Synthesis strategies of iron nitrides at carbon cloth as battery-like electrode for hybrid supercapacitors
- Department of Process Engineering and Technology of Polymer and Carbon Materials, Wrocław University of Science and Technology, Wrocław, Poland
In recent years, hybrid supercapacitors (HSCs) or supercapatteries which combine a capacitor-type electrode with an electrode based on materials exhibiting a Faradaic (battery-like) response have been intensively investigated for next-generation energy storage applications. HSCs attracted great attention due to a significant increase of maximum energy density stored while providing stable long-term performance and good rate capability. However, the electrochemical performance of the device is closely related to the inherent properties of the electrode material, including morphology and structure. In this paper, we present synthesis protocols for iron oxide/hydrophilic carbon cloth (Fe2O3@hCC) composite electrodes and their electrochemical performance as a negative electrode operating in an alkaline electrolyte. Two environmentally friendly, scalable and facile synthesis approaches were applied, including hydrothermal treatment and direct electrodeposition. Next, the Fe2O3@hCC electrodes were treated to convert iron oxide to iron nitride (Fe2N). The results showed that the synthesis of the precursor for iron nitride has a direct impact on morphology, crystalline structure and electrochemical performance. Furthermore, the amorphous Fe2N obtained from electrodeposition exhibited significantly better Faradaic behavior, achieving a specific capacity up to 186 mAh g-1, 66% higher than the composite electrode with Fe2N from the hydrothermal approach.
1 Introduction
The growing demand for clean and sustainable energy sources on a global scale has induced significant research efforts in the field of electrochemical energy storage. Simultaneously required are advances in energy conversion devices and the development of efficient and multipurpose energy storage solutions for the ubiquitous adoption of renewable energy systems, electric transportation, and smart grid integration. Batteries and supercapacitors play a crucial role in the efficient capture, storage, and delivery of electrical energy on demand (Yadlapalli et al., 2022). They provide an essential connection between intermittent energy sources, such as solar and wind, and the constant power demands of contemporary society.
As a result, a great deal of focus has been placed on the development of supercapacitor electrode materials to improve energy storage performance and meet the high demand for modern electronic devices. Electrode materials based on transition metal compounds, such as metal oxides, metal hydroxides, metal sulfides, and metal nitrides, are regarded as optimal for highly efficient electrochemical capacitors and hybrid supercapacitors (HSCs) (Zhi et al., 2013; Augustyn et al., 2014; Chi et al., 2018; Moyseowicz, 2019). Transition metal nitrides have the ability to substitute standard materials in a variety of applications, including electrical devices, gas sensing, ceramics, and environmental remediation. Due to their low density, large surface area, electrical properties, enhanced chemical activity, and high conductivity, nanoparticles with diverse morphologies and structures have recently attracted the interest of researchers (Zhou et al., 2019; Idrees et al., 2021). Nevertheless, similar to other conversion-type materials, obstacles such as repeated volume variation, a highly air-sensitive surface, and simple oxidation significantly degrade their properties (Adalati et al., 2022). Among the various metal nitrides, Fe2N is a very promising electroactive material because of its high electrical conductivity, low cost, easy preparation and environmental friendliness, however, limited amount of publications on its application in energy storage devices (Xu et al., 2017; Śliwak et al., 2017; Feng et al., 2023; Zhao et al., 2023).
The choice of iron is primarily motivated by its remarkable electrochemical capabilities and its abundance in nature, making it an economically feasible choice. Iron exhibits many valence states, such as Fe0, Fe2+, Fe3+, etc., and possesses a diverse range of redox chemistry (Feng et al., 2023). These characteristics contribute to its ability to achieve high specific capacitance values. The majority of transition metal oxides/hydroxides are commonly utilized as positive electrodes, but iron oxides/hydroxides/nitrides exhibit a stable and extensive operating range in the negative potential. This characteristic renders them highly promising as negative electrodes for asymmetric or hybrid supecapacitors (Zhang et al., 2016).
Carbon materials are extensively employed in energy storage devices, particularly in electric double-layer capacitors (EDLCs) (Borenstein et al., 2017). Carbon-based materials are a very interesting group in materials science for their superior mechanical strength, structural stability, and electrical conductivity, while also being lightweight and cost-effective (Moyseowicz et al., 2021). Furthermore, carbon cloth, which is a crisscrossed interweaving structure of carbon threads that are composed of carbon fibers or nanofibers, is the most widely used current collector in the field of supercapacitors, particularly flexible supercapacitors (Chen et al., 2018). They increase the surface area of the electrodes and offer flexibility (Gong et al., 2021).
Current research on supercapacitors indicates that new strategies must be implemented to achieve comparable energy density to lithium-ion batteries. Consequently, a new strategic design for HSCs has been developed (Jin et al., 2008; Dubal et al., 2015; Moyseowicz and Moyseowicz, 2021). In such a device, the electrode configuration consists of one capacitive or pseudo-capacitive electrode and one battery-type electrode. By combining the benefits of supercapacitor and battery electrodes, this type of electrode configuration in supercapacitors makes it possible to get high energy and power densities (Cherusseri et al., 2019). HSCs employing battery electrodes can bridge the divide between standard supercapacitors and lithium-ion batteries.
In the present study, we investigated the influence of the synthesis procedure on the morphology, crystalline structure, and electrochemical performance of Fe2O3 deposited on the carbon cloth substrate and their iron nitride analogues. Two environmentally friendly, scalable, and straightforward synthesis methods, including hydrothermal treatment and direct electrodeposition, were utilized. The results show that the precursor synthesis for iron nitride has a direct effect on the iron-based nanoparticle coating properties, which greatly impacts the electrode electrochemical behavior in a 6 mol L-1 KOH electrolyte. The binder-less electrodes prepared from the direct electrodeposition method displayed much superior Faradaic behavior, yielding a specific capacity of up to 186 mAh g-1, greater than the composite electrode containing Fe2N from the hydrothermal method.
2 Experimental section
2.1 Hydrophilic carbon cloth preparation
Carbon fiber cloth, supplied by the FuelCellStore, was used as a substrate for the deposition of iron oxides. Before any treatment, the commercial carbon cloth (CC) was cut into 2 × 1 cm2 pieces and washed sequentially in acetone, isopropanol and water. Its surface was oxidized by the procedure applied in other study (Kordek et al., 2019). Pieces of carbon cloth were placed in an alumina crucible and calcined in an air atmosphere at 500°C for 2 h. The obtained material was labelled hCC (hydrophilic carbon cloth).
2.2 Synthesis of Fe2O3 nanostructures on carbon cloth
2.2.1 Hydrothermal precipitation
All the chemical reagents in this experiment were used as received without any further purification. The synthesis of Fe2O3 was performed in a stainless steel autoclave using a hydrothermal approach. Firstly, a 3 mL of 2.5 mM mL-1 of Fe(NO3)3 aqueous solution to yield was dissolved in 200 mL Mili-Q water on the magnetic stirrer. Then, the solution was placed into the stainless-steel autoclave and finally 5 mL of 10% ammonia solution was dropped. Hydrothermal treatment was performed at 180°C for 6 h. After the reaction, the autoclave was allowed to cool down to a room temperature. The obtained product was centrifuged and washed with Milli-Q water several times and dried in a vacuum oven at 60°C for 24 h.
Fe2O3 from hydrothermal synthesis was deposited on a hCC using drop-casting technique. Fe2O3-HT was dispersed in acetone using an ultrasonic bath for 30 min. The suspension of Fe2O3 was dropped into the hCC surface to obtain binder-free electrode with the 20 wt.% amount Fe2O3. Finally, the Fe2O3@hCC-HT was dried until the acetone completely evaporated.
2.2.2 Electrochemical deposition
The Fe2O3 films were plated on pieces of hCC by cathodic electrodeposition. Piece of carbon cloth was immersed in electroplating bath composed of 20 mM Fe(NO3)3 aqueous solution and connected as working electrode in a standard three-electrode setup with Ag/AgCl as a reference and graphite rod as a counter electrode. A cathodic potential of −1.4 V vs. Ag/AgCl was supplied to the working electrode for 3 h. After the deposition, the sample labelled Fe2O3@hCC-E was washed thoroughly with water and dried at the temperature of 60°C overnight. The increase in the mass of the carbon cloth after electrodeposition of Fe2O3 was 20.2 wt%.
2.3 Preparation of the Fe2N nanostructures at carbon cloth
The resultant products (Fe2O3@hCC-E and Fe2O3@hCC-HT) were introduced in the bottom of a quartz boat and inserted into the center of a horizontal reactor. The reactor was heated up to 700 °C in the nitrogen flow of 9 L h-1 with a heating rate of 10 ° min-1. Afterwards, the ammonia (9 L h-1) was introduced into the reactor for 2 h at the targeted temperature. Then, the reactor was cooled down to a room temperature under a nitrogen flow. The Fe2N@hCC samples were kept under inert atmosphere before their application in the electrochemical system.
2.4 Structural and chemical characterization
The morphologies of iron compounds on carbon cloth were analyzed by field-emission scanning electron microscopy (FESEM, Merlin Zeiss) with an accelerating voltage of 3 kV. The structural characteristics of iron compounds on carbon cloth were revealed X-ray diffraction (XRD), performed with an Ultima IV Rigaku diffractometer using a Cu Ka2 radiation source (λ = 1.54056 Å) and high resolution transmission electron microscopy (HRTEM, FEI Titan G3). The chemical compositions of iron oxides and nitrides on carbon cloth were determined by X-ray photoelectron spectroscopy (XPS) using a PHI 5000 VersaProbe (ULVAC-PHI) spectrometer.
2.5 Electrochemical measurements
The binder-free electrodes were cut out of carbon cloth with iron-based coating in the form of pellets with a geometric surface area of 0.64 cm2. For electrochemical measurements, a three-electrode configuration was assembled in a Swagelok system. The measurements were performed in a 6 mol L -1 KOH aqueous solution using gold current collectors to avoid corrosion and to preserve comparable experimental conditions. Measurements were conducted with a potentiostat–galvanostat VSP Biologic in a voltage range of −1.1÷0 V with a pitch-based activated carbon counter electrode and a mercury oxide reference electrode. The electrochemical properties of iron-based samples were determined by cyclic voltammetry at a voltage scan rate of 1–100 mV s-1 and galvanostatic charge-discharge at current densities in the range 0.5–10 A g-1 and EIS measurements were recorded under an open circuit potential in the frequency range of 200 kHz to 10 mHz. The specific capacity was expressed in mAh per mass of active material in one electrode. The specific capacity values (Qs/mAh g -1) were calculated from the cyclic voltammetry curves and galvanostatic discharge profiles using Eqs 1, 2, respectively.
where I is the current (A), Δt is the discharge time (s), ν is the scan rate (V s-1), and mel is the mass of the active material in the electrode (g).
For the electrochemically active surface area, electrodes were measured in the non-faradaic potential region. The measurements were performed in an three-neck electrochemical cell filled with 6 mol L-1 KOH as an electrolyte in a standard 3-electrode system. 1 cm × 1 cm pieces of carbon cloth with deposited Fe-based material and held by a Pt clip were used directly as working electrodes, while Hg|HgO electrode was used as a reference and a graphite rod as a counter electrode. The capacitive currents were derived from cyclic voltammetry plots acquired in a potential window between −0.1 and 0.1 V vs. Hg|HgO at different scan rates.
3 Results and discussion
In Figure 1 the comparison between the morphologies of the iron-based films deposited by the two techniques, namely from electrodeposition and hydrothermal/drop casting technique is presented, both before and after nitridation. Comparison between Figures 1A, B show the distinct difference between Fe2O3 obtained by the two methods. As can be seen, the morphology of the electrodeposited film is composed of continuously interconnected nanosheets, while hydrothermal technique led to formation of the film in the form of rather uniform nanoparticles. Partially uncovered carbon cloth surfaces also suggests that the deposition of Fe2O3 on the substrate is not homogeneous within the sample.
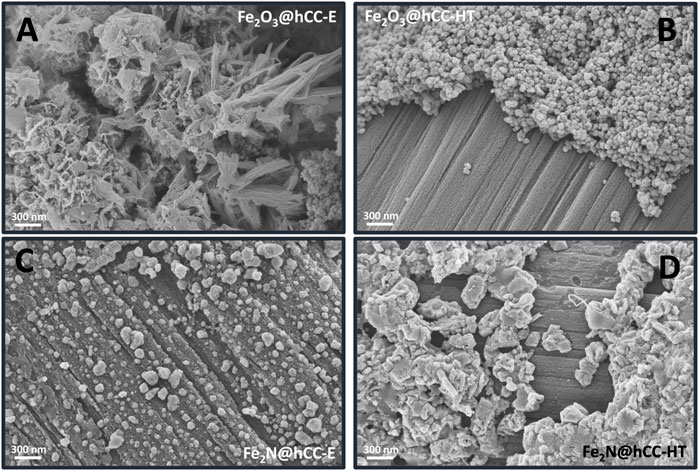
FIGURE 1. SEM images of iron oxides and iron nitrides materials on a carbon cloth prepared by hydrothermal and electrodeposition techniques.
Figure 1C reveals that thermal treatment of the Fe2O3@hCC-E sample in an ammonia atmosphere resulted in a radical change in the film morphology. As can be seen, a porous interconnected structure was converted into polydisperse micro- and nanoparticles evenly distributed on the carbon cloth surface. On the other hand, the thermal conversion of Fe2O3@hCC-HT in ammonia resulted in the aggregation of nanoparticles and formation of bulk particles (Figure 1D). As can be seen, the nitridation procedure, even if it is performed under the identical conditions, had a very different effect on two oxide materials and the morphology of nitride samples obtained by the two techniques is also distinctly different.
Furthermore, XRD diffraction patterns (Figure 2) present very different structures for the obtained samples. As can be seen, the iron oxide sample obtained by the hydrothermal technique shows a typical pattern characteristic for hematite (α-Fe2O3—JCPDS 33–0664) phase with reflexes located at 2Θ of 24.2°, 33.2°, 35.7°, 40.8°, 49.5°, 54.1°, 57.5°, 62.3°, 64.0°, 71.9° and 75.4° (Geng et al., 2012; Wu et al., 2013; Moyseowicz et al., 2017). The deposited film is definitely well-crystallized due to sharp feature of the reflexes. Besides that, broad signals located at 2Θ of c.a. 26° and 44° can be seen in this diffractogram, that are characteristic for carbon cloth (Walendzik et al., 2023). The nitridation of the Fe2O3@hCC-HT sample also resulted in the formation of well-crystallized product. Diffractogram of Fe2N@hCC-HT shows, besides signals derived from carbon cloth, a diffraction pattern typical for ζ-Fe2N phase (JCPDS 50–0958), with reflexes located at 2Θ of 35.4°, 37.4°, 40.1°, 40.9°, 43.0°, 56.9°, 68.1° and 76.0° (Śliwak et al., 2017). The narrow width of the reflexes suggests the big nitride crystallite size. On the other hand, diffractograms of both samples prepared with aid of electrodeposition technique are very different from those of materials prepared hydrothermally. Despite the presence of the thick electrodeposited film in the Fe2O3@hCC-E sample (as evidenced by SEM), diffractogram of this sample has only signals that can be attributed to carbon cloth. This leads to the conclusion that the deposited film is amorphous or sub-nanocrystalline (Valvoda, 1996). What is more, an iron nitride (Fe2N@hCC-E) formed upon amination of this sample shows similar diffractogram, therefore the nanoparticles that can be seen in its SEM image are also of amorphous structure.
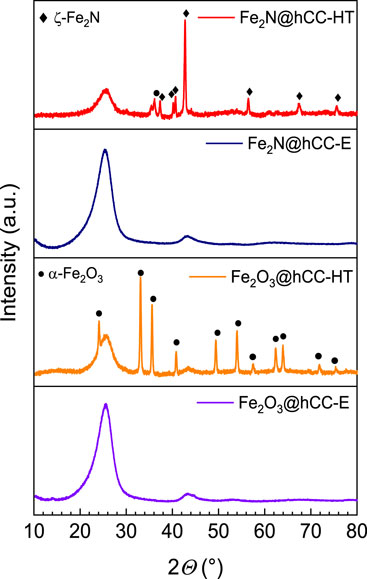
FIGURE 2. XRD patterns of the iron oxides and iron nitrides materials prepared by hydrothermal and electrodeposition techniques.
In order to further characterize the chemical states of elements on the surfaces of the 4 electrodes, XPS technique was applied. As can be noticed in Table 1, iron is present in all samples, indicating that in all samples, iron-based films are formed. The iron concentration in the samples obtained by hydrothermal treatment is evidently higher than in electrodeposited materials, suggesting that more active material was deposited by the former technique. Fe/O atomic ratio for both samples with iron oxide films is similar (0.54 and 0.49 for hydrothermal and electrodeposition techniques, respectively), indicating similar surface chemical states of both oxides.
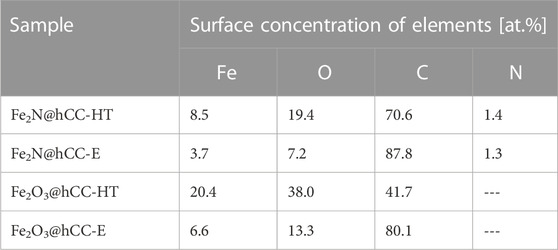
TABLE 1. Surface atomic concentrations of elements in nitride and oxide samples prepared by hydrothermal and electrodeposition techniques.
Similar atomic concentrations of nitrogen can be found in both samples obtained after nitridation. For both samples Fe/N ratio (of 6.1 for Fe2N@hCC-HT and 2.8 for Fe2N@hCC-E) is higher than stoichiometric for Fe2N (2) suggesting that in both materials iron can partially be found in the surface in different form. It is possible that upon the thermal treatment, iron nitride with lower nitrogen content was formed (Park et al., 2016). It can be pointed out here, that metal nitrides usually undergo surface oxidation with the formation of metal oxides (Dong et al., 2017).
Furthermore, high resolution XPS spectra in the Fe 2p and N 1s regions are presented in Figure 3 and Figure 4. In all spectra distinct Fe 2p3/2 and Fe 2p1/2 peaks can be seen at binding energies of 711.6 and 725.4 eV, respectively, that are characteristic for α-Fe2O3 phase. Typical for this phase are also the satellite features located at binding energies of 719.5 and 733.0 eV (Cao et al., 2015; Yan et al., 2017). The fact that both oxide and nitride materials possess nearly identical XPS spectra in the Fe 2p region can be explained by the oxidation of surface Fe2N with the formation of thin Fe2O3 layer. XPS is a surface sensitive technique which analyzes only a few atomic monolayers, so majority of the signal comes from this oxidized layer. Surface oxidation of Fe2N into oxide phase was widely discussed in the literature (Dong et al., 2017). As oxidized layer is very thin, therefore its diffraction reflexes cannot be distinguished in XRD patterns of nitride samples.
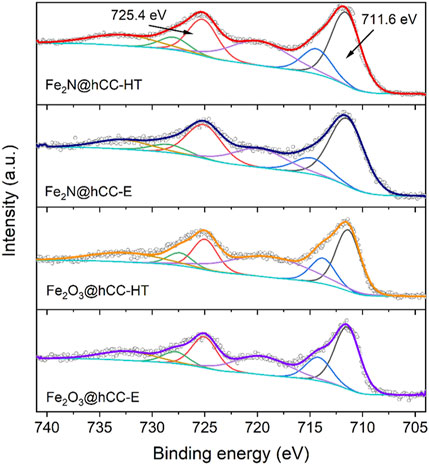
FIGURE 3. High-resolution XPS spectra in Fe 2p region of the iron oxides and iron nitrides materials prepared by hydrothermal and electrodeposition techniques.
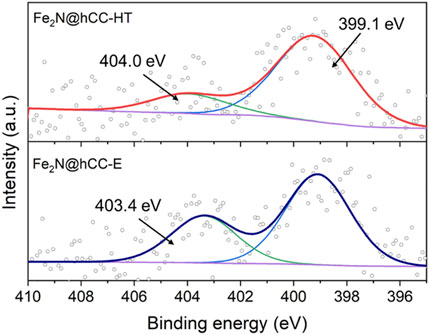
FIGURE 4. High-resolution XPS spectra in N 1s region of the iron nitrides materials prepared by hydrothermal and electrodeposition techniques.
High resolution N 1s spectra are presented in Figure 4 for both nitride samples. The spectra can be deconvoluted into two peaks. In both spectra, the most intensive component is located at the binding energy of 399.1 eV and can be attributed to metal-nitrogen bonds or pyridinic N, as their binding energy values are almost the same (Wu et al., 2011; Peng et al., 2013; Yu et al., 2018; Kohila Rani et al., 2020). The other peak can be distinguished at 404.0 and 403.4 eV for Fe2N@hCC-HT and Fe2N@hCC-E, respectively. Signals in this spectral region (between 403 and 406 eV) are usually attained to nitrogen-oxygen bonds (Arrigo et al., 2010; Kordek-Khalil et al., 2022). Characterizations of all samples show that the two nitride samples are both composed of Fe2N phase covered by thin Fe2O3 layer generated by atmospheric oxidation of the nitrides. What differs between the samples is the film morphology as well as structure, which in the case of the sample prepared by hydrothermal technique is crystalline with distinct XRD reflexes and is amorphous for the electrodeposited film. Similar is the case with source metal oxides, which also possess similar surface characteristics but differ in morphology and structure. In general, electrodeposition leads to the formation of amorphous films, and the hydrothermal method forms well-crystallized nanocrystalline products.
Figure 5 presents HRTEM images of the samples after the nitridation procedure. As can be seen in the bright-field images, the Fe2N@hCC-HT sample is composed of smaller nanoparticles than Fe2N@hCC-E, which is consistent with SEM images. Furthermore, high-resolution TEM images of the samples were also acquired to get an insight into the structure of the materials. As can be seen in Figure 5B, the sample Fe2N@hCC-E possesses small crystalline domains (with a diameter of ca. 10 nm) in a rather amorphous matrix. The interplanar distance of 0.210 nm in these domains corresponds to the (011) crystalline plane of hexagonal Fe2N (Jiang et al., 2019), which is also confirmed by the SAED ring (Supplementary Figure S1). On the other hand, Fe2N@hCC-HT sample is evidently more crystalline with a fringe spacing of 0.25 nm (Figure 5D) and can be attributed to Fe2O3, which based on XPS results, is a product of surface oxidation of Fe2N (Chen et al., 2016). Additionally, the SAED ring patterns also implies the Fe2O3 presence on the surface of the Fe2N@hCC-HT (Supplementary Figure S1).
Figure 6 displays the findings of the CV measurements, which reveal distinct differences in the electrochemical behavior between iron oxides and iron nitrides, as well as comparision between materials from electrodeposition and hydrothermal/drop casting method. The iron-based compounds exhibit clear redox peaks when a lower cutoff potential is used (i.e., below −1.0 V vs. Hg|HgO). The Fe2O3@hCC-HT electrode shows poor electrochemical behavior (Figure 6A), with a very weak peaks of an Fe3+ ⇋ Fe2+ redox pair and a small peak related to Fe2+ reduction to metallic Fe0 at a potential of −1.05 V vs. Hg|HgO electrode (Zhang et al., 2016). However, the Fe2O3 obtained from the electrodeposition process presents improved electrochemical performance compared to its hydrothermal counterpart (Figure 6B). The CV curve of Fe2O3@hCC-E shows the cathodic peak attributed to Fe2+ reduction to metallic Fe0, however due to its nanosheet-like morphology, the additional peak appeared at −0.71 V vs. Hg|HgO electrode, related to the conversion of the intermediate phase of adsorbed iron hydroxide to Fe2+ (Li et al., 2015). The effect of a high temperature nitridation of the Fe2O3@hCC electrodes is very pronounced and greatly improves the electrochemical response of converted iron oxides to nitrides. Due to the increase in conductivity of the Fe2N form, the CV profile of the Fe2N@hCC-HT presents distinct redox pair related to the transition of Fe3+ to Fe2+ at the potentials value of −0.97 V vs. Hg|HgO for cathodic and −0.64 V vs. Hg|HgO for anodic peak (Xu et al., 2017; Zhao et al., 2023), therefore indicating a battery-type behavior of the Fe2N in an alkaline electrolyte. Furthermore, the CV curve revealed that during electrochemical measurement, the Fe2N@hCC-E sample undergoes further reduction to metallic iron, showing a peak above 1.0 V vs. Hg|HgO, which may influence a Fe3+ ⇋ Fe2+ redox potential, shifting it towards more positive values by 70 mV (Li et al., 2015). Finally, even at a low scan rate of 5 mV s−, the peak correlated to the hydrogen evolution reaction is not observed for the Fe2N@hCC samples, due to the increased hydrogen evolution potential of iron nitride (Theerthagiri et al., 2020).
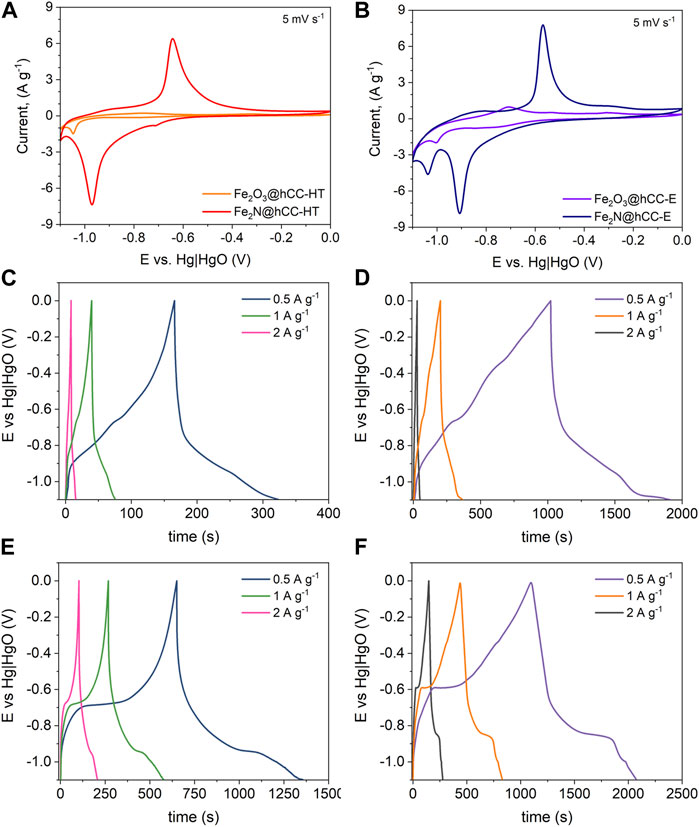
FIGURE 6. Electrochemical measurements results from a three-electrode cell: CV curves at a scan rate of 5 mV s-1 (A,B) and GCD profiles at different current densities of the Fe2O3@hCC-HT (C), Fe2O3@hCC-E (D), Fe2N@hCC-HT (E) and Fe2N@hCC-E (F).
The GCD measurements confirm the results obtained from the CV measurements. The Fe2O3@hCC electrodes show a distorted, quasi-triangular shape, with an linear part above −0.8 V vs. Hg|HgO, which is mostly related to the charge stored in the Fe2+ to Fe0 transition (Figures 6C, D). Moreover, the time required for discharge of the Fe2O3@hCC-E is over 6 times longer than that of Fe2O3@hCC-HT at a current density of 0.5 A g-1. The nitridation of the Fe2O3@hCC electrodes changes significantly the GCD profiles, as visible plateaus are observed, which correspond to the redox peaks of cyclic voltammograms at −0.71 ÷ −0.64 V and −1.05 ÷ −0.97 V vs. Hg|HgO (Figures 6E, F), thus confirming the Fe2N battery-like electrochemical behavior (Simon et al., 2014; Xu et al., 2017).
The relationships between the specific capacity and scan rate are presented in Figure 7A. The highest specific capacity at a scan rate of 1 mV s−1 is achieved by the Fe2N@hCC-E sample, with a value of 186 mAh g−1, followed by Fe2O3@hCC-E (177 mAh g−1), showing the superiority of the electrodeposition method over the hydrothermal synthesis combined with drop casting technique. The specific capacity of Fe2N@hCC-HT was 112 mAh g−1, 66% less than its counterpart from the electrodeposition procedure, while the lowest specific capacity value of 43 mAh g-1 was measured for Fe2O3@hCC-HT sample. Furthermore, from the discharge time of the GCD profiles, the specific capacity values (Qs) can be calculated, accordingly to the Equation 2 and at a current density of 0.5 A g-1 the Qs were 22, 126, 98 and 135 mAh g-1, Fe2O3@hCC-HT, Fe2O3@hCC-E, Fe2N@hCC-HT and Fe2N@hCC-E, respectively, corresponding to the performance between 2 and 5 mV s-1 from the CV measurements. The aggregated, spherical-like morphology does not provide enough active sites for redox reactions; thus, iron-based films from hydrothermal treatment have lower charge storage capability. Moreover, the small nanoparticle size of a thin iron nitride film in the Fe2N@hCC-E sample and the amorphous nature of the iron oxide precursor led to the improved electrochemical response (Zhang et al., 2016; Eftekhari and Mohamedi, 2017). Under increasing scan rate up to 100 mV s-1, the Fe2N@hCC electrodes show over 5-times higher specific capacity than their Fe2O3 precursors.
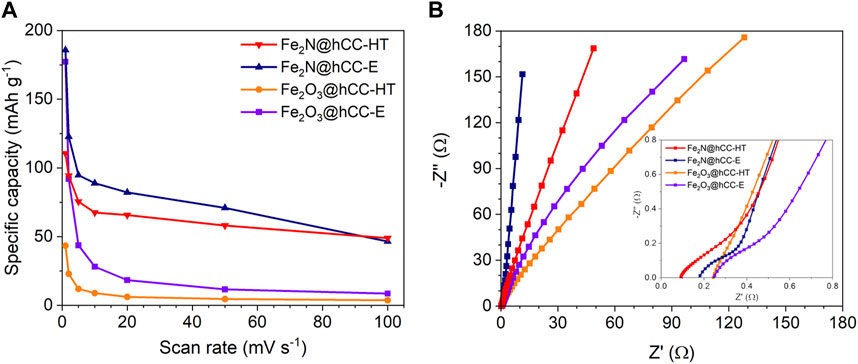
FIGURE 7. Specific capacity versus scan rate (A) and Nyquist plots with an inset representing the high-frequency region (B) of the iron oxides and iron nitrides materials prepared by hydrothermal and electrodeposition techniques.
The electrochemically active surface areas of the samples do not directly correlate with material activities as supercapacitor electrodes (Supplementary Figure S2). In general, the areas of electrodes fabricated using electrodeposition are higher than those of the samples prepared by the hydrothermal technique. Furthermore, as can be seen the nitridation of electrodeposited film results in decrease of electrochemically active surface while the effect is reverse for hydrothermal materials. This is consistent with the SEM images that show material shrinking in case of electrodeposited electrode and particles growth in case of hydrothermally obtained films. The limited electrochemically active surface area seen in the Fe2O3@hCC-HT electrode may be attributed to the nanoscale dimensions of its particles, which could potentially lead to a lack of electrical contact between some nanoparticles and the hCC electrode.
EIS measurements were performed to gain further insight into the electrochemical characteristics of the synthesized materials, and the results are presented in the form of a Nyquist plots (Figure 7B). Supplementary Figure S3 presents the fitted equivalet circuit which consists of bulk resistance (Rb), a constant phase elements (CPE), resistance related to surface porosity (Rp), a charge transfer resistance (Rct) and the Warburg impedance (Wu et al., 2021). The joint electrolyte resistance and current collector resistance defined as bulk resistance (Rb), determined from the intersection of the Z′ were 0.09, 0.18 and 0.25 Ω for Fe2N@hCC-HT, Fe2N@hCC-E and both Fe2O3@hCC, respectively (Gajewska et al., 2023). The Rp values were 0.23, 0.19, 0.61 and 58.4 Ω for Fe2N@hCC-HT, Fe2N@hCC-E, Fe2O3@hCC-E and Fe2O3@hCC-HT, respectively, suggesting improved conductivity within iron-based films obtained from electrodeposition technique. No distinct semicircle in the high-frequency range of the Nyquist plots was observed, which suggests minimized resistance between the binder-free electrode made of iron compounds deposited at the hCC and a current collector (Allison and Andreas, 2019). The charge transfer resistance Rct was significantly lower for iron nitrides than their Fe2O3 counterparts, which implies that nitrides exhibit much faster electron transfer during the electrochemical reaction (Fe2N@hCC-HT (0.22 Ω) vs. Fe2O3@hCC-HT (0.41 Ω) and Fe2N@hCC-E (0.05 Ω) vs. Fe2O3@hCC-E (0.21 Ω)) (Jiang et al., 2016). Next, a straight 45° line indicates the presence of Warburg impedance, which corresponds to diffuse layer resistance, and then in the low-frequency region, the more inclined the plot is towards the -Z″ axis, the lower the resistance related to the equilibrium differential capacitance. Among all tested samples, Fe2N@hCC-E exhibits the most vertical line, confirming the formation of an electric double layer at the electrode/electrolyte interface, further indicating a good electric charge storage performance (Mei et al., 2018; Zhao et al., 2023). The EIS data is in good agreement with the CV and GCD measurements, showing that Fe2N exhibits better electrochemical performance than Fe2O3, an additionally confirming that the electrodeposition method for iron nitride precursor is more efficient and promising approach than hydrothermal and drop casting methods.
The long-term performance of the electrode material is another factor in the evaluation of its applicability in energy storage devices. The results of the cyclic stability tests for 2000 cycles at a current density of 1 A g-1 are presented in Supplementary Figure S4. While the long-term performance of the Fe2N@hCC electrodes from both preparation methods is very close, the Fe2O3-based electrodes show significant discrepancies, emphasizing the superiority of the electrodeposition method over the hydrothermal/drop casting technique. The highest capacity retention was observed for Fe2O3@hCC-E (65%), followed by Fe2N@hCC-E (61%), Fe2N@hCC-E (56%), and Fe2O3@hCC-HT (36%). The trend is similar to the results of the electrochemically active surface area, where materials for electrodeposition exhibit a higher surface accessible for EDL charge storage, resulting in slower electrochemical dissolution of Fe-based compounds (Wang et al., 2021).
4 Conclusion
This work presents a study on the influence of the synthesis protocol of Fe2O3 films deposited on carbon cloth, which are the precursors for Fe2N on carbon cloth binder-free electrodes. The results of the as-prepared iron-based electrodes show that electrodeposition and hydrothermal/drop casting techniques both before and after nitridation reveal distinct differences between the two methods. Hydrothermal synthesis yields highly crystalline α-Fe2O3, while XRD diffraction patterns show that from the direct electrodeposition, amorphous Fe2O3 interconnected nanosheets are present on the surface of a carbon cloth. XPS studies revealed a superficial oxidation of the Fe2N to Fe2O3 due to the exposure to air. The study demonstrates that both iron oxide and iron nitride from the electrodeposition synthesis exhibit different and superior electrochemical behavior and performance compared to their hydrothermal counterparts. The Fe2N@hCC-E sample exhibits the highest specific capacity at a scan rate of 1 mV s−1, with a value of 186 mAh g−1. The obtained results show promising potential for the optimization and application of Fe2N@hCC from combined electrodeposition and nitridation protocols as a binder-free negative electrode for hybrid supercapacitors.
Data availability statement
The raw data supporting the conclusion of this article will be made available by the authors, without undue reservation.
Author contributions
KK-K: Conceptualization, Formal Analysis, Investigation, Methodology, Writing–original draft. AM: Formal Analysis, Investigation, Methodology, Writing–original draft. AKM: Conceptualization, Funding acquisition, Investigation, Supervision, Writing–review and editing.
Funding
The author(s) declare financial support was received for the research, authorship, and/or publication of this article. The following research was financially supported by the Small Grant Scheme–NOR/SGS/DesignHyCap/0189/2020 funded by the National Centre for Research and Development, Poland.
Conflict of interest
The authors declare that the research was conducted in the absence of any commercial or financial relationships that could be construed as a potential conflict of interest.
Publisher’s note
All claims expressed in this article are solely those of the authors and do not necessarily represent those of their affiliated organizations, or those of the publisher, the editors and the reviewers. Any product that may be evaluated in this article, or claim that may be made by its manufacturer, is not guaranteed or endorsed by the publisher.
Supplementary material
The Supplementary Material for this article can be found online at: https://www.frontiersin.org/articles/10.3389/fmats.2023.1273628/full#supplementary-material
References
Adalati, R., Sharma, M., Sharma, S., Kumar, A., Malik, G., Boukherroub, R., et al. (2022). Metal nitrides as efficient electrode material for supercapacitors: A review. J. Energy Storage 56, 105912. doi:10.1016/J.EST.2022.105912
Allison, A., and Andreas, H. A. (2019). Minimizing the Nyquist-plot semi-circle of pseudocapacitive manganese oxides through modification of the oxide-substrate interface resistance. J. Power Sources 426, 93–96. doi:10.1016/J.JPOWSOUR.2019.04.029
Arrigo, R., Hävecker, M., Wrabetz, S., Blume, R., Lerch, M., McGregor, J., et al. (2010). Tuning the acid/base properties of nanocarbons by functionalization via amination. J. Am. Chem. Soc. 132, 9616–9630. doi:10.1021/ja910169v
Augustyn, V., Simon, P., and Dunn, B. (2014). Pseudocapacitive oxide materials for high-rate electrochemical energy storage. Energy Environ. Sci. 7, 1597–1614. doi:10.1039/c3ee44164d
Borenstein, A., Hanna, O., Attias, R., Luski, S., Brousse, T., and Aurbach, D. (2017). Carbon-based composite materials for supercapacitor electrodes: a review. J. Mat. Chem. A 5, 12653–12672. doi:10.1039/C7TA00863E
Cao, K., Jiao, L., Liu, H., Liu, Y., Wang, Y., Guo, Z., et al. (2015). 3D hierarchical porous α-Fe2O3 nanosheets for high-performance lithium-ion batteries. Adv. Energy Mater 5. doi:10.1002/AENM.201401421
Chen, D., Zhou, S., Quan, H., Zou, R., Gao, W., Luo, X., et al. (2018). Tetsubo-like α-Fe2O3/C nanoarrays on carbon cloth as negative electrode for high-performance asymmetric supercapacitors. Chem. Eng. J. 341, 102–111. doi:10.1016/J.CEJ.2018.02.021
Chen, M., Zhao, E., Yan, Q., Hu, Z., Xiao, X., and Chen, D. (2016). The effect of crystal face of Fe2O3 on the electrochemical performance for lithium-ion batteries. Sci. Rep. 6, 1–9. doi:10.1038/srep29381
Cherusseri, J., Sambath Kumar, K., Choudhary, N., Nagaiah, N., Jung, Y., Roy, T., et al. (2019). Novel mesoporous electrode materials for symmetric, asymmetric and hybrid supercapacitors. Nanotechnology 30, 202001. doi:10.1088/1361-6528/AB0685
Chi, H. Z., Wu, Y. Q., Shen, Y. K., Zhang, C., Xiong, Q., and Qin, H. (2018). Electrodepositing manganese oxide into a graphene hydrogel to fabricate an asymmetric supercapacitor. Electrochim Acta 289, 158–167. doi:10.1016/J.ELECTACTA.2018.09.025
Dong, Y., Wang, B., Zhao, K., Yu, Y., Wang, X., Mai, L., et al. (2017). Air-stable porous Fe2N encapsulated in carbon microboxes with high volumetric lithium storage capacity and a long cycle life. Nano Lett. 17, 5740–5746. doi:10.1021/ACS.NANOLETT.7B02698
Dubal, D. P., Ayyad, O., Ruiz, V., and Gómez-Romero, P. (2015). Hybrid energy storage: the merging of battery and supercapacitor chemistries. Chem. Soc. Rev. 44, 1777–1790. doi:10.1039/c4cs00266k
Eftekhari, A., and Mohamedi, M. (2017). Tailoring pseudocapacitive materials from a mechanistic perspective. Mater Today Energy 6, 211–229. doi:10.1016/j.mtener.2017.10.009
Feng, T., Liu, G., Li, G., Li, Y., Liang, J., and Wang, K. (2023). Engineering iron-rich nanomaterials for supercapacitors. Chem. Eng. J. 2023, 145045. doi:10.1016/J.CEJ.2023.145045
Gajewska, K., Moyseowicz, A., Minta, D., Zyna Gryglewicz, G., and Sci, J. M. (2023). Effect of electrolyte and carbon material on the electrochemical performance of high-voltage aqueous symmetric supercapacitors. J. Mater. Sci. 2023, 1. doi:10.1007/S10853-023-08148-5
Geng, B., Tao, B., Li, X., and Wei, W. (2012). Ni2+/surfactant-assisted route to porous α-Fe2O3 nanoarchitectures. Nanoscale 4, 1671. doi:10.1039/c2nr12102f
Gong, S. G., Shi, Y. H., Su, Y., Li, Y. F., Ding, L., Lin, J., et al. (2021). In situ growth of 3D lamellar Mn(OH)2on CuO-coated carbon cloth for flexible Asymmetric supercapacitors with a high working voltage of 2.4 v. ACS Sustain Chem. Eng. 9, 13385–13394. doi:10.1021/ACSSUSCHEMENG.1C05164
Idrees, M., Mukhtar, A., Ata-ur-Rehman, , Abbas, S. M., Zhang, Q., and Li, X. (2021). Transition metal nitride electrodes as future energy storage devices: A review. Mater Today Commun. 27, 102363. doi:10.1016/J.MTCOMM.2021.102363
Jiang, H., Huang, L., Wei, Y., Wang, B., Wu, H., Zhang, Y., et al. (2019). Bio-derived hierarchical multicore–shell Fe2N-Nanoparticle-Impregnated N-doped carbon nanofiber bundles: A host material for lithium-/potassium-ion storage. Nanomicro Lett. 11, 1–17. doi:10.1007/S40820-019-0290-0/FIGURES/7
Jiang, M., Li, Y., Lu, Z., Sun, X., and Duan, X. (2016). Binary nickel–iron nitride nanoarrays as bifunctional electrocatalysts for overall water splitting. Inorg. Chem. Front. 3, 630–634. doi:10.1039/C5QI00232J
Jin, W. H., Cao, G. T., and Sun, J. Y. (2008). Hybrid supercapacitor based on MnO2 and columned FeOOH using Li2SO4 electrolyte solution. J. Power Sources 175, 686–691. doi:10.1016/J.JPOWSOUR.2007.08.115
Kohila Rani, K., Karuppiah, C., Wang, S. F., Alaswad, S. O., Sireesha, P., Devasenathipathy, R., et al. (2020). Direct pyrolysis and ultrasound assisted preparation of N, S co-doped graphene/Fe3C nanocomposite as an efficient electrocatalyst for oxygen reduction and oxygen evolution reactions. Ultrason. Sonochem 66, 105111. doi:10.1016/J.ULTSONCH.2020.105111
Kordek, K., Jiang, L., Fan, K., Zhu, Z., Xu, L., Al-Mamun, M., et al. (2019). Two-step Activated carbon cloth with oxygen-rich functional groups as a high-performance additive-free Air electrode for flexible zinc–Air batteries. Adv. Energy Mater 9, 1802936. doi:10.1002/AENM.201802936
Kordek-Khalil, K., Walendzik, I., and Rutkowski, P. (2022). Low-overpotential full water splitting with metal-free self-supported electrodes obtained by amination of oxidised carbon cloth. Sustain. Energy Technol. Assessments 53, 102569. doi:10.1016/J.SETA.2022.102569
Li, R., Wang, Y., Zhou, C., Wang, C., Ba, X., Li, Y., et al. (2015). Carbon-stabilized high-capacity ferroferric oxide nanorod array for flexible solid-state Alkaline battery–supercapacitor hybrid device with high environmental suitability. Adv. Funct. Mater 25, 5384–5394. doi:10.1002/ADFM.201502265
Mei, B. A., Munteshari, O., Lau, J., Dunn, B., and Pilon, L. (2018). Physical interpretations of nyquist plots for EDLC electrodes and devices. J. Phys. Chem. C 122, 194–206. doi:10.1021/ACS.JPCC.7B10582
Moyseowicz, A., Gryglewicz, S., and Gryglewicz, G. (2021). Enhancing electrochemical capacitor performance through the application of nanostructured carbon materials as conducting additives. Chem. Eng. Process. - Process Intensif. 169. doi:10.1016/j.cep.2021.108647
Moyseowicz, A., and Moyseowicz, A. (2021). One-pot synthesis of bismuth sulfide nanostructures as an active electrode material for aqueous hybrid capacitors. Energies (Basel) 14, 2670. doi:10.3390/EN14092670/S1
Moyseowicz, A. (2019). Scalable one-pot synthesis of bismuth sulfide nanorods as an electrode active material for energy storage applications. J. Solid State Electrochem. 23, 1191–1199. doi:10.1007/s10008-019-04215-7
Moyseowicz, A., Śliwak, A., Miniach, E., and Gryglewicz, G. (2017). Polypyrrole/iron oxide/reduced graphene oxide ternary composite as a binderless electrode material with high cyclic stability for supercapacitors. Compos B Eng. 109, 23–29. doi:10.1016/j.compositesb.2016.10.036
Park, M. J., Lee, J. H., Hembram, K. P. S. S., Lee, K. R., Han, S. S., Yoon, C. W., et al. (2016). Oxygen reduction electrocatalysts based on coupled iron nitride nanoparticles with nitrogen-doped carbon. Catalysts 6, 86. doi:10.3390/CATAL6060086
Peng, H., Mo, Z., Liao, S., Liang, H., Yang, L., Luo, F., et al. (2013). High performance Fe- and N- doped carbon catalyst with graphene structure for oxygen reduction. Sci. Rep. 3, 1–7. doi:10.1038/srep01765
Simon, P., Gogotsi, Y., and Dunn, B. (2014). Where do batteries end and supercapacitors begin? Science 343, 1210–1211. doi:10.1126/SCIENCE.1249625
Śliwak, A., Moyseowicz, A., and Gryglewicz, G. (2017). Hydrothermal-assisted synthesis of an iron nitride-carbon composite as a novel electrode material for supercapacitors. J. Mater Chem. A Mater 5, 5680–5684. doi:10.1039/C6TA10985C
Theerthagiri, J., Lee, S. J., Murthy, A. P., Madhavan, J., and Choi, M. Y. (2020). Fundamental aspects and recent advances in transition metal nitrides as electrocatalysts for hydrogen evolution reaction: A review. Curr. Opin. Solid State Mater Sci. 24, 100805. doi:10.1016/J.COSSMS.2020.100805
Valvoda, V. (1996). About Some Practical Aspects Of X-Ray Diffraction: From Powder To Thin Film. LNF-IR--96-049.
Walendzik, I., Kordek-Khalil, K., and Rutkowski, P. (2023). Quick-to-synthesize hybrid electrodes composed of activated carbon cloth with Co/Fe-based films as bifunctional electrocatalysts for water splitting. Int. J. Hydrogen Energy 48, 25741–25754. doi:10.1016/J.IJHYDENE.2023.03.092
Wang, H., Li, J., Li, K., Lin, Y., Chen, J., Gao, L., et al. (2021). Transition metal nitrides for electrochemical energy applications. Chem. Soc. Rev. 50, 1354–1390. doi:10.1039/D0CS00415D
Wu, G., Johnston, C. M., MacK, N. H., Artyushkova, K., Ferrandon, M., Nelson, M., et al. (2011). Synthesis–structure–performance correlation for polyaniline–Me–C non-precious metal cathode catalysts for oxygen reduction in fuel cells. J. Mater Chem. 21, 11392–11405. doi:10.1039/C0JM03613G
Wu, L., Shi, D., Yan, S., Qiao, W., Zhong, W., and Du, Y. (2021). Iron-doped cobalt nitride nanoparticles (Fe–Co3N): an efficient electrocatalyst for water oxidation. Int. J. Hydrogen Energy 46, 2086–2094. doi:10.1016/J.IJHYDENE.2020.10.089
Wu, W., Hao, R., Liu, F., Su, X., and Hou, Y. (2013). Single-crystalline α-Fe2O3 nanostructures: controlled synthesis and high-index plane-enhanced photodegradation by visible light. J. Mater Chem. A Mater 1, 6888–6894. doi:10.1039/C3TA10886D
Xu, L., Sun, L., Feng, J., Qi, L., Muhammad, I., Maher, J., et al. (2017). Nanocasting synthesis of an iron nitride-ordered mesopore carbon composite as a novel electrode material for supercapacitors. RSC Adv. 7, 44619–44625. doi:10.1039/C7RA08704G
Yadlapalli, R. T., Alla, R. K. R., Kandipati, R., and Kotapati, A. (2022). Super capacitors for energy storage: progress, applications and challenges. J. Energy Storage 49, 104194. doi:10.1016/J.EST.2022.104194
Yan, Y., Tang, H., Wu, F., Wang, R., and Pan, M. (2017). One-step self-assembly synthesis α-Fe2O3 with carbon-coated nanoparticles for stabilized and enhanced supercapacitors electrode. Energies 10, 1296. doi:10.3390/EN10091296
Yu, Y., Xiao, D., Ma, J., Chen, C., Li, K., Ma, J., et al. (2018). The self-template synthesis of highly efficient hollow structure Fe/N/C electrocatalysts with Fe–N coordination for the oxygen reduction reaction. RSC Adv. 8, 24509–24516. doi:10.1039/C8RA03672A
Zhang, Z., Zhang, H., Chen, Y., Li, Z., Li, Y., Luo, T., et al. (2016). Ultrasmall Fe2O3 nanoparticles anchored on three-dimensional hierarchical porous graphene-like networks for high rate capability supercapacitors. ChemElectroChem 3, 1820–1826. doi:10.1002/CELC.201600393
Zhao, G., Ning, K., Wei, M., Zhang, L., Han, L., Zhu, G., et al. (2023). Fabrication and enhanced supercapacitive performance of Fe2N@Cotton-based porous carbon fibers as electrode material. Resour. Chem. Mater. 2, 277–287. doi:10.1016/J.RECM.2023.07.005
Zhi, M., Xiang, C., Li, J., Li, M., and Wu, N. (2013). Nanostructured carbon-metal oxide composite electrodes for supercapacitors: a review. Nanoscale 5, 72–88. doi:10.1039/c2nr32040a
Keywords: iron nitride, carbon cloth, electrochemical energy storage, supercapacitor, hydrothermal synthesis, electrodeposition
Citation: Kordek-Khalil K, Moyseowicz A and Moyseowicz AK (2023) Synthesis strategies of iron nitrides at carbon cloth as battery-like electrode for hybrid supercapacitors. Front. Mater. 10:1273628. doi: 10.3389/fmats.2023.1273628
Received: 06 August 2023; Accepted: 02 October 2023;
Published: 12 October 2023.
Edited by:
Noel Diez, Spanish National Research Council (CSIC), SpainReviewed by:
Manisha Das, CIC energigune, SpainRudra Kumar, Monterrey Institute of Technology and Higher Education (ITESM), Mexico
Copyright © 2023 Kordek-Khalil, Moyseowicz and Moyseowicz. This is an open-access article distributed under the terms of the Creative Commons Attribution License (CC BY). The use, distribution or reproduction in other forums is permitted, provided the original author(s) and the copyright owner(s) are credited and that the original publication in this journal is cited, in accordance with accepted academic practice. No use, distribution or reproduction is permitted which does not comply with these terms.
*Correspondence: Agata K. Moyseowicz, agata.moyseowicz@pwr.edu.pl
†These authors have contributed equally to this work