Formulation and evaluation of ophthalmic microemulsion for enhanced topical administration of brinzolamide
- 1Department of Chemistry, Division of Science and Technology, University of Education, Lahore, Pakistan
- 2Department of Chemistry, University of Gujrat, Gujrat, Pakistan
- 3Chemical Engineering Department, College of Engineering, King Saud University, Riyadh, Saudi Arabia
- 4Department of Chemical Engineering Materials Environment Sapienza University of Rome, Rome, Italy
- 5Department of Chemistry, Government College Women University Sialkot, Sialkot, Pakistan
- 6Department of Physics, Division of Science and Technology, University of Education, Lahore, Pakistan
Microemulsions (μEs) are more effective than conventional formulations for ophthalmic use due to their optical transparency, thermodynamic stability, structural flexibility and higher bioavailability. In addition, μE formulations can increase the water solubility of the drug and improve drug absorption in the eye. Herein, we report the development of three new biocompatible μE formulations containing an antihypertensive drug brinzolamide (BZD) and their evaluation for topical ocular administration. For this, Formulations A, B and C were optimized using an appropriate ratio of isopropyl myristate (IPM) as oil phase, water as aqueous phase and 2-propanol as co-surfactant, while Tween-80, Tween-20 and Tween-60 were selected as surfactant for each formulation, respectively. Preliminary, pseudoternary phase diagrams were delineated and then electrical conductivity and optical microscopy were used to establish optimal formulation for each μE to upheld the appropriate amount of BZD, i.e., 2.0 wt%, 2.0 wt%, and 1.0 wt% in formulation A, B and C, respectively. Dynamic light scattering demonstrated very fine monomodal assembly of BZD-μE nanodroplets (∼50 nm), while FTIR analysis showed effective encapsulation of BZD into hydrophobic microenvironment with no observable chemical interaction between BZD and μE excipients, which was further verified by the peak-to-peak concomitant measurement of fluorescence. Further, in-vitro release of BZD-μE showed enhanced and persistent topical ocular administration (>99%) within 10 h demonstrating the appropriate formulation for topical instillation.
Introduction
Glaucoma is a serious chronic disease that causes weakening of the retinal nerves and ganglion cells, leading to pathophysiological changes in the structure of the eye. Glaucoma can cause vision loss and, if left untreated, lead to complete blindness. Intraocular pressure is the main cause of the development of glaucoma due to the increased production of aqueous humour (Loiselle et al., 2020). Brinzolamide (BZD) is used as the first-line drug for the treatment of glaucoma (Silver and Group, 2000). BZD is an ocular carbonic anhydrase inhibitor and reduces the production of aqueous humour and directly lowers intraocular pressure when applied to the target area (Eissa, 2016; Choradiya and Patil, 2022). BZD being a lipophilic drug, has very poor solubility in aqueous media and the commercial BZD formulation contains only 1% of BZD in the water suspension. This suspension has many side effects on the eyes, including blurred vision, pain and dry eyes, and this limitation significantly reduces the importance of BZD as an ophthalmic medication (Gohil et al., 2020). Apart from the side effects, it has other limitations like small residence time, poor bioavailability and pre-corneal drug loss. The delivery of drugs to the eye is a challenging process due to the unique physiology and anatomy of the eye (Urtti, 2006).
Topical administration of eye drops is the most common method of treating eye diseases due to the presence of blood–retinal barrier that makes systemic drug administration ineffective. Various studies have been conducted to develop novel methods such as micellar systems, contact lenses, hydrogels and microemulsions (μE) that can increase the bioavailability of the drug to the eye (Abd El Wahab et al., 2022; Qadri et al., 2022a; Saleem et al., 2023). μE has emerged as an effective alternative to conventional ocular drug delivery methods (Grampurohit et al., 2011; Patel et al., 2013; Nazar et al., 2018). μEs are being explored as an effective drug delivery vehicle for ophthalmic medications, offering advantages such as structural flexibility, optical transparency, thermodynamic stability, enhanced solubilization, higher bioavailability and ease of formation (Constantinides, 1995; Gasco, 1997; Tenjarla, 1999; Kantaria et al., 2003).
μEs are stable liquid mixtures containing oil and aqueous phases as well as surfactants and co-surfactants and are isotropic and clear in appearance (Saleem et al., 2020). Their formation is easy compared to ordinary emulsions and does not require high shear conditions (Danielsson, 1981; Yuan et al., 2006; Narang et al., 2007). Depending on the composition of the formulation, there are three types of μE. In the oil-in-water (o/w) μE, there is a continuous water phase in which oil droplets are dispersed. In contrast, the dispersion of water droplets in the continuous oil phase leads to the formation of water-in-oil (w/o) μE (Yasir Siddique et al., 2021). However, if the aqueous and oil phases are inter dispersed, this is an indication of a bicontinuous μE (Scriven, 1976; Danielsson, 1981). An aim of the present study is to increase the bioavailability of BZD and improve the ophthalmic formulation for therapeutic relief to reduce intraocular pressure. In the present work, three new μE formulations were optimized using appropriate ratio of isopropyl myristate (IPM), water and 2-propanol, while Tween-80, Tween-20 and Tween-60 were selected as surfactant for each formulation respectively, to improve the solubility and load of BZD (basic molecular structure of BZD, Tween-20, Tween-60, Tween-80 are given in the Supplementary Material S1). By delineating the pseudoternary phase diagram and employing several complementary characterization techniques, optimal μE formulations were developed to upheld the appropriate amount of BZD, i.e., 2.0 wt%, 2.0 wt%, and 1.0 wt% in formulation A, B and C, respectively. The as-formulated smart template is a promising colloidal carrier for effective topical ocular administration of BZD with extended retention interval.
Materials and methods
Materials and chemicals
Isopropyl myristate (99.99%) and 2-propanol (99.99%) were purchased from Sigma-Aldrich, while Tween-80® (99.99%), Tween-60® (99.99%) and Tween-20® (99.99%) were purchased from VWR Chemicals. Schazoo Zaka Lab (PVT), Lahore, Pakistan, generously provided Brinzolamide (working standards). Distilled and deionized water was used for dilution.
Microemulsion formulation and mapping of ternary phase diagram
μEs of three different formulations were prepared using the titration method by mixing IPM as oil, 2-propanol as cosurfactant, Tween-80, Tween-20 and Tween-60 as surfactants. Surfactant/co-surfactant (Smix) were used in a 1:1 ratio. Water was then added to form the optimized μE formulation. Formulation A consists of IPM, Tween-80, 2-propanol and water. Formulation B, on the other hand, comprised of IPM, Tween-20, 2-propanol and water. While, Formulation C consisted of IPM, Tween-60, 2-propanol and water. Ternary phase diagrams were constructed using random ratios of oil, water and Smix. A dilution line AB as shown in Figure 1, was created at a constant Smix ratio to investigate the structural changes of the μE from w/o to o/w phase. The optimal μEs were selected from the dilution line.
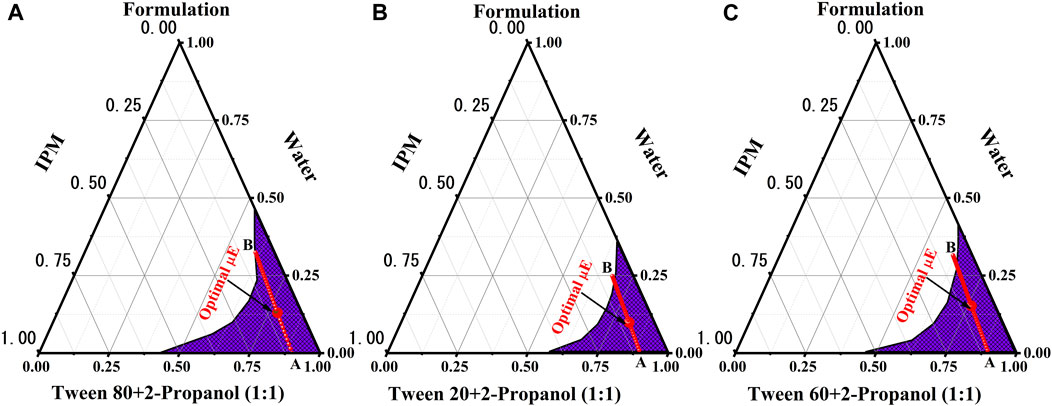
FIGURE 1. Pseudo ternary phase diagram of 2-propanol/Tween-80/Tween-20/Tween-60/IPM/oil/water for formulation (A–C). (A, B) represents the dilution line. The highlighted dot on dilution line indicates the optimal µE.
Incorporation of drug in µE
BZD was dissolved in each optimized μE formulation under continuous stirring at pH 7.2, 7.4 and 7.8 respectively at room temperature (30°C). Optimized μEs were consist of 38% Tween-80, 38% 2-propanol, 8% IPM and 16% water for formulation A, 40% Tween-20, 40% 2-propanol, 9% IPM and 11% water for formulation B and 40% Tween-60, 40% 2-propanol, 8% IPM and 12% water for formulation C μE A, B and C dissolved 2.0wt%, 2.0wt%, and 1.0wt% of BZD, respectively. All drug-free and drug-loaded optimal formulations were stable and transparent over 9 months of storage.
Characterization of microemulsion
Physical features, optical microscopic and stability study
The developed μE formulations were centrifuged for 15 min at 5,500 rpm using Hermle Z200 centrifuge equipment to check the stability of each drug-free and drug-loaded μE. Meanwhile, a biological microscope (LABOMED FLR Lx 400; Jenoptic, Germany) with 4x/10x/40x/×100 magnification was used to reveal the structural transitions in all drug free μE formulations. Viscosity was measured at 25°C ± 0.5°C with a calibrated Brookfield viscometer (LVDV-2T) at 150 rpm by washing and cleaning the viscometer at each measurement. Electrical conductivity measurement has been used to study and validate different types of phase inversion and continuous phase of μEs using a conductivity meter (ADWA AD-3000, Hungry).
Size distribution analysis
Using a Zetasizer (Malvern, Nano ZSP), the average droplet size of BZD-free and BZD-loaded formulation and the polydispersity index (PDI) were determined at room temperature without sample filtration. The equipment had a laser with a wavelength of 635 nm and backscattering technology, also known as NIBS (Non-Invasive Back-Scatter), was used to measure light scattering at an angle of 173°. For accuracy, each individual measurement was performed three times.
Spectroscopic measurements
The IR spectra of drug-free and drug-loaded μEs in the range of 500–4,000 cm-1 were recorded with a resolution of 2 cm-1 using a Bruker FTIR (Alpha series), while the steady-state fluorescence was measured using a spectrofluorophotometer (manufactured by Shimadzu RF-6000). The fluorescence spectra of BZD were recorded in the range of 300–800 nm. These spectra were recorded in aqueous phase, single oil phase, Smix (1:1), and in each of the optimal μE systems.
In-Vitro drug release studies
The in-vitro release of all formulations was evaluated using simulated tear fluid (STF) at 50 rpm. The 2 mL freshly prepared STF medium used consisted of several salts, i.e.,; sodium chloride 0.65 g, sodium bicarbonate 0.25 g, calcium chloride dehydrate 0.009 g with 100 mL of water at a simulating eye temperature of 34ºC ± 0.5°C. The fixed amount of all formulations, which was 1 g, was added into the dialysis membrane and sealed. The medium was sampled at the prescribed intervals (0, 5, 1, 2, 4, 8, 10, 12 and 24 h). An equal amount of STF medium was removed from it and added to maintain the volume, and the sinking condition was achieved. The BZD concentration in the retained samples was determined using the High performance liquid chromatography (HPLC) method.
HPLC analysis
The released BZD content was examined using HPLC. Briefly, 25 μL of solvent system was injected into the column before samples were diluted with the same volume. The column used was a C18 column (Zorbax SB, 4.6 mm250 cm, 5 m packing-L1, Agilent). Triethylamine phosphate buffer/acetonitrile/methanol was used as the mobile phase in a ratio of 70/20/10 v/v with a flow rate of 1.5 mL/min. The detector wavelength was 254 nm and the sample run time was approximately 10 min.
Results and discussions
Phase behaviour and physiochemical properties of μΕs
The phase behavior of μE formulations consisting of oil, water and surfactant was studied using the ternary phase diagram as shown in Figure 1. Mapping the pseudoternary phase diagram is important in formulating μEs (Rahman et al., 2017; Saleem et al., 2018). It enables the phase compatibility of the ingredients and the average area of μE formation to be determined. Different ratios of oil, Smix and water were used (Lawrence and Rees, 2012; Subramanian et al., 2005; Rahdar et al., 2018). The behavior of each phase in the μE system was investigated through water dilution as this offers the advantages of fast, accurate and cost-effective operation at room temperature (Takamura et al., 1979). The present study comprised of oil (∼8%), water (∼16%), Tween-80 (∼38%) and 2-propanol (∼38%) as Formulation-A, oil (∼9%), water (∼11%), Tween-20 (∼40%) and 2-propanol (∼40%) as Formulation-B and oil (∼8%), Water (∼12%), Tween-60 (∼40%) and 2-propanol (∼40%) as Formulation-C. Figure 1 represents μE region (shaded area), while AB represent the dilution line and highlighted mark represents the optimal μE selected for further studies on physical stability based on the visual appearance for all formulations. The final formulation contained an excessive proportion of water compared to oil phase. This demonstrates the development of an o/w μE, as supported by previous studies (Saleem et al., 2019; Siddique et al., 2021). Table 1 lists some measured physical parameters of the optimal BZD-free μE and BZD-loaded μE of all μE sets.
Conductivity measurements
Electrical conductivity is convenient tool that determines the type of µE formed and the phase transitions of µE formation from water-in-oil (w/o) to oil-in-water (o/w) through the bicontinuous network channel (Yadav et al., 2018). µEs exhibit a sudden change in electrical conductivity when the composition is varied at a fixed temperature (Kahlweit et al., 1993). Figure 2 represents the changes in electrical conductivity for formulation A, B and C with increasing water content as a function of the weight fraction of the aqueous component (Φw) along the dilution line AB of the oil/Smix. The bicontinuous channel of formulation A starts at ∼16% Φw, which is called the percolation threshold (Φp), below this point the w/o µE at Φw < 16%. At a Φw value of 28%, the abrupt change occurs, indicating the formation of o/w µE with increasing water content and triggering the Φb phase transition (Pal et al., 2017; Nazar et al., 2018). As the Φw value increases, the first derivative (dσ/dφ) becomes even more helpful in determining the µE domain phase transition (Zavgorodnya et al., 2017). At Φw ∼25%, the maximum value of the first derivative was observed, indicating the existence of a stable bicontinuous microstructure in this particular region.
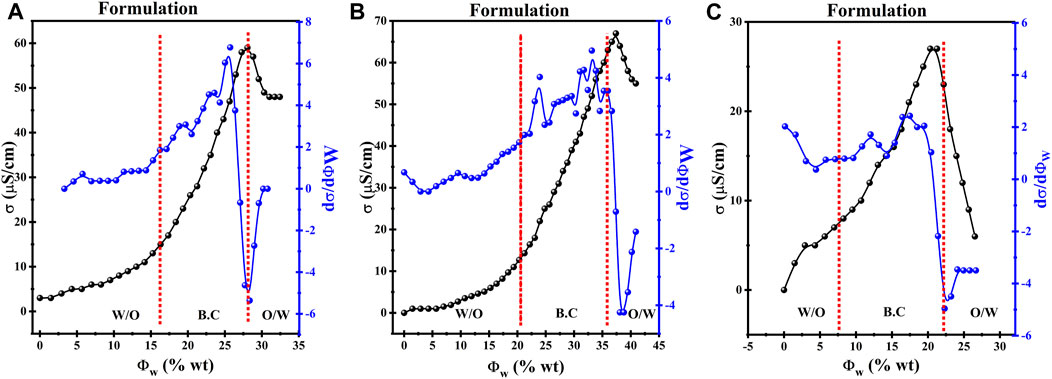
FIGURE 2. Discrepancy of electrical conductivity (σ) and the first derivative of the electric conductivity (do/dΦ) with Φw (wt%) alongside AB dilution line for BZD freeformulation (A–C).
Formulation-B showed the corresponding values of Φw for all suggested transitions in the microstructure are the percolation threshold (Φp ∼ 21%), phase inversion (Φb ∼ 36%), and bicontinuous channel (dσ/dΦ ∼ 30%). Similarly the Formulation-C showed values of Φw for are the percolation threshold (Φp ∼ 7%), phase inversion (Φb ∼ 22%), and bicontinuous channel (dσ/dΦ ∼ 15%).
Optical microscopic studies
An optical microscope was used to visualize the variations in microstructure of μE from w/o to o/w via a bicontinuous network channel. Microstructural transitions in µE were studied under a biological microscope. The expected phase changes present in µE containing w/o, o/w, and bicontinuous network are shown along with the proposed microstructure, also sketched in Figure 3 (Nazar et al., 2020). Dispersed spherical oil and water droplets in their respective continuous phases constructing w/o and o/w μE are shown in Figures 3A, C respectively, while Figure 3B depicts a bicontinuous channel formed by a network of spherical droplets. The results agree well with previously reported observations by researchers (Nazar et al., 2018). The oil-rich composition showed the presence of μE with water droplets dispersed in the continuous oil phase (w/o), as shown in Figure 3A. Conversely, water-rich compositions exhibited μE with oil droplets dispersed in water (o/w), shown in Figure 3C. Between these two extremes, a bicontinuous μE emerged, revealing an aqueous phase-dependent microdomains, as shown in Figure 3B. The distinctive microstructures are influenced by the hydrophilic-lipophilic balance (HLB) value of surfactant and co-surfactant (Paria and Khilar, 2000; Rahdar et al., 2018; Qadri et al., 2022b). When the HLB leans towards the hydrophilic side, a two-layer o/w system is formed in which oil is dispersed in the water phase and vice versa (Khan et al., 2016). For μEs in which neither water nor oil droplets form, the term bicontinuous emerged, signifying the percolation behavior (Dasilva-Carvalhal et al., 2003; Rahman et al., 2016).
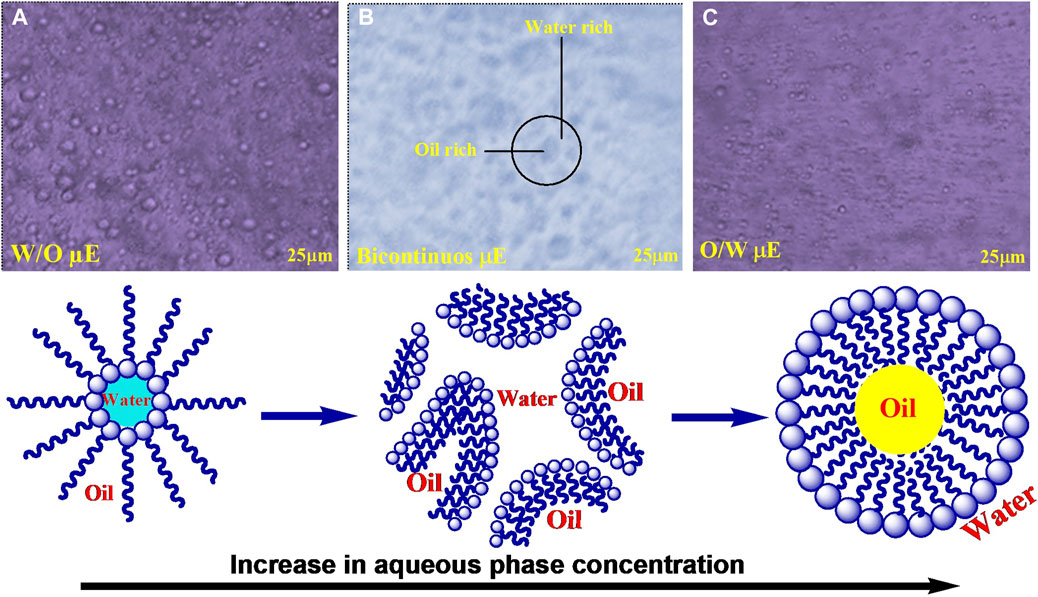
FIGURE 3. Optical micrographs of different types of the phase behavior on dilution of the μE (A) w/o μΕ; (B) bicontinuous μΕ; (C) o/w μΕ; along with the proposed microstructure variation.
Size distribution analysis
Size distribution analysis of BZD-free and BZD-loaded μE of all formulations was performed to check the stability of the dispersed partciles using dynamic light scattering (DLS) (Khan et al., 2016). As shown in Figure 4, the particle size of BZD-free μE droplet was 26.3 ± 1.4, 45.3 ± 2.8 and 36.5 ± 1.8 nm for formulation A, B and C, respectively each represented monostructured droplets by a single peak (Soomro et al., 2016). When BZD was loaded into the μE, the average partcile size increased to 36.2 ± 2.5, 53.5 ± 2.5 and 58.2 ± 2.3 nm for formulation A, B and C respectively, indicating the accumulation and encapsulation of BZD inside the interfacial layers of microstructres (Richard et al., 2017; Xiang et al., 2023). The low polydispersity index (PDI <0.3) indicates uniform homogeneity of the droplet distribution of the μE formulation (Khan et al., 2016). Furthermore, the negative ζ-potential values indicated the high colloidal stability, as the highly stable μE has a ζ-potential value of either >30 mV or < −30 mV due to the electrostatic repulsion between the droplets (Nazar et al., 2017; Pavoni et al., 2020). Other researchers have found and reported similar results (Nazar et al., 2018; Siddique et al., 2024). The values of diffusion coefficients D) are listed in Table 1, which were calculated using the Stokes−Einstein relationship (
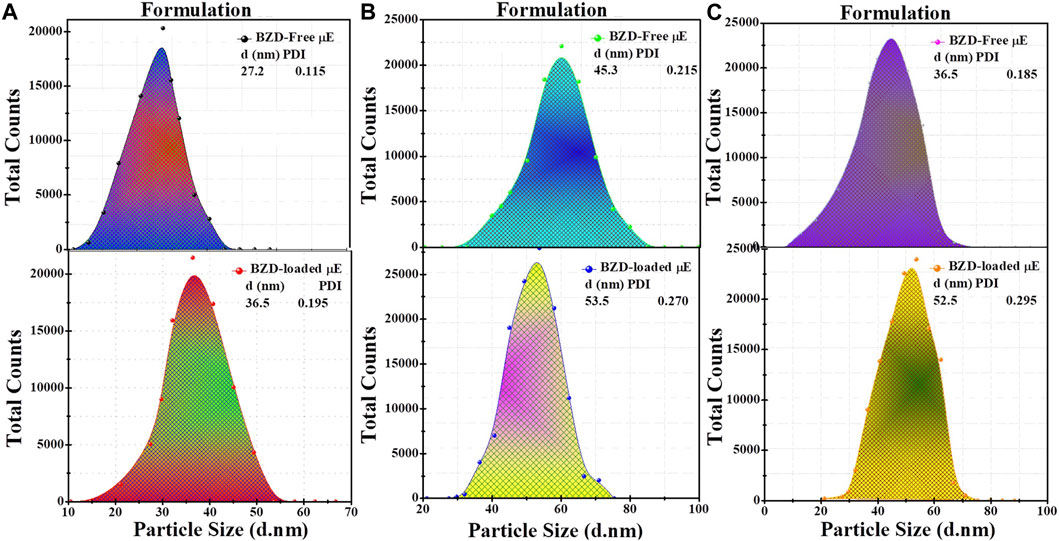
FIGURE 4. Particle size distribution of BZD-free μE, BZD-loaded μE of formulation (A–C) respectively analyzed by DLS.
FTIR analysis
FTIR analysis was performed to check the compatibility of BZD with μE excipients. The FTIR spectra of pure drug, BZD-free and BZD-loaded μE of all formulation are shown in Figure 5. In the FTIR spectrum of pure BZD drug, a signal in the range of 3,110–3,350 cm−1 was observed, probably due to N–H stretching vibrations, while a weak peak in the range of 3,100–3,000 cm−1 is attributed to the C–H stretching mode. The peak observed at 1,644 cm−1 was due to the asymmetric and symmetric C–C stretching vibrations, while a strong band at 1,450 cm-1 corresponds to the bending of the C–H bond. The FTIR spectrum of the BZD-loaded μE is completely different from that of the BZD-pure powder; however the spectrum of BZD-free μE and BZD-loaded μE showed no significant change in all formulations, indicating that no interaction with μE excipients could be observed, demonstrating the stability of BZD within the microstructures (Schneider et al., 2011; Dinache et al., 2020).
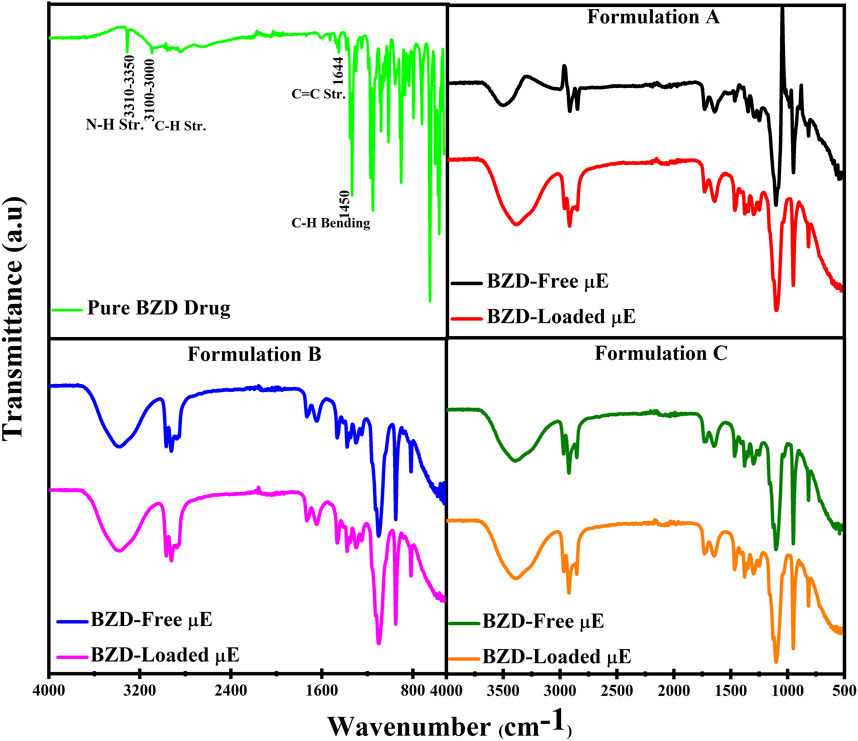
FIGURE 5. FTIR spectra of pure BZD drug, BZD-free μE, BZD-loaded μE, of formulation (A–C) respectively.
The FTIR spectrum of BZD-loaded μE demonstrates that the BZD is completely dissolved in μE and the peak-to-peak correlation showed that there are no larger aggregates due to the absence of any additional peak (Nazar et al., 2009). However, some slight changes in intensities are observed, which are most likely due to the different molecular environments experienced by BZD in different μE formulations due to the weak physical interactions such as hydrogen bonding or intermolecular forces between the functional groups of the BZD and excipients (Saleem et al., 2019). However, these connections may facilitate sustained release of the BZD.
Fluorescence studies
The steady-state spectrophotometry is widley used to measure the partioning of the drug in microdomains of the μE as it depends on the polarity of medium provided (Lissi et al., 2000; Pal et al., 2011). The emission spectra of BZD in water, IPM, Smix of all formulations are depicted in Figure 6. The maximum emission (λem) of BZD in water was observed at 345 nm, while in IPM it was at 360 nm. The λem of BZD in Smix A, B and C were at 422 nm, 456 nm and 465 nm, while the λem of optimal μE-A, μE-B and μE-C was at 408 nm, 406 nm and 404 nm. A red shift was observed in Smix and optimal μE formulations as compared to water. These results suggests that BZD molecules are firmly contained to the non-polar portion of the interface and are shielded by any pure bulk domain, such as water or oil (Pal et al., 2011). All of these results indicate that micro-aggregate-assembled probes may be found close to the μE interface, where excitation propagation was inhibited and additive rotation is further limited (Nazar et al., 2020; Saleem et al., 2020).
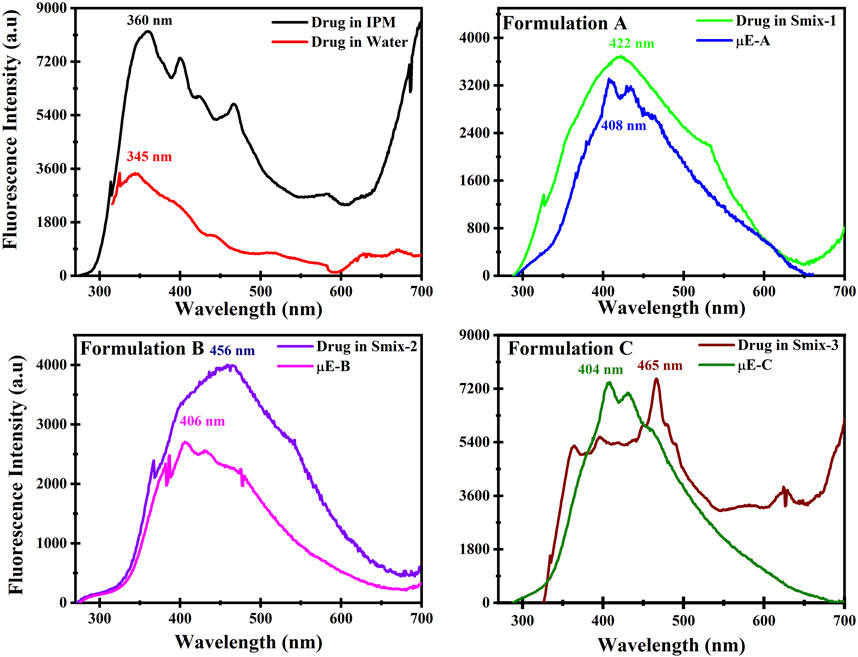
FIGURE 6. Fluorescence spectrum of drug in isopropyl myristate (IPM), drug in water, drug in Smix and in optimal μE formulations (A–C) respectively.
In-Vitro drug release study
New formulations are created and their efficacy is examined in term of in-vitro release also an effort to reduce the use of animal models (Bachhav and Patravale, 2009; Yasir Siddique et al., 2021). The in-vitro release of BZD from μE was assessed by STF as a release medium that simulated the ocular environment after several interval of time (Wu et al., 2013). The release of BZD was found >99.9% of formulation A in interval of 10 h, while the interval for the formulation B and C was 4 and 7 h demonstrating faster release than formulation A owing various factors, such as viscosity, surfactant, oil phase and drug solubility which can affect the release rate (Siafaka et al., 2015). 2-Propanol tends to lower the surface tension of the surfactant ultimately enhances the drug release as it makes the surface of droplet smooth and dynamic (Figueiredo et al., 2016). In Figure 7, the release rate of BZD from all three optimal μE has shown different BZD release at different time due to change in surfactant. In start, all μE showed a slow release of BZD until 5 h. After that a sudden increase in release rate has been observed (Siafaka et al., 2021).
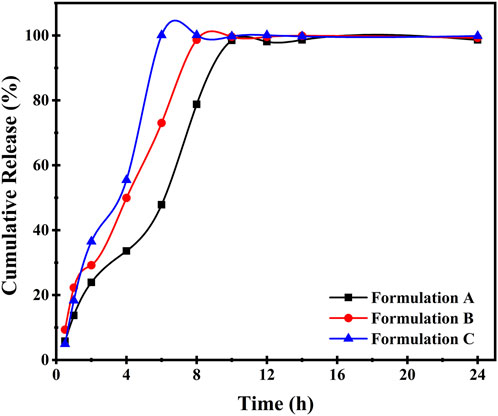
FIGURE 7. In vitro release studies of prepared μE containing BZD of Formulation (A–C) during 0–24 h.
Formulation A showed ∼35% BZD release at 4 h and rest of the 60% get released round about 10 h. In formulation B, 50% BZD was released at 4 h and rest of the BZD was released at 8 h, similar trend was followed by formulations C. Additionally, formulation A showed a sustained release in 10 h among all 3 μE formulation indicating lower dosage quantity because of sustained release.
Conclusion
Three new formulations were made which were consist of formulation A of Tween-80, 2-propanol, IPM and water, formulation B of Tween-20, 2-propanol, IPM and water and formulation C of Tween-60, 2-propanol, IPM and water to enhance the solubility and bioavailability of the antihypertensive drug BZD. Transformation of phases in μE was studied through electrical conductivity, microscopic images and viscosity measurements. To check the stability and presence of BZD fluorescence and DLS study was done. The findings show that BZD-loaded μE with low and desirable thin globule sizes were successfully generated. Evidently, the developed optimized microemulsions can be used as ocular carriers for glaucoma therapy; nevertheless, formulation A, which contained Tween-80/2-propanol as the surfactant, cosurfactant, IPM as the oil phase, was the most ideal of all. In actuality, formulation A offers superior physicochemical values and delayed release, which are both noteworthy benefits. Traditional topical treatments can be expensive, but this new technology may offer some benefits, making it more affordable and sustainable in the long term.
Data availability statement
The original contributions presented in the study are included in the article/Supplementary Material, further inquiries can be directed to the corresponding authors.
Author contributions
SZ: Conceptualization, Formal Analysis, Investigation, Methodology, Software, Writing–original draft. MN: Conceptualization, Funding acquisition, Project administration, Supervision, Writing–original draft, Writing–review and editing. MS: Formal Analysis, Methodology, Software, Writing–review and editing. SH: Data curation, Formal Analysis, Methodology, Writing–review and editing. KA: Formal Analysis, Methodology, Writing–review and editing. MS: Formal Analysis, Methodology, Software, Writing–review and editing. SS: Formal Analysis, Writing–review and editing. HA: Formal Analysis, Software, Writing–review and editing. ZU: Formal Analysis, Funding acquisition, Software, Supervision, Writing–review and editing.
Funding
The author(s) declare that financial support was received for the research, authorship, and/or publication of this article. The research work was supported by project #RSP 2024R399, King Saud University, Riyadh, Saudi Arabia.
Acknowledgments
The author, MN, express their gratitude to the Higher Education Commission of Pakistan for providing financial support through NRPU Project No. 20-17321/NRPU/R&D/HEC/2021. The authors sincerely appreciate funding from Researchers Supporting Project number (RSP 2024R399), King Saud University, Riyadh, Saudi Arabia. Furthermore, the authors express their appreciation to the Department of Chemistry, University of Education, Multan Campus, Pakistan and the Department of Chemical Engineering, King Saud University, Saudi Arabia for providing laboratory facilities to conduct their research.
Conflict of interest
The authors declare that the research was conducted in the absence of any commercial or financial relationships that could be construed as a potential conflict of interest.
The author(s) declared that they were an editorial board member of Frontiers, at the time of submission. This had no impact on the peer review process and the final decision.
Publisher’s note
All claims expressed in this article are solely those of the authors and do not necessarily represent those of their affiliated organizations, or those of the publisher, the editors and the reviewers. Any product that may be evaluated in this article, or claim that may be made by its manufacturer, is not guaranteed or endorsed by the publisher.
Supplementary material
The Supplementary Material for this article can be found online at: https://www.frontiersin.org/articles/10.3389/fmats.2024.1363138/full#supplementary-material
References
Abd El Wahab, L. M., Essa, E. A., El Maghraby, G. M., and Arafa, M. F. (2022). The development and evaluation of phase transition microemulsion for ocular delivery of acetazolamide for glaucoma treatment. AAPS PharmSciTech 24 (1), 1. doi:10.1208/s12249-022-02459-7
Bachhav, Y. G., and Patravale, V. B. (2009). Microemulsion based vaginal gel of fluconazole: formulation, in vitro and in vivo evaluation. Int. J. Pharm. 365 (1), 175–179. doi:10.1016/j.ijpharm.2008.08.021
Choradiya, B. R., and Patil, S. B. (2022). Design, development, and characterization of brinzolamide and brimonidine tartrate nanoemulsion for ophthalmic drug delivery. Thai J. Pharm. Sci. 46 (4), 413–424.
Constantinides, P. P. (1995). Lipid microemulsions for improving drug dissolution and oral absorption: physical and biopharmaceutical aspects. Pharm. Res. 12, 1561–1572. doi:10.1023/a:1016268311867
Danielsson, I. (1981). The definition of microemulsion. Colloids Surfaces 3 (4), 391–392. doi:10.1016/0166-6622(81)80064-9
Dasilva-Carvalhal, J., García-Río, L., Gómez-Díaz, D., Mejuto, J. C., and Rodríguez-Dafonte, P. (2003). Influence of crown ethers on the electric percolation of AOT/isooctane/water (w/o) microemulsions. Langmuir 19 (15), 5975–5983. doi:10.1021/la026857m
Dinache, A., Tozar, T., Smarandache, A., Andrei, I. R., Nistorescu, S., Nastasa, V., et al. (2020). Spectroscopic characterization of emulsions generated with a new laser-assisted device. Molecules 25 (7), 1729. doi:10.3390/molecules25071729
Eissa, M. (2016). Validated spectrophotometric methods for simultaneous determination of brinzolamide and timolol maleate in their pure form and ophthalmic preparation. Al-Azhar J. Pharm. Sci. 54 (1), 188–202. doi:10.21608/ajps.2018.6643
Erdal, M. S., Özhan, G., Mat, C., Özsoy, Y., and Güngör, S. (2016). Colloidal nanocarriers for the enhanced cutaneous delivery of naftifine: characterization studies and in vitro and in vivo evaluations. Int. J. nanomedicine 11, 1027–1037. doi:10.2147/ijn.s96243
Figueiredo, K. A., Neves, J. K. O., Silva, J. A. d., Freitas, R. M. d., and Carvalho, A. L. M. (2016). Phenobarbital loaded microemulsion: development, kinetic release and quality control. Braz. J. Pharm. Sci. 52, 251–264. doi:10.1590/s1984-82502016000200003
Gasco, M. (1997). Microemulsions in the pharmaceutical field: perspectives and applications. Surfactant Sci. Ser. 66, 97–122.
Gohil, R., (2020). Optimization of brinzolamide loaded microemulsion using formulation by design approach: characterization and in-vitro evaluation. Curr. Drug Ther. 15 (1), 37–52. doi:10.2174/22123903otu1zotc6tcvy
Grampurohit, N., Ravikumar, P., and Mallya, R. (2011). Microemulsions for topical use–a review. Ind. J. Pharm. Edu Res. 45 (1), 100–107.
Kahlweit, M., Busse, G., and Winkler, J. (1993). Electric conductivity in microemulsions. J. Chem. Phys. 99 (7), 5605–5614. doi:10.1063/1.465953
Kantaria, S., Rees, G. D., and Lawrence, M. J. (2003). Formulation of electrically conducting microemulsion-based organogels. Int. J. Pharm. 250 (1), 65–83. doi:10.1016/s0378-5173(02)00491-x
Khan, M. F., Singh, M. K., and Sen, S. (2016). Measuring size, size distribution, and polydispersity of water-in-oil microemulsion droplets using fluorescence correlation spectroscopy: comparison to dynamic light scattering. J. Phys. Chem. B 120 (5), 1008–1020. doi:10.1021/acs.jpcb.5b09920
Lawrence, M. J., and Rees, G. D. (2012). Microemulsion-based media as novel drug delivery systems. Adv. drug Deliv. Rev. 64, 175–193. doi:10.1016/j.addr.2012.09.018
Lissi, E., Abuin, E. B., Rubio, M. A., and Cerón, A. (2000). Fluorescence of Prodan and Laurdan in AOT/heptane/water microemulsions: partitioning of the probes and characterization of microenvironments. Langmuir 16 (1), 178–181. doi:10.1021/la990720n
Loiselle, A. R., de Kleine, E., van Dijk, P., and Jansonius, N. M. (2020). Intraocular and intracranial pressure in glaucoma patients taking acetazolamide. PLoS One 15 (6), e0234690. doi:10.1371/journal.pone.0234690
Nakamura, Y., Mochida, A., Choyke, P. L., and Kobayashi, H. (2016). Nanodrug delivery: is the enhanced permeability and retention effect sufficient for curing cancer? Bioconjugate Chem. 27 (10), 2225–2238. doi:10.1021/acs.bioconjchem.6b00437
Narang, A. S., Delmarre, D., and Gao, D. (2007). Stable drug encapsulation in micelles and microemulsions. Int. J. Pharm. 345 (1-2), 9–25. doi:10.1016/j.ijpharm.2007.08.057
Nazar, M. F., Khan, A. M., and Shah, S. S. (2009). Microemulsion system with improved loading of piroxicam: a study of microstructure. AAPS PharmSciTech 10 (4), 1286. doi:10.1208/s12249-009-9328-9
Nazar, M. F., Mujeed, A., Siddique, M. Y., Zafar, M., Saleem, M. A., Khan, A. M., et al. (2020). Structural dynamics of tween-based microemulsions for antimuscarinic drug mirabegron. Colloid Polym. Sci. 298 (3), 263–271. doi:10.1007/s00396-020-04603-w
Nazar, M. F., Saleem, M. A., Bajwa, S. N., Yameen, B., Ashfaq, M., Zafar, M. N., et al. (2017). Encapsulation of antibiotic levofloxacin in biocompatible microemulsion formulation: insights from microstructure analysis. J. Phys. Chem. B 121 (2), 437–443. doi:10.1021/acs.jpcb.6b09326
Nazar, M. F., Yasir Siddique, M., Saleem, M. A., Zafar, M., Nawaz, F., Ashfaq, M., et al. (2018). Fourth-generation antibiotic gatifloxacin encapsulated by microemulsions: structural and probing dynamics. Langmuir 34 (36), 10603–10612. doi:10.1021/acs.langmuir.8b01775
Pal, N., Saxena, N., and Mandal, A. (2017). Phase behavior, solubilization, and phase transition of a microemulsion system stabilized by a novel surfactant synthesized from castor oil. J. Chem. Eng. Data 62 (4), 1278–1291. doi:10.1021/acs.jced.6b00806
Pal, N., Verma, S. D., Singh, M. K., and Sen, S. (2011). Fluorescence correlation spectroscopy: an efficient tool for measuring size, size-distribution and polydispersity of microemulsion droplets in solution. Anal. Chem. 83 (20), 7736–7744. doi:10.1021/ac2012637
Paria, S., and Khilar, K. (2000). Microemulsion-based media as novel drug delivery systems. Adv. Drug Deliv. Rev. 45, 89–121. doi:10.1016/s0169-409x(00)00103-4
Patel, A., (2013). Ocular drug delivery systems: an overview. World J. Pharmacol. 2 (2), 47. doi:10.5497/wjp.v2.i2.47
Pavoni, L., Perinelli, D. R., Bonacucina, G., Cespi, M., and Palmieri, G. F. (2020). An overview of micro- and nanoemulsions as vehicles for essential oils: formulation, preparation and stability. Nanomaterials 10 (1), 135. doi:10.3390/nano10010135
Qadri, H. K., Shaheen, A., Rashid, S., Ahmad Bhat, I., Mohammad Rather, G., and Ahmad Dar, A. (2022a). Micellization and gelation characteristics of Pluronic P123 and single ester-bonded cleavable cationic gemini surfactant: a potential system for solubilization and release of ibuprofen. J. Mol. Liq. 366, 120311. doi:10.1016/j.molliq.2022.120311
Qadri, H. K., Shaheen, A., Rashid, S., Ahmad Bhat, I., Mohammad Rather, G., and Ahmad Dar, A. (2022b). Micellization and gelation characteristics of Pluronic P123 and single ester-bonded cleavable cationic gemini surfactant: a potential system for solubilization and release of ibuprofen. J. Mol. Liq. 366, 120311. doi:10.1016/j.molliq.2022.120311
Rahdar, A., Almasi-Kashi, M., Khan, A. M., Aliahmad, M., Salimi, A., Guettari, M., et al. (2018). Effect of ion exchange in NaAOT surfactant on droplet size and location of dye within Rhodamine B (RhB)-containing microemulsion at low dye concentration. J. Mol. Liq. 252, 506–513. doi:10.1016/j.molliq.2018.01.004
Rahman, A., Rahman, M. M., Mollah, M. Y. A., and Susan, M. A. B. H. (2016). Dynamic percolation and swollen behavior of nanodroplets in 1-ethyl-3-methylimidazolium trifluoromethanesulfonate/triton X-100/cyclohexane microemulsions. J. Phys. Chem. B 120 (28), 6995–7002. doi:10.1021/acs.jpcb.6b04763
Rahman, H. M. A. U., Afzal, S., Nazar, M. F., Alvi, D. A., Khan, A. M., and Asghar, M. N. (2017). Phase behavior of a TX-100/oleic acid/water based ternary system: a microstructure study. J. Mol. Liq. 230, 15–19. doi:10.1016/j.molliq.2017.01.011
Richard, B., Lemyre, J.-L., and Ritcey, A. M. (2017). Nanoparticle size control in microemulsion synthesis. Langmuir 33 (19), 4748–4757. doi:10.1021/acs.langmuir.7b00773
Saleem, M., Nazar, M. F., Yameen, B., Khan, A. M., Hussain, S. Z., and Khalid, M. R. (2018). Structural insights into the microemulsion-mediated formation of fluoroquinolone nanoantibiotics. ChemistrySelect 3, 11616–11621. doi:10.1002/slct.201801925
Saleem, M. A., (2023). “Chapter 11 - self-nanoemulsifying drug delivery systems with bioavailability potential,” in Novel platforms for drug delivery applications. Editors S. Das, S. Thomas, and P. P. Das (Woodhead Publishing, England, UK), 257–275.
Saleem, M. A., Yasir Siddique, M., Nazar, M. F., Khan, S. U. D., Ahmad, A., Khan, R., et al. (2020). Formation of antihyperlipidemic nano-ezetimibe from volatile microemulsion template for enhanced dissolution profile. Langmuir 36 (27), 7908–7915. doi:10.1021/acs.langmuir.0c01016
Saleem, M. N., Nazar, M. F., Siddique, M. Y., Khan, A., Ashfaq, M., Hussain, S. Z., et al. (2019). Soft-templated fabrication of antihypertensive nano-Irbesartan: structural and dissolution evaluation. J. Mol. Liq. 292, 111388. doi:10.1016/j.molliq.2019.111388
Schneider, C., Hanisch, M., Wedel, B., Jusufi, A., and Ballauff, M. (2011). Experimental study of electrostatically stabilized colloidal particles: colloidal stability and charge reversal. J. Colloid Interface Sci. 358 (1), 62–67. doi:10.1016/j.jcis.2011.02.039
Scriven, L. E. (1976). Equilibrium bicontinuous structure. Nature 263 (5573), 123–125. doi:10.1038/263123a0
Siafaka, P. I., Çağlar, E. Ş., Sipahi, H., Charehsaz, M., Aydın, A., and Üstündağ Okur, N. (2021). Ocular microemulsion of brinzolamide: formulation, physicochemical characterization, and in vitro irritation studies based on EpiOcular™ eye irritation assay. Pharm. Dev. Technol. 26 (7), 765–778. doi:10.1080/10837450.2021.1944206
Siafaka, P. I., Titopoulou, A., Koukaras, E. N., Kostoglou, M., Koutris, E., Karavas, E., et al. (2015). Chitosan derivatives as effective nanocarriers for ocular release of timolol drug. Int. J. Pharm. 495 (1), 249–264. doi:10.1016/j.ijpharm.2015.08.100
Siddique, M. Y., Alamgir, I., Nazar, M. F., Sumrra, S. H., Ashfaq, M., Safdar, M., et al. (2021). Structural and probing dynamics of Brij-35-based microemulsion for fluoroquinolone antibiotics. Colloid Polym. Sci. 299 (9), 1479–1488. doi:10.1007/s00396-021-04871-0
Siddique, M. Y., Nazar, M. F., Saleem, M. A., Haider, S., Sumrra, S. H., Akhtar, M. S., et al. (2024). Formulation of gelled microemulsion for effective permeation of celecoxib across the skin barrier. ChemistrySelect 9 (3), e202302841. doi:10.1002/slct.202302841
Silver, L. H., and Group, B. C. S. (2000). Ocular comfort of brinzolamide 1.0% ophthalmic suspension compared with dorzolamide 2.0% ophthalmic solution: results from two multicenter comfort studies. Surv. Ophthalmol. 44, S141–S145. doi:10.1016/s0039-6257(99)00111-3
Soomro, R., Memon, N., Bhanger, M. I., and Denizli, A. (2016). Horseradish peroxidase immobilized into organogel-silica composite for transformation of chlorophenols to biodegradable organic acids. Hacettepe J. Biol. Chem. 44 (3), 361–374. doi:10.15671/hjbc.20164420579
Subramanian, N., Ghosal, S. K., Acharya, A., and Moulik, S. P. (2005). Formulation and physicochemical characterization of microemulsion system using isopropyl myristate, medium-chain glyceride, polysorbate 80 and water. Chem. Pharm. Bull. 53 (12), 1530–1535. doi:10.1248/cpb.53.1530
Takamura, A., Minowa, T., Noro, S., and Kubo, T. (1979). Effects of tween and span group emulsifiers on the stability of o/w emulsions. Chem. Pharm. Bull. 27 (12), 2921–2926. doi:10.1248/cpb.27.2921
Tenjarla, S. (1999). Microemulsions: an overview and pharmaceutical applications. Crit. Reviews™ Ther. Drug Carr. Syst. 16 (5), 62. doi:10.1615/critrevtherdrugcarriersyst.v16.i5.20
Urtti, A. (2006). Challenges and obstacles of ocular pharmacokinetics and drug delivery. Adv. drug Deliv. Rev. 58 (11), 1131–1135. doi:10.1016/j.addr.2006.07.027
Wu, W., Li, J., Wu, L., Wang, B., Wang, Z., Xu, Q., et al. (2013). Ophthalmic delivery of brinzolamide by liquid crystalline nanoparticles: in vitro and in vivo evaluation. Aaps Pharmscitech 14, 1063–1071. doi:10.1208/s12249-013-9997-2
Xiang, H., Xu, S., Zhang, W., Li, Y., Zhou, Y., and Miao, X. (2023). Skin permeation of curcumin nanocrystals: effect of particle size, delivery vehicles, and permeation enhancer. Colloids Surfaces B Biointerfaces 224, 113203. doi:10.1016/j.colsurfb.2023.113203
Yadav, V., Jadhav, P., Kanase, K., Bodhe, A., and Dombe, S. (2018). Preparation and evaluation of microemulsion containing antihypertensive drug. Int. J. Appl. Pharm. 10 (5), 138–146. doi:10.22159/ijap.2018v10i5.27415
Yasir Siddique, M., Nazar, M. F., Mahmood, M., Saleem, M. A., Alwadai, N., Almuslem, A. S., et al. (2021). Microemulsified gel formulations for topical delivery of clotrimazole: structural and in vitro evaluation. Langmuir 37 (46), 13767–13777. doi:10.1021/acs.langmuir.1c02590
Yuan, Y., Li, S. m., Mo, F. k., and Zhong, D. f. (2006). Investigation of microemulsion system for transdermal delivery of meloxicam. Int. J. Pharm. 321 (1-2), 117–123. doi:10.1016/j.ijpharm.2006.06.021
Keywords: microemulsion, topical, ocular, monomodal, nanodroplets
Citation: Zafar S, Nazar MF, Siddique MY, Haider S, Alam K, Saleem MA, Shaukat S, Abd Ur Rahman HM and Ullah Z (2024) Formulation and evaluation of ophthalmic microemulsion for enhanced topical administration of brinzolamide. Front. Mater. 11:1363138. doi: 10.3389/fmats.2024.1363138
Received: 29 December 2023; Accepted: 05 February 2024;
Published: 27 February 2024.
Edited by:
Zhiyong Gao, Central South University, ChinaCopyright © 2024 Zafar, Nazar, Siddique, Haider, Alam, Saleem, Shaukat, Abd Ur Rahman and Ullah. This is an open-access article distributed under the terms of the Creative Commons Attribution License (CC BY). The use, distribution or reproduction in other forums is permitted, provided the original author(s) and the copyright owner(s) are credited and that the original publication in this journal is cited, in accordance with accepted academic practice. No use, distribution or reproduction is permitted which does not comply with these terms.
*Correspondence: Muhammad Faizan Nazar, faizan.nazar@ue.edu.pk; Zaka Ullah, zaka.ullah@ue.edu.pk