Biofilm contamination in confined space stations: reduction, coexistence or an opportunity?
- 1DICMaPI, Università di Napoli Federico II, Naples, Italy
- 2CEINGE, Advanced Biotechnologies, Naples, Italy
The prolonged human permanence in confined environments in space, such as in the case of the International Space Station, has inadvertently fostered conditions leading to uncontrolled microbial proliferation on surfaces, known as biofilm. Biofilm presence represents a challenge in critical spacecraft systems, that can lead to contamination issues and systems loss of function due to biofouling phenomena. This scenario is further complicated by microgravity that has a controversial role on biofilm growth and formation. Biocontamination can be a limiting factor in human long-term mission in outer Earth orbit and an economic and health issue on ISS. This study addresses the pressing need for effective antimicrobial strategies against such resilient biofilms in confined environments where the usage of biocidal chemical compounds is strictly controlled due to toxicity dangers. Traditional methods can be complemented by advanced antimicrobial coatings techniques. A promising approach is based on the oxygen plasma as coating platform. The technology can be potentially extended to a wide range of antibiofilm agents (e.g., peptides, bacteriophages, nanoparticles, quorum sensing disrupting agents, etc.) and substrates (e.g., metal, plastic, ceramic) showing an exceptional flexibility. An alternative vision of the biofilm challenge can be inspired by the dual nature of biofilms, addressed as “good” or “bad” depending on the specific application. Indeed, biofilm have a great potential in closed systems as small space habitat (e.g., ISS) that can be inspired by their role in “large space habitat” as planet Earth itself. The replication of such a complex biological equilibrium is an open challenge.
1 Introduction
Biofilms are aggregates of microorganisms that proliferate at interfaces by self-synthesizing extracellular polymeric substances (EPS) (Hall-Stoodley et al., 2004). Biofilms formation is ubiquitarians constituting a risk due to microbial pathogen presence in food related facilities, drinking water systems colonization, and medical devices and implants contamination (Novikova, 2004a; Hall-Stoodley et al., 2004; Høiby et al., 2015). Moreover, biofilm is a resilient structure due to its enhanced resistance to chemical compound employed for surfaces detergency, but also for its active role in microbial resistance against antibiotics (Penesyan et al., 2020).
Microbial contamination on solid surfaces can significantly compromise the lifespan and functionality of industrial equipment, including heat exchangers, air, and water recycling systems (Stoodley et al., 1999; Zea et al., 2018). Notably, the presence of biofilms has been detected aboard the International Space Station (ISS) (Venkateswaran et al., 2014; Zea et al., 2018; Zea et al., 2020; Marra et al., 2023a), highlighting the challenges of equipment maintenance in space and emphasizing broader concerns in human space exploration. Prolonged habitation in the confined environments of spacecraft, such as the ISS, inadvertently creates ideal conditions for microbial growth (Coil et al., 2016), posing a significant risk to both equipment and human health. This situation is exacerbated by the unique microgravity conditions, combined with the presence of atmospheric moisture and nutrients in habitable pressurized cabins (Klaus et al., 1997; Kim et al., 2013; Venkateswaran et al., 2014). Such environmental conditions prove to be ideal for biofilm formation leading to critical spacecraft systems loss of functions. In general biofilm can develop at different types of interfaces (liquid-air, solid-air or solid-liquid). Liquid-air interfaces require the presence of free liquid surfaces, not often encountered, unless explicitly needed, and are typically easily removed together with the contaminated liquid. In HEPA filters, solid-air interfaces can be colonized by bacterial and fungi, as reported on Mir orbital station (Checinska et al., 2015). These are called airborne biofilm. Diverse species of microorganisms have been identified in the aforementioned environments with prevalent genera including Staphylococcus sp., Corynebacterium sp., and Bacillus species. Airborne fungi also were isolated, with Penicillium and Aspergillus as the dominant genera. Opportunistic pathogenic bacteria species were also isolated, including Flavobacterium meningosepticum, Pseudomonas aeruginosa, Escherichia coli, Klebsiella pneumoniae, Staphylococcus sp (Novikova, 2004b). Regrettably, certain of these species have been linked to infectious diseases affecting the respiratory and digestive systems.
A recurring type of biofilm contamination is the one occurring at solid-liquid interface. An example is the wastewater collection reservoirs unit, also known as the Water Process Assembly (WPA) a part of the Water Recovery System (WRS). This component was fouled by biofilm growth in several occasions, and replacement material were furnished by Earth to solve this problem (Weir et al., 2012). Most common microbial organisms isolated from this biomass are Ralstonia picketii, Bulkholderia sp. and Cupriavidus metallidurans (Zea et al., 2020). The WRS is one of the so-called Environmental Control and Life Support System (ECLSS), a network of facility dedicated to astronauts’ life support abroad the ISS. The ECLSS is necessary for water recycling, thermal control mechanisms, and waste management. One of the few advantages of confined environments is the ability to control physical conditions of wastewater, such as temperature, pH, and ionic strength, or at least be aware of those parameters (Flemming, 2011). In the case of biofilm management, the right combination of these conditions can either promote or inhibit biofilm growth. However, the control of these conditions is limited by the need for compatibility with human life. Additionally, the use of chemical compounds to control biofilm is restricted by concerns about human toxicity. Currently, on the ISS, biocontrol is achieved by adding iodine to drinking water (Wang et al., 2021); however, the long-term effects on astronaut health are a concern, and alternative approaches to reduce toxicity are currently under investigation and discussed in this perspective.
This rise in microbial colonization and the emergence of antibiotic-resistant pathogens aboard spacecraft have profoundly shifted our understanding of microbial behavior in the realm of space exploration. This shift underscores an urgent need to develop novel and effective antimicrobial strategies, particularly considering the altered immune responses of astronauts in-flight, which heightens their susceptibility to infectious diseases (Siems et al., 2021).
Microgravity conditions further complicate this scenario. It is still unclear its role in biofilm enhanced abroad as it tends to enhance microbial activity and biofilm robustness, increasing the risk of microbial-induced corrosion and biofouling and compromising long-term space missions (Benoit and Klaus, 2007; Horneck et al., 2010). Moreover, recent works highlight how biofilm spatial organization heavily affects bacterial gene expression, suggesting a hidden role of gravity (Sanchez-Vizuete et al., 2021; Dergham et al., 2023; Flores et al., 2023).
Addressing these challenges calls for a spectrum of new antimicrobial strategies aimed to prevent as long as possible biofilm formation (Flemming, 2020). While traditional methods like biocides, ionizing radiation, and biofilm detachment techniques have been employed, their effectiveness is often limited against the resilient nature of biofilms, especially in confined environment where only few substances categories can be employed (Wang et al., 2021). This limitation has spurred the exploration of more innovative approaches, particularly in the development of advanced antimicrobial coatings. These coatings are increasingly seen as promising solutions due to their diverse mechanisms of action, including anti-adhesion/microbe-repelling, antimicrobial agent release, and contact-killing properties (Campoccia et al., 2013; Wang et al., 2021; Yazdani-Ahmadabadi et al., 2022).
Recent advancements in this domain have been groundbreaking, particularly the integration of metal ions and nanoparticles into these coatings (Marra et al., 2023b). These elements target essential microbial structures and processes, enhancing the coatings’ antimicrobial efficacy. Moreover, the generation of reactive oxygen species through photoexcitation has emerged as a potent method for combating a broad spectrum of microorganisms, adding another layer of defense against microbial threats.
Despite these advancements, a significant technological gap remains in the scale-up from lab-scale to specific testing on real situations, particularly in space environments. The integration of these advanced antimicrobial coatings, is still poorly tested abroad ISS (Wang et al., 2021), and the synergistic implementation alongside other sterilization technologies, can significantly improve biocontamination management. However, to fill this gap, the need for further research focused on the space field is mandatory. As human ventures into space continue to advance, the evolution and adaptation of antimicrobial strategies will play a crucial role in safeguarding astronaut health and ensuring the integrity of spacecraft systems under these challenging conditions.
2 “Promising coating” technologies: nanoparticle and peptides
Recent advancements in nano-coatings methodologies improved the efficacy of antimicrobial and antibiofilm agents against biofilm growth on surfaces (Dhandapani et al., 2012; Lim et al., 2015; Marra et al., 2023b). Biofilm growth is summarized in five key steps illustrated at the top of Figure 1.
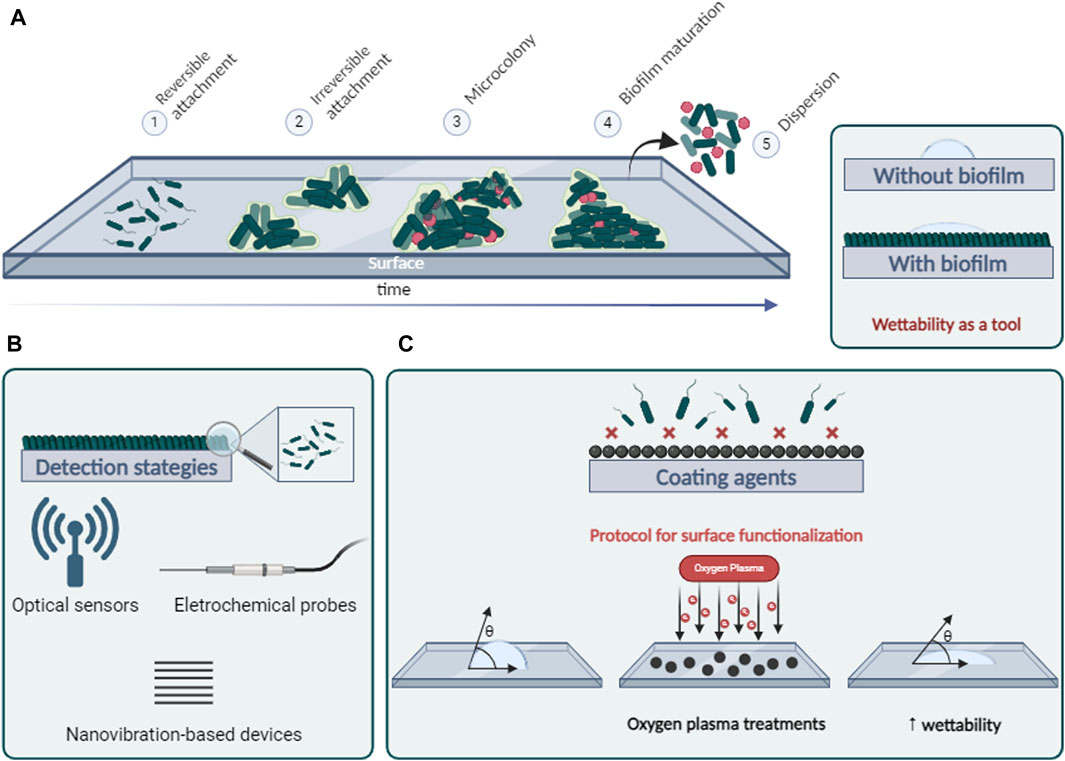
Figure 1. (A) Stages of biofilm development on the surface over time (from attachment to dispersion, labeled 1 to 5, respectively); (B) Detection strategies for the produced biofilm, such as optical sensors, electrochemical probes, and nanovibration-based devices; (C) Coating agents, such as peptides, bacteriophages, nanoparticles, quorum sensing disrupting agents, and so on, combined with oxygen plasma used for surface functionalization. Plasma treatment, where the surface is placed in a vacuum chamber and bombarded by ionized gas, is a safe and effective technique for increasing wettability without producing harmful gases or high temperatures. Created with BioRender.com.
A primary approach is based on preventing bacterial adhesion on surfaces, delaying the first step of biofilm formation (Speziale et al., 2009; Gorth et al., 2012; Campoccia et al., 2013; Wang et al., 2021). A promising work following this approach demonstrated an antiadhesion properties of lubricant impregnated surfaces abroad the ISS (Flores et al., 2023). Another complementary or synergistic route is the implementation of bacterial-killing agents to prevent microbial proliferation after the first phase of adhesion (Campoccia et al., 2013).
Notable focus is recently placed on metal oxide nanoparticles (MNPs), and in particular on TiO₂ (Alavi et al., 2019; Liu et al., 2019). TiO₂ nanoparticles demonstrate significant photocatalytic activity, generating reactive oxygen species that effectively penetrate and disintegrate biofilm matrices (Jalvo et al., 2017). Iron oxide nanoparticles show best results when coated with biocompatible materials such as dextran (Naha et al., 2019). In parallel to nanoparticle-based strategies, antimicrobial peptides (AMPs) offer a promising alternative for biofilms control (Gaglione et al., 2017; Di Somma et al., 2020; Hancock et al., 2021). Their capacity to penetrate and destabilize biofilms at multiple levels makes them a versatile and effective tool against biofilm-related infections (de la Fuente-Núñez et al., 2012; Lim et al., 2015; Hancock et al., 2021). AMPs disrupt microbial membranes through various mechanisms, which often leads to cell death. Beyond these actions, AMPs can impair intracellular signaling systems, thereby influencing critical biofilm control pathways. Notably, families of AMPs like Cathelicidins (Van Harten et al., 2018) and their derivatives, including LL-37, have shown considerable anti-biofilm activity (Bandurska et al., 2015). These peptides effectively inhibit both the formation and growth of biofilms. Furthermore, peptides derived from amphibians have demonstrated robust anti-biofilm properties, in some instances surpassing the efficacy of conventional antibiotics (Ding et al., 2020). The reason under the interest of both TiO2 and AMPs in space field, is their proven low toxicity towards humans (Warheit et al., 2007; Nordström and Malmsten, 2017). Others unconventional biobased antimicrobial agents can be represented by bacteriophages and phages. Phages and phage-derived products have gained scientists interest as an alternative to antibiotics in preventing and treating biofilms and associated infections (Amankwah et al., 2021). The development of efficient phage-based treatments requires a deeper understanding of specific bacteria strain resistance to phages and the co-evolutionary mechanisms between phages and bacteria. Phage-based treatments for bacterial biofilm destruction includes the use of mono phages, phage cocktails, genetically engineered phages, and phage-derived enzymes (Pires et al., 2017).
Whichever antimicrobial agent is chosen to ensure a stable bond between antimicrobial agent and a desired substrate, the easiest way is to use the right chemical linker (e.g., in the case of peptides, dopamine shows excellent properties (Lim et al., 2015)). However, toxicity and additional costs must be considered, and in the case of confined environment are not negligible. Following this field of view, a promising technique is represented by Cold Plasma (CP) employment (Lieberman and Lichtenberg, 1994; Fridman, 2008; Vandenabeele and Lucas, 2020). CP was already employed as an effective methodology to bond nanoparticles to a variety of surfaces with technological relevance, such as glass (Marra et al., 2023b), and metal (Dong et al., 2019). This innovative method eliminates the need for high temperatures and chemical precursors, thus preserving the integrity of the surfaces. The performance of these nanoparticle coatings is rigorously evaluated through comprehensive wetting analysis (Evgenidis et al., 2017; Zabiegaj et al., 2021; Kampouraki et al., 2022) and biofilm growth assessments, employing techniques like confocal laser scanning microscopy (CLSM) (Di Somma et al., 2020). The results, depicted at the bottom of Figure 1, from these evaluations reveal a dual benefit: a robust contact-killing effect and enhanced anti-adhesion properties. These properties contribute to a significant reduction in both live and dead biofilm biomasses (Recupido et al., 2020). Moreover, the omniphilic behavior observed during the wetting analysis suggests a strong correlation with the coatings’ anti-adhesion capabilities. In the pursuit of employing coatings for space applications, Wang et al. (Wang et al., 2021) conducted a comprehensive review of various coatings suitable for testing within the specified parameters of space environments. Among the most promising candidates is the silver nanocluster–silica composite coating developed by Balagna et al. (Balagna et al., 2012), currently proposed as an antimicrobial coating in the aerospace sector. The efficacy of this coating was evaluated on a commercial polymeric film, utilized in space applications to construct the inner section of inflatable modules that come into contact with crew members. Notably, this coating exhibits inherent air impermeability. Various bacteria (such as Staphylococcus aureus, B. cereus, Morganella, Klebsiella pneumoniae, E. coli) and fungi (Candida parapsilosis, Candida albicans, Candida glabrata) identified on board the ISS and Mir station have exhibited sensitivity to the silver nanocluster–silica composite coating (Zea et al., 2020; Marra et al., 2023a).
Durability tests further demonstrated the coating’s resilience, remaining active for the formation of an inhibition halo and displaying an absence of microorganism growth even after 84 days of air-aging. These findings underscore the potential of coating as a robust and durable solution for antimicrobial protection in space applications. In the pursuit of enhancing the durability of coatings for extended space missions, a particular type of coating warrants consideration: self-healing coatings. Inspired by the regenerative processes observed in living organisms, self-healing materials have the ability to autonomously or non-autonomously repair damage, effectively restoring the original structure and function (Wang et al., 2020). Intrinsic self-healing materials, which are cross-linked by supramolecular non-covalent bonds (e.g., hydrogen bonds, host-guest interactions, ionic interactions, etc.), can repair damaged regions through dynamic recombination of these supramolecular bonds (Shchukin et al., 2006; Chen et al., 2015).
A groundbreaking development in antimicrobial strategies is the synergistic integration of metal nanoparticles (MNPs) and AMPs, leveraging their distinct properties to devise a comprehensive and highly effective treatment approach (Ding et al., 2020). This synergy arises from the complementary mechanisms of MNPs and AMPs, each targeting different facets of bacterial physiology, thereby culminating in an overarching antimicrobial effect. MNPs primarily exert their antibacterial impact by disrupting the structural integrity of bacterial cell membranes and inducing the generation of reactive oxygen species (ROS) (Campoccia et al., 2013). This activity increases the permeability of bacterial cells, rendering them more vulnerable to external agents. Conversely, AMPs engage bacterial cells through various mechanisms, including the direct disruption of cell membranes, inhibition of critical enzymes, and interference with DNA, RNA, and protein synthesis processes (Campoccia et al., 2013; Wang et al., 2021).
Despite those promising techniques must be fully explored, at the best of our knowledge both for Earth and space applications, a definitive antibiofilm coating is far from being realized, and the term “promising” is still too vague.
3 Redefining biofilm challenges in space: from reduction to coexistence strategies
In the ever-evolving field of antimicrobial and antibiofilm research, a significant shift has been observed in the general approach. This evolution intertwines traditional practices with cutting-edge scientific approaches. Given the billions of years over which microorganisms have adapted to a variety of stresses, it becomes evident that completely eradicating them is an unfeasible goal. In our vision, the emphasis should be focused on understanding how to deal with biofilm presence in complex confined environments. In all efforts to combat biofouling (Cirillo et al., 2021), it is crucial to recognize that biofilms have evolved versatile defense mechanisms against various stresses, including those induced by toxic metals, irradiation, antibiotics, and host immune systems. Expecting an easy and enduring victory over biofouling is unrealistic. Rather, a possible and reasonable achievement is to prolong the period during which biofilms do not pose problems with the final goal to obtain a steady state coexistence with these complex biological systems, as indeed it is in natural environment. Any anti-fouling technology is time-dependent and not permanent. The temporal requirements vary, ranging from hours to days for applications like removable catheters and the food, beverage, and pharmaceutical industries, to months and years for systems like desalination plants, membrane systems, steam condensers. Following this point of view in the peculiar case of the ISS or other spacecraft environment, a final solution against biofouling is unachievable. Unfortunately, to the best of our knowledge, no coating, antibiotics, detergent operation can be applied to permanently solve the issue of biofilm growth on surfaces.
Nevertheless, the biofilm’s indomitable resilience should not deter our efforts. As mentioned earlier, biofilm growth is probably impossible to permanently eradicate, but it can be slowed to the extent that it ceases to be a significant concern. In the context of extended space missions, its growth must be decelerated, aiming to minimize the frequency and invasiveness of extraordinary cleaning operations.
Possible approaches to handle biofouling taking into account the peculiarities of space exploration needs should include:
• Redesigning the WPA to withstand organic loads, preventing complete blockage by biofilm. Not all flow systems are susceptible to biofilm; in fact, there are instances of systems successfully coexisting with biofilms (Flemming, 2011). The occurrence of biofouling is strictly dictated by flow conditions. As a general law, an increase in hydrodynamic forces promotes both the attachment of planktonic cells to surfaces, and the detachment of sessile cells from biofilm (Stoodley et al., 1999; Stoodley, 2016; Fanesi et al., 2021). Typically, an enhancement of flow increases disposal of metabolites, delivery of nutrients and oxygen promoting biofilms. However, after a critical value the detachment process overcome the attachment process and the transport phenomena benefits (Stoodley, 2016). The redesign of the WPA should consider these two processes, considering potential organic loads and, critically, the significant unknown factor of the system: microgravity. Further studies are imperative to comprehend how microgravity influences biofilm growth (Marra et al., 2023a).
• The application of specific coatings with antimicrobial and antibiofilm properties aims to manage and delay the growth of microorganisms. The introduction of substances required for coating materials is meticulously regulated aboard the International Space Station (ISS). The unique and confined environment poses considerable challenges. Any chemical compound used must not only be deemed safe to ensure the health and safety of astronauts but also exhibit sufficient effectiveness against bacterial proliferation to obviate the need for periodic reintroduction processes. All coating techniques must adhere to the European Cooperation for Space Standardization’s (ECSS) standards, which govern the materials permitted in confined space stations and specifically address requirements for antimicrobial materials (European Cooperation for Space Standardization, https://ecss.nl/(accessed: December 2020)).
• Detection strategies to identify early stages of biofilm formation on surfaces. Monitoring biofilms formation is a valuable method for timely recognition of fouling layer development and can be crucial for cleaning operation timing, in order to guarantee a good anti-fouling protocol.
An ideal monitoring device should offer fast, accurate, real-time, non-destructive, on-line, and cost-effective information about fouling layer development. To the best of our knowledge this ambitious goal has not been reached, but several techniques have been proposed, including nanovibration-based devices (Salazar et al., 2023) and spectroscopy technique for online biofilm monitoring (Kampouraki et al., 2024), nanoparticles probes for detection purpose (Bazsefidpar et al., 2023).
• Smart data analysis and mathematical model. The implementation of machine learning and/or artificial intelligence in bioinformatics can outclass human ability to manage vast amount of data (Srivastava et al., 2020). This instrument can be especially useful for biofilm growth for long-period of time. In general, in literature there is a huge viability of data regarding biovolume, surface coverage, strains, substrate, and coating implementation on biofilm. However, due to the great biofilm heterogeneity there is a lack of connection and relationship between those features. The implementation of IT tools can potentially lead to mathematicals model useful to predict for example, the fouling phenomena in systems like pipes or tubes. These novel methods can identify crucial parameters to be identify a can be as useful in microgravity conditions as on Earth application. For instance, the complexity of biofilm structures can be compared to other three-dimensional or bi-dimensional biological structures like tumours or skin (Lowengrub et al., 2009; Deisboeck et al., 2011), where mathematical models, such as cellular automata (Migliaccio et al., 2023), have already been successfully employed.
The combination of the aforementioned interdisciplinary approaches would lead to a holistic management of biofilm prevention within a specific field of application. A particular attention should be placed in the case of complex environments such as the ISS, or possible future permanent human colonies on the Moon or Mars, where a major attention should be paid to investigate the role of modified gravity conditions on evolution of complex biological systems. The next steps towards achieving possible coexistence with biofilm should focus on designing specific biofilm sensors to schedule maintenance operations for ECLSS facilities. Future research should also encompass the redesign of liquid and air flow systems to identify the stress conditions range (e.g., wall shear stresses) that trigger biofilm formation. Most of available literature is focused on the study of biofilm in static condition in lab-environments, such as Petri dishes or agarose gels, stressing only the role of biological or chemical stimuli as shaping driving force of biofilm. We suggest to extend this investigation and consider also mechanical stimuli, such as flow or bulk stresses, as sources of microbial three-dimensional organization. In this vision it is important to extend experimental investigation to conditions mimicking biofilm growth in real conditions, such as pipes, tubes and reservoirs, where submerged biofilm is growth typically under the presence of an external flow, on materials such as metals, glass or plastic.
Furthermore, it is imperative for future research to focus on identifying a range of antimicrobial and antibiofilm agents to treat surfaces prone to contamination. When evaluating these coatings, critical considerations should include their low toxicity, durability, and the ability not to cause damage to the substrate on which they are applied.
4 Conclusion
Biofilm in the peculiar conditions typical of confined space habitats represents a potential criticism for long term space exploration. A full comprehension of the role of external stresses on biofilm formation is still not available, new approaches are now being used to investigate the role of anisotropic and bulk stresses, such as gravity, on bacteria. It is now evident that a complete eradication of biofilm is not feasible. Moreover, even its reduction poses significant complexities, given the resilience of these microbial communities and the unique constraints of space environments. This understanding necessitates a paradigm shift in our approach to space biofouling: from attempting total elimination to seeking innovative ways of coexistence that minimize risks to human health. In this perspective, we have emphasized potential guidelines to mitigate biofilm formation, recognizing its presence as a dual health and technical risk in confined environments. This consideration is particularly applicable to what is often referred to as ‘bad’ biofilms as counterposed to ‘good’ ones where biofilms are used as a biotechnological tool for applications such as bioreactors or bioremediation. In particular, the concept of “good” biofilms finds practical application in the recent Biorock project (Cockell et al., 2021). Biorock, is a European Space Agency (ESA) project conducted aboard the ISS, that explores the feasibility of large-scale biomining in space. The study focuses on the bioleaching of vanadium from basalt rock, showcasing the advantageous aspects of utilizing biofilms in space (Sheoran et al., 2009). This approach has as aim the utilization of in-situ resources to support space exploration in extraterrestrial planet. Strains considered potential candidate for this task are here reported: Acidithiobacillus ferroxidans, Bacillus subtilis, Cupriavidus metallidurans, Shewanella oneidensis, and Sphingomonas desiccabilis (Cockell et al., 2021). Aside from biomining, biofilms can be utilized in space exploration as a part of the life-support systems (Fahrion et al., 2021). For example, bacteria can be involved in the production of methane, needed as fuel for some propulsion systems. Cyanobacterial biofilms have been considered suitable candidates for methane production and subsequent use as fuel (Justiniano et al., 2023).
Nature provides numerous examples where biofilm is crucial for environmental homeostasis, as seen in aquariums (as mentioned earlier). On a long time perspective, it would be good to reproduce in confined space environments this complex equilibrium, giving to biofilm a positive role in the overall biological balance of the system. This coexistence strategy not only seeks to minimize the adverse impacts of biofilm but also explores the prospect of leveraging biofilm as a beneficial tool. By comprehending the intricate dynamics of biofilm formation and its interaction with diverse surfaces and environments, especially in microgravity, we will be able to design systems where biofilms can be harnessed for our advantage. This could have implications for various space applications, including life support systems, waste management, and other critical operations. The knowledge acquired thanks to the space application research will also have sound implications in standard non-space conditions, providing novel approaches that will improve sustainability of human technology on Earth.
In conclusion, the journey of managing biofilm in space is not just about combating a challenge, but also about embracing an opportunity. It invites a broader perspective where biofilm, once seen as a formidable foe, can be transformed into a valuable ally in our quest for sustainable and long-term space exploration. This shift in perspective opens up new avenues for research and innovation, paving the way for more effective and holistic approaches to biofouling management in space.
Data availability statement
The original contributions presented in the study are included in the article/Supplementary material, further inquiries can be directed to the corresponding author.
Author contributions
DM: Conceptualization, Writing–original draft. RF: Visualization, Writing–original draft. SC: Conceptualization, Funding acquisition, Project administration, Supervision, Writing–review and editing.
Funding
The author(s) declare financial support was received for the research, authorship, and/or publication of this article. Financial support was provided from ESA (research projects OSIP IDEA: I-2021-03382) to University of Naples. This study was conducted under the umbrella of the European Space Agency Topical Team: “Biofilms from an interdisciplinary perspective”.
Conflict of interest
The authors declare that the research was conducted in the absence of any commercial or financial relationships that could be construed as a potential conflict of interest.
Publisher’s note
All claims expressed in this article are solely those of the authors and do not necessarily represent those of their affiliated organizations, or those of the publisher, the editors and the reviewers. Any product that may be evaluated in this article, or claim that may be made by its manufacturer, is not guaranteed or endorsed by the publisher.
References
Alavi, M., Karimi, N., and Valadbeigi, T. (2019). Antibacterial, antibiofilm, antiquorum sensing, antimotility, and antioxidant activities of green fabricated Ag, Cu, TiO2, ZnO, and Fe3O4 NPs via protoparmeliopsis muralis lichen aqueous extract against multi-drug-resistant bacteria. ACS Biomaterials Sci. Eng. 5, 4228–4243. doi:10.1021/acsbiomaterials.9b00274
Amankwah, S., Abdella, K., and Kassa, T. (2021). Bacterial biofilm destruction: a focused review on the recent use of phage-based strategies with other antibiofilm agents. Nanotechnol. Sci. Appl. Vol. 14, 161–177. doi:10.2147/nsa.s325594
Balagna, C., Perero, S., Ferraris, S., Miola, M., Fucale, G., Manfredotti, C., et al. (2012). Antibacterial coating on polymer for space application. Mater. Chem. Phys. 135, 714–722. doi:10.1016/j.matchemphys.2012.05.049
Bandurska, K., Berdowska, A., Barczyńska-Felusiak, R., and Krupa, P. (2015). Unique features of human cathelicidin LL-37. Biofactors 41, 289–300. doi:10.1002/biof.1225
Bazsefidpar, S., Freitas, M., Pereira, C. R., Gutiérrez, G., Serrano-Pertierra, E., Nouws, H. P. A., et al. (2023). Fe3O4@ Au core–shell magnetic nanoparticles for the rapid analysis of E. coli O157: H7 in an electrochemical immunoassay. Biosensors 13, 567. doi:10.3390/bios13050567
Benoit, M. R., and Klaus, D. M. (2007). Microgravity, bacteria, and the influence of motility. Adv. Space Res. 39, 1225–1232. doi:10.1016/j.asr.2006.10.009
Campoccia, D., Montanaro, L., and Arciola, C. R. (2013). A review of the biomaterials technologies for infection-resistant surfaces. Biomaterials 34, 8533–8554. doi:10.1016/j.biomaterials.2013.07.089
Checinska, A., Probst, A. J., Vaishampayan, P., White, J. R., Kumar, D., Stepanov, V. G., et al. (2015). Microbiomes of the dust particles collected from the international space station and spacecraft assembly facilities. Microbiome 3, 50–18. doi:10.1186/s40168-015-0116-3
Chen, D., Wu, M., Li, B., Ren, K., Cheng, Z., Ji, J., et al. (2015). Layer-by-layer-assembled healable antifouling films. Adv. Mater. 27, 5882–5888. doi:10.1002/adma.201501726
Cirillo, A. I., Tomaiuolo, G., and Guido, S. (2021). Membrane fouling phenomena in microfluidic systems: from technical challenges to scientific opportunities. Micromachines 12, 820. doi:10.3390/mi12070820
Cockell, C. S., Santomartino, R., Finster, K., Waajen, A. C., Nicholson, N., Moeller, R., et al. (2021). Microbially-enhanced vanadium mining and bioremediation under micro-and Mars gravity on the International Space Station. Front. Microbiol. 12, 641387. doi:10.3389/fmicb.2021.641387
Coil, D. A., Neches, R. Y., Lang, J. M., Brown, W. E., Severance, M., Cavalier, D., et al. (2016). Growth of 48 built environment bacterial isolates on board the International Space Station (ISS). PeerJ 4, e1842. doi:10.7717/peerj.1842
Deisboeck, T. S., Wang, Z., Macklin, P., and Cristini, V. (2011). Multiscale cancer modeling. Annu. Rev. Biomed. Eng. 13, 127–155. doi:10.1146/annurev-bioeng-071910-124729
de la Fuente-Núñez, C., Korolik, V., Bains, M., Nguyen, U., Breidenstein, E. B. M., Horsman, S., et al. (2012). Inhibition of bacterial biofilm formation and swarming motility by a small synthetic cationic peptide. Antimicrob. agents Chemother. 56, 2696–2704. doi:10.1128/AAC.00064-12
Dergham, Y., Le Coq, D., Nicolas, P., Bidnenko, E., Dérozier, S., Deforet, M., et al. (2023). Direct comparison of spatial transcriptional heterogeneity across diverse Bacillus subtilis biofilm communities. Nat. Commun. 14, 7546. doi:10.1038/s41467-023-43386-w
Dhandapani, P., Maruthamuthu, S., and Rajagopal, G. (2012). Bio-mediated synthesis of TiO2 nanoparticles and its photocatalytic effect on aquatic biofilm. J. Photochem. Photobiol. B Biol. 110, 43–49. doi:10.1016/j.jphotobiol.2012.03.003
Ding, S.-Y., Faraj, Y., Wei, J., Wang, W., Xie, R., Liu, Z., et al. (2020). Antimicrobial peptide-functionalized magnetic nanoparticles for rapid capture and removal of pathogenic bacteria. Microchem. J. 159, 105493. doi:10.1016/j.microc.2020.105493
Di Somma, A., Recupido, F., Cirillo, A., Romano, A., Romanelli, A., Caserta, S., et al. (2020). Antibiofilm properties of temporin-L on Pseudomonas fluorescens in static and in-flow conditions. Int. J. Mol. Sci. 21, 8526. doi:10.3390/ijms21228526
Dong, P., Cheng, X., Jin, Z., Huang, Z., Nie, X., Wang, X., et al. (2019). The green synthesis of Ag-loaded photocatalyst via DBD cold plasma assisted deposition of Ag nanoparticles on N-doped TiO2 nanotubes. J. Photochem. Photobiol. A Chem. 382, 111971. doi:10.1016/j.jphotochem.2019.111971
Evgenidis, S. P., Kalić, K., Kostoglou, M., and Karapantsios, T. D. (2017). Kerberos: a three camera headed centrifugal/tilting device for studying wetting/dewetting under the influence of controlled body forces. Colloids Surfaces A Physicochem. Eng. Aspects 521, 38–48. doi:10.1016/j.colsurfa.2016.07.079
Fahrion, J., Mastroleo, F., Dussap, C.-G., and Leys, N. (2021). Use of photobioreactors in regenerative life support systems for human space exploration. Front. Microbiol. 12, 699525. doi:10.3389/fmicb.2021.699525
Fanesi, A., Lavayssière, M., Breton, C., Bernard, O., Briandet, R., and Lopes, F. (2021). Shear stress affects the architecture and cohesion of Chlorella vulgaris biofilms. Sci. Rep. 11, 4002–4011. doi:10.1038/s41598-021-83523-3
Flemming, H.-C. (2011). Microbial biofouling: unsolved problems, insufficient approaches, and possible solutions. Biofilm highlights, 81–109. doi:10.1007/978-3-642-19940-0_5
Flemming, H.-C. (2020). Biofouling and me: my Stockholm syndrome with biofilms. Water Res. 173, 115576. doi:10.1016/j.watres.2020.115576
Flores, P., McBride, S. A., Galazka, J. M., Varanasi, K. K., and Zea, L. (2023). Biofilm formation of Pseudomonas aeruginosa in spaceflight is minimized on lubricant impregnated surfaces. npj Microgravity 9, 66. doi:10.1038/s41526-023-00316-w
Gaglione, R., Dell'Olmo, E., Bosso, A., Chino, M., Pane, K., Ascione, F., et al. (2017). Novel human bioactive peptides identified in Apolipoprotein B: evaluation of their therapeutic potential. Biochem. Pharmacol. 130, 34–50. doi:10.1016/j.bcp.2017.01.009
Gorth, D. J., Puckett, S., Ercan, B., Webster, T. J., Rahaman, M., and Bal, B. S. (2012). Decreased bacteria activity on Si₃N₄ surfaces compared with PEEK or titanium. Int. J. nanomedicine 7, 4829–4840. doi:10.2147/ijn.s35190
Hall-Stoodley, L., Costerton, J. W., and Stoodley, P. (2004). Bacterial biofilms: from the natural environment to infectious diseases. Nat. Rev. Microbiol. 2, 95–108. doi:10.1038/nrmicro821
Hancock, R. E. W., Alford, M. A., and Haney, E. F. (2021). Antibiofilm activity of host defence peptides: complexity provides opportunities. Nat. Rev. Microbiol. 19, 786–797. doi:10.1038/s41579-021-00585-w
Høiby, N., Bjarnsholt, T., Moser, C., Bassi, G. L., Coenye, T., Donelli, G., et al. (2015). ESCMID guideline for the diagnosis and treatment of biofilm infections 2014. Clin. Microbiol. Infect. 21, S1–S25. doi:10.1016/j.cmi.2014.10.024
Horneck, G., Klaus, D. M., and Mancinelli, R. L. (2010). Space microbiology. Microbiol. Mol. Biol. Rev. 74, 121–156. doi:10.1128/MMBR.00016-09
Jalvo, B., Faraldos, M., Bahamonde, A., and Rosal, R. (2017). Antimicrobial and antibiofilm efficacy of self-cleaning surfaces functionalized by TiO2 photocatalytic nanoparticles against Staphylococcus aureus and Pseudomonas putida. J. Hazard. Mater. 340, 160–170. doi:10.1016/j.jhazmat.2017.07.005
Justiniano, Y.-A. V., Goeres, D. M., Sandvik, E. L., Kjellerup, B. V., Sysoeva, T. A., Harris, J. S., et al. (2023). Mitigation and use of biofilms in space for the benefit of human space exploration. Biofilm 5, 100102. doi:10.1016/j.bioflm.2022.100102
Kampouraki, Z. C., Petala, M., Boumpakis, A., Skordaris, G., Michailidis, N., Deliyanni, E., et al. (2022). Wetting and imbibition characteristics of Pseudomonas fluorescens biofilms grown on stainless steel. Langmuir 38, 9810–9821. doi:10.1021/acs.langmuir.2c00828
Kampouraki, Z. C., Petala, M., Zacharias, K., Konstantinidis, A., Zabulis, X., Karamaounas, P., et al. (2024). Highly sensitive resistance spectroscopy technique for online monitoring of biofilm growth on metallic surfaces. Environ. Res. 240, 117401. doi:10.1016/j.envres.2023.117401
Kim, W., Tengra, F. K., Young, Z., Shong, J., Marchand, N., Chan, H. K., et al. (2013). Spaceflight promotes biofilm formation by Pseudomonas aeruginosa. PLoS One 8, e62437. doi:10.1371/journal.pone.0062437
Klaus, D., Simske, S., Todd, P., and Stodieck, L. (1997). Investigation of space flight effects on Escherichia coli and a proposed model of underlying physical mechanisms. Microbiology 143, 449–455. doi:10.1099/00221287-143-2-449
Lieberman, M. A., and Lichtenberg, A. J. (1994). Principles of plasma discharges and materials processing. MRS Bull. 30, 899–901. doi:10.1557/mrs2005.242
Lim, K., Chua, R. R. Y., Ho, B., Tambyah, P. A., Hadinoto, K., and Leong, S. S. J. (2015). Development of a catheter functionalized by a polydopamine peptide coating with antimicrobial and antibiofilm properties. Acta biomater. 15, 127–138. doi:10.1016/j.actbio.2014.12.015
Liu, Y., Shi, L., Su, L., van der Mei, H. C., Jutte, P. C., Ren, Y., et al. (2019). Nanotechnology-based antimicrobials and delivery systems for biofilm-infection control. Chem. Soc. Rev. 48, 428–446. doi:10.1039/c7cs00807d
Lowengrub, J. S., Frieboes, H. B., Jin, F., Chuang, Y.-L., Li, X., Macklin, P., et al. (2009). Nonlinear modelling of cancer: bridging the gap between cells and tumours. Nonlinearity 23, R1–R91. doi:10.1088/0951-7715/23/1/r01
Marra, D., Karapantsios, T., Caserta, S., Secchi, E., Holynska, M., Labarthe, S., et al. (2023a). Migration of surface-associated microbial communities in spaceflight habitats. Biofilm 5, 100109. doi:10.1016/j.bioflm.2023.100109
Marra, D., Perna, I., Pota, G., Vitiello, G., Pezzella, A., Toscano, G., et al. (2023b). Nanoparticle coatings on glass surfaces to prevent Pseudomonas fluorescens AR 11 biofilm formation. Microorganisms 11, 621. doi:10.3390/microorganisms11030621
Migliaccio, G., Ferraro, R., Wang, Z., Cristini, V., Dogra, P., and Caserta, S. (2023). Exploring cell migration mechanisms in cancer: from wound healing assays to cellular automata models. Cancers 15, 5284. doi:10.3390/cancers15215284
Naha, P. C., Liu, Y., Hwang, G., Huang, Y., Gubara, S., Jonnakuti, V., et al. (2019). Dextran-coated iron oxide nanoparticles as biomimetic catalysts for localized and pH-activated biofilm disruption. ACS nano 13, 4960–4971. doi:10.1021/acsnano.8b08702
Nordström, R., and Malmsten, M. (2017). Delivery systems for antimicrobial peptides. Adv. colloid interface Sci. 242, 17–34. doi:10.1016/j.cis.2017.01.005
Novikova, N. D. (2004a). Review of the knowledge of microbial contamination of the Russian manned spacecraft. Microb. Ecol. 47, 127–132. doi:10.1007/s00248-003-1055-2
Novikova, N. D. (2004b). Review of the knowledge of microbial contamination of the Russian manned spacecraft. Microb. Ecol. 47, 127–132. doi:10.1007/s00248-003-1055-2
Penesyan, A., Paulsen, I. T., Gillings, M. R., Kjelleberg, S., and Manefield, M. J. (2020). Secondary effects of antibiotics on microbial biofilms. Front. Microbiol. 11, 2109. doi:10.3389/fmicb.2020.02109
Pires, D. P., Melo, L. D. R., Boas, D. V., Sillankorva, S., and Azeredo, J. (2017). Phage therapy as an alternative or complementary strategy to prevent and control biofilm-related infections. Curr. Opin. Microbiol. 39, 48–56. doi:10.1016/j.mib.2017.09.004
Recupido, F., Toscano, G., Tatè, R., Petala, M., Caserta, S., Karapantsios, T. D., et al. (2020). The role of flow in bacterial biofilm morphology and wetting properties. Colloids Surf. B Biointerfaces 192, 111047. doi:10.1016/j.colsurfb.2020.111047
Salazar, J., Amer, M.-À., Turó, A., Castro, N., Navarro, M., Soto, S., et al. (2023). Real-time detection of the bacterial biofilm formation stages using QCM-based sensors. Chemosensors 11, 68. doi:10.3390/chemosensors11010068
Sanchez-Vizuete, P., Dergham, Y., Bridier, A., Deschamps, J., Dervyn, E., Hamze, K., et al. (2021). The coordinated population redistribution between Bacillus subtilis submerged biofilm and liquid-air pellicle. Biofilm 4, 100065. doi:10.1016/j.bioflm.2021.100065
Shchukin, D. G., Zheludkevich, M., Yasakau, K., Lamaka, S., Ferreira, M. G. S., and Möhwald, H. (2006). Layer-by-layer assembled nanocontainers for self-healing corrosion protection. Adv. Mater. 18, 1672–1678. doi:10.1002/adma.200502053
Sheoran, V., Sheoran, A. S., and Poonia, P. (2009). Phytomining: a review. Miner. Eng. 22, 1007–1019. doi:10.1016/j.mineng.2009.04.001
Siems, K., Müller, D., Maertens, L., Van Houdt, R., Mancinelli, R. L., Caplin, N., et al. (2021). “Fighting microbial biofilms in space by ESA's upcoming space microbiology and material science experiment BIOFILMS,” in 23rd IAA Humans in Space, Virtual Conference, April, 2021.
Speziale, P., Pietrocola, G., Rindi, S., Provenzano, M., Provenza, G., Di Poto, A., et al. (2009). Structural and functional role of Staphylococcus aureus surface components recognizing adhesive matrix molecules of the host. Future Microbiol. 4, 1337–1352. doi:10.2217/fmb.09.102
Srivastava, G. N., Malwe, A. S., Sharma, A. K., Shastri, V., Hibare, K., and Sharma, V. K. (2020). Molib: a machine learning based classification tool for the prediction of biofilm inhibitory molecules. Genomics 112, 2823–2832. doi:10.1016/j.ygeno.2020.03.020
Stoodley, P. (2016). Biofilms: flow disrupts communication. Nat. Microbiol. 1, 15012–2. doi:10.1038/nmicrobiol.2015.12
Stoodley, P., Lewandowski, Z., Boyle, J. D., and Lappin-Scott, H. M. (1999). Structural deformation of bacterial biofilms caused by short-term fluctuations in fluid shear: an in situ investigation of biofilm rheology. Biotechnol. Bioeng. 65, 83–92. doi:10.1002/(sici)1097-0290(19991005)65:1<83::aid-bit10>3.0.co;2-b
Vandenabeele, C. R., and Lucas, S. (2020). Technological challenges and progress in nanomaterials plasma surface modification–a review. Mater. Sci. Eng. R Rep. 139, 100521. doi:10.1016/j.mser.2019.100521
Van Harten, R. M., Van Woudenbergh, E., Van Dijk, A., and Haagsman, H. P. (2018). Cathelicidins: immunomodulatory antimicrobials. Vaccines 6, 63. doi:10.3390/vaccines6030063
Venkateswaran, K., Vaishampayan, P., Cisneros, J., Pierson, D. L., Rogers, S. O., and Perry, J. (2014). International Space Station environmental microbiome—microbial inventories of ISS filter debris. Appl. Microbiol. Biotechnol. 98, 6453–6466. doi:10.1007/s00253-014-5650-6
Wang, C., Wang, T., Hu, P., Shen, T., Xu, J., Ding, C., et al. (2020). Dual-functional anti-biofouling coatings with intrinsic self-healing ability. Chem. Eng. J. 389, 123469. doi:10.1016/j.cej.2019.123469
Wang, M., Duday, D., Scolan, E., Perbal, S., Prato, M., Lasseur, C., et al. (2021). Antimicrobial surfaces for applications on confined inhabited space stations. Adv. Mater. Interfaces 8, 2100118. doi:10.1002/admi.202100118
Warheit, D. B., Hoke, R. A., Finlay, C., Donner, E. M., Reed, K. L., and Sayes, C. M. (2007). Development of a base set of toxicity tests using ultrafine TiO2 particles as a component of nanoparticle risk management. Toxicol. Lett. 171, 99–110. doi:10.1016/j.toxlet.2007.04.008
Weir, N., Wilson, M., Yoets, A., Molina, T., Bruce, R., and Carter, L. (2012). “Microbiological characterization of the international space station water processor assembly external filter assembly S/N 01,” in 42nd International Conference on Environmental Systems (ICES), San Diego, CA, USA, July, 2012, 3595.
Yazdani-Ahmadabadi, H., Yu, K., Khoddami, S., F. Felix, D., Yeh, H. H., Luo, H. D., et al. (2022). Robust nanoparticle-derived lubricious antibiofilm coating for difficult-to-coat medical devices with intricate geometry. ACS Nanosci. Au 3, 67–83. doi:10.1021/acsnanoscienceau.2c00040
Zabiegaj, D., Hajirasouliha, F., Duilio, A., Guido, S., Caserta, S., Kostoglou, M., et al. (2021). Wetting/spreading on porous media and on deformable, soluble structured substrates as a model system for studying the effect of morphology on biofilms wetting and for assessing anti-biofilm methods. Curr. Opin. Colloid and Interface Sci. 53, 101426. doi:10.1016/j.cocis.2021.101426
Zea, L., McLean, R. J. C., Rook, T. A., Angle, G., Carter, D. L., Delegard, A., et al. (2020). Potential biofilm control strategies for extended spaceflight missions. Biofilm 2, 100026. doi:10.1016/j.bioflm.2020.100026
Keywords: biofilm, nanoparticles, antimicrobial coatings, space, biofilm detection, management strategies, wetting, bacteria
Citation: Marra D, Ferraro R and Caserta S (2024) Biofilm contamination in confined space stations: reduction, coexistence or an opportunity?. Front. Mater. 11:1374666. doi: 10.3389/fmats.2024.1374666
Received: 22 January 2024; Accepted: 18 March 2024;
Published: 28 March 2024.
Edited by:
Malgorzata Holynska, European Space Research and Technology Centre (ESTEC), NetherlandsReviewed by:
Thodoris D. Karapantsios, Aristotle University of Thessaloniki, GreeceDavid Duday, Luxembourg Institute of Science and Technology (LIST), Luxembourg
Copyright © 2024 Marra, Ferraro and Caserta. This is an open-access article distributed under the terms of the Creative Commons Attribution License (CC BY). The use, distribution or reproduction in other forums is permitted, provided the original author(s) and the copyright owner(s) are credited and that the original publication in this journal is cited, in accordance with accepted academic practice. No use, distribution or reproduction is permitted which does not comply with these terms.
*Correspondence: Sergio Caserta, sergio.caserta@unina.it