The metabolic plasticity of B cells
- Metabolism and Cell Signalling Laboratory, Spanish National Cancer Research Centre, Madrid, Spain
The humoral response requires rapid growth, biosynthetic capacity, proliferation and differentiation of B cells. These processes involve profound B-cell phenotypic transitions that are coupled to drastic changes in metabolism so as to meet the extremely different energetic requirements as B cells switch from resting to an activated, highly proliferative state and to plasma or memory cell fates. Thus, B cells execute a multi-step, energetically dynamic process of profound metabolic rewiring from low ATP production to transient and large increments of energy expenditure that depend on high uptake and consumption of glucose and fatty acids. Such metabolic plasticity is under tight transcriptional and post-transcriptional regulation. Alterations in B-cell metabolism driven by genetic mutations or by extrinsic insults impair B-cell functions and differentiation and may underlie the anomalous behavior of pathological B cells. Herein, we review molecular switches that control B-cell metabolism and fuel utilization, as well as the emerging awareness of the impact of dynamic metabolic adaptations of B cells throughout the different phases of the humoral response.
Introduction
Naïve B cells maintain a state of quiescence coupled to an extremely low basal metabolism that is interrupted when antigen encounter triggers a massive uptake of nutrients and biomass accumulation to sustain a sudden burst in proliferation and a several-fold increase in cell size. Different phases of the humoral response, including the Germinal Center (GC) cyclic transitions from cell growth in the light zone to proliferation in the dark zone, the formation of long-lived, resting but alert memory cells and the antibody production and secretion by Plasma Cells (PC), demand the rewiring of metabolic fluxes to match the functions and energetic requirements of the different phenotypic B-cell states (Boothby and Rickert, 2017).
At the molecular level, key regulators of activated B-cell functions exert a parallel control on metabolism. Therefore, alterations in B cells leading to diseases such as autoimmunity, immune deficiency and B-cell lymphomagenesis occur in the context of abnormal B-cell metabolism, as recently reviewed (Boothby and Rickert, 2017; Iperi et al., 2021; Raza and Clarke, 2021; Shiraz et al., 2022). Thus, understanding normal and dysfunctional B-cell metabolism may uncover potential avenues for interventions to harness pathogenic activities of B cells.
An interesting feature of B-cell metabolism is that it is multifaceted and the reprogramming subsequent to activation is not exclusively based on upregulating a specific metabolic route, such glucose uptake and consumption via glycolysis (Dufort et al., 2007; Caro-Maldonado et al., 2014). Instead, increased in B-cell metabolism comprise the involvement of multiple metabolic routes.
We herein analyze the main metabolic adaptations that occur in B cells through maturation, activation and differentiation, highlighting different metabolic routes, energetic substrates, as well as molecular effectors shaping B-cell metabolism (Figure 1).
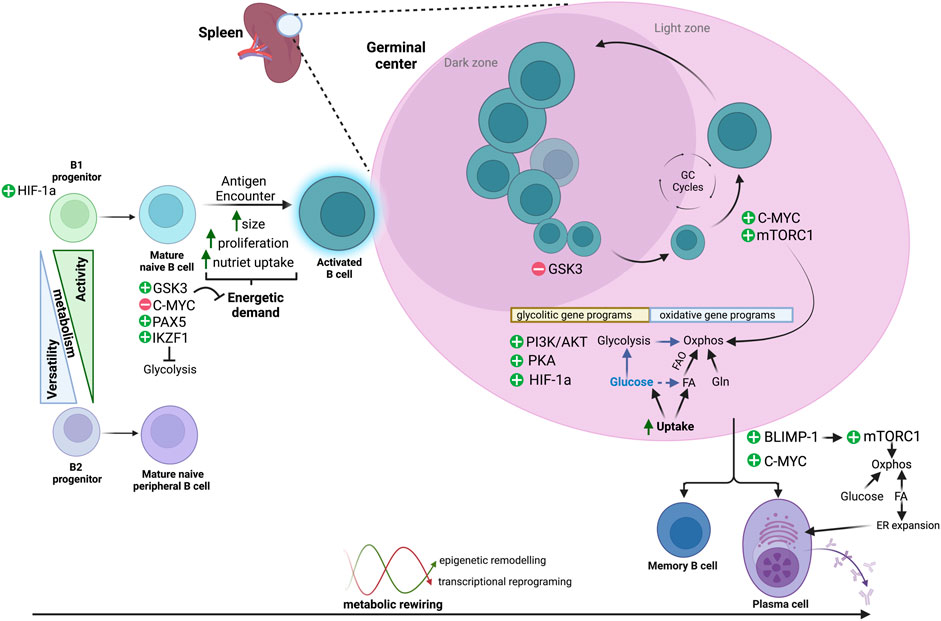
FIGURE 1. Metabolic routes across B-cell differentiation. B cells display dynamic metabolic changes to meet fluctuating energetic demands as they transition from distinct B-cell lineages, through resting states of low metabolic activity, and following antigen encounter, to an activated and highly proliferative state with increased metabolic activity. B cells activate specific transcriptional programs and regulatory pathways that allow them to either maintain a low metabolic profile or to use a wide range of substrates [glucose, fatty acids (FA) and/or glutamine (Gln)] and different metabolic routes (i.e., glycolysis, Oxphos, FAO). These metabolic transitions are reversible and tightly regulated, and an interplay between epigenetic remodelling and metabolic fluctuations may control transitions throughout the different phenotypic states.
The metabolism of B-cell maturation
B-cell development from lymphoid progenitors derived from hematopoietic stem cells occurs within the fetal liver and bone marrow (BM), and involves subsequent stages of maturation in secondary lymphoid organs such as spleen and lymph nodes. A crucial event in B-cell differentiation is immunoglobulin gene rearrangement, a process that occurs in progenitor pro-B cells and results in precursor pre-B cells that express an immature B-cell receptor (BCR). Further gene rearrangements yield the expression of a mature BCR and the consequent transition to immature B-cell stage. BCR reactivity against self-antigens is then censored via apoptosis, while cells expressing non-self-reactive BCRs continue the B-cell maturation process (Martin et al., 2016).
Along with this sequence of events, as early as the B-cell linage is specified, phenotypic heterogeneity goes hand-in-hand with metabolic disparities that will determine distinct B-cell lineages (Sanz et al., 2019; Iperi et al., 2021). For example, B-cell lineages established either from perinatal and peripheral B1 progenitors or from conventional BM B2 progenitors, show distinct fates and metabolic specifications, being tissue-resident B1 B-cells metabolically more active with higher rates of glycolysis, oxidative phosphorylation (Oxphos) and fatty-acid (FA) metabolism, but less versatile than resting follicular (FO) B2 B cells (Clarke et al., 2018).
Beyond lineage-dependent metabolic specificities, pro-B cells in the BM preferentially display a glycolytic metabolism established through the transcriptional control of glycolytic genes in a hypoxia inducible factor 1α (HIF-1α) -dependent manner. Deletion of HIF-1α in mice results in the upregulation of genes involved in Oxphos and the tricarboxylic acid (TCA) cycle, impairing the transition from pro- to pre-B-cell stage (Kojima et al., 2010). Selected transitional B cells continue their maturation process toward resting FO B cells. The phenotypic switch towards mature naïve follicular stages relies on metabolic adjustments that facilitate a quiescence-like metabolic program maintained by the downregulation of Oxphos and inhibition of the mechanistic target of rapamycin complex 1 (mTORC1), in an AMP-activated protein kinase (AMPK)-dependent manner (Farmer et al., 2019).
Metabolic switches in B-cell activation and the germinal center reaction
The broad repertoire of B-cell phenotypic states is tightly regulated by the combination of specific transcriptional programs and microenvironmental cues that impose metabolic dependencies. The GC are transient micro-anatomical structures that cluster several clones of FO cells that have been activated by a T cell dependent antigen, together with T follicular helper (Tfh) cells and other cell types. Through sequential rounds of Tfh-mediated activation and selection of competing clones of GC B cells, followed by clonal expansion and antibody diversification, the GC reaction facilitates an increase in the affinity of the selected antibody-producing cells, while also yielding memory B cells. Each phase of the GC reaction and the fates of the exiting GC B cells are shaped by profound metabolic changes.
Glucose and glycolysis: Leading role or supporting actor
Highly proliferative cells increase glucose uptake and its breakdown through glycolysis, even in normoxia, a phenomenon called “Warburg effect” (Warburg, 1956). This metabolic feature, deeply studied in cancer cells, is also relevant during the clonal expansion phase of GC B cells. In this context, the serial reactions of glucose catabolism yield only two ATPs but provide numerous intermediate metabolites (Vander Heiden et al., 2009) used by the growing B cells as bricks for macromolecule synthesis and biomass accumulation.
Before antigen encounter, glucose metabolism is transcriptionally repressed by specific B-cell lineage factors such as PAX5 and IKZF1 (Chan et al., 2017), but following antigen stimulation, B cells maximize glucose uptake through the upregulation of the glucose transporters GLUT1, GLUT4, GLUT8 and GLUT11 (McBrayer et al., 2012), while conclusions on the expression of GLUT3 are divergent (Doughty et al., 2006; Dufort et al., 2007; McBrayer et al., 2012; Caro-Maldonado et al., 2014). Antigen-mediated induction of glucose transporters is framed within a broader transcriptional reprograming of glycolytic genes (Cho et al., 2016) to guarantee B-cell survival and alleviate the burden of several energetically onerous processes downstream of B-cell activation, such as proliferation and, in case of PC differentiation, to fuel antibody production (Dufort et al., 2007; Caro-Maldonado et al., 2014; Jellusova et al., 2017). These transcriptional changes favor the increase in the glycolytic rate, concomitantly with the activation of pathways that orchestrate B-cell metabolic reprogramming downstream of BCR activation, such as PI3K/Akt signaling (Doughty et al., 2006; Patke et al., 2006; Woodland et al., 2008). In addition, the IL-4/STAT6 axis, with established systemic effects on nutrient uptake and insulin signaling (Ricardo-Gonzalez et al., 2010), also increases glycolysis in activated B cells (Dufort et al., 2007).
The glycogen synthase kinase 3 (GSK3) integrates cytokine and nutrient signaling. GSK3 is a metabolic modulator in resting cells with limited nutrient content and is rapidly inhibited via a PI3K/Akt-dependent phosphorylation downstream of BCR and by co-stimulatory signals such as the CD40-CD40L tandem during B-cell-T cell synapse in the GC (Cross et al., 1995). Active GSK3 maintains a low steady-state metabolism in naïve B cells by restraining increments in cell mass and proliferation through the inhibition of the transcription factor C-MYC (Jellusova et al., 2017; Varano et al., 2017). Consequently, GSK3 preserves a restricted energetic status to ensure B-cell survival and allows the early increase in metabolic activity following antigen encounter. B-cell activation results in inhibition of GSK3 by, in addition to the aforementioned PI3K-Akt dependent signals, cAMP- and PKA-dependent stimuli that result in metabolic activation and proliferation (Cato et al., 2011). Importantly, even in conditions of GSK3 inhibition such as after T cell-dependent-stimulation, residual GSK3 activity may be important to harness B-cell proliferation by tuning down glycolysis and mitochondrial biogenesis and to prevent metabolic exhaustion and ROS-mediated cell death (Jellusova et al., 2017; Varano et al., 2017). These antagonistic roles of GSK3, critical for preventing a metabolic collapse of GC B cells, may be conditioned by a dark-zone-to-light-zone decreasing oxygen tension gradient across the GC (Cho et al., 2016). In the normoxic dark zone, higher metabolic rates sustain clonal expansion associated with repressed GSK3 activity while the moderate hypoxic conditions of the light zone contribute to maintain GSK3 activity and its restrictive metabolic effects. Such on-off cycles of GSK3 activity and the repressive effect on C-MYC have been proposed as a main tumor suppressive axis in the GC (Calado et al., 2012; Dominguez-Sola et al., 2012; Finkin et al., 2019).
The interplay of oxygen availability and the cyclic metabolic behavior of GC B cells is mediated by HIF-1α-dependent upregulation of glycolytic gene programs to support anabolism (Cho et al., 2016; Jellusova et al., 2017; Li et al., 2021). It is conceivable that HIF-1α behaves as a metabolic rheostat that adjusts humoral immune responses in a context dependent manner, since both positive and detrimental effects of hypoxia on antibody class-switch recombination have been reported (Abbott et al., 2016; Cho et al., 2016). In contrast to its critical impact during affinity maturation in the GC, the HIF-1α transcriptional program is intriguingly dispensable for the initial glycolytic reprogramming in LPS-activated B cells (Caro-Maldonado et al., 2014).
An emergent concept in GC biology is that the increased metabolic demand coupled to rapid growth and proliferation of GC B cells happens under an environment of transient limitations in nutrients and oxygen (Abbott et al., 2016; Cho et al., 2016), and GC B cells count with an overlapping repertoire of signaling pathways to cope with cyclic metabolic stress. We still need to understand how antibody affinity, metabolic limitations and energetic demands are integrated to maximize the expansion of selected clones without reaching a threshold of deleterious metabolic stress.
The mTORC1 signaling pathway links nutrient availability, anabolism, positive selection and clonal expansion. mTORC1 is involved in GC formation, GC B-cell expansion, metabolic reprogramming and PC differentiation, and both pharmacological and genetic inhibition of mTORC1 largely disrupt antigen-induced cell growth and impair antibody secretion (Cho et al., 2016; Jones et al., 2016; Jellusova et al., 2017; Tsui et al., 2018). The physical interaction of a Tfh and a GC B cell in the light zone is proportional to the amount of antigen presented by the B cell and thus, to the affinity of the antibody expressed. This interaction, called “synapse”, results in rapid activation of mTORC1 and ultimately, in positive selection of the interacting B cell (Ersching et al., 2017). mTORC1 activation in light zone B cells is critical for ribosome biogenesis and anabolic cell growth, processes that precede the proliferative burst that takes place only after B cells have migrated to the dark zone (Victora and Nussenzweig, 2022) (Figure 1). Importantly, mTORC1 activity is strictly required to trigger cell growth but is largely dispensable after activated B cells increase in size and start to execute rapid rounds of cell division (Ersching et al., 2017). The inability to shut off mTORC1 activity by means of constitutively-active nutrient or growth factor signaling to mTORC1 is detrimental and decreases fitness of GC B cells (Goldfinger et al., 2011; Ersching et al., 2017; Long et al., 2022). Interestingly, a moderate, partial increase in nutrient-Rag GTPase signaling results in enhanced B-cell activation, enlarged GC and increased high-affinity antibody production (Ortega-Molina et al., 2019). Moreover, moderately activating mutations in RagC have been exclusively found in human follicular lymphoma (FL) and diffuse large B-cell lymphoma (DLBCL) samples (Green et al., 2015; Okosun et al., 2016), while a mild inhibition of nutrient signaling to mTORC1 impairs the GC response (Ortega-Molina et al., 2021). In addition to its function controlling B-cell growth and proliferation, mTORC1 plays a key role in regulating antibody class-switch recombination, a process that is also tuned by nutrient availability and oxygen tension (Keating et al., 2013; Zhang et al., 2013; Cho et al., 2016). Collectively, these results strongly suggest the existence of exquisitely refined sensitivity of B cells to moderate fluctuations in the nutrient signaling pathway. Finally, beyond the control of the GC reaction, activation of mTORC1 also fuels the rapid extra-follicular proliferation of activated B cells, and this effect is independent from the differentiation into antibody-secreting cells, suggesting that B-cell differentiation and cell division can be uncoupled (Gaudette et al., 2021).
Oxidative metabolism in B cells: Beyond mitochondrial oxidation of glucose
Despite the relevance of a dynamic control of mTORC1 in B-cell functions, this pathway is not directly involved in the regulation of glycolysis upon antigen-stimulation (Doughty et al., 2006), suggesting that pathways other than glycolysis support the remodeling that allows B-cell differentiation and function.
Increased glucose uptake following antigen stimulation is not merely purposed to feed glycolytic flux, but it also yields glucose-derived pyruvate, ultimately shunted to the mitochondria for an oxidative process that is further supported by an increase in mitochondrial mass (Jang et al., 2015; Jellusova et al., 2017) and by the upregulation of gene programs involved in mitochondrial respiration. Such parallel increase in Oxphos sustains the energetic costs of several consecutive rounds of proliferation during clonal expansion and it is also critical to support PC differentiation by alleviating the energetic burden imposed by massive antibody secretion (Adams et al., 2016; Cho et al., 2016; Price et al., 2018). This increased metabolic capacity of PC is driven by the expression of specific lineage transcriptions factors, such as BLIMP1, required for PC differentiation (Price et al., 2018) and the activation of anabolic factors that positively regulate Oxphos by driving mitochondrial remodeling, such as the aforementioned mTORC1 and C-MYC (Tsui et al., 2018).
Glucose-derived pyruvate substantially feeds Oxphos, but other substrates also contribute to fuel respiration. For example, while hampering glutamine metabolism has minimal impact on FO B cells (Choi et al., 2018), glutamine consumption via Oxphos is largely increased and is required for GC B-cell proliferation and for PC cells (Garcia-Manteiga et al., 2011; Caro-Maldonado et al., 2014; Waters et al., 2018) highlighting the existence of multiple parallel fuel dependencies in active B cells. In addition to glycolysis and glutaminolysis, recent evidence shows that FA oxidation (FAO) may be a main energetic source for GC B cells (Weisel et al., 2020) in contrast to previous studies pointing to reduced lipid oxidation and increased pyruvate oxidation in B cells stimulated in vitro (Caro-Maldonado et al., 2014). The use of lipids as fuel in GC B cells involves mitochondrial and peroxisomal FAO, being both endogenous and exogenously uptaken FA burned preferentially over glucose (Weisel et al., 2020). This FAO-centric vision of the GC is in agreement with the simultaneous transcriptional selective upregulation of genes related with FAO and lipid metabolism in GC B cells (Cho et al., 2016). However, the energetic dependence on exogenous FA has been observed in intestinal PC (Kunisawa et al., 2015; Kim et al., 2016) and B1 B cells (Clarke et al., 2018; Muri et al., 2019), suggesting that lipid metabolism may be relevant beyond GC B cells during the humoral response.
Interestingly, in an apparent contradiction to the increased catabolism, de novo synthesis of FA from glucose also constitutes a metabolic adaptation essential to engage PC differentiation (Dufort et al., 2014). Concomitant synthesis and breakdown of FA may be perceived as a futile cycle, but simultaneous catabolism and anabolism of the same substrates is not uncommon in highly proliferative cells such as cancer cells and may enable the profound structural changes that B cells undertake during their PC differentiation process. PC must expand the endoplasmic reticulum (ER) and Golgi apparatus in order to boost the synthesis (and secretion) of antibodies, which may account for 90% of total protein translation in an extremely energetically onerous process. To sustain such biosynthetic and secretory capacity, PC must largely expand the endomembrane compartment, while simultaneously produce large quantities of ATP from FAO, so FA are acquired from external sources and endogenously produced to fulfill both structural and metabolic fates of FA. Of note, the transcriptional regulator of PC lineage differentiation is BLIMP1, positive regulator of mTORC1 activity and critical mediator of the Unfolded Protein Response (UPR) (Tellier et al., 2016). Activation of mTORC1 boosts mRNA translation but can also induce proteostatic stress (Goldfinger et al., 2011). In turn, Blimp1-mediated control of the UPR helps counteract the stress resulting from increased translation through the expansion of the ER and Golgi apparatus and thus, the biosynthetic capacity of the antibody-producing cell. Moreover, a link between the UPR effector ATF4, amino acid metabolism and autophagy has been recently established in lymphoma cells, suggesting the existence of a functional regulatory connection between UPR and mTORC1 signaling in B cells (Li et al., 2022).
Cancer research has established a solid link between metabolism and epigenetic control of transcription and this concept is emerging in B-cell physiology. Metabolism not only supplies energy and anabolic bricks to support structural remodeling during B-cell differentiation, but also impacts on the epigenetic regulation of specific transcriptional programs. Thus, metabolic rewiring controls B-cell functions and fate decisions by changing the availability of metabolites such as the TCA cycle intermediate α-ketoglutarate, cofactor of the UTX demethylase that regulates expression of the master regulator for GC B-cell differentiation BCL6 (Haniuda et al., 2020). The phenotypic plasticity that B cells show throughout the GC reaction and the rapid transitions through changes in the expression of specific gene programs are achieved via reversible epigenetic remodeling of specific histone marks. These modifications are frequently anomalous in DLBCL and FL due to mutations in genes encoding histone remodelers such as EZH2, CREBBP, EP300 or KMT2D (Green, 2018; Pasqualucci and Dalla-Favera, 2018; Schmitz et al., 2018; Mondello et al., 2022). Moreover, serine-glycine metabolism cooperates with BCL2 driving lymphomagenesis through epigenetic silencing of tumour suppressor genes (Parsa et al., 2020). Latent epigenetic differences exist in resting naïve B cells of lupus patients, involving differential expression of metabolic gene sets with roles in Oxphos, UPR and the response to hypoxia (Scharer et al., 2019). Short-chain FA derived from gut microbiota do not merely act as energetic substrates in B cells, but as HDAC inhibitors, thus disrupting antibody responses in healthy mice and murine models of lupus (Sanchez et al., 2020). Thus, awareness of the functional impact of B-cell metabolism as a modulator of epigenetic alterations during lymphomagenesis and in other pathogenic B cells is beginning to emerge.
Concluding remarks
Throughout each transition in the entire multifaceted lifespan of a B cell, profound metabolic rearrangements occur (Figure 1). We currently count with a limited number of photograms of B-cell metabolism, so the plot of metabolic control of the humoral response remains to be revealed. We need to deepen our understanding on how metabolism is integrated with increasing antibody affinity during clonal evolution, on how conflicting signals are resolved and to what extent does B-cell metabolism dictate the cyclic behavior on the GC. GC B cells show profuse synaptic interactions with the GC microenvironment, but metabolic cross-talk of B cells and other components of the GC remain poorly understood. We also need to learn how aberrant metabolism of B cells precipitates self-reactivity or B-cell transformation. In this regard, deregulated activity of epigenetic modifiers is a hallmark of B-cell lymphomas and our awareness of metabolite availability as a key layer of control for epigenetic modifiers is emerging. The inability to recapitulate the cellular interactions of the humoral response in culture has undermined our insight on B-cell physiology. However, recent technological advances in single-cell technologies, high-content in vivo microscopy and in temporal and spatial tracing of metabolites will enable a better understanding of B-cell metabolism and continue settling the perception of its determinant role in dictating B-cell behavior.
Author contributions
YV-G and AE conceived, wrote and edited the text and figures.
Funding
AE lab is supported by the Retos Projects Program of the Spanish Ministry of Science, Innovation and Universities, the Spanish State Research Agency (AEI/10.13039/501100011033) co-funded by the European Regional Development Fund (PID 2019-104012RB-I00), a FERO Grant for Research in Oncology, and La Caixa Foundation (HR21-00046). AE is an EMBO Young Investigator. YV-G is a recipient of a CNIO Friends Fellowship supported by the Fundación Domingo Martinez.
Conflict of interest
The authors declare that the research was conducted in the absence of any commercial or financial relationships that could be construed as a potential conflict of interest.
Publisher’s note
All claims expressed in this article are solely those of the authors and do not necessarily represent those of their affiliated organizations, or those of the publisher, the editors and the reviewers. Any product that may be evaluated in this article, or claim that may be made by its manufacturer, is not guaranteed or endorsed by the publisher.
References
Abbott, R. K., Thayer, M., Labuda, J., Silva, M., Philbrook, P., Cain, D. W., et al. (2016). Germinal center hypoxia potentiates immunoglobulin class switch recombination. J. Immunol. 197, 4014–4020. doi:10.4049/JIMMUNOL.1601401
Adams, W. C., Chen, Y. H., Kratchmarov, R., Yen, B., Nish, S. A., Lin, W. H. W., et al. (2016). Anabolism-associated mitochondrial stasis driving lymphocyte differentiation over self-renewal. Cell Rep. 17, 3142–3152. doi:10.1016/J.CELREP.2016.11.065
Boothby, M., and Rickert, R. C. (2017). Metabolic regulation of the immune humoral response. Immunity 46, 743–755. doi:10.1016/J.IMMUNI.2017.04.009
Calado, D. P., Sasaki, Y., Godinho, S. A., Pellerin, A., Köchert, K., Sleckman, B. P., et al. (2012). The cell-cycle regulator c-Myc is essential for the formation and maintenance of germinal centers. Nat. Immunol. 13, 1092–1100. doi:10.1038/NI.2418
Caro-Maldonado, A., Wang, R., Nichols, A. G., Kuraoka, M., Milasta, S., Sun, L. D., et al. (2014). Metabolic reprogramming is required for antibody production that is suppressed in anergic but exaggerated in chronically BAFF-exposed B cells. J. Immunol. 192, 3626–3636. doi:10.4049/jimmunol.1302062
Cato, M. H., Chintalapati, S. K., Yau, I. W., Omori, S. A., and Rickert, R. C. (2011). Cyclin D3 is selectively required for proliferative expansion of germinal center B cells. Mol. Cell. Biol. 31, 127–137. doi:10.1128/MCB.00650-10
Chan, L. N., Chen, Z., Braas, D., Lee, J. W., Xiao, G., Geng, H., et al. (2017). Metabolic gatekeeper function of B-lymphoid transcription factors. Nature 542 (7642), 479–483. doi:10.1038/nature21076
Cho, S. H., Raybuck, A. L., Stengel, K., Wei, M., Beck, T. C., Volanakis, E., et al. (2016). Germinal centre hypoxia and regulation of antibody qualities by a hypoxia response system. Nature 537, 234–238. doi:10.1038/nature19334
Choi, S. C., Titov, A. A., Abboud, G., Seay, H. R., Brusko, T. M., Roopenian, D. C., et al. (2018). Inhibition of glucose metabolism selectively targets autoreactive follicular helper T cells. Nat. Commun. 9, 4369. doi:10.1038/S41467-018-06686-0
Clarke, A. J., Riffelmacher, T., Braas, D., Cornall, R. J., and Simon, A. K. (2018). B1a B cells require autophagy for metabolic homeostasis and self-renewal. J. Exp. Med. 215, 399–413. doi:10.1084/JEM.20170771
Cross, D. A. E., Alessi, D. R., Cohen, P., Andjelkovich, M., and Hemmings, B. A. (1995). Inhibition of glycogen synthase kinase-3 by insulin mediated by protein kinase B. Nature 378, 785–789. doi:10.1038/378785A0
Dominguez-Sola, D., Victora, G. D., Ying, C. Y., Phan, R. T., Saito, M., Nussenzweig, M. C., et al. (2012). The proto-oncogene MYC is required for selection in the germinal center and cyclic reentry. Nat. Immunol. 13, 1083–1091. doi:10.1038/NI.2428
Doughty, C. A., Bleiman, B. F., Wagner, D. J., Dufort, F. J., Mataraza, J. M., Roberts, M. F., et al. (2006). Antigen receptor-mediated changes in glucose metabolism in B lymphocytes: Role of phosphatidylinositol 3-kinase signaling in the glycolytic control of growth. Blood 107 (11), 4458–4465. doi:10.1182/blood-2005-12-4788
Dufort, F. J., Bleiman, B. F., Gumina, M. R., Blair, D., Wagner, D. J., Roberts, M. F., et al. (2007). Cutting edge: IL-4-mediated protection of primary B lymphocytes from apoptosis via stat6-dependent regulation of glycolytic metabolism. J. Immunol. 179, 4953–4957. doi:10.4049/jimmunol.179.8.4953
Dufort, F. J., Gumina, M. R., Ta, N. L., Tao, Y., Heyse, S. A., Scott, D. A., et al. (2014). Glucose-dependent de novo lipogenesis in B lymphocytes: A requirement for atp-citrate lyase in lipopolysaccharide-induced differentiation. J. Biol. Chem. 289, 7011–7024. doi:10.1074/JBC.M114.551051
Ersching, J., Efeyan, A., Mesin, L., Jacobsen, J. T., Pasqual, G., Grabiner, B. C., et al. (2017). Germinal center selection and affinity maturation require dynamic regulation of mTORC1 kinase. Immunity 46, 1045–1058. e6. doi:10.1016/J.IMMUNI.2017.06.005
Farmer, J. R., Allard-Chamard, H., Sun, N., Ahmad, M., Bertocchi, A., Mahajan, V. S., et al. (2019). Induction of metabolic quiescence defines the transitional to follicular B cell switch. Sci. Signal. 12, eaaw5573. doi:10.1126/SCISIGNAL.AAW5573
Finkin, S., Hartweger, H., Oliveira, T. Y., Kara, E. E., and Nussenzweig, M. C. (2019). Protein amounts of the MYC transcription factor determine germinal center B cell division capacity. Immunity 51, 324–336. e5. doi:10.1016/J.IMMUNI.2019.06.013
Garcia-Manteiga, J. M., Mari, S., Godejohann, M., Spraul, M., Napoli, C., Cenci, S., et al. (2011). Metabolomics of B to plasma cell differentiation. J. Proteome Res. 10, 4165–4176. doi:10.1021/PR200328F
Gaudette, B. T., Roman, C. J., Ochoa, T. A., Atria, D. G., Jones, D. D., Siebel, C. W., et al. (2021). Resting innate-like B cells leverage sustained Notch2/mTORC1 signaling to achieve rapid and mitosis-independent plasma cell differentiation. J. Clin. Invest. 131, e151975. doi:10.1172/JCI151975
Goldfinger, M., Shmuel, M., Benhamron, S., and Tirosh, B. (2011). Protein synthesis in plasma cells is regulated by crosstalk between endoplasmic reticulum stress and mTOR signaling. Eur. J. Immunol. 41, 491–502. doi:10.1002/EJI.201040677
Green, M. R. (2018). Chromatin modifying gene mutations in follicular lymphoma. Blood 131, 595–604. doi:10.1182/BLOOD-2017-08-737361
Green, M. R., Kihira, S., Liu, C. L., Nair, R. V., Salari, R., Gentles, A. J., et al. (2015). Mutations in early follicular lymphoma progenitors are associated with suppressed antigen presentation. Proc. Natl. Acad. Sci. U. S. A. 112, E1116–E1125. doi:10.1073/PNAS.1501199112
Haniuda, K., Fukao, S., and Kitamura, D. (2020). Metabolic reprogramming induces germinal center B cell differentiation through Bcl6 locus remodeling. Cell Rep. 33, 108333. doi:10.1016/j.celrep.2020.108333
Iperi, C., Bordron, A., Dueymes, M., Pers, J. O., and Jamin, C. (2021). Metabolic program of regulatory B lymphocytes and influence in the control of malignant and autoimmune situations. Front. Immunol. 12, 735463. doi:10.3389/FIMMU.2021.735463
Jang, K. J., Mano, H., Aoki, K., Hayashi, T., Muto, A., Nambu, Y., et al. (2015). Mitochondrial function provides instructive signals for activation-induced B-cell fates. Nat. Commun. 6, 6750. doi:10.1038/NCOMMS7750
Jellusova, J., Cato, M. H., Apgar, J. R., Ramezani-Rad, P., Leung, C. R., Chen, C., et al. (2017). Gsk3 is a metabolic checkpoint regulator in B cells. Nat. Immunol. 18, 303–312. doi:10.1038/ni.3664
Jones, D. D., Gaudette, B. T., Wilmore, J. R., Chernova, I., Bortnick, A., Weiss, B. M., et al. (2016). mTOR has distinct functions in generating versus sustaining humoral immunity. J. Clin. Invest. 126, 4250–4261. doi:10.1172/JCI86504
Keating, R., Hertz, T., Wehenkel, M., Harris, T. L., Edwards, B. A., McClaren, J. L., et al. (2013). The kinase mTOR modulates the antibody response to provide cross-protective immunity to lethal infection with influenza virus. Nat. Immunol. 14, 1266–1276. doi:10.1038/NI.2741
Kim, M., Qie, Y., Park, J., and Kim, C. H. (2016). Gut microbial metabolites fuel host antibody responses. Cell Host Microbe 20, 202–214. doi:10.1016/J.CHOM.2016.07.001
Kojima, H., Kobayashi, A., Sakurai, D., Kanno, Y., Hase, H., Takahashi, R., et al. (2010). Differentiation stage-specific requirement in hypoxia-inducible factor-1alpha-regulated glycolytic pathway during murine B cell development in bone marrow. J. Immunol. 184, 154–163. doi:10.4049/JIMMUNOL.0800167
Kunisawa, J., Arita, M., Hayasaka, T., Harada, T., Iwamoto, R., Nagasawa, R., et al. (2015). Dietary ω3 fatty acid exerts anti-allergic effect through the conversion to 17, 18-epoxyeicosatetraenoic acid in the gut. Sci. Rep. 5, 9750. doi:10.1038/SREP09750
Li, L., Feng, C., Qin, J., Li, D., Liu, M., Han, S., et al. (2021). Regulation of humoral immune response by HIF-1α-dependent metabolic reprogramming of the germinal center reaction. Cell. Immunol. 367, 104409. doi:10.1016/J.CELLIMM.2021.104409
Li, M., Teater, M. R., Hong, J. Y., Park, N. R., Duy, C., Shen, H., et al. (2022). Translational activation of ATF4 through mitochondrial anaplerotic metabolic pathways is required for DLBCL growth and survival. Blood Cancer Discov. 3, 50–65. doi:10.1158/2643-3230.BCD-20-0183
Long, Z., Phillips, B., Radtke, D., Meyer-Hermann, M., and Bannard, O. (2022). Competition for refueling rather than cyclic reentry initiation evident in germinal centers. Sci. Immunol. 7, eabm0775. doi:10.1126/SCIIMMUNOL.ABM0775
Martin, V. G., Wu, Y. C. B., Townsend, C. L., Lu, G. H. C., O’Hare, J. S., Mozeika, A., et al. (2016). Transitional B cells in early human B cell development - time to revisit the paradigm? Front. Immunol. 7, 546. doi:10.3389/FIMMU.2016.00546
McBrayer, S. K., Cheng, J. C., Singhal, S., Krett, N. L., Rosen, S. T., and Shanmugam, M. (2012). Multiple myeloma exhibits novel dependence on GLUT4, GLUT8, and GLUT11: Implications for glucose transporter-directed therapy. Blood 119, 4686–4697. doi:10.1182/BLOOD-2011-09-377846
Mondello, P., Ansell, S. M., and Nowakowski, G. S. (2022). Immune epigenetic crosstalk between malignant B cells and the tumor microenvironment in B cell lymphoma. Front. Genet. 13, 826594. doi:10.3389/FGENE.2022.826594
Muri, J., Thut, H., Bornkamm, G. W., and Kopf, M. (2019). B1 and marginal zone B cells but not follicular B2 cells require Gpx4 to prevent lipid peroxidation and ferroptosis. Cell Rep. 29, 2731–2744. e4. doi:10.1016/J.CELREP.2019.10.070
Okosun, J., Wolfson, R. L., Wang, J., Araf, S., Wilkins, L., Castellano, B. M., et al. (2016). Recurrent mTORC1-activating RRAGC mutations in follicular lymphoma. Nat. Genet. 48, 183–188. doi:10.1038/NG.3473
Ortega-Molina, A., Deleyto-Seldas, N., Carreras, J., Sanz, A., Lebrero-Fernández, C., Menéndez, C., et al. (2019). Oncogenic Rag GTPase signaling enhances B cell activation and drives follicular lymphoma sensitive to pharmacological inhibition of mTOR. Nat. Metab. 1, 775–789. doi:10.1038/S42255-019-0098-8
Ortega-Molina, A., Lebrero-Fernández, C., Sanz, A., Deleyto-Seldas, N., Belén Plata-Gómez, A., Menéndez, C., et al. (2021). Inhibition of Rag GTPase signaling in mice suppresses B cell responses and lymphomagenesis with minimal detrimental trade-offs. Cell Rep. 36, 109372. doi:10.1016/j.celrep.2021.109372
Parsa, S., Ortega-Molina, A., Ying, H. Y., Jiang, M., Teater, M., Wang, J., et al. (2020). The serine hydroxymethyltransferase-2 (SHMT2) initiates lymphoma development through epigenetic tumor suppressor silencing. Nat. cancer 1, 653–664. doi:10.1038/S43018-020-0080-0
Pasqualucci, L., and Dalla-Favera, R. (2018). Genetics of diffuse large B-cell lymphoma. Blood 131, 2307–2319. doi:10.1182/BLOOD-2017-11-764332
Patke, A., Mecklenbräuker, I., Erdjument-Bromage, H., Tempst, P., and Tarakhovsky, A. (2006). BAFF controls B cell metabolic fitness through a PKC beta- and Akt-dependent mechanism. J. Exp. Med. 203, 2551–2562. doi:10.1084/JEM.20060990
Price, M. J., Patterson, D. G., Scharer, C. D., and Boss, J. M. (2018). Progressive upregulation of oxidative metabolism facilitates plasmablast differentiation to a T-independent antigen. Cell Rep. 23, 3152–3159. doi:10.1016/J.CELREP.2018.05.053
Raza, I. G. A., and Clarke, A. J. (2021). B cell metabolism and autophagy in autoimmunity. Front. Immunol. 12, 2157. doi:10.3389/fimmu.2021.681105
Ricardo-Gonzalez, R. R., Eagle, A. R., Odegaard, J. I., Jouihan, H., Morel, C. R., Heredia, J. E., et al. (2010). IL-4/STAT6 immune axis regulates peripheral nutrient metabolism and insulin sensitivity. Proc. Natl. Acad. Sci. U. S. A. 107, 22617–22622. doi:10.1073/PNAS.1009152108
Sanchez, H. N., Moroney, J. B., Gan, H., Shen, T., Im, J. L., Li, T., et al. (2020). B cell-intrinsic epigenetic modulation of antibody responses by dietary fiber-derived short-chain fatty acids. Nat. Commun. 11 (1), 60–19. doi:10.1038/s41467-019-13603-6
Sanz, I., Wei, C., Jenks, S. A., Cashman, K. S., Tipton, C., Woodruff, M. C., et al. (2019). Challenges and opportunities for consistent classification of human B cell and plasma cell populations. Front. Immunol. 10, 2458. doi:10.3389/FIMMU.2019.02458
Scharer, C. D., Blalock, E. L., Mi, T., Barwick, B. G., Jenks, S. A., Deguchi, T., et al. (2019). Epigenetic programming underpins B cell dysfunction in human SLE. Nat. Immunol. 20, 1071–1082. doi:10.1038/S41590-019-0419-9
Schmitz, R., Wright, G. W., Huang, D. W., Johnson, C. A., Phelan, J. D., Wang, J. Q., et al. (2018). Genetics and pathogenesis of diffuse large B-cell lymphoma. N. Engl. J. Med. 378, 1396–1407. doi:10.1056/NEJMOA1801445
Shiraz, A. K., Panther, E. J., and Reilly, C. M. (2022). Altered germinal-center metabolism in B cells in autoimmunity. Metabolites 12, 40. doi:10.3390/METABO12010040
Tellier, J., Shi, W., Minnich, M., Liao, Y., Crawford, S., Smyth, G. K., et al. (2016). Blimp-1 controls plasma cell function through the regulation of immunoglobulin secretion and the unfolded protein response. Nat. Immunol. 17 (3), 323–330. doi:10.1038/ni.3348
Tsui, C., Martinez-Martin, N., Gaya, M., Maldonado, P., Llorian, M., Legrave, N. M., et al. (2018). Protein kinase C-β dictates B cell fate by regulating mitochondrial remodeling, metabolic reprogramming, and heme biosynthesis. Immunity 48, 1144–1159. e5. doi:10.1016/J.IMMUNI.2018.04.031
Vander Heiden, M. G., Cantley, L. C., and Thompson, C. B. (2009). Understanding the warburg effect: The metabolic requirements of cell proliferation. Science 324, 1029–1033. doi:10.1126/science.1160809
Varano, G., Raffel, S., Sormani, M., Zanardi, F., Lonardi, S., Zasada, C., et al. (2017). The B-cell receptor controls fitness of MYC-driven lymphoma cells via GSK3β inhibition. Nature 546, 302–306. doi:10.1038/NATURE22353
Victora, G. D., and Nussenzweig, M. C. (2022). Germinal centers. Annu. Rev. Immunol. 40, 413–442. doi:10.1146/ANNUREV-IMMUNOL-120419-022408
Warburg, O. (1956). On the origin of cancer cells. Science 123, 309–314. doi:10.1126/SCIENCE.123.3191.309
Waters, L. R., Ahsan, F. M., Wolf, D. M., Shirihai, O., and Teitell, M. A. (2018). Initial B cell activation induces metabolic reprogramming and mitochondrial remodeling. iScience 5, 99–109. doi:10.1016/J.ISCI.2018.07.005
Weisel, F. J., Mullett, S. J., Elsner, R. A., Menk, A. V., Trivedi, N., Luo, W., et al. (2020). Germinal center B cells selectively oxidize fatty acids for energy while conducting minimal glycolysis. Nat. Immunol. 21, 331–342. doi:10.1038/S41590-020-0598-4
Woodland, R. T., Fox, C. J., Schmidt, M. R., Hammerman, P. S., Opferman, J. T., Korsmeyer, S. J., et al. (2008). Multiple signaling pathways promote B lymphocyte stimulator dependent B-cell growth and survival. Blood 111, 750–760. doi:10.1182/BLOOD-2007-03-077222
Keywords: metabolic plasticity, B-cell activation, humoral response, glycolysis, Oxphos, anabolism
Citation: Vivas-García Y and Efeyan A (2022) The metabolic plasticity of B cells. Front. Mol. Biosci. 9:991188. doi: 10.3389/fmolb.2022.991188
Received: 12 July 2022; Accepted: 22 August 2022;
Published: 23 September 2022.
Edited by:
Francesca Sacco, University of Rome Tor Vergata, ItalyReviewed by:
Guoyu Meng, Shanghai Jiao Tong University, ChinaCopyright © 2022 Vivas-García and Efeyan. This is an open-access article distributed under the terms of the Creative Commons Attribution License (CC BY). The use, distribution or reproduction in other forums is permitted, provided the original author(s) and the copyright owner(s) are credited and that the original publication in this journal is cited, in accordance with accepted academic practice. No use, distribution or reproduction is permitted which does not comply with these terms.
*Correspondence: Alejo Efeyan, aefeyan@cnio.es