mode for monitoring of the adsorbent; the adsorbent in the gas tube was above the planar microstrip line, which is shown in Figure 11. The permittivity change of adsorbent influenced the electromagnetic waves propagated in the microstrip line (Reiß et al., 2011; Zarifi et al., 2015a, 2017). This contactless technique is attractive for dangerous and toxic gas detection as the sensing platform does not need to be in the gas-flowing medium.
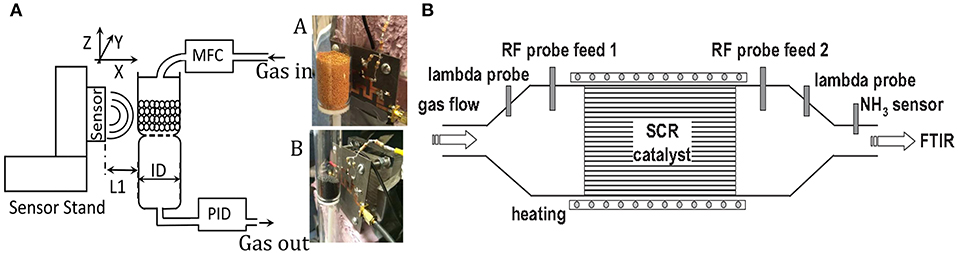
Figure 11. (A) Schematic of the measurement setup of the sensor to perform noncontact gas sensing, (B) illustration of the setup for the resonance cavity of catalyst. Reproduced with permissions from Zarifi et al. (2015a) and Dietrich et al. (2017). Copyright 2017 John Wiley and Sons; copyright 2015 AIP Publishing.
Signal Analysis
The gas sensing tests of the microwave sensors were carried out by vector network analyzer (VNA). The sensitive signal was derived from various parameters of the VNA measurement. Despite the lack of permittivity measurements of materials in microwave range, the permittivity of sensitive materials applied in microwave gas sensors were distributed over a wide range, polymers demonstrated measurements between 2 and 5, and the permittivity of oxides and zeolites could be adjusted from 3 to 15. The materials with lower initial permittivity are assumed to possess higher sensitivity for small permittivity changes, because the dielectric response is usually calculated by the ratio of the permittivity change and the initial value. In most microwave gas sensors, the reflection characteristics of electromagnetic waves are extracted as signals, which include the S parameter, reflection coefficient, return loss, and input impedance. All these parameters describe the degree of mismatch in impedance. No comparative study about different parameters on gas sensing has been conducted.
The alteration of parameters can be classified as a frequency shift in the frequency spectra or magnitude variation at a fixed frequency. To analyze the response and recovery time, each parameter was measured at a fixed frequency with the evolution of time. The time taken by a sensor to achieve 90% of the total parameter change is defined as response time in the case of adsorption or the recovery time in the case of desorption as shown in Figure 12a (Zheng et al., 2015; Bailly et al., 2016b). Combining both the real and imaginary parts of a parameter is considered as a powerful tool to assess the overall performance of a sensor (Rossignol et al., 2013). Figures 12b,c present the real vs. imaginary plot for S11 measurement. Here, each concentration outlines a full ellipsoid, whose radius is correlated to the concentration of ammonia. The obtained ellipsoids could facilitate the optimization of the sensor performance in predicting the concentration. Besides optimizing the sensor performance, the imaginary part of the parameter as a function of the real part shows a set of aligned points for a concentration at fixed frequency (Barochi et al., 2011). This assists the discriminated power of sensors in concentration prediction through the use of pattern recognition algorithms (Chen et al., 2017).
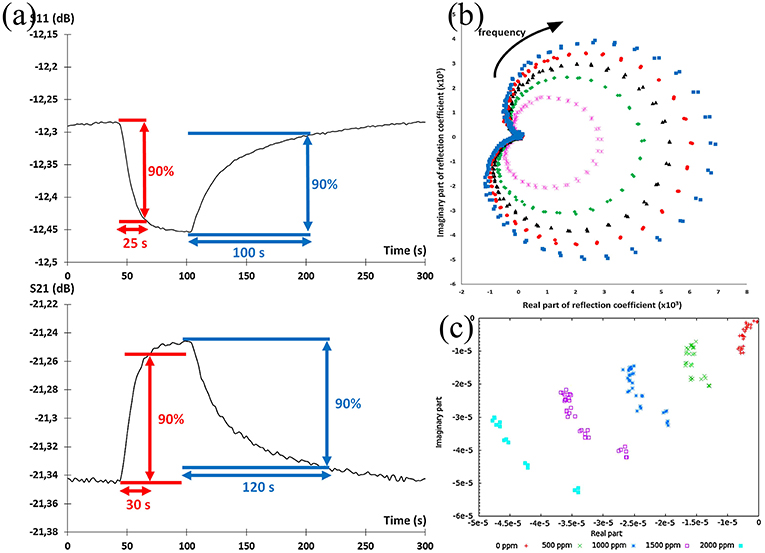
Figure 12. (a) Evolution of S-parameters during the gas sensing, and determination of response and recovery times, (b) imaginary part as a function of real part of S11 for different gas concentrations between 2 and 2.5 GHz, frequency evolution follows the clockwise direction, (c) imaginary part as a function of real part of signal in linear scale at fixed frequency. Reproduced with permissions from Rossignol et al. (2013) and Bailly et al. (2016b). Copyright 2013, 2016 Elsevier.
Outlook
We have demonstrated the presented progress of microwave gas sensors. This is a considerably vigorous and fast evolving field and continuous rapid growth can be expected with advances in materials and device fabrication, characterization, and signal processing. The use of the novel microwave transduction process in a gas sensor is still in its infancy. Based on the examples discussed in the review, most sensors were developed to serve the environmental industry; however, important parameters for gas sensors, such as selectivity, sensitivity, and long-term stability as well as reproduction were not satisfactorily reported in the literatures. Other relevant aspects include operational conditions such as temperature, humidity, and packaging. It is now necessary to improve our fundamental knowledge on microwave gas sensors and consider the future trends. Although we may overlook certain important issues, it is useful to consider which developments and challenges may deserve careful scrutiny.
A key challenge is the gas sensitive mechanism for different materials pertaining to permittivity change. The interactions of materials with gas molecules related to the conductivity change were extensively studied as conductometric gas sensors are the most popular in recent decades. Nevertheless, it is unsuitable for microwave gas sensors. The signal of the microwave gas sensor originates from the permittivity change. However, to the best of our knowledge, no study has reported the permittivity change of materials because of the complicated methods required for measuring permittivity in the microwave range. Therefore, designing a novel experiment is necessary for directly measuring the permittivity changes for gas sensing. The permittivity reflects various polarizations and the gas sensitive process is related to the polarization processes. A fundamental study is urgently required to understand the relation between the polarization changes of materials in gas sensing. Consequently, “permittivity synthesis” can become possible. This introduces the possibility of adapting the desired dielectric interactions with gas molecules by ordering the proper atoms and molecules into specified materials.
The dielectric properties of materials in gas sensing attracted the most attention, but the design of the propagative structure is important for practical applications. Propagative structures with high sensitivity can be designed, as long as the permittivity of materials is measured. High quality factor is also desired for precise measurement, but the permittivity change in gas sensing will degrade the quality factor. The degrading quality factor narrows the test range of gas concentrations. Simultaneously, the bandwidth impacts the concentration range of sensors. Therefore, the contradiction between high quality factor and wide bandwidth requires balanced optimization. Various functions are demanded for better suitability in different fields. The omnidirectional polarized sensor is desired for the ease of use in wireless measurements. The coupling effects of electromagnetic waves is a critical issue for developing a sensor array. Flexibility and compact size maybe necessary for integrating the sensors into wearable devices. Digestible antenna is required for sensing in human body. Furthermore, the resonant cavity method lends itself well for in situ and non-contact measuring gas adsorption of materials in pipe.
In conclusion, there appears to be considerable advantages for microwave transduction in gas sensing. There are several fundamental challenges that need to be addressed before microwave gas sensors can become viable candidates for commercial implementation. The future of microwave gas sensors looks bright, and continued progress in this field will overcome the current challenges and lead to a class of sensors that can be used in a wide range of environments and applications.
Author Contributions
YZ and CH conceived the paper. FL and YZ analyzed the references and wrote the paper. JJ reviewed the paper.
Funding
This work was supported by the National Natural Science Foundation of China [grant numbers 61871245]; the Natural Science Foundation of Ningbo Municipality [grant number 2018A610021], and K. C. Wong Magna Fund in Ningbo University.
Conflict of Interest Statement
The authors declare that the research was conducted in the absence of any commercial or financial relationships that could be construed as a potential conflict of interest.
References
Alshamma'a, A., Mason, A., and Korostynska, O. J. S. R. (2014). Microwave sensors for the non-invasive monitoring of industrial and medical applications. Sens. Rev. 34, 182–191. doi: 10.1108/SR-11-2012-725
Auerbach, S. M., Carrado, K. A., and Dutta, P. K. (2003). Handbook of Zeolite Science and Technology. New York, NY: Marcel Dekker Inc. doi: 10.1201/9780203911167
Babeva, T., Andreev, A., Grand, J., Vasileva, M., Karakoleva, E., Zafirova, B. S., et al. (2017). Optical fiber-Ta2O5 waveguide coupler covered with hydrophobic zeolite film for vapor sensing. Sens. Actuat. B-Chem. 248, 359–366. doi: 10.1016/j.snb.2017.03.157
Bahoumina, P., Hallil, H., Lachaud, J. L., Abdelghani, A., Frigui, K., Bila, S., et al. (2017). Microwave flexible gas sensor based on polymer multi wall carbon nanotubes sensitive layer. Sens. Actuat. B Chem. 249, 708–714. doi: 10.1016/j.snb.2017.04.127
Bai, H., and Shi, G. (2007). Gas sensors based on conducting polymers. Sensors 7, 267–307. doi: 10.3390/s7030267
Bailly, G., Harrabi, A., Rossignol, J., Domenichini, B., Bellat, J. P., Bezverkhyy, I., et al. (2016a). Influence of the design in microwave-based gas sensors: ammonia detection with titania nanoparticles. Proc. Eng. 168, 264–267. doi: 10.1016/j.proeng.2016.11.185
Bailly, G., Harrabi, A., Rossignol, J., Stuerga, D., and Pribetich, P. (2016b). Microwave gas sensing with a microstrip interDigital capacitor: detection of NH3 with TiO2 nanoparticles. Sens. Actuat. B Chem. 236, 554–564. doi: 10.1016/j.snb.2016.06.048
Bailly, G., Rossignol, J., Fonseca, B. D., Pribetich, P., and Stuerga, D. (2016c). Microwave gas sensing with hematite: shape effect on ammonia detection using pseudocubic, rhombohedral and spindle-like particles. ACS Sens. 1, 656–662. doi: 10.1021/acssensors.6b00297
Barochi, G., Rossignol, J., and Bouvet, M. (2011). Development of microwave gas sensors. Sens. Actuat. B Chem. 157, 374–379. doi: 10.1016/j.snb.2011.04.059
Black, N. C. G., Rungger, I., Li, B., Maier, S., Cohen, L. F., Gallop, J. C., et al. (2018). Adsorption dynamics of CVD graphene investigated by a contactless microwave method. 2D Mater. 5:035024. doi: 10.1088/2053-1583/aac231
Bogner, A., Steiner, C., Walter, S., Kita, J., Hagen, G., and Moos, R. (2017). Planar microstrip ring resonators for microwave-based gas sensing: design aspects and initial transducers for humidity and ammonia sensing. Sensors 17:2422. doi: 10.3390/s17102422
Chen, W. T. S., and Mansour, R. R. (2018). Miniature gas sensor and sensor array with single- and dual-mode RF dielectric resonators. IEEE Trans. Microw. Theory Tech. 66, 1–8. doi: 10.1109/TMTT.2018.2854551
Chen, Z., Zheng, Y., Chen, K., Li, H., and Jian, J. (2017). Concentration estimator of mixed VOC gases using sensor array with neural networks and decision tree learning. IEEE Sens. J. 17, 1884–1892. doi: 10.1109/JSEN.2017.2653400
Chopra, S., Pham, A., Gaillard, J., Parker, A., and Rao, A. M. (2002). Carbon-nanotube-based resonant-circuit sensor for ammonia. Appl. Phys. Lett. 80, 4632–4634. doi: 10.1063/1.1486481
Cismaru, A., Aldrigo, M., Radoi, A., and Dragoman, M. (2016). Carbon nanotube-based electromagnetic band gap resonator for CH4 gas detection. J. Appl. Phys. 119, 1801–1804. doi: 10.1063/1.4944708
Dietrich, M., Hagen, G., Reitmeier, W., Burger, K., Hien, M., Grass, P., et al. (2017). Radio-frequency-based NH3-selective catalytic reduction catalyst control: studies on temperature dependency and humidity influences. Sensors 17:1615. doi: 10.3390/s17071615
Dragoman, M., Grenier, K., Dubuc, D., Bary, L., Plana, R., Fourn, E., et al. (2007). Millimeter wave carbon nanotube gas sensor. J. Appl. Phys. 101:106103. doi: 10.1063/1.2734873
El Bouazzaoui, S., Achour, M. E., and Brosseau, C. (2011). Microwave effective permittivity of carbon black filled polymers: Comparison of mixing law and effective medium equation predictions. J. Appl. Phys. 110:074105. doi: 10.1063/1.3644947
Fayaz, M., Jahandar Lashaki, M., Abdolrazzaghi, M., Zarifi, M. H., Hashisho, Z., Daneshmand, M., et al. (2019). Monitoring the residual capacity of activated carbon in an emission abatement system using a non-contact, high resolution microwave resonator sensor. Sens. Actuat. B Chem. 282, 218–224. doi: 10.1016/j.snb.2018.11.038
Fei, Y., Yu, H. X., Bo, Z., Ying, Z., and Zhu, Z. X. (2011). “Substrate integrated waveguide phase shifter,” in International Conference on Electronics (Ningbo: IEEE).
Fonseca, B. D., Rossignol, J., Bezverkhyy, I., Bellat, J. P., Stuerga, D., and Pribetich, P. (2014). VOCs detection by microwave transduction using zeolites as sensitive material. Proc. Eng. 87, 1019–1022. doi: 10.1016/j.proeng.2014.11.334
Fonseca, B. D., Rossignol, J., Bezverkhyy, I., Bellat, J. P., Stuerga, D., and Pribetich, P. (2015a). Detection of VOCs by microwave transduction using dealuminated faujasite DAY zeolites as gas sensitive materials. Sens. Actuat. B Chem. 213, 558–565. doi: 10.1016/j.snb.2015.02.006
Fonseca, B. D., Rossignol, J., Stuerga, D., and Pribetich, P. J. U. C. (2015b). Microwave signature for gas sensing: 2005 to present. Urban Clim. 14, 502–515. doi: 10.1016/j.uclim.2014.10.010
Gao, X., and Zhang, T. (2018). An overview: facet-dependent metal oxide semiconductor gas sensors. Sens. Actuat. B Chem. 277, 604–633. doi: 10.1016/j.snb.2018.08.129
Huang, X., Leng, T., Georgiou, T., Abraham, J., Raveendran Nair, R., Novoselov, K. S., et al. (2018). Graphene oxide dielectric permittivity at ghz and its applications for wireless humidity sensing. Sci. Rep. 8:43. doi: 10.1038/s41598-017-16886-1
Jun, J., Oh, J., Shin, D. H., Kim, S. G., Lee, J. S., Kim, W., et al. (2016). Wireless, room temperature volatile organic compound sensor based on polypyrrole nanoparticle immobilized ultrahigh frequency radio frequency identification tag. ACS Appl. Mater. Interfaces 8, 33139–33147. doi: 10.1021/acsami.6b08344
Kauffman, D. R., and Star, A. (2008). Carbon nanotube gas and vapor sensors. Angew. Chem. 47, 6550–6570. doi: 10.1002/anie.200704488
Kim, I.-D., Rothschild, A., and Tuller, H. L. (2013). Advances and new directions in gas-sensing devices. Acta Mater. 61, 974–1000. doi: 10.1016/j.actamat.2012.10.041
Korotcenkov, G. (2012). Handbook of Gas Sensor Materials Properties, Advantages and Shortcomings for Applications Volume 1: Conventional Approaches. New York, NY: Springer. doi: 10.1007/978-1-4614-7165-3
Kubersky, P., Syrovy, T., Hamacek, A., Nespurek, S., and Syrova, L. (2015). Towards a fully printed electrochemical NO2 sensor on a flexible substrate using ionic liquid based polymer electrolyte. Sens. Actuat. B-Chem. 209, 1084–1090. doi: 10.1016/j.snb.2014.12.116
Lee, J. S., Oh, J., Jun, J., and Jang, J. (2015). Wireless hydrogen smart sensor based on Pt/graphene-immobilized radio-frequency identification tag. ACS Nano 9, 7783–7790. doi: 10.1021/acsnano.5b02024
Lv, Y., Yu, H., Xu, P., Xu, J., and Li, X. (2018). Metal organic framework of MOF-5 with hierarchical nanopores as micro-gravimetric sensing material for aniline detection. Sens. Actuat. B-Chem. 256, 639–647. doi: 10.1016/j.snb.2017.09.195
Mitroy, J., Safronova, M. S., and Clark, C. W. (2010). Theory and applications of atomic and ionic polarizabilities. J. Phys. B Atom. Mol. Opt. Phys. 43:202001. doi: 10.1088/0953-4075/43/20/202001
Moos, R., Sahner, K., Fleischer, M., Guth, U., Barsan, N., and Weimar, U. (2009). Solid state gas sensor research in Germany - a status report. Sensors 9, 4323–4365. doi: 10.3390/s90604323
Moseley, P. T., and Williams, D. E. (1990). A selective ammonia sensor. Sens. Actuat. B-Chem. 1, 113–115. doi: 10.1016/0925-4005(90)80183-Z
Nicolas, A., Devautour-Vinot, S., Maurin, G., Giuntini, J. C., and Henn, F. (2008). Location and de-trapping energy of sodium ions in dehydrated X and Y faujasites determined by dielectric relaxation spectroscopy. Microporous Mesoporous Mater. 109, 413–419. doi: 10.1016/j.micromeso.2007.05.039
Occhiuzzi, C., Rida, A., Marrocco, G., and Tentzeris, M. (2011). RFID passive gas sensor integrating carbon nanotubes. IEEE Trans. Microw. Theory Tech. 59, 2674–2684. doi: 10.1109/TMTT.2011.2163416
Reiß, S., Schönauer, D., Hagen, G., Fischerauer, G., and Moos, R. (2011). Monitoring the ammonia loading of zeolite-based ammonia SCR catalysts by a microwave method. Chem. Eng. Technol. 34, 791–796. doi: 10.1002/ceat.201000546
Rossignol, J., Barochi, G., Fonseca, B. D., Brunet, J., Bouvet, M., Pauly, A., et al. (2012). Development of gas sensors by microwave transduction with phthalocyanine film. Proc. Eng. 47, 1191–1194. doi: 10.1016/j.proeng.2012.09.365
Rossignol, J., Barochi, G., Fonseca, B. D., Brunet, J., Bouvet, M., Pauly, A., et al. (2013). Microwave-based gas sensor with phthalocyanine film at room temperature. Sens. Actuat. B-Chem. 189, 213–216. doi: 10.1016/j.snb.2013.03.092
Rossignol, J., Stuerga, D., and Jouhannaud, J. (2010). Broadband microwave gas sensor: a coaxial design. Microw. Opt. Technol. Lett. 52, 1739–1741. doi: 10.1002/mop.25359
Rydosz, A., Brudnik, A., and Staszek, K. (2019). Metal oxide thin films prepared by magnetron sputtering technology for volatile organic compound detection in the microwave frequency range. Materials 12:877. doi: 10.3390/ma12060877
Sahner, K., Hagen, G., Schonauer, D., Reiss, S., and Moos, R. (2008). Zeolites–versatile materials for gas sensors. Solid State Ionics 179, 2416–2423. doi: 10.1016/j.ssi.2008.08.012
Staszek, K., Rydosz, A., Maciak, E., Wincza, K., and Gruszczynski, S. (2017). Six-port microwave system for volatile organic compounds detection. Sens. Actuat. B-Chem. 245, 882–894. doi: 10.1016/j.snb.2017.01.194
Tooski, S. B. (2010). Sense toxins/sewage gases by chemically and biologically functionalized single-walled carbon nanotube sensor based microwave resonator. J. Appl. Phys. 107, 14702–14708. doi: 10.1063/1.3277020
Tooski, S. B. (2011). Theoretical study of carbon-nanotube-based gas pressure sensors. J. Appl. Phys. 109, 622–236. doi: 10.1063/1.3525059
Tu, M., Wannapaiboon, S., Khaletskaya, K., and Fischer, R. A. (2015). Engineering zeolitic-imidazolate framework (ZIF) thin film devices for selective detection of volatile organic compounds. Adv. Funct. Mater. 25, 4470–4479. doi: 10.1002/adfm.201500760
Wang, D., Tian, L., Li, H., Wan, K., Yu, X., Wang, P., et al. (2019). Mesoporous ultrathin SnO2 nanosheets in situ modified by graphene oxide for extraordinary formaldehyde detection at low temperatures. ACS Appl. Mater. Interfaces 11, 12808–12818. doi: 10.1021/acsami.9b01465
Wang, Z., Huang, L., Zhu, X., Zhou, X., and Chi, L. (2017). An ultrasensitive organic semiconductor NO2 sensor based on crystalline TIPS-pentacene films. Adv. Mater. 11:1703192. doi: 10.1002/adma.201703192
Wei, T.-C., and Hillhouse, W. H. (2006). Ion transport in the microporous titanosilicate ETS-10. J. Phys. Chem. B 110, 13728–13733. doi: 10.1021/jp061037u
Xie, C., Oganov, A. R., Dong, D., Liu, N., Li, D., and Debela, T. T. (2015). Rational design of inorganic dielectric materials with expected permittivity. Sci. Rep. 5:16769. doi: 10.1038/srep16769
Xing, R., Xu, L., Song, J., Zhou, C., Li, Q., Liu, D., et al. (2015). Preparation and gas sensing properties of In2O3/Au nanorods for detection of volatile organic compounds in exhaled breath. Sci. Rep. 5:10717. doi: 10.1038/srep10717
Xu, X. W., Wang, J., and Long, Y. C. (2006). Zeolite-based materials for gas sensors. Sensors 6, 1751–1764. doi: 10.3390/s6121751
Yamazoe, N., and Shimanoe, K. (2009). New perspectives of gas sensor technology. Sens. Actuat. B Chem. 138, 100–107. doi: 10.1016/j.snb.2009.01.023
Yuan, W. J., and Shi, G. Q. (2013). Graphene-based gas sensors. J. Mater. Chem. A 1, 10078–10091. doi: 10.1039/c3ta11774j
Zarifi, M. H., Ayaz, M., Goldthorp, J., Abdolrazzaghi, M., Hashisho, Z., and Daneshmand, M. (2015a). Microbead-assisted high resolution microwave planar ring resonator for organic-vapor sensing. Appl. Phys. Lett. 106, 12868–12882. doi: 10.1063/1.4907944
Zarifi, M. H., Gholidoust, A., Abdolrazzaghi, M., Shariaty, P., Hashisho, Z., and Daneshmand, M. (2018). Sensitivity enhancement in planar microwave active-resonator using metal organic framework for CO2 detection. Sens. Actuat. B Chem. 255, 1561–1568. doi: 10.1016/j.snb.2017.08.169
Zarifi, M. H., Rahimi, M., Daneshmand, M., and Thundat, T. (2016). Microwave ring resonator-based non-contact interface sensor for oil sands applications. Sens. Actuat. B Chem. 224, 632–639. doi: 10.1016/j.snb.2015.10.061
Zarifi, M. H., Shariaty, P., Hashisho, Z., and Daneshmand, M. (2017). A non-contact microwave sensor for monitoring the interaction of zeolite 13X with CO2 and CH4 in gaseous streams. Sens. Actuat. B Chem. 238, 1240–1247. doi: 10.1016/j.snb.2016.09.047
Zarifi, M. H., Sohrabi, A., Shaibani, P. M., Daneshmand, M., and Thundat, T. (2015b). Detection of volatile organic compounds using microwave sensors. Sens. J. IEEE 15, 248–254. doi: 10.1109/JSEN.2014.2345477
Zarifi, M. H., Thundat, T., and Daneshmand, M. (2015c). High resolution microwave microstrip resonator for sensing applications. Sens. Actuat. A Phys. 233, 224–230. doi: 10.1016/j.sna.2015.06.031
Zheng, Y., Li, X., and Dutta, P. K. (2012). Exploitation of unique properties of zeolites in the development of gas sensors. Sensors (Basel) 12, 5170–5194. doi: 10.3390/s120405170
Keywords: gas sensor, microwave transduction, polarization, propagative structure, electromagnetic wave
Citation: Li F, Zheng Y, Hua C and Jian J (2019) Gas Sensing by Microwave Transduction: Review of Progress and Challenges. Front. Mater. 6:101. doi: 10.3389/fmats.2019.00101
Received: 16 March 2019; Accepted: 17 April 2019;
Published: 03 May 2019.
Edited by:
Xiaogan Li, Dalian University of Technology (DUT), ChinaReviewed by:
Jianxin Yi, University of Science and Technology of China, ChinaDing Wang, University of Shanghai for Science and Technology, China
Copyright © 2019 Li, Zheng, Hua and Jian. This is an open-access article distributed under the terms of the Creative Commons Attribution License (CC BY). The use, distribution or reproduction in other forums is permitted, provided the original author(s) and the copyright owner(s) are credited and that the original publication in this journal is cited, in accordance with accepted academic practice. No use, distribution or reproduction is permitted which does not comply with these terms.
*Correspondence: Yangong Zheng, zhengyangong@nbu.edu.cn
Changzhou Hua, huachangzhou@nbu.edu.cn