Advanced materials and biofabrication technologies to design in vitro functional central nervous system models
- 1Department of Mechanical and Aerospace Engineering, Politecnico di Torino, Turin, Italy
- 2PolitoBIOMed Lab, Politecnico di Torino, Turin, Italy
- 3Interuniversity Center for the Promotion of the 3Rs Principles in Teaching and Research, Turin, Italy
- 4Department of Neuroscience “Rita Levi Montalcini”, Neuroscience Institute Cavalieri Ottolenghi, University of Turin, Turin, Italy
Nowadays, the pathophysiology of several central nervous system (CNS) disorders is still poorly understood, making difficult the identification of efficient treatments. CNS damages, due to neurodegenerative conditions or injuries, often result in permanent neuronal dysfunctions and serious impairments of motor, sensory and cognitive capacities. Despite the many attempts of pharmaceutical research to promote neural regeneration, poor progresses have been made in effectively restoring nervous functionality. Indeed, most of the experimental drugs show limited efficacy in the clinical trials, also due to existing preclinical models’ inability in fully replicating the complexity of CNS pathophysiology. Therefore, tissue-engineered three-dimensional (3D) models are being extensively explored to develop novel representative in vitro platforms, which more carefully replicate the architecture of neural microenvironment, including both cellular and extracellular components. In this respect, 3D in vitro models are expected to be promising and comprehensive tools for investigating CNS diseases and testing new drug compounds, as they overcome some of the common limitations of traditional two-dimensional (2D) cultures. This review discusses the main challenges to be addressed in CNS modeling, analyzing the key elements involved in neural tissue engineering. Specifically, an overview of the mostly used neural cell sources and biomaterials is provided, focusing on the critical aspects to consider in selecting the appropriate components according to the application. Different methods adopted to modulate the structural and functional properties of the engineered microenvironment are also presented, aimed at fostering in vitro tissue maturation. Lastly, the latest advances in biofabrication technologies are outlined, reviewing the most recent 3D bioprinted in vitro systems and microfluidic-based 3D platforms, starting from the modeling of distinctive CNS pathophysiological mechanisms to the designing of refined and functional in vivo-like neural microtissues.
1 Introduction
The CNS has unique and highly specialized structural and functional characteristics, which are not fully understood yet. CNS damages, either by disease or injury, can determine severe impairments and, in the severe cases, even lead to death (Azari and Reynolds, 2016). On one hand, neurodegenerative diseases (for example, Alzheimer’s, Parkinson’s and Huntington’s disease, amyotrophic lateral sclerosis) can lead to cell loss in specific CNS areas, representing the most common causes of morbidity worldwide (Boni et al., 2018; Accardo et al., 2019). On the other hand, CNS impairments can occur due to traumatic events (including car accidents and falls), often leading to spinal cord injury (SCI) or traumatic brain injury, with different consequences based on the damage severity. Overall, these disorders affect not only the individual’s health but also create a financial burden for society.
In the last decade, significant efforts have been made for understanding and stimulating the poor regenerative potential of CNS, but no therapies are available to cure these disorders yet. In this scenario, in vitro models can represent a powerful tool to both recapitulate and study disease mechanisms, and easily progress in the understanding of the pathological cascades and in the screening of drug candidates. Until recently, the research in this field has mainly relied on 2D cell culture systems, being the predominant models for the investigation of CNS pathophysiology. However, 2D cell cultures, although representing useful tools for preliminary in vitro studies, show several limitations which could lead to inaccuracies in the in vitro tissue reproduction and the prediction of drug response (Carletti et al., 2011). For this reason, in vitro 3D models have been introduced as powerful tools for achieving more predictable results. Indeed, 3D models can better recapitulate the heterogeneity of CNS, mimicking the structure of native tissue, thus recreating a more physiological environment for cell culture. Indeed, CNS microenvironment is characterized by several specialized cell types with various roles and functions, surrounded by the extracellular matrix in well-defined hierarchical structure, essential for maintaining the proper functionality of the neural tissue.
In this context, neural tissue engineering aims to reconstruct in vitro bioartificial tissues, combining cell biology and biomaterial science. Specifically, this research field is based on the development of 3D engineered porous constructs, commonly referred as scaffolds, intended to mimic the architecture and the properties of the native environment. Such scaffolds were initially applied to foster nervous tissue regeneration, however, recently, these approaches have found extensive application in developing both healthy and pathological in vitro models, capable of reproducing in vivo conditions with higher accuracy and reliability than traditional 2D models. For example, these systems help in investigating essential molecular pathways, neurogenesis and neural network formation, but are also useful for replicating more complex physiological structures and tissue interfaces.
The aim of this review is to recapitulate the different aspects to consider in the in vitro CNS modeling (Figure 1). In the first chapter, a summary of the current sources of neural cells employed in CNS tissue engineering will be presented, underlying pros and cons for each of them. The second chapter provides an overview of biomaterials employed for specific cell subtype cultures. Then, the biomaterial-based strategies for inducing in vitro tissue maturation will be introduced. Finally, the advances in 3D bioprinting and microfluidic devices for CNS modeling will be discussed, highlighting future trends. Overall, this review summarizes the key aspects to face during CNS in vitro model design and the current strategies applied to mimic some of the physiological features towards the development of refined and physiologically relevant in vitro platforms.
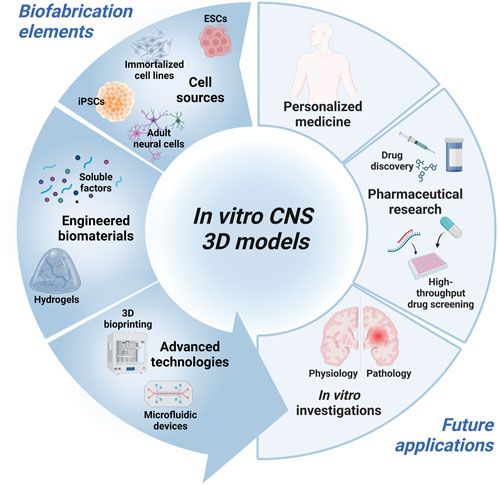
FIGURE 1. Schematic diagram of the key elements for the design of in vitro 3D CNS models and intended application. Created with BioRender.com.
2 Cell types and sources used for in vitro CNS modeling
Choosing the appropriate cell types and sources is essential for in vitro CNS modeling. Indeed, each cell type is characterized by specific biochemical, morphological and functional properties: 1) biochemical signaling encloses gene/protein expression or specific reactivity to different factors, 2) morphological requirements are essential for proper growth analysis, while 3) functional properties mean that cells successfully connect each other to form a complex and physiologically-relevant construct (Hopkins et al., 2015). Overall, the desirable cellular model should closely mimic the main in vivo characteristics, eventually by using more cell types: the available sources and the main pros and cons of each one are described in the next paragraphs (Figure 2; Table 1). We listed them from the less to the most convenient and impactful one, in the field of biomaterial-based in vitro CNS models, overall considering their advantages/disadvantages.
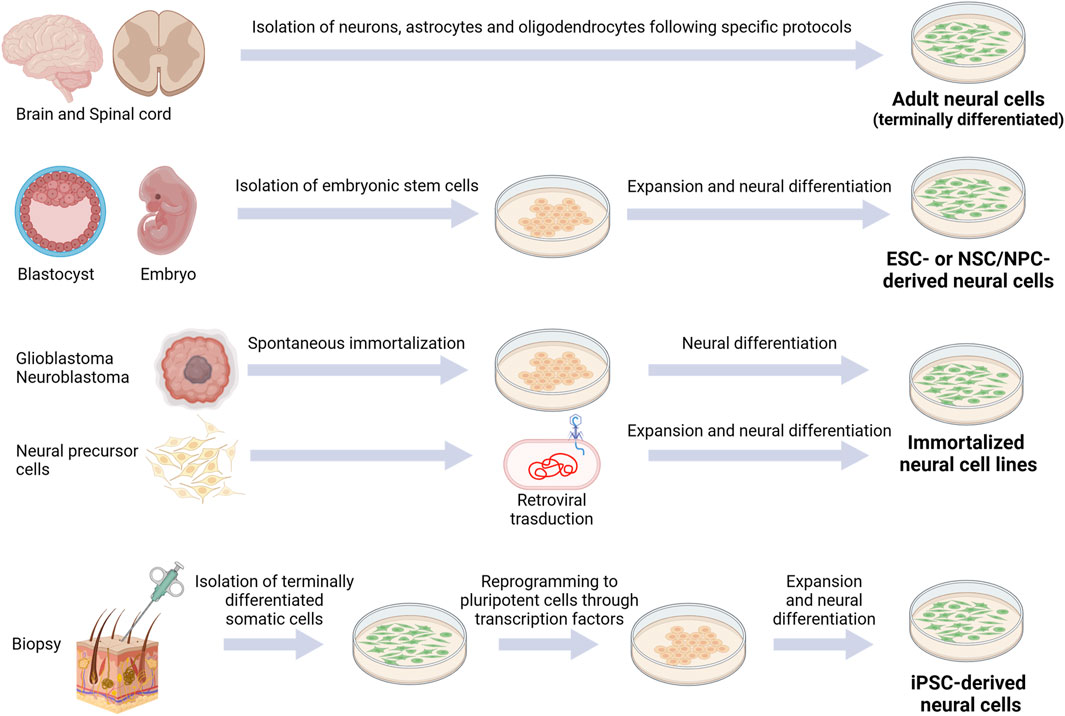
FIGURE 2. Schematic representation of currently available cell sources for CNS modeling. Created with BioRender.com.
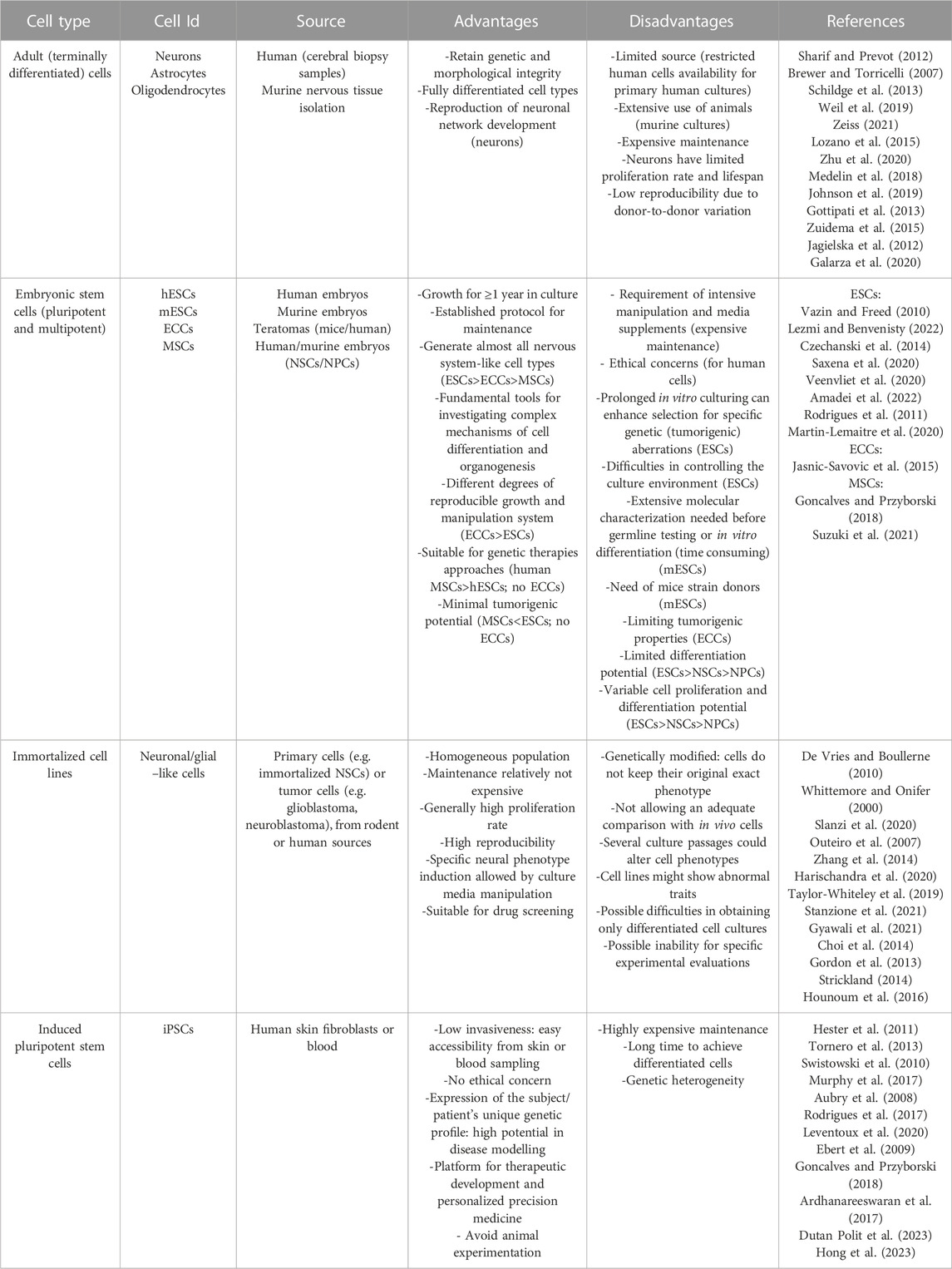
TABLE 1. Main cell types and sources used for in vitro CNS modeling: for any category of cells, common advantages and disadvantages in their use are shown (eventual cell-specific properties are indicated into the brackets).
2.1 Adult cells
Adult cells are terminally differentiated cells (with the exception of adult stem cells, represented in the CNS by resident stem cells maintaining a self-renewal and proliferative capacity to generate new neurons, astrocytes, and oligodendrocytes throughout adulthood (de Morree and Rando, 2023)). To date, their employment in the neurological field is limited due to restricted human cell availability. However, for example, neurons, astrocytes and oligodendrocytes can be collected from adult patients undergoing craniotomy and cerebral biopsy (Sharif and Prevot, 2012).
Instead, rodent primary cultures are more accessible and represent a fundamental source for studying CNS physiological and pathological pathways. Since the first primary explant by Harrison in 1907, many specific protocols have been developed to separate the neural cell type of interest from the others (i.e., neurons (Brewer and Torricelli, 2007), astrocytes (Schildge et al., 2013) and oligodendrocytes (Weil et al., 2019)). However, primary rodent cell culture could imply differences among species (i.e., number of neurons, different time scale of CNS development (Zeiss, 2021)), and lead to technical problems and costly investment to reproduce high quality viable neuronal cultures; furthermore, the extensive use of animals remains a critical point.
Neurons and glial cells have been employed in the most diverse applications. For instance, primary rodent cortical neurons were employed to model the 3D brain structure (Lozano et al., 2015), to investigate the neuronal network development (Moschetta et al., 2021) and the neuro-microglia interaction in a 3D environment (Zhu et al., 2020); primary rat hippocampal neurons were utilized for evaluating the synaptic network formation into bioactive polymeric materials (Medelin et al., 2018). Primary cortical rodent astrocytes have been employed to study the influence of the substrate on their morpho-functional behavior (Gottipati et al., 2013; Zuidema et al., 2015; Johnson et al., 2019). Oligodendrocyte progenitor cells were isolated from rodent neonatal cortex or spinal cord to study their biological properties and their differentiation process in 3D models (Jagielska et al., 2012; Egawa et al., 2017; Geissler et al., 2018). Concerning human cells, cortical astrocytes have been used for studying quiescence and activation pathways (Galarza et al., 2020).
2.2 Embryonic stem cells
Since the discovery of embryonic stem cells (ESCs), multiple attempts have been made to reproduce physiological and pathological models of CNS. Indeed, ESCs are self-renewing cells, representing the main promising wellspring for in vitro research. ESCs can be obtained from the inner mass of the blastocyst: they can generate cells from each of the three germ layers and, for this reason, are classified as pluripotent. Due to their pluripotency, ESCs (murine or human) are a fundamental tool for investigating the complex mechanisms of cell differentiation and organ structure formations. The first stable murine ESC (mESC) line was developed in 1981 (Evans and Kaufman, 1981; Martin, 1981), while the first human ESC (hESC) line in 1998 (Thomson et al., 1998). Since then, many hESC lines have been generated from donated embryos by immunosurgery or mechanical dissection. After isolation, ESCs have to be cultivated in vitro with a maintenance medium, and then differentiated in the desired cell lineage through a combination of inducing factors. Many protocols have been developed for inducing differentiation of ESCs into neural lineages, such as oligodendrocytes, astrocytes and different types of neurons (glutamatergic and GABAergic neurons, motor neurons, etc.) (Vazin and Freed, 2010). However, the obligated disruption of the human embryo has raised many ethical and political concerns over the years, hampering the use of hESCs in research. Furthermore, hurdles also regard the tumorigenic potential of this cell type, based on distinct intrinsic molecular characteristics as their self-renewal and pluripotency capacity, and their gene expression profile (with high expression of onco-fetal genes) (Lezmi and Benvenisty, 2022).
On the other hand, the use of mESCs, as key tools for genetic engineering of stem cell–based therapies and basic research (widely used to introduce mutations and generate transgenic mice) circumvents the limited availability of human samples and the ethical concerns described. Although previously limited in their commercial availability to a small number of inbred mouse strains donors, in the last decade there has been considerable progress in obtaining mESCs even from once non-permissive mouse strains, enabling the cultivation of mESCs from different transgenic mice for modeling neuronal diseases or neurodevelopmental biology studies within months (Czechanski et al., 2014; Saxena et al., 2020; Veenvliet et al., 2020). However, it is important to take into account, for the in vitro expansion of mESCs, the relative difficulties in controlling the culture environment (possibly due to excessive cell aggregation problems), the requirement of intensive manipulation and media supplements, the needed constant (and time consuming) characterization of newly derived ESC lines before germline testing or in vitro differentiation (e.g., by assays for mycoplasma, bacteria, fungi or mouse pathogens, SNP paneling analysis and periodic Karyotipic assessments), not disregarding mice employment issues (related to the production or renewal of mESCs from specific strains) (Rodrigues et al., 2011; Czechanski et al., 2014; Martin-Lemaitre et al., 2020). Among the pluripotent stem cells, it is also possible to mention the embryonal carcinoma cells (ECCs), derived from teratomas, which generally, upon culture with retinoic acid (RA), could differentiate into well-developed, morphologically and immunophenotypically CNS-like neurons, providing a more efficient and more reproducible system in terms of growth and manipulation, compared to ESCs (Jasnic-Savovic et al., 2015). However, ECCs are rarely employed in regenerative therapies due to their tumorigenic properties, but can be used for in vitro disease modeling.
Also multipotent stem cells (MSCs), including neural stem cells (NSCs) and neural precursor cells (NPCs), are unspecialized cells, which have the capacity to self-renew. NSCs are uncommitted cells, able to generate all neural lineages, while NPCs (the non-stem cell progeny of NSCs) are committed to give rise to neurons or astrocytes/oligodendrocytes that are regionally and spatially different. For this reason, NSCs have unlimited proliferation potential, while NPCs can undergo a lower number of replication cycles (Homem et al., 2015; Martínez-Cerdeño and Noctor, 2018; Suzuki et al., 2021). However, both cells display minimal tumorigenic potential (Goncalves and Przyborski, 2018). There are several examples where these cells (in particular NSCs) have been successfully used to replace lost neurons (e.g., for the treatment of ischemic stroke) (Goncalves and Przyborski, 2018; Suzuki et al., 2021).
2.3 Immortalized cell lines
Immortalized cell line represents an enormous source for in vitro analysis. In order to reduce the use of primary cells (i.e., isolated or harvested directly from living tissues), immortalized cells have been largely developed, mainly generated by modifying primary cells or tumor cells, derived from rodent or human sources. For instance, neural cell lines can derive from glioblastoma and neuroblastoma cell lines: due to their intrinsic tumorigenic nature, they can be only employed in vitro. As an alternative, primary cells as NPCs and NSCs can be immortalized by retroviral transduction (Whittemore and Onifer, 2000; De Vries and Boullerne, 2010).
Their maintenance is less expensive compared to primary cells as long as they have higher viability and proliferation rate. Numerous immortalized cell lines have been developed and employed for studying neurodegenerative disorders or regeneration mechanisms after injury. For instance, the pathogenesis of Parkinson’s disease has been investigated with rat pheochromocytoma cells (PC12 (Slanzi et al., 2020)), human neuroglioma cells (H4 (Outeiro et al., 2007)), human mesencephalic cells (Lund Human Mesencephalic cells, LUHMES (Zhang et al., 2014; Harischandra et al., 2020)) and human neuroblastoma cells (SH-SY5Y (Taylor-Whiteley et al., 2019)): in particular, the SH-SY5Y and LUHMES cell lines have been largely employed because they are able to reproduce the dopaminergic phenotype involved in Parkinson’s disease. NSC-34 is a mouse motor neuron-like hybrid cell line widely employed in the study of neurodegenerative motor neuron diseases, including amyotrophic lateral sclerosis (Gyawali et al., 2021; Stanzione et al., 2021). Regarding Alzheimer’s disease (AD) research, human NPCs (ReNcell) have been used to recapitulate amyloid-β and tau pathology (Choi et al., 2014). In the field of neural tissue regeneration, murine NSCs (C17.2 and NE-4C), rat neuronal cell line (PC12), human NSCs (ReNcell CX), human NPCs (NT2) have been used in different studies of neural tissue engineering. Moreover, many astrocyte and oligodendrocyte cell lines are available on the market, such as human astrocytes (CCF-STTG1) and oligodendrocytes (MO3.13), and rodent astrocytes (C8D1A, C8-S, CTX TNA2) and oligodendrocytes (Oli-neu, Mouse Oligodendrocyte Precursor Cells MLGPCs): they are largely used for 2D cell cultures, but still scarcely employed in 3D systems.
The major disadvantage of immortalized cell lines is that they cannot be compared to any cell type in vivo given their unique gene expression, which allows for indefinite division. Moreover, even if specific neuronal phenotypes could be induced in these cells by manipulations of the culture conditions, they could differ widely from the in vivo cells or from the primary cells, not allowing an adequate comparison (Gordon et al., 2013; Strickland, 2014; Hounoum et al., 2016). Furthermore, after several passages, the cellular characteristics of the immortalized cell lines might change or even show abnormal traits (such as the unusual combination of neurotransmitter production). For example, the work of Hounoum and colleagues suggested that the NSC-34 cell model, while representing a valuable tool for research on motor neuron diseases, did not allow to obtain cultures containing only differentiated cells and was not suitable for the study of glutamate-induced excitotoxicity (Hounoum et al., 2016).
2.4 Induced pluripotent stem cells
Induced pluripotent stem cells (iPSCs) have recently emerged as an exciting alternative to embryonic stem cells. Indeed, they are derived from terminally differentiated somatic cells (generally skin fibroblasts or peripheral blood mononuclear cells). They can be genetically reprogrammed into embryonic-like cells expressing pluripotency genes using a cocktail of transcription factors (Figure 2), in this way limiting ethical concerns compared to embryonic cells. Since their discovery (Takahashi et al., 2007), in vitro differentiation of human iPSCs has been established for motor neurons (Hester et al., 2011), cortical neurons (Tornero et al., 2013), dopaminergic neurons (Swistowski et al., 2010), striatal neurons (Aubry et al., 2008; Murphy et al., 2017), oligodendrocytes (Rodrigues et al., 2017) and astrocytes (Leventoux et al., 2020). The stemness or the differentiated cell state can be monitored through specific markers. For instance, Nestin and SOX2 or betaIII-Tubulin are markers for neural progenitor cells, NG2 for oligodendrocyte precursor cells, MAP2 for neurons, O4 for oligodendrocytes and GFAP for astrocytes.
Originating from adult human cells, iPSCs can express the patient’s unique genetic profile: therefore, their potential encloses disease modeling and drug screening as a personalized cell therapy. Indeed, maintaining the patient genetic background ensures the in vitro reproduction of different mutations of numerous diseases: the first successful in vitro model of a genetically inherited disease was on spinal muscular atrophy in 2008 (Ebert et al., 2009).
Overall, iPSCs can be employed to model neural differentiation or specific disease pathogenesis, helping to elucidate biomolecular mechanisms involved in neural degeneration and identify new drugs (Goncalves and Przyborski, 2018). Moreover, they are the main promising candidates for neural regeneration and for investigating molecular pathways involved in this process.
Despite all the relevant advantages, the experimental reproducibility remains one of the main challenges in hiPSC-based research, and must be taken into account: approaches to limit variability in protocols and data need to be addressed. It is mainly due to: genetic heterogeneity from donors, reprogramming methods of the isolation source (skin or blood cells), changes induced during passages in culture and differentiation protocols (Ardhanareeswaran et al., 2017; Dutan Polit et al., 2023; Hong et al., 2023).
3 Tailored biomaterials to support the culture of specific cell subtypes
The extracellular matrix (ECM) is a dynamic environment composed of structural and functional molecules where neurons and glial cells. It occupies almost 20% of the entire volume of the brain and it has a key role in regulating tissue homeostasis. Indeed, The ECM influences and directs cell behavior and fate, therefore the ECM composition and structure are tissue-specific and vary during tissue development and maturation to steer cell behavior. Moreover, pathological pathways lead to several modifications of the ECM compositions, contributing to the pathogenesis of CNS disorders.
In the CNS, the fundamental chemical components of ECM are hyaluronan acid (HA), tenascin-R, sulfated proteoglycans, mainly chondroitin sulfate and heparan sulfate (HS) and link proteins such as cartilage link protein and brain-derived link protein; the molecules constituting the ECM are organized to form a random matrix or a specific framework, such as perineuronal nets (PNNs), perisynaptic nets or the basement membrane of the blood-brain barrier (BBB) (Benarroch, 2015).
In the next section, we will focus on biomaterials applied to artificially reproduce the composition of the ECM of the CNS, supporting cell adhesion and growth as well as specific neural subtype differentiation. Indeed, the wide range of available biomaterials allows mimicking many ECM properties and, depending on the final application, the most suitable biomaterial is employed. Regarding natural biomaterials, many components are actually found in the native ECM of the CNS. For instance, HA is extensively employed in neural tissue engineering because is a major component of the ECM in the CNS; collagen type I, despite not being abundant in the CNS, is widely used thanks to its cell-binding sites; and chitosan resembles glycosaminoglycans thanks to its molecular structure. Overall, natural polymers have remarkable biological properties but lack batch-to-batch properties control and reproducibility. For these reasons, also synthetic biomaterials, such as polyethylene glycol (PEG) and polycaprolactone (PCL) have been largely employed in this research field, showing interesting results thanks to their highly controlled physical and chemical properties (Boni et al., 2018).
3.1 Neurons
Neurons represent the primary functional units of the CNS, and they are responsible for the propagation of the nervous signals in an organized and rapid manner. Hence, the main CNS diseases are associated with neuronal dysfunctions, making the understanding of the pathological pathways a crucial issue. Neuronal cultures are used to model the pathogenesis of neurodegenerative diseases and to investigate regenerative therapies that foster tissue repair and functional recovery after CNS injury. Therefore, custom-designed scaffolds could improve the neuron commitment to specific subtypes.
Among available biomaterials, chitosan, a natural polysaccharide derived from chitin, has been selected to develop neuron substrates thanks to its well-documented biocompatibility, easy processability, low immunogenicity and ability to foster cell adhesion.
For instance, chitosan with the addition of β-glycerophosphate has been used to develop a thermosensitive hydrogel where NSC-34 cell line proliferates and differentiates under retinoic acid (RA) induction toward motor neuron cells. High viability was demonstrated by Live/Dead assay, underlying the biocompatibility of the material and motor neuron differentiation was achieved by increased expression of neurofilament-H (Stanzione et al., 2021). Furthermore, chitosan was also able to support the differentiation of progenitor cells into motor neuronal lineage when modified with lactose moieties to improve its surface hydrophilicity (Medelin et al., 2018). More often, the developed 3D models allow to assess the role of chitosan hydrogels in promoting neuronal differentiation and/or the neurite formation, without evaluating the differentiation into a specific neuronal subtype. For example, the work of Guan and al. highlights how blending chitosan with gelatin, HS and HA can support the growth of NSCs and enhance their neuronal differentiation upon induction (Guan et al., 2013). These studies demonstrated that chitosan supports the growth of neuron cells, but that different modification of the biomaterial could ameliorate biological properties to better support neuronal cells.
Alternatively to chitosan, also alginate has been largely applied (Gilmozzi et al., 2021). In a recent paper, researchers reprogrammed peripheral blood mononuclear cells of amyotrophic lateral sclerosis patients and healthy controls into iPSCs and differentiated them into motor neuron cells encapsulated in a 3D hydrogel composed of cellulose nanofibrils and alginate (Scarian et al., 2022). Similarly, Gilmozzi and colleagues established a novel system to promote the differentiation of human iPSCs into dopaminergic (DA) neurons, exploiting alginate/fibronectin beads: the electrophysiological and metabolic parameters were evaluated showing increased functionality compared to monolayer cultures. Protein levels of tyrosine hydroxylase (TH) and G protein-gated inwardly rectifying potassium channel 2 (GIRK2), commonly used as markers for mature DA neurons, were evaluated showing high expression (Gilmozzi et al., 2021). In another work, a synthetic polymer composed of Poly (N-isopropylacrylamide)-PEG has been also used to differentiate stem cells in DA neurons: indeed, 25% of cells exhibited midbrain DA neuron-like spiking behavior, indicating a functional phenotype (Adil et al., 2017).
Finally, a recent study evaluated the neuronal differentiation of rat ectomesenchimal stem cells isolated from the cranial neural crest in a sodium alginate-Matrigel hydrogel: it was promoted the growth and differentiation of stem cells enhancing neurite elongation compared to 2D controls, making this biomaterial a valuable candidate for neuroregeneration (Li Y. et al., 2021).
Overall, a gold-standard biomaterial for neuronal differentiation is still not established, but many are the compositions under investigation to elucidate how each biochemical properties influence molecular mechanisms and cell fate in 3D environments. To achieve this, peptide sequences were investigated for supporting neuronal differentiation, thanks to the high specificity of these short amino acid sequences. For instance, the Ile-Lys-Val-Ala-Val (IKVAV) functional peptide derived by the α1 chain of ECM-protein laminin, a major component of the CNS ECM, and RADA16, a self-assembling peptide appended with IKVAV and arginylglycylaspartic acid (RGD) peptide motif have been engineered. It was found that they promote the adhesion and differentiation of encapsulated NSCs (Sun et al., 2016; Yang et al., 2021).
3.2 Astrocytes
The percentage of astrocytes is still debated, but their number is estimated around 20% of the total number of cells in any CNS region (Sun et al., 2017; Valério-Gomes et al., 2018).They play a key role in the system homeostasis, including control of neurotransmitters and intra/extracellular ion concentrations. Indeed, astrocytes are involved in several CNS functions and have a key role in disease progression, from neurodegenerative disorders to injuries. In particular, after injury, a process named astrogliosis occurs with astrocytes changing dramatically their gene expression and morphology, showing a reactive phenotype which enhances their proliferation rate and upregulates the production of GFAP and Vimentin. Moreover, reactive astrocytes increase proteoglycans expression leading to the formation of the glial scar, a physical obstacle for axon regeneration (Gottipati et al., 2020). Therefore, biomaterials and astrocyte cultures (eventually in combination with other CNS cells) allow to study and modulate the glial activation, but also to analyze cell-cell interactions, efficacy of drugs and biocompatibility of the materials. The main two parameters to take into consideration working with 3D astrocyte models are (i) maintaining a non-activated phenotype of cells of cells and avoiding upregulation of GFAP, and (ii) achieving a physiological morphology (Gradišnik et al., 2021).
Lau and colleagues demonstrated that PCL scaffolds allowed primary astrocytes to mimic in vivo phenotype and stellated morphology. The evaluation of F-/G-actin and GFAP expression demonstrated a decrease in cytoskeleton stress, while the presence of mature and healthy astrocytes was confirmed by the upregulation of genes coding the brain-derived neurotrophic factor, the excitatory amino acid transporter 2 and anti-oxidants. Therefore, PCL scaffolds could be used for CNS repair strategies, supporting the growth of non-reactive astrocytes (Lau et al., 2014). Likewise, reduction of GFAP immunoreactivity was achieved by Gottipatti et al. by carbon nanotube films, meaning that this material could be used as a coating for electrodes in brain machine interface (Gottipati et al., 2013). Similarly, Placone et al. demonstrated that an optimized hydrogel of collagen, HA and Matrigel, did not upregulate GFAP and maintained star-shaped morphology of primary astrocytes (Placone et al., 2015). This was also recently obtained with a synthetic hydrogel, that could maintain astrocyte quiescence, but on demand could also activate them. In particular, authors developed a specific model that resembles many characteristics of human brain ECM: the model was a modulus-tunable PEG hydrogel with matrix metalloprotease (MMP) - degradable and integrin-binding peptides (Galarza et al., 2020). However, additional studies are required to better describe the on demand activation mechanism.
3.3 Oligodendrocytes
Oligodendrocytes (OLs) derive from oligodendrocyte progenitor cells (OPCs) and are the CNS cell population responsible for myelin sheath formation around neuronal axons. OLs are mainly involved in SCI pathology: indeed, after injury, the process of remyelination is impaired as OPCs are abundantly recruited to the lesion site but the myelin produced is usually thinner than the physiological one hampering the efficacy of neuronal activities. Therefore, it is fundamental to study the remyelination pathway after SCI in vitro for preserving neurological functions. Nonetheless, OLs are involved also in multiple sclerosis, where demyelination occurs due to an autoimmune response by activation of macrophages, T and B cells. Spontaneous remyelination in vivo is limited due to restricted availability and proliferation of OPCs and poor differentiation into OLs. Many cell therapies based on precursor or stem cells have been explored; however, injection strategies often fail because cells do not survive or do not differentiate into functional OLs (Russell and Lampe, 2016). Therefore, biomaterials can have a beneficial role both in the survival and differentiation of OLs, improving their remyelination capability.
In 2014, Shah et al. evaluated the effect of graphene oxide (GO) coating on PCL nanofibrous scaffold in inducing NSC selective differentiation into OLs: the authors found an increase of the expression of myelin basic protein proportional to the GO concentration (Shah et al., 2014).
However, regarding the 3D in vitro study of OLs, hydrogels have been the most investigated as they hold numerous essential properties for the survival, proliferation and differentiation of OLs. For instance, Furhmann and colleagues investigated the influence of RGD peptide in the growth of OPCs within hyaluronan and methylcellulose hydrogel, showing significantly greater survival (Führmann et al., 2016). Geissler employed collagen I to tailor mechanical properties, HA to mimic brain ECM and laminin to promote OLs maturation, demonstrating that NPCs increased their differentiation into OLs compared to the 2D milieu (Geissler et al., 2018). Interestingly, Egawa and colleagues obtained the complete differentiation of rat primary OPCs into OLs by using differentiation media in a 3D hydrogel of collagen and hyaluronan: this hydrogel allows OPC to extend in all directions and therefore to finely analyze cell-cell contacts (Egawa et al., 2017). Finally, human pluripotent stem cells were successfully differentiated in OPCs thanks to a Poly (N-isopropylacrylamide)-PEG thermoresponsive hydrogel and the addition of Smoothened agonist and RA (Rodrigues et al., 2017).
4 Modeling neural microenvironment to guide in vitro tissue maturation
Nowadays, neural tissue engineering approaches are extensively used in the modeling of CNS, aiming at reproducing the composition, structural complexity and functionality of CNS tissue with greater reliability. The combination of the latest biofabrication technologies with cell biology and biomaterial science has resulted in substantial progress in modeling the neural microenvironment, as several strategies have been proposed to promote the maturation of microtissues towards in vivo-like physiological conditions. Indeed, beyond scaffold composition, also the mechanical and physical properties, such as stiffness and topography have to be finely-tuned to reproduce ECM architecture and to guide cell fate and behavior.
This section provides a summary of the most common methods used for directing neural cell differentiation and enhancing axonal growth and neural network formation (Figure 3).
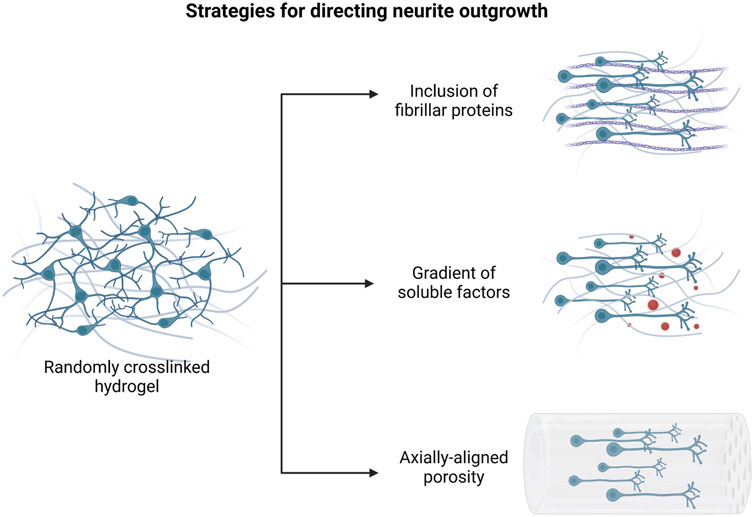
FIGURE 3. Main approaches to promote directional axonal elongation in engineered in vitro neural microenvironments. Created with BioRender.com.
4.1 Tuning biomaterial properties to direct cell behavior
Among the key parameters to be considered in model designing, the physical, mechanical and biochemical properties of the biomaterials are fundamental in modulating cell response. Indeed, several studies have demonstrated the high influence of the hydrogel stiffness in directing neural differentiation. It has been reported that NSCs preferentially differentiate into neurons in matrix with elastic modulus lower than 1 kPa, while stiffer materials induce their differentiation into astrocytes (Fan et al., 2018; Zhou P. et al., 2020; Liu X. et al., 2021). Thus, that feature would be exploited to create multicellular constructs starting from a single stem cell population simply by changing hydrogel concentrations to define cell phenotypes.
Additionally, the mechanical properties of the culture matrix have an important role in promoting or inhibiting neurite outgrowth (Woods et al., 2022). Hippocampal neural progenitor cells cultured on HA-based hydrogels showed different behavior according to the variation of hydrogel storage modulus between 400 Pa and 800 Pa. Indeed, a significant increment of neurite density and extension into HA matrix has been detected in the case of the softer hydrogel (Tarus et al., 2016). Similarly, human iPSC-NPC spheroids encapsulated in methacrylated HA hydrogel showed enhanced neurite outgrowth in soft hydrogel (with a stiffness of 0.51 ± 0.20 kPa), compared to the stiffer one (with a stiffness of 1.41 ± 0.27 kPa) (Wu et al., 2017).
As regards to the strategies based on biochemical cues, the incorporation of neurotrophic factors within scaffolds has been largely used to induce neural differentiation and promote directional axonal elongation. For example, it has been demonstrated that the neuronal/astroglial differentiation of NSCs may be adjusted by modulating nerve growth factor concentration in the hydrogel matrix (Gao et al., 2022). It has also been reported that the inclusion of a RA gradient into PCL-gelatin methacryloyl (GelMA) scaffolds can induce the differentiation of embryoid bodies into different neuronal identities (Hamid et al., 2021). By contrast, the incorporation of stromal-derived factor 1 spatial gradient into a hydrogel scaffold did not significantly affect NSC behavior comparing with uniformly distributed growth factor hydrogels, suggesting the importance of the gradient range selection and its long-term maintenance (Li C. et al., 2021).
4.2 Designing model geometry and topographical cues
Several reports have already demonstrated as the geometrical and topographical features of the culture substrate impact on neural cell behavior in many respects, providing specific stimuli for cell adhesion, migration and differentiation (Farrukh et al., 2018). Hence, the inclusion of anisotropic patterners, both at microscale and nanoscale level, has been widely investigated to promote engineered tissue maturation and neural functionality.
For instance, the integration of fibrillar proteins as hydrogel matrix components has been proved to direct aligned axon extension, due to the presence of specific cell binding sites for neurite attachment and outgrowth. Woods et al. reported that the functionalization of HA hydrogel scaffolds with collagen type-IV and fibronectin results in increased metabolic activity of SH-SY5Y cells and in the formation of neurite interconnections into the cell networks (Woods et al., 2022).
On the other hand, many studies have focused on the fabrication of bioinspired scaffolds, containing axially-aligned channels or oriented porosity, intended for providing physical support to axon elongation and tissue regeneration. Several strategies have been optimized in order to modulate the geometrical properties of the constructs, including both conventional and more advanced biofabrication methods. As examples, Winter and colleagues created an engineered microtissue constituted by hydrogel micro-columns which support the formation of an aligned astrocyte network. Their results suggested that structural parameters, such as channel radius or angle of curvature, influence astrocyte morphology and alignment (Winter et al., 2016). Similarly, Onoe et al. provided a deeper analysis of the influence of scaffold geometry on NSC differentiation: specifically, they developed coaxially extruded hydrogel microtubes and they assessed the effects of the variation of core diameter and shell thickness on the morphology and mRNA expression of encapsulated cells (Onoe et al., 2016).
Furthermore, it has been shown that the creation of density patterns in the matrix architecture also results in oriented axonal growth (Jang et al., 2015). In the work of Bang and colleagues, the application of a hydrostatic pressure during Matrigel gelation phase allowed for the modulation of the hydrogel crosslinking, resulting in a controlled alignment of ECM fibers in the flow direction. Spatially dependent neurite extension and formation of neural bundles were observed in the patterned hydrogel, inside which axons were longer and concentrated at the center of channels. On the contrary, no differences in neurite distribution were detected in randomly crosslinked Matrigel (Bang et al., 2016).
4.3 Enhancing the electroconductive properties of biomaterials
ECM is characterized by electroconductive properties, that are essential for the correct transmission of intercellular signals and the preservation of neural network functionality. Hence, various hydrogel-based systems integrating electrically conductive components have been developed to mimic the electrophysiological characteristics of neural microenvironment and foster tissue maturation. Importantly, these systems are also attractive as suitable platforms for external electrical stimulation, whose application in 2D culture has led to good results in terms of neuronal expression and neurite outgrowth (Warren et al., 2021).
Few studies reported the inclusion of carbon-based materials into 3D scaffold-based models. McNamara et al. investigated the influence of graphene-alginate microfibers on dopaminergic neurons, by analyzing the genetic expression of cells up to 6 days after encapsulation. Their findings suggested that the presence of graphene increased the conductivity of wet hydrogel microfibers to a value of the same magnitude as that of the native brain tissue. The introduction of graphene led to the upregulation of TH gene, indicating an increased dopaminergic activity, and to significant changes in the level of βIII-tubulin expression, commonly associated to neuronal maturity (McNamara et al., 2021). Similarly, Zhu et al. optimized a bioink consisting of GelMA, graphene nanoplatelets and NSCs for stereolithography-based 3D bioprinting: according to their results, the nano-composite hydrogel supported cell viability and proliferation, as well as promoted neuronal differentiation and neurite elongation (Zhu et al., 2016). In the work of Huang and colleagues, the addition of graphene, in a Pluronic-stabilized form, into a polyurethanes (PU)-based bioink improved the oxygen metabolism of the embedded NSCs and effectively promoted their differentiation (Huang et al., 2017).
Moreover, several electroactive hydrogels have been designed, involving the use of electrically conductive polymers, such as poly (pyrrole) (PPy), PEDOT or polyaniline (PANI). Wu et al. proposed a cell-laden hydrogel microfiber matrix that combines collagen and PPy nanoparticles: this system was shown to promote the neurogenesis of the encapsulated PC12 cells due to the enhanced conductivity of the substrate, as demonstrated by the increased expression of proteins related to calcium ion channels. Additionally, its capacity of mediating external electrical stimulation was assessed, highlighting the importance of matrix conductivity for signal transmission (Wu et al., 2019). Luo et al. reported that NSCs preferentially differentiated into neurons and oligodendrocytes instead of astrocytes on the surface of electroconductive ECM-based hydrogels containing PPy (Luo et al., 2022). Concerning PEDOT, it has also been proved that its incorporation, modified by chondroitin sulfate and tannic acid as doping agents, into a photo-crosslinkable bioink is beneficial for NSC proliferation and differentiation towards neuron phenotype (Song et al., 2022). Lastly, Liu et al. investigated the use of a thermosensitive hydrogel including PANI as electroactive component in combination with biochemical and electrical stimulation: in vitro assays showed the synergic effects of external electric stimulation and growth factor release on neural differentiation of PC12 cells and axonal growth (Liu W. et al., 2021).
5 3D in vitro systems to recreate the CNS
One of the major limitations in the comprehension of neurodegenerative diseases and the development of novel regenerative treatments is the lack of reliable preclinical in vitro models (Slanzi et al., 2020). Indeed, they fail to reproduce the complexity of CNS tissue, resulting in a poor predictability of drug therapeutic response in patients. For many years, the heterogeneity of the CNS microenvironment has hindered progress in this field, as the maturation of the neural network is closely connected to the presence of several specific biological and mechanical cues which are difficult to recreate in vitro. In this context, the construction of 3D engineered tissue has shown great promise in the development of more reliable in vitro models, as it provides an artificial environment for cell growth and interactions with the surrounding matrix with higher physiological relevance. Thus, a wide range of biofabrication techniques, including the use of supporting biomaterials and living cells, have been explored to develop complex in vitro systems mimicking the architecture and composition of nervous tissue. Conventional methods, such as solvent casting, freeze-drying, phase-separation (Papadimitriou et al., 2020), or simple hydrogel-based systems (Madhusudanan et al., 2020), have been employed to fabricate microporous structures, constituted by both natural and synthetic materials with tailored biochemical and mechanical properties. However, most of these techniques present many disadvantages, mainly associated with the impossibility of modeling complex tissue geometry and cytoarchitecture, long processing time, and poor reproducibility (Papadimitriou et al., 2020).
Recently, technological advancements in additive manufacturing technologies and microfluidic devices have led to the development of novel approaches aimed at more faithfully replicating neural microenvironment with different grades of complexity. Indeed, additive manufacturing technologies rely on the use of rapid prototyping processes that allow for the automated fabrication of customizable structures starting from computer-aided design objects. In this way, precise control of the final geometry and model composition can be achieved, along with constant monitoring of process parameters and times (Melchels et al., 2012). Hence, the integration of these methods in neural tissue engineering approaches offers the chance to develop multifunctional systems, useful for the in vitro modeling of CNS, both in healthy and pathological conditions, and drug screening. Additionally, the standardization of these processes would ensure high model reproducibility and simple handling which are essential requirements for subsequent applications in preclinical trials and medical practice.
Among the latest biofabrication techniques, 3D bioprinting has recently gained increasing attention, as it allows for the creation of complex engineered constructs with precisely arranged biomaterials, living cells, and bioactive factors according to predesigned patterns. Thus, 3D bioprinted systems are emerging as effective tools for the modeling of many distinctive mechanisms of CNS pathophysiology, the reproduction of physiological structures, and the development of implantable scaffolds (Cadena et al., 2021).
Microfluidic-based platforms, meanwhile, have been extensively used because of their inherent capability of closer replicating the dynamicity of in vivo environment, by including perfusable microchannels into the model. Additionally, they can be specially designed to incorporate multiple culture chambers, that can be interconnected or isolated from each other, thus enabling the establishment of different cell culture conditions. These features make them attractive solutions for many applications, such as the modeling of CNS tissue-specific interfaces or neurodegenerative diseases and high-throughput drug screening (Teixeira et al., 2020).
Furthermore, the combination of the aforementioned technologies with existing 3D cell biology-based models, such as organoids, could be a significant step toward the realization of comprehensive and relevant CNS models. Notably, organoids derived from PSCs have been proven to successfully model key mechanisms of neural development and neurodegenerative diseases, due to their multicellular structure and self-organizing capability (Kelava and Lancaster, 2016; Grenier et al., 2020). It has also been reported that the use of ECM-like biomaterials (i.e., Matrigel (Lancaster et al., 2013) and collagen hydrogel (Zafeiriou et al., 2020)) can promote neuronal maturation in brain organoids, providing environmental cues for their growth. However, several limitations are commonly associated with organoids, including their inherent variability and the lack of vasculature which hinder nutrient exchanges inside the structures. In that regard, the use of 3D bioprinting could bring significant advantages in the development of organoid-based systems, both in terms of reproducibility and cellular complexity, by enabling precise cell positioning into more sophisticated architectures. Otherwise, the inclusion of organoids into microfluidic 3D platforms allows for controlled vascular perfusion and the establishment of compartmentalized co-culture systems (Adlakha, 2023).
This section provides a brief overview of the biomaterials and latest biofabrication techniques employed in CNS research (Table 2), focusing on 3D bioprinting technologies and microfluidics, and a selection of the most recent 3D in vitro systems for CNS modeling.
5.1 3D bioprinting for CNS applications
3D bioprinting is commonly referred to a set of rapid prototyping techniques which consist in the controlled deposition of a cell-laden biomaterial, defined as bioink, following a predesigned geometry (Askari et al., 2021). Compared to other additive manufacturing approaches, mostly based on the establishment of culture systems starting from cell-free scaffolds, this technology brings several advantages. Firstly, it enhances the establishment of cell-matrix interactions through the direct combination of cells and biomaterials as well as the inclusion of bioactive factors, that can be distributed creating physical and biochemical gradients (Hamid et al., 2021). Moreover, this method allows the possibility to mimic tissue morphology with higher reproducibility and spatial resolution and to integrate multiple cell types according to the in vivo cytoarchitecture (Joung et al., 2018).
As previously discussed, the neural microenvironment is characterized by the presence of different specialized compartments including the PNNs, the perisynaptic nets, and the basement membrane, where the ECM components arrange themselves in anisotropic and hierarchical structures, essential for maintaining a good functionality of the neural circuit (Benarroch, 2015). For this reason, 3D bioprinting technologies are gaining popularity in the field of CNS tissue engineering, as they offer the opportunity of more accurately modeling the neural environment, providing new platforms to investigate the mechanisms involved in neurodegenerative diseases and tissue regeneration.
5.1.1 Tailored bioinks for CNS
Among the key factors to consider in the engineering of neural tissue constructs, an appropriate selection of scaffold biomaterials is crucial for efficiently supporting neural cell culture over time. Indeed, encapsulated cells interact with the surrounding matrix, modifying their phenotype and behavior in response to environmental stimuli, directly related to the biological and physical features of the bioink. Due to its special composition, rich of highly hydrophilic molecules, the nervous tissue displays relatively low stiffness which is difficult to achieve in vitro without affecting the structural integrity of the culture system (Axpe et al., 2020). Thus, soft hydrogels have been largely employed in neural tissue modeling as they enable the establishment of an in vivo-like environment characterized by relatively low stiffness and availability of cell-binding sites (Madhusudanan et al., 2020). Hydrogels are highly hydrophilic meshes composed by polymers of synthetic or natural origin, capable of incorporating a large amount of water, which makes them a particularly suitable substrate for cell culture. Indeed, their porous structure promotes cell adhesion and proliferation in a more physiological 3D condition, while allowing a sufficient diffusion of oxygen and nutrients in absence of vascular perfusion. According to their composition and the crosslinking strategy used, hydrogels can be specifically modulated to guide neural cell differentiation and maturation.
Natural hydrogels, constituted by single polymers or more often by a combination of them, have been mostly selected as bioinks because of their inherent biocompatibility and bioactivity. Common polysaccharides such as HA, chitosan, alginate and gellan gum are chosen to fabricate extrudable hydrogels (Lozano et al., 2015; Gu et al., 2016; Salaris et al., 2019), along with purified fibrillar proteins like collagen, fibrin or silk fibroin, useful for enhancing cell adhesion and guiding neurite outgrowth (Abelseth et al., 2019; Ngo et al., 2020; Sanz et al., 2021). In addition, many of these polymers can be chemically modified by the introduction of functional groups to allow fast gelation of the hydrogel precursor solution and further regulate its degradation rate. Indeed, these are fundamental requirements for achieving high shape fidelity and ensuring long-term stability of the printed construct. Specifically, semi-synthetic polymers like GelMA or HA methacrylate, obtained by adding photosensitive methacrylate groups to gelatin or hyaluronic acid respectively, have been successfully used in a wide variety of tissue engineering applications, including photocurable hydrogels scaffold and 3D bioprinting (Liu et al., 2017; Wu et al., 2017; Yin et al., 2018; Spearman et al., 2020). As example, it has been reported that NSCs encapsulated into soft GelMA hydrogels exhibited high viability and proliferation after 7 days in vitro and were able to differentiate into neurons (Zhou P. et al., 2020).
Lastly, hybrid hydrogels composed of natural and synthetic polymers have been recently investigated with the aim of increasing bioink performance in terms of viscosity and processability. For instance, the combination of thiolated Pluronic F-127 with dopamine-conjugated gelatin and HA resulted in a thermally gelling bioink used for the bioprinting both of PC-12 cells and human glioma cells with excellent control of the geometry despite of the hydrogel low elastic modulus (Haring et al., 2019).
An additional approach involves the use of PU, a class of synthetic biodegradable polymers largely employed in the biomedical field because of their biocompatibility and tunable physical properties. Hsieh et al. developed a thermoresponsive PU hydrogel system, starting from two different formulas of PU dispersion, characterized by easy gelation at physiological conditions and appropriate rheological behavior for 3D bioprinting. NSCs embedded in the bioinks display high viability after the printing process and enhanced expression of neuronal or glial genes according to the hydrogel stiffness (Hsieh et al., 2015).
5.1.2 3D bioprinted in vitro systems for CNS modeling
Modeling the CNS remains extremely challenging, and many key issues are still under investigation to validate the reliability of 3D bioprinted models as alternative platforms for pre-clinical and clinical studies. However, actual research is making numerous efforts and progress in the development of efficient bioprinting strategies and the creation of neural functional tissues. To date, numerous examples of neural-like constructs have been designed, intended for replicating many features and mechanisms existing in CNS, starting from neurogenesis and neural network formation to more sophisticated tissue geometry and cytoarchitecture.
5.1.2.1 3D bioprinting for steering neural differentiation and maturation
Several studies are focusing on the encapsulation of NSCs into printable hydrogel systems aiming at inducing their differentiation and the establishment of mature networks. Gu et al. 3D-printed frontal cortical human NSCs within a bioink composed by alginate, carboxymethyl-chitosan, and agarose. The bioink composition was optimized to maximize hydrogel printability and obtain well-defined mechanical properties and high permeability after crosslinking. This led to uniform distribution of NSCs within the printed construct and a high level of cell viability and proliferation up to 10 days after printing. In situ differentiation of encapsulated cells mainly results in GABAergic neurons and neuroglia, as confirmed by immunocytochemistry and gene expression analysis. The functional maturation of differentiated neurons was also demonstrated by calcium imaging results and by the presence of extending neurite outgrowth shown in the SEM images (Gu et al., 2016). Interestingly, the same multi-component hydrogel was also used to create 3D bioprinted neural tissue from iPSCs. These cells are extremely sensitive to environmental stimuli, and their integration in 3D bioprinting models requires an accurate optimization of the fabrication parameters. Gu et al. successfully bioprinted iPSCs without affecting their pluripotent state, as confirmed by their subsequent differentiation to multiple germ lineages. Then, a direct differentiation of iPSCs, driven by brain-derived neurotrophic factor-supplemented medium, was obtained, resulting in different neuronal and glial cell subtypes (Gu et al., 2017). The reported results highlighted the efficiency and flexibility of the bioprinting platform developed by the authors, who suggested a further application in the modeling of neural development and diseases.
In another study, by contrast, the 3D bioprinting technique was tested as a potential tool for reprogramming human dermal fibroblasts into neural crest-like stem cells. Fibroblasts were encapsulated into a thermoresponsive PU hydrogel together with FoxD3 plasmid transcriptional factor and in situ cell transfection was achieved, mediated by the extrusion forces. The shear stress generated by the extrusion process was optimized to induce a transient membrane permeability which allows the transfection without impacting cell viability. In addition, the differentiation of reprogrammed cells was investigated to produce neural tissue constructs after induction (Ho and Hsu, 2018).
3D bioprinting has been also employed by Lee et al. to create multicellular neural constructs including rat embryonic neurons and astrocytes. Cells suspended in the culture media were extruded on a printed collagen hydrogel layer, according to different geometries to obtain single-layered or multi-layered composites. Various parameters such as cell density, collagen concentration and the printing resolution were optimized, investigating their influence on cell viability and neurite outgrowth and interconnections (Lee et al., 2009).
5.1.2.2 3D bioprinting to model cerebral cortex
The possibility of accurately positioning cells into a layered structure was also explored to reproduce brain-like constructs. Indeed, human cerebral isocortex is constituted of six neuronal layers including different cell subtypes with distinct functions in brain activity. Thus, replicating this specific feature in vitro would be crucial for a deeper understanding of brain functioning and neurodegenerative disease pathogenesis. Lozano and colleagues developed a 3D bioprinted scaffold simulating the laminated architecture of the cerebral cortex. An RGD-modified gellan gum hydrogel was used for the encapsulation of primary cortical neurons and the subsequent bioprinting through a hand-held printing process. They validated the ability of this technique to deposit up to six distinguished layers of biomaterials, without affecting the integrity of the structure. Moreover, a three-layered construct, that alternates cell-loaded and acellular regions, was used to investigate neural maturation and network formation. As shown by immunofluorescence analysis, discrete layers of neuronal cells were maintained and axonal growth inside the acellular layer was detected at 5 days of culture (Lozano et al., 2015).
An alternative, but equally effective approach has been experimented by Zhou et al., who exploited patterned cell arrangement to investigate neuron and astrocyte interactions in human brain development. They employed an innovative bioprinting method, based on the deposition of cell-loaded Matrigel droplets in a lipid oil bath. This technique allows for the construction of engineered soft tissue, with high control of the overall geometry and cell distribution. By encapsulating iPSC-derived NSCs and human primary astrocytes in different patterns, they were able to simulate some cortical developmental processes, including cell migration or segregation and neuronal differentiation and astrogenesis (Zhou L. et al., 2020).
5.1.2.3 3D bioprinting for spinal cord modeling
A large number of studies have been also focused on the fabrication of 3D bioprinted spinal cord models for reproducing its cytoarchitecture and, in particular, for developing valuable platforms for the investigation of SCI pathophysiology and the development of novel treatments. Considering the complexity of spinal cord structure and its heterogeneity in the cellular composition, a good control of cell spatial arrangement within the printed scaffold turns out to be crucial for the biomimicry and functionality of the model. Liu et al. employed 3D bioprinting technology to develop a neural construct inspired to the white matter of spinal cord tissue. A multimaterial bioink (composed of hydroxypropyl chitosan, thiolated hyaluronic acid, vinyl sulfonated hyaluronic acid and Matrigel) was optimized to support the growth of NSCs and their subsequent neural differentiation. Viability analysis revealed a high proliferation rate of encapsulated cells up to 7 days after bioprinting, thus confirming that the extrusion process did not affect their stemness. In addition, immunocytochemistry data showed that NSCs expressed both astrocyte and neuronal-specific markers and formed a mature neural network after 21 days in vitro. As it was expected, the increase of hydrogel concentration led to an overexpression of glial-specific marker, suggesting that changes in hydrogel mechanical properties would direct NSC fate (Liu X. et al., 2021).
A different approach was proposed by Joung et al. where a multicellular scaffold was fabricated starting from two distinct cells populations. Specifically, they combined the use of human iPSC-derived spinal NPCs and OPCs with a point-dispensing bioprinting technology. Neural cells encapsulated in Matrigel were precisely printed inside the channels of a biocompatible hydrogel scaffold, which provided mechanical stability and facilitated directional axon propagation. Additionally, the detection of intracellular calcium influxes confirmed cell differentiation into mature functional neurons (Joung et al., 2018).
Similarly, Firouzian et al. created a biomimetic rat spinal cord model by using intrascaffold cell assembly. According to this method, the bioink could be printed within a prefabricated acellular scaffold that closely meets the stiffness of spinal cord tissue while supporting neural cell culture in a softer substrate. Specifically, a collagen-coated poly (lactic-co-glycolic acid) scaffold based on rat spinal cord was made by using the thermally induced phase separation casting technique to achieve a directional microporous structure. Then, primary neural cells incorporated in a multimaterial hydrogel (obtained by blending gelatin, alginate and fibrinogen) were seeded within the channels through an image-guided 3D printing technology. Results showed that the scaffold displays heterogeneous mechanical properties among the cellular and acellular compartments, resembling the native tissue. The viability analysis and immunostaining imaging revealed a uniform distribution of neural cells inside the construct with high viability and maturation over 14 culture days in vitro (Firouzian et al., 2020).
A further example of this multimaterial approach was reported by Hamid et al. who developed a spinal cord model aimed at reproducing the neurogenesis process by the 3D bioprinting of embryoid body-loaded GelMA bioink in a PCL tubular scaffold. The geometry of the construct was carefully designed to ensure appropriate oxygen and nutrient diffusion inside the hydrogel and to provide the formation of a concentration gradient of biomolecules through the structure. The immunocytochemistry analysis demonstrated the differentiation of embryoid bodies into different neural cell identities according to the presence or not of RA, highlighting the importance of growth factor gradients for enhancing tissue biomimicry (Hamid et al., 2021).
5.1.3 Novel technologies for 3D bioprinting of neural tissues
Although the promising results mentioned, innovative biofabrication technologies are emerging to overcome some limitations associated to traditional 3D bioprinting techniques. Indeed, several issues are commonly related to the extrusion process, such as high shear stress resulting in low cell viability or poor geometry definition and reproducibility. Moreover, it is very difficult to achieve an optimal balance between printing fidelity and cell response, especially when soft matrices are required for tissue modeling (Qiu et al., 2020).
For this reason, alternative bioprinting systems, including the use of microfluidic printheads and core-shell nozzles (Figure 4), are considered of particular interest, as their application in CNS engineering would facilitate the printing of low-moduli hydrogels and would provide new crosslinking strategies to achieve a higher cell viability and more refined structure fidelity and stability.
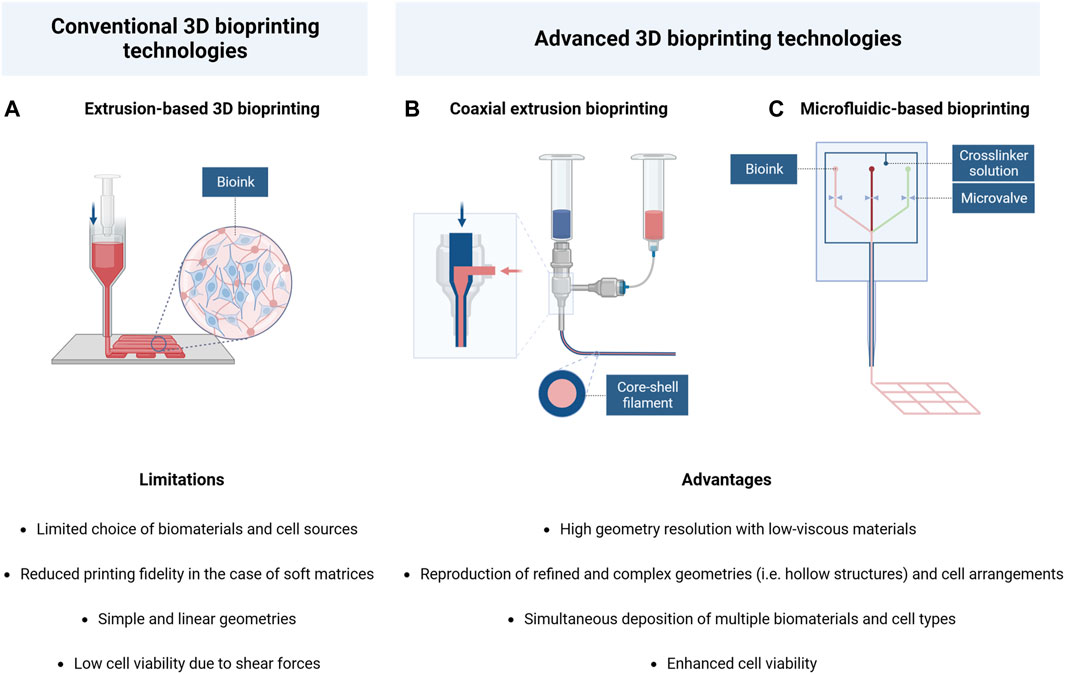
FIGURE 4. Schematic illustration and comparison of conventional and advanced 3D bioprinting technologies: (A) Extrusion-based bioprinting; (B) coaxial extrusion bioprinting; (C) microfluidic-based bioprinting. Created with BioRender.com.
Specifically, the coaxial bioprinting technology is characterized by the presence of a cylindrical nozzle constituted by an inner core channel surrounded by a concentric outer shell, which allows the simultaneous flow of two different materials to form the final filament. Hence, this technique has the advantage of allowing the reconstruction of physiological hollow structures (such as blood vessels or nervous fibers) by respecting the in vivo cellular arrangement (Jia et al., 2016; Kjar et al., 2021).
In addition, this technique has been also exploited to bioprint highly concentrated cell suspension surrounded by an external supporting hydrogel shell. It has been reported that this approach could facilitate cell self-assembling in the core portion, without affecting the structural integrity of the model (Li et al., 2019). Zhang et al. investigated the role of coaxial bioprinted microenvironment on NSC behavior by comparing it with traditional hydrogel encapsulation. Their work was focused on the implementation of a relevant in vitro platform to study AD. The model consisted in a core-shell construct constituted by an outer layer of alginate hydrogel with inside a NSC suspension enriched with Matrigel. According to the results, cells exhibit a major tendency to self-clustering in the coaxial bioprinted structure, long-term viability, and higher expression of typical neuronal and astrocyte differentiation markers. Moreover, the protein analysis revealed that cellular environment influences the biosynthesis of many amino acids involved in metabolic pathways, with different effects on NSC growth and maturation. Lastly, the 3D model demonstrated amyloid-β protein (Aβ) aggregation and increased expression of Aβ and tau isoform genes, which are commonly considered significant AD pathological hallmarks (Zhang et al., 2022).
Microfluidic-based bioprinting is a second technique derived from traditional 3D bioprinting, which includes the use of specific printheads constituted by multiple microchannels merging into a single one in the extrusion phase. This technology allows the creation of highly defined geometry as it can combine the integration of different materials and cell types with high control of flow parameters in each channel. These systems are commonly applied for the simultaneous extrusion of soft hydrogel precursor solutions and crosslinking agents, resulting in fast gelation and good shape fidelity of the bioink, even in the case of low-viscous materials (Warren et al., 2021). In addition, these characteristics make microfluidic bioprinting particularly advantageous for cellular viability because it provides the possibility of reducing the pressure applied in the printing process, thus protecting the encapsulated cells from high shear stress.
In some recent studies, this technology has been frequently exploited to bioprint iPSC-derived neural cells, being particularly susceptible to environmental stimuli due to traditional extrusion processes. Salaris et al. developed a 3D neural construct by using iPSC-derived cortical neurons and glial precursors and a microfluidic-based bioprinter. The bioink solution, composed of a mixture of alginate and Matrigel, was rapidly crosslinked by a CaCl2 solution extruded in a coaxial configuration. This method led to high viability of the bioprinted cells and ensured their long-term maintenance and differentiation into neuronal and glial phenotypes (Salaris et al., 2019). Actually, this work reported the longest time of survival (up to 70 days in vitro) of iPSC-derived neurons compared to other bioprinted scaffolds (Gu et al., 2017; Joung et al., 2018). Similarly, a commercial Lab-on-a-Printer technology has been employed by de la Vega et al. who developed mature human iPSC-derived neural constructs mimicking spinal cord morphology. They successfully bioprinted iPSC-derived NPCs according to a cylindrical geometry, encapsulating them in a multicomponent fibrin bioink. The quantitative analysis of cell viability showed percentages of viable cells above 81% at 7 days of culture (de la Vega et al., 2018). These values were higher than those obtained by Joung et al. (above 75% after 4 days in vitro), who printed iPSC-derived neural cells by microextrusion 3D bioprinting (Joung et al., 2018). Then, cell differentiation towards motor neurons was achieved by treating the printed construct with different specific factors, as confirmed by the expression of motor neuron-associated markers (de la Vega et al., 2018).
The same device has been also used by Sharma and colleagues to model brain tissue. They combined the just mentioned fibrin bioink with the incorporation of drug-releasing microspheres to trigger the differentiation of encapsulated cells. Specifically, the controlled release of guggulsterone induced the differentiation of human iPSC-derived NPCs towards dopaminergic neurons, thus validating the ability of microsphere-based bioinks to promote neural maturation in 3D bioprinted constructs (Sharma et al., 2020). This strategy represents a significant improvement compared to common neural differentiation methods, mostly based on the use of supplemented culture media. Indeed, the inclusion of these delivery microsystems into bioinks would create a specific microenvironment for different cell subtypes and enhance the efficiency of cell differentiation.
To conclude, the advancements in 3D bioprinting technologies offer many new opportunities for CNS tissue engineering, by introducing innovative cell-friendly printing approaches that allow for overcoming the traditional limitations in the selection of biomaterials and cell sources.
5.2 Microfluidic 3D systems for CNS modeling
In the last years, great interest has grown on developing microfluidic-based culture systems, because of their potential in neurodegenerative disease modeling and drug screening applications. These platforms differ from other 3D in vitro models due to the possibility of integrating a controlled fluid flow, which ensures the diffusion of nutrients and biomolecules even with different concentration gradients, thus contributing to the creation of a more physiological and dynamic microenvironment (Zhuang et al., 2018). These devices can be developed in different grades of complexity, starting from a single chamber chip to more elaborate systems, in which different chambers are interconnected to recreate functional tissue-tissue interfaces (Bhatia and Ingber, 2014). Importantly, microfluidic systems could be also optimized to simulate tissue-specific stimuli, such as mechanical or electrical cues, useful for achieving good functionality of the integrated microtissue.
In particular, microfluidic technology has been employed for the establishment of 3D neural culture platforms, where cells and biomaterials are carefully positioned to replicate cellular and extracellular matrix interactions (Figure 5). Indeed, these systems combine the advantages of microfluidics with those of hydrogel-based culture, thus providing relevant environmental cues for neural cell growth and maturation.
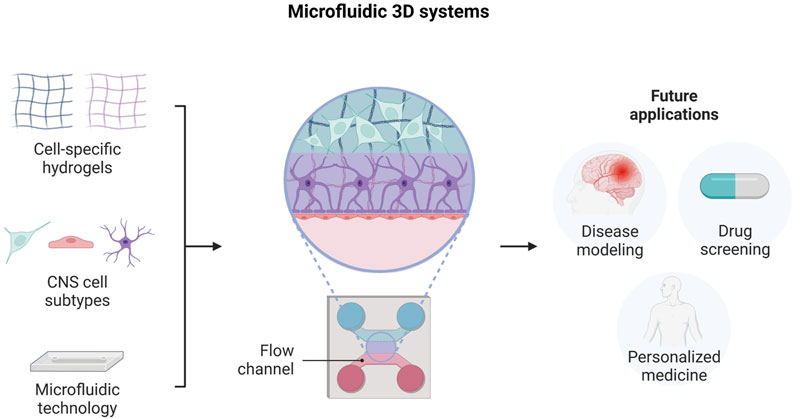
FIGURE 5. Engineering neural tissue by using microfluidic-based 3D platforms. Illustration of the different system components, design and potential future applications. Created with BioRender.com.
Hence, many studies have focused their research on the development of microfluidic-based 3D platforms, aimed at modeling neural circuit formation or neurovascular unit structure, along with investigating the mechanisms involved in neurodegenerative diseases or neuronal injury (Shrirao et al., 2018; Teixeira et al., 2020; Caffrey et al., 2021).
5.2.1 Microfluidic technology to model cerebral cortex
The possibility of designing a compartmentalized structure has been exploited to replicate the layered organization of the cerebral cortex. Kunze and colleagues engineered a multi-layered construct, constituted of alternating cell-loaded and acellular hydrogel layers, by the use of microfluidic technology. The chip design and the seeding protocol were optimized to respect the same scale dimension of neural microtissue. Primary cortical neurons, embedded in a mixture of agarose and alginate, were cultured under different conditions. Hence, they demonstrated the influence of nutrient concentrations on the length and density of neurites, modifying the initial amount of B27 supplement in the hydrogel solutions (Kunze et al., 2011). In the work of Odawara et al., a 3D neuronal network was recreated using polydimethylsiloxane (PDMS) microchambers and collagen hydrogels, intended to mimic the cortical environment. PDMS patterning allows for the creation of separated cell blocks, in which rat hippocampal neurons were cultured in collagen type I matrix gels. To modulate the orientation of collagen fibrils, the system was tilted at 45° during hydrogel gelation. In this way, the position of the somata was fixed inside the structure and directional control of neurite elongation was achieved, according to collagen fibril orientation. The functionality of neural networks was also assessed by calcium imaging and the detection of action potential propagation and synaptic transmission. Furthermore, the establishment of the neural network was also demonstrated using human iPSC-derived neurons (Odawara et al., 2013).
5.2.2 Microfluidic technology to model 3D neural networks
As mentioned above, the formation of synaptic connections strictly depends on topographical and biochemical cues, which influence axon growth and extension and hence the establishment of synaptic contacts. In this regard, microfluidic technology can be employed to create engineered platforms with tailored structures and a highly controlled environment by creating specifically-interconnected micrometric compartments. For example, by appropriately modulating the chip design, multiple culture chambers with defined interconnections can be integrated, thus allowing for direct control of neuronal cell body positions and axon directions. Van de Wijdeven et al. developed a microfluidic chip composed of four or six cellular interconnected compartments to support the formation of complex neural networks with multiple nodes. Specifically, they established a 3D cell culture system by seeding motor neuron aggregates derived from ChiPS18 human iPSC line. Based on the results, this platform enables the formation of neural networks, with a high level of structural complexity and connections. Indeed, axonal growth and extension towards the opposite compartments were observed, suggesting that the design of the chip facilitates neuron connectivity and provides directional control for axonal guidance (van de Wijdeven et al., 2018). Similarly, Bang et al. fabricated a microfluidic culture system for the in vitro modeling of neural network maturation. The device, constituted by multiple channels filled with Matrigel, was optimized to enable the seeding of cortical neurons on the sidewall of the hydrogel and to promote the directional growth of neurites inside the chamber. Then, the establishment of effective synapsis was investigated by coculturing the neurons on the sidewall with neurons loaded into Matrigel, as presynaptic and postsynaptic neurons respectively (Bang et al., 2016).
The importance of anisotropy in vitro tissue modeling has been highlighted by Kim et al., who developed a microfluidic-based 3D culture platform supporting the reconstruction of a hippocampal cell network. Their system comprises three inlets merging in a singular channel, filled with adjacent streams of collagen hydrogel. Matrix alignment was obtained by stretching and releasing the chip during hydrogel crosslinking, resulting in the orientation of collagen fibrils perpendicularly to the strain direction. Primary hippocampal neurons, isolated from CA3 and CA1 regions of rat embryonic hippocampi, were embedded in the two external collagen solutions, while the central collagen stream was intended as axonal compartment. Their results demonstrated that this method significantly enhances directional axon extension and supports the establishment of synaptic connections between the two cell populations (Kim et al., 2017). Lastly, Lee et al. developed an innovative culture system (Neuro-IMPACT), including a microfluidic approach in a 96-well plate design, which could facilitate high-throughput analysis in simple and flexible conditions. To validate their platform, they successfully reconstructed a functional neural network, by seeding primary cortical neurons on both sides of a collagen hydrogel-filled channel. The presence of mature synapses was detected by immunocytochemistry analysis after 21 days in vitro, which revealed the expression of synaptophysin in proximity to the neuronal marker. Additionally, the functional properties of the network were assessed by calcium imaging. In particular, spontaneous and synchronous signals were observed after the injection of BAPTA-AM solution, indicating the generation of action potentials by neurons (Lee et al., 2019).
5.2.3 Microfluidic technology to model the neurovascular unit
The major innovation in microfluidic technology is the possibility of simulating in vivo vascular perfusion, a feature hardly to be replicated by other 3D in vitro models. The interaction between neuronal cells, glia, and vascular cells in CNS, commonly known as neurovascular unit (NVU), is of great importance for tissue homeostasis and its dysfunction is often strictly correlated to many pathological conditions (Caffrey et al., 2021). Therefore, the research interest in developing microfluidic-based NVU in vitro models has increased significantly in the last years, as these technologies would offer new opportunities for a deeper understanding of the neurovascular interplay and the testing of novel therapeutic strategies.
Adriani et al. proposed a microfluidic system consisting of four communicating channels, intended for the co-culture of neurons, astrocytes, and endothelial cells, in order to replicate the interface between the brain and the vascular tissue. The two central channels, including neural cell populations, were filled with a collagen-based hydrogel, whose concentration was modulated according to cell response, while the endothelial cells were located in the adjacent channel, to form a monolayer. The device successfully supports the growth and maturation of all the cell types, as demonstrated by the distinctive cell morphologies and by the expression of cell-specific markers. The functionality of neural and endothelial cells was also assessed by calcium imaging and permeability test respectively, thus confirming the reliability of this model for further therapeutic studies (Adriani et al., 2017).
Similarly, in the aforementioned work of Lee and coll., Neuro-IMPACT platform has been also employed to model the BBB. Human brain microvascular endothelial cells and fibroblast cells, embedded in a fibrin hydrogel, were plated into two different channels. Astrocytes were then seeded on the side of endothelial cells. Thus, the construction of the engineered BBB was assessed by immunostaining and permeability analysis, which confirmed the presence of tight junctions and effective membrane functions (Lee et al., 2019).
5.2.4 Microfluidic technology to model neurodegenerative diseases
As previously discussed, microfluidic platforms have found extensive applications in the development of physiologically relevant neural environments. However, these models could also support the investigation of many neurodegenerative disorders thanks to the inclusion of genetically manipulated cells or cells derived from patients. A pioneering example in this field is the work of the Park’ group, who developed a microfluidic-based 3D culture system to model some of the key features of AD. They conducted a deeper analysis of the interactions between AD neurons, astrocytes and human adult microglia cells to investigate the mechanisms of neuroinflammation proper of AD pathology. AD neural cells were obtained from the differentiation of transduced NPCs or iPSCs, genetically modified to express high levels of pathogenic Aβ species. To respect 3D in vivo conditions, neural cells were embedded in Matrigel before being entered into the microfluidic device. According to the reported results, the model is able to relevantly recapitulate many biological events involved in AD pathogenesis, including microglia activation and recruitment, the secretion of pro-inflammatory factors, and the influence of these processes on neuronal cell damage (Park et al., 2018).
6 Conclusion and future perspective
The innovation induced by in vitro three-dimensional models has the potential to revolutionize the neuroscience field, developing both healthy and pathological models, capable of reproducing in vivo conditions with higher accuracy and reliability than traditional 2D models. On one hand, these models have the aim of drastically reducing the number of animals employed in scientific research. On the other hand, the research use of cells directly obtained from patients can highly increase therapies’ effectiveness. Indeed, personalized therapy is a milestone for modern medicine and involves also the development of 3D models centered on the unique patient genetic profile.
In this review, several important aspects of in vitro CNS modeling have been highlighted. Despite numerous advancements, designing physiologically relevant constructs remain challenging for several reasons. First of all, any cell source available, from iPSCs to cell lines, has different advantages and disadvantages, as previously reported (Table 1). Secondly, each neural cell type has specific characteristics and needs that cannot be completely satisfied by a single biomaterial. Lastly, the structural complexity of the neural microenvironment makes the precise tuning of biomaterial properties necessary.
However, recent innovations in 3D bioprinting and in microfluidic-based platforms offer the chance to develop advanced systems, useful for the in vitro modeling of complex CNS constructs. Special emphasis has been placed on recapitulating the state-of-the-art of these techniques, with the aim to help capture real-time dynamic innovation in the neural tissue engineering field.
Author contributions
CT: Conceptualization, Visualization, Writing–original draft, Writing–review and editing. VC: Conceptualization, Visualization, Writing–original draft, Writing–review and editing. GM: Data curation, Writing–original draft. CT-T: Conceptualization, Funding acquisition, Supervision, Writing–review and editing. MB: Conceptualization, Funding acquisition, Supervision, Writing–review and editing.
Funding
The author(s) declare financial support was received for the research, authorship, and/or publication of this article. This work was supported by Fondazione CRT “Richieste Ordinarie 2020” grant (no. 73239) to MB and CT-T.
Acknowledgments
Authors are grateful to Fondazione CRT “Richieste Ordinarie 2020” grant (no. 73239) for funding VC fellowship.
Conflict of interest
The authors declare that the research was conducted in the absence of any commercial or financial relationships that could be construed as a potential conflict of interest.
The authors (MB) and (CT-T) declared that they were editorial board members of Frontiers at the time of submission. This had no impact on the peer review process and the final decision.
Publisher’s note
All claims expressed in this article are solely those of the authors and do not necessarily represent those of their affiliated organizations, or those of the publisher, the editors and the reviewers. Any product that may be evaluated in this article, or claim that may be made by its manufacturer, is not guaranteed or endorsed by the publisher.
References
Abelseth, E., Abelseth, L., De La Vega, L., Beyer, S. T., Wadsworth, S. J., and Willerth, S. M. (2019). 3D printing of neural tissues derived from human induced pluripotent stem cells using a fibrin-based bioink. ACS Biomater. Sci. Eng. 5, 234–243. doi:10.1021/acsbiomaterials.8b01235
Accardo, A., Cirillo, C., Lionnet, S., Vieu, C., and Loubinoux, I. (2019). Interfacing cells with microengineered scaffolds for neural tissue reconstruction. Brain Res. Bull. 152, 202–211. doi:10.1016/j.brainresbull.2019.07.020
Adil, M. M., Rodrigues, G. M. C., Kulkarni, R. U., Rao, A. T., Chernavsky, N. E., Miller, E. W., et al. (2017). Efficient generation of hPSC-derived midbrain dopaminergic neurons in a fully defined, scalable, 3D biomaterial platform. Sci. Rep. 7, 40573. doi:10.1038/srep40573
Adlakha, Y. K. (2023). Human 3D brain organoids: steering the demolecularization of brain and neurological diseases. Cell Death Discov. 9, 221. doi:10.1038/s41420-023-01523-w
Adriani, G., Ma, D., Pavesi, A., Kamm, R. D., and Goh, E. L. K. (2017). A 3D neurovascular microfluidic model consisting of neurons, astrocytes and cerebral endothelial cells as a blood-brain barrier. Lab. Chip 17, 448–459. doi:10.1039/c6lc00638h
Amadei, G., Handford, C. E., Qiu, C., De Jonghe, J., Greenfeld, H., Tran, M., et al. (2022). Embryo model completes gastrulation to neurulation and organogenesis. Nature 610, 143–153. doi:10.1038/s41586-022-05246-3
Ardhanareeswaran, K., Mariani, J., Coppola, G., Abyzov, A., and Vaccarino, F. M. (2017). Human induced pluripotent stem cells for modelling neurodevelopmental disorders. Nat. Rev. Neurol. 13, 265–278. doi:10.1038/nrneurol.2017.45
Askari, M., Afzali Naniz, M., Kouhi, M., Saberi, A., Zolfagharian, A., and Bodaghi, M. (2021). Recent progress in extrusion 3D bioprinting of hydrogel biomaterials for tissue regeneration: A comprehensive review with focus on advanced fabrication techniques. Biomater. Sci. 9, 535–573. doi:10.1039/d0bm00973c
Aubry, L., Bugi, A., Lefort, N., Rousseau, F., Peschanski, M., and Perrier, A. L. (2008). Striatal progenitors derived from human ES cells mature into DARPP32 neurons in vitro and in quinolinic acid-lesioned rats. Proc. Natl. Acad. Sci. 105, 16707–16712. doi:10.1073/pnas.0808488105
Axpe, E., Orive, G., Franze, K., and Appel, E. A. (2020). Towards brain-tissue-like biomaterials. Nat. Commun. 11, 3423–3513. doi:10.1038/s41467-020-17245-x
Azari, H., and Reynolds, B. A. (2016). In vitro models for neurogenesis. Cold Spring Harb. Perspect. Biol. 8, 0212799–a21312. doi:10.1101/cshperspect.a021279
Bang, S., Na, S., Jang, J. M., Kim, J., and Jeon, N. L. (2016). Engineering-aligned 3D neural circuit in microfluidic device. Adv. Healthc. Mater. 5, 159–166. doi:10.1002/adhm.201500397
Benarroch, E. E. (2015). Extracellular matrix in the CNS. Neurology 85, 1417–1427. doi:10.1212/WNL.0000000000002044
Bhatia, S. N., and Ingber, D. E. (2014). Microfluidic organs-on-chips. Nat. Biotechnol. 32, 760–772. doi:10.1038/nbt.2989
Boni, R., Ali, A., Shavandi, A., and Clarkson, A. N. (2018). Current and novel polymeric biomaterials for neural tissue engineering. J. Biomed. Sci. 25, 90–21. doi:10.1186/s12929-018-0491-8
Brewer, G. J., and Torricelli, J. R. (2007). Isolation and culture of adult neurons and neurospheres. Nat. Protoc. 2, 1490–1498. doi:10.1038/nprot.2007.207
Cadena, M., Ning, L., King, A., Hwang, B., Jin, L., Serpooshan, V., et al. (2021). 3D bioprinting of neural tissues. Adv. Healthc. Mater. 10, e2001600. doi:10.1002/adhm.202001600
Carletti, E., Motta, A., and Migliaresi, C. (2011). “Scaffolds for tissue engineering and 3D cell culture BT - 3D cell culture: Methods and protocols,” in, ed. J. W. Haycock (Totowa, NJ: Humana Press), 17–39. doi:10.1007/978-1-60761-984-0_2
Choi, S. H., Kim, Y. H., Hebisch, M., Sliwinski, C., Lee, S., D’Avanzo, C., et al. (2014). A three-dimensional human neural cell culture model of Alzheimer’s disease. Nature 515, 274–278. doi:10.1038/nature13800
Czechanski, A., Byers, C., Greenstein, I., Schrode, N., Donahue, L. R., Hadjantonakis, A.-K., et al. (2014). Derivation and characterization of mouse embryonic stem cells from permissive and nonpermissive strains. Nat. Protoc. 9, 559–574. doi:10.1038/nprot.2014.030
de la Vega, L., Gómez, D. A. R., Abelseth, E., Abelseth, L., da Silva, V. A., and Willerth, S. M. (2018). 3D bioprinting human induced pluripotent stem cell-derived neural tissues using a novel lab-on-a-printer technology. Appl. Sci. 8, 1–13. doi:10.3390/app8122414
de Morree, A., and Rando, T. A. (2023). Regulation of adult stem cell quiescence and its functions in the maintenance of tissue integrity. Nat. Rev. Mol. Cell Biol. 24, 334–354. doi:10.1038/s41580-022-00568-6
De Vries, G. H., and Boullerne, A. I. (2010). Glial cell lines: an overview. Neurochem. Res. 35, 1978–2000. doi:10.1007/s11064-010-0318-9
Dutan Polit, L., Eidhof, I., McNeill, R. V., Warre-Cornish, K. M., Yde Ohki, C. M., Walter, N. M., et al. (2023). Recommendations, guidelines, and best practice for the use of human induced pluripotent stem cells for neuropharmacological studies of neuropsychiatric disorders. Neurosci. Appl. 2, 101125. doi:10.1016/j.nsa.2023.101125
Ebert, A. D., Yu, J., Rose, F. F. J., Mattis, V. B., Lorson, C. L., Thomson, J. A., et al. (2009). Induced pluripotent stem cells from a spinal muscular atrophy patient. Nature 457, 277–280. doi:10.1038/nature07677
Egawa, N., Shindo, A., Liang, A. C., Du, Y., Xing, C., Lo, E. K., et al. (2017). A novel three-dimensional culture system for oligodendrocyte precursor cells. Stem Cells Dev. 26, 1078–1085. doi:10.1089/scd.2016.0306
Evans, M. J., and Kaufman, M. H. (1981). Establishment in culture of pluripotential cells from mouse embryos. Nature 292, 154–156. doi:10.1038/292154a0
Fan, L., Liu, C., Chen, X., Zou, Y., Zhou, Z., Lin, C., et al. (2018). Directing induced pluripotent stem cell derived neural stem cell fate with a three-dimensional biomimetic hydrogel for spinal cord injury repair. ACS Appl. Mat. Interfaces 10, 17742–17755. doi:10.1021/acsami.8b05293
Farrukh, A., Zhao, S., and del Campo, A. (2018). Microenvironments designed to support growth and function of neuronal cells. Front. Mat. 5, 1–22. doi:10.3389/fmats.2018.00062
Firouzian, K. F., Song, Y., Lin, F., and Zhang, T. (2020). Fabrication of a biomimetic spinal cord tissue construct with heterogenous mechanical properties using intrascaffold cell assembly. Biotechnol. Bioeng. 117, 3094–3107. doi:10.1002/bit.27459
Führmann, T., Tam, R. Y., Ballarin, B., Coles, B., Elliott Donaghue, I., van der Kooy, D., et al. (2016). Injectable hydrogel promotes early survival of induced pluripotent stem cell-derived oligodendrocytes and attenuates longterm teratoma formation in a spinal cord injury model. Biomaterials 83, 23–36. doi:10.1016/j.biomaterials.2015.12.032
Galarza, S., Crosby, A. J., Pak, C. H., and Peyton, S. R. (2020). Control of astrocyte quiescence and activation in a synthetic brain hydrogel. Adv. Healthc. Mater. 9, 19014199–e1901512. doi:10.1002/adhm.201901419
Gao, X., Cheng, W., Zhang, X., Zhou, Z., Ding, Z., Zhou, X., et al. (2022). Nerve growth factor-laden anisotropic silk nanofiber hydrogels to regulate neuronal/astroglial differentiation for scarless spinal cord repair. ACS Appl. Mat. Interfaces 14, 3701–3715. doi:10.1021/acsami.1c19229
Geissler, S. A., Sabin, A. L., Besser, R. R., Gooden, O. M., Shirk, B. D., Nguyen, Q. M., et al. (2018). Biomimetic hydrogels direct spinal progenitor cell differentiation and promote functional recovery after spinal cord injury. J. Neural Eng. 15, 025004. doi:10.1088/1741-2552/aaa55c
Gilmozzi, V., Gentile, G., Riekschnitz, D. A., Von Troyer, M., Lavdas, A. A., Kerschbamer, E., et al. (2021). Generation of hiPSC-derived functional dopaminergic neurons in alginate-based 3D culture. Front. Cell Dev. Biol. 9, 708389–708416. doi:10.3389/fcell.2021.708389
Goncalves, K., and Przyborski, S. (2018). The utility of stem cells for neural regeneration. Brain Neurosci. Adv. 2, 239821281881807. doi:10.1177/2398212818818071
Gordon, J., Amini, S., and White, M. K. (2013). General overview of neuronal cell culture. Methods Mol. Biol. Clift. N.J.) 1078, 1–8. doi:10.1007/978-1-62703-640-5_1
Gottipati, M. K., Samuelson, J. J., Kalinina, I., Bekyarova, E., Haddon, R. C., and Parpura, V. (2013). Chemically functionalized single-walled carbon nanotube films modulate the morpho-functional and proliferative characteristics of astrocytes. Nano Lett. 13, 4387–4392. doi:10.1021/nl402226z
Gottipati, M. K., Zuidema, J. M., and Gilbert, R. J. (2020). Biomaterial strategies for creating in vitro astrocyte cultures resembling in vivo astrocyte morphologies and phenotypes. Curr. Opin. Biomed. Eng. 14, 67–74. doi:10.1016/j.cobme.2020.06.004
Gradišnik, L., Bošnjak, R., Maver, T., and Velnar, T. (2021). Advanced bio-based polymers for astrocyte cell models. Mater. (Basel) 14, 3664. doi:10.3390/ma14133664
Grenier, K., Kao, J., and Diamandis, P. (2020). Three-dimensional modeling of human neurodegeneration: brain organoids coming of age. Mol. Psychiatry 25, 254–274. doi:10.1038/s41380-019-0500-7
Gu, Q., Tomaskovic-Crook, E., Lozano, R., Chen, Y., Kapsa, R. M., Zhou, Q., et al. (2016). Functional 3D neural mini-tissues from printed gel-based bioink and human neural stem cells. Adv. Healthc. Mater. 5, 1429–1438. doi:10.1002/adhm.201600095
Gu, Q., Tomaskovic-Crook, E., Wallace, G. G., and Crook, J. M. (2017). 3D bioprinting human induced pluripotent stem cell constructs for in situ cell proliferation and successive multilineage differentiation. Adv. Healthc. Mater. 6, 1700175–1700211. doi:10.1002/adhm.201700175
Guan, S., Zhang, X.-L., Lin, X.-M., Liu, T.-Q., Ma, X.-H., and Cui, Z.-F. (2013). Chitosan/gelatin porous scaffolds containing hyaluronic acid and heparan sulfate for neural tissue engineering. J. Biomaterials Sci. Polym. Ed. 24, 999–1014. doi:10.1080/09205063.2012.731374
Gyawali, A., Gautam, S., Hyeon, S. J., Ryu, H., and Kang, Y.-S. (2021). L-citrulline level and transporter activity are altered in experimental models of amyotrophic lateral sclerosis. Mol. Neurobiol. 58, 647–657. doi:10.1007/s12035-020-02143-6
Hamid, O. A., Eltaher, H. M., Sottile, V., and Yang, J. (2021). 3D bioprinting of a stem cell-laden, multi-material tubular composite: an approach for spinal cord repair. Mater. Sci. Eng. C 120, 111707. doi:10.1016/j.msec.2020.111707
Haring, A. P., Thompson, E. G., Tong, Y., Laheri, S., Cesewski, E., Sontheimer, H., et al. (2019). Process- and bio-inspired hydrogels for 3D bioprinting of soft free-standing neural and glial tissues. Biofabrication 11, 025009. doi:10.1088/1758-5090/ab02c9
Harischandra, D. S., Rokad, D., Ghaisas, S., Verma, S., Robertson, A., Jin, H., et al. (2020). Enhanced differentiation of human dopaminergic neuronal cell model for preclinical translational research in Parkinson’s disease. Biochimica Biophysica Acta (BBA) - Mol. Basis Dis. 1866, 165533. doi:10.1016/j.bbadis.2019.165533
Hester, M. E., Murtha, M. J., Song, S., Rao, M., Miranda, C. J., Meyer, K., et al. (2011). Rapid and efficient generation of functional motor neurons from human pluripotent stem cells using gene delivered transcription factor codes. Mol. Ther. 19, 1905–1912. doi:10.1038/mt.2011.135
Ho, L., and Hsu, S. H (2018). Cell reprogramming by 3D bioprinting of human fibroblasts in polyurethane hydrogel for fabrication of neural-like constructs. Acta Biomater. 70, 57–70. doi:10.1016/j.actbio.2018.01.044
Homem, C. C. F., Repic, M., and Knoblich, J. A. (2015). Proliferation control in neural stem and progenitor cells. Nat. Rev. Neurosci. 16, 647–659. doi:10.1038/nrn4021
Hong, Y., Yang, Q., Song, H., and Ming, G. (2023). Opportunities and limitations for studying neuropsychiatric disorders using patient-derived induced pluripotent stem cells. Mol. Psychiatry 28, 1430–1439. doi:10.1038/s41380-023-01990-8
Hopkins, A. M., DeSimone, E., Chwalek, K., and Kaplan, D. L. (2015). 3D in vitro modeling of the central nervous system. Prog. Neurobiol. 125, 1–25. doi:10.1016/j.pneurobio.2014.11.003
Hounoum, B. M., Vourc’h, P., Felix, R., Corcia, P., Patin, F., Guéguinou, M., et al. (2016). NSC-34 motor neuron-like cells are unsuitable as experimental model for glutamate-mediated excitotoxicity. Front. Cell. Neurosci. 10, 1–12. doi:10.3389/fncel.2016.00118
Hsieh, F. Y., Lin, H. H., and Hsu, S. hui (2015). 3D bioprinting of neural stem cell-laden thermoresponsive biodegradable polyurethane hydrogel and potential in central nervous system repair. Biomaterials 71, 48–57. doi:10.1016/j.biomaterials.2015.08.028
Huang, C. T., Kumar Shrestha, L., Ariga, K., and Hsu, S. H. (2017). A graphene-polyurethane composite hydrogel as a potential bioink for 3D bioprinting and differentiation of neural stem cells. J. Mat. Chem. B 5, 8854–8864. doi:10.1039/c7tb01594a
Jagielska, A., Norman, A. L., Whyte, G., Vliet, K. J. V., Guck, J., and Franklin, R. J. M. (2012). Mechanical environment modulates biological properties of oligodendrocyte progenitor cells. Stem Cells Dev. 21, 2905–2914. doi:10.1089/scd.2012.0189
Jang, J. M., Tran, S. H. T., Na, S. C., and Jeon, N. L. (2015). Engineering controllable architecture in Matrigel for 3D cell alignment. ACS Appl. Mat. Interfaces 7, 2183–2188. doi:10.1021/am508292t
Jasnic-Savovic, J., Klajn, A., Milivojevic, M., Mojsin, M., and Nikcevic, G. (2015). Human embryonal carcinoma cells in serum-free conditions as an in vitro model system of neural differentiation. Altern. Laboratory Animals 43, 9–18. doi:10.1177/026119291504300105
Jia, W., Gungor-Ozkerim, P. S., Zhang, Y. S., Yue, K., Zhu, K., Liu, W., et al. (2016). Direct 3D bioprinting of perfusable vascular constructs using a blend bioink. Biomaterials 106, 58–68. doi:10.1016/j.biomaterials.2016.07.038
Johnson, C. D. L., Zuidema, J. M., Kearns, K. R., Maguire, A. B., Desmond, G. P., Thompson, D. M., et al. (2019). The effect of electrospun fiber diameter on astrocyte-mediated neurite guidance and protection. ACS Appl. Bio Mat. 2, 104–117. doi:10.1021/acsabm.8b00432
Joung, D., Truong, V., Neitzke, C. C., Guo, S. Z., Walsh, P. J., Monat, J. R., et al. (2018). 3D printed stem-cell derived neural progenitors generate spinal cord scaffolds. Adv. Funct. Mater. 28, 1801850–1801910. doi:10.1002/adfm.201801850
Kelava, I., and Lancaster, M. A. (2016). Stem cell models of human brain development. Cell Stem Cell 18, 736–748. doi:10.1016/j.stem.2016.05.022
Kim, S. H., Im, S. K., Oh, S. J., Jeong, S., Yoon, E. S., Lee, C. J., et al. (2017). Anisotropically organized three-dimensional culture platform for reconstruction of a hippocampal neural network. Nat. Commun. 8, 14346. doi:10.1038/ncomms14346
Kjar, A., McFarland, B., Mecham, K., Harward, N., and Huang, Y. (2021). Engineering of tissue constructs using coaxial bioprinting. Bioact. Mater. 6, 460–471. doi:10.1016/j.bioactmat.2020.08.020
Kunze, A., Giugliano, M., Valero, A., and Renaud, P. (2011). Micropatterning neural cell cultures in 3D with a multi-layered scaffold. Biomaterials 32, 2088–2098. –2098. doi:10.1016/j.biomaterials.2010.11.047
Lancaster, M. A., Renner, M., Martin, C. A., Wenzel, D., Bicknell, L. S., Hurles, M. E., et al. (2013). Cerebral organoids model human brain development and microcephaly. Nature 501, 373–379. doi:10.1038/nature12517
Lau, C. L., Kovacevic, M., Tingleff, T. S., Forsythe, J. S., Cate, H. S., Merlo, D., et al. (2014). 3D Electrospun scaffolds promote a cytotrophic phenotype of cultured primary astrocytes. J. Neurochem. 130, 215–226. doi:10.1111/jnc.12702
Lee, S. R., Hyung, S., Bang, S., Lee, Y., Ko, J., Lee, S., et al. (2019). Modeling neural circuit, blood-brain barrier, and myelination on a microfluidic 96 well plate. Biofabrication 11, 035013. doi:10.1088/1758-5090/ab1402
Lee, W., Pinckney, J., Lee, V., Lee, J. H., Fischer, K., Polio, S., et al. (2009). Three-dimensional bioprinting of rat embryonic neural cells. Neuroreport 20, 798–803. doi:10.1097/WNR.0b013e32832b8be4
Leventoux, N., Morimoto, S., Imaizumi, K., Sato, Y., Takahashi, S., Mashima, K., et al. (2020). Human astrocytes model derived from induced pluripotent stem cells. Cells 9, 2680. doi:10.3390/cells9122680
Lezmi, E., and Benvenisty, N. (2022). The tumorigenic potential of human pluripotent stem cells. Stem Cells Transl. Med. 11, 791–796. doi:10.1093/stcltm/szac039
Li, C., Kuss, M., Kong, Y., Nie, F., Liu, X., Liu, B., et al. (2021a). 3D printed hydrogels with aligned microchannels to guide neural stem cell migration. ACS Biomater. Sci. Eng. 7, 690–700. doi:10.1021/acsbiomaterials.0c01619
Li, X., Wang, X., Chen, H., Jin, Z., Dai, X., Zhang, X., et al. (2019). A comparative study of the behavior of neural progenitor cells in extrusion-based in vitro hydrogel models. Biomed. Mat. 14, 065001. doi:10.1088/1748-605X/ab3b4b
Li, Y., Cao, X., Deng, W., Yu, Q., Sun, C., Ma, P., et al. (2021b). 3D printable Sodium alginate-Matrigel (SA-MA) hydrogel facilitated ectomesenchymal stem cells (EMSCs) neuron differentiation. J. Biomater. Appl. 35, 709–719. doi:10.1177/0885328220961261
Liu, W., Heinrich, M. A., Zhou, Y., Akpek, A., Hu, N., Liu, X., et al. (2017). Extrusion bioprinting of shear-thinning gelatin methacryloyl bioinks. Adv. Healthc. Mater. 6, 1–21. doi:10.1002/adhm.201601451
Liu, W., Luo, Y., Ning, C., Zhang, W., Zhang, Q., Zou, H., et al. (2021a). Thermo-sensitive electroactive hydrogel combined with electrical stimulation for repair of spinal cord injury. J. Nanobiotechnology 19, 286–316. doi:10.1186/s12951-021-01031-y
Liu, X., Hao, M., Chen, Z., Zhang, T., Huang, J., Dai, J., et al. (2021b). 3D bioprinted neural tissue constructs for spinal cord injury repair. Biomaterials 272, 120771. doi:10.1016/j.biomaterials.2021.120771
Lozano, R., Stevens, L., Thompson, B. C., Gilmore, K. J., Gorkin, R., Stewart, E. M., et al. (2015). 3D printing of layered brain-like structures using peptide modified gellan gum substrates. Biomaterials 67, 264–273. doi:10.1016/j.biomaterials.2015.07.022
Luo, Y., Fan, L., Liu, C., Wen, H., Wang, S., Guan, P., et al. (2022). An injectable, self-healing, electroconductive extracellular matrix-based hydrogel for enhancing tissue repair after traumatic spinal cord injury. Bioact. Mater. 7, 98–111. doi:10.1016/j.bioactmat.2021.05.039
Madhusudanan, P., Raju, G., and Shankarappa, S. (2020). Hydrogel systems and their role in neural tissue engineering. J. R. Soc. Interface 17, 20190505. doi:10.1098/rsif.2019.0505
Martin, G. R. (1981). Isolation of a pluripotent cell line from early mouse embryos cultured in medium conditioned by teratocarcinoma stem cells. Proc. Natl. Acad. Sci. U. S. A. 78, 7634–7638. doi:10.1073/pnas.78.12.7634
Martin-Lemaitre, C., Alcheikh, Y., Naumann, R., and Honigmann, A. (2020). Optimization of mouse embryonic stem cell culture for organoid and chimeric mice production. bioRxiv. doi:10.1101/2020.03.13.990135
Martínez-Cerdeño, V., and Noctor, S. C. (2018). Neural progenitor cell terminology. Front. Neuroanat. 12, 104. doi:10.3389/fnana.2018.00104
McNamara, M. C., Aykar, S. S., Alimoradi, N., Niaraki Asli, A. E., Pemathilaka, R. L., Wrede, A. H., et al. (2021). Behavior of neural cells post manufacturing and after prolonged encapsulation within conductive graphene-laden alginate microfibers. Adv. Biol. 5, e2101026. doi:10.1002/adbi.202101026
Medelin, M., Porrelli, D., Aurand, E. R., Scaini, D., Travan, A., Borgogna, M. A., et al. (2018). Exploiting natural polysaccharides to enhance in vitro bio-constructs of primary neurons and progenitor cells. Acta Biomater. 73, 285–301. doi:10.1016/j.actbio.2018.03.041
Melchels, F. P. W., Domingos, M. A. N., Klein, T. J., Malda, J., Bartolo, P. J., and Hutmacher, D. W. (2012). Additive manufacturing of tissues and organs. Prog. Polym. Sci. 37, 1079–1104. doi:10.1016/j.progpolymsci.2011.11.007
Moschetta, M., Chiacchiaretta, M., Cesca, F., Roy, I., Athanassiou, A., Benfenati, F., et al. (2021). Graphene nanoplatelets render poly(3-hydroxybutyrate) a suitable scaffold to promote neuronal network development. Front. Neurosci. 15, 731198–731212. doi:10.3389/fnins.2021.731198
Murphy, A. R., Laslett, A., O’Brien, C. M., and Cameron, N. R. (2017). Scaffolds for 3D in vitro culture of neural lineage cells. Acta Biomater. 54, 1–20. doi:10.1016/j.actbio.2017.02.046
Ngo, T. B., Spearman, B. S., Hlavac, N., and Schmidt, C. E. (2020). Three-dimensional bioprinted hyaluronic acid hydrogel test beds for assessing neural cell responses to competitive growth stimuli. ACS Biomater. Sci. Eng. 6, 6819–6830. doi:10.1021/acsbiomaterials.0c00940
Odawara, A., Gotoh, M., and Suzuki, I. (2013). A three-dimensional neuronal culture technique that controls the direction of neurite elongation and the position of soma to mimic the layered structure of the brain. RSC Adv. 3, 23620–23630. doi:10.1039/c3ra44757j
Onoe, H., Kato-Negishi, M., Itou, A., and Takeuchi, S. (2016). Differentiation induction of mouse neural stem cells in hydrogel tubular microenvironments with controlled tube dimensions. Adv. Healthc. Mater. 5, 1104–1111. doi:10.1002/adhm.201500903
Outeiro, T. F., Kontopoulos, E., Altmann, S. M., Kufareva, I., Strathearn, K. E., Amore, A. M., et al. (2007). Sirtuin 2 inhibitors rescue α-synuclein-mediated toxicity in models of Parkinson's disease. Science 317, 516–519. doi:10.1126/science.1143780
Papadimitriou, L., Manganas, P., Ranella, A., and Stratakis, E. (2020). Biofabrication for neural tissue engineering applications. Mater. Today Bio 6, 100043. doi:10.1016/j.mtbio.2020.100043
Park, J., Wetzel, I., Marriott, I., Dréau, D., D’Avanzo, C., Kim, D. Y., et al. (2018). A 3D human triculture system modeling neurodegeneration and neuroinflammation in Alzheimer’s disease. Nat. Neurosci. 21, 941–951. doi:10.1038/s41593-018-0175-4
Placone, A. L., McGuiggan, P. M., Bergles, D. E., Guerrero-Cazares, H., Quiñones-Hinojosa, A., and Searson, P. C. (2015). Human astrocytes develop physiological morphology and remain quiescent in a novel 3D matrix. Biomaterials 42, 134–143. doi:10.1016/j.biomaterials.2014.11.046
Qiu, B., Bessler, N., Figler, K., Buchholz, M. B., Rios, A. C., Malda, J., et al. (2020). Bioprinting neural systems to model central nervous system diseases. Adv. Funct. Mater. 30, 1910250. doi:10.1002/adfm.201910250
Robert, J., Caffrey, T., and Button, E. (2021). Toward three-dimensional in vitro models to study neurovascular unit functions in health and disease. Neural Regen. Res. 16, 2132–2140. doi:10.4103/1673-5374.310671
Rodrigues, C. A. V., Fernandes, T. G., Diogo, M. M., da Silva, C. L., and Cabral, J. M. S. (2011). Stem cell cultivation in bioreactors. Biotechnol. Adv. 29, 815–829. doi:10.1016/j.biotechadv.2011.06.009
Rodrigues, G. M. C., Gaj, T., Adil, M. M., Wahba, J., Rao, A. T., Lorbeer, F. K., et al. (2017). Defined and scalable differentiation of human oligodendrocyte precursors from pluripotent stem cells in a 3D culture system. Stem Cell Rep. 8, 1770–1783. doi:10.1016/j.stemcr.2017.04.027
Russell, L. N., and Lampe, K. J. (2016). Engineering biomaterials to influence oligodendroglial growth, maturation, and myelin production. Cells Tissues Organs 202, 85–101. doi:10.1159/000446645
Salaris, C., Brighi, S., Turris, B., Soloperto, , Turris, , Benedetti, , et al. (2019). 3D bioprinted human cortical neural constructs derived from induced pluripotent stem cells. J. Clin. Med. 8, 1595. doi:10.3390/jcm8101595
Sanz, B., Albillos Sanchez, A., Tangey, B., Gilmore, K., Yue, Z., Liu, X., et al. (2020). Light cross-linkable marine collagen for coaxial printing of a 3D model of neuromuscular junction formation. Biomedicines 9, 16–19. doi:10.3390/biomedicines9010016
Saxena, S., Choudhury, S., and Mohan, K. N. (2020). Reproducible differentiation and characterization of neurons from mouse embryonic stem cells. MethodsX 7, 101073. doi:10.1016/j.mex.2020.101073
Scarian, E., Bordoni, M., Fantini, V., Jacchetti, E., Raimondi, M. T., Diamanti, L., et al. (2022). Patients’ stem cells differentiation in a 3D environment as a promising experimental tool for the study of amyotrophic lateral sclerosis. Int. J. Mol. Sci. 23, 5344. doi:10.3390/ijms23105344
Schildge, S., Bohrer, C., Beck, K., and Schachtrup, C. (2013). Isolation and culture of mouse cortical astrocytes. J. Vis. Exp. JoVE 1, 50079. –7. doi:10.3791/50079
Shah, S., Yin, P. T., Uehara, T. M., Chueng, S. T. D., Yang, L., and Lee, K. B. (2014). Guiding stem cell differentiation into oligodendrocytes using graphene-nanofiber hybrid scaffolds. Adv. Mat. 26, 3673–3680. doi:10.1002/adma.201400523
Sharif, A., and Prevot, V. (2012). Isolation and culture of human astrocytes. Methods Mol. Biol. 814, 137–151. doi:10.1007/978-1-61779-452-0_11
Sharma, R., Smits, I. P. M., De La Vega, L., Lee, C., and Willerth, S. M. (2020). 3D bioprinting pluripotent stem cell derived neural tissues using a novel fibrin bioink containing drug releasing microspheres. Front. Bioeng. Biotechnol. 8, 57–12. doi:10.3389/fbioe.2020.00057
Shrirao, A. B., Kung, F. H., Omelchenko, A., Schloss, R. S., Boustany, N. N., Zahn, J. D., et al. (2018). Microfluidic platforms for the study of neuronal injury in vitro. Biotechnol. Bioeng. 115, 815–830. doi:10.1002/bit.26519
Slanzi, A., Iannoto, G., Rossi, B., Zenaro, E., and Constantin, G. (2020). In vitro models of neurodegenerative diseases. Front. Cell Dev. Biol. 8, 328. doi:10.3389/fcell.2020.00328
Song, S., Liu, X., Huang, J., and Zhang, Z. (2022). Neural stem cell-laden 3D bioprinting of polyphenol-doped electroconductive hydrogel scaffolds for enhanced neuronal differentiation. Biomater. Adv. 133, 112639. doi:10.1016/j.msec.2021.112639
Spearman, B. S., Agrawal, N. K., Rubiano, A., Simmons, C. S., Mobini, S., and Schmidt, C. E. (2020). Tunable methacrylated hyaluronic acid-based hydrogels as scaffolds for soft tissue engineering applications. J. Biomed. Mater. Res. Part A 108, 279–291. doi:10.1002/jbm.a.36814
Stanzione, A., Polini, A., La Pesa, V., Quattrini, A., Romano, A., Gigli, G., et al. (2021). Thermosensitive chitosan-based hydrogels supporting motor neuron-like NSC-34 cell differentiation. Biomater. Sci. 9, 7492–7503. doi:10.1039/d1bm01129d
Strickland, J. C. (2014). Guide to research techniques in neuroscience by matt carter and jennifer shieh. J. Undergrad. Neurosci. Educ. 13, 1–2.
Sun, W., Cornwell, A., Li, J., Peng, S., Osorio, M. J., Aalling, N., et al. (2017). SOX9 is an astrocyte-specific nuclear marker in the adult brain outside the neurogenic regions. J. Neurosci. 37, 4493–4507. doi:10.1523/JNEUROSCI.3199-16.2017
Sun, Y., Li, W., Wu, X., Zhang, N., Zhang, Y., Ouyang, S., et al. (2016). Functional self-assembling peptide nanofiber hydrogels designed for nerve degeneration. ACS Appl. Mat. Interfaces 8, 2348–2359. doi:10.1021/acsami.5b11473
Suzuki, H., Imajo, Y., Funaba, M., Nishida, N., Sakamoto, T., and Sakai, T. (2021). Current concepts of neural stem/progenitor cell therapy for chronic spinal cord injury. Front. Cell. Neurosci. 15, 794692. doi:10.3389/fncel.2021.794692
Swistowski, A., Peng, J., Liu, Q., Mali, P., Rao, M. S., Cheng, L., et al. (2010). Efficient generation of functional dopaminergic neurons from human induced pluripotent stem cells under defined conditions. Stem Cells 28, 1893–1904. doi:10.1002/stem.499
Takahashi, K., Tanabe, K., Ohnuki, M., Narita, M., Ichisaka, T., Tomoda, K., et al. (2007). Induction of pluripotent stem cells from adult human fibroblasts by defined factors. Cell 131, 861–872. doi:10.1016/j.cell.2007.11.019
Tarus, D., Hamard, L., Caraguel, F., Wion, D., Szarpak-Jankowska, A., Van Der Sanden, B., et al. (2016). Design of hyaluronic acid hydrogels to promote neurite outgrowth in three dimensions. ACS Appl. Mat. Interfaces 8, 25051–25059. doi:10.1021/acsami.6b06446
Taylor-Whiteley, T. R., Le Maitre, C. L., Duce, J. A., Dalton, C. F., and Smith, D. P. (2019). Recapitulating Parkinson’s disease pathology in a three-dimensional human neural cell culture model. Dis. Models Mech. 12, dmm038042. doi:10.1242/dmm.038042
Teixeira, M. I., Amaral, M. H., Costa, P. C., Lopes, C. M., and Lamprou, D. A. (2020). Recent developments in microfluidic technologies for central nervous system targeted studies. Pharmaceutics 12, 542–637. doi:10.3390/pharmaceutics12060542
Thomson, J. A., Itskovitz-Eldor, J., Shapiro, S. S., Waknitz, M. A., Swiergiel, J. J., Marshall, V. S., et al. (1998). Embryonic stem cell lines derived from human blastocysts. Science 282, 1145–1147. doi:10.1126/science.282.5391.1145
Tornero, D., Wattananit, S., Grønning Madsen, M., Koch, P., Wood, J., Tatarishvili, J., et al. (2013). Human induced pluripotent stem cell-derived cortical neurons integrate in stroke-injured cortex and improve functional recovery. Brain 136, 3561–3577. doi:10.1093/brain/awt278
Valério-Gomes, B., Guimarães, D. M., Szczupak, D., and Lent, R. (2018). The absolute number of oligodendrocytes in the adult mouse brain. Front. Neuroanat. 12, 90. doi:10.3389/fnana.2018.00090
van de Wijdeven, R., Ramstad, O. H., Bauer, U. S., Halaas, Ø., Sandvig, A., and Sandvig, I. (2018). Structuring a multi-nodal neural network in vitro within a novel design microfluidic chip. Biomed. Microdevices 20, 9. doi:10.1007/s10544-017-0254-4
Vazin, T., and Freed, W. J. (2010). Human embryonic stem cells: derivation, culture, and differentiation: a review. Restor. Neurology Neurosci. 28, 589–603. doi:10.3233/RNN-2010-0543
Veenvliet, J. V., Bolondi, A., Kretzmer, H., Haut, L., Scholze-Wittler, M., Schifferl, D., et al. (2020). Mouse embryonic stem cells self-organize into trunk-like structures with neural tube and somites. Science 370, eaba4937. doi:10.1126/science.aba4937
Warren, D., Tomaskovic-Crook, E., Wallace, G. G., and Crook, J. M. (2021). Engineering in vitro human neural tissue analogs by 3D bioprinting and electrostimulation. Apl. Bioeng. 5, 020901–020925. doi:10.1063/5.0032196
Weil, M. T., Schulz-Ëberlin, G., Mukherjee, C., Kuo-Elsner, W. P., Schäfer, I., Müller, C., et al. (2019). Isolation and culture of oligodendrocytes. Methods Mol. Biol. Clift. N.J.) 1936, 79, 95. doi:10.1007/978-1-4939-9072-6_5
Whittemore, S. R., and Onifer, S. M. (2000). Immortalized neural cell lines for CNS transplantation. Prog. Brain Res. 127, 49–65. doi:10.1016/S0079-6123(00)27005-2
Winter, C. C., Katiyar, K. S., Hernandez, N. S., Song, Y. J., Struzyna, L. A., Harris, J. P., et al. (2016). Transplantable living scaffolds comprised of micro-tissue engineered aligned astrocyte networks to facilitate central nervous system regeneration. Acta Biomater. 38, 44–58. doi:10.1016/j.actbio.2016.04.021
Woods, I., O’Connor, C., Frugoli, L., Kerr, S., Gutierrez Gonzalez, J., Stasiewicz, M., et al. (2022). Biomimetic scaffolds for spinal cord applications exhibit stiffness-dependent immunomodulatory and neurotrophic characteristics. Adv. Healthc. Mater. 11, 21016633–e2101717. doi:10.1002/adhm.202101663
Wu, C., Liu, A., Chen, S., Zhang, X., Chen, L., Zhu, Y., et al. (2019). Cell-laden electroconductive hydrogel simulating nerve matrix to deliver electrical cues and promote neurogenesis. ACS Appl. Mat. Interfaces 11, 22152–22163. doi:10.1021/acsami.9b05520
Wu, S., Xu, R., Duan, B., and Jiang, P. (2017). Three-dimensional hyaluronic acid hydrogel-based models for in vitro human iPSC-derived NPC culture and differentiation. J. Mat. Chem. B 5, 3870–3878. doi:10.1039/c7tb00721c
Yang, C. Y., Huang, W. Y., Chen, L. H., Liang, N. W., Wang, H. C., Lu, J., et al. (2021). Neural tissue engineering: the influence of scaffold surface topography and extracellular matrix microenvironment. J. Mat. Chem. B 9, 567–584. doi:10.1039/d0tb01605e
Yin, J., Yan, M., Wang, Y., Fu, J., and Suo, H. (2018). 3D bioprinting of low-concentration cell-laden gelatin methacrylate (GelMA) bioinks with a two-step cross-linking strategy. ACS Appl. Mat. Interfaces 10, 6849–6857. doi:10.1021/acsami.7b16059
Zafeiriou, M. P., Bao, G., Hudson, J., Halder, R., Blenkle, A., Schreiber, M. K., et al. (2020). Developmental GABA polarity switch and neuronal plasticity in Bioengineered Neuronal Organoids. Nat. Commun. 11, 3791–3812. doi:10.1038/s41467-020-17521-w
Zeiss, C. J. (2021). Comparative milestones in rodent and human postnatal central nervous system development. Toxicol. Pathol. 49, 1368–1373. doi:10.1177/01926233211046933
Zhang, X., Yin, M., and Zhang, M. (2014). Cell-based assays for Parkinson’s disease using differentiated human LUHMES cells. Acta Pharmacol. Sin. 35, 945–956. doi:10.1038/aps.2014.36
Zhang, Y., Chen, H., Long, X., and Xu, T. (2022). Three-dimensional-engineered bioprinted in vitro human neural stem cell self-assembling culture model constructs of Alzheimer’s disease. Bioact. Mater. 11, 192–205. doi:10.1016/j.bioactmat.2021.09.023
Zhou, L., Wolfes, A. C., Li, Y., Chan, D. C. W., Ko, H., Szele, F. G., et al. (2020a). Lipid-bilayer-supported 3D printing of human cerebral cortex cells reveals developmental interactions. Adv. Mater. 32, e2002183. doi:10.1002/adma.202002183
Zhou, P., Xu, P., Guan, J., Zhang, C., Chang, J., Yang, F., et al. (2020b). Promoting 3D neuronal differentiation in hydrogel for spinal cord regeneration. Colloids Surfaces B Biointerfaces 194, 111214. doi:10.1016/j.colsurfb.2020.111214
Zhu, H., Qiao, X., Liu, W., Wang, C., and Zhao, Y. (2020). Microglia play an essential role in synapse development and neuron maturation in tissue-engineered neural tissues. Front. Neurosci. 14, 586452. doi:10.3389/fnins.2020.586452
Zhu, W., Harris, B. T., and Zhang, L. G. (2016). Gelatin methacrylamide hydrogel with graphene nanoplatelets for neural cell-laden 3D bioprinting. Annu. Int. Conf. IEEE Eng. Med. Biol. Soc. IEEE Eng. Med. Biol. Soc. Annu. Int. Conf. 2016, 4185–4188. –4188. doi:10.1109/EMBC.2016.7591649
Zhuang, P., Sun, A. X., An, J., Chua, C. K., and Chew, S. Y. (2018). 3D neural tissue models: from spheroids to bioprinting. Biomaterials 154, 113–133. doi:10.1016/j.biomaterials.2017.10.002
Zuidema, J. M., Desmond, G. P., Rivet, C. J., Kearns, K. R., Thompson, D. M., and Gilbert, R. J. (2015). Nebulized solvent ablation of aligned PLLA fibers for the study of neurite response to anisotropic-to-isotropic fiber/film transition (AFFT) boundaries in astrocyte-neuron co-cultures. Biomaterials 46, 82–94. doi:10.1016/j.biomaterials.2014.12.046
Keywords: CNS modeling, cell sources, microfluidic, 3D bioprinting, in vitro models
Citation: Traldi C, Chiappini V, Menduti G, Tonda-Turo C and Boido M (2023) Advanced materials and biofabrication technologies to design in vitro functional central nervous system models. Front. Med. Eng. 1:1270943. doi: 10.3389/fmede.2023.1270943
Received: 01 August 2023; Accepted: 19 September 2023;
Published: 04 October 2023.
Edited by:
Akhilesh Rai, University of Coimbra, PortugalReviewed by:
Gemma Palazzolo, Italian Institute of Technology (IIT), ItalyYaqing Wang, university of science and technology of china, China
Jelena Ban, University of Rijeka, Croatia
Copyright © 2023 Traldi, Chiappini, Menduti, Tonda-Turo and Boido. This is an open-access article distributed under the terms of the Creative Commons Attribution License (CC BY). The use, distribution or reproduction in other forums is permitted, provided the original author(s) and the copyright owner(s) are credited and that the original publication in this journal is cited, in accordance with accepted academic practice. No use, distribution or reproduction is permitted which does not comply with these terms.
*Correspondence: Chiara Tonda-Turo, chiara.tondaturo@polito.it
†These authors have contributed equally to this work