Optimizing PEEK implant surfaces for improved stability and biocompatibility through sandblasting and the platinum coating approach
- 1Research Center for Biomedical Engineering, Faculty of Engineering, Universitas Indonesia, Depok, West Java, Indonesia
- 2Institute of Functional Surfaces (iFS), School of Mechanical Engineering, University of Leeds, Leeds, United Kingdom
- 3Biomedical Engineering Program Study, Department of Electrical Engineering, Faculty of Engineering, Universitas Indonesia, Depok, West Java, Indonesia
- 4Department of Microbiology, Faculty of Medicine, Universitas Indonesia, Salemba, Jakarta, Indonesia
- 5Department of Mechanical Engineering, Faculty of Engineering, Universitas Indonesia, Depok, West Java, Indonesia
Polyether–ether–ketone (PEEK) is a commonly employed biomaterial for spinal, cranial, and dental implant applications due to its mechanical properties, bio-stability, and radiolucency, especially when compared to metal alloys. However, its biologically inert behavior poses a substantial challenge in osseointegration between host bone and PEEK implants, resulting in implant loosening. Previous studies identified PEEK surface modification methods that prove beneficial in enhancing implant stability and supporting cell growth, but simultaneously, those modifications have the potential to promote bacterial attachment. In this study, sandblasting and sputter coating are performed to address the aforementioned issues as preclinical work. The aim is to investigate the effects of surface roughness through alumina sandblasting and a platinum (Pt) sputtered coating on the surface friction, cell viability, and bacterial adhesion rates of PEEK material. This study reveals that a higher average surface roughness of the PEEK sample (the highest was 1.2 μm obtained after sandblasting) increases the coefficient of friction, which was 0.25 compared to the untreated PEEK of 0.14, indicating better stability performance but also increased bacterial adhesion. A novelty of this study is that the method of Pt coating after alumina sandblasting is seen to significantly reduce the bacterial adhesion by 67% when compared to the sandblasted PEEK sample after 24 h immersion, implying better biocompatibility without changing the cell viability performance.
1 Introduction
Polyether–ether–ketone (PEEK) is a leading polymeric biomaterial used in orthopedics and bone tissue fields. Specifically, PEEK has been performing well in implant applications, such as spinal fusion, neurosurgical, dental, and cranio-maxillofacial replacements (Faadhila et al., 2022; Dondani et al., 2023; Jia et al., 2023). This performance is due to its mechanical properties, biological stability, radiolucency, and ability to biomechanically mimic a bone (Gu et al., 2021; Dondani et al., 2023). These properties are considered to tackle drawbacks and decrease post-implantation biological reactions caused by previous conventional biomaterials, such as metallic alloys (Ananth et al., 2015; AlOtaibi et al., 2020; Sarfraz et al., 2022). Titanium (Ti) alloy, as an example, possesses limitations to the biocompatibility aspects because it can cause metal ion toxicity, osteolysis, and allergenic effects, as well as stress shielding and bone resorption due to its relatively high elastic modulus (Huiskes et al., 1992; Fage et al., 2016). Therefore, PEEK-based biomaterial has emerged and been developed as an alternative. Despite those advantages compared to the metals, PEEK suffers from hydrophobicity, bioactivity, and osseointegration performance (Najeeb et al., 2016; Fogel et al., 2022). The biologically inert behavior of PEEK material creates a constraint to integrating with its host bone when implanted, causing the implant to loosen (Gu et al., 2021). Moreover, PEEK material does not have antibacterial behavior, so many bacteria from the human body can easily adhere to its surface (Sarfraz et al., 2022). This lack of ability potentially generates PEEK implant-related infections and surgical failures (Wang et al., 2016; Ouyang et al., 2018).
Many studies have aimed to optimize the bonding initiation between PEEK implants and the host bone for the osseointegration process. The common methods involve PEEK surface alterations to obtain higher adhesion ability through physical and chemical treatments, surface coatings, and composite modifications (Whulanza et al., 2016; Nadhif et al., 2017; Dondani et al., 2023). Plasma ion implantation is one of the common physical change techniques used as a pre-treatment method, showing sufficient compatibility with the PEEK surface and the other surface treatments. This treatment has been observed to reduce the contact angle during the wettability test (Ha et al., 1997; Tsougeni et al., 2009; Akkan et al., 2014), increase PEEK bioactivity (Gan et al., 2016; Wakelin et al., 2018), and cell adhesion and osseointegration (Liu et al., 2017; Ouyang et al., 2019). However, the plasma treatments have limitations in increasing the hardness of the surface and are challenging for implants with a complex geometry due to electromagnetic radiation (Jemat et al., 2015; Lu et al., 2015). Accelerated neutron atom beam (ANAB) (Khoury et al., 2019), photodynamic therapy (Peng et al., 2021), laser radiation (Xie et al., 2021), and sandblasting (Qosim et al., 2018; Sunarso et al., 2018) are alternatives included in the physical treatment group which are still underdeveloped but highly prospective. However, it is noted that some methods only improve the interlocking without increasing the hydrophilicity and antimicrobial performance of the PEEK material.
The sandblasting technique was broadly employed in the surface modification of Ti-based implants to roughen the surface through a spray of abrasive particles and effectively enhance bone–implant integration (Grassi et al., 2006; Elias et al., 2008). These successful in vivo studies have encouraged recent work in modifying the PEEK implant surface roughness due to the technique’s procedures, cost, and impacts. A previous study (Sunarso et al., 2018) has shown positive impacts on the cell responses and osseointegration ability using a sandblasting approach to the PEEK surface. By spraying alumina particles, the study brought evidence that the micro-roughened PEEK increased adhesion, proliferation, and differentiation of rat bone marrow-derived mesenchymal stem cells and mitigation of inflammatory chemokine ligand 2 when compared to the untreated PEEK samples. However, microbial adhesion was not evaluated in that study. A combination of surface modification methods is required to reduce the limitations of each individual approach.
Mechanical and/or chemical coating approaches have attempted to make PEEK surface more antibacterial (Meng et al., 2020; Mo et al., 2020; Ishihama et al., 2021; Mo et al., 2021; Yang et al., 2023). The objective is to utilize the coating behavior by either inhibiting bacterial adhesion or chemically reducing the live bacteria (Gao et al., 2022). A platinum-based coating applied through magnetron sputtering or electrodeposition on various biomaterials has been developed in several studies (Scholz et al., 2005; Abuayyash et al., 2020; Czerwińska-Główka et al., 2020; Brown et al., 2021; Devendra et al., 2022) due to platinum’s antimicrobial ability. Platinum is renowned for its resistance to corrosion, oxidation, and heat. Its biocompatibility sets it apart from other metals like nickel or copper as platinum remains inert within the human body, making it both hypoallergenic and biocompatible. These attributes position platinum as an ideal material for biomedical and semi-conductive applications (Guarnieri et al., 2014; Boehler et al., 2017; Cassar et al., 2019; Raj et al., 2022). Nevertheless, the effect of platinum coating on the PEEK implant performance has not yet been investigated. Therefore, this study utilizes a platinum coating to introduce a novel coating material that enhances the antibacterial adhesion performance of PEEK.
Studies have shown several PEEK surface modification methods that can improve the stability and cell growth of implants and also potentially escalate bacterial attachment. In this study, sandblasting and magnetron sputtering are employed to address both issues as preclinical work. The aim is to investigate the effects of surface characteristics through alumina sandblasting and a platinum (Pt) sputtered coating on implant stability, cell growth, and bacterial adhesion rates in PEEK material. Untreated PEEK samples are compared to the different groups of surface-treated samples regarding (a) surface roughness average and friction coefficient using a contacting probe and a tribometer for stability performance, as well as (b) cell viability and bacterial adhesion, respectively, using stem cells and Staphylococcus aureus bacteria for biocompatibility aspects. It is hypothesized that the bacterial adhesion on the PEEK surface could be reduced without changing its implant stability performance through the coupled methods of Pt coating after alumina sandblasting.
2 Materials and methodology
2.1 PEEK sample preparation
PEEK rods (Jiangsu Junhua Ltd., China) with a diameter of 30 mm were cut to obtain thicknesses of 2 mm and 5 mm, as seen in Figure 1A. Table 1 shows the material properties of the PEEK used in this study provided by the supplier. The samples were polished using a DAP-7 machine (Struer, Denmark) to homogenize the surface roughness average (
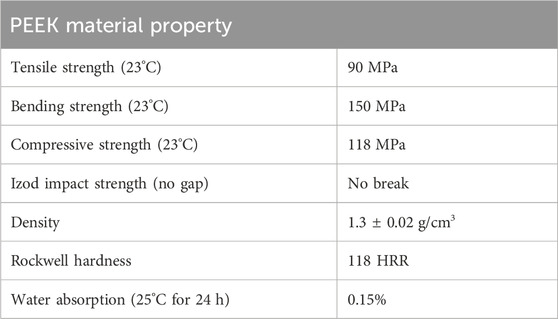
TABLE 1. Material properties of PEEK (Jiangsu Junhua ChinaPEEK, 2016).
Sandblasting was performed using a spray gun positioned to face downward, connected to an air compressor and a particle chamber, and used 250-μm alumina (Al2O3) as the sprayed material. The sample was placed on a stainless steel holder and positioned directly below the spray gun at an 8-cm distance. Once the pressure on the regulator reached 50 psi, the spray gun was triggered to spray the sample for 10 s, 20 s, and 30 s to observe the duration effect. The pressure effect was also compared with samples sprayed at 75 psi and 100 psi for the mentioned durations.
Sputter coating was performed using the LUXOR Platinum Coater for SEM (Luxor Tech, Belgium). The sandblasted and non-sandblasted PEEK samples were attached to the top cover of the chamber. A platinum (Pt) block, which served as the coating material, and a magnetron were placed at the bottom of the closed chamber. The coating mode was set for a thickness of 100 nm, working in a vacuum and filling the chamber with argon gas at a pressure of 10 Pa. At the end, groups of PEEK samples, that is, untreated, sandblasted, Pt-coated, and sandblasted with Pt coating, were obtained.
2.2 Testing methodology
This study used in vitro laboratory-scale research, as shown in Figure 2. Following sample preparation, the PEEK materials were characterized and tested through both mechanical and biological approaches.
2.2.1 Surface characterization
Several modified surface characterizations were investigated, including surface roughness average (
To assess the impact of surface roughness on material stability, the CoF of the PEEK samples was measured using a sliding tribometer instrument (Rtec Instruments, USA) against a stainless steel ball. The friction test was performed continuously, tracing a circular path for a duration of 60 s, using a force of 20 N and a rotational speed of 100 rpm. This testing procedure was repeated three times on three different PEEK samples.
All Pt-coated PEEK samples were examined in the coating adhesion test by making cross-shaped scratches on the material surface using a scalpel surgical knife. Then, the surface was attached with adhesive tape and pulled in one swift motion (Whulanza et al., 2022). A Hitachi SU-3500 scanning electron microscope (SEM) (Hitachi, Japan) at magnifications of 500× and 1000×, along with energy dispersion x-ray spectroscopy (EDS), were employed to observe surface topography and platinum concentration, respectively. The selections of three points on each sample before testing and three points near the scratched area after testing were made during EDS observation. SEM magnification, speed rate, area of interest, and lighting adjustments were monitored to obtain proper visualizations.
2.2.2 Biological aspect testing
Observations for biological compatibility aspects were performed using cell viability and bacterial adhesion techniques to investigate the influence of PEEK modification on cell growth and bacterial components. Cell viability in this study was assessed using the indirect dye compound 3-(4,5-dimethylthiazol-2-yl)-2,5-diphenyltetrazolium bromide (MTT) assay in triplicate on umbilical cord mesenchymal stem cells (UCMSCs). The samples were sterilized using an autoclave for 1 h. Then, they were placed in a complete medium (heparin, glutamine, fungizone, and pen strep) to soak the samples and stored at 37°C for 24 h, 48 h, and 72 h. At the same time, cells and 100 µL of complete medium were mixed in a 96-well plate and incubated in a CO2 incubator at 37°C and 5% CO2. Several laboratory tools were also used, such as P-200 and P-1000 micropipettes, P-200 and P-1000 micropipette tips, microtubes, centrifuge machine, glass tubes, microcentrifuge tubes, forceps, spatulas, falcon tubes, sterilized cups, and a vortex mixer. The next day, the complete medium was removed and replaced with the soaking medium. Three wells were left with cells without soaking medium as controls. The plate was then returned to the incubator at 37°C and 5% CO2 for 24 h. The MTT solution was then added to each well and incubated at 37°C for 4 h. The medium was removed from the wells and replaced with dimethyl sulfoxide (DMSO). The measurements were performed using a spectrophotometer at a wavelength of 570 nm to determine the absorbance values of each sample. The percentage viability was calculated by normalizing the viability values of the test samples to the control using Eq. 1 (Whulanza et al., 2019).
Bacterial adhesion testing was performed to assess the implant’s ability to bind bacteria within the body. In this experiment, we used Staphylococcus aureus bacteria as the primary causative agent of bone inflammation. Samples were immersed in a Staphylococcus aureus bacterial suspension of 0.5 McFarland for 15 min, 60 min, and 24 h. The immersion was carried out in an incubator at a temperature of 35°C. Then, the samples were placed in tubes containing sodium chloride (NaCl), followed by rinsing and draining protocols. The samples were transferred to microtubes containing another NaCl solution and centrifuged for 10 min at 4,000 rpm to release the bacteria binding, then diluted to a 1:10 ratio. Subsequently, 10 µL of the solution was placed in a Petri dish containing a blood agar plate (BAP) using an inoculating loop and spread evenly. The BAP plates were then placed in an incubator at 35°C for 24 h. The microbial growth in the BAP was counted by calculating the colony-forming units (CFUs) to compare the four samples. The bacterial adhesion assay was conducted in duplicate for three different time variations and four different samples. Each type of sample was tested twice.
2.3 Statistical analysis
The results are presented as averages, with error bars representing the standard deviation (SD). Statistical analysis was performed using a two-way analysis of variance (ANOVA) with a post hoc Dunnett test. ANOVA was employed to determine the significance of the treatment methods on the testing parameters of the PEEK surface. A significance level of α = 0.05 was used, and the p-value was compared in the mentioned comparison. Results with a p-value less than 0.05 were considered significantly different and indicated by an asterisk (*).
3 Results
3.1 Surface characterization after sandblasting
Figure 3 shows the comparison between the
Figure 4 shows the statistical comparison of CoF values that were automatically calculated by the instrument between the untreated and sandblasted (at 100 psi for 30 s) PEEK samples. The results presented that the coating method significantly elevated the average CoF of PEEK (p-value = 0.0222), as well as the sandblasting method (p-value = 0.0158). The combination of both methods demonstrates the highest increase of CoF (p-value = 0.0018).
Figure 5 visualizes the surface topography via SEM images of un-sandblasted samples at magnifications of 1000×, as well as samples sandblasted for 20 s and 30 s at 50 psi, 75 psi, and 100 psi. When compared to the sandblasted samples, the surface topography of the untreated samples appears smoother, and there is no indication of micro-level structures, such as holes or intricate surface texture profiles. The presence of fibers or straight lines on the surface was caused by the traces of sanding during the smoothing process. Meanwhile, the black spots that appeared were due to inadequate sample cleaning from dirt and dust before coating. In contrast, the sandblasted samples exhibited an irregular and uneven surface. This uneven surface will impact surface roughness, which is also related to the coefficient of friction in determining implant stability. Furthermore, deeper grooves will be more easily populated by cells, thus creating micro-locking between the implant and cells. The surface visualization underwent a distinct change in the samples after being pressurized at 100 psi for 20 and 30 s, exhibiting more apparent roughness in the profile, as observed via SEM images than the lower pressure settings (50 and 75 psi). The morphology appeared relatively similar when comparing the samples after sandblasting at 75 psi for 20 s to those samples sandblasted at 50 psi. These visualization trends align with the roughness average comparison, supporting the quantitative results.
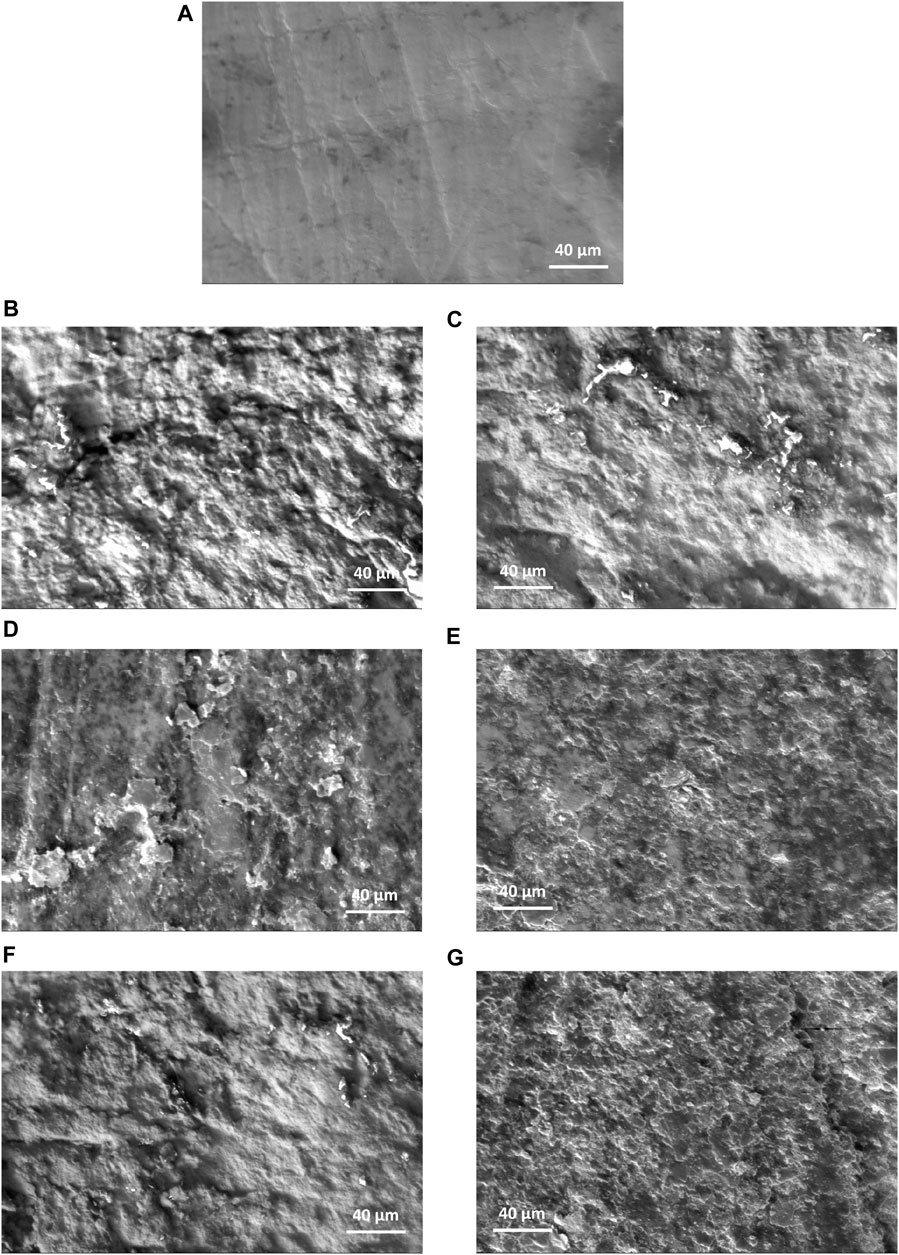
FIGURE 5. Surface morphology via SEM images at 1000× magnification of (A) un-sandblasted samples, as well as samples sandblasted for 20 s and 30 s, respectively at (B, C) 50 psi, (D, E) 75 psi, and (F, G) 100 psi.
3.2 Platinum coating adhesion
In this test, the Pt-coated samples were scratched using a scalpel, and then the coating was peeled off with adhesive tape. Visible damage due to scratching was apparent, but damage around the scratches was not visible, according to the visualization through SEM in Figure 6. Following this, the EDS was used to quantify the decrease in Pt (wt%) concentration on the samples with and without sandblasting. The examination was performed on the samples before and after the test at three random points within a radius of <100 µm around the scratches.
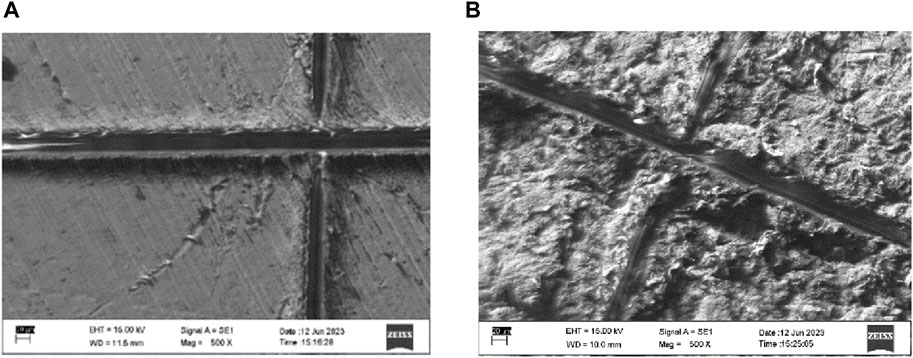
FIGURE 6. Damage due to the coating adhesion test on the PEEK sample (A) without and (B) with sandblasting.
From the t-test analysis, there was no significant difference between Pt (wt%) concentration before and after the peel-off in the samples without sandblasting (p-value = 0.9671). The sandblasted samples also experienced no significant difference between before and after the test (p-value = 0.2354). These findings substantiate that the presence of scratches did not induce a notable impact on the surrounding Pt coating, thus demonstrating its robust resistance against both scratching and peeling.
To assess the distribution of the coating, a one-way ANOVA analysis was executed on the three measurement points within the specimens. The result indicated that there was no significant difference (p-value = 0.8753) in Pt concentration among the random points within the samples without sandblasting and represented a consistent distribution. The results also imply a uniformly distributed Pt concentration within the sandblasted samples (p-value = 0.6202). All comparisons are shown in Figure 7. Additionally, a t-test analysis was carried out to compare the depth of Pt coating between samples with and without sandblasting, as determined by concentration analysis. The outcome of this analysis revealed no substantial difference in coating depth between samples subjected to the sandblasting process and those that were not sandblasted (p-value = 0.6346).
3.3 Cell viability
Figure 8 shows the comparison of cell viability across all samples, that is, the untreated, sandblasted, and coated surfaces, compared to the cell control after 24 h, 48 h, and 72 h. It showed that some samples exhibited fluctuating absorbance values. However, these fluctuations in viability were not significantly different, suggesting a stable number of viable cells during the three time periods. The normalized viability values compared to the control after 24 h, 48 h, and 72 h were 85.53%, 95.47%, and 82.48%, respectively.
Significantly fluctuating results were observed in samples treated with sandblasting, both with and without Pt coating. In the sandblasted sample without Pt coating, viability significantly decreased at 48 h and then increased again at 72 h. On the other hand, viability significantly increased at 48 h and then decreased slightly at 72 h in the sandblasted samples with Pt coating. The viability calculations for the sandblasted samples without coating compared to the control at 24 h, 48 h, and 72 h were 98.87%, 67.29%, and 86.97%, respectively, while for the sandblasted samples with coating, the values were 61.72%, 100.82%, and 80.16%, respectively.
The viability results for Pt-coated samples indicated good cell growth. The viability of the cells gradually increased, although not significantly. The viability percentages for the time periods of 24 h, 48 h, and 72 h are 70.81%, 88.66%, and 92.65%. Generally, the shortest period showed relatively low viability values for all samples. The Pt coating was associated with an increased amount of cell growth.
3.4 Bacterial adhesion
Figure 9 compares the number of bacteria per volume on the untreated and treated samples. The results indicated that the sandblasting method significantly increased the bacterial adhesion rate (p-value = 0.0152), while coating significantly reduced bacterial adhesion (p-value = 0.0016) compared to the untreated PEEK samples. This behavior was also seen when the two treatments were coupled, where the sandblasted, coated samples had a significantly lower rate than the sandblasted-without-coating samples (p-value = 0.1170). Therefore, there was no significant difference between the untreated material and the material treated with both sandblasting and coating. The results also demonstrated that the longer the PEEK samples were immersed in the medium, the more bacteria adhered to the sample. However, a small quantity of bacteria tends to be effectively countered by the immune system and does not cause other diseases, such as bone inflammation.
4 Discussion
Considering the advantages of using PEEK material compared to Ti alloy in the dental and orthopedic fields recently, the osseointegration and infectious factors of PEEK material remain of significant interest to the biomaterial research groups due to the clinical issues of implant loosening and less biocompatible material. While the method of physically modifying the PEEK surface roughness through sandblasting has been investigated previously (Sunarso et al., 2018), the behavior of the treatment combined with other coating material and its effect on bacterial adhesion is still unknown. For the first time, this study employed sandblasting and sputter coating to address PEEK material’s osseointegration and microbial issues as part of a preclinical investigation. The surface-treated PEEK samples are compared with regard to the surface roughness and friction assessed using a contacting probe and tribometer to evaluate stability performance. To assess biocompatibility, cell viability was evaluated via UCMSCs, and bacterial adhesion was evaluated using S. aureus. The major finding of this study is that bacterial adhesion on the PEEK surface was highly influenced through the combined application of Pt coating following alumina sandblasting without compromising implant stability performance.
In this study, alumina sandblasting of PEEK implants was shown to effectively increase the surface roughness average in accordance with the valleys and rough surface observed in the implant surface topography, corresponding to an improved coefficient of friction. Higher pressure and longer spraying duration resulted in higher surface roughness. The increased surface friction theoretically means that the resistant force of a surface that encounters another surface is higher, justifying the enhanced implant stability (Stachowiak and Batchelor, 2014). This also determines that the true contact area and the specific resistance to the shearing of the asperities between the PEEK surface and the counter body have increased (Williams, 2005). The goal is to have good contact and interlocking mechanisms between the PEEK surface and the host bone or tissue, as well as to promote protein adsorption during the integration process. Similar to the concept of other physical modification techniques that have been developed, higher surface texture can accommodate these mechanisms to be more effective.
A surface treatment combined with coating is recommended to activate PEEK implant surfaces. PEEK implants with moderately rough surfaces, characterized by roughness average values ranging between 1 μm and 1.5 μm, facilitate the adhesion of osteoblasts, promoting high proliferation rates and optimal osseointegration (Carlos Nelson and Ilser, 2011; Elawadly et al., 2017). Studies on PEEK modification through various techniques, including the sandblasting method, have been reviewed by Dondani et al. (2023) and AlOtaibi et al. (2020), summarizing the current and future perspectives on surface treatments for osseointegration to bone. A previous investigation by Sunarso et al. (2018) reported the effect of micro-roughening of PEEK on bone marrow-derived stem cell and macrophage responses, showing a positive impact of the alumina sandblasting approach in the osseointegration performance. The study compared PEEK samples with a surface roughness of approximately 2.3 μm for the sandblasted samples to untreated mirror-polished PEEK samples with a surface roughness of 0.06 μm. The integration assessment was done through the pull-out force test on both PEEK groups when implanted in rat femur bone cavities. The sandblasted PEEK required four times higher forces than the untreated ones. This effect aligns with the result shown in this study, in which the osseointegration was represented through the friction coefficient measurement using a tribometer. Even though the surface roughness obtained in the current study was much lower, approximately 1.2 μm, the results still agree that the sandblasting significantly increases the friction coefficient from the untreated PEEK of ∼1.5–2.5. Another validation technique could involve the wettability test, where the roughened surface will increase the contact angle of the water droplet, as mentioned in many works (Ha et al., 1997; Tsougeni et al., 2009; Kubiak et al., 2011; Akkan et al., 2014; Ourahmoune et al., 2014). Previous studies (Gittens et al., 2014; Fogel et al., 2022; Kia et al., 2022; Jia et al., 2023) have developed various surface engineering techniques, specifically for spinal fusion applications, that had the same hypothesis and objective in addressing the osteointegration issue of PEEK material. However, a limited number of works discuss the effect of PEEK surface treatments on the attachment of microorganisms mimicking the human body environment.
In this study, the increased bacterial adhesion after sandblasting was detected on the PEEK surface. As a novel finding, the platinum presence at the PEEK surface deposited through a magnetron sputtered coating was seen to significantly reduce the bacterial adhesion rate and also improved the coefficient of friction on both the untreated and the sandblasted samples. These coupled treatments indicated a higher biocompatibility of the PEEK implants, whilst they did not have any significant effect on the cell growth as determined using stem cell viability. Additionally, the platinum coating shows a benefit in visual imaging through electron microscopy by increasing the conductivity of PEEK material. Gao et al. (2022) made a systematic overview of the modification strategies for advancing the antibacterial ability of PEEK. Previous works (Meng et al., 2020; Mo et al., 2020; Ishihama et al., 2021; Mo et al., 2021; Chen et al., 2022; Yang et al., 2023) across research groups have made efforts to develop an antibacterial surface modification of PEEK, most often through coating approach. The intention generally is to reduce the contact area between PEEK and bacteria and/or reduce the viability of the surrounding bacteria through surface morphology alteration and antibacterial coating. The modifications on the micro and nanostructure of the PEEK surface were reported to play different roles in disrupting bacterial cell membrane (Sun et al., 2009; Ivanova et al., 2012; Mo et al., 2020), reducing the adhesion contact area (Lu et al., 2014; Rochford et al., 2014), and isolating and trapping them from other strains (Lu et al., 2016; Ouyang et al., 2016; Wang et al., 2016; Wang et al., 2018). The fundamental idea is to postpone and prevent the formation of biofilm created by bacteria on the implant surface. Wang et al. (2016) and Lu et al. (2014) used a plasma immersion ion implantation to construct a multistage titanium oxide nanostructure on the PEEK surface. The results showed that the coating effectively has a long-term antibacterial ability, specifically for oral bacterial strains, and facilitates better tissue integration and implant stability due to a high coefficient of friction. It was described that the nanoparticles prevented the bacteria attachment at the early stage by limiting the contact area between the PEEK and the bacteria, thus reducing the adhesion rate of S. aureus. A pitting structure on the PEEK surface was also developed by Rochford et al. (2014) through oxygen plasma for an orthopedic implant. An aligned concept was applied that the pits could weaken the bacterial activity contacting with the PEEK surface by creating pits that are the same size as the bacteria. However, none of these studies has used platinum-based coating on the PEEK material in implant applications to compare with the current study.
Applying a platinum-based coating through magnetron sputtering or electrodeposition on various materials other than PEEK has been examined due to its antibacterial ability. Abuayyash et al. (2020) demonstrated a novel thin coating combining silver (Ag) and Pt through sputter deposition on Ti-based material. The coating formation works electrochemically to improve antibacterial adhesion after implantation and tissue cell attachment by a sacrificing mechanism using silver as the anode. Scholz et al. (2005) characterized the antimicrobial ability of various metallic coating materials on silicon oxide fabrics. They reported that Pt coating is a useful asset for medical device applications that effectively stops bacterial and fungal activities. Czerwińska-Główka et al. (2020) found that a platinum coating only had short-term effectiveness in reducing the bacterial activity on Pt-coated glass slides. It was hypothesized to have a similarity in biocidal behavior upon bacterial colonization as in the sputter-coated layer of copper (Cu) and Ag (McLean et al., 1993; Wang et al., 2008). However, the detailed mechanism of platinum coating in hindering the bacterial strain of implant devices is still unclear. Further investigation is needed to obtain an effective combination of surface treatment and coating materials to protect the PEEK material from infectious factors when used in implants.
While this study has successfully identified the effect of modified PEEK material surface on stability and biocompatibility, some limitations must be considered. It is known that the in vitro conditions do not directly agree with the clinical situations where many bodily substances and environmental factors interact at the same time. It was noted that the higher pressure and longer spraying duration result in higher surface roughness, but optimum parameters for the sandblasting process and their correlations with changes in the coefficient of changes must be determined. Further characterization is required. The friction coefficient test used a sliding tribometer but was limited by using a steel ball as the counterpart and a 1-min test duration. Nevertheless, this short duration has been sufficient to have a valid measurement because all attempts showed consistency. This study only used one type of bacterial strain, whereas, in real conditions, multiple microbial types can interact with the PEEK-bone integration. Platinum coating performance on the live/dead bacteria comparison has not been evaluated in this study; only the bacterial adhesion rate has been reported. Similarly, with regard to cell viability, this study only reports the percentage of cell growth over 72 h. Further research is required to investigate cell growth under both treatments over a longer time period.
5 Conclusion
The effect of modifying the surface of PEEK material by alumina sandblasting and platinum sputtered coating on stability performance and bacterial adhesion was successfully investigated. The following points are drawn from the obtained results:
• Alumina sandblasting of PEEK implants has been shown to effectively increase surface roughness. Higher pressure and longer shooting times result in greater surface roughness.
• Increased surface roughness corresponds to an improved coefficient of friction, indicating enhanced implant stability. However, it is essential to note that sandblasting alone significantly increases bacterial adhesion.
• To address the issue of increased bacterial adhesion resulting from sandblasting, a subsequent platinum coating can be applied to significantly reduce the adhesion and increase the coefficient of friction compared to using the sandblast method alone.
• These sandblasting and platinum-coated treatments also support cell growth and provide higher biocompatibility of the PEEK implants.
Future studies may need to investigate other combinations of surface treatment to enable more alternatives for osseointegration when implanting PEEK material. Broader parameters for optimizing the sandblasting and sputter coating applications need to be characterized in polymer surface modification. Those suggestions may contribute to a deeper understanding of PEEK surface bone integration and those interactions with the surrounding microbial environment in vivo.
Data availability statement
The original contributions presented in the study are included in the article/supplementary material; further inquiries can be directed to the corresponding author.
Ethics statement
Ethical approval was not required for any studies on animals in accordance with the local legislation and institutional requirements because only commercially available established cell lines were used.
Author contributions
AF: investigation, visualization, and writing–original draft. MT: data curation and writing–review and editing. PK: formal analysis, supervision, writing–review and editing. SR: resources, software, writing–review and editing. DL: methodology, validation, and writing–original draft. YW: conceptualization, funding acquisition, project administration, and writing–review and editing.
Funding
The author(s) declare that financial support was received for the research, authorship, and/or publication of this article. The study was funded by Universitas Indonesia PUTI Grant NKB-795/UN2.RST/HKP.05.00/2020 Nomor: 626/SK/R/UI/2020.
Conflict of interest
The authors declare that the research was conducted in the absence of any commercial or financial relationships that could be construed as a potential conflict of interest.
Publisher’s note
All claims expressed in this article are solely those of the authors and do not necessarily represent those of their affiliated organizations, or those of the publisher, the editors, and the reviewers. Any product that may be evaluated in this article, or claim that may be made by its manufacturer, is not guaranteed or endorsed by the publisher.
References
Abuayyash, A., Ziegler, N., Meyer, H., Meischein, M., Sengstock, C., Moellenhoff, J., et al. (2020). Enhanced antibacterial performance of ultrathin silver/platinum nanopatches by a sacrificial anode mechanism. Nanomedicine Nanotechnol. Biol. Med. 24, 102126. doi:10.1016/j.nano.2019.102126
Akkan, C. K., Hammadeh, M. E., May, A., Park, H. W., Abdul-Khaliq, H., Strunskus, T., et al. (2014). Surface topography and wetting modifications of PEEK for implant applications. Lasers Med. Sci. 29 (5), 1633–1639. doi:10.1007/s10103-014-1567-7
AlOtaibi, N., Naudi, K., Conway, D., and Ayoub, A. (2020). The current state of PEEK implant osseointegration and future perspectives: a systematic review. Eur. Cell Mater 40, 1–20. doi:10.22203/ecm.v040a01
Ananth, H., Mankar, S., Mohammed, H. S., Anand, M., and Amarnath, G. S. (2015). A review on biomaterials in dental implantology. Int. J. Biomed. Sci. 11 (3), 113–120. doi:10.59566/ijbs.2015.11113
Boehler, C., Oberueber, F., Schlabach, S., Stieglitz, T., and Asplund, M. (2017). Long-term stable adhesion for conducting polymers in biomedical applications: IrOx and nanostructured platinum solve the chronic challenge. ACS Appl. Mater. Interfaces 9 (1), 189–197. doi:10.1021/acsami.6b13468
Brown, R., Lönn, B., Pfeiffer, R., Frederiksen, H., and Wickman, B. (2021). Plasma-Induced heating effects on platinum nanoparticle size during sputter deposition synthesis in polymer and ionic liquid substrates. Langmuir 37 (29), 8821–8828. doi:10.1021/acs.langmuir.1c01190
Carlos Nelson, E. (2011). “Factors affecting the success of dental implants,” in Implant dentistry. IntechOpen: rijeka. Editor T Ilser. Ch. 14.
Cassar, I. R., Yu, C., Sambangi, J., Lee, C. D., Whalen, J. J., Petrossians, A., et al. (2019). Electrodeposited platinum-iridium coating improves in vivo recording performance of chronically implanted microelectrode arrays. Biomaterials 205, 120–132. doi:10.1016/j.biomaterials.2019.03.017
Chen, J., Xie, L., Ruan, Q., Gao, A., Liao, Q., Mo, S., et al. (2022). Diamond-like carbon coating and surface grafting of osteoprotegerin and alendronate on polyetheretherketone to ameliorate the mechanical performance and osseointegration simultaneously. Compos. Part B Eng. 236, 109815. doi:10.1016/j.compositesb.2022.109815
Czerwińska-Główka, D., Przystaś, W., Zabłocka-Godlewska, E., Student, S., Cwalina, B., Łapkowski, M., et al. (2020). Bacterial surface colonization of sputter-coated platinum films. Mater. (Basel) 13 (12), 2674. doi:10.3390/ma13122674
Devendra, B. K., Praveen, B., Tripathi, V., Kumar, H. P., and Chethana, K. (2022). The development of platinum-rhodium alloy coatings on SS304 using a pulse/direct electrodeposition technique and their application to antibacterial activity. J. Indian Chem. Soc. 99 (6), 100466. doi:10.1016/j.jics.2022.100466
Dondani, J. R., Iyer, J., and Tran, S. D. (2023). Surface treatments of PEEK for osseointegration to bone. Biomolecules 13 (3), 464. doi:10.3390/biom13030464
Elawadly, T., Radi, I. A. W., El Khadem, A., and Osman, R. B. (2017). Can PEEK Be an implant material? Evaluation of surface topography and wettability of filled versus unfilled PEEK with different surface roughness. J. Oral Implant. 43 (6), 456–461. doi:10.1563/aaid-joi-d-17-00144
Elias, C. N., Oshida, Y., Lima, J., and Muller, C. (2008). Relationship between surface properties (roughness, wettability and morphology) of titanium and dental implant removal torque. J. Mech. Behav. Biomed. Mater 1 (3), 234–242. doi:10.1016/j.jmbbm.2007.12.002
Faadhila, A., Rahman, S. F., Whulanza, Y., Supriadi, S., Tampubolon, J. Y., Wicaksana, S. I., et al. (2022). Design of a transforaminal lumbar interbody fusion (TLIF) spine cage. Int. J. Technol. 13 (8), 1663–1671. doi:10.14716/ijtech.v13i8.6152
Fage, S. W., Muris, J., Jakobsen, S. S., and Thyssen, J. P. (2016). Titanium: a review on exposure, release, penetration, allergy, epidemiology, and clinical reactivity. Contact Dermat. 74 (6), 323–345. doi:10.1111/cod.12565
Fogel, G., Martin, N., Williams, G. M., Unger, J., Yee-Yanagishita, C., Pelletier, M., et al. (2022). Choice of spinal interbody fusion cage material and design influences subsidence and osseointegration performance. World Neurosurg. 162, e626–e634. doi:10.1016/j.wneu.2022.03.087
Gan, K., Liu, H., Jiang, L., Liu, X., Song, X., Niu, D., et al. (2016). Bioactivity and antibacterial effect of nitrogen plasma immersion ion implantation on polyetheretherketone. Dent. Mater. 32 (11), e263–e274. doi:10.1016/j.dental.2016.08.215
Gao, W., Han, X., Li, Y., Zhou, Z., Wang, J., Shi, R., et al. (2022). Modification strategies for improving antibacterial properties of polyetheretherketone. J. Appl. Polym. Sci. 139 (36), e52847. doi:10.1002/app.52847
Gittens, R. A., Olivares-Navarrete, R., Schwartz, Z., and Boyan, B. D. (2014). Implant osseointegration and the role of microroughness and nanostructures: lessons for spine implants. Acta Biomater. 10 (8), 3363–3371. doi:10.1016/j.actbio.2014.03.037
Grassi, S., Piattelli, A., de Figueiredo, L. C., Feres, M., de Melo, L., Iezzi, G., et al. (2006). Histologic evaluation of early human bone response to different implant surfaces. J. Periodontol. 77 (10), 1736–1743. doi:10.1902/jop.2006.050325
Gu, X., Sun, X., Sun, Y., Wang, J., Liu, Y., Yu, K., et al. (2021). Bioinspired modifications of PEEK implants for bone tissue engineering. Front. Bioeng. Biotechnol. 8, 631616. doi:10.3389/fbioe.2020.631616
Guarnieri, V., Biazi, L., Marchiori, R., and Lago, A. (2014). Platinum metallization for MEMS application. Focus on coating adhesion for biomedical applications, Biomatter 4, e28822. doi:10.4161/biom.28822
Ha, S. W., Kirch, M., Birchler, F., Eckert, K. L., Mayer, J., Wintermantel, E., et al. (1997). Surface activation of polyetheretherketone (PEEK) and formation of calcium phosphate coatings by precipitation. J. Mater. Sci. Mater. Med. 8 (11), 683–690. doi:10.1023/a:1018535923173
Huiskes, R., Weinans, H., and Van Rietbergen, B. (1992). The relationship between stress shielding and bone resorption around total hip stems and the effects of flexible materials. Clin. Orthop. Relat. Research® 274, 124–134. doi:10.1097/00003086-199201000-00014
Ishihama, H., Ishii, K., Nagai, S., Kakinuma, H., Sasaki, A., Yoshioka, K., et al. (2021). An antibacterial coated polymer prevents biofilm formation and implant-associated infection. Sci. Rep. 11 (1), 3602. doi:10.1038/s41598-021-82992-w
Ivanova, E. P., Hasan, J., Webb, H. K., Truong, V. K., Watson, G. S., Watson, J. A., et al. (2012). Natural bactericidal surfaces: mechanical rupture of Pseudomonas aeruginosa cells by cicada wings. Small 8 (16), 2489–2494. doi:10.1002/smll.201200528
Jemat, A., Ghazali, M. J., Razali, M., and Otsuka, Y. (2015). Surface modifications and their effects on titanium dental implants. BioMed Res. Int. 2015, 1–11. doi:10.1155/2015/791725
Jia, C.-Q., Zhang, Z., Cao, S. Q., Wang, T. J., Yu, H. C., Wang, W. X., et al. (2023). A biomimetic gradient porous cage with a micro-structure for enhancing mechanical properties and accelerating osseointegration in spinal fusion. Bioact. Mater. 23, 234–246. doi:10.1016/j.bioactmat.2022.11.003
Jiangsu Junhua ChinaPEEK (2016). PEEK grade and typical properties. Changzhou City, Jiangsu Province, China: China. https://en.chinapeek.com.
Khoury, J., Selezneva, I., Pestov, S., Tarassov, V., Ermakov, A., Mikheev, A., et al. (2019). Surface bioactivation of PEEK by neutral atom beam technology. Bioact. Mater. 4, 132–141. doi:10.1016/j.bioactmat.2019.02.001
Kia, C., Antonacci, C. L., Wellington, I., Makanji, H. S., and Esmende, S. M. (2022). Spinal implant osseointegration and the role of 3D printing: an analysis and review of the literature. Bioengineering 9 (3), 108. doi:10.3390/bioengineering9030108
Kubiak, K. J., Wilson, M. C. T., Mathia, T. G., and Carras, S. (2011). Dynamics of contact line motion during the wetting of rough surfaces and correlation with topographical surface parameters. Scanning 33 (5), 370–377. doi:10.1002/sca.20289
Liu, X., Han, F., Zhao, P., Lin, C., Wen, X., and Ye, X. (2017). Layer-by-layer self-assembled multilayers on PEEK implants improve osseointegration in an osteoporosis rabbit model. Nanomedicine Nanotechnol. Biol. Med. 13 (4), 1423–1433. doi:10.1016/j.nano.2017.01.011
Lu, T., Li, J., Qian, S., Cao, H., Ning, C., and Liu, X. (2016). Enhanced osteogenic and selective antibacterial activities on micro-/nano-structured carbon fiber reinforced polyetheretherketone. J. Mater. Chem. B 4 (17), 2944–2953. doi:10.1039/c6tb00268d
Lu, T., Liu, X., Qian, S., Cao, H., Qiao, Y., Mei, Y., et al. (2014). Multilevel surface engineering of nanostructured TiO2 on carbon-fiber-reinforced polyetheretherketone. Biomaterials 35 (22), 5731–5740. doi:10.1016/j.biomaterials.2014.04.003
Lu, T., Wen, J., Qian, S., Cao, H., Ning, C., Pan, X., et al. (2015). Enhanced osteointegration on tantalum-implanted polyetheretherketone surface with bone-like elastic modulus. Biomaterials 51, 173–183. doi:10.1016/j.biomaterials.2015.02.018
McLean, R. J. C., Hussain, A. A., Sayer, M., Vincent, P. J., Hughes, D. J., and Smith, T. J. N. (1993). Antibacterial activity of multilayer silver–copper surface films on catheter material. Can. J. Microbiol. 39 (9), 895–899. doi:10.1139/m93-134
Meng, X., Zhang, J., Chen, J., Nie, B., Yue, B., Zhang, W., et al. (2020). KR-12 coating of polyetheretherketone (PEEK) surface via polydopamine improves osteointegration and antibacterial activity in vivo. J. Mater. Chem. B 8 (44), 10190–10204. doi:10.1039/d0tb01899f
Mo, S., Mehrjou, B., Tang, K., Wang, H., Huo, K., Qasim, A. M., et al. (2020). Dimensional-dependent antibacterial behavior on bioactive micro/nano polyetheretherketone (PEEK) arrays. Chem. Eng. J. 392, 123736. doi:10.1016/j.cej.2019.123736
Mo, S., Zhao, F., Gao, A., Wu, Y., Liao, Q., Xie, L., et al. (2021). Simultaneous application of diamond-like carbon coating and surface amination on polyether ether ketone: towards superior mechanical performance and osseointegration. Smart Mater. Med. 2, 219–228. doi:10.1016/j.smaim.2021.07.004
Nadhif, M. H., Whulanza, Y., Istiyanto, J., and Bachtiar, B. M. (2017). Delivery of amphotericin B to Candida albicans by using biomachined lab-on-a-chip. J. Biomimetics, Biomaterials Biomed. Eng. 30, 24–30. doi:10.4028/www.scientific.net/jbbbe.30.24
Najeeb, S., Bds, Z. K., Bds, S. Z., and Bds, M. S. Z. (2016). Bioactivity and osseointegration of PEEK are inferior to those of titanium: a systematic review. J. Oral Implant. 42 (6), 512–516. doi:10.1563/aaid-joi-d-16-00072
Ourahmoune, R., Salvia, M., Mathia, T., and Mesrati, N. (2014). Surface morphology and wettability of sandblasted PEEK and its composites. Scanning 36 (1), 64–75. doi:10.1002/sca.21089
Ouyang, L., Chen, M., Wang, D., Lu, T., Wang, H., Meng, F., et al. (2019). Nano textured PEEK surface for enhanced osseointegration. ACS Biomaterials Sci. Eng. 5 (3), 1279–1289. doi:10.1021/acsbiomaterials.8b01425
Ouyang, L., Deng, Y., Yang, L., Shi, X., Dong, T., Tai, Y., et al. (2018). Graphene-oxide-decorated microporous polyetheretherketone with superior antibacterial capability and in vitro osteogenesis for orthopedic implant. Macromol. Biosci. 18 (6), e1800036. doi:10.1002/mabi.201800036
Ouyang, L., Zhao, Y., Jin, G., Lu, T., Li, J., Qiao, Y., et al. (2016). Influence of sulfur content on bone formation and antibacterial ability of sulfonated PEEK. Biomaterials 83, 115–126. doi:10.1016/j.biomaterials.2016.01.017
Peng, T.-Y., Lin, D. J., Mine, Y., Tasi, C. Y., Li, P. J., Shih, Y. H., et al. (2021). Biofilm Formation on the surface of (Poly)Ether-Ether-Ketone and in vitro antimicrobial efficacy of photodynamic therapy on peri-implant mucositis. Polymers 13 (6), 940. doi:10.3390/polym13060940
Qosim, N., Supriadi, S, Saragih, A. S., and Whulanza, Y. (2018). Surface treatments of ti-alloy based bone implant manufactured by electrical discharge machining, 22. Bogotá, Colombia: Ingenieria y Universidad, 59–70.
Raj, V., Gopakumar, A., Vaidya, G., Scott, J., Toth, M., Jagadish, C., et al. (2022). High-density individually addressable platinum nanoelectrodes for biomedical applications. Discov. Mater. 2 (1), 6. doi:10.1007/s43939-022-00027-1
Rochford, E. T., Poulsson, A., Salavarrieta Varela, J., Lezuo, P., Richards, R., and Moriarty, T. (2014). Bacterial adhesion to orthopaedic implant materials and a novel oxygen plasma modified PEEK surface. Colloids Surf. B Biointerfaces 113, 213–222. doi:10.1016/j.colsurfb.2013.09.012
Sarfraz, S., Mäntynen, P. H., Laurila, M., Rossi, S., Leikola, J., Kaakinen, M., et al. (2022). Comparison of titanium and PEEK medical plastic implant materials for their bacterial biofilm formation properties. Polymers 14 (18), 3862. doi:10.3390/polym14183862
Scholz, J., Nocke, G., Hollstein, F., and Weissbach, A. (2005). Investigations on fabrics coated with precious metals using the magnetron sputter technique with regard to their anti-microbial properties. Surf. Coatings Technol. 192 (2), 252–256. doi:10.1016/j.surfcoat.2004.05.036
Stachowiak, G. W., and Batchelor, A. W. (2014). Engineering tribology. Fourth;4;4th. Oxford: Butterworth-Heinemann.
Sun, M., Watson, G. S., Zheng, Y., Watson, J. A., and Liang, A. (2009). Wetting properties on nanostructured surfaces of cicada wings. J. Exp. Biol. 212 (19), 3148–3155. doi:10.1242/jeb.033373
Sunarso, , Tsuchiya, A., Fukuda, N., Toita, R., Tsuru, K., and Ishikawa, K. (2018). Effect of micro-roughening of poly(ether ether ketone) on bone marrow derived stem cell and macrophage responses, and osseointegration. J. Biomaterials Sci. Polym. Ed. 29 (12), 1375–1388. doi:10.1080/09205063.2018.1461448
Tsougeni, K., Vourdas, N., Tserepi, A., Gogolides, E., and Cardinaud, C. (2009). Mechanisms of oxygen plasma nanotexturing of organic polymer surfaces: from stable super hydrophilic to super hydrophobic surfaces. Langmuir 25 (19), 11748–11759. doi:10.1021/la901072z
Wakelin, E. A., Yeo, G. C., McKenzie, D. R., Bilek, M. M. M., and Weiss, A. S. (2018). Plasma ion implantation enabled bio-functionalization of PEEK improves osteoblastic activity. Apl. Bioeng. 2 (2), 026109. doi:10.1063/1.5010346
Wang, H. B., Wei, Q. F., Wang, J. Y., Hong, J. H., and Zhao, X. Y. (2008). Sputter deposition of nanostructured antibacterial silver on polypropylene non-wovens. Surf. Eng. 24 (1), 70–74. doi:10.1179/174329408x277493
Wang, S., Deng, Y., Yang, L., Shi, X., Yang, W., and Chen, Z. G. (2018). Enhanced antibacterial property and osteo-differentiation activity on plasma treated porous polyetheretherketone with hierarchical micro/nano-topography. J. Biomaterials Sci. Polym. Ed. 29 (5), 520–542. doi:10.1080/09205063.2018.1425181
Wang, X., Lu, T., Wen, J., Xu, L., Zeng, D., Wu, Q., et al. (2016). Selective responses of human gingival fibroblasts and bacteria on carbon fiber reinforced polyetheretherketone with multilevel nanostructured TiO2. Biomaterials 83, 207–218. doi:10.1016/j.biomaterials.2016.01.001
Whulanza, Y., Arafat, Y., Rahman, S., Utomo, M., and Kassegne, S. (2022). On-chip testing of a carbon-based platform for electro-adsorption of glutamate. Heliyon 8 (5), e09445. doi:10.1016/j.heliyon.2022.e09445
Whulanza, Y., Harahap, I. S., Istiyanto, J., Kurniawati, T., and Rahyussalim, A. J. (2019). Realization of photo-curing gelatin hydrogel using a commercial projector for culturing mesenchymal cells. Int. J. Adv. Sci. Eng. Inf. Technol. 9, 1643–1648. doi:10.18517/ijaseit.9.5.10215
Whulanza, Y., Nadhif, H., Istiyanto, J., Supriadi, S., and Bachtiar, B. (2016). PDMS surface modification using biomachining method for biomedical application. J. Biomimetics, Biomaterials Biomed. Eng. 26, 66–72. doi:10.4028/www.scientific.net/jbbbe.26.66
Xie, H., Zhang, C., Wang, R., Tang, H., Mu, M., Li, H., et al. (2021). Femtosecond laser-induced periodic grooves and nanopore clusters make a synergistic effect on osteogenic differentiation. Colloids Surfaces B Biointerfaces 208, 112021. doi:10.1016/j.colsurfb.2021.112021
Keywords: PEEK biomaterial, sandblasting, platinum sputtered coating, implant, cell viability
Citation: Faadhila A, Taufiqurrakhman M, Katili PA, Rahman SF, Lestari DC and Whulanza Y (2024) Optimizing PEEK implant surfaces for improved stability and biocompatibility through sandblasting and the platinum coating approach. Front. Mech. Eng 10:1360743. doi: 10.3389/fmech.2024.1360743
Received: 24 December 2023; Accepted: 26 February 2024;
Published: 19 March 2024.
Edited by:
Alessandro Ruggiero, University of Salerno, ItalyReviewed by:
Yoshitaka Nakanishi, Kumamoto University, JapanJitendra Kumar Katiyar, Chitkara University, India
Copyright © 2024 Faadhila, Taufiqurrakhman, Katili, Rahman, Lestari and Whulanza. This is an open-access article distributed under the terms of the Creative Commons Attribution License (CC BY). The use, distribution or reproduction in other forums is permitted, provided the original author(s) and the copyright owner(s) are credited and that the original publication in this journal is cited, in accordance with accepted academic practice. No use, distribution or reproduction is permitted which does not comply with these terms.
*Correspondence: Yudan Whulanza, yudan.whulanza@ui.ac.id