Effectiveness of low-intensity pulsed ultrasound on osteoarthritis: molecular mechanism and tissue engineering
- 1Orthopedics and Sports Medicine Center, The Affiliated Suzhou Hospital of Nanjing Medical University, Suzhou, China
- 2Gusu School, Nanjing Medical University, Suzhou, China
Osteoarthritis (OA) is distinguished by pathological alterations in the synovial membrane, articular cartilage, and subchondral bone, resulting in physical symptoms such as pain, deformity, and impaired mobility. Numerous research studies have validated the effectiveness of low-intensity pulsed ultrasound (LIPUS) in OA treatment. The periodic mechanical waves generated by LIPUS can mitigate cellular ischemia and hypoxia, induce vibration and collision, produce notable thermal and non-thermal effects, alter cellular metabolism, expedite tissue repair, improve nutrient delivery, and accelerate the healing process of damaged tissues. The efficacy and specific mechanism of LIPUS is currently under investigation. This review provides an overview of LIPUS’s potential role in the treatment of OA, considering various perspectives such as the synovial membrane, cartilage, subchondral bone, and tissue engineering. It aims to facilitate interdisciplinary scientific research and further exploration of LIPUS as a complementary technique to existing methods or surgery. Ongoing research is focused on determining the optimal dosage, frequency, timing, and treatment strategy of LIPUS for OA. Additional research is required to clarify the precise mechanism of action and potential impacts on cellular, animal, and human systems prior to its integration into therapeutic applications.
1 Introduction
Osteoarthritis (OA) is the most prevalent degenerative joint disease affecting the elderly population, imposing a substantial burden on patients, families, and society. The primary risk factors comprise advanced age, obesity, female gender, genetic predisposition, and severe joint injuries (1). OA not only induces joint inflammation, pain, stiffness, and impaired function, but in advanced cases, it can also lead to muscle wasting, joint malformation, and ultimately, disability. Additionally, it can result in a cascade of complications, such as cardiovascular events, deep vein thrombosis in the lower extremities, hip fractures, and all-cause mortality (2). Consequently, the development of effective prevention and treatment strategies for OA has become a crucial challenge in the field of public health management.
Currently, conservative management of OA predominantly involves the use of symptomatic therapies, such as anti-inflammatory analgesics and cartilage-supporting nutrition. Although these interventions may provide temporary relief from pain, they are unable to arrest or reverse the progression of OA. Extended pharmacological treatment plans can result in negative side effects such as gastrointestinal issues, potential kidney damage, and the risk of developing a dependency on medication (3, 4). In addition, joint replacement surgery is frequently utilized to address advanced OA, despite its invasive nature, financially burdensome, likelihood of complications, and the finite durability of artificial joint prostheses. Despite years of research, the etiology of this prevalent and complex disease remains elusive, and there is a notable absence of efficacious treatments. Consequently, there is a pressing need for comprehensive exploration of a secure and efficient supplementary approach for managing OA. Since receiving initial approval from the US Food and Drug Administration (FDA) in 1994 for fracture treatment, therapeutic ultrasound has been acknowledged as a non-invasive, safe and non-carcinogenic modality in the management of musculoskeletal disease (5). This modality is known to enhance local circulation and lymphatic circulation, improve metabolism, promote tissue regeneration and nutrient status, and decrease muscle and connective tissue tension as well as sensory nerve excitability. Recently, low-intensity pulsed ultrasound (LIPUS) has emerged as a therapeutic modality for the treatment of OA. LIPUS is characterized by pulsed waves with frequencies ranging from 1 to 3 MHz and intensities below 1 w/cm2, making it a non-invasive physical stimulus for therapeutic purposes. Due to its low intensity and pulsed output mode, LIPUS exhibits minimal thermal effects, allowing for the targeted delivery of acoustic energy to tissues. LIPUS emits low intensity ultrasound in a pulsed wave mode, delivering mechanical energy to targeted tissue through high-frequency pressure waves, resulting in vibrations and collisions induced by the periodic acoustic waves. LIPUS has emerged as a promising therapeutic approach originally utilized for skeletal muscle disorders, demonstrating efficacy in enhancing fracture healing and promoting recovery from tendon and ligament injuries. Numerous research studies have demonstrated the significant impact of LIPUS on cellular metabolism and tissue repair in OA.
This review seeks to consolidate the existing body of research on the use of LIPUS in the treatment of OA over the past several decades, with a specific focus on application, outcomes and mechanisms of action on different affected tissues. The ultimate goal is to enhance the clinical effectiveness of LIPUS in the management of OA by elucidating its therapeutic principles and effects on synovial membranes, cartilage, and subchondral bones.
2 Definition and mechanism of LIPUS
2.1 Definition of LIPUS
LIPUS is a therapeutic modality that utilizes ultrasonic pulsed waves to address various pathological conditions by leveraging mechanical, thermal, physical, chemical, and biochemical mechanisms, while preserving the integrity and viability of human tissues. For applications in both “in vitro” and non-invasive settings, LIPUS is subjected to sonication by diverse generators, transduced, and administered to the targeted treatment site. Currently, as shown in Figure 1A, LIPUS devices typically operate at frequencies of 1, 1.5, or 3 MHz, with some devices offering the option of 0.75 MHz for deeper lesions. LIPUS equipment commonly used today typically emits ultrasonic waves at a pulse rate of 1,000 Hz with a duty cycle of 20%, resulting in 200 μs of ultrasonic action time and 800 μs of intermittent time, and 1,000 cycles per second. The dosage of LIPUS can be subdivided into three categories: low dose (<1 W/cm2), medium dose (1–2 W/cm2), and high dose (2–3 W/cm2). In the majority of studies, LIPUS employs a dosage of 30–60 mW/cm2, a duty cycle of 20%, and a frequency of 1.5 MHz.
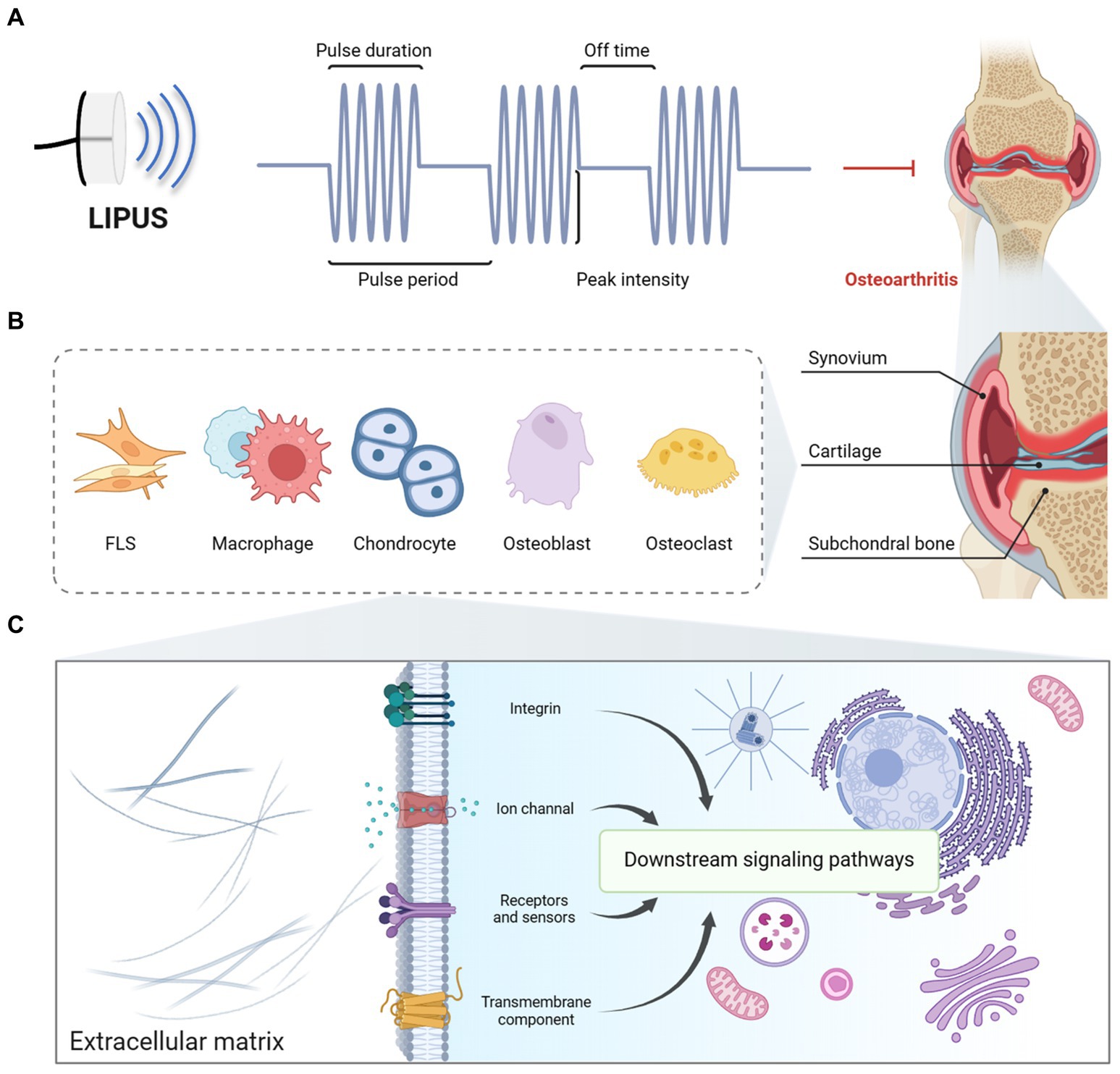
Figure 1. The typical features and potential mechanism of LIPUS. (A) Overall, the duty cycle of LIPUS in existing studies is 20%, the frequency ranges from 1 to 3 MHz, and the intensity ranges from 0.02 to 1 W/cm2. (B) LIPUS acts on FLSs, macrophages, chondrocytes, osteoblasts, and osteoclasts in the synovium, cartilage, and subchondral bone. (C) LIPUS facilitates the mediation of integrin, ion channel, and other cellular processes through mechano-biochemical signal transduction. This regulation subsequently influences downstream signaling pathways, ultimately impacting the pathophysiology of OA in the synovium, cartilage, and subchondral bone. Figures created with BioRender.com.
2.2 Cavitation and mechanical effects of LIPUS
LIPUS primarily operates via both thermal and non-thermal mechanisms. LIPUS emits periodic mechanical sound waves that penetrate tissues and cells, inducing vibration and collisions (6) (Figure 1B). By eliciting minimal thermal effects and substantial non-thermal effects within the targeted tissue, it modulates cellular metabolism, accelerates tissue healing processes, and ameliorates cellular ischemia and hypoxia, ultimately enhancing tissue nutrition and promoting tissue repair (7). LIPUS produces minimal thermal impact, as evidenced by a modest temperature of 0.5°C after a 10-min exposure in both in vivo and ex vivo conditions. The primary mechanisms of action for LIPUS are non-thermal in nature, specifically cavitation and mechanical effects, which have garnered significant attention from researchers.
2.2.1 Cavitation effects of LIPUS
The ultrasonic cavitation effect mainly encompasses a sequence of dynamic events in which small gas-core cavitation bubbles undergo expansion and collapse in response to acoustic pressure generated by ultrasonic waves within the liquid medium. This phenomenon manifests in two distinct manifestations: stable and transient cavitation. Transient cavitation, which is predominantly utilized in the treatment of cancer and palliative care (8), involves the unstable oscillation of bubbles that expand to 2–3 times their resonance size before rapidly collapsing, releasing substantial energy that causes cellular damage and rapid tissue destruction. Both types of cavitation serve as the principal mechanisms responsible for ultrasound’s biological effects on targeted tissues. Nonetheless, the maintenance of a stable cavitation effect appears to be more efficacious in achieving favorable outcomes in ultrasound treatment.
2.2.2 Mechanical effects of LIPUS
Mechanical effects mainly include acoustic flow and mass transfer enhancement. Acoustic flow pertains to the circulation that occurs around a liquid when a cavitation bubble vibrates and can be divided into macroscopic and microscopic flows. Macroscopic flow refers to the substantial movement of a fluid in alignment with the propagation of an ultrasound wave within a liquid. Comparatively, microscopic flows are characterized by a small vortices that form in close proximity to the point of oscillation. Furthermore, the mechanical impact of macroscopic flows is comparatively weaker than that of microscopic flows. Microscopic flows are a consequence of cavitation and have the potential to alter membrane permeability, influence diffusion ion rates, impact protein synthesis, and modulate cell secretion processes (9). In the realm of employing non-thermal effects in applications of LIPUS it is crucial to sustain stable cavitation and acoustic flows.
Improving mass transfer is a crucial component of the non-thermal effects of LIPUS. Ultrasound plays a key role in augmenting the movement of the fluid medium by increasing mass transfer and reaction rates. This phenomenon is particularly noticeable in cells and tissues treated with LIPUS, where it predominantly affects the cell membrane, cytosol, and boundary layers. The oscillation of bubbles creates micro-flows within the encompassing sound field, guiding reagents toward enzyme active sites or cells, thereby aiding in the release of biological substances into the medium and leading to discernible biological outcomes (10).
2.3 Conversion of mechanical signal into biochemical signaling
Acoustic signals elicit various interactions with tissues, including absorption, reflection, refraction, and transmission of mechanical pulse energy. Variations in acoustic impedance among tissues lead to differing absorption and propagation efficiencies of LIPUS, thereby impacting its therapeutic efficacy. LIPUS specifically targets tissues to induce a cavitation effect, facilitating enhanced acoustic flow and mass transfer, ultimately eliciting biophysical effects within the desired tissue. Currently, there is a lack of comprehensive understanding regarding the mechanisms by which tissues and cells perceive and respond to acoustic energy, leading to the translation of intercellular signals that regulate biological functions and reshape the microenvironment. Some researchers have sought to elucidate these biological processes through the framework of cellular mechanical transduction, as illustrated in Figure 1C. LIPUS, acting as a non-invasive form of mechanical stimulation, has the ability to dynamically modulate the cytoskeleton and induce extracellular matrix (ECM)-cytoplasm signal transduction by interacting with receptors such as integrins and ion channels located on the cell membrane. Subsequently, these signals are transmitted through the cytoskeleton to the nucleus, ultimately impacting gene expression and modifications, thereby initiating downstream cellular responses.
3 Mechanism of action of LIPUS on OA
3.1 Effects of LIPUS on synovium
The synovium plays a crucial role in the joint structure by working in conjunction with cartilage through an immune-inflammatory network, thereby contributing to the maintenance of joint cavity homeostasis (11). The various types of synoviocytes, such as fibroblast-like synoviocytes (FLSs), macrophages, and synovial stem cells, secrete synovial fluid, vesicles, and cytokines to facilitate lubrication, immunity and inflammation regulation, creating a specialized homeostatic microenvironment within the synovial membrane and joint cavity. Chronic low-grade synovial inflammation is present in all stages of OA and is closely associated with the clinical symptoms experienced by patients, including synovial hypertrophy, abnormal growth, vascular proliferation, and inflammatory reactions (12). The disruption of joint integrity during OA is attributed to an imbalance in the synovial inflammatory microenvironment, caused by the aberrant secretion of inflammatory factors within the synovium.
Recent research findings indicate that LIPUS may have the potential to serve as an efficacious intervention for mitigating synovial inflammation and fibrosis (Figure 2 and Table 1). Early implementation of LIPUS has been shown to delay the degradation of cartilage by decreasing synovial inflammation, suppressing MMP13 expression, and enhancing Col II expression within the cartilage (13). Several studies have demonstrated that LIPUS has the ability to suppress the formation of Toll-related receptor-myeloid differentiation factor in OA animal models and cell experiments, thereby controlling the synovial inflammatory response induced by lipopolysaccharide (LPS) (14). Additionally, LIPUS has been shown to effectively inhibit the proliferation, growth, and DNA cleavage of synoviocytes through the modulation of cytokines such as nitric oxide synthase (iNOS) and interleukin-1 beta (IL-1β). Moreover, in comparation to the control group, the LIPUS treatment group exhibited significantly reduced levels of inflammatory cell infiltration, synovial hyperplasia, pannus formation, and cartilage destruction, ultimately delaying the degeneration of OA articular cartilage (15). Furthermore, LIPUS was found to enhance the synthesis of hyaluronan (HA) and suppress the expression of hyaluronidase (HYAL) 2 HYAL2, resulting in the accumulation of high molecular weight HA (16). Nakamura et al. (17) have demonstrated that LIPUS intervention could effectively reduce knee tissue damage and decrease the levels of COX-2 positive cells in the knee compared to a control group in MRL/lpr mice. Additionally, LIPUS has been found to upregulate the phosphorylation of focal adhesion kinase (FAK) in synoviocytes and inhibit FAK phosphorylation to downregulate ERK, JNK, and p38 phosphorylation. These results suggest that LIPUS may regulate synoviocyte apoptosis and survival via the integrin/FAK/MAPK pathway (18).
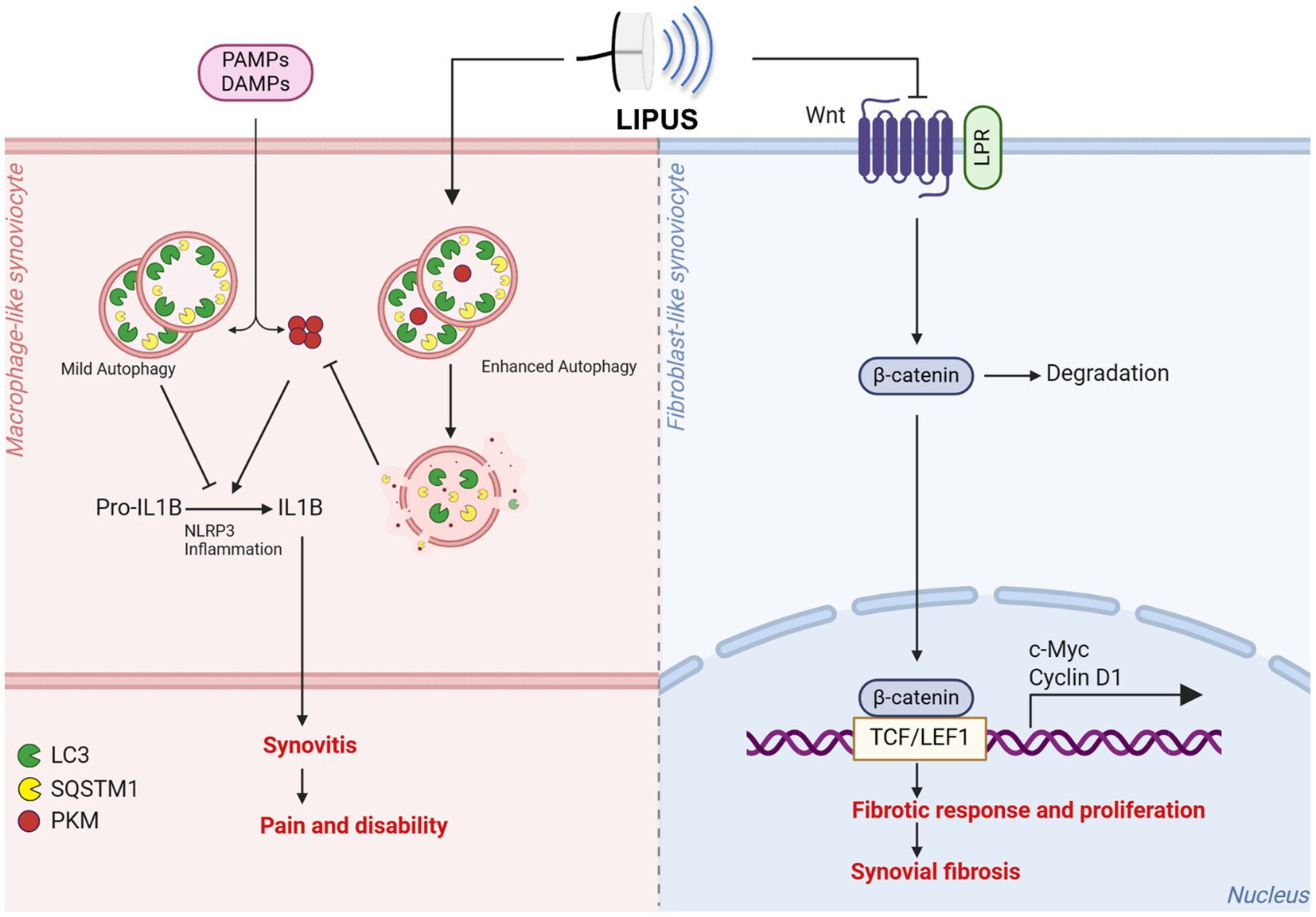
Figure 2. LIPUS holds potential as an effective approach to counteract synovial inflammation and fibrosis. LIPUS demonstrates the ability to mitigate synovial inflammation and joint dysfunction through the enhancement of autophagy in macrophage-like synoviocytes and the inhibition of mature IL-1B production. Additionally, LIPUS has the capacity to modulate the Wnt/β-catenin signaling pathway in fibroblast-like synoviocytes, facilitating β-catenin degradation and nuclear translocation, regulating downstream gene transcription, and ultimately impeding synovial fibrosis. Figures created with BioRender.com.
FLSs are essential mesenchymal components in synovial tissue and their dysregulated activation and resulting alterations in biological functions are significant contributors to OA pathogenesis. In vitro studies have shown that LIPUS can mitigate TGF-β1-induced fibrotic reactions and FLS proliferation. Furthermore, LIPUS has been found to suppress the Wnt/β-catenin signaling pathway and its associated downstream proteins in the synovial tissue of destabilization of the medial meniscus (DMM) mouse model. Collectively, the combined effects of this dual mechanism effectively inhibit synovial fibrosis and the downstream proteins associated with the Wnt/β-catenin pathway (19). Macrophages are identified as a crucial cell type within the synovium, playing a central role in the pathogenesis of OA. Studies have demonstrated a high concentration of activated macrophages in the affected joints of OA patients, which release pro-inflammatory factors that contribute to the acceleration of disease progression. The intervention of LIPUS has been shown to decrease the recruitment of macrophages. Following a 2-day treatment with LIPUS, it was observed that while the number of M1 macrophages decreased in the injury model, the number of M2 macrophages increased (20). This suggests that LIPUS plays a role in influencing on macrophage polarization, promoting the transition toward anti-inflammatory M2 macrophages and consequently reducing inflammatory responses in the vicinity of the joints. After a 4-week LIPUS intervention in a rat model of post-traumatic arthritis affecting the knee joint, there was a decrease in leukocyte infiltration within the synovium. Furthermore, LIPUS stimulation resulted in a significant reduction in the number of CD68+ macrophages and restricted their localization within the subintimal synovium (21). Moreover, LIPUS was found to enhance autophagy levels and expedite the formation of the SQSTM1 (sequestosome1)-PKM (pyruvate kinase, muscle) complex in macrophages treated with LPS-ATP. Through the regulation of SQSTM1-dependent autophagic degradation of muscle-type pyruvate kinase 2 in macrophages, LIPUS effectively suppresses the production of mature IL-1β, consequently improving synovial inflammation and gait performance in animal models (22).
3.2 Effects of LIPUS on cartilage
Degeneration of articular cartilage is a key pathological feature of OA and plays a pivotal role in the diagnosis of OA. Multiple biological factors, such as inflammation and mechanical forces, can disrupt the balance of chondrocytes, leading to decreased synthesis of the ECM of cartilage and diminished resistance of articular cartilage to stress, ultimately causing injury (Figure 3 and Table 2).
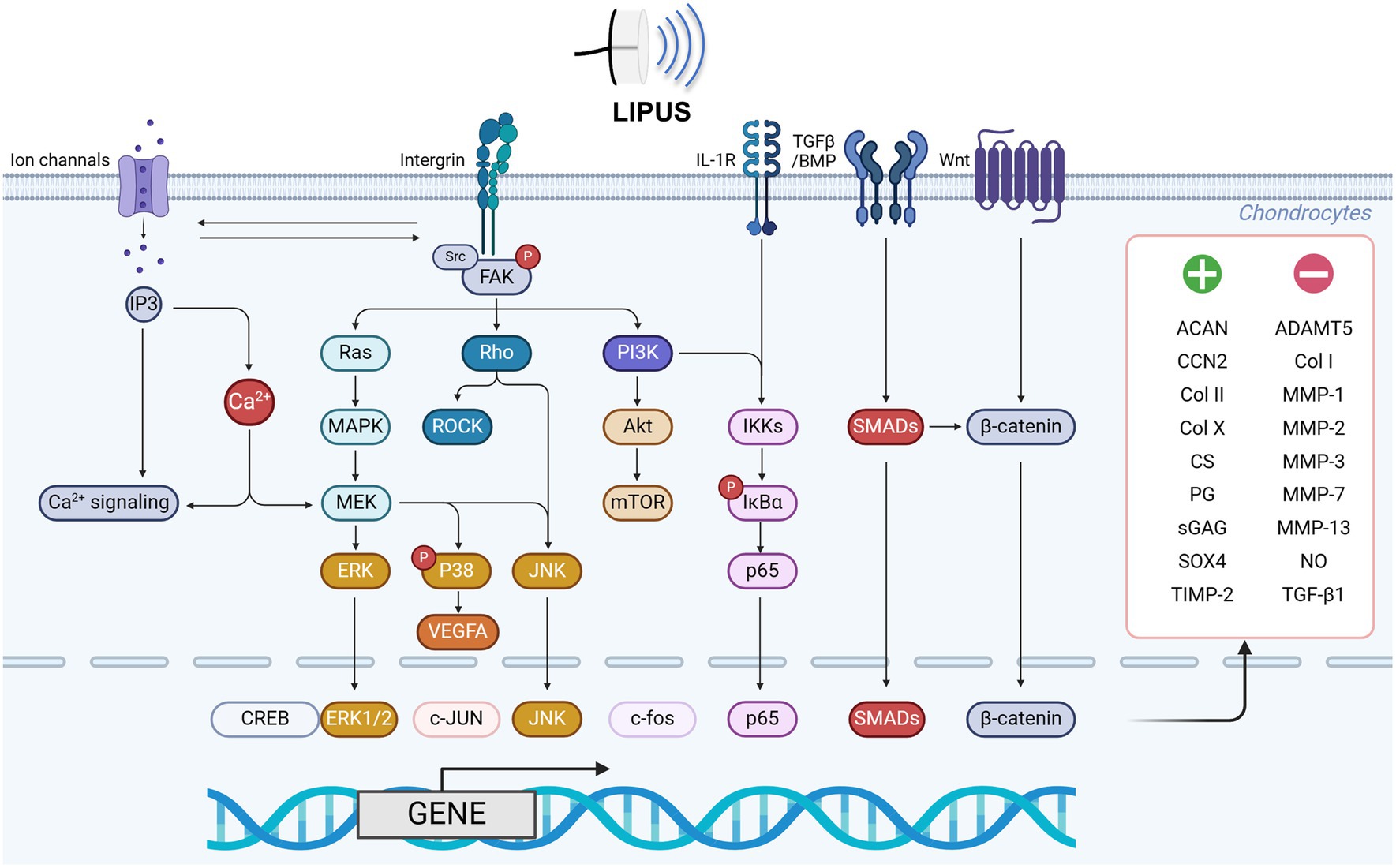
Figure 3. LIPUS regulates chondrocyte function via multiple pathways. LIPUS exerts regulatory effects on chondrocyte proliferation, differentiation, and metabolism through modulation of ion channels and integrin-related FAK/PI3K signaling pathways. Specifically, the activation of ion channels facilitates calcium influx, cytoskeletal regulation, and MEK/ERK pathway activation. Additionally, LIPUS suppresses MMP13 and p38 phosphorylation in healthy chondrocytes by activating the mechanosensitive integrin/FAK pathway and modulating the Ras/MEK/ERK, Rho/ROCK, and FAK/PI3K signaling cascades. In OA chondrocytes, LIPUS may impede chondrocyte proliferation and differentiation by inhibiting p38 phosphorylation. Furthermore, LIPUS modulates the catabolic and inflammatory effects of IL-1β by inhibiting the binding of IL-1β to the IL-1β receptor complex and the downstream NF-κB pathway. Conversely, LIPUS was found to regulate TGF-β/BMP receptors on the chondrocyte membrane, activating the SMADs pathway and influencing the downstream Wnt/β-catenin signaling pathway to exert its biological regulatory functions. Figures created with BioRender.com.
Cartilage tissue demonstrates sensitivity to mechanical stimuli, with LIPUS having the potential to influence the proliferation and differentiation of chondrocytes. Studies have shown that LIPUS can transmit sustained wave energy and modulate the increased expression of TGF-β, thereby promoting the proliferation of bovine chondrocytes and the upregulation of cartilage-related markers such as MMP-1 and Col I (23). Additionally, research has indicated (24) that the CCN protein family 2 may play a role in enhancing the proliferation and differentiation of articular cartilage and osteoblasts affected by OA, thereby potentially supporting the proliferation of injured articular cartilage. Khanna et al. (25) conducted experiments using rabbit and dog models with articular cartilage defects, demonstrating that LIPUS significantly improved cartilage repair, morphological changes, and chondrocyte proliferation. In a separate study (26), LIPUS was applied to stimulate articular chondrocytes in OA rat models, resulting in the synthesis of chondrocyte DNA, upregulation of the ERK signaling pathway and β1 integrin expression, and enhanced chondrocyte proliferation and differentiation through the activation of focal adhesion kinase (FaK) signaling. The inhibition of FaK has been shown to impede lipase-mediated cell proliferation and differentiation in vitro, as well as reduce inflammation in OA mice (27). Xie et al. (28) further confirmed that LIPUS enhanced the proliferation of BMSC through the regulation of phosphoinositide 3-kinase (PI3K)/protein kinase B (AKt) pathway proteins and the upregulation of cyclin D1 expression. Adipose-derived stem cells also exhibited differentiation into chondrocytes following 2 weeks of LIPUS stimulation. These collective findings suggest that LIPUS may have the potential to delay OA progression by promoting chondrocyte proliferation in injured joints and accelerating soft tissue repair.
In the realm of OA cartilage metabolism, the degradation of joint tissue occurs as a result of a imbalance between catabolic and anabolic processes. Specifically, there is a notable increase in ECM degradation of OA articular cartilage, with MMPs playing a pivotal role in this process (29). Studies have shown that LIPUS stimulation of OA articular cartilage can attenuate the expression of catabolic enzymes, such as MMP-13, and the platelet response protein disintegrant and metallopeptidase 5 in chondrocytes (30). Moreover, research has demonstrated that ultrasound has the capability to initiate mechanoconductive cell signaling pathways, leading to the stimulation of chondrocytes for the production of Col II, integrin β1 (31), and proteoglycan, as well as mesenchymal stem cells to facilitate the restoration of damaged tissues in OA joints (32). LIPUS has been shown to promote collagen synthesis and aggrecan remodeling, thereby providing therapeutic benefits via the activation of ERK1/2, enhancement of hypertrophic chondrocytes, inhibition of endochondral osteogenesis, and induction of ECM regeneration (33). LIPUS can also inhibit disruptions in cartilage ECM metabolism and chondrocyte hypertrophy mediated by VEGF during OA progression by regulating VEGFA expression in OA chondrocytes and suppressing p38 MAPK activity (34). Kojima et al. (35) abserved a notable reduction in Osteoarthritis Research Society International (OARSI) scores was noted in the group treated with LIPUS irradiation. Conversely, the non-LIPUS group exhibited extensive areas of dual positivity for collagen II and denatured collagen detection reagent (DCDR), while the LIPUS group displayed a limited number of DCDR-positive areas. Furthermore, a significant decrease in macrophage numbers within the articular capsule was observed in the LIPUS irradiated group. Cook et al. (36) further established that extended LIPUS treatment duration enhanced joint function and facilitated joint function recovery in OA. Furthermore, LIPUS stimulation of OA cartilage led to a notable increase in Col II synthesis and mRNA expression, thereby expediting soft tissue repair. Wu et al. (37) indicated that LIPUS modulated primary cilia expression and enhanced synthetic matrix metabolism in articular chondrocytes, with a concomitant association with primary cilia. Furthermore, LIPUS demonstrated a significant impact on OA by activating Transient Receptor Potential Vanilloid 4 (TRPV4), prompting calcium influx, and facilitating nuclear translocation of NF-κB to regulate gene transcription related to synthetic matrix production. Inhibition of TRPV4 influenced primary cilia expression following LIPUS stimulation, while depletion of primary cilia similarly affected TRPV4 activity.
Tanaka et al. (38) showed that the level of autophagy is modified in OA-affected cartilages, with a decrease in cartilage damage and an increase in the expression levels of chondrocyte autophagy markers Beclin-1, ATG7, fibroblast growth factor 18 and fibroblast growth factor receptor 4 observed under the influence of LIPUS. LIPUS was found to stimulate the release of MSC exosomes through the activation of autophagy, thereby augmenting the beneficial impact of MSCs on OA cartilage by inhibiting GW4869, a known exosome release inhibitor (39). In a study by Wang et al. (40), it was shown that LIPUS had a discernible influence on autophagy in chondrocytes, leading to the conclusion that autophagy activation could facilitate the chondrogenic differentiation of mesenchymal stem cells. LIPUS was also found to reduce the expression of Yes-associated protein (YAP) through restoration of impaired autophagy capacity and inhibition of the interaction between YAP and receptor-interacting protein kinase 1 (RIPK1), consequently leading to a delay in the advancement of OA. Results from animal experimentation demonstrated that LIPUS could effectively impede cartilage degeneration and mitigate the progression of OA (41).
In summary, LIPUS regulates chondrocyte proliferation, differentiation, autophagy levels, and the balance between synthesis and catabolism through complex signaling pathways to enhance the function of OA joints and promote recovery.
3.3 Effects of LIPUS on subchondral bone
During the early stages of OA, patients undergo subchondral bone loss, with progressive subchondral osteopenia developing as the disease advances. Subchondral sclerosis disrupts the normal mechanical distribution of loads within the joint, hindering the ability of the overlying cartilage to effectively cushion pressure. Research has demonstrated that osteoblasts play a crucial role in the inflammatory response and can produce chemokines that trigger inflammatory reactions (42) (Table 3). Given the sensitivity of osteoblasts to mechanical stimulation, the use of LIPUS has been considered an effective treatment for subchondral OA.
Yamaguchi et al. (43) conducted a study examining the synergistic effects of LIPUS and MSC therapy for OA, finding that the combined intervention result in greater improvements in cartilage repair scores and bone volume (BV)/tissue volume (TV) compared to their individual applications. Yılmaz et al. (44) developed a monoiodoacetate (MIA) rat model to investigate the systemic regenerative effects of LIPUS on cartilage and subchondral bone, demonstrating increased bone mineral density (BMD) values. Lee et al. (45) indicated that early intervention with LIPUS treatment can provide protective benefits against the advancement of OA, such as decreased tissue degradation, alleviated pain symptoms, enhanced subchondral bone microarchitecture, and reduced sensory innervation. Additionally, regular LIPUS therapy appears to inhibit osteoclastogenesis, potentially through its impact on sensory innervation suppression in OA. A separate study (46) investigated the effects of LIPUS on changes in articular cartilage and subchondral bone under both normal and functionally disused conditions. The study found that OA was associated with reduced cartilage thickness and glycosaminoglycan sulfate content, as well as increased thickness of the subchondral cortical bone plate and heightened subchondral trabecular bone density during periods regular joint use. Furthermore, the density and spatial distribution of chondrocytes exhibit an increase during the progression of OA, leading to alterations in both cartilage degradation and subchondral bone structure. These changes are associated with a decline in mechanical properties of osteoarthritic cartilage, which can be significantly alleviated by LIPUS treatment, particularly under conditions of regular joint loading. In summary, LIPUS contributes to mitigating cartilage degeneration and subchondral alterations during the progression of OA.
4 LIPUS and tissue engineering
Stem cells and tissue engineering approaches are key areas of study in regenerative medicine, particularly in the context of regeneration. The combination of stem cells and tissue engineering techniques offers the potential to create cartilage tissue that closely resembles native hyaline cartilage. The essential components of artificial cartilage tissue engineering include seed cells, scaffolds, and growth factors. This review provides a comprehensive analysis of the application of LIPUS in cartilage tissue engineering, focusing on these critical elements (Figure 4 and Table 4).
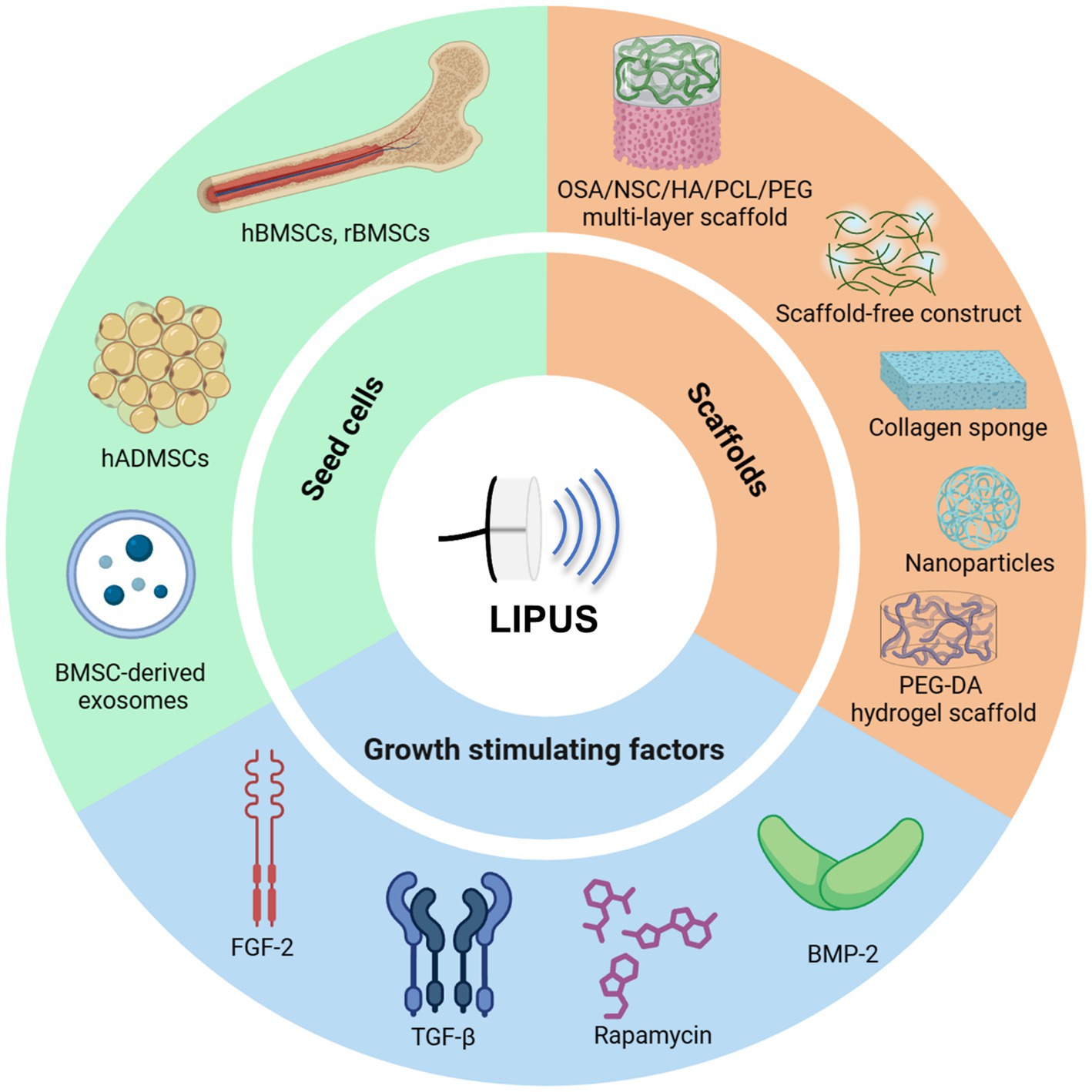
Figure 4. Tissue engineering, particularly with LIPUS, has witnessed significant advancements and is now extensively employed for joint repair. Researchers have identified bone marrow and adipose-derived mesenchymal stem cells along with their exosomes, induced their differentiation into specialized cells, developed diverse biocompatible scaffolds, incorporated various growth factors to enhance the synergistic effects of tissue engineering technology and LIPUS, ultimately facilitating OA tissue repair. Figures created with BioRender.com.
4.1 Seed cells
Selecting the appropriate seed cells is imperative in the development of an optimal tissue-engineered articular cartilage. The chosen cells must possess robust capacities for proliferation and differentiation, enabling them to mature while preserving the chondrocyte phenotype. Mesenchymal stem cells are a favorable choice for seed cell selection in tissue engineering applications due to their accessibility from multiple sources, such as bone marrow, adipose tissue, periosteum, synovial fluid, dermis and blood, all of which provide substantial potential for proliferation and differentiation.
Nasb et al. (47) conducted a study with 96 OA patients, who were divided into three groups in a 1: 1:1 ratio to receive intraarticular injections of human adipose-derived mesenchymal stem cells (HADMSCs) with LIPUS, HADMSCs with sham LIPUS, or normal saline with LIPUS. They observed that LIPUS enhanced the tissue repair function in MSCs. Xia et al. (48) discovered that LIPUS increased the expression of Stromal cell-derived factor-1 (SDF-1) and C-X-C chemokine receptor type 4 (CXCR4) proteins, thereby promoting MSC migration. They also found that autophagy inhibitors and agonists could influence this effect. The findings from in vivo experiments revealed that LIPUS significantly enhanced the therapeutic effects of MSCs on OA by promoting cartilage repair. This effect was found to be modulated by specific inhibitors. Through the activation of autophagy, LIPUS facilitated the migration of MSC and strengthened their protective effects on OA cartilage. In a study by Yamaguchi et al. (43), the combined treatment of LIPUS and MSC injection was shown to improve cartilage repair and promote subchondral bone reconstruction in osteochondral defects. Additionally, MSC injection was found to enhance cartilage repair scores, while LIPUS irradiation increased BV/TV. The synergistic effects of combined treatment involving MSC injection and LIPUS irradiation have been shown to enhance cartilage repair and BV/TV improvement. This combined approach is more effective in promoting concurrent cartilage repair and subchondral reconstruction compared to either treatment alone. Furthermore, LIPUS has been found to augment the regenerative effects of BMSC-derived exosomes on OA cartilage by suppressing inflammation, stimulating chondrocyte proliferation, and enhancing cartilage matrix synthesis, potentially through the inhibition of the NF-κB pathway activation induced by IL-1β (49).
4.2 Scaffolds
Scaffolds play a role in cartilage tissue engineering by offering a structure framework for the adhesion, growth, and specialization of seed cells, necessitating characteristics such as biocompatibility, biodegradability, and biomechanical stability. Biocompatibility ensures the scaffold’s compatibility with the surrounding biological milieu, while biodegradability facilitates gradual decomposition as new tissue develops. Furthermore, it is imperative for the biomechanical properties of the scaffold to closely resemble those of natural cartilage in order to facilitate structural support during the process of regeneration. Consequently, scaffolds serve as a conducive environment for seed cells to flourish, thereby promoting the synthesis of regenerated hyaline cartilage and facilitating the successful repair of cartilage defects.
The utilization of three-dimensional (3D) printing technology and ultrasound therapy has garnered significant interest in the field of cartilage tissue engineering. Takeuchi et al. (50) employed type-I honeycomb collagen sponges as a 3D scaffold for chondrocyte culture, observing enhanced chondrocyte proliferation and Col IX synthesis following LIPUS stimulation via the integrin/PI3K/Akt signaling pathway. Uenaka et al. (51) developed a semi-open static culture system to enhance the generation of scaffold-free constructs from monolayer-cultured chondrocytes, with the additional stimulation of ECM production through LIPUS. Aliabouzar et al. (52) investigated the seeding of human mesenchymal stem cells (hMSCs) on 3D printed poly-(ethylene glycol)-diacrylate (PEG-DA) scaffolds with varying pore sizes. Their findings indicated that square porous scaffolds exhibited superior effects on cell proliferation and chondrogenic differentiation compared to solid or hexagonal scaffolds, showing a significant increase in the production of GAG, Col II, and other anabolic products after 24 h of LIPUS stimulation. Researchers combined LIPUS with lip-coated microbubbles to improve ultrasound imaging and drug delivery, as well as to stimulate cell growth and chondrogenic differentiation (53). Babaei et al. (54) cultured chondrocytes on a 3D printed polyurethane scaffold infused with gelled glue, hyaluronic acid and glucosamine, and observed that LIPUS treatment enhanced cell proliferation, viability, and gene expression related to chondrocyte phenotype. Chen et al. (55) conducted optimization and design a single-layer integrated multi-layer functional structure functional bionic scaffold for the repair of osteochondral defects, utilizing rabbit model experiments to evaluate the efficacy of the repair. The findings indicated successful integration at the interface of the scaffold material and host tissue, as well as between the newly formed subchondral bone and cartilage. In this study, osteochondral repair demonstrated the highest total histological score. The experimental model involved the use of cylindrical fully repaired full-thickness defects measuring 5 mm in diameter in rabbits. Of particular note is the similarity in thickness between the regenerated cartilage and the surrounding normal cartilage, as well as the resemblance in cell arrangement and number the superficial cartilage region to that of normal hyaline cartilage. Additionally, distinct lacunae were observed in the regenerated cartilage.
4.3 Growth stimulating factors
Growth-stimulating factors are crucial in facilitating and enhancing the tissue engineering process. With the concurrent application of these factors with LIPUS stimulation has shown promising results in enhancing vascularization and facilitating the development of new bone and cartilage, thereby creating a conductive environment for comprehensive mitochondrial repair (55). Chen et al. (56) conducted a study in which drug carriers were substituted with liposomes to prolong drug effectiveness and decrease possible adverse effects, resulting in decreased effective dosage and frequency of administration for OA repair through the concurrent use of LIPUS. This approach ultimately improved the consistency of therapeutic outcomes. Additionally, Zuo et al. (57) found that the combination of LIPUS with Prussian blue nanoparticles (PBNPs) led to reduced cellular reactive oxygen species (ROS), apoptosis, and MMPs, thereby preventing articular cartilage damage via the PI3K/Akt/mTOR signaling pathway. The utilization of dexamethasone and transforming growth factor-b1 (TD) in the treatment of hMSCs resulted in the manifestation of characteristic cartilage cell morphology. The incorporation of LIPUS further increased the effects of TD on the differentiation of hMSCs (58). Although several growth-stimulating factors have shown efficacy in promoting cell proliferation and differentiation in vitro, the suitability of these stimuli necessitates comprehensive validation and careful selection.
5 Perspectives, future outlook and remarks
Originally employed for the management of delayed fracture union and non-union cases, the utilization of LIPUS has evolved to include a wide range of applications in the fields of musculoskeletal, neurological, urinary, cardiovascular, and other systemic disorders. According to the mechano-biochemical transduction theory, LIPUS impact cellular function through the induction of cavitation and mechanical responses within various cellular components, including the extracellular matrix, cell membrane, cytoplasm, and nucleus, via mechanisms involved in structural integrity and motility. This ultimately results in a range of biological effects, including alterations in cellular morphology, regulation of gene expression, and metabolic adaptations. Furthermore, LIPUS enhances the synthesis of crucial factors (90, 91) essential for cellular proliferation and tissue regeneration, such as TGF, FGF, VEGF, and BMP. Collectively, these intricate biological mechanisms suggest that LIPUS holds potential as a therapeutic intervention for conditions such as OA.
Currently, numerous in vitro research provides evidence for the efficacy of LIPUS as a non-invasive therapeutic intervention for modulating synthesis/catabolism levels in OA joints and facilitating changes in the synovial membrane, cartilage, and subchondral skeletal microenvironment. Given the avascular nature of cartilage and the hypoxic conditions (92–94) in which chondrocytes function intra-articularly, the majority of in vitro and ex vivo studies have been conducted under standard 21% O2 and 5% CO2 concentrations. The heightened oxygen levels present in the incubator exceed the physiological levels found in live cartilage potentially leading to alterations in chondrocyte response to LIPUS and subsequent biochemical changes. Additionally, it is crucial to consider that many in vitro studies have not yet investigated the potential influence of cell culture materials and media on the transmission of LIPUS waves. Nevertheless, the intrinsic attributes of these cultural elements possess the capacity to affect the transmission of LIPUS waves, thereby significantly influencing cellular biochemical responses. Thus, a comprehensive comprehension of the synergistic effects of LIPUS and the extracellular milieu is imperative for the development of a culture system that more accurately replicates the intracellular microenvironment.
It is imperative to perform preclinical studies utilizing appropriate animal models in order to investigate the effects of LIPUS on the entire joint and its potential chondroprotective properties. Risk factors associated with OA can be classified into mechanical and non-mechanical factors, each leading to unique manifestations of the disease. Synovial inflammation persists throughout the progression of OA, while cartilage degeneration is a prominent pathological characteristic in the intermediate and advanced stages. The initial phases of OA involve the activation of osteoclasts in the subchondral bone, progressing to osteoporosis in advanced stages. Given the differences in load-bearing capacities between various animal species and human joints, careful consideration must be given to the selection of appropriate experimental animals for both surgical and non-surgical modeling at different stages OA development.
LIPUS is characterized by acoustic radiation whose power diminishes as a function of distance. Upon encountering tissues within a joint, LIPUS undergoes reflection and refraction, thereby modifying the mechano-biochemical transduction effects on various tissues, cells, and molecules. The efficacy of LIPUS interventions in activating molecular pathways and cellular activities is contingent upon factors such as duration, strength, and energy parameters, which may vary across studies, posing challenges in the analysis and comparison of results. Additionally, it is important to note that treatment outcomes may differ depending on the specific LIPUS machines, probe types, intervention techniques, and individual variations. Therefore, there is a critical necessity for additional high-quality evidence to substantiate its effectiveness. In the development of LIPUS devices for both animal and clinical trials, medical researchers should engage in collaboration with professionals in acoustic engineering, software engineering and AI algorithms to guarantee the creation of experimental instruments capable of managing acoustic peak pressure, radiation pressure, and acoustic flow force, thereby accurately controlling the LIPUS intensity.
Tissue engineering, specifically utilizing LIPUS, has experienced notable progress and is widely utilized for joint repair. Nevertheless, there are several persistent challenges. One such challenge is the diminished differentiation potential of mesenchymal stem cells with age, coupled with limitations in the quantity of cells that can be obtained from these stem cells due to the difficulty in maintaining their chondrocyte phenotype post-differentiation. Consequently, there is a potential for hypertrophic differentiation in these cells, which could result in apoptosis or ossification. Moreover, the precise cartilage subtype that may be generated remains uncertain. The field of materials science has experienced significant progress, facilitating the discovery and application of contemporary natural and synthetic materials for potential use as scaffold materials in tissue engineering. Through the utilization of current theoretical models and methodologies, the processes and outcomes of tissue engineering have undergone continuous enhancement. Techniques such as 3D cell culture in vitro epitaxy and 3D bioprinting have played a crucial role in the development of cartilage tissues with improved properties. However, additional validation studies and clinical trials are necessary to confirm their practical advantages. Growth factors, including TGF, BMP, and others, are thought to promote the growth and specialization of precursor cells in vitro. Despite encouraging preliminary results, the optimization and selection of these stimulating factors require further exploration for practical implementation.
The current state of clinical application of LIPUS therapy is characterized by a complex interplay of potential benefits, challenges, and limitations. These include the high cost of LIPUS treatment devices, which restricts accessibility to a wider patient demographic, variability in treatment outcomes leading to inconsistent efficacy, and the time-intensive nature of the therapy requiring multiple visits over an extended duration, potentially impacting patient compliance and quality of life. While LIPUS treatment is commonly perceived as noninvasive and safe, certain patients may encounter adverse effects such as skin irritation or discomfort. Furthermore, the efficacy of this therapy is constrained by the specific characteristics and site of the injury or illness, thereby diminishing its overall effectiveness. Lastly, the utilization of LIPUS therapy necessitates healthcare professionals to undergo specialized training for its appropriate administration, consequently augmenting the intricacy and expenses associated with delivering this treatment. In summary, LIPUS presents numerous advantages compared to traditional pharmacological treatments, including its localized application, non-invasiveness, ease of use, cost-effectiveness, and efficacy. As further research is conducted, LIPUS is poised to significantly enhance the management of OA for a large population of patients globally. It is essential to acknowledge that despite the promising results of LIPUS therapy in specific clinical contexts, its widespread acceptance and implementation are contingent upon addressing substantial obstacles and constraints.
Author contributions
JZ: Formal analysis, Writing – review & editing, Writing – original draft. EN: Data curation, Writing – review & editing. LL: Data curation, Writing – review & editing. HZ: Data curation, Writing – review & editing. XY: Conceptualization, Supervision, Writing – review & editing. YH: Conceptualization, Supervision, Writing – review & editing.
Funding
The author(s) declare that financial support was received for the research, authorship, and/or publication of this article. Financial supports from the Program of Jiangsu science and technology Department (Nos. BK20211083 and BE2022737), Jiangsu social development project (No. BE2021673), the Program of Suzhou Health Commission (Nos. GSWS2020078 and SZXK202111), and Jiangsu Graduate Student Cultivation Innovative Engineering Graduate Research and Practice Innovation Program (No. SJCX23_0683).
Acknowledgments
We thank the authors of the references for providing the data of the final manuscript.
Conflict of interest
The authors declare that the research was conducted in the absence of any commercial or financial relationships that could be construed as a potential conflict of interest.
Publisher’s note
All claims expressed in this article are solely those of the authors and do not necessarily represent those of their affiliated organizations, or those of the publisher, the editors and the reviewers. Any product that may be evaluated in this article, or claim that may be made by its manufacturer, is not guaranteed or endorsed by the publisher.
References
1. Katz, JN, Arant, KR, and Loeser, RF. Diagnosis and treatment of hip and knee osteoarthritis: a review. JAMA. (2021) 325:568–78. doi: 10.1001/jama.2020.22171
2. Quicke, JG, Conaghan, PG, Corp, N, and Peat, G. Osteoarthritis year in review 2021: epidemiology & therapy. Osteoarthr Cartil. (2022) 30:196–206. doi: 10.1016/j.joca.2021.10.003
3. Arden, NK, Perry, TA, Bannuru, RR, Bruyere, O, Cooper, C, Haugen, IK, et al. Non-surgical management of knee osteoarthritis: comparison of ESCEO and OARSI 2019 guidelines. Nat Rev Rheumatol. (2021) 17:59–66. doi: 10.1038/s41584-020-00523-9
4. Bannuru, RR, Osani, MC, Vaysbrot, EE, Arden, NK, Bennell, K, Bierma-Zeinstra, SMA, et al. OARSI guidelines for the non-surgical management of knee, hip, and polyarticular osteoarthritis. Osteoarthr Cartil. (2019) 27:1578–89. doi: 10.1016/j.joca.2019.06.011
5. Poolman, RW, Agoritsas, T, Siemieniuk, RA, Harris, IA, Schipper, IB, Mollon, B, et al. Low intensity pulsed ultrasound (LIPUS) for bone healing: a clinical practice guideline. BMJ. (2017) 356:j576. doi: 10.1136/bmj.j576
6. Doll, J, Moghaddam, A, Daniel, V, Biglari, B, Heller, R, Schmidmaier, G, et al. LIPUS vs. reaming in non-union treatment: cytokine expression course as a tool for evaluation and differentiation of non-union therapy. J Orthop. (2020) 17:208–14. doi: 10.1016/j.jor.2019.08.018
7. Liufu, C, Li, Y, Lin, Y, Yu, J, Du, M, Chen, Y, et al. Synergistic ultrasonic biophysical effect-responsive nanoparticles for enhanced gene delivery to ovarian cancer stem cells. Drug Deliv. (2020) 27:1018–33. doi: 10.1080/10717544.2020.1785583
8. Azagury, A, Khoury, L, Enden, G, and Kost, J. Ultrasound mediated transdermal drug delivery. Adv Drug Deliv Rev. (2014) 72:127–43. doi: 10.1016/j.addr.2014.01.007
9. Watson, T. Ultrasound in contemporary physiotherapy practice. Ultrasonics. (2008) 48:321–9. doi: 10.1016/j.ultras.2008.02.004
10. Lin, G, Reed-Maldonado, AB, Lin, M, Xin, Z, and Lue, TF. Effects and mechanisms of low-intensity pulsed ultrasound for chronic prostatitis and chronic pelvic pain syndrome. Int J Mol Sci. (2016) 17:1057. doi: 10.3390/ijms17071057
11. De Roover, A, Escribano-Nunez, A, Monteagudo, S, and Lories, R. Fundamentals of osteoarthritis: inflammatory mediators in osteoarthritis. Osteoarthr Cartil. (2023) 31:1303–11. doi: 10.1016/j.joca.2023.06.005
12. Sanchez-Lopez, E, Coras, R, Torres, A, Lane, NE, and Guma, M. Synovial inflammation in osteoarthritis progression. Nat Rev Rheumatol. (2022) 18:258–75. doi: 10.1038/s41584-022-00749-9
13. Hsieh, YL, Chen, HY, and Yang, CC. Early intervention with therapeutic low-intensity pulsed ultrasound in halting the progression of post-traumatic osteoarthritis in a rat model. Ultrasound Med Biol. (2018) 44:2637–45. doi: 10.1016/j.ultrasmedbio.2018.08.007
14. Zhao, Y, Huang, Q, Yang, J, Lou, M, Wang, A, Dong, J, et al. Autophagy impairment inhibits differentiation of glioma stem/progenitor cells. Brain Res. (2010) 1313:250–8. doi: 10.1016/j.brainres.2009.12.004
15. Chung, JI, Barua, S, Choi, BH, Min, BH, Han, HC, and Baik, EJ. Anti-inflammatory effect of low intensity ultrasound (LIUS) on complete Freund's adjuvant-induced arthritis synovium. Osteoarthr Cartil. (2012) 20:314–22. doi: 10.1016/j.joca.2012.01.005
16. Nakamura, T, Fujihara, S, Katsura, T, Yamamoto, K, Inubushi, T, Tanimoto, K, et al. Effects of low-intensity pulsed ultrasound on the expression and activity of hyaluronan synthase and hyaluronidase in IL-1beta-stimulated synovial cells. Ann Biomed Eng. (2010) 38:3363–70. doi: 10.1007/s10439-010-0104-5
17. Nakamura, T, Fujihara, S, Yamamoto-Nagata, K, Katsura, T, Inubushi, T, and Tanaka, E. Low-intensity pulsed ultrasound reduces the inflammatory activity of synovitis. Ann Biomed Eng. (2011) 39:2964–71. doi: 10.1007/s10439-011-0408-0
18. Sato, M, Nagata, K, Kuroda, S, Horiuchi, S, Nakamura, T, Karima, M, et al. Low-intensity pulsed ultrasound activates integrin-mediated mechanotransduction pathway in synovial cells. Ann Biomed Eng. (2014) 42:2156–63. doi: 10.1007/s10439-014-1081-x
19. Liao, B, Guan, M, Tan, Q, Wang, G, Zhang, R, Huang, J, et al. Low-intensity pulsed ultrasound inhibits fibroblast-like synoviocyte proliferation and reduces synovial fibrosis by regulating Wnt/beta-catenin signaling. J Orthop Translat. (2021) 30:41–50. doi: 10.1016/j.jot.2021.08.002
20. da Silva Junior, EM, Mesquita-Ferrari, RA, Franca, CM, Andreo, L, Bussadori, SK, and Fernandes, KPS. Modulating effect of low intensity pulsed ultrasound on the phenotype of inflammatory cells. Biomed Pharmacother. (2017) 96:1147–53. doi: 10.1016/j.biopha.2017.11.108
21. Feltham, T, Paudel, S, Lobao, M, Schon, L, and Zhang, Z. Low-intensity pulsed ultrasound suppresses synovial macrophage infiltration and inflammation in injured knees in rats. Ultrasound Med Biol. (2021) 47:1045–53. doi: 10.1016/j.ultrasmedbio.2020.12.019
22. Zhang, B, Chen, H, Ouyang, J, Xie, Y, Chen, L, Tan, Q, et al. SQSTM1-dependent autophagic degradation of PKM2 inhibits the production of mature IL1B/IL-1beta and contributes to LIPUS-mediated anti-inflammatory effect. Autophagy. (2020) 16:1262–78. doi: 10.1080/15548627.2019.1664705
23. Ting, SY, Montagne, K, Nishimura, Y, Ushida, T, and Furukawa, KS. Modulation of the effect of transforming growth factor-beta 3 by low-intensity pulsed ultrasound on scaffold-free dedifferentiated articular bovine chondrocyte tissues. Tissue Eng Part C Methods. (2015) 21:1005–14. doi: 10.1089/ten.tec.2014.0428
24. Nishida, T, Kubota, S, Aoyama, E, Yamanaka, N, Lyons, KM, and Takigawa, M. Low-intensity pulsed ultrasound (LIPUS) treatment of cultured chondrocytes stimulates production of CCN family protein 2 (CCN2), a protein involved in the regeneration of articular cartilage: mechanism underlying this stimulation. Osteoarthr Cartil. (2017) 25:759–69. doi: 10.1016/j.joca.2016.10.003
25. Khanna, A, Nelmes, RT, Gougoulias, N, Maffulli, N, and Gray, J. The effects of LIPUS on soft-tissue healing: a review of literature. Br Med Bull. (2009) 89:169–82. doi: 10.1093/bmb/ldn040
26. Ansari, MY, Khan, NM, Ahmad, I, and Haqqi, TM. Parkin clearance of dysfunctional mitochondria regulates ROS levels and increases survival of human chondrocytes. Osteoarthr Cartil. (2018) 26:1087–97. doi: 10.1016/j.joca.2017.07.020
27. Sang, F, Xu, J, Chen, Z, Liu, Q, and Jiang, W. Low-intensity pulsed ultrasound alleviates osteoarthritis condition through focal adhesion kinase-mediated chondrocyte proliferation and differentiation. Cartilage. (2021) 13:196S–203S. doi: 10.1177/1947603520912322
28. Xie, S, Jiang, X, Wang, R, Xie, S, Hua, Y, Zhou, S, et al. Low-intensity pulsed ultrasound promotes the proliferation of human bone mesenchymal stem cells by activating PI3K/AKt signaling pathways. J Cell Biochem. (2019) 120:15823–33. doi: 10.1002/jcb.28853
29. Phillips, R. Targeting articular Mmp 13 in OA. Nat Rev Rheumatol. (2021) 17:645. doi: 10.1038/s41584-021-00697-w
30. Uddin, SM, Richbourgh, B, Ding, Y, Hettinghouse, A, Komatsu, DE, Qin, YX, et al. Chondro-protective effects of low intensity pulsed ultrasound. Osteoarthr Cartil. (2016) 24:1989–98. doi: 10.1016/j.joca.2016.06.014
31. Xia, P, Ren, S, Lin, Q, Cheng, K, Shen, S, Gao, M, et al. Low-intensity pulsed ultrasound affects chondrocyte extracellular matrix production via an integrin-mediated p 38 MAPK signaling pathway. Ultrasound Med Biol. (2015) 41:1690–700. doi: 10.1016/j.ultrasmedbio.2015.01.014
32. Zhou, HY, Li, Q, Wang, JX, Xie, YJ, Wang, SQ, Lei, L, et al. Low-intensity pulsed ultrasound repair in mandibular condylar cartilage injury rabbit model. Arch Oral Biol. (2019) 104:60–6. doi: 10.1016/j.archoralbio.2019.05.018
33. Sekino, J, Nagao, M, Kato, S, Sakai, M, Abe, K, Nakayama, E, et al. Low-intensity pulsed ultrasound induces cartilage matrix synthesis and reduced MMP13 expression in chondrocytes. Biochem Biophys Res Commun. (2018) 506:290–7. doi: 10.1016/j.bbrc.2018.10.063
34. Guan, M, Zhu, Y, Liao, B, Tan, Q, Qi, H, Zhang, B, et al. Low-intensity pulsed ultrasound inhibits VEGFA expression in chondrocytes and protects against cartilage degeneration in experimental osteoarthritis. FEBS Open Bio. (2020) 10:434–43. doi: 10.1002/2211-5463.12801
35. Kojima, Y, and Watanabe, T. Low-intensity pulsed ultrasound irradiation attenuates collagen degradation of articular cartilage in early osteoarthritis-like model mice. J Exp Orthop. (2023) 10:106. doi: 10.1186/s40634-023-00672-2
36. Cook, SD, Salkeld, SL, Patron, LP, Doughty, ES, and Jones, DG. The effect of low-intensity pulsed ultrasound on autologous osteochondral plugs in a canine model. Am J Sports Med. (2008) 36:1733–41. doi: 10.1177/0363546508316766
37. Wu, S, Zhou, H, Ling, H, Sun, Y, Luo, Z, Ngo, T, et al. LIPUS regulates the progression of knee osteoarthritis in mice through primary cilia-mediated TRPV4 channels. Apoptosis. (2024). doi: 10.1007/s10495-024-01950-9
38. Tanaka, E, Liu, Y, Xia, L, Ogasawara, N, Sakamaki, T, Kano, F, et al. Effectiveness of low-intensity pulsed ultrasound on osteoarthritis of the temporomandibular joint: a review. Ann Biomed Eng. (2020) 48:2158–70. doi: 10.1007/s10439-020-02540-x
39. Xia, P, Wang, Q, Song, J, Wang, X, Wang, X, Lin, Q, et al. Low-intensity pulsed ultrasound enhances the efficacy of bone marrow-derived MSCs in osteoarthritis cartilage repair by regulating autophagy-mediated exosome release. Cartilage. (2022) 13:194760352210930. doi: 10.1177/19476035221093060
40. Wang, X, Lin, Q, Zhang, T, Wang, X, Cheng, K, Gao, M, et al. Low-intensity pulsed ultrasound promotes chondrogenesis of mesenchymal stem cells via regulation of autophagy. Stem Cell Res Ther. (2019) 10:41. doi: 10.1186/s13287-019-1142-z
41. Pan, C, Lu, F, Hao, X, Deng, X, Liu, J, Sun, K, et al. Low-intensity pulsed ultrasound delays the progression of osteoarthritis by regulating the YAP-RIPK1-NF-kappa B axis and influencing autophagy. J Transl Med. (2024) 22:286. doi: 10.1186/s12967-024-05086-x
42. Hugle, T, and Geurts, J. What drives osteoarthritis?-synovial versus subchondral bone pathology. Rheumatology. (2017) 56:1461–71. doi: 10.1093/rheumatology/kew389
43. Yamaguchi, S, Aoyama, T, Ito, A, Nagai, M, Iijima, H, Tajino, J, et al. Effect of low-intensity pulsed ultrasound after mesenchymal stromal cell injection to treat osteochondral defects: an in vivo study. Ultrasound Med Biol. (2016) 42:2903–13. doi: 10.1016/j.ultrasmedbio.2016.07.021
44. Yilmaz, V, Karadas, O, Dandinoglu, T, Umay, E, Cakci, A, and Tan, AK. Efficacy of extracorporeal shockwave therapy and low-intensity pulsed ultrasound in a rat knee osteoarthritis model: a randomized controlled trial. Eur J Rheumatol. (2017) 4:104–8. doi: 10.5152/eurjrheum.2017.160089
45. Lee, W, Georgas, E, Komatsu, DE, and Qin, YX. Daily low-intensity pulsed ultrasound stimulation mitigates joint degradation and pain in a post-traumatic osteoarthritis rat model. J Orthop Translat. (2024) 44:9–18. doi: 10.1016/j.jot.2023.09.002
46. Li, X, Sun, Y, Zhou, Z, Zhang, D, Jiao, J, Hu, M, et al. Mitigation of articular cartilage degeneration and subchondral bone sclerosis in osteoarthritis progression using low-intensity ultrasound stimulation. Ultrasound Med Biol. (2019) 45:148–59. doi: 10.1016/j.ultrasmedbio.2018.08.022
47. Nasb, M, Liangjiang, H, Gong, C, and Hong, C. Human adipose-derived mesenchymal stem cells, low-intensity pulsed ultrasound, or their combination for the treatment of knee osteoarthritis: study protocol for a first-in-man randomized controlled trial. BMC Musculoskelet Disord. (2020) 21:33. doi: 10.1186/s12891-020-3056-4
48. Xia, P, Wang, X, Wang, Q, Wang, X, Lin, Q, Cheng, K, et al. Low-intensity pulsed ultrasound promotes autophagy-mediated migration of mesenchymal stem cells and cartilage repair. Cell Transplant. (2021) 30:096368972098614. doi: 10.1177/0963689720986142
49. Liao, Q, Li, BJ, Li, Y, Xiao, Y, Zeng, H, Liu, JM, et al. Low-intensity pulsed ultrasound promotes osteoarthritic cartilage regeneration by BMSC-derived exosomes via modulating the NF-kappa B signaling pathway. Int Immunopharmacol. (2021) 97:107824. doi: 10.1016/j.intimp.2021.107824
50. Takeuchi, R, Ryo, A, Komitsu, N, Mikuni-Takagaki, Y, Fukui, A, Takagi, Y, et al. Low-intensity pulsed ultrasound activates the phosphatidylinositol 3 kinase/Akt pathway and stimulates the growth of chondrocytes in three-dimensional cultures: a basic science study. Arthritis Res Ther. (2008) 10:R77. doi: 10.1186/ar2451
51. Uenaka, K, Imai, S, Ando, K, and Matsusue, Y. Relation of low-intensity pulsed ultrasound to the cell density of scaffold-free cartilage in a high-density static semi-open culture system. J Orthop Sci. (2010) 15:816–24. doi: 10.1007/s00776-010-1544-3
52. Aliabouzar, M, Lee, SJ, Zhou, X, Zhang, GL, and Sarkar, K. Effects of scaffold microstructure and low intensity pulsed ultrasound on chondrogenic differentiation of human mesenchymal stem cells. Biotechnol Bioeng. (2018) 115:495–506. doi: 10.1002/bit.26480
53. Aliabouzar, M, Zhang, LG, and Sarkar, K. Lipid coated microbubbles and low intensity pulsed ultrasound enhance Chondrogenesis of human mesenchymal stem cells in 3D printed scaffolds. Sci Rep. (2016) 6:37728. doi: 10.1038/srep37728
54. Babaei, M, Jamshidi, N, Amiri, F, and Rafienia, M. Effects of low-intensity pulsed ultrasound stimulation on cell seeded 3D hybrid scaffold as a novel strategy for meniscus regeneration: an in vitro study. J Tissue Eng Regen Med. (2022) 16:812–24. doi: 10.1002/term.3331
55. Chen, T, Bai, J, Tian, J, Huang, P, Zheng, H, and Wang, J. A single integrated osteochondral in situ composite scaffold with a multi-layered functional structure. Colloids Surf B Biointerfaces. (2018) 167:354–63. doi: 10.1016/j.colsurfb.2018.04.029
56. Chen, CH, Kuo, SM, Tien, YC, Shen, PC, Kuo, YW, and Huang, HH. Steady augmentation of anti-osteoarthritic actions of rapamycin by liposome-encapsulation in collaboration with low-intensity pulsed ultrasound. Int J Nanomedicine. (2020) 15:3771–90. doi: 10.2147/IJN.S252223
57. Zuo, D, Tan, B, Jia, G, Wu, D, Yu, L, and Jia, L. A treatment combined prussian blue nanoparticles with low-intensity pulsed ultrasound alleviates cartilage damage in knee osteoarthritis by initiating PI3K/Akt/mTOR pathway. Am J Transl Res. (2021) 13:3987–4006.
58. Lai, CH, Chen, SC, Chiu, LH, Yang, CB, Tsai, YH, Zuo, CS, et al. Effects of low-intensity pulsed ultrasound, dexamethasone/TGF-beta 1 and/or BMP-2 on the transcriptional expression of genes in human mesenchymal stem cells: chondrogenic vs. osteogenic differentiation. Ultrasound Med Biol. (2010) 36:1022–33. doi: 10.1016/j.ultrasmedbio.2010.03.014
59. Cook, SD, Salkeld, SL, Popich-Patron, LS, Ryaby, JP, Jones, DG, and Barrack, RL. Improved cartilage repair after treatment with low-intensity pulsed ultrasound. Clin Orthop Relat Res. (2001) 391:S231–43. doi: 10.1097/00003086-200110001-00022
60. Nishikori, T, Ochi, M, Uchio, Y, Maniwa, S, Kataoka, H, Kawasaki, K, et al. Effects of low-intensity pulsed ultrasound on proliferation and chondroitin sulfate synthesis of cultured chondrocytes embedded in Atelocollagen gel. J Biomed Mater Res. (2002) 59:201–6. doi: 10.1002/jbm.1226
61. Zhang, Z, Huckle, J, Francomano, CA, and Spencer, RG. The influence of pulsed low-intensity ultrasound on matrix production of chondrocytes at different stages of differentiation: an explant study. Ultrasound Med Biol. (2002) 28:1547–53. doi: 10.1016/S0301-5629(02)00659-2
62. Zhang, ZJ, Huckle, J, Francomano, CA, and Spencer, RG. The effects of pulsed low-intensity ultrasound on chondrocyte viability, proliferation, gene expression and matrix production. Ultrasound Med Biol. (2003) 29:1645–51. doi: 10.1016/j.ultrasmedbio.2003.08.011
63. Duda, GN, Kliche, A, Kleemann, R, Hoffmann, JE, Sittinger, M, and Haisch, A. Does low-intensity pulsed ultrasound stimulate maturation of tissue-engineered cartilage? J Biomed Mater Res B Appl Biomater. (2004) 68:21–8. doi: 10.1002/jbm.b.10075
64. Jia, XL, Chen, WZ, Zhou, K, and Wang, ZB. Effects of low-intensity pulsed ultrasound in repairing injured articular cartilage. Chin J Traumatol. (2005) 8:175–8.
65. Tien, YC, Lin, SD, Chen, CH, Lu, CC, Su, SJ, and Chih, TT. Effects of pulsed low-intensity ultrasound on human child chondrocytes. Ultrasound Med Biol. (2008) 34:1174–81. doi: 10.1016/j.ultrasmedbio.2007.12.019
66. Korstjens, CM, van der Rijt, RH, Albers, GH, Semeins, CM, and Klein-Nulend, J. Low-intensity pulsed ultrasound affects human articular chondrocytes in vitro. Med Biol Eng Comput. (2008) 46:1263–70. doi: 10.1007/s11517-008-0409-9
67. Naito, K, Watari, T, Muta, T, Furuhata, A, Iwase, H, Igarashi, M, et al. Low-intensity pulsed ultrasound (LIPUS) increases the articular cartilage type II collagen in a rat osteoarthritis model. J Orthop Res. (2010) 28:361–9. doi: 10.1002/jor.20995
68. Gurkan, I, Ranganathan, A, Yang, X, Horton, WE Jr, Todman, M, Huckle, J, et al. Modification of osteoarthritis in the guinea pig with pulsed low-intensity ultrasound treatment. Osteoarthr Cartil. (2010) 18:724–33. doi: 10.1016/j.joca.2010.01.006
69. Vaughan, NM, Grainger, J, Bader, DL, and Knight, MM. The potential of pulsed low intensity ultrasound to stimulate chondrocytes matrix synthesis in agarose and monolayer cultures. Med Biol Eng Comput. (2010) 48:1215–22. doi: 10.1007/s11517-010-0681-3
70. Li, X, Li, J, Cheng, K, Lin, Q, Wang, D, Zhang, H, et al. Effect of low-intensity pulsed ultrasound on MMP-13 and MAPKs signaling pathway in rabbit knee osteoarthritis. Cell Biochem Biophys. (2011) 61:427–34. doi: 10.1007/s12013-011-9206-4
71. Ito, A, Aoyama, T, Yamaguchi, S, Zhang, X, Akiyama, H, and Kuroki, H. Low-intensity pulsed ultrasound inhibits messenger RNA expression of matrix metalloproteinase-13 induced by interleukin-1beta in chondrocytes in an intensity-dependent manner. Ultrasound Med Biol. (2012) 38:1726–33. doi: 10.1016/j.ultrasmedbio.2012.06.005
72. Li, X, Lin, Q, Wang, D, Dai, Y, Cheng, K, Yu, J, et al. The effects of low-intensity pulsed ultrasound and nanomagnet applications on the expressions of MMP-13 and MAPKs in rabbit knee osteoarthritis. J Nanosci Nanotechnol. (2013) 13:722–7. doi: 10.1166/jnn.2013.7182
73. Yang, SW, Kuo, CL, Chang, SJ, Chen, PC, Lin, YT, Manousakas, I, et al. Does low-intensity pulsed ultrasound treatment repair articular cartilage injury? A rabbit model study. BMC Musculoskelet Disord. (2014) 15:36. doi: 10.1186/1471-2474-15-36
74. Jang, KW, Ding, L, Seol, D, Lim, TH, Buckwalter, JA, and Martin, JA. Low-intensity pulsed ultrasound promotes chondrogenic progenitor cell migration via focal adhesion kinase pathway. Ultrasound Med Biol. (2014) 40:1177–86. doi: 10.1016/j.ultrasmedbio.2013.12.007
75. Cheng, K, Xia, P, Lin, Q, Shen, S, Gao, M, Ren, S, et al. Effects of low-intensity pulsed ultrasound on integrin-FAK-PI3K/Akt mechanochemical transduction in rabbit osteoarthritis chondrocytes. Ultrasound Med Biol. (2014) 40:1609–18. doi: 10.1016/j.ultrasmedbio.2014.03.002
76. Xu, SY, Zhang, LM, Yao, XM, Zhou, GQ, Li, X, He, BJ, et al. Effects and mechanism of low-intensity pulsed ultrasound on extracellular matrix in rabbit knee osteoarthritis. Zhongguo Gu Shang. (2014) 27:766–71.
77. Tan, L, Ren, Y, van Kooten, TG, Grijpma, DW, and Kuijer, R. Low-intensity pulsed ultrasound (LIPUS) and pulsed electromagnetic field (PEMF) treatments affect degeneration of cultured articular cartilage explants. Int Orthop. (2015) 39:549–57. doi: 10.1007/s00264-014-2542-4
78. Xia, P, Shen, S, Lin, Q, Cheng, K, Ren, S, Gao, M, et al. Low-intensity pulsed ultrasound treatment at an early osteoarthritis stage protects rabbit cartilage from damage via the integrin/focal adhesion kinase/mitogen-activated protein kinase signaling pathway. J Ultrasound Med. (2015) 34:1991–9. doi: 10.7863/ultra.14.10016
79. Ji, JB, Li, XF, Liu, L, Wang, GZ, and Yan, XF. Effect of low intensity pulsed ultrasound on expression of TIMP-2 in serum and expression of mmp-13 in articular cartilage of rabbits with knee osteoarthritis. Asian Pac J Trop Med. (2015) 8:1043–8. doi: 10.1016/j.apjtm.2015.11.003
80. Du, D, Chen, S, Yi, G, Wang, P, Tang, Y, Zheng, L, et al. Low-intensity pulsed ultrasound promotes extracellular matrix synthesis of human osteoarthritis chondrocytes. Xi Bao Yu Fen Zi Mian Yi Xue Za Zhi. (2016) 32:1536–40.
81. Zahoor, T, Mitchell, R, Bhasin, P, Guo, Y, Paudel, S, Schon, L, et al. Effect of low-intensity pulsed ultrasound on joint injury and post-traumatic osteoarthritis: an animal study. Ultrasound Med Biol. (2018) 44:234–42. doi: 10.1016/j.ultrasmedbio.2017.09.014
82. Tang, ZF, and Li, HY. Effects of fibroblast growth factors 2 and low intensity pulsed ultrasound on the repair of knee articular cartilage in rabbits. Eur Rev Med Pharmacol Sci. (2018) 22:2447–53. doi: 10.26355/eurrev_201804_14838
83. Pan, YL, Ma, Y, Guo, Y, Tu, J, Guo, GP, Ma, SM, et al. Effects of Clematis chinensis Osbeck mediated by low-intensity pulsed ultrasound on transforming growth factor-beta/Smad signaling in rabbit articular chondrocytes. J Med Ultrason. (2001). 2019) 46:177–86. doi: 10.1007/s10396-018-0920-z
84. Vahedi, P, Hosainzadegan, H, Brazvan, B, Roshangar, L, Shafaei, H, and Salimnejad, R. Treatment of cartilage defects by low-intensity pulsed ultrasound in a sheep model. Cell Tissue Bank. (2021) 22:369–78. doi: 10.1007/s10561-020-09880-x
85. Tavakoli, J, Torkaman, G, Ravanbod, R, and Abroun, S. Regenerative effect of low-intensity pulsed ultrasound and platelet-rich plasma on the joint friction and biomechanical properties of cartilage: a non-traumatic osteoarthritis model in the Guinea pig. Ultrasound Med Biol. (2022) 48:862–71. doi: 10.1016/j.ultrasmedbio.2022.01.008
86. Sabanci, S, Sabanci, S, Sendur OFSakarya, S, and Yilmaz, O. The effectiveness of therapeutic ultrasound to the mechanically damaged chondrocyte culture. Physiother Theory Pract. (2022) 40:21–30. doi: 10.1080/09593985.2022.2107466,
87. Yi, X, Wu, L, Liu, J, Qin, YX, Li, B, and Zhou, Q. Low-intensity pulsed ultrasound protects subchondral bone in rabbit temporomandibular joint osteoarthritis by suppressing TGF-beta 1/Smad 3 pathway. J Orthop Res. (2020) 38:2505–12. doi: 10.1002/jor.24628
88. Yi, X, Liu, J, Cheng, MS, and Zhou, Q. Low-intensity pulsed ultrasound inhibits IL-6 in subchondral bone of temporomandibular joint osteoarthritis by suppressing the TGF-beta 1/Smad 3 pathway. Arch Oral Biol. (2021) 125:105110. doi: 10.1016/j.archoralbio.2021.105110
89. Schumann, D, Kujat, R, Zellner, J, Angele, MK, Nerlich, M, Mayr, E, et al. Treatment of human mesenchymal stem cells with pulsed low intensity ultrasound enhances the chondrogenic phenotype in vitro. Biorheology. (2006) 43:431–43.
90. Jamtvedt, G, Dahm, KT, Christie, A, Moe, RH, Haavardsholm, E, Holm, I, et al. Physical therapy interventions for patients with osteoarthritis of the knee: an overview of systematic reviews. Phys Ther. (2008) 88:123–36. doi: 10.2522/ptj.20070043
91. Uddin, SMZ, and Komatsu, DE. Therapeutic potential low-intensity pulsed ultrasound for osteoarthritis: pre-clinical and clinical perspectives. Ultrasound Med Biol. (2020) 46:909–20. doi: 10.1016/j.ultrasmedbio.2019.12.007
92. Oliveira, S, Andrade, R, Silva, FS, Espregueira-Mendes, J, Hinckel, BB, Leal, A, et al. Effects and mechanotransduction pathways of therapeutic ultrasound on healthy and osteoarthritic chondrocytes: a systematic review of in vitro studies. Osteoarthr Cartil. (2023) 31:317–39. doi: 10.1016/j.joca.2022.07.014
93. Zeng, C, Li, H, Yang, T, Deng, ZH, Yang, Y, Zhang, Y, et al. Effectiveness of continuous and pulsed ultrasound for the management of knee osteoarthritis: a systematic review and network meta-analysis. Osteoarthr Cartil. (2014) 22:1090–9. doi: 10.1016/j.joca.2014.06.028
Keywords: low-intensity pulsed ultrasound, osteoarthritis, therapeutic potential, molecular mechanisms, tissue regeneration
Citation: Zhou J, Ning E, Lu L, Zhang H, Yang X and Hao Y (2024) Effectiveness of low-intensity pulsed ultrasound on osteoarthritis: molecular mechanism and tissue engineering. Front. Med. 11:1292473. doi: 10.3389/fmed.2024.1292473
Edited by:
Xianyi Cai, Hefeng Central Hospital, ChinaReviewed by:
Mengcun Chen, Thomas Jefferson University, United StatesFabiola Ojeda, Parc de Salut Mar, Spain
Copyright © 2024 Zhou, Ning, Lu, Zhang, Yang and Hao. This is an open-access article distributed under the terms of the Creative Commons Attribution License (CC BY). The use, distribution or reproduction in other forums is permitted, provided the original author(s) and the copyright owner(s) are credited and that the original publication in this journal is cited, in accordance with accepted academic practice. No use, distribution or reproduction is permitted which does not comply with these terms.
*Correspondence: Yuefeng Hao, haoyuefeng@njmu.edu.cn; Xing Yang, xingyangsz@126.com