Iron-organic carbon coprecipitates reduce nitrification by restricting molybdenum in agricultural soils
- 1Department of Land, Air and Water Resources, University of California Davis, Davis, CA, United States
- 2AgroBioSciences Program, Mohammed VI Polytechnic University, Ben Guerir, Morocco
- 3Department of Soil Science, University of Wisconsin-Madison, Madison, WI, United States
- 4University of California Cooperative Extension, Woodland, CA, United States
Nitrification converts ammonium (NH4+) to nitrate (NO3−) using metalloenzymes, the activity of which depends on iron (Fe), molybdenum (Mo), and copper (Cu) availability. Iron-organic carbon coprecipitates (or Fe-OC flocs) are key byproducts of wastewater treatment industry and natural components of soil that may affect nitrification by changing the bioavailability of these metals. Here, we used flocs of different chemistry (aromatic and aliphatic) and known Fe and C composition to investigate their effects on nitrification in soils along a soil C gradient. Both aromatic and aliphatic flocs reduced net nitrification, but the magnitude of their effect was more pronounced in soils with low C content as opposed to those with high C content. Within each soil, both flocs reduced net nitrification similarly. In the presence of flocs, the bioavailability of Mo (assessed by changes in the concentration of water-soluble Mo) was dramatically decreased in low C soils, possibly because Mo was incorporated into or adsorbed to flocs or their decomposition products. In contrast, Mo bioavailability in high C soils was decreased to a lesser extent by flocs, likely because organic matter limited floc adsorption capacity and released Mo through mineralization. The depletion of bioavailable Mo by flocs in agricultural soils has the potential to impede soil nitrification and extend the residence time of NH4+ and its availability to plants and microbes.
1 Introduction
Excess nitrification, an important pathway of nitrogen (N) transformation in soils, can negatively affect ecosystem health, by increasing atmospheric nitrous oxide (N2O) levels and NO3− leaching to water bodies (Nevison et al., 2016; Ayiti and Babalola, 2022). Thus, decreasing nitrification in agricultural soils is of interest (Norton and Ouyang, 2019). Organo-Fe complexes, commonly termed flocs, may impede nitrification. These complexes contribute up to 38% of total organic carbon (OC) in some soils (Zhao et al., 2016) and result from the coprecipitation of dissolved organic matter (DOM) with minerals; an ubiquitous process in soils experiencing significant changes in pH or redox conditions. Flocs are also key byproducts of the water treatment industry, which uses Fe-based salts to coprecipitate DOM and remove it prior to disinfection (Dempsey et al., 1984; Siéliéchi et al., 2008). While industry generated flocs are generally disposed of in landfills, they have drawn much focus due to their potential for reversing land subsidence and sequestering C in constructed wetlands (Henneberry et al., 2012).
There have been several studies demonstrating the inhibitory effects of Fe on soil nitrification (Butler and Gordon, 1986), However, the effects of flocs are not well characterized. Iron-OC, the coprecipitation process is critical to stabilizing C against microbial decay and determining Fe reactivity, which may limit its participation in nitrification. Nevertheless, the stability of flocs, defined here as resistance to both microbial and chemical degradation, can be influenced by OC chemical composition as well as soil conditions and properties. First, the chemical composition of OC influences the binding strength with mineral surfaces (Newcomb et al., 2017). High-molecular-weight OC containing abundant aromatic functional groups is preferentially adsorbed by either strong or irreversible processes (Lehmann et al., 1987; McBride, 1987) leading to highly stable flocs. In contrast, aliphatic compounds such as carbohydrates are biodegradable and yield less stable flocs. Second, changes in soil pH can compromise the stability of flocs such as by inducing the dissolution of Fe and the subsequent release of OC. Third, soil C and clays present in soil can stabilize flocs against microbial decay (You et al., 2022). Therefore, OC chemistry as well as soil properties and conditions are expected to determine floc stability and reactivity; the more stable a floc is, the less influence it is expected to have towards nitrification.
Beyond controlling Fe and OC availability, flocs may impede soil nitrification by controlling the availability of metals and nutrients that are required for microbial growth and metabolism. It is well known that soil nitrification is regulated by different metalloenzymes, each requiring specific cofactors (Lancaster et al., 2018). These cofactors play a crucial role in catalyzing key steps of the nitrification process. In fact, Cu, Fe and Mo are necessary for the hydrolysis of ammonia (NH3) to NH2OH, the oxidation of NH2OH to NO2− or N2O, and of NO2− to NO3−, respectively (Ensign et al., 1993; Chicano et al., 2021). Because flocs have presumably higher stability in soil environments, few studies have considered their effects on metals bioavailability. However, interactions of metals with OM and the highly sorptive Fe minerals through sorption processes can control their bioavailability. Prior work found that Fe-OM coprecipitates increase the retention of Cu in soils through the incorporation of Cu into the floc structure during floc formation or by the surface attachment (i.e., adsorption) of Cu onto previously formed floc (Seda, 2014). Moreover, depending on floc stability level and the nature of floc C, floc decomposition may yield compounds with metal chelating properties in soil. For instance, glucose oxidation may yield gluconic acid which can effectively chelate cations in soils (Duff et al., 1963).
In addition to Fe, Mo and Cu, other metals and nutrients may also influence nitrification, such as calcium (Ca), phosphorus (P), magnesium (Mg), nickel (Ni), cobalt (Co), manganese (Mn), zinc (Zn), aluminum (Al), and potassium (K) (Meiklejohn, 1952; Van Droogenbroeck and Laudelout, 1967; Loveless and Painter, 1968; Cela and Sumner, 2002; Çeçen et al., 2010; Bhunia, 2014; Raglin et al., 2022). Although there is limited available information regarding the interactions between flocs and these elements, it is noteworthy to mention that many of these elements can be influenced by the presence of chelating agents (Schwertmann et al., 1986; Fischer and Bipp, 2002). Other noteworthy information from the literature includes the fact that P availability is influenced by the effects of OM on P adsorption to Fe oxide (Barrón et al., 1988; Mikutta et al., 2006).
The purpose of this study is to explore the effects of Fe-OC flocs on nitrification in soils with different properties, such as C, N, and clay content. To do so, we created model Fe-OC flocs of known Fe and C composition (aromatic and aliphatic C) to understand how flocs affect nitrification in soils. Our hypotheses are:
(a) Fe-OC flocs reduce nitrification. Aliphatic floc reduce nitrification more than aromatic floc because it is less stable and more reactive.
(b) The effect of Fe-OC flocs on nitrification are less pronounced in soil with high C content relative to those with low C content.
(c) Fe-OC flocs or their decomposition products decrease the bioavailability of metal cofactors Mo, Fe and Cu and other nutrients for nitrification.
2 Materials and methods
2.1 Preparation of Fe-OC flocs
All chemicals used were high purity. Tannic acid (TA) and carboxymethyl cellulose (CMC) were used to prepare the aromatic and aliphatic Fe-OC flocs, respectively. To prepare the aromatic floc, 2% (w/v) TA solution and 2% (w/v) Fe (III) sulfate (Fe2(SO4)3) solution were prepared using deionized water (DI). In an Erlenmeyer flask, 18 mL Fe2(SO4)3 solution was added to 10 mL TA solution under vigorous stirring, and the pH was adjusted to 7 with 1 M NaOH. The resulting black solution was centrifuged at 10,000 rpm for 15 min, after which the supernatant was discarded, and the precipitate (floc) was washed 3 times with DI water to remove excess Fe. The floc was oven-dried overnight at 30°C and ground using a pestle and mortar to obtain a fine powder. Powdered flocs were stored at room temperature in a closed container. To prepare the aliphatic floc, 3 mL of 2% Fe2(SO4)3 solution was added to 10 mL of a 2% (v/v) CMC solution. The resulting solution was shaken manually and centrifuged at 10,000 rpm for 15 min, after which the supernatant was discarded, and the floc was washed 3 times with DI water. The floc was freeze-dried and ground to a fine powder before storing at room temperature in a closed container.
2.2 Characterization of Fe-C flocs composition
Total C and N analyses were performed on 3 samples of powdered Fe-OC flocs using an elemental analyzer (EAS 4010, Costech Analytical Technologies Inc., Valencia, CA). Flocs Fe content was determined by digesting 30 mg of floc in 1 mL nitric acid HNO3 (69%, 16 M) followed by determination of dissolved Fe with a ferrozine-based colorimetric assay. The results of these analyses are presented in Table 1.
2.3 Characterization of Fe-OC flocs stability
To characterize Fe-OC floc stability, we combined thermal stability analyses, which serves as a proxy of binding strength between the mineral and the organic fractions, with stability assessments in incubation experiments, measured by the amount of Fe and OC released from flocs. Thermal stability was measured by thermal gravimetric analysis (TGA) using an SDT Q600 V20.9 Build 20 and was performed under N2 purge (20 mL/min) from 25 to 15,00°C with temperature increments of 20°C/min.
2.4 Soil preparation
Soils along a C gradient (1% C, 3% C, 11% C and 16% C) were collected from the top 0–15 cm of Twitchell Island (latitude: 38.11, longitude: −121.64), located on the western portion of the San Francisco Bay Delta (California, United States). An additional 1% C soil was collected from Russell Ranch (latitude: 38.24, longitude: −121.29), at the University of California at Davis. Most soil from Twitchell Island is a Rindge mucky silt loam (Euic, thermic Typic Haplosaprist), formed from Tule and reed deposition. The Twitchell Island 1% C soil has a sandy texture, whereas Russell Ranch soil is a clay (1% C-CL). For each soil, five samples were randomly collected, composited, and sieved (2 mm). Visible plant residues and roots were removed, and soils were air-dried. Gravimetric water content (GWC) and Water holding Capacity (WHC) of soils were determined. The percentages of clay, silt and sand were determined by hydrometer method (Gee and Or, 2002), with a pre-treatment with H2O2 to remove OM. pH was determined in 1:5 soil: water slurries. Selected soil properties and initial metal concentrations are presented in Table 2 and Table 3, respectively.
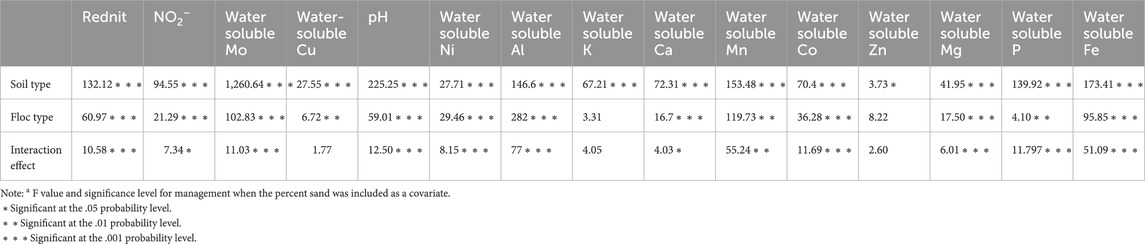
Table 3. F values and Tukey-adjusted p values for the main effects of soil type, floc type and their interaction on reduction in net nitrification (Rednit), pH, NO2− concentration, and water-soluble Mo, Cu, Al, Ca, K, Mn, Co, Ni, Zn, Mg, and P concentrations.
2.5 Soil incubations
2.5.1 Main incubation
Flocs were applied to 6 g air-dried soil at a rate providing 2 mg C g-1 soil. Flocs were thoroughly mixed with soils and incubated at 65% of WHC at 30°C for 30 days. Control samples were prepared by incubating soil without flocs. Each treatment was replicated four times. Soil-floc mixtures were placed in specimen cups, which were then placed in a mason jar containing 2 mL of DI water to maintain moisture. The jars were closed with a foam lid to allow gas exchange. NH4+, NO3−, total dissolved Fe, dissolved organic C (DOC) were analyzed on days 0, 3, 5, 7, 10, 17 and 30 of the incubation. Briefly 30 mL of 0.5 M potassium sulfate (K2SO4) were added to soil-floc mixture (Mulvaney, 1996). Samples were shaken for 1 h on a reciprocal shaker and the suspension filtered (Fisherbrand, Q5) for the analysis of NH4+, NO3+, dissolved organic C (DOC) and total dissolved Fe. NO3− was analyzed colorimetrically using a single reagent method (Doane and Horwáth, 2003). The NH4+ concentration was determined using the salicylate method (Verdouw et al., 1978; Foster, 1995). DOC was analyzed on a UVPersulfate Total Organic C Analyzer (model Phoenix 8,000, Tekmar Dohrmann, Cincinnati, Ohio). Total dissolved Fe was analyzed using the ferrozine method. Net N mineralization and nitrification (mg N kg−1 dry soil) in each treatment was calculated as the difference between the final and initial NH4+ and NO3−-N contents, respectively. Reduction in net nitrification (Rednit) was calculated as the percentage difference between NO3−-N contents in treatments and controls (equation 1). We also calculated the net DOC and Fe release from floc as percentage of floc C and Fe, respectively (equation 2 and 3).
We also measured NO2− concentrations on the same extracts as NH4+, NO3+, but only in 1% C-Sand, 1% C-CL and 11% C soils. This was done calorimetrically using the Griess reagent (Zimmermann (1979). Hot water extraction of Mo and Cu and other metals and nutrients (Al, Ca, K, Mn, Co, Ni, Zn, Mg, and P) was performed on samples incubated for 30 days at 30°C: 20 mL of DI were added to samples and placed in a hot water bath for 1 h with occasional shaking. Then, samples were shaken for 1 h, centrifuged (10.000 g for 10 min) and passed through 0.45-μm syringe filter to remove particulate material. Dilution with 3% nitric acid (HNO3) followed (the dilution factor was 1.03), after which samples were analyzed by Inductively Coupled Plasma Atomic Emission Spectroscopy (ICP-AES) at the Interdisciplinary Center for Plasma Mass Spectrometry laboratory at the University of California at Davis.
2.5.2 Additional incubation
To confirm our hypothesis 3, we performed a laboratory incubation to test the effects of adding a metal cofactor on Rednit. Flocs were applied to 4 g of 1% C-Sand receiving 10, 50 and 100 mg kg-1 Mo as sodium molybdate (Na2MoO4). Control samples were prepared by incubating soil without flocs, and without the addition of Mo. We measured net nitrification (mg N kg−1 dry soil) as the difference between the final and initial NO3−-N contents in each treatment.
2.6 Statistical analyses
Experiments were evaluated using R statistical software (version 3.6.2). We performed analysis of variance (ANOVA) and Partial Least Square Regression (PLSR) on the data from day 30 of the incubation. PLSR integrates principal component analysis (PCA) and multiple linear regression to maximize covariance between the predictor and response variables. This method is particularly effective in situations where (1) strong collinearity occurs between predictor variables (Supplementary Figure S1) and (2) a relatively small dataset size could otherwise restrict model performance. Notably, many of the variables used in this study exhibit pronounced multi-collinearity (Supplementary Figure S1). We also performed regression analysis using Excel to highlight important relationships in our dataset.
3 Results
3.1 Assessment of Fe-OC flocs stability by thermal analyses and in incubation experiment
Our results for thermal stability analysis showed that T50 of the aromatic floc, which is the temperature at which half of the OC is removed, is greater than that of the aliphatic floc (Figure 1). Thus, to remove the same fraction of C mass, the aromatic floc required higher temperature (more than 1000°C) than the aliphatic floc (380°C), which indicates that the former is more thermally stable. These results are in general agreement with stability assessments of Fe-OC floc in the main incubation experiment. In fact, we found that both Fe and OC increased in soils treated with the aliphatic floc (Figure 2), but not with the aromatic floc, indicating a possible decomposition of the aliphatic floc. Our results also show that net total Fe and net total DOC released were positively correlated (r2 = 0.80, p < 0.001) across all soils and floc treatments (Figure 3). However, this relationship was not consistent across individual soils (Supplementary Table S1).
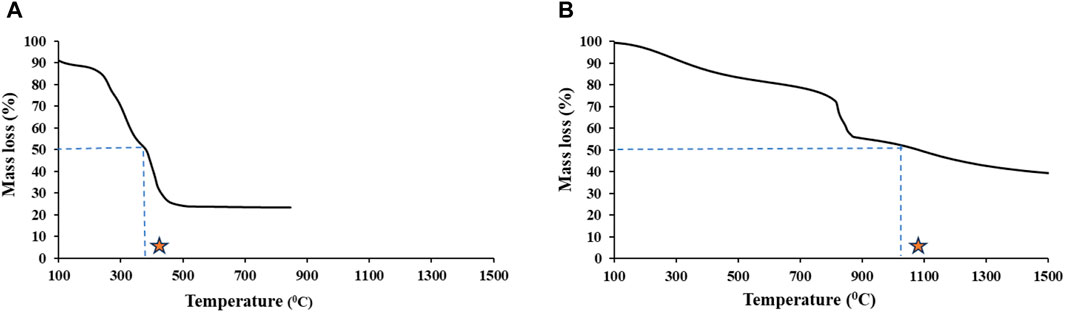
Figure 1. Thermal stability of (A) the aliphatic and (B) the aromatic flocs. Star symbol highlights T50.
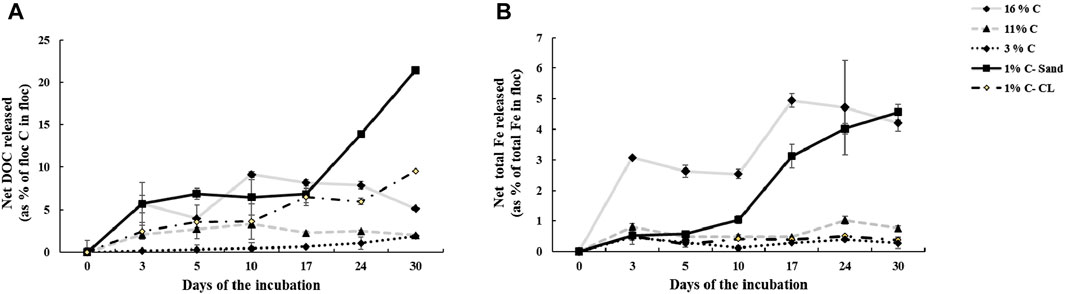
Figure 2. (A) Net DOC and (B) net total Fe released from the aliphatic floc in soils in the main incubation experiment. The aromatic floc cannot be represented by the same graphs because the calculations result in “zeros.”
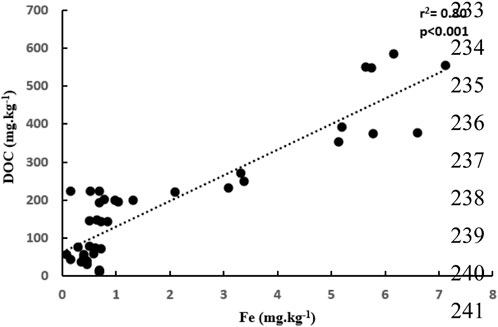
Figure 3. Relationship between net DOC and net total Fe released across all soils and floc treatments.
3.2 Flocs reduced net nitrification more in soils with low C than in soils with high C content
We measured net nitrification and calculated Rednit in soils amended with both floc types (Figure 4). Both flocs significantly reduced net nitrification in all soils (Table 3). The interaction between soil and floc type was also significant, suggesting that the ability of floc to affect nitrification depends on soil type. As such, the magnitude of floc effects on nitrification was more pronounced in with low C content (≤3% C) than in soils with high C content (>3% C). For instance, Rednit was 50% in 1% C-Sand compared to 20% in 16% C soil with both flocs. Interestingly, within the same soil, both flocs reduced net nitrification similarly (p = 0.33, F = 0.97). As assessed by the F value, the main effect of floc type on Rednit was larger than that of soil type and interaction effect.
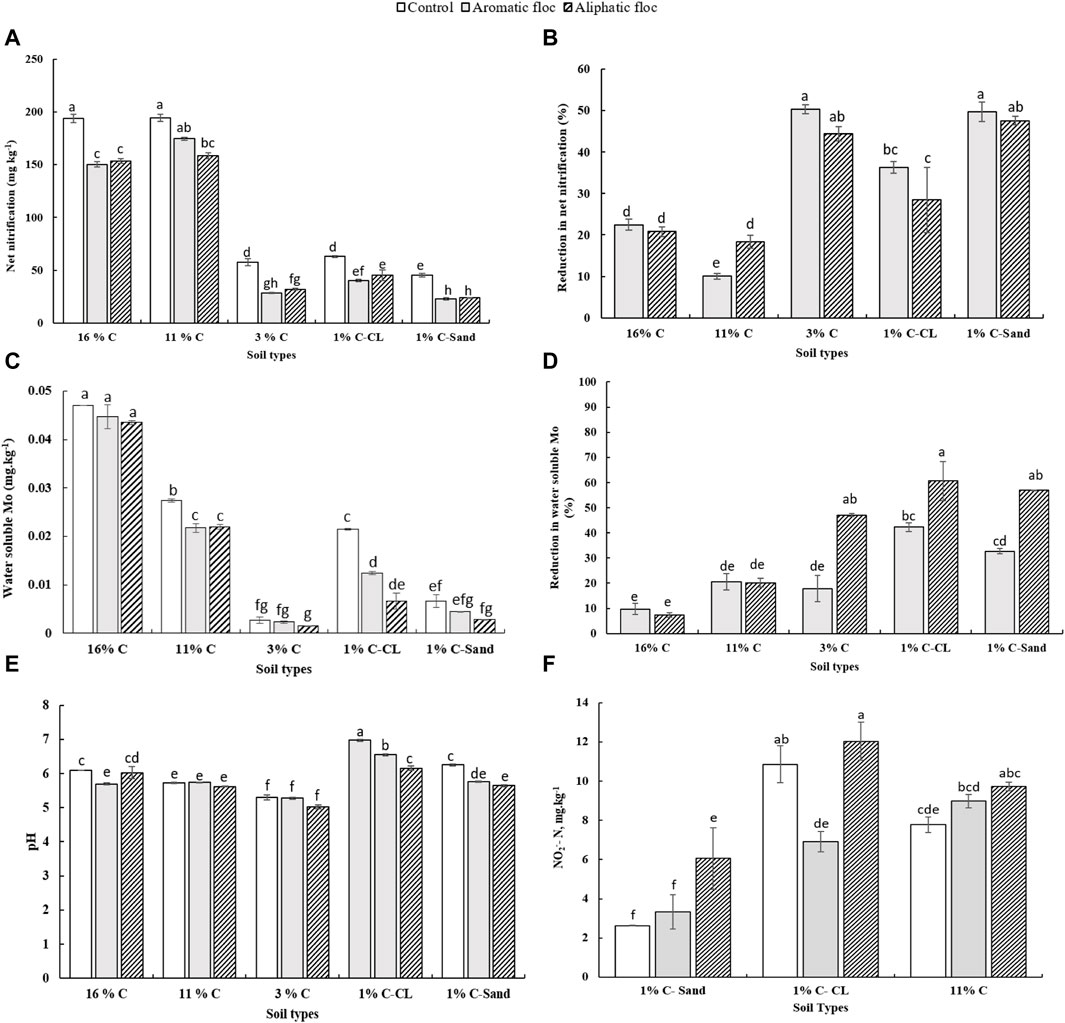
Figure 4. (A) Net nitrification, (B) reduction in net nitrification, (C) water soluble Mo, (D) reduction in water-soluble Mo, (E) pH and (F) NO2- concentrations in soils treated with flocs at day 30 of the incubation.
3.3 Rednit was accompanied by significant alterations in pH, NO2− concentrations, nutrients and metals
Rednit was also accompanied by significant alterations in pH, NO2− and water-soluble Mo, Cu, Al, Ca, K, Mn, Co, Ni, Zn, Mg, and P concentrations in flocs amended with soils (Figure 4, Supplementary Figure S2). A two-way ANOVA analysis showed that soil type, floc type and their interaction was significant for most measured parameters (Table 3). In fact, changes in element concentrations were more pronounced in soils with low C compared to those with high C, and in the aliphatic than in the aromatic floc treatments, with few exceptions. However, the main effect of soil type on element concentrations change was larger than that of floc and interaction effects (Table 3), indicating the strong influence of the soil type on pH, NO2− and water-soluble elements. Indeed, low C soils were more responsive to flocs input than high C soils. Other noteworthy information is that while pH and water-soluble Mo decreased, NO2− concentrations and water-soluble elements increased in low C soils. For instance, in the 1% C-CL soil, water-soluble Mo was reduced by 42.2% and 62.6%, whereas water-soluble Cu was increased by 40.3% and 90.2%, respectively, in the aromatic and aliphatic floc treatments. As for NO2− concentrations, they increased significantly in 1% C-Sand and 1% C-Clay (except in the aromatic floc treatment). Although a similar trend was observed in 11% C soil amended with flocs, the accumulation of NO2− was not significant.
3.4 Possible mechanistic drivers of Rednit in flocs-amended soils
We conducted a PLSR analysis to describe Rednit in soils amended with flocs by covarying its possible mechanistic drivers (Figure 5). Our findings demonstrate that incorporating Mo in a predictive model more accurately represented Rednit than the same predictive model with Mo omitted. When Mo was omitted, the proportion of Rednit variance explained by the model dropped from 26.16% to 15.71% on the second component of PLSR (Figure 5). The effects of incorporating or omitting the other factors (Ni, Co, etc.) in the predictive model were not as pronounced as with Mo (Supplementary Figure S3). Regression analysis between Rednit and water-soluble Mo also showed that the latter explained 53% of variability in Rednit across all soils and floc treatments (p = 0.01, Figure 6F).
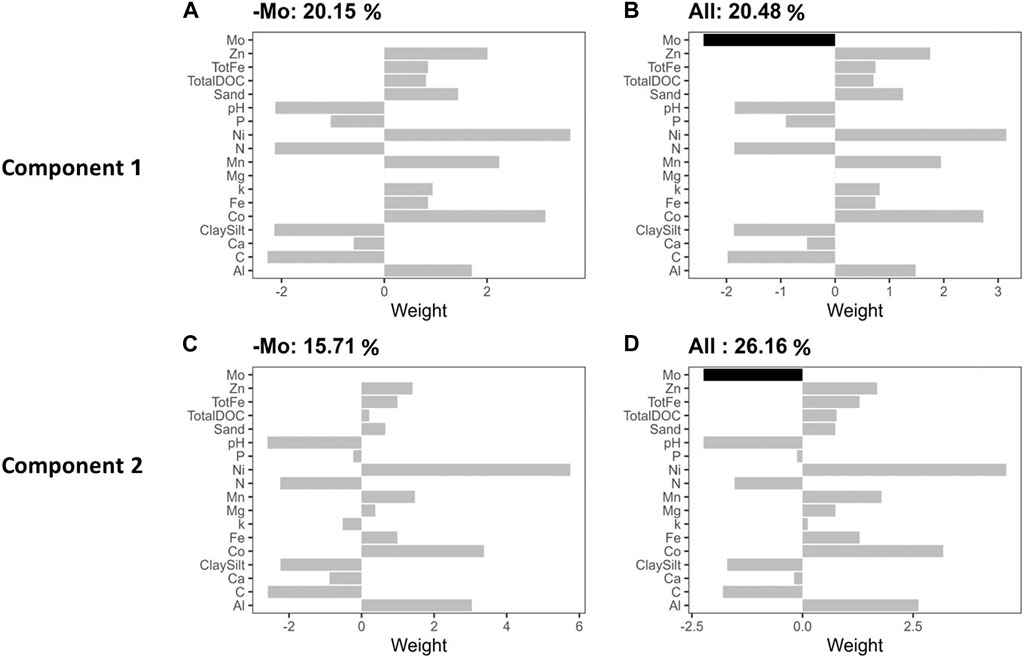
Figure 5. Component 1 (top row) and component 2 partial least squares regression (PLSR) x weights (buttom row) of the mechanistic drivers of Rednit. The titles represent the proportion of reduction in net nitrification (Rednit) variance explained by the model without (B,D) and with (A,C) Mo omitted.
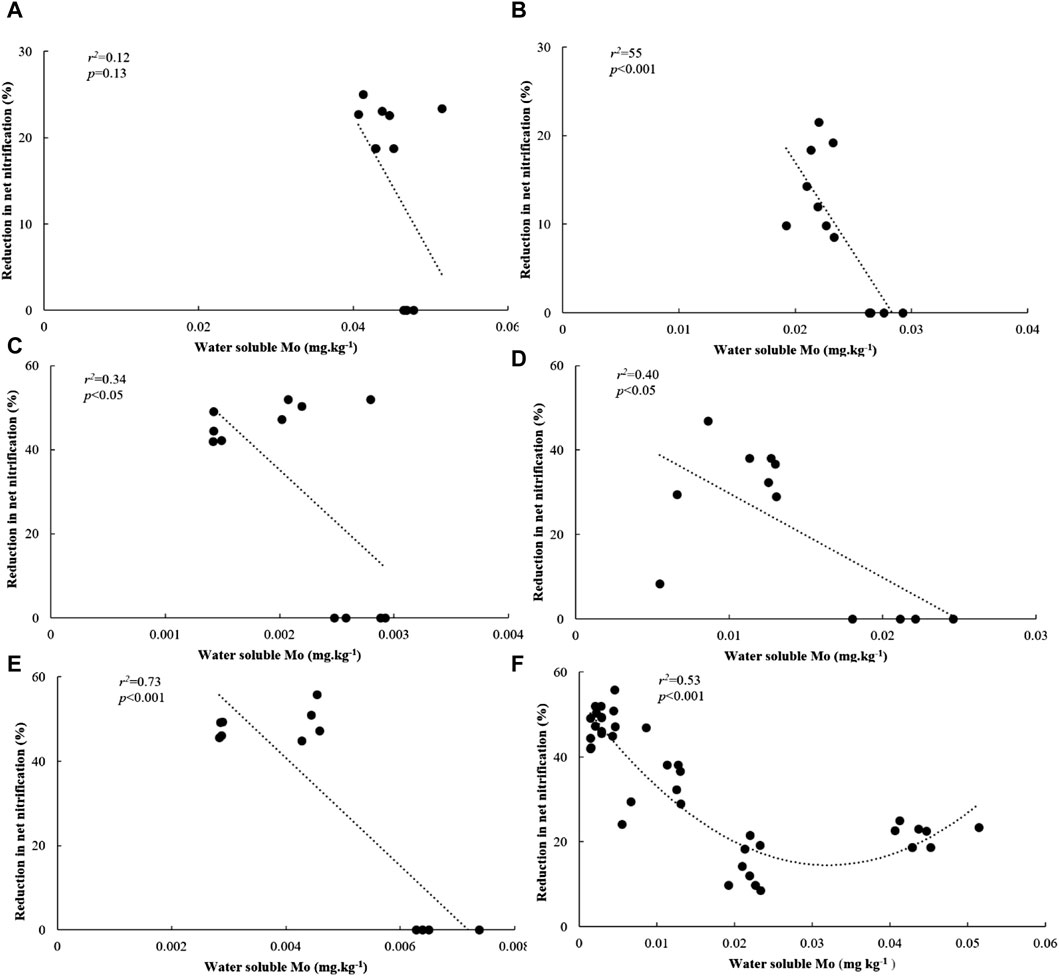
Figure 6. Relationship between water soluble Mo and reduction in net nitrification in (A) 16% C soil, (B) 11% C soil, (C) 3% C soil, (D) 1% C-CL soil, (E) 1%C-Sand soil and (F) across all soils amended with flocs.
3.5 Relationships between water soluble Mo and soil properties
We investigated relationships between water soluble Mo and its underlying drivers in soils amended with flocs. Our analysis revealed significant and positive correlations between water soluble Mo, soil C and N, and clay + silt content across all soils (Supplementary Figure S4). Additionally, across all low C soils, water-soluble Mo showed strong correlations with DOC (Figure 7F). However, these correlations were not observed in high C soils (Figure 7F). Moreover, water-soluble Mo exhibited a positive and significant correlation with pH across all soils with less than 3% C, but not in soils with higher C content (Figure 8F). When considering individual low C soils, we found strong positive correlations between water-soluble Mo and pH (Figures 8A E), while negative correlations were observed between Mo and DOC (Figures 7A E). These correlations, however, were not present in individual high C soils. Notably, there was no correlation between water-soluble Mo and total dissolved Fe in soils with C content of 11%, 16% and 3%, but a positive and significant correlation was evident in the case of 1% C-Sand soil and 1% C-Clay (Supplementary Table S2).
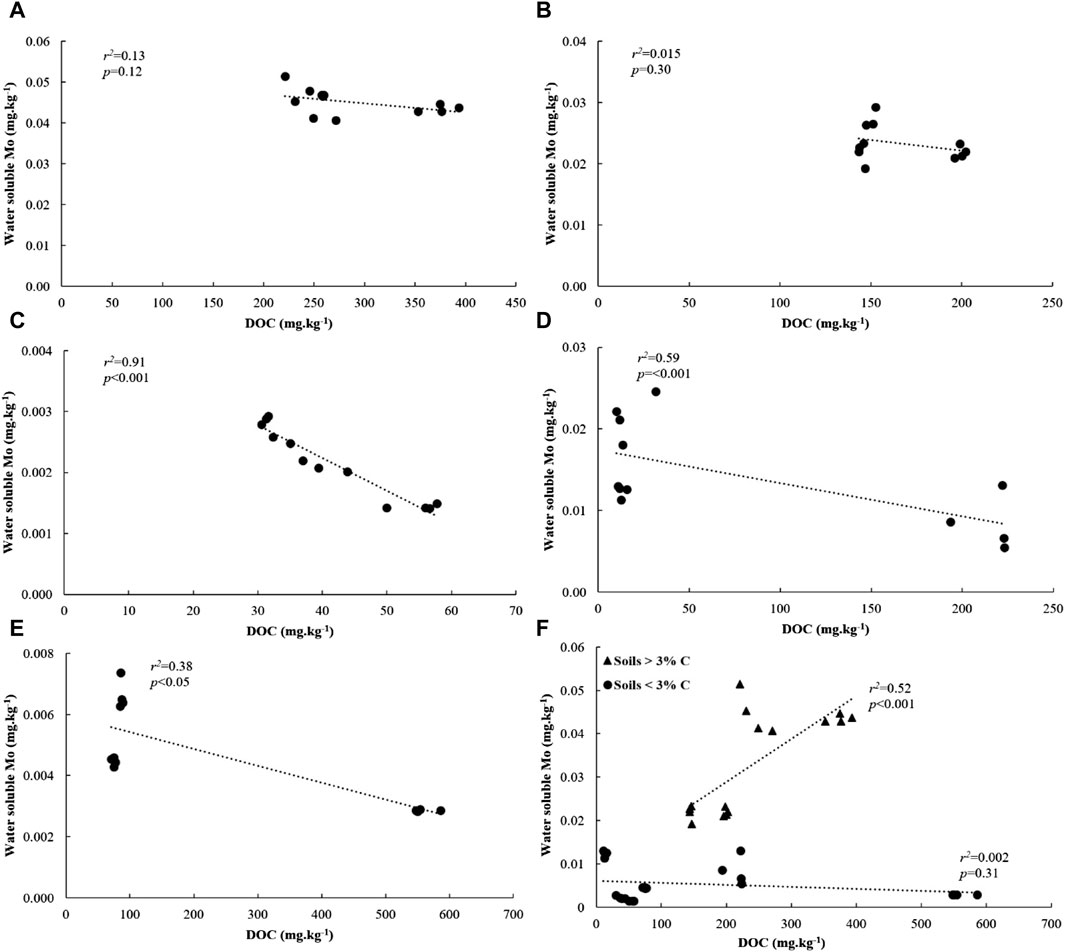
Figure 7. Relationship between water-soluble Mo and DOC in (A) 16% C soil, (B) 11% C soil, (C) 3% C soil, (D) 1% C-CL soil, (E) 1%C-Sand soil and (F) based on soil type after 30 days of incubation.
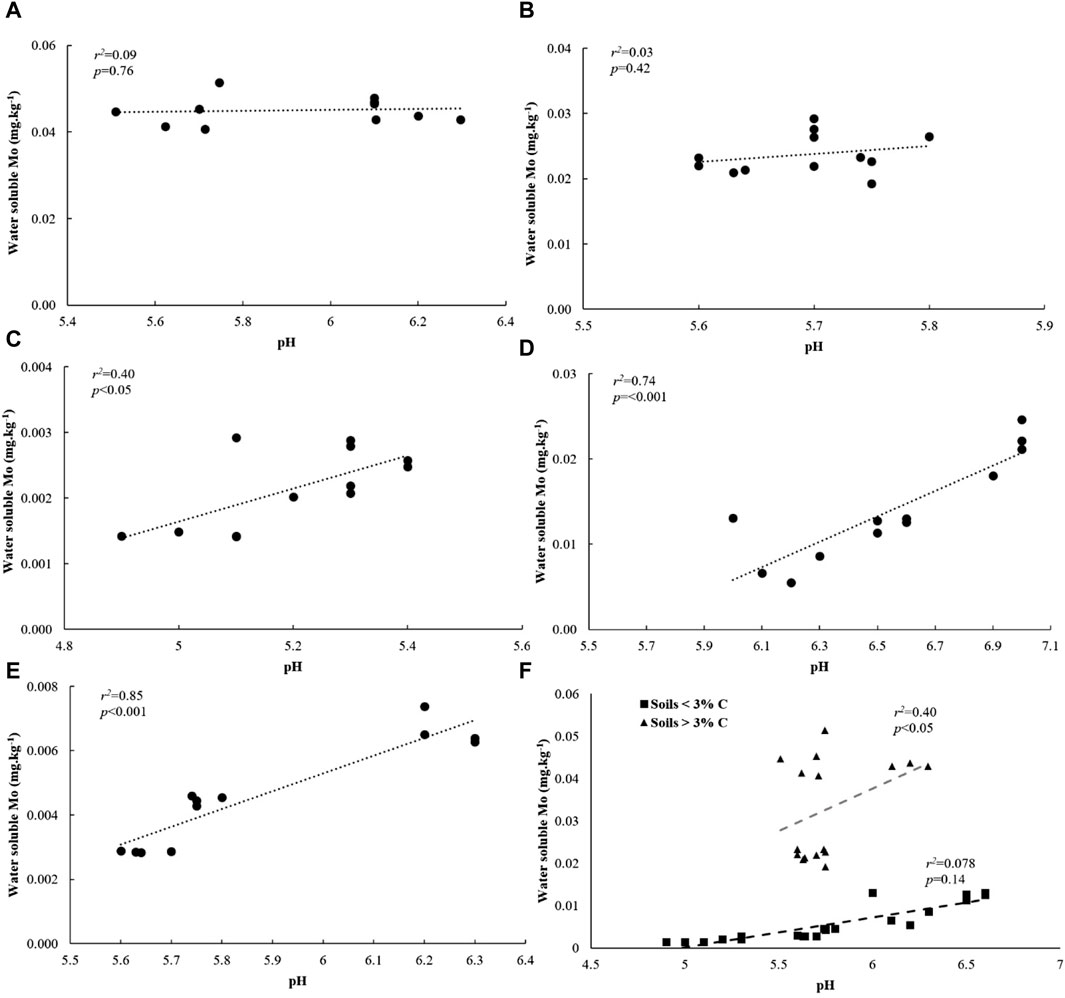
Figure 8. Relationship between water soluble Mo and pH in (A) 16% C soil, (B) 11% C soil, (C) 3% C soil, (D) 1% C-CL soil, (E) 1%C-Sand soil and (F) based on soil type after 30 days of incubation.
3.6 Effects of adding Mo on Rednit
Supplemental Mo additions did not increase net nitrification compared to the control treatments (Figure 9A). There was also no discernible correlation between water-soluble Mo concentrations and NO3− concentrations across all Mo addition treatments (r2 = 0.017, p = 0.27). Water-soluble Mo concentration increased with Mo addition only after the addition of 50 mg kg-1 (Figure 9B).
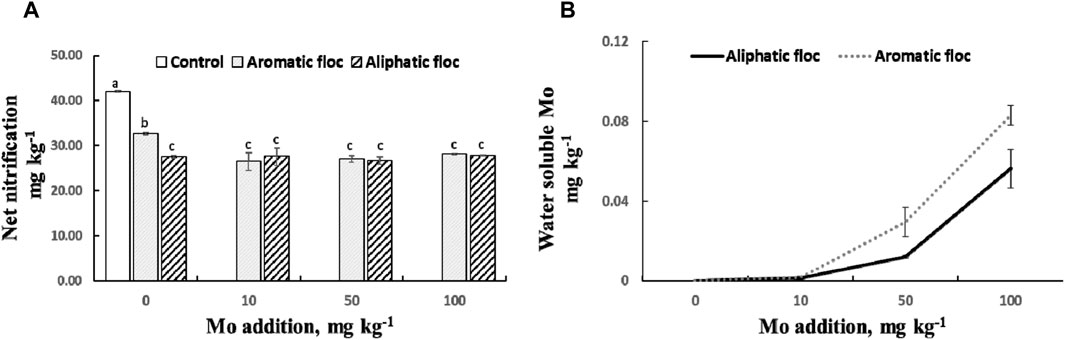
Figure 9. (A) Net nitrification and (B) water soluble Mo in flocs amended soils subjected to increased Mo addition at day 30 of the incubation. Values are average of 4 repetitions per treatment. Bars represent standart errors.
4 Discussion
4.1 Stability of Fe-C flocs is not equated with reactivity
The paradigm that stable flocs are not reactive, or have low reactivity originates from studies indicating that sorption processes protect OM against microbial degradation (Wagai and Mayer, 2007) and modify the structural properties of Fe oxides, making them less reactive towards metals and nutrients (Henneberry et al., 2012). However, our findings challenge this paradigm and do not support the hypothesis that stable aromatic floc induce lower Rednit compared to non-stable aliphatic floc. Instead, we discovered that regardless of their stability, flocs similarly reduced net nitrification within each soil. This outcome was unexpected for the following reasons:
❖ Differences in floc C chemistry: the dissimilarities in floc C chemistry between aromatic and aliphatic flocs are anticipated to impact their stability. Previous research has documented the preferential sorption of aromatic compounds (e.g., TA) over aliphatic compounds (e.g., CMC) onto minerals. Both compounds possess functional groups, such as phenolic groups (-OH) for TA and carboxymethyl groups (-CH2COOH) for CMC, which enable strong chelation with Fe(III). However, thermal analysis has revealed that the bonds between CMC and Fe(III) are weaker than those formed between TA and Fe(III).
❖ Floc stability assessment: evaluating floc stability in soil by measuring the release of Fe and DOC from the floc structure revealed that Fe and DOC were released in all soils amended with aliphatic flocs but not with aromatic flocs. Floc decomposition likely resulted from Fe solubilization, a process primarily regulated by soil pH, mineral dissolution-precipitation dynamics, and chelation (Colombo et al., 2014).
Taken together, we propose that the aliphatic floc is more likely to release Fe and DOC into the soil environment, which are more likely to engage in chemical reactions compared to Fe and OC sequestered in the aromatic floc structure. Accordingly, one would expect higher Rednit in the non-stable aliphatic floc treatment. However, our observations contradict this expectation, as we did not observe any significant differences in Rednit between the stable and non-stable floc treatments.
4.2 Rednit is related to water soluble Mo
Extensive research has been conducted to investigate the factors that drive nitrification in soils, with OM, pH, and ammonium (NH4+) often taking the spotlight (Li et al., 2020). Notably, the availability of N substrates plays a pivotal role in stimulating the activity of nitrifying microorganisms and their enzymes, as well as promoting N mineralization, thereby enhancing soil nitrification (Li et al., 2020). However, Sahrawat (1982) found that in a set of eight tropical soils, nitrification was not strongly correlated with OC and total N content, but rather with soil pH. While direct comparisons between the factors controlling Rednit in our study and nitrification in the aforementioned studies cannot be made, due to the lack of normalization to a control soil in the latter, we found that, compared to soils with less than 3% C, net nitrification was higher in soils characterized by high C and N content. This trend is similar to the one observed by Li et al. (2020), but not consistent with Sahrawat’s findings (1982). Although NH4+ concentrations decreased in all soils amended with floc, this decrease was not statistically significant within each soil type (Supplementary Table S3). This suggests that nitrification was not limited by substrate availability and that other factors may have influenced the observed patterns.
Notably, our study sheds light on an often-overlooked factor: Mo, whose bioavailability appears to have influenced nitrification in flocs amended soils. Although many other nutrients and metals concentrations were affected in flocs amended soils, PLSR analysis revealed that Mo emerged as the most influential factor impacting Rednit, justifying our focused attention on Mo in this study. While Mo’s role in N2 fixation and NO3− reduction is well-known, its potential involvement in nitrification has received limited attention in soil research. However, Mo plays a critical role in nitrification through various mechanisms. First, Mo is an essential micronutrient for nitrifying microorganisms (Zavarzin, 1957; Finstein and Delwiche, 1965; Tandon and Mishra, 1968). In fact, studies using growth media have demonstrated that the synthesis of a single Nitrobacter cell requires a minimum of 2000 atoms of Mo, with no other metal able to substitute for Mo in the media. Furthermore, Mo stimulates the growth of Nitrobacter cells and enhances their utilization of nitrite (NO2−), albeit at inhibitory levels when present in excess (Finstein and Delwiche, 1965). Secondly, Mo is critical for the enzymatic oxidation of NO2− to NO3− carried out by the NXR enzyme (Zavarzin, 1958). A deficiency in Mo halts this crucial process (Yanase et al., 2000). The NXR enzyme contains a Mo center (Figure 10), along with several Fe-S clusters, which acts as a binding site for the substrate (NO2−). This center facilitates the transfer of two electrons from NO2− to the Mo (VI) ion, leading to the oxidation of NO2− to NO3− (Meincke et al., 1992). Considering these observations and acknowledging that other mechanisms may have contributed to reduced nitrification in floc-amended soils, we propose Mo availability may have been influenced by floc and soil properties, subsequently affecting nitrification.
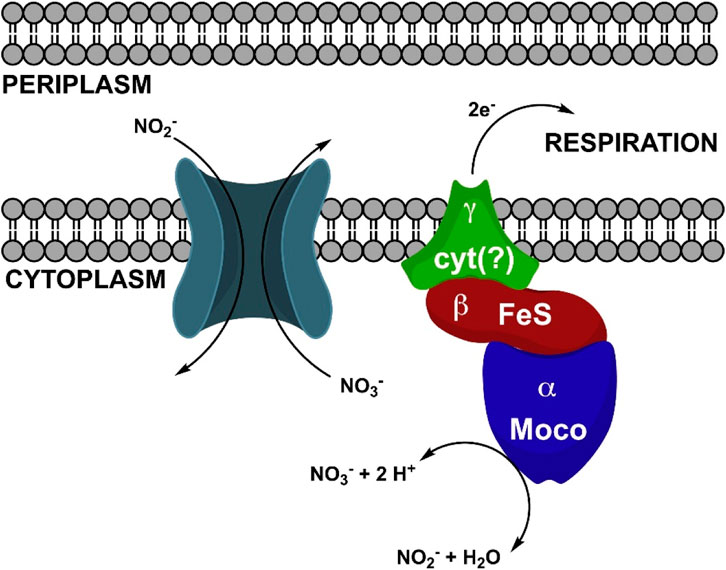
Figure 10. Schematic illustrating NO2− oxidation by Nitrobacter hamburgensis NO2− oxidoreductase (NXR) (Lancaster et al., 2018). Reproduced with permission.
4.3 Mo bioavailability is limited in floc amended soils
Mo bioavailability is controlled by sorption and desorption dynamics in soil. In our experiment, soil C content, DOC, pH, and Fe were related to Mo bioavailability:
4.3.1 Relationship between water soluble Mo, DOC, and total soil C
In agreement with our results, previous studies have found a positive relationship between soil C content and the availability of Mo (Lombin, 1985; Marks et al., 2015; Rutkowska et al., 2017), indicating that water-soluble Mo originates from SOM mineralization and that high levels of water-soluble Mo are generally associated with a high content of OM. This is in accordance with the observation that high C soils had more water-soluble Mo than those with low C content at day 30 of the incubation experiment. In fact, SOM serves as a reservoir for Mo and mediates its retention, storage, and bioavailability (Karimian and Cox, 1978; Wichard et al., 2009; Marks et al., 2015). It was also found that Mo adsorption prevents Mo leaching and promotes its acquisition by N2-fixing microorganisms in the topsoil (Wichard et al., 2009). These microorganisms employ small complexing agents called metallophores that capture Mo from SOM-Mo complexes prior to incorporation into the nitrogenase enzyme. Additionally, OM can enhance Mo bioavailability by inducing the aggregation of Fe oxides, reducing Mo penetration into their micropores (Lang and Kaupenjohann, 2003). This mechanism was proposed following the observation that higher MoO42− desorption occurred in soil samples with high C concentrations compared to desorption from low-C subsoil (Lang and Kaupenjohann, 2003). OM can also increase Mo bioavailability to plants by restricting Mo interactions with other metal oxides (Jenne, 1977). These processes potentially slow down Mo immobilization on flocs or its decomposition products in high C soils, explaining why water-soluble Mo is not reduced as significantly as in low C soils.
In contrast, in low C soils, dissolved organic carbon (DOC) appears to have a negative effect on Mo bioavailability. This finding aligns with previous research indicating that the addition of organic materials decreases exchangeable soil Mo, indicating Mo fixation with increasing OM content (Gupta, 1971). Similarly, Xu et al. (2013) found that the concentration of Mo in soil solution decreases with increasing soil OC. It is possible that in low C soils, DOC restricted Mo bioavailability through strong binding mechanisms such as ligand exchange and specific adsorption processes. (Xu et al., 2013). Furthermore, Fe bound in dissolved organic matter may be responsible for Mo adsorption (Lombin, 1985). Moreover, it is possible that Mo was incorporated into floc structure. For instance, molybdate ion can be incorporated into hydrotalcite minerals that are formed by the precipitation of Fe or Al oxides (Allada et al., 2002; Smith et al., 2005; Paikaray and Hendry, 2013). Natural Mo mineralization in these soils is likely low due to low C content and is unable to overcome the negative effects of added C on Mo bioavailability.
4.3.2 Relationship between water soluble Mo and clay + silt and sand contents
Our findings are not consistent with previous research indicating that the availability of Mo increases with higher clay and silt content in the soil texture (Srivastava and Gupta, 1996). According to the literature, Mo has a strong affinity for adsorption onto clay minerals such as kaolinite, illite, and montmorillonite (Goldberg et al., 1996), and soils with a higher percentage of clay tend to have higher available Mo content (Lombin, 1985). However, we observed that soils with the highest clay content had the lowest Mo bioavailability in our study. This discrepancy can be attributed to the charges present on clay minerals, which are influenced by pH and the point of zero charge (PZC). Positively charged clays are more likely to adsorb MoO42- and reduce its bioavailability compared to negatively charged clays.
Sandy soils, on the other hand, are typically nutrient-poor, which may explain the low Mo bioavailability observed in the 1% C-Sand soil in our study. Previous studies by Bloomfield and Kelso (1973), Karimian and Cox (1978), and Riley et al. (1987) have also reported that Mo is prone to leaching in sandy soils, with the extent of leaching depending on soil pH.
4.3.3 Relationship among water-soluble Mo, pH, and Fe
Soil pH has been cited as an important control on Mo bioavailability. It has been observed that Mo bioavailability is limited in soils with pH below 5.5, primarily due to adsorption with soil colloids such as Fe oxides (Duval et al., 2015). Lindsay (1979) reported that the concentration of MoO42− increases by a factor of 100 for each unit increase above pH 3. This is attributed to reduced activity of Fe and Al minerals, an increase in free negatively charged soil colloids, and stronger competition between molybdate and hydroxyl anions for adsorption sites (Jarrell and Dawson, 1978; Jiang et al., 2015). In our study, most of the floc-amended low and high C soils had an average pH of 5.5 at day 30 of the incubation, indicating potential adsorption of Mo on Fe species. This adsorption is likely to occur in low C soils. For instance, we found that in 1% C- Sand soil, the decrease in water-soluble Mo concentrations (from 0.0066 to 0.0029 mg kg-1 soil) was accompanied by a decrease in pH (from 6.2 to 5.6) and increased soluble Fe (from 0.24 to 6.16 mg kg-1). Water-soluble Mo and Fe were positively and significantly correlated (r2 = 0.94***) indicating that Fe species adsorb Mo in this soil. In contrast, Marks et al. (2015) found that SOM played a more significant role in Mo complexation compared to short-range Fe, manganese (Mn), and Al oxides. In soils spanning a wide range of pH (6.45–4.55), they observed that 33% of bulk soil Mo was associated with SOM, while only 1.4% was associated with these minerals. Unlike in low C soils, water-soluble Mo was not correlated with pH in high C soils, despite having similar pH levels. This finding aligns with the studies by Perakis and Sinkhorn (2011) and Marks et al. (2015), suggesting that pH restricts Mo availability primarily in soils with lower OM content and relatively low soil C levels.
4.4 Connecting the dots: potential connections between floc-induced Mo depletion and nitrification in soils
This study combines floc-mediated Mo availability and nitrification into one environmental model (Figure 11). The results presented here indicate that floc or its decomposition products (Fe and DOC) are effective at removing Mo from soil solution, thereby reducing nitrification in mineral soils. We posit that Mo removal occurs as a result of either incorporation into the stable floc structure in the case of the aromatic floc, or sorption onto DOC and/or Fe in the aliphatic floc treatment; both processes are promoted by decreased pH caused by the presence of floc. We also posit that nitrification is halted at the final step: NO2− oxidation to NO3−. This was confirmed by the fact that NO2− concentrations accumulated in low C soils (Figure 4F). Overall, this mechanism appears to be active in soils with ≤3% C content.
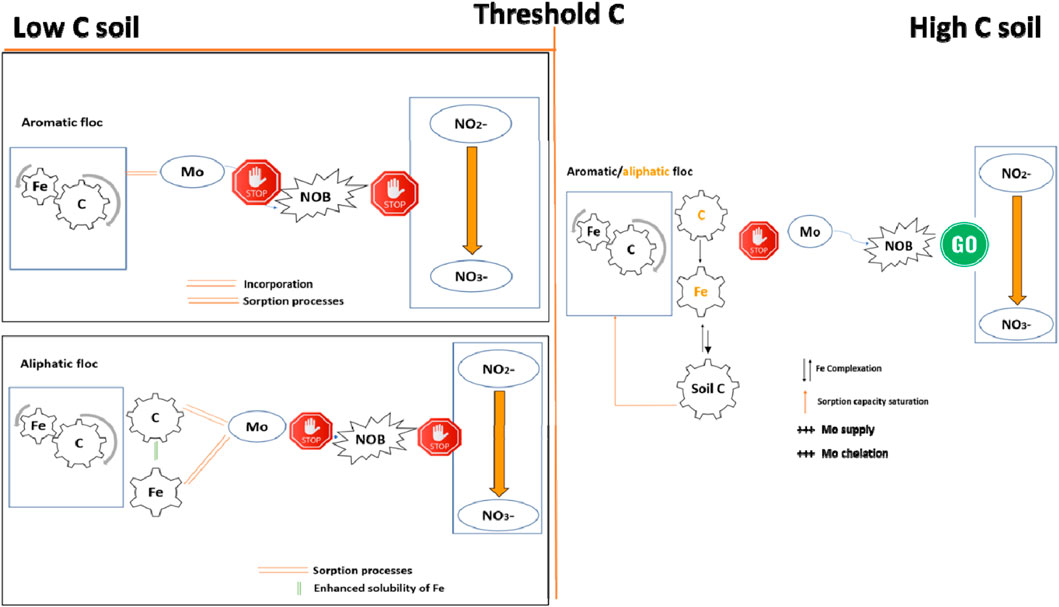
Figure 11. Mechanisms of Fe-OC floc-mediated Rednit in soils across a soil C gradient. NOB: Nitrite oxidizing bacteria.
In soils with high C, net nitrification was not affected by floc-mediated Mo reduction due to their high OM content. Indeed, OM may increase Mo availability in several ways: (a) by enhancing Mo chelation, (b) by enhancing the supply of Mo by SOM mineralization, and/or (c) by occupying adsorption sites on flocs and/or causing the micro aggregation of Fe oxides, thereby limiting the capacity of flocs to adsorb Mo. As a result, nitrification was not inhibited and NO2− did not accumulate, as demonstrated for the 11% C- soil.
4.5 Response of nitrification to Mo addition in 1%C- soil amended with Fe-OC flocs
In our study, none of the supplemental Mo additions increased net nitrification (Figure 9) compared to the control treatments. This raises an intriguing question: if we posit that flocs limited nitrification by decreasing Mo availability, Why did the addition of Mo not eliminate this limitation and increase nitrification?
These results can have three possible explanations:
(a) The amount of Mo required to saturate the adsorption capacity of floc or its decomposition products, while still leaving enough Mo available for microbial utilization, may not have been reached, even with the maximum amount of supplemental Mo. Previous research by Wen et al. (2019) demonstrated that nitrification can resume when a sufficient amount of Mo is applied. In their study, the application of 0.3 mg kg-1 Mo increased NO3− concentrations in an acidic soil (pH = 4.99). This is a very small amount of Mo added in comparison to the levels added in the present study to increase water-soluble Mo. This observation suggests a large capacity for Mo adsorption by flocs.
(b) The added Mo could have been toxic to nitrifiers. Liang and Tabatabai, (1978) found that the application of 480 mg kg-1 Mo inhibited nitrification by 39%–74% and led to the accumulation of nitrite (NO2−) in three different soils. However, this possibility was discarded in our soils since net nitrification in floc-amended soil was similar with and without added Mo (Figure 9), indicating that the decrease in net nitrification originated from the floc addition rather than the added Mo. Moreover, Ueda et al. (1988) demonstrated that Mo addition up to 1,000 mg kg-1 as sodium molybdate was not toxic, and Buekers et al. (2010) determined that the toxicity threshold is 3,129 (2,910–3,363) mg.kg-1 expressed as total Mo in soil.
(c) The presence of floc or its decomposition products may have caused an imbalance in the concentrations of one or more other nutrients/metals required for the growth of nitrifiers or the nitrification process. For example, the growth of a denitrifying microorganism in a medium initially depleted in Mo by Fe and Al precipitation was not fully restored by the addition of Mo, potentially due to a lack of other necessary nutrients (Ge et al., 2018). In our study, it appears that many other nutrients and metals concentrations were affected, which may have contributed to the effects of flocs on nitrification in this study.
4.6 Influence of changes in soil nutrient status on nitrification
Changes in the water soluble fraction of other nutrient and metal concentrations in flocs amended soils could have contributed to flocs effects on nitrification, considering that (1) the nitrifying communities are highly sensitive to the levels of both growth and non-growth substrates (Smith, 1964; Killham, 1990; Daims et al., 2016; Koch et al., 2019; Fujitani et al., 2020) and (2) when present in excess, many nutrients become toxic to the nitrifying community (Loveless and Painter, 1968; Liang and Tabatabai, 1977; Giashuddin and Cornfield, 1979; Cela and Sumner, 2002; Çeçen et al., 2010; Huang et al., 2016; Aslan and Sozudogru, 2017). However, the lack of established toxicity thresholds for soil nitrifiers makes definitive conclusions challenging. Moreover, The cumulative effects of soil nutrients on nitrification are complex, as interactions between different elements can exhibit various synergistic, additive, or antagonistic effects, further complicating the understanding of their combined impact on nitrifying communities (Gikas, 2008). For instance, Ca and Mg have been found to alleviate cobalt (Co) toxicity to nitrifiers in a medium (Loveless and Painter, 1968).
4.7 The effects of flocs on nitrification in low C soils: The theory
The effects of flocs on nitrification in low C soils can be explained using the concept of Liebig’s Law of the Minimum. This law states that the nutrient which is present in its minimum concentration can affect and regulate plant growth. This law applies to microbial processes as well, including nitrification. The growth of nitrifiers, like all microbes, can be described by the following relationship (C + N + P + other nutrients (e.g., Mo, Fe, Cu, etc.) - > microbial biomass). In this study, we found that the bioavailability of Mo was decreased in flocs-amended soils. Considering the importance of Mo for both nitrifier growth and activity, as backed up by our PLSR analyses, we posit that Mo was a limiting nutrient for nitrification in soils ≤3% C (Figure 12). However, it is important to acknowledge that nutrient toxicity and changes in nutrient status presents another potential pathway through which Fe-OC flocs may have influenced nitrification in low C soils. As mentioned earlier, the lack of established toxicity thresholds specific to nitrifiers in soils and the uncertainties surrounding the determination of the cumulative effects of soil nutrients and metals on nitrification make it challenging to draw definitive conclusions. Further studies are necessary to confirm and gain a better understanding of these interactions.
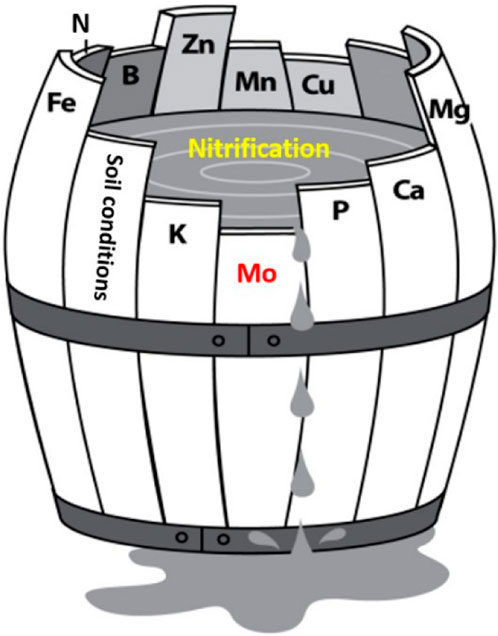
Figure 12. Liebig’s Barrel is used to illustrate the application of Liebig’s “Law of the Minimum” to flocs-driven nitrification in low C soils. The Liebig’s barrel illustrates that if Mo is missing or deficient, nitrification rates will be poor, regardless of the availability of other nutrients. If the shortest stave is lengthened sufficiently, then another nutrient can become the “limiting nutrient” depending on the needs of the nitrifying population.
5 Conclusion
Flocs in soil can originate from the natural coprecipitation of metals and DOM. Floc formation is routinely used in engineered systems, such as wastewater treatment or on-site agricultural drainage treatment facilities, which use iron-based coagulants to remove DOM from the waters or constructed wetlands. Flocs are shown to reverse land subsidence and enhance C sequestration due to their high stability in wetland environments. However, the present study raises questions on the importance of stability of flocs for soil C and N sequestration. It is often assumed that stable flocs are not reactive, based on the observation that C bioavailability is constrained, resulting in slower C cycling. Our study shows that stable structures can be reactive towards other nutrients (i.e., Mo), which may constrain C and N sequestration. Indeed, Van Groenigen et al. (2006) found that soil C sequestration is restricted by N availability and nutrients that support N2 fixation, such as Mo, P, and K. Therefore, adopting a more holistic approach that considers the effect of flocs on other soil nutrients can bring new insight into mechanisms of C and N sequestration and offer new possibilities for the use of flocs in management. Soil properties and conditions should also be considered in this holistic approach. In fact, our study showed that nitrification in high C soils appeared to be less sensitive to the application of flocs compared to low C soils.
This study also suggests that the application of flocs decreases soil nitrification by inducing Mo deficiency in soils with low C content. This decrease in net nitrification was accompanied by a minimal decrease in NH4+ (Supplementary Table S3). More broadly, these results imply that flocs could be useful for decreasing nitrification in agricultural systems and control N bioavailability to plants. Decreased nitrification from agricultural fields can alleviate other environmental issues such as eutrophication of lakes and water pollution. Additionally, we suggest that the fertilizer industry could also use flocs as a coating during fertilizer preparations to enhance their efficiency.
Because flocs can limit nitrification and extend the residence time of NH4+ in agricultural soils, data on flocs-driven changes in soil nutrient status is also useful to understand their possible effects on plant growth and development. In general, a deficiency in one or more of soil nutrients can decrease plant growth and development (Merchant, 2010), or can cause toxicity when present in excess (Eid et al., 2012). However, a study by Liang et al. (2019) found that coagulation treatment with Al- and Fe-based coagulants did not disrupt the growth of Typha plant grown in a constructed wetland, despite a slight change in soil nutrient content within a 2-year period (Liang et al., 2019). More studies are needed to explore the usefulness of flocs in agriculture or as wetland restoration strategy, especially in terms of their effects on both plant growth and other N cycling transformations, such as denitrification and N fixation.
Data availability statement
The raw data supporting the conclusion of this article will be made available by the authors, without undue reservation.
Author contributions
IS: Conceptualization, Data curation, Formal Analysis, Methodology, Visualization, Writing–original draft, Writing–review and editing. TD: Conceptualization, Methodology, Writing–review and editing. XZ-B: Conceptualization, Investigation, Methodology, Validation, Writing–review and editing. PL: Writing–review and editing. RL: Writing–review and editing. DZ: Writing–review and editing. WH: Conceptualization, Funding acquisition, Investigation, Methodology, Supervision, Validation, Writing–review and editing.
Funding
The author(s) declare that financial support was received for the research, authorship, and/or publication of this article. We acknowledge funding from Mohammed VI Polytechnic University and the J. G. Boswell Endowed Chair in Soil Science.
Acknowledgments
We acknowledge the contributions of Afafe Slimani in editing this paper.
Conflict of interest
The authors declare that the research was conducted in the absence of any commercial or financial relationships that could be construed as a potential conflict of interest.
Publisher’s note
All claims expressed in this article are solely those of the authors and do not necessarily represent those of their affiliated organizations, or those of the publisher, the editors and the reviewers. Any product that may be evaluated in this article, or claim that may be made by its manufacturer, is not guaranteed or endorsed by the publisher.
Supplementary material
The Supplementary Material for this article can be found online at: https://www.frontiersin.org/articles/10.3389/fmats.2024.1346112/full#supplementary-material
References
Allada, R. K., Navrotsky, A., Berbeco, H. T., and Casey, W. H. (2002). Thermochemistry and aqueous solubilities of hydrotalcite-like solids. Science 296, 721–723. doi:10.1126/science.1069797
Aslan, S., and Sozudogru, O. (2017). Individual and combined effects of nickel and copper on nitrification organisms. Ecol. Eng. 99, 126–133. doi:10.1016/j.ecoleng.2016.11.019
Ayiti, O. E., and Babalola, O. O. (2022). Factors influencing soil nitrification process and the effect on environment and health. Front. Sustain. Food Syst. 6, 821994. doi:10.3389/fsufs.2022.821994
Barrón, V., Herruzo, M., and Torrent, J. (1988). Phosphate adsorption by aluminous hematites of different shapes. Soil Sci. Soc. Am. J. 52, 647–651. doi:10.2136/sssaj1988.03615995005200030009x
Bhunia, P. (2014). “Fundamentals of biological treatment,” in Comprehensive water quality and purification. Editor S. Ahuja (Waltham, Elsevier), 47–73. doi:10.1016/b978-0-12-382182-9.00048-7
Bloomfield, C., and Kelso, W. I. (1973). The mobilisation and fixation of molybdenum, vanadium, and uranium by decomposing plant matter. J. Soil. Sci. 24, 368–379. doi:10.1111/j.1365-2389.1973.tb00772.x
Bolan, N. S., Adriano, D. C., and Curtin, D. (2003). Soil acidification and liming interactions with nutrient and heavy metal transformation and bioavailability. Adv. Agron. 78, 215–272. doi:10.1016/s0065-2113(02)78006-1
Buekers, J., Mertens, J., and Smolders, E. (2010). Toxicity of the molybdate anion in soil is partially explained by effects of the accompanying cation or by soil pH. Environ. Toxicol. Chem. 29, 1274–1278. doi:10.1002/etc.162
Butler, J. H., and Gordon, L. I. (1986). Rates of nitrous oxide production in the oxidation of hydroxylamine by iron (III). Inorg. Chem. 25, 4573–4577. doi:10.1021/ic00245a024
Çeçen, F., Semerci, N., and Geyik, A. G. (2010). Inhibitory effects of Cu, Zn, Ni and Co on nitrification and relevance of speciation. J. Chem. Technol. Biot. 85, 520–528. doi:10.1002/jctb.2321
Cela, S., and Sumner, M. (2002). Soil zinc fractions determine inhibition of nitrification, water. Air. Soil. Poll. 141, 91–104. doi:10.1023/a:1021379421878
Chicano, T. M., Dietrich, L., de Almeida, N. M., Akram, M., Hartmann, E., Leidreiter, F., et al. (2021). Structural and functional characterization of the intracellular filament-forming nitrite oxidoreductase multiprotein complex. Nat. Microbiol. 6, 1129–1139. doi:10.1038/s41564-021-00934-8
Colombo, C., Palumbo, G., He, J.-Z., Pinton, R., and Cesco, S. (2014). Review on iron availability in soil: interaction of Fe minerals, plants, and microbes. J. Soil. Sediment. 14, 538–548. doi:10.1007/s11368-013-0814-z
Daims, H., Lücker, S., and Wagner, M. (2016). A New Perspective on microbes formerly known as nitrite-oxidizing bacteria. Trends. Microbiol. 24, 699–712. doi:10.1016/j.tim.2016.05.004
Dempsey, B. A., Ganho, R. M., and O'Melia, C. R. (1984). The coagulation of humic substances by means of aluminum salts. J. Am. Water. Works. Ass. 76, 141–150. doi:10.1002/j.1551-8833.1984.tb05315.x
Doane, T. A., and Horwáth, W. R. (2003). Spectrophotometric determination of nitrate with a single reagent. Anal. Lett. 36, 2713–2722. doi:10.1081/al-120024647
Duff, R. B., Webley, D. M., and Scott, R. O. (1963). Solubilization of minerals and related materials by 2-ketogluconic acid-producing bacteria. Soil Sci. 95, 105–114. doi:10.1097/00010694-196302000-00004
Duval, B. D., Natali, S. M., and Hungate, B. A. (2015). What constitutes plant-available molybdenum in sandy acidic soils? Commun. Soil. Sci. Plan. 46, 318–326. doi:10.1080/00103624.2014.969405
Eid, E. M., Shaltout, K. H., El-Sheikh, M. A., and Asaeda, T. (2012). Seasonal courses of nutrients and heavy metals in water, sediment and above- and below-ground Typha domingensis biomass in Lake Burullus (Egypt): perspectives for phytoremediation. Flora 207, 783–794. doi:10.1016/j.flora.2012.09.003
Ensign, S. A., Hyman, M. R., and Arp, D. J. (1993). In vitro activation of ammonia monooxygenase from Nitrosomonas europaea by copper. J. Bacteriol. 175, 1971–1980. doi:10.1128/jb.175.7.1971-1980.1993
Finstein, M. S., and Delwiche, C. C. (1965). Molybdenum as a micronutrient for nitrobacter. J. Bacteriol. 89, 123–128. doi:10.1128/jb.89.1.123-128.1965
Fischer, K., and Bipp, H. P. (2002). Removal of heavy metals from soil components and soils by natural chelating agents. Part II. Soil extraction by sugar acids, water. Air. Soil. Poll. 138, 271–288. doi:10.1023/A:1015566207849
Foster, J. C. (1995). “Soil nitrogen,” in Methods in applied soil microbiology and biochemistry. Editors K. Alef, and P. Nannipieri (San Diego, CA: Academic Press), 79–87.
Fujitani, H., Nomachi, M., Takahashi, Y., Hasebe, Y., Eguchi, M., and Tsuneda, S. (2020). Successful enrichment of low-abundant comammox Nitrospira from nitrifying granules under ammonia-limited conditions. FEMS. Microbiol. Lett. 367, fnaa025. doi:10.1093/femsle/fnaa025
Ge, X., Vaccaro, B. J., Thorgersen, M. P., Poole, F. L., Majumder, E. L., Zane, G. M., et al. (2018). Iron-and aluminium-induced depletion of molybdenum in acidic environments impedes the nitrogen cycle: Mo depletion by Fe/Al precipitation at ORR. Environ. Microbiol. 21, 152–163. doi:10.1111/1462-2920.14435
Gee, G. W., and Or, D. (2002). “Particle size analysis,” in Methods of soil analysis, Part 4, physical methods. Editors J. H. Dane, and G. C. Topp (Madison: Soils Science Society of America), 255–293. Book Series No. 5.
Giashuddin, M., and Cornfield, A. (1979). Effects of adding nickel (as oxide) to soil on nitrogen and carbon mineralisation at different pH values. Environ. Pollut. 19, 67–70. doi:10.1016/0013-9327(79)90113-7
Gikas, P. (2008). Single and combined effects of nickel (Ni(II)) and cobalt (Co(II)) ions on activated sludge and on other aerobic microorganisms: a review. J. Hazard. Mat. 159, 187–203. doi:10.1016/j.jhazmat.2008.02.048
Goldberg, S., Forster, H. S., and Godfrey, C. L. (1996). Molybdenum adsorption on oxides, clay minerals, and soils. Soil. Sci. Soc. Am. J. 60, 425–432. doi:10.2136/sssaj1996.03615995006000020013x
Gupta, U. C. (1971). Influence of various organic materials on the recovery of molybdenum and copper added to a sandy clay loam soil. Plant. Soil. 34, 249–253. doi:10.1007/bf01372781
Henneberry, Y. K., Kraus, T. E., Nico, P. S., and Horwath, W. R. (2012). Structural stability of coprecipitated natural organic matter and ferric iron under reducing conditions. Org. Geochem. 48, 81–89. doi:10.1016/j.orggeochem.2012.04.005
Huang, X., Zhu-Barker, X., Horwath, W., Faeflen, S., Luo, H., Xin, X., et al. (2016). Effect of iron oxide on nitrification in two agricultural soils with different pH. Biogeosciences 13, 5609–5617. doi:10.5194/bg-13-5609-2016
Jarrell, W. M., and Dawson, M. D. (1978). Sorption and availability of molybdenum in soils of Western Oregon. Soil. Sci. Soc. Am. J. 42, 412–415. doi:10.2136/sssaj1978.03615995004200030007x
Jenne, E. A. (1977). “Trace element sorption by sediments and soils: sites and processes,” in Molybdenum in the environment. Editor R. F. Gould (New York: Marcel Dekker), 425–553.
Jiang, W., Yang, Z. F., Yu, T., Hou, Q. Y., Zhong, C., Zheng, G. D., et al. (2015). Evaluation of the potential effects of soil properties on molybdenum availability in soil and its risk estimation in paddy rice. J. Soil. Sediment. 15, 1520–1530. doi:10.1007/s11368-015-1107-5
Karimian, N., and Cox, F. R. (1978). Adsorption and extractability of molybdenum in relation to some chemical properties of soil. Soil, Sci. Soc. Am. J. 42, 757–761. doi:10.2136/sssaj1978.03615995004200050021x
Killham, K. (1990). Nitrification in coniferous forest soils. Plant. Soil. 128, 31–44. doi:10.1007/bf00009394
Koch, H., van Kessel, M. A., and Lücker, S. (2019). Complete nitrification: insights into the ecophysiology of comammox Nitrospira. Appl. Microbiol. Biot. 103, 177–189. doi:10.1007/s00253-018-9486-3
Lancaster, K. M., Caranto, J. D., Majer, S. H., and Smith, M. A. (2018). Alternative bioenergy: updates to and challenges in nitrification metalloenzymology. Joule 2, 421–441. doi:10.1016/j.joule.2018.01.018
Lang, F., and Kaupenjohann, M. (2003). Immobilisation of molybdate by iron oxides: effects of organic coatings. Geoderma 113, 31–46. doi:10.1016/s0016-7061(02)00314-2
Lehmann, R. G., Cheng, H. H., and Harsh, J. B. (1987). Oxidation of phenolic acids by soil iron and manganese oxides. Soil. Sci. Soc. Am. J. 51, 352–356. doi:10.2136/sssaj1987.03615995005100020017x
Li, Y., Wang, M., Zhang, Y., Koopal, L. K., and Tan, W. (2020). Goethite effects on transport and activity of lysozyme with humic acid in quartz sand. Colloid. Surf. A 604, 125319. doi:10.1016/j.colsurfa.2020.125319
Liang, C. N., and Tabatabai, M. A. (1977). Effects of trace elements on nitrogen mineralisation in soils. Environ. Pollut. 12, 141–147. doi:10.1016/0013-9327(77)90017-9
Liang, C. N., and Tabatabai, M. A. (1978). Effects of trace elements on nitrification in soils. J. Environ. Qual. 7, 291–293. doi:10.2134/jeq1978.00472425000700020028x
Liang, Y. L., Kraus, T. E. C., Silva, L. C. R., Bachand, P. A. M., Bachand, S. M., Doane, T. A., et al. (2019). Effects of ferric sulfate and polyaluminum chloride coagulation enhanced treatment wetlands on Typha growth, soil and water chemistry. Sci. Total. Environ. 648, 116–124. doi:10.1016/j.scitotenv.2018.07.341
Lindsay, W. L. (1979). Chemical equilibria in soils. Chichester, Sussex, UK: John Wiley and Sons Ltd, 449.
Lombin, G. A. (1985). Evaluation of the micronutrient fertility of Nigeria’s semi-arid savannah soils: boron and molybdenum. Soil. Sci. Plant. Nutr. 31, 13–25. doi:10.1080/17470765.1985.10555213
Loveless, J. E., and Painter, H. A. (1968). The influence of metal ion concentrations and pH value on the growth of a Nitrosomonas strain isolated from activated sludge. Microbiology 52, 1–14. doi:10.1099/00221287-52-1-1
Marks, J. A., Perakis, S. S., King, E. K., and Pett-Ridge, J. (2015). Soil organic matter regulates molybdenum storage and mobility in forests. Biogeochemistry 125, 167–183. doi:10.1007/s10533-015-0121-4
McBride, M. B. (1987). Adsorption and oxidation of phenolic compounds by iron and manganese oxides. Soil. Sci. Soc. Am. J. 51, 1466–1472. doi:10.2136/sssaj1987.03615995005100060012x
Meiklejohn, J. (1952). Minimum phosphate and magnesium requirements of nitrifying bacteria. Nature 170, 1131. doi:10.1038/1701131a0
Meincke, M., Bock, E., Kastrau, D., and Kroneck, P. M. (1992). Nitrite oxidoreductase from Nitrobacter hamburgensis: redox centers and their catalytic role. Arc. Microbiol. 158, 127–131. doi:10.1007/bf00245215
Merchant, S. S. (2010). The elements of plant micronutrients. Plant. Physiol. 154, 512–515. doi:10.1104/pp.110.161810
Mikutta, R., Kleber, M., Torn, M. S., and Jahn, R. (2006). Stabilization of soil organic matter: association with minerals or chemical recalcitrance? Biogeochemistry 77, 25–56. doi:10.1007/s10533-005-0712-6
Mulvaney, R. L. (1996). “Nitrogen-inorganic forms,” in Methods of soil analysis. Part 3. Chemical methods. Editor D. L. Sparks (Madison, WI: Soil Science Society of America, American Society of Agronomy), 1123–1184.
Nevison, C., Hess, P., Riddick, S., and Ward, D. (2016). Denitrification, leaching, and river nitrogen export in the community earth system model. J. Adv. Model. Earth Syst. 8, 272–291. doi:10.1002/2015MS000573
Newcomb, C. J., Qafoku, N. P., Grate, J. W., Bailey, V. L., and De Yoreo, J. J. (2017). Author Correction: developing a molecular picture of soil organic matter–mineral interactions by quantifying organo–mineral binding. Nat. Commun. 8, 2017–2018. doi:10.1038/s41467-017-02125-8
Norton, J., and Ouyang, Y. (2019). Controls and adaptive management of nitrification in agricultural soils. Front. Microbiol. 10, 1931. doi:10.3389/fmicb.2019.01931
Paikaray, S., and Hendry, M. J. (2013). In situ incorporation of arsenic, molybdenum, and selenium during precipitation of hydrotalcite-like layered double hydroxides. Appl. Clay. Sci. 77–78, 33–39. doi:10.1016/j.clay.2013.03.016
Perakis, S. S., and Sinkhorn, E. R. (2011). Biogeochemistry of a temperate forest nitrogen gradient. Ecology 92, 1481–1491. doi:10.1890/10-1642.1
Raglin, S., Soman, C., Ma, Y., and Kent, A. (2022). Long term influence of fertility and rotation on soil nitrification potential and nitrifier communities. Front. Soil. Sci. 2, 838497. doi:10.3389/fsoil.2022.838497
Riley, M. M., Robson, A. D., Gartrell, J. W., and Jeffery, R. C. (1987). The absence of leaching of molybdenum in acidic soils from Western Australia. Aust. J. Soil. Res. 25, 179. doi:10.1071/sr9870179
Rutkowska, B., Szulc, W., Spychaj-Fabisiak, E., and Pior, N. (2017). Prediction of molybdenum availability to plants in differentiated soil conditions. Plant. Soil. Environ. 63, 491–497. doi:10.17221/616/2017-pse
Sahrawat, K. L. (1982). Nitrification in some tropical soils. Plant. Soil. 65, 281–286. doi:10.1007/bf02374659
Sato, C., Schnoor, J. L., and McDonald, D. B. (1986). Effects of copper and nickel on the growth of Nitrosomonas europaea. Toxic. Assess. 1, 357–376. doi:10.1002/tox.2540010307
Schwertmann, U., Kodama, H., and Fischer, W. R. (1986). “Mutual interactions between organics and iron oxides,” in Interactions of soil minerals with natural organics and microbes. Editors P. M. Huang, and M. Schnitzer (John Wiley and Sons), 223–250.
Seda, N. N. (2014). Iron oxide-organic matter coprecipitates and controls on copper availability. master's thesis. United States: University of Connecticut.
Siéliéchi, J. M., Lartiges, B. S., Kayem, G. J., Hupont, S., Frochot, C., Thieme, J., et al. (2008). Changes in humic acid conformation during coagulation with ferric chloride: implications for drinking water treatment. Water. Res. 42, 2111–2123. doi:10.1016/j.watres.2007.11.017
Smith, H. D., Parkinson, G. M., and Hart, R. D. (2005). In situ absorption of molybdate and vanadate during precipitation of hydrotalcite from sodium aluminate solutions. J. Cryst. Growth. 275, e1665–e1671. doi:10.1016/j.jcrysgro.2004.11.227
Smith, J. H. (1964). Relationships between soil cation-exchange capacity and the toxicity of ammonia to the nitrification process. Soil. Sci. Soc. Am. J. 28, 640–644. doi:10.2136/sssaj1964.03615995002800050019x
Srivastava, P. C., and Gupta, U. C. (1996). Trace elements in crop production. Lebanon: Science Publishers, Inc.
Tandon, S. P., and Mishra, M. M. (1968). Effect of molybdenum, vanadium and tungsten on the activity of Nitrobacter agilis. Agr. Biol. Chem. 32, 1190–1192. doi:10.1080/00021369.1968.10859207
Ueda, K., Kobayashi, M., and Takahashi, E. (1988). Effect of anionic heavy metals on ammonification and nitrification in soil. Soil. Sci. Plant. Nutr. 34, 139–146. doi:10.1080/00380768.1988.10415587
Van Droogenbroeck, R., and Laudelout, H. (1967). Phosphate requirements of the nitrifying bacteria. Ant. Leeuw. 33, 287–296. doi:10.1007/bf02045574
Van Groenigen, K. J., Six, J., Hungate, B. A., de Graaff, M. A., Van Breemen, N., and Van Kessel, C. (2006). Element interactions limit soil carbon storage. P. Natl. A. Sci. 103, 6571–6574. doi:10.1073/pnas.0509038103
Verdouw, H., van Echteld, C. J. A., and Dekkers, E. M. J. (1978). Ammonia determination based on indophenol formation with sodium salicylate. Water Res. 12, 399–402. doi:10.1016/0043-1354(78)90107-0
Wagai, R., and Mayer, L. M. (2007). Sorptive stabilization of organic matter in soils by hydrous iron oxides. Geochim. Cosmochim. Ac. 71, 25–35. doi:10.1016/j.gca.2006.08.047
Wen, X., Hu, C., Sun, X., Zhao, X., and Tan, Q. (2019). Research on the nitrogen transformation in rhizosphere of winter wheat (Triticum aestivum) under molybdenum addition. Environ. Sci. Pollut. R. 26, 2363–2374. doi:10.1007/s11356-018-3565-y
Wichard, T., Mishra, B., Myneni, S. C., Bellenger, J. P., and Kraepiel, A. M. (2009). Storage and bioavailability of molybdenum in soils increased by organic matter complexation. Nat. Geosci. 2, 625–629. doi:10.1038/ngeo589
Xu, N., Braida, W., Christodoulatos, C., and Chen, J. P. (2013). A Review of molybdenum adsorption in soils/bed sediments: speciation, mechanism, and model applications. Soil. Sediment. Contam. 22, 912–929. doi:10.1080/15320383.2013.770438
Yanase, M., Takahashi, M., Tanaka, T., and Matsuo, Y. (2000). Nitrification denitrification under molybdenum deficiency. Environm. Eng. Res. 37, 17–27. doi:10.11532/proes1992.37.17
You, M., Zhu-Barker, X., Doane, T. A., and Horwath, W. R. (2022). Decomposition of carbon adsorbed on iron (III)-treated clays and their effect on the stability of soil organic carbon and external carbon inputs. Biogeochemistry 157, 259–271. doi:10.1007/s10533-021-00873-w
Zavarzin, G. A. (1957). The participation of molybdenum in the oxidation of nitrites by nitrifying bacteria. Dokl. Akad. Nauk. SSSR 113, 1361–1362.
Zavarzin, G. A. (1958). On the Inducer of the Second Phase of Nitrification. Concerning the participation of respiratory pigments in nitrification. Mikrobiologiya 21, 395–399.
Zhao, Q., Poulson, S., Obrist, D., Sumaila, S., Dynes, J., McBeth, J., et al. (2016). Iron-bound organic carbon in forest soils: quantification and characterization. Biogeosciences 13, 4777–4788. doi:10.5194/bg-13-4777-2016
Zimmermann, K., and von Lengerken, J. (1979). Analytical methods for nitrate and nitrite determination in foods. 3. Spectrophotometric determination of nitrate and nitrite using sulphanilic acid/1-naphylamine, and of nitrite using resorcinol/zirconium (IV) oxychloride. Die Nahr. 23 (9–10), 929–934. doi:10.1002/food.19790230910
Keywords: nitrification, iron-organic matter coprecipitates, flocs, molybdenum, soils
Citation: Slimani I, Doane T, Zhu-Barker X, Lazicki P, Lybrand RA, Zaharescu DG and Horwath W (2024) Iron-organic carbon coprecipitates reduce nitrification by restricting molybdenum in agricultural soils. Front. Mater. 11:1346112. doi: 10.3389/fmats.2024.1346112
Received: 28 November 2023; Accepted: 26 February 2024;
Published: 05 April 2024.
Edited by:
Renji Zheng, Central South University, ChinaReviewed by:
Lin Wei, Lam Research, United StatesFengxia Yang, Ministry of Agriculture and Rural Affairs, China
Copyright © 2024 Slimani, Doane, Zhu-Barker, Lazicki, Lybrand, Zaharescu and Horwath. This is an open-access article distributed under the terms of the Creative Commons Attribution License (CC BY). The use, distribution or reproduction in other forums is permitted, provided the original author(s) and the copyright owner(s) are credited and that the original publication in this journal is cited, in accordance with accepted academic practice. No use, distribution or reproduction is permitted which does not comply with these terms.
*Correspondence: Imane Slimani, islimani@ucdavis.edu