The underlying mechanisms of arenaviral entry through matriglycan
- Department of Chemical and Structural Biology, Weizmann Institute of Science, Rehovot, Israel
Matriglycan, a recently characterized linear polysaccharide, is composed of alternating xylose and glucuronic acid subunits bound to the ubiquitously expressed protein α-dystroglycan (α-DG). Pathogenic arenaviruses, like the Lassa virus (LASV), hijack this long linear polysaccharide to gain cellular entry. Until recently, it was unclear through what mechanisms LASV engages its matriglycan receptor to initiate infection. Additionally, how matriglycan is synthesized onto α-DG by the Golgi-resident glycosyltransferase LARGE1 remained enigmatic. Recent structural data for LARGE1 and for the LASV spike complex informs us about the synthesis of matriglycan as well as its usage as an entry receptor by arenaviruses. In this review, we discuss structural insights into the system of matriglycan generation and eventual recognition by pathogenic viruses. We also highlight the unique usage of matriglycan as a high-affinity host receptor compared with other polysaccharides that decorate cells.
1 Introduction
The cellular glycocalyx is a densely packed environment made of polysaccharides that extend out into the extracellular space. All human cells contain this external layer of sugars, which is critical for membrane remodeling, cellular morphology, and adherence to the extracellular matrix (ECM) (Shurer et al., 2019; Möckl, 2020). Long-chained polysaccharides such as heparan sulfate (HS), chondroitin sulfate (CS), and hyaluronan are known to decorate the cellular surface. These glycosaminoglycans (GAGs) interact with various binding partners from the ECM in addition to chemokines, growth factors, and proteases (Gandhi and Mancera, 2008). Another abundant polysaccharide that contributes to the eukaryotic glycocalyx is matriglycan. Although not containing an aminosugar and hence not considered a GAG, matriglycan is a GAG-like biopolymer that shares structural and chemical properties with other members of the glycocalyx (Sheikh et al., 2017; Hohenester, 2019). Matriglycan is uniquely present on the protein complex, dystroglycan, which is composed of an extracellular subunit, α-dystroglycan (α-DG) that interacts with ECM proteins, and a transmembrane subunit, β-dystroglycan, that binds to dystrophin via its C-terminal region (Jung et al., 1995; Endo, 2014). Dystroglycan plays a major role in the formation of the basement membrane and is essential in preserving healthy muscle function (Nickolls and Bönnemann, 2018). It connects ligands of the ECM with the actin cytoskeleton, thereby facilitating intracellular communication (Ervasti and Campbell, 1993).
In the early 1990s, the Campbell lab observed that the dystroglycan complex is highly glycosylated and interacts with laminin globular (LG) domains in the extracellular matrix (Ervasti and Campbell, 1991; Ibraghimov-Beskrovnaya et al., 1992; Ervasti and Campbell, 1993). It was later understood that α-DG displays matriglycan, a unique form of O-mannosylation (Chiba et al., 1997; Yoshida-Moriguchi and Campbell, 2015) containing the M3 core glycan (Ervasti and Campbell, 1993; Yoshida-Moriguchi et al., 2010; Yoshida-Moriguchi et al., 2013; Praissman and Wells, 2014). This extensive degree of α-DG’s glycosylation can account for over 50% of its total mass (Barresi and Campbell, 2006; Endo, 2014). Additionally, when these glycans on α-DG are not properly formed, debilitating phenotypes are known to occur, including types of congenital muscular dystrophies (CMDs) like Walker-Warburg syndrome (Longman et al., 2003; Godfrey et al., 2011). Impairments of α-DG glycosylation have also been linked to epithelium-derived cancers (de Bernabé et al., 2009; Quereda et al., 2022). Matriglycan is synthesized by the glycosyltransferase Like-acetylglucosaminyltransferase 1 (LARGE1) (Grewal et al., 2001; Barresi et al., 2004; Kanagawa et al., 2004) and is made of xylose (Xyl) and glucuronic acid (GlcA) in a long linear chain of repeating [-3GlcAβ1,3Xylα1-] heterodisaccharide subunits (Inamori and Hara, 2012; Goddeeris et al., 2013).
Matriglycan is covalently bound to two different motifs, Thr317/319 and Thr379/381, within the mucin-like domain of human α-DG (Yoshida-Moriguchi et al., 2010; Hara et al., 2011; Yang et al., 2023). Overall, more than 18 different enzymes are necessary for the proper formation of matriglycan (Beltrán et al., 2019; Hohenester, 2019; Kanagawa, 2021). The synthesis of full-length matriglycan is dependent upon LARGE1 recognition of the phosphorylation of the M3 glycan (Walimbe et al., 2020). LARGE1 must also be properly oriented through specific binding to the N-terminal domain of α-DG (Kanagawa et al., 2004; Fujimura et al., 2005; Okuma et al., 2023). Upon the sequential addition of β1,4-Xyl and β1,4-GlcA, by priming enzymes TMEM5 (Manya et al., 2016; Praissman et al., 2016) and B4GAT1 (Praissman et al., 2014; Willer et al., 2014), respectively, to the M3 core substrate, LARGE1 can initiate matriglycan synthesis (Sheikh et al., 2017; Endo, 2019).
Matriglycan plays a critical role in both cellular adhesion and differentiation (Moore and Winder, 2012; Goddeeris et al., 2013; Quereda et al., 2022), which is mediated by various laminin isoforms and other LG-domain-containing ECM proteins like agrin, perlecan, and neurexin (Hohenester, 2019). Interestingly, on top of its endogenous functions, matriglycan serves as the primary host receptor for highly pathogenic viruses. The Lassa virus (LASV), which causes the Lassa hemorrhagic fever (LHF) disease, as well as other viruses from the Arenaviridae family like the Lymphocytic choriomeningitis virus (LCMV), attach to this polysaccharide to initiate cell entry. Although α-DG itself was originally implicated to be the host receptor for the ‘Old World’ (OW) (i.e., endemic to Africa) group of arenaviruses (Cao et al., 1998), the labs of Kunz (Kunz et al., 2005) and Oxenius and Brancaccio (Imperiali et al., 2005) found that it was the glycans on α-DG that facilitate this viral-host interaction. LASV is a pathogenic virus endemic to West Africa that infects hundreds of thousands of people annually (McCormick et al., 1987; McCormick and Fisher-Hoch, 2002; Hallam et al., 2018). The virus preferentially infects and replicates within macrophages, dendritic cells, and endothelial cells (Lukashevich et al., 1999; Baize et al., 2004; Schaeffer et al., 2018). Transmission typically occurs via the excrement of the Mastomys natalensis rat (Lecompte et al., 2006), but human-to-human transmission is also well documented (Fisher-Hoch et al., 1995; Lo Iacono et al., 2015; Akhmetzhanov et al., 2019). LASV infection can be fatal (McCormick et al., 1987; Yaro et al., 2021), and survivors are often left with permanent debilitating symptoms, such as deafness (Mateer et al., 2018) and vision impairment (Li et al., 2020). There is currently no FDA-approved vaccine against LASV nor any highly effective therapeutics (Eberhardt et al., 2019; Salam et al., 2022). It is, therefore, of major interest to elucidate the mechanisms used for arenaviral infection and to develop novel therapeutics against these pathogens.
How exactly α-DG-tropic arenaviruses recognize their host receptor has, until recently, remained a mystery. A wealth of knowledge has been generated over the past few decades, but challenges in isolating and visualizing an arenaviral spike have prevented a more holistic understanding. With the resolution revolution in cryo-EM (Kühlbrandt, 2014), we now have a clearer picture of the viral lifecycle of pathogenic viruses like LASV. This review will discuss recent developments, from a structural perspective, in determining how matriglycan is generated and later hijacked by arenaviruses for cellular entry.
2 Matriglycan: an infrequent glycocalyx entity with tissue-specific binding partners
Unlike most protein-based polysaccharides in the glycocalyx, matriglycan is almost exclusively found (besides several notable exceptions) (Inamori et al., 2016; Katz et al., 2022) covalently bound to a single protein, α-DG. The length of matriglycan is heterogeneous and tissue-specific (Goddeeris et al., 2013), but it is thought to contain at least 100 disaccharide repeats that extend off the surface of α-DG by more than ∼80 nm (Briggs et al., 2016; Hohenester, 2019). There seems to be preferential binding of certain laminin isoforms, like laminin 10/11, to shorter lengths of matriglycan (McDearmon et al., 2006). The ECM binding partners of matriglycan are effectively determined by their affinity to a specific polysaccharide length, where KD values range between 1–100 nM (Goddeeris et al., 2013; Sciandra et al., 2013; Okuma et al., 2023). Thus, α-DG in particular tissues will have its preferred LG-domain containing ECM receptors.
A paralogue of LARGE1, called LARGE2 (from the GYLTL1B gene), also generates matriglycan yet has a narrower tissue distribution (Grewal et al., 2005; Ashikov et al., 2012; Inamori and Yoshida-Moriguchi, 2012). Collectively, these enzymes are expressed in a wide array of tissue types. As LARGE1 alone is thought to be sufficient for healthy tissue, LARGE2 is believed to often be dispensable, but it may function in a compensatory manner when LARGE1 is not expressed (Fujimura et al., 2005; Inamori et al., 2014). Although it has been implicated in serving a secondary role in maintaining α-DG glycosylation, recent studies have demonstrated abnormal activity of LARGE2 as a signature of colorectal cancer (Dietinger et al., 2020). Furthermore, aberrant expression of LARGE1/2, which has been detected in various types of cancers, may act to destabilize the binding between epithelial cells and the ECM (de Bernabé et al., 2009; Esser et al., 2013; Quereda et al., 2022). One notable difference between LARGE1 and its paralogue, LARGE2, is that the latter can generate matriglycan on other substrates. It was demonstrated that in α-DG−/− embryonic stem cells, other heparan sulfate proteoglycans (HSPGs), such as biglycan and syndecan-2, could be modified by LARGE2 (Inamori et al., 2016). Therefore, LARGE2 likely has a broader specificity than LARGE1 and can act upon other proteoglycans rather than only on α-DG.
Matriglycan chain termination is carried out by the sulfotransferase HNK-1 through the addition of a sulfate group to the final GlcA residue (Sheikh et al., 2020). This terminal sulfate effectively eliminates the binding substrate of LARGE1/2 to prevent further matriglycan synthesis. Levels of HNK-1 expression are also highly heterogeneous and tissue-based. Thus, LARGE1, LARGE2, and HNK-1 likely function in parallel to generate specific matriglycan lengths that arise from the variable expression levels of these enzymes in each tissue. For instance, HNK-1 transcript abundance was found to be significantly higher in brain tissue, when compared to LARGE1/2, resulting in shorter matriglycan on α-DG (Goddeeris et al., 2013; Sheikh et al., 2020).
2.1 Mechanistic insights into LARGE1 synthesis of matriglycan
LARGE1 is a bifunctional enzyme that is localized in the Golgi (Grewal et al., 2005; Percival and Froehner, 2007). This enzyme contains a cytoplasmic/transmembrane region, a coiled-coil (stem) domain, a xylosyltransferase (Xyl-T) domain, a linker region, and a glucuronyltransferase (GlcA-T) domain (Figure 1). The ectodomain structure of LARGE1 (residues 29–756) (Peyrard et al., 1999) was recently solved (Joseph et al., 2022; Katz and Diskin, 2022), providing insights into how matriglycan is synthesized. This enzyme exists as a homodimer, with two copies of each catalytic domain. Both catalytic domains consist of a canonical GT-A fold that contains an α/β/α sandwich, similar to a Rossman fold for nucleotide-binding proteins (Harrus et al., 2018; Taujale et al., 2020; Joseph et al., 2022; Katz and Diskin, 2022). Furthermore, the catalytic domains both contain a canonical ‘DXD’ motif, which forms part of each active site. This motif coordinates a divalent cation that interacts with the phosphate groups of the UDP-sugar donor (Breton et al., 2006; Taujale et al., 2020). The DXD motif of each domain straddles between the major and minor β-sheets where it engages with incoming monosaccharide-UDP substrates. The Xyl-T domain, a retaining transferase member of the GT 8 family (Drula et al., 2022), contains a DXD motif with a bound Mn2+ ion that facilitates the addition of a Xyl residue to the growing matriglycan chain (Katz and Diskin, 2022). The GlcA-T domain, an inverting transferase member of the GT 49 family (Drula et al., 2022), is predicted to coordinate a Mn2+ ion (or another divalent ion) in its DXD domain.
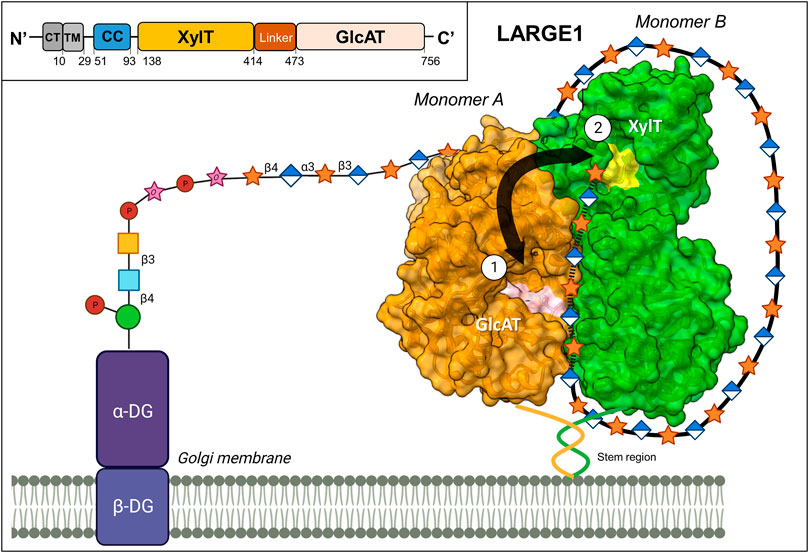
FIGURE 1. Proposed mechanism for matriglycan synthesis. LARGE1 is a homodimeric enzyme that synthesizes matriglycan in a processive manner on α-DG (PDB 7ZVJ). The growing polysaccharide chain alternates between the xylosyltransferase (XylT) domain on one monomer (shown in green), and the glucuronyltransferase (GlcAT) domain on the second monomer (shown in orange). The ‘DXD’ motifs, which comprise part of the catalytic sites, are colored in yellow and pink for the XylT and GlcAT domains, respectively. The matriglycan chain likely wraps around the perimeter of the enzyme, adhering to positively charged patches on the protein’s surface, aiding the enzyme’s processivity. Inset: A schematic diagram of the domain organization of LARGE1, CT: Cytoplasmic, TM: Transmembrane, CC: Coiled Coil. This image was partially created by BioRender.com.
LARGE1 is organized in a parallel dimer. In this form, two LARGE1 monomers are associated such that each catalytic domain interacts with its cognate domain on the second monomer (Figure 1). Oligomerization in a parallel-dimeric form was found to be dependent upon the presence of the coiled-coil (CC) domain (Katz and Diskin, 2022). On each monomer, the two catalytic pockets face in opposite directions. Therefore, the closest distance between the two types of active sites is ∼40 Å, coming from catalytic domains on adjacent monomers. To access the two types of catalytic domains on the same monomer, the matriglycan chain would need to loop around to the opposite face of the enzyme after each subunit addition. Thus, the most efficient method for matriglycan generation is to use one domain contributed from each monomer in an alternating manner. Interestingly, several positively charged patches exist along the perimeter of the enzyme. These areas likely facilitate the growing (negatively charged) matriglycan to wrap around LARGE1 while having its non-reducing end stay near the two active sites (Katz and Diskin, 2022). Furthermore, LARGE1 synthesizes matriglycan processively, rather than distributively, through the use of these adjacent catalytic domains (Figure 1). The resulting matriglycan is generated in discrete lengths, which may be dictated by the inherent length of the enzyme-substrate complex (Joseph et al., 2022).
2.2 Architecture of the Lassa virus spike complex and mechanism for matriglycan recognition
Arenaviruses are equipped with trimeric class-I spike complexes (Burns and Buchmeier, 1993; Burri and da Palma, 2012). Such spikes are translated as single polypeptide chains that trimerize and require maturation through proteolytic cleavage by cellular proteases to become active (Buchmeier and Parekh, 1987; Lenz et al., 2000; Schlie et al., 2010). The cleavage yields N-terminal receptor binding domains, termed glycoprotein-1 (GP1), C-terminal membrane fusion domains, termed glycoprotein-2 (GP2), and (unique to arenaviruses) a structured signal peptide (SSP) (Eichler et al., 2003; Burri and da Palma, 2012). For many years, it was unclear how arenaviruses like LASV utilize their spike complexes to bind the matriglycan receptor. The mechanism of matriglycan binding was recently revealed through the determination of the cryo-EM structure of a complete, trimeric LASV spike complex (Katz et al., 2022). The receptor binding site (RBS), where matriglycan binds, is quaternary and forms through the shared interactions between each of the spike’s three monomers at the apex of the spike (Figure 2A). At the heart of the binding site are the three C-terminal regions of the GP1 domains from each adjacent monomer, which undergo mutual domain-swapping with a neighboring subunit. This interlocked domain-swapping phenomenon provides structural stability and generates a surface-exposed cleft where matriglycan binds. Key residues that engage with matriglycan are contributed from the recognition sequence of the subtilisin kexin isozyme-1/site-1 (SKI-1/S1P) protease (i.e., an “RRLL” sequence (Lenz et al., 2000)) that cleaves between the GP1 and GP2 subunits (Figure 2D). Although the general vicinity of the RBS was previously known through mutational analyses (Burri et al., 2013; Hastie et al., 2016; Acciani et al., 2017; Pennington and Lee, 2022), the function of the SKI-1/S1P recognition sequence for receptor binding (Katz et al., 2022) was unknown. This discovery helps to explain the exclusive use of the SKI-1/S1P protease by arenaviruses over more common cellular proteases, like furin, which is also considered to be a virulence-increasing factor in other viruses (Basak et al., 2001; Johnson et al., 2021).
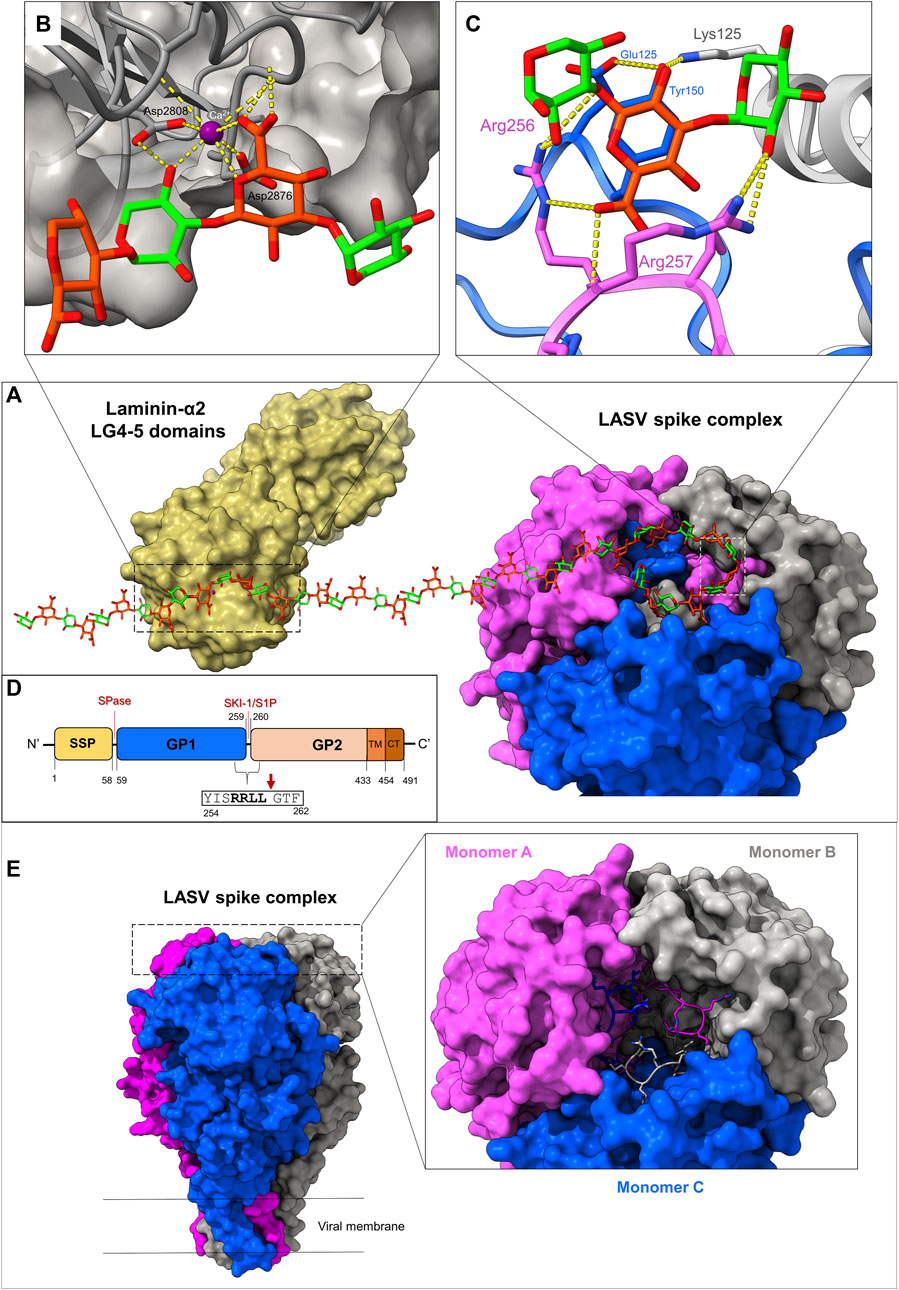
FIGURE 2. Mechanisms of matriglycan recognition by the LASV spike complex and LG4,5-domain-containing proteins. (A) Surface representations of the LG domains 4–5 of laminin α-2 chain, in complex with matriglycan (PDB 5IK5) alongside the Lassa spike complex, bound to matriglycan (PDB 7PVD). (B) The CA2+-dependent mechanism of the LG4-5 domain recognition of matriglycan, which chiefly relies on metal coordination. (C) The mechanism of LASV spike complex recognition of matriglycan, which depends on residues of a domain-swapped SKI-1/S1P site. This interaction is dominated by hydrogen bonds and electrostatic interactions. (D) A schematic diagram for the domain organization of the LASV spike complex. SSP: structured signal peptide, GP1: Glycoprotein-1 subunit, GP2: glycoprotein-2 subunit, TM: Transmembrane domain, CT: Cytoplasmic domain. The signal peptidase-1 (SPase) and SKI-1/S1P cleavage sites are indicated. The specific cleavage site by SKI-1/S1P is shown with a red arrow directly after the “RRLL” motif. Note that the use of a single matriglycan chain between the structures, along with only a partial description of key residues used for binding, was carried out for artistic purposes. (E) Stabilization of the LASV spike complex through domain swapping of the terminal GP1 regions (PDB: 7PUY). Monomers (A–C) of the trimeric spike complex are shown in pink, gray, and blue, respectively. The final ∼10 residues of GP1, which include the SKI-1/S1P recognition motif, are shown in stick representation. These segments are interlocked with a neighboring monomer through hydrophobic interactions, while exposing critical residues for matriglycan binding.
Three equivalent matriglycan binding sites form on the apex of the spike. Since the RBS is generated through the interaction of the GP1 termini with neighboring monomers, it indicates that LASV cannot bind its host receptor unless the spike is fully matured (i.e., cleaved by SKI-1/S1P), rationalizing previous observations that soluble GP1 is alone unable to bind matriglycan (Jae et al., 2014; Cohen-Dvashi et al., 2015; Hastie et al., 2016; Acciani et al., 2017). Each binding site can accommodate two disaccharide repeats through the combined interactions of several key residues: Arg256, Arg257, and Leu258 (of the SKI-1/S1P site) from an “A”-monomer, and Tyr150 from a “B”-monomer (Figure 2C). In addition, GlcA subunits within matriglycan act as N-caps for short ⍺-helices on each GP1 that start with Leu120 and Ser121. Other important residues include Lys125 from an adjacent ‘C’-monomer that plays a critical role in positioning Tyr150 such that it can interact with a GlcA monosaccharide. Of these residues, Arg257 seems to be the most critical for matriglycan binding, as it interacts with both a GlcA and a Xyl residue, by making two hydrogen bonds through its guanidinium group and one via its main chain amine. Tyr150, which was recognized as an important residue for binding by genetic analyses (Hastie et al., 2016; Acciani et al., 2017), interacts directly with matriglycan and further stabilizes Arg257 through its main-chain carbonyl (Katz et al., 2022). Arg256 binds an entire disaccharide subunit and makes two hydrogen bonds with hydroxyl groups on matriglycan. The receptor binding site is preconfigured for binding through a series of other residues within GP1 that make secondary interactions. The bound matriglycan chain is approximately 40 Å in length and comprises 2,113 Å2 total buried surface area (903 Å2 within the RBS and 1,210 Å2 within matriglycan) (Katz et al., 2022). Interestingly, despite extensive N-linked glycosylation on the LASV spike (Watanabe et al., 2018), these glycans are not believed to play a role in matriglycan binding. Nonetheless, one particular N-linked glycan, Asn119, is highly conserved and may protect against immune detection of critical residues used for matriglycan binding (Sommerstein et al., 2015; Borenstein-Katz et al., 2019).
The molecular mechanism that LASV uses to bind matriglycan differs significantly from that of laminin G-like domains (Figure 2B). LG domains utilize a Ca2+ ion coordinated by two acidic side chains and main chain carbonyl groups to engage with matriglycan that completes an octahedral coordination geometry (Briggs et al., 2016). Additional residues of LG domains are involved in matriglycan binding, contributing to either direct or water-mediated polar interactions. The high affinity of LG domains to matriglycan may be due to the protein not requiring conformational changes for binding, reducing the binding’s entropic cost. Ligand binding, for both the LASV spike and LG domains, is facilitated by matriglycan being bound in a low-energy conformation with intramolecular hydrogen bonding within the polysaccharide chain. However, unlike LG domains, LASV does not engage in ion coordination and instead relies on a network of hydrogen bonding via positively charged residues in its SKI-1/S1P motif. The matriglycan binding interface of an LG domain is also much smaller, as only one Xyl-GlcA disaccharide repeat is bound, whereas LASV can fit at least five disaccharide subunits within the apex of its spike (Briggs et al., 2016; Katz et al., 2022).
2.3 The role of the SKI-1/S1P cleavage site in receptor recognition and the spike’s stability
Since the 1980s, it has been understood that arenaviral spikes require proteolytic maturation by the SKI-1/S1P protease for spike activation (Buchmeier et al., 1987) although SKI-1/S1P is not a common protease in the viral world (Lenz et al., 2000; Eichler et al., 2003). Besides arenaviruses, the use of the SKI-1/S1P protease has only been found in one other viral family, the Nairoviridae (which, like the Arenaviridae, is also in the Bunyavirales order) (Hulswit et al., 2021), where it activates the Gn envelope proteins of viruses like the Crimean-Congo hemorrhagic fever virus (CCHFV) (Vincent et al., 2003; Sanchez et al., 2006; Bost et al., 2023). In the absence of a functional SKI-1/S1P recognition motif, virions expressing the LCMV or the LASV spikes are no longer infectious (Lenz et al., 2001; Beyer et al., 2003). Even though arenaviruses can use other cellular receptors besides matriglycan, and thus do not necessarily need the SKI-1/S1P recognition motif for generating the receptor binding site, it seems that all human-infecting arenaviruses nevertheless contain a SKI-1/S1P proteolytic motif (Spiropoulou et al., 2002; Rojek and Lee, 2008; Pasquato et al., 2018). The Machupo virus (MACV), for instance, is a member of the New World (NW) arenaviruses (Charrel et al., 2002), which are primarily endemic to South America. MACV uses transferrin receptor 1 (TfR1) rather than matriglycan for cellular entry (Radoshitzky et al., 2007) in a mechanism that does not rely on the SKI-1/S1P recognition motif (Abraham et al., 2010; Diskin, 2023), but it preserves the SKI-1/S1P site for activation (Rojek and Lee, 2008). These observations suggest additional functions for the SKI-1/S1P recognition motif on top of forming the matriglycan binding site.
A possible hint for the additional functions of the SKI-1/S1P sites in arenaviral spike complexes comes from the extensive work leading up to obtaining structural information for the full-length LASV spike. Besides structural data for bits and pieces of these spikes (Bowden et al., 2009; Abraham et al., 2010; Igonet et al., 2011; Briknarová, 2012; Cohen-Dvashi et al., 2015; Hastie et al., 2016; Israeli et al., 2017; Shimon et al., 2017; Shulman et al., 2019; Zhang et al., 2019), low-resolution structural data for a complete spike first became available by EM subtomogram averaging from actual virions of the LASV, indicating its trimeric nature (Li et al., 2016). Later studies of the spike’s ectodomain required stapling antibodies to discern its architecture (Hastie et al., 2017) due to an apparent instability of these ectodomain constructs. However, an inherent stability of the spikes is a prerequisite for infectious particles that are transmitted through aerosol, as the spikes need to withstand the harsh external conditions required for transmission (Stephenson et al., 1984; Kenyon et al., 1992). Indeed, the WT full-length spike was found to be stable and hence suitable for structural studies (Katz et al., 2022). The cryo-EM structure of the LASV spike indicates that this stabilization originates from a two-sided interlocking of the protein complex (Katz et al., 2022). Inside the membrane, the GP2 helices are twisted around each other in a conformation that is locked by the SSP subunit, forming a stable six-helical bundle. At the apex of the spike, the SKI-1/S1P recognition motifs, contributed from each GP1 domain, interlocks the spike and stabilizes its trimeric form (Figure 2E). This additional identified role of the SKI-1/S1P recognition motif establishes a direct link between the spike’s stability and its ability to bind matriglycan and provides a possible explanation for its usage by non-matriglycan-tropic arenaviruses.
Interestingly, while all arenaviruses preserve the use of SKI-1/S1P for cleavage, sequence variations in the recognition motif do exist, and these variations may affect the maturation of newly formed spike complexes. SKI-1/S1P cleaves after hydrophobic residues in a general (K/R)-X-Xh-Xh motif (Seidah and Prat, 2007). The ‘P4’ site in arenaviruses has an invariant arginine residue, but the other positions do vary, mostly within the NW arenaviruses (Pasquato et al., 2011; Burri et al., 2013). The OW arenaviruses are relatively more conserved, composed of either “RRLL”, “RRLA”, or “RRLM” motifs (Burri et al., 2013). The “RRLL” motif is the consensus sequence of LASV strains (Lenz et al., 2000), and the mutation to “RRLA” in LASV prevents proteolytic processing (Burri and Pasqual, 2012). Although the evolutionary benefit of SKI-1/S1P sequence variation is unclear, a particular SKI-1/S1P sequence can dictate the intracellular compartments for post-translational processing (Burri et al., 2013). Noteworthy is that the LASV spike is cleaved in the ER/cis-Golgi, whereas the LCMV spike is cleaved in the late-Golgi (Beyer et al., 2003; Burri and Pasqual, 2012). When the SKI-1/S1P motif of LASV (‘RRLL’) is moved to LCMV’s spike, this mutant spike is then translocated to the ER/cis-Gogli as well (Burri and Pasqual, 2012; Oppliger and da Palma, 2016). Interestingly, the ‘RRLL’ motif of LASV mimics the SKI-1/S1P autoproteolysis site C and is the only known arenaviral motif that is identical to a cellular substrate (Pasquato et al., 2011; Burri and Pasqual, 2012). Most host substrates that are cleaved by SK1-1/S1P undergo processing in the medial Golgi, a distinct intracellular location relative to all known viral substrates. The alternative compartments for arenaviral processing may act to isolate viral spike maturation and thus prevent interruption of the processing of essential host proteins (Burri and Pasqual, 2012).
2.4 The lassa virus has high specificity for matriglycan
Many OW arenaviruses, and even several non-pathogenic NW arenaviruses, utilize matriglycan for cellular entry (Cao et al., 1998; Spiropoulou et al., 2002; Kunz et al., 2005; Rojek and Kunz, 2008). Virions of LASV and some LCMV strains engage matriglycan with high affinity, having reported half-maximal binding values of <10 nM (Cao et al., 1998; Kunz et al., 2004; Imperiali et al., 2005; Kunz et al., 2005; Kunz et al., 2005; Rojek and Sanchez, 2008). The binding strength between LASV and matriglycan likely stems from the extensive hydrogen bonding network that each spike forms with matriglycan and a capacity to bind several polysaccharide chains simultaneously over multiple spikes in the context of the complete virion. In addition, the C3-symmetry of each spike creates numerous degenerate binding states to the repeating linear matriglycan, which collectively decrease the entropic cost of binding (Lortat-Jacob et al., 2001; Erlendsson and Teilum, 2021; Katz et al., 2022). Kinetic data, alongside competition assays (Kunz et al., 2001; Rambukkana et al., 2003; Kunz et al., 2005), suggest that LASV (and some LCMV) virions have a matriglycan affinity on the same order of magnitude as many ECM proteins, inferring that arenaviruses can compete for free matriglycan in the extracellular environment. Matriglycan is relatively uncommon within the sea of glycans that surround the cellular membrane. (Pries et al., 2000; Reitsma et al., 2007; Gao and Lipowsky, 2010). Nonetheless, LASV has obtained specificity towards its polysaccharide receptor (Kunz et al., 2005; Rojek and Sanchez, 2008). Despite the similarities in structure, LASV and “high affinity” LCMV strains show minimal or no inhibition by soluble heparin (HP) and HS during cellular entry. LASV is seemingly unperturbed when infecting a cell line lacking HS- and chondroitin-containing proteoglycans in the glycocalyx (Rojek et al., 2007; Fedeli et al., 2018). Nonetheless, switching from matriglycan to other GAGs for cell entry is possible for some arenaviruses.
Unlike LASV, which exclusively utilizes matriglycan as a primary host receptor, some LCMV strains are able to use an alternative host receptor. This capability alters disease phenotypes and only requires minute changes in the spike’s sequence (Southern and Bishop, 1987; Suprunenko and Hofer, 2019). Specifically, while LCMV strains like cl-13 have high specificity for matriglycan (Kunz et al., 2004; Kunz et al., 2005), three mutations within the GP1 subunit of the LCMV spike complex are each independently sufficient for the virus to gain access to HS for cellular entry: Y155H (Hastie et al., 2016), S153F (Teng et al., 1996), L260F (Sevilla et al., 2000; Volland et al., 2021). Furthermore, these single-point mutations alter tissue tropism, with HS-tropic LCMV strains often being non-immunosuppressive and substantially less pathogenic (Salvato et al., 1991; Sevilla et al., 2000; Smelt et al., 2001). What is the evolutionary benefit of these LCMV strains that can enter cells via HSPGs, but do not generate disease? The gain of HSPG-mediated cell entry does allow for increased tropism for cells that have negligible matriglycan expression. Despite the ability of these LCMV strains to utilize another receptor, many of these variants have still maintained the capability to bind matriglycan, and some of them only utilize HS in the absence of α-DG (Kunz et al., 2004). Although LCMV receptor choice is not a direct predictor of disease severity, as there are exceptions like the highly pathogenic strain, WE2.2, it plays a critical role in determining the infected cell types and subsequent immune response (Teng et al., 1996; Kunz et al., 2004; Plume et al., 2019).
2.5 Viral entry through the use of GAGs and other glycans
High specificity binding to matriglycan by arenaviruses is not the only case for specific usage of linear glycans by viruses. While some viruses utilize GAGs as low-affinity attachment factors during cell entry (Xu and Esko, 2014), other viruses can generate high-affinity and tissue-specific interactions by taking advantage of the heterogeneous degree of N-sulfation, O-sulfation, and N-acetylation in the glycocalyx (Gallagher and Walker, 1985; Casu and Lindahl, 2001; Spillmann, 2001; Kobayashi et al., 2012). A subset of pathogenic viruses that engage HSPGs, like the Herpes Simplex Virus-1 (HSV-1) (Herold et al., 1995), rabies lyssavirus (Sasaki et al., 2018), and Dengue virus (DENV) (Chen et al., 1997), have a specific preference for the type/degree of HS sulfation (Xu and Esko, 2014). This specificity can result in high affinity, characterized by KD values as high as 15 nM for DENV/HS (Chen et al., 1997), for example. Subsequently, viruses such as HSV-1 (O’Donnell et al., 2006; O’Donnell and Shukla, 2008; Madavaraju et al., 2020; Koganti et al., 2021), AAV-2 (Summerford and Samulski, 1998; Qiu et al., 2000), AcMNPV (Makkonen et al., 2013), and potentially DENV (Chen et al., 1997; Hilgard and Stockert, 2000; Dalrymple and Mackow, 2011; Cruz-Oliveira et al., 2015) may utilize GAGs alone for cell entry.
Noteworthy, besides long-linear polysaccharides, other types of cell-surface glycans could be used by viruses for cell entry. A known example is the influenza virus which uses α2-6-linked sialic acids for cellular entry via the hemagglutinin spikes on its viral surface. The affinity between one hemagglutinin spike to sialic acid is very low (0.5–5 mM) (Sauter et al., 1989; Gamblin and Skehel, 2010; Liu et al., 2022). However, influenza is equipped with 900–1,200 hemagglutinin trimers per virion, enabling numerous interactions with host sialic acids, resulting in a 104–106-fold increase in avidity (Kosik et al., 2018; Kosik and Yewdell, 2019). The sialic acid–binding adenovirus 37 (Ad37), for instance, is believed to increase its avidity 250-fold when it engages with a second sialic acid via a bivalent interaction (Nilsson et al., 2011). Thus, multivalent binding, which may act cooperatively, is used by many viruses to achieve high avidity interactions with their host receptors.
2.6 Cellular internalization via matriglycan
Recognition of the target cell via binding to the cell-surface receptor is a critical step for viral infection. However, merely attaching to the host cell is not sufficient, and viruses also need to penetrate cells either on the plasma membrane or alternatively inside some internal compartments following internalization. The usage of specific host receptors by viruses can alternate their cellular internalization/signaling pathways, as is the case for the human echovirus 1 with α2β1 integrin receptor (Jokinen et al., 2010; Boulant et al., 2015; Hussein et al., 2015; Salmela et al., 2017). Furthermore, other pathogens, like the poliovirus (Brandenburg et al., 2007; Coyne et al., 2007; Kalia and Jameel, 2011), modify their internalization strategy depending on the tissue being infected. In this regard, binding to matriglycan can facilitate two different pathways for internalization; LG domain-containing ECM proteins, like laminin-111, rely on a dynamin-dependent endocytic (pinocytotic) pathway for internalization (Leonoudakis et al., 2014) whereas α-DG tropic arenaviruses enter their host cell via the dynamin-independent process of macropinocytosis (Goncalves et al., 2013; Oppliger and Torriani, 2016). Numerous other viruses use macropinocytosis for cellular entry, such as HIV-1 (Liu et al., 2002), Vaccinia (Mercer and Helenius, 2008), and the Ebola virus (Mulherkar et al., 2011; Mercer and Helenius, 2012; Kuroda et al., 2020). Internalization via macropinocytosis is likely a form of apoptotic mimicry, in which cells detect phosphatidylserine (PS) on viral envelopes through PS-receptors, such as Axl, and Tyro3 (Shimojima et al., 2012; Shimojima and Kawaoka, 2012), that subsequently activate intracellular signaling cascades (Soares et al., 2008; Amara and Mercer, 2015). As macropinocytosis is used by the cell for the removal of apoptotic bodies, this pathway could be an effective way for viruses to evade immune detection, as it triggers intracellular pathways that modulate proinflammatory and anti-inflammatory cytokines (Mercer and Helenius, 2009; Amara and Mercer, 2015). For successful internalization, the virion needs to be in close proximity to the cell surface. How LASV or other matriglycan-tropic viruses ensure such proximity is not yet clear. An interesting possibility is that these viruses may slide on matriglycan toward the cellular membrane (i.e., viral surfing) (Katz et al., 2022); whether or not this is the mechanism remains an open question.
2.7 Balancing cellular entry and viral egress
Given the abundance of a glycan receptor and the multiple spikes each virion has, the binding of the virion will have high avidity, potentially immobilizing viruses within the glycocalyx during cell exit. Some viruses, like HSV-1, overcome this issue by upregulating heparanase to the cellular surface to degrade heparan sulfate, and thus facilitating viral egress (Hadigal et al., 2015; Agelidis et al., 2017; Thakkar et al., 2017; Hadigal et al., 2020). Influenza, on the other hand, achieves viral egress by using neuraminidase to degrade sialic acids in the cellular periphery. Thus, the influenza virus requires a properly “tuned” hemagglutinin:neuraminidase ratio, ranging from 200:1 to 50:1 (dependent on the viral serotype and target tissue), in order to successfully escape the cell (Air and Laver, 1989; Harris et al., 2006; Xu et al., 2012; Gaymard et al., 2016). By contrast, LASV uses an entirely different mechanism for improving the likelihood of viral egress. Once new virions are formed within a host cell, they are equipped with a small fragment of matriglycan, which is bound within its spike complex (Katz et al., 2022). This “placeholder” would prevent LASV from binding with endogenous α-DG-bound matriglycan in the glycocalyx and re-infecting its host cell. Once the virion has successfully escaped, via budding (Murphy and Whitfield, 1975), to the extracellular environment (where free matriglycan is lacking), this receptor fragment is expected to dissociate so the virion can engage with another cell. An interesting open question is from where this piece of matriglycan-placeholder originates. This fragment may be synthesized by LARGE2 or the product of another yet-to-be-discovered cellular process.
3 Conclusion
The use of long-chained GAGs has historically been viewed as a general viral strategy to adhere to the host membrane through low affinity/non-specific interactions. Only recently has it become clear the complex role of these GAG interactions and the potential use of long-chained glycans as stand-alone host receptors. The Lassa virus engages in high-affinity interactions with its specific glycan receptor, matriglycan. Since the discovery that arenaviruses contain the rare SKI-1/S1P site within their viral spike complex, the preference for this particular proteolytic motif, as opposed to furin, has remained unclear. From the recent structure of the full-length native LASV spike complex, it is now understood that many OW arenaviruses contain this rare SKI-1/S1P motif because it serves the additional purpose of forming the matriglycan binding site. The very high degree of conservation of the SKI-1/S1P site among OW arenaviruses further validates the importance of this motif for viral entry (Burri et al., 2013; Katz et al., 2022). The additional role of the SKI-1/S1P sequence motif in stabilizing the trimeric form of the LASV spike complex may explain why all known human-infecting arenaviruses contain this site, while not all members of this viral family bind matriglycan. Understanding the structural basis for matriglycan recognition by LASV, and other arenaviruses, further paves the way to the design of new inhibitory molecules that will compete for the receptor binding site and thereby prevent viral attachment and entry. While we now have a better view of how pathogenic arenaviruses exploit matriglycan, many open questions remain regarding subsequent steps in the infection process.
4 Scope statement
A central attribute of the dystroglycan axis is matriglycan, the unique post-translational modification of α-dystroglycan. In this minireview, we discuss recent structural insights for the synthesis of matriglycan and the role of this polymeric sugar as a cell surface receptor for pathogenic viruses from the Arenaviridae family. Understanding how viruses, like the Lassa virus, recognize matriglycan provides invaluable data on the molecular mechanisms behind the infection process. The recently obtained structures of LARGE1, the enzyme responsible for matriglycan synthesis, and of the Lassa virus’s spike complex, bound to matriglycan, show how this polysaccharide is both generated and later hijacked by pathogens. Highlighting the unique role that the dystroglycan axis plays in infectious diseases is particularly relevant and would make an important contribution to this research topic.
Author contributions
MK: Visualization, Writing–original draft. RD: Supervision, Writing–review and editing.
Funding
The author(s) declare that financial support was received for the research, authorship, and/or publication of this article. The RD lab is supported by research grants from the Pasteur-Weizmann Council and from the Institut Pasteur and the Weizmann Institute of Science, the Ernst I Ascher Foundation, Ben B. and Joyce E. Eisenberg Foundation, Estate of Emile Mimran, Jeanne and Joseph Nissim Center for Life Sciences Research, Donald Rivin, Stanley and Tanya Rossby Endowment Fund, as well as from the Israel Science Foundation (grant No. 209/20).
Acknowledgments
We thank Matthew Rosenthal and Ariel Tennenhouse for commenting on this review.
Conflict of interest
The authors declare that the research was conducted in the absence of any commercial or financial relationships that could be construed as a potential conflict of interest.
Publisher’s note
All claims expressed in this article are solely those of the authors and do not necessarily represent those of their affiliated organizations, or those of the publisher, the editors and the reviewers. Any product that may be evaluated in this article, or claim that may be made by its manufacturer, is not guaranteed or endorsed by the publisher.
References
Abraham, J., Corbett, K. D., Farzan, M., Choe, H., and Harrison, S. C. (2010). Structural basis for receptor recognition by New World hemorrhagic fever arenaviruses. Nat. Struct. Mol. Biol. 17 (4), 438–444. doi:10.1038/nsmb.1772
Acciani, M., Alston, J. T., Zhao, G., Reynolds, H., Ali, A. M., Xu, B., et al. (2017). Mutational analysis of lassa virus glycoprotein highlights regions required for alpha-dystroglycan utilization. J. virology 91 (18), e00574-17. doi:10.1128/JVI.00574-17
Agelidis, A. M., Hadigal, S. R., Jaishankar, D., and Shukla, D. (2017). Viral activation of heparanase drives pathogenesis of herpes simplex virus-1. Cell Rep. 20 (2), 439–450. doi:10.1016/j.celrep.2017.06.041
Air, G. M., and Laver, W. G. (1989). The neuraminidase of influenza virus. Proteins 6 (4), 341–356. doi:10.1002/prot.340060402
Akhmetzhanov, A. R., Asai, Y., and Nishiura, H. (2019). Quantifying the seasonal drivers of transmission for Lassa fever in Nigeria. Ser. B, Biol. Sci. 374 (1775), 20180268. doi:10.1098/rstb.2018.0268
Amara, A., and Mercer, J. (2015). Viral apoptotic mimicry. Nat. Rev. Microbiol. 13 (8), 461–469. doi:10.1038/nrmicro3469
Ashikov, A., Buettner, F. F. R., Tiemann, B., Gerardy-Schahn, R., and Bakker, H. (2012). LARGE2 generates the same xylose- and glucuronic acid-containing glycan structures as LARGE. Glycobiology 23 (3), 303–309. doi:10.1093/glycob/cws153
Baize, S., Kaplon, J., Faure, C., Pannetier, D., Georges-Courbot, M. C., and Deubel, V. (2004). Lassa virus infection of human dendritic cells and macrophages is productive but fails to activate cells. J. Immunol. 172 (5), 2861–2869. doi:10.4049/jimmunol.172.5.2861
Barresi, R., and Campbell, K. P. (2006). Dystroglycan: from biosynthesis to pathogenesis of human disease. J. Cell Sci. 119 (Pt 2), 199–207. doi:10.1242/jcs.02814
Barresi, R., Michele, D. E., Kanagawa, M., Harper, H. A., Dovico, S. A., Satz, J. S., et al. (2004). LARGE can functionally bypass alpha-dystroglycan glycosylation defects in distinct congenital muscular dystrophies. Nat. Med. 10, 696–703. doi:10.1038/nm1059
Basak, A., Zhong, M., Munzer, J. S., Chrétien, M., and Seidah, N. G. (2001). Implication of the proprotein convertases furin, PC5 and PC7 in the cleavage of surface glycoproteins of Hong Kong, Ebola and respiratory syncytial viruses: a comparative analysis with fluorogenic peptides. Biochem. J. 353 (Pt 3), 537–545. doi:10.1042/0264-6021:3530537
Beltrán, D., Anderson, M. E., Bharathy, N., Settelmeyer, T. P., Svalina, M. N., Bajwa, Z., et al. (2019). Exogenous expression of the glycosyltransferase LARGE1 restores α-dystroglycan matriglycan and laminin binding in rhabdomyosarcoma. Skelet. muscle 9 (1), 11. doi:10.1186/s13395-019-0195-0
Beyer, W. R., Pöpplau, D., Garten, W., von Laer, D., and Lenz, O. (2003). Endoproteolytic processing of the lymphocytic choriomeningitis virus glycoprotein by the subtilase SKI-1/S1P. J. virology 77 (5), 2866–2872. doi:10.1128/jvi.77.5.2866-2872.2003
Borenstein-Katz, A., Shulman, A., Hamawi, H., Leitner, O., and Diskin, R. (2019). Differential antibody-based immune response against isolated GP1 receptor-binding domains from lassa and junin viruses. J. virology 93 (8), e00090-19. doi:10.1128/JVI.00090-19
Bost, C., Hartlaub, J., Pinho Dos Reis, V., Strecker, T., Seidah, N. G., Groschup, M. H., et al. (2023). The proprotein convertase SKI-1/S1P is a critical host factor for Nairobi sheep disease virus infectivity. Virus Res. 329, 199099. doi:10.1016/j.virusres.2023.199099
Boulant, S., Stanifer, M., and Lozach, P.-Y. (2015). Dynamics of virus-receptor interactions in virus binding, signaling, and endocytosis. Viruses 7 (6), 2794–2815. doi:10.3390/v7062747
Bowden, T. A., Crispin, M., Graham, S. C., Harvey, D. J., Grimes, J. M., Jones, E. Y., et al. (2009). Unusual molecular architecture of the machupo virus attachment glycoprotein. J. virology 83 (16), 8259–8265. doi:10.1128/JVI.00761-09
Brandenburg, B., Lee, L. Y., Lakadamyali, M., Rust, M. J., Zhuang, X., and Hogle, J. M. (2007). Imaging poliovirus entry in live cells. PLoS Biol. 5 (7), 1833–e213. doi:10.1371/journal.pbio.0050183
Breton, C., Snajdrová, L., Jeanneau, C., Koca, J., and Imberty, A. (2006). Structures and mechanisms of glycosyltransferases. Glycobiology 16 (2), 29R–37R. doi:10.1093/glycob/cwj016
Briggs, D. C., Yoshida-Moriguchi, T., Zheng, T., Venzke, D., Anderson, M. E., Strazzulli, A., et al. (2016). Structural basis of laminin binding to the LARGE glycans on dystroglycan. Nat. Chem. Biol. 12 (10), 810–814. doi:10.1038/nchembio.2146
Briknarová, K. (2012). “Zinc-binding domain junín virus arenavirus envelope glycoprotein,” in Encyclopedia of inorganic and bioinorganic chemistry (United States: John Wiley and Sons, Ltd).
Buchmeier, M. J., and Parekh, B. S. (1987). Protein structure and expression among arenaviruses. Curr. Top. Microbiol. Immunol. 133, 41–57. doi:10.1007/978-3-642-71683-6_4
Buchmeier, M. J., Southern, P. J., Parekh, B. S., Wooddell, M. K., and Oldstone, M. B. (1987). Site-specific antibodies define a cleavage site conserved among arenavirus GP-C glycoproteins. J. virology 61 (4), 982–985. doi:10.1128/JVI.61.4.982-985.1987
Burns, J. W., and Buchmeier, M. J. (1993). “Glycoproteins of the arenaviruses,” in The Arenaviridae. Editor M. S. Salvato (Boston, MA: Springer US), 17–35.
Burri, D. J., da Palma, J. R., Kunz, S., and Pasquato, A. (2012). Envelope glycoprotein of arenaviruses. Viruses 4 (10), 2162–2181. doi:10.3390/v4102162
Burri, D. J., da Palma, J. R., Seidah, N. G., Zanotti, G., Cendron, L., Pasquato, A., et al. (2013). Differential recognition of old world and new world arenavirus envelope glycoproteins by subtilisin kexin isozyme 1 (SKI-1)/Site 1 protease (S1P). J. virology 87 (11), 6406–6414. doi:10.1128/JVI.00072-13
Burri, D. J., Pasqual, G., Rochat, C., Seidah, N. G., Pasquato, A., and Kunz, S. (2012). Molecular characterization of the processing of arenavirus envelope glycoprotein precursors by subtilisin kexin isozyme-1/site-1 protease. J. virology 86 (9), 4935–4946. doi:10.1128/JVI.00024-12
Cao, W., Henry, M. D., Borrow, P., Yamada, H., Elder, J. H., Ravkov, E. V., et al. (1998). Identification of alpha-dystroglycan as a receptor for lymphocytic choriomeningitis virus and Lassa fever virus. Science 282, 2079–2081. doi:10.1126/science.282.5396.2079
Casu, B., and Lindahl, U. (2001). Structure and biological interactions of heparin and heparan sulfate. Adv. Carbohydr. Chem. Biochem. 57, 159–206. doi:10.1016/s0065-2318(01)57017-1
Charrel, R. N., Feldmann, H., Fulhorst, C. F., Khelifa, R., de Chesse, R., and de Lamballerie, X. (2002). Phylogeny of New World arenaviruses based on the complete coding sequences of the small genomic segment identified an evolutionary lineage produced by intrasegmental recombination. Biochem. biophysical Res. Commun. 296 (5), 1118–1124. doi:10.1016/s0006-291x(02)02053-3
Chen, Y., Maguire, T., Hileman, R. E., Fromm, J. R., Esko, J. D., Linhardt, R. J., et al. (1997). Dengue virus infectivity depends on envelope protein binding to target cell heparan sulfate. Nat. Med. 3 (8), 866–871. doi:10.1038/nm0897-866
Chiba, A., Matsumura, K., Yamada, H., Inazu, T., Shimizu, T., Kusunoki, S., et al. (1997). Structures of sialylated O-linked oligosaccharides of bovine peripheral nerve α-dystroglycan: the role of A novel O-Mannosyl-Type oligosaccharide in the binding of Α-dystroglycan with laminin*. J. Biol. Chem. 272 (4), 2156–2162. doi:10.1074/jbc.272.4.2156
Cohen-Dvashi, H., Cohen, N., Israeli, H., and Diskin, R. (2015). Molecular mechanism for LAMP1 recognition by lassa virus. J. virology 89 (15), 7584–7592. doi:10.1128/JVI.00651-15
Coyne, C. B., Kim, K. S., and Bergelson, J. M. (2007). Poliovirus entry into human brain microvascular cells requires receptor-induced activation of SHP-2. EMBO J. 26 (17), 4016–4028. doi:10.1038/sj.emboj.7601831
Cruz-Oliveira, C., Freire, J. M., Conceição, T. M., Higa, L. M., Castanho, M. A. R. B., and Da Poian, A. T. (2015). Receptors and routes of dengue virus entry into the host cells. FEMS Microbiol. Rev. 39 (2), 155–170. doi:10.1093/femsre/fuu004
Dalrymple, N., and Mackow, E. R. (2011). Productive dengue virus infection of human endothelial cells is directed by heparan sulfate-containing proteoglycan receptors. J. virology 85 (18), 9478–9485. doi:10.1128/JVI.05008-11
de Bernabé, D.B.-V., Inamori, K. I., Yoshida-Moriguchi, T., Weydert, C. J., Harper, H. A., Willer, T., et al. (2009). Loss of alpha-dystroglycan laminin binding in epithelium-derived cancers is caused by silencing of LARGE. J. Biol. Chem. 284 (17), 11279–11284. doi:10.1074/jbc.C900007200
Dietinger, V., García de Durango, C. R., Wiechmann, S., Boos, S. L., Michl, M., Neumann, J., et al. (2020). Wnt-driven LARGE2 mediates laminin-adhesive O-glycosylation in human colonic epithelial cells and colorectal cancer. Cell Commun. Signal. CCS 18 (1), 102. doi:10.1186/s12964-020-00561-6
Diskin, R. (2023). A structural perspective on the evolution of viral/cellular macromolecular complexes within the arenaviridae family of viruses. Curr. Opin. Struct. Biol. 79, 102561. doi:10.1016/j.sbi.2023.102561
Drula, E., Garron, M. L., Dogan, S., Lombard, V., Henrissat, B., and Terrapon, N. (2022). The carbohydrate-active enzyme database: functions and literature. Nucleic acids Res. 50 (D1), D571–D577. doi:10.1093/nar/gkab1045
Eberhardt, K. A., Mischlinger, J., Jordan, S., Groger, M., Günther, S., and Ramharter, M. (2019). Ribavirin for the treatment of Lassa fever: a systematic review and meta-analysis. Int. J. Infect. Dis. IJID 87, 15–20. doi:10.1016/j.ijid.2019.07.015
Eichler, R., Lenz, O., Strecker, T., Eickmann, M., Klenk, H. D., and Garten, W. (2003). Identification of Lassa virus glycoprotein signal peptide as a trans-acting maturation factor. EMBO Rep. 4 (11), 1084–1088. doi:10.1038/sj.embor.embor7400002
Endo, T. (2014). Glycobiology of α-dystroglycan and muscular dystrophy. J. Biochem. 157 (1), 1–12. doi:10.1093/jb/mvu066
Endo, T. (2019). Mammalian O-mannosyl glycans: Biochemistry and glycopathology. Proc. Jpn. Acad. Ser. B, Phys. Biol. Sci. 95 (1), 39–51. doi:10.2183/pjab.95.004
Erlendsson, S., and Teilum, K. (2021). Binding revisited—avidity in cellular function and signaling. Front. Mol. Biosci. 7, 615565. doi:10.3389/fmolb.2020.615565
Ervasti, J. M., and Campbell, K. P. (1991). Membrane organization of the dystrophin-glycoprotein complex. Cell 66 (6), 1121–1131. doi:10.1016/0092-8674(91)90035-w
Ervasti, J. M., and Campbell, K. P. (1993). A role for the dystrophin-glycoprotein complex as a transmembrane linker between laminin and actin. J. Cell Biol. 122 (4), 809–823. doi:10.1083/jcb.122.4.809
Esser, A. K., Miller, M. R., Huang, Q., Meier, M. M., Beltran-Valero de Bernabé, D., Stipp, C. S., et al. (2013). Loss of LARGE2 disrupts functional glycosylation of α-dystroglycan in prostate cancer. J. Biol. Chem. 288 (4), 2132–2142. doi:10.1074/jbc.M112.432807
Fedeli, C., Torriani, G., Galan-Navarro, C., Moraz, M. L., Moreno, H., Gerold, G., et al. (2018). Axl can serve as entry factor for lassa virus depending on the functional glycosylation of dystroglycan. J. virology 92 (5), e01613-17. doi:10.1128/jvi.01613-17
Fisher-Hoch, S. P., Tomori, O., Nasidi, A., Perez-Oronoz, G. I., Fakile, Y., Hutwagner, L., et al. (1995). Review of cases of nosocomial Lassa fever in Nigeria: the high price of poor medical practice. BMJ 311, 857–859. doi:10.1136/bmj.311.7009.857
Fujimura, K., Sawaki, H., Sakai, T., Hiruma, T., Nakanishi, N., Sato, T., et al. (2005). LARGE2 facilitates the maturation of alpha-dystroglycan more effectively than LARGE. Biochem. biophysical Res. Commun. 329 (3), 1162–1171. doi:10.1016/j.bbrc.2005.02.082
Gallagher, J. T., and Walker, A. (1985). Molecular distinctions between heparan sulphate and heparin. Analysis of sulphation patterns indicates that heparan sulphate and heparin are separate families of N-sulphated polysaccharides. Biochem. J. 230 (3), 665–674. doi:10.1042/bj2300665
Gamblin, S. J., and Skehel, J. J. (2010). Influenza hemagglutinin and neuraminidase membrane glycoproteins. J. Biol. Chem. 285 (37), 28403–28409. doi:10.1074/jbc.R110.129809
Gandhi, N. S., and Mancera, R. L. (2008). The structure of glycosaminoglycans and their interactions with proteins. Chem. Biol. drug Des. 72 (6), 455–482. doi:10.1111/j.1747-0285.2008.00741.x
Gao, L., and Lipowsky, H. H. (2010). Composition of the endothelial glycocalyx and its relation to its thickness and diffusion of small solutes. Microvasc. Res. 80 (3), 394–401. doi:10.1016/j.mvr.2010.06.005
Gaymard, A., Le Briand, N., Frobert, E., Lina, B., and Escuret, V. (2016). Functional balance between neuraminidase and haemagglutinin in influenza viruses. official Publ. Eur. Soc. Clin. Microbiol. Infect. Dis. 22 (12), 975–983. doi:10.1016/j.cmi.2016.07.007
Goddeeris, M. M., Wu, B., Venzke, D., Yoshida-Moriguchi, T., Saito, F., Matsumura, K., et al. (2013). LARGE glycans on dystroglycan function as a tunable matrix scaffold to prevent dystrophy. Nature 503 (7474), 136–140. doi:10.1038/nature12605
Godfrey, C., Foley, A. R., Clement, E., and Muntoni, F. (2011). Dystroglycanopathies: coming into focus. Curr. Opin. Genet. Dev. 21 (3), 278–285. doi:10.1016/j.gde.2011.02.001
Goncalves, A.-R., Moraz, M. L., Pasquato, A., Helenius, A., Lozach, P. Y., and Kunz, S. (2013). Role of DC-SIGN in lassa virus entry into human dendritic cells. J. virology 87 (21), 11504–11515. doi:10.1128/JVI.01893-13
Grewal, P. K., Holzfeind, P. J., Bittner, R. E., and Hewitt, J. E. (2001). Mutant glycosyltransferase and altered glycosylation of alpha-dystroglycan in the myodystrophy mouse. Nat. Genet. 28 (2), 151–154. doi:10.1038/88865
Grewal, P. K., McLaughlan, J. M., Moore, C. J., Browning, C. A., and Hewitt, J. E. (2005). Characterization of the LARGE family of putative glycosyltransferases associated with dystroglycanopathies. Glycobiology 15 (10), 912–923. doi:10.1093/glycob/cwi094
Hadigal, S., Koganti, R., Yadavalli, T., Agelidis, A., Suryawanshi, R., and Shukla, D. (2020). Heparanase-regulated syndecan-1 shedding facilitates herpes simplex virus 1 egress. J. virology 94 (6), e01672-19. doi:10.1128/JVI.01672-19
Hadigal, S. R., Agelidis, A. M., Karasneh, G. A., Antoine, T. E., Yakoub, A. M., Ramani, V. C., et al. (2015). Heparanase is a host enzyme required for herpes simplex virus-1 release from cells. Nat. Commun. 6, 6985. doi:10.1038/ncomms7985
Hallam, H. J., Hallam, S., Rodriguez, S. E., Barrett, A. D. T., Beasley, D. W. C., Chua, A., et al. (2018). Baseline mapping of Lassa fever virology, epidemiology and vaccine research and development. NPJ vaccines 3, 11. doi:10.1038/s41541-018-0049-5
Hara, Y., Kanagawa, M., Kunz, S., Yoshida-Moriguchi, T., Satz, J. S., Kobayashi, Y. M., et al. (2011). Like-acetylglucosaminyltransferase (LARGE)-dependent modification of dystroglycan at Thr-317/319 is required for laminin binding and arenavirus infection. Proc. Natl. Acad. Sci. 108 (42), 17426–17431. doi:10.1073/pnas.1114836108
Harris, A., Cardone, G., Winkler, D. C., Heymann, J. B., Brecher, M., White, J. M., et al. (2006). Influenza virus pleiomorphy characterized by cryoelectron tomography. Proc. Natl. Acad. Sci. U. S. A. 103 (50), 19123–19127. doi:10.1073/pnas.0607614103
Harrus, D., Kellokumpu, S., and Glumoff, T. (2018). Crystal structures of eukaryote glycosyltransferases reveal biologically relevant enzyme homooligomers. Cell. Mol. life Sci. CMLS 75 (5), 833–848. doi:10.1007/s00018-017-2659-x
Hastie, K. M., Igonet, S., Sullivan, B. M., Legrand, P., Zandonatti, M. A., Robinson, J. E., et al. (2016). Crystal structure of the prefusion surface glycoprotein of the prototypic arenavirus LCMV. Nat. Struct. Mol. Biol. 23 (6), 513–521. doi:10.1038/nsmb.3210
Hastie, K. M., Zandonatti, M. A., Kleinfelter, L. M., Heinrich, M. L., Rowland, M. M., Chandran, K., et al. (2017). Structural basis for antibody-mediated neutralization of Lassa virus. Science 356 (6341), 923–928. doi:10.1126/science.aam7260
Herold, B. C., Gerber, S. I., Polonsky, T., Belval, B. J., Shaklee, P. N., and Holme, K. (1995). Identification of structural features of heparin required for inhibition of herpes simplex virus type 1 binding. Virology 206 (2), 1108–1116. doi:10.1006/viro.1995.1034
Hilgard, P., and Stockert, R. (2000). Heparan sulfate proteoglycans initiate dengue virus infection of hepatocytes. Hepatology 32 (5), 1069–1077. doi:10.1053/jhep.2000.18713
Hohenester, E. (2019). Laminin G-like domains: dystroglycan-specific lectins. Curr. Opin. Struct. Biol. 56, 56–63. doi:10.1016/j.sbi.2018.11.007
Hulswit, R. J. G., Paesen, G. C., Bowden, T. A., and Shi, X. (2021). Recent advances in bunyavirus glycoprotein research: precursor processing, receptor binding and structure. Recept. Bind. Structure’, Viruses 13 (2), 353. doi:10.3390/v13020353
Hussein, H. A. M., Walker, L. R., Abdel-Raouf, U. M., Desouky, S. A., Montasser, A. K. M., and Akula, S. M. (2015). Beyond RGD: virus interactions with integrins. Archives virology 160 (11), 2669–2681. doi:10.1007/s00705-015-2579-8
Ibraghimov-Beskrovnaya, O., Ervasti, J. M., Leveille, C. J., Slaughter, C. A., Sernett, S. W., and Campbell, K. P. (1992). Primary structure of dystrophin-associated glycoproteins linking dystrophin to the extracellular matrix. Nature 355 (6362), 696–702. doi:10.1038/355696a0
Igonet, S., Vaney, M. C., Vonrhein, C., Vonhrein, C., Bricogne, G., Stura, E. A., et al. (2011). X-ray structure of the arenavirus glycoprotein GP2 in its postfusion hairpin conformation. Proc. Natl. Acad. Sci. U. S. A. 108 (50), 19967–19972. doi:10.1073/pnas.1108910108
Imperiali, M., Thoma, C., Pavoni, E., Brancaccio, A., Callewaert, N., and Oxenius, A. (2005). O Mannosylation of alpha-dystroglycan is essential for lymphocytic choriomeningitis virus receptor function. J. virology 79 (22), 14297–14308. doi:10.1128/JVI.79.22.14297-14308.2005
Inamori, K.-I., Beedle, A. M., de Bernabé, D. B. V., Wright, M. E., and Campbell, K. P. (2016). LARGE2-dependent glycosylation confers laminin-binding ability on proteoglycans. Glycobiology 26 (12), 1284–1296. doi:10.1093/glycob/cww075
Inamori, K.-I., Hara, Y., Willer, T., Anderson, M. E., Zhu, Z., Yoshida-Moriguchi, T., et al. (2012). Xylosyl- and glucuronyltransferase functions of LARGE in α-dystroglycan modification are conserved in LARGE2. Glycobiology 23 (3), 295–302. doi:10.1093/glycob/cws152
Inamori, K.-I., Willer, T., Hara, Y., Venzke, D., Anderson, M. E., Clarke, N. F., et al. (2014). Endogenous glucuronyltransferase activity of LARGE or LARGE2 required for functional modification of α-dystroglycan in cells and tissues. J. Biol. Chem. 289 (41), 28138–28148. doi:10.1074/jbc.M114.597831
Inamori, K.-I., Yoshida-Moriguchi, T., Hara, Y., Anderson, M. E., Yu, L., and Campbell, K. P. (2012). Dystroglycan function requires xylosyl- and glucuronyltransferase activities of LARGE. Science 335 (6064), 93–96. doi:10.1126/science.1214115
Israeli, H., Cohen-Dvashi, H., Shulman, A., Shimon, A., and Diskin, R. (2017). Mapping of the Lassa virus LAMP1 binding site reveals unique determinants not shared by other old world arenaviruses. PLoS Pathog. 13 (4), e1006337. doi:10.1371/journal.ppat.1006337
Jae, L. T., Raaben, M., Herbert, A. S., Kuehne, A. I., Wirchnianski, A. S., Soh, T. K., et al. (2014). Virus entry. Lassa virus entry requires a trigger-induced receptor switch. Science 344, 1506–1510. doi:10.1126/science.1252480
Johnson, B. A., Xie, X., Bailey, A. L., Kalveram, B., Lokugamage, K. G., Muruato, A., et al. (2021). Loss of furin cleavage site attenuates SARS-CoV-2 pathogenesis. Nature 591 (7849), 293–299. doi:10.1038/s41586-021-03237-4
Jokinen, J., White, D. J., Salmela, M., Huhtala, M., Käpylä, J., Sipilä, K., et al. (2010). Molecular mechanism of alpha2beta1 integrin interaction with human echovirus 1. EMBO J. 29 (1), 196–208. doi:10.1038/emboj.2009.326
Joseph, S., Schnicker, N. J., Xu, Z., Yang, T., Hopkins, J., Watkins, M., et al. (2022). Structure and mechanism of LARGE1 matriglycan polymerase. bioRxiv [Preprint]. doi:10.1101/2022.05.12.491222
Jung, D., Yang, B., Meyer, J., Chamberlain, J. S., and Campbell, K. P. (1995). Identification and characterization of the dystrophin anchoring site on beta-dystroglycan. J. Biol. Chem. 270 (45), 27305–27310. doi:10.1074/jbc.270.45.27305
Kalia, M., and Jameel, S. (2011). Virus entry paradigms. Amino acids 41 (5), 1147–1157. doi:10.1007/s00726-009-0363-3
Kanagawa, M. (2021). Dystroglycanopathy: from elucidation of molecular and pathological mechanisms to development of treatment methods. Int. J. Mol. Sci. 22 (23), 13162. doi:10.3390/ijms222313162
Kanagawa, M., Saito, F., Kunz, S., Yoshida-Moriguchi, T., Barresi, R., Kobayashi, Y. M., et al. (2004). Molecular recognition by LARGE is essential for expression of functional dystroglycan. Cell 117 (7), 953–964. doi:10.1016/j.cell.2004.06.003
Katz, M., and Diskin, R. (2022). Structural basis for matriglycan synthesis by the LARGE1 dual glycosyltransferase. PloS one 17 (12), e0278713–e0278720. doi:10.1371/journal.pone.0278713
Katz, M., Weinstein, J., Eilon-Ashkenazy, M., Gehring, K., Cohen-Dvashi, H., Elad, N., et al. (2022). Structure and receptor recognition by the Lassa virus spike complex. Nature 603 (7899), 174–179. doi:10.1038/s41586-022-04429-2
Kenyon, R. H., McKee, K. T., Zack, P. M., Rippy, M. K., Vogel, A. P., York, C., et al. (1992). Aerosol infection of rhesus macaques with Junin virus. Intervirology 33 (1), 23–31. doi:10.1159/000150227
Kobayashi, F., Yamada, S., Taguwa, S., Kataoka, C., Naito, S., Hama, Y., et al. (2012). Specific interaction of the envelope glycoproteins E1 and E2 with liver heparan sulfate involved in the tissue tropismatic infection by hepatitis C virus. Glycoconj. J. 29 (4), 211–220. doi:10.1007/s10719-012-9388-z
Koganti, R., Memon, A., and Shukla, D. (2021). Emerging roles of heparan sulfate proteoglycans in viral pathogenesis. Seminars thrombosis hemostasis 47 (3), 283–294. doi:10.1055/s-0041-1725068
Kosik, I., Ince, W. L., Gentles, L. E., Oler, A. J., Kosikova, M., Angel, M., et al. (2018). Influenza A virus hemagglutinin glycosylation compensates for antibody escape fitness costs. PLoS Pathog. 14 (1), e1006796. doi:10.1371/journal.ppat.1006796
Kosik, I., and Yewdell, J. W. (2019). Influenza hemagglutinin and neuraminidase: yin−Yang proteins coevolving to thwart immunity. Viruses 11 (4), 346. doi:10.3390/v11040346
Kühlbrandt, W. (2014). Biochemistry. The resolution revolution. Science 343 (6178), 1443–1444. doi:10.1126/science.1251652
Kunz, S., Rojek, J. M., Kanagawa, M., Spiropoulou, C. F., Barresi, R., Campbell, K. P., et al. (2005). Posttranslational modification of alpha-dystroglycan, the cellular receptor for arenaviruses, by the glycosyltransferase LARGE is critical for virus binding. J. virology 79 (22), 14282–14296. doi:10.1128/JVI.79.22.14282-14296.2005
Kunz, S., Rojek, J. M., Perez, M., Spiropoulou, C. F., and Oldstone, M. B. A. (2005). Characterization of the interaction of lassa fever virus with its cellular receptor alpha-dystroglycan. J. virology 79, 5979–5987. doi:10.1128/jvi.79.10.5979-5987.2005
Kunz, S., Sevilla, N., McGavern, D. B., Campbell, K. P., and Oldstone, M. B. (2001). Molecular analysis of the interaction of LCMV with its cellular receptor [alpha]-dystroglycan. J. Cell Biol. 155 (2), 301–310. doi:10.1083/jcb.200104103
Kunz, S., Sevilla, N., Rojek, J. M., and Oldstone, M. B. A. (2004). Use of alternative receptors different than alpha-dystroglycan by selected isolates of lymphocytic choriomeningitis virus. Virology 325 (2), 432–445. doi:10.1016/j.virol.2004.05.009
Kuroda, M., Halfmann, P., and Kawaoka, Y. (2020). HER2-mediated enhancement of Ebola virus entry. PLoS Pathog. 16 (10), e1008900–e1008925. doi:10.1371/journal.ppat.1008900
Lecompte, E., Fichet-Calvet, E., Daffis, S., Koulémou, K., Sylla, O., Kourouma, F., et al. (2006). Mastomys natalensis and lassa fever, West Africa. Emerg. Infect. Dis. 12 (12), 1971–1974. doi:10.3201/eid1212.060812
Lenz, O., ter Meulen, J., Feldmann, H., Klenk, H. D., and Garten, W. (2000). Identification of a novel consensus sequence at the cleavage site of the lassa virus glycoprotein. J. virology 74 (23), 11418–11421. doi:10.1128/jvi.74.23.11418-11421.2000
Lenz, O., ter Meulen, J., Klenk, H. D., Seidah, N. G., and Garten, W. (2001). The Lassa virus glycoprotein precursor GP-C is proteolytically processed by subtilase SKI-1/S1P. Proc. Natl. Acad. Sci. U. S. A. 98, 12701–12705. doi:10.1073/pnas.221447598
Leonoudakis, D., Huang, G., Akhavan, A., Fata, J. E., Singh, M., Gray, J. W., et al. (2014). Endocytic trafficking of laminin is controlled by dystroglycan and is disrupted in cancers. J. Cell Sci. 127 (Pt 22), 4894–4903. doi:10.1242/jcs.152728
Li, A. L., Grant, D., Gbakie, M., Kanneh, L., Mustafa, I., Bond, N., et al. (2020). Ophthalmic manifestations and vision impairment in Lassa fever survivors. PloS one 15 (12), 02437666–e243812. doi:10.1371/journal.pone.0243766
Li, S., Sun, Z., Pryce, R., Parsy, M. L., Fehling, S. K., Schlie, K., et al. (2016). Acidic pH-induced conformations and LAMP1 binding of the lassa virus glycoprotein spike. PLoS Pathog. 12, e1005418. doi:10.1371/journal.ppat.1005418
Liu, M., Huang, L. Z. X., Smits, A. A., Büll, C., Narimatsu, Y., van Kuppeveld, F. J. M., et al. (2022). Human-type sialic acid receptors contribute to avian influenza A virus binding and entry by hetero-multivalent interactions. Nat. Commun. 13 (1), 4054. doi:10.1038/s41467-022-31840-0
Liu, N. Q., Lossinsky, A. S., Popik, W., Li, X., Gujuluva, C., Kriederman, B., et al. (2002). Human immunodeficiency virus type 1 enters brain microvascular endothelia by macropinocytosis dependent on lipid rafts and the mitogen-activated protein kinase signaling pathway. J. virology 76 (13), 6689–6700. doi:10.1128/jvi.76.13.6689-6700.2002
Lo Iacono, G., Cunningham, A. A., Fichet-Calvet, E., Garry, R. F., Grant, D. S., Khan, S. H., et al. (2015). Using modelling to disentangle the relative contributions of zoonotic and anthroponotic transmission: the case of lassa fever. PLoS neglected Trop. Dis. 9 (1), e3398. doi:10.1371/journal.pntd.0003398
Longman, C., Brockington, M., Torelli, S., Jimenez-Mallebrera, C., Kennedy, C., Khalil, N., et al. (2003). Mutations in the human LARGE gene cause MDC1D, a novel form of congenital muscular dystrophy with severe mental retardation and abnormal glycosylation of alpha-dystroglycan. Hum. Mol. Genet. 12 (21), 2853–2861. doi:10.1093/hmg/ddg307
Lortat-Jacob, H., Chouin, E., Cusack, S., and van Raaij, M. J. (2001). Kinetic analysis of adenovirus fiber binding to its receptor reveals an avidity mechanism for trimeric receptor-ligand interactions. J. Biol. Chem. 276 (12), 9009–9015. doi:10.1074/jbc.M009304200
Lukashevich, I. S., Maryankova, R., Vladyko, A. S., Nashkevich, N., Koleda, S., Djavani, M., et al. (1999). Lassa and Mopeia virus replication in human monocytes/macrophages and in endothelial cells: different effects on IL-8 and TNF-alpha gene expression. J. Med. virology 59 (4), 552–560. doi:10.1002/(sici)1096-9071(199912)59:4<552::aid-jmv21>3.0.co;2-a
Madavaraju, K., Koganti, R., Volety, I., Yadavalli, T., and Shukla, D. (2020). Herpes simplex virus cell entry mechanisms: an update. Front. Cell. Infect. Microbiol. 10, 617578. doi:10.3389/fcimb.2020.617578
Makkonen, K.-E., Turkki, P., Laakkonen, J. P., Ylä-Herttuala, S., Marjomäki, V., and Airenne, K. J. (2013). 6-o- and N-sulfated syndecan-1 promotes baculovirus binding and entry into Mammalian cells. J. virology 87 (20), 11148–11159. doi:10.1128/JVI.01919-13
Manya, H., Yamaguchi, Y., Kanagawa, M., Kobayashi, K., Tajiri, M., Akasaka-Manya, K., et al. (2016). The muscular dystrophy gene TMEM5 encodes a ribitol β1,4-xylosyltransferase required for the functional glycosylation of dystroglycan. J. Biol. Chem. 291 (47), 24618–24627. doi:10.1074/jbc.M116.751917
Mateer, E. J., Huang, C., Shehu, N. Y., and Paessler, S. (2018). Lassa fever-induced sensorineural hearing loss: a neglected public health and social burden. PLoS neglected Trop. Dis. 12 (2), e0006187. doi:10.1371/journal.pntd.0006187
McCormick, J. B., and Fisher-Hoch, S. P. (2002). “Lassa fever,” in Arenaviruses I: the epidemiology, molecular and cell biology of arenaviruses. Editor M. B. A. Oldstone (Berlin, Heidelberg: Springer Berlin Heidelberg), 75–109.
McCormick, J. B., Webb, P. A., Krebs, J. W., Johnson, K. M., and Smith, E. S. (1987). A prospective study of the epidemiology and ecology of Lassa fever. J. Infect. Dis. 155 (3), 437–444. doi:10.1093/infdis/155.3.437
McDearmon, E. L., Combs, A. C., Sekiguchi, K., Fujiwara, H., and Ervasti, J. M. (2006). Brain alpha-dystroglycan displays unique glycoepitopes and preferential binding to laminin-10/11. FEBS Lett. 580 (14), 3381–3385. doi:10.1016/j.febslet.2006.05.010
Mercer, J., and Helenius, A. (2008). Vaccinia virus uses macropinocytosis and apoptotic mimicry to enter host cells. Science 320 (5875), 531–535. doi:10.1126/science.1155164
Mercer, J., and Helenius, A. (2009). Virus entry by macropinocytosis. Nat. Cell Biol. 11 (5), 510–520. doi:10.1038/ncb0509-510
Mercer, J., and Helenius, A. (2012). Gulping rather than sipping: macropinocytosis as a way of virus entry. Curr. Opin. Microbiol. 15 (4), 490–499. doi:10.1016/j.mib.2012.05.016
Möckl, L. (2020). The emerging role of the mammalian glycocalyx in functional membrane organization and immune system regulation. Front. Cell Dev. Biol. 8, 253. doi:10.3389/fcell.2020.00253
Moore, C. J., and Winder, S. J. (2012). The inside and out of dystroglycan post-translational modification. Neuromuscul. Disord. NMD 22 (11), 959–965. doi:10.1016/j.nmd.2012.05.016
Mulherkar, N., Raaben, M., de la Torre, J. C., Whelan, S. P., and Chandran, K. (2011). The Ebola virus glycoprotein mediates entry via a non-classical dynamin-dependent macropinocytic pathway. Virology 419 (2), 72–83. doi:10.1016/j.virol.2011.08.009
Murphy, F. A., and Whitfield, S. G. (1975). Morphology and morphogenesis of arenaviruses. Bull. World Health Organ. 52 (4-6), 409–419.
Nickolls, A. R., and Bönnemann, C. G. (2018). The roles of dystroglycan in the nervous system: insights from animal models of muscular dystrophy. Dis. models Mech. 11 (12), dmm035931. doi:10.1242/dmm.035931
Nilsson, E. C., Storm, R. J., Bauer, J., Johansson, S. M. C., Lookene, A., Ångström, J., et al. (2011). The GD1a glycan is a cellular receptor for adenoviruses causing epidemic keratoconjunctivitis. Nat. Med. 17 (1), 105–109. doi:10.1038/nm.2267
O’Donnell, C. D., and Shukla, D. (2008). The importance of heparan sulfate in herpesvirus infection. Virol. Sin. 23 (6), 383–393. doi:10.1007/s12250-008-2992-1
O’Donnell, C. D., Tiwari, V., Oh, M. J., and Shukla, D. (2006). A role for heparan sulfate 3-O-sulfotransferase isoform 2 in herpes simplex virus type 1 entry and spread. Virology 346 (2), 452–459. doi:10.1016/j.virol.2005.11.003
Okuma, H., Hord, J. M., Chandel, I., Venzke, D., Anderson, M. E., Walimbe, A. S., et al. (2023). N-terminal domain on dystroglycan enables LARGE1 to extend matriglycan on α-dystroglycan and prevents muscular dystrophy. eLife 12, e82811. doi:10.7554/eLife.82811
Oppliger, J., da Palma, J. R., Burri, D. J., Bergeron, E., Khatib, A. M., Spiropoulou, C. F., et al. (2016). A molecular sensor to characterize arenavirus envelope glycoprotein cleavage by subtilisin kexin isozyme 1/site 1 protease. J. virology 90 (2), 705–714. doi:10.1128/JVI.01751-15
Oppliger, J., Torriani, G., Herrador, A., and Kunz, S. (2016). Lassa virus cell entry via dystroglycan involves an unusual pathway of macropinocytosis. J. virology 90 (14), 6412–6429. doi:10.1128/JVI.00257-16
Pasquato, A., Burri, D. J., Traba, E. G. I., Hanna-El-Daher, L., Seidah, N. G., and Kunz, S. (2011). Arenavirus envelope glycoproteins mimic autoprocessing sites of the cellular proprotein convertase subtilisin kexin isozyme-1/site-1 protease. Virology 417, 18–26. doi:10.1016/j.virol.2011.04.021
Pasquato, A., Cendron, L., and Kunz, S. (2018). Cleavage of the glycoprotein of arenaviruses. Activation Viruses by Host Proteases, 47–70. doi:10.1007/978-3-319-75474-1_3
Pennington, H. N., and Lee, J. (2022). Lassa virus glycoprotein complex review: insights into its unique fusion machinery. Biosci. Rep. 42 (2). doi:10.1042/BSR20211930
Percival, J. M., and Froehner, S. C. (2007). Golgi complex organization in skeletal muscle: a role for golgi-mediated glycosylation in muscular dystrophies? Traffic 8 (3), 184–194. doi:10.1111/j.1600-0854.2006.00523.x
Peyrard, M., Seroussi, E., Sandberg-Nordqvist, A. C., Xie, Y. G., Han, F. Y., Fransson, I., et al. (1999). The human LARGE gene from 22q12.3-q13.1 is a new, distinct member of the glycosyltransferase gene family. Proc. Natl. Acad. Sci. U. S. A. 96 (2), 598–603. doi:10.1073/pnas.96.2.598
Plume, J. M., Todd, D., and Bonthius, D. J. (2019). Viral strain determines disease symptoms, pathology, and immune response in neonatal rats with lymphocytic choriomeningitis virus infection. Viruses 11 (6), 552. doi:10.3390/v11060552
Praissman, J. L., Live, D. H., Wang, S., Ramiah, A., Chinoy, Z. S., Boons, G. J., et al. (2014). B4GAT1 is the priming enzyme for the LARGE-dependent functional glycosylation of α-dystroglycan. eLife 3, e03943. doi:10.7554/eLife.03943
Praissman, J. L., and Wells, L. (2014). Mammalian O-mannosylation pathway: glycan structures, enzymes, and protein substrates. Biochemistry 53 (19), 3066–3078. doi:10.1021/bi500153y
Praissman, J. L., Willer, T., Sheikh, M. O., Toi, A., Chitayat, D., Lin, Y. Y., et al. (2016). The functional O-mannose glycan on α-dystroglycan contains a phospho-ribitol primed for matriglycan addition. eLife 5, e14473. doi:10.7554/eLife.14473
Pries, A. R., Secomb, T. W., and Gaehtgens, P. (2000). The endothelial surface layer. Pflugers Archiv Eur. J. physiology 440 (5), 653–666. doi:10.1007/s004240000307
Qiu, J., Handa, A., Kirby, M., and Brown, K. E. (2000). The interaction of heparin sulfate and adeno-associated virus 2. Virology 269 (1), 137–147. doi:10.1006/viro.2000.0205
Quereda, C., Pastor, À., and Martín-Nieto, J. (2022). Involvement of abnormal dystroglycan expression and matriglycan levels in cancer pathogenesis. Cancer Cell Int. 22 (1), 395. doi:10.1186/s12935-022-02812-7
Radoshitzky, S. R., Abraham, J., Spiropoulou, C. F., Kuhn, J. H., Nguyen, D., Li, W., et al. (2007). Transferrin receptor 1 is a cellular receptor for New World haemorrhagic fever arenaviruses. Nature 446 (7131), 92–96. doi:10.1038/nature05539
Rambukkana, A., Kunz, S., Min, J., Campbell, K. P., and Oldstone, M. B. A. (2003). Targeting Schwann cells by nonlytic arenaviral infection selectively inhibits myelination. Proc. Natl. Acad. Sci. U. S. A. 100 (26), 16071–16076. doi:10.1073/pnas.2232366100
Reitsma, S., Slaaf, D. W., Vink, H., van Zandvoort, M. A. M. J., and oude Egbrink, M. G. A. (2007). The endothelial glycocalyx: composition, functions, and visualization. Pflügers Archiv - Eur. J. Physiology 454 (3), 345–359. doi:10.1007/s00424-007-0212-8
Rojek, J. M., and Kunz, S. (2008). Cell entry by human pathogenic arenaviruses. Cell. Microbiol. 10 (4), 828–835. doi:10.1111/j.1462-5822.2007.01113.x
Rojek, J. M., Lee, A. M., Nguyen, N., Spiropoulou, C. F., and Kunz, S. (2008). Site 1 protease is required for proteolytic processing of the glycoproteins of the South American hemorrhagic fever viruses Junin, Machupo, and Guanarito. J. virology 82 (12), 6045–6051. doi:10.1128/JVI.02392-07
Rojek, J. M., Sanchez, A. B., Nguyen, N. T., de la Torre, J. C., and Kunz, S. (2008). Different mechanisms of cell entry by human-pathogenic Old World and New World arenaviruses. J. virology 82 (15), 7677–7687. doi:10.1128/JVI.00560-08
Rojek, J. M., Spiropoulou, C. F., Campbell, K. P., and Kunz, S. (2007). Old World and clade C New World arenaviruses mimic the molecular mechanism of receptor recognition used by alpha-dystroglycan’s host-derived ligands. J. virology 81 (11), 5685–5695. doi:10.1128/JVI.02574-06
Salam, A. P., Duvignaud, A., Jaspard, M., Malvy, D., Carroll, M., Tarning, J., et al. (2022). Ribavirin for treating Lassa fever: a systematic review of pre-clinical studies and implications for human dosing. PLoS neglected Trop. Dis. 16 (3), e0010289. doi:10.1371/journal.pntd.0010289
Salmela, M., Jokinen, J., Tiitta, S., Rappu, P., Cheng, R. H., and Heino, J. (2017). Integrin α2β1 in nonactivated conformation can induce focal adhesion kinase signaling. Sci. Rep. 7 (1), 3414. doi:10.1038/s41598-017-03640-w
Salvato, M., Borrow, P., Shimomaye, E., and Oldstone, M. B. (1991). Molecular basis of viral persistence: a single amino acid change in the glycoprotein of lymphocytic choriomeningitis virus is associated with suppression of the antiviral cytotoxic T-lymphocyte response and establishment of persistence. J. virology 65 (4), 1863–1869. doi:10.1128/JVI.65.4.1863-1869.1991
Sanchez, A. J., Vincent, M. J., Erickson, B. R., and Nichol, S. T. (2006). Crimean-Congo hemorrhagic fever virus glycoprotein precursor is cleaved by Furin-like and SKI-1 proteases to generate a novel 38-kilodalton glycoprotein. J. virology 80 (1), 514–525. doi:10.1128/JVI.80.1.514-525.2006
Sasaki, M., Anindita, P. D., Ito, N., Sugiyama, M., Carr, M., Fukuhara, H., et al. (2018). The role of heparan sulfate proteoglycans as an attachment factor for rabies virus entry and infection. J. Infect. Dis. 217 (11), 1740–1749. doi:10.1093/infdis/jiy081
Sauter, N. K., Bednarski, M. D., Wurzburg, B. A., Hanson, J. E., Whitesides, G. M., Skehel, J. J., et al. (1989). Hemagglutinins from two influenza virus variants bind to sialic acid derivatives with millimolar dissociation constants: a 500-MHz proton nuclear magnetic resonance study. Biochemistry 28 (21), 8388–8396. doi:10.1021/bi00447a018
Schaeffer, J., Carnec, X., Reynard, S., Mateo, M., Picard, C., Pietrosemoli, N., et al. (2018). Lassa virus activates myeloid dendritic cells but suppresses their ability to stimulate T cells. PLoS Pathog. 14 (11), e1007430. doi:10.1371/journal.ppat.1007430
Schlie, K., Maisa, A., Lennartz, F., Ströher, U., Garten, W., and Strecker, T. (2010). Characterization of Lassa virus glycoprotein oligomerization and influence of cholesterol on virus replication. J. virology 84 (2), 983–992. doi:10.1128/JVI.02039-09
Sciandra, F., Bozzi, M., Bigotti, M. G., and Brancaccio, A. (2013). The multiple affinities of α-dystroglycan. Curr. protein and peptide Sci. 14, 626–634. doi:10.2174/1389203711209070644
Seidah, N. G., and Prat, A. (2007). The proprotein convertases are potential targets in the treatment of dyslipidemia. J. Mol. Med. 85 (7), 685–696. doi:10.1007/s00109-007-0172-7
Sevilla, N., Kunz, S., Holz, A., Lewicki, H., Homann, D., Yamada, H., et al. (2000). Immunosuppression and resultant viral persistence by specific viral targeting of dendritic cells. J. Exp. Med. 192 (9), 1249–1260. doi:10.1084/jem.192.9.1249
Sheikh, M. O., Halmo, S. M., and Wells, L. (2017). Recent advancements in understanding mammalian O-mannosylation. Glycobiology 27 (9), 806–819. doi:10.1093/glycob/cwx062
Sheikh, M. O., Venzke, D., Anderson, M. E., Yoshida-Moriguchi, T., Glushka, J. N., Nairn, A. V., et al. (2020). HNK-1 sulfotransferase modulates α-dystroglycan glycosylation by 3-O-sulfation of glucuronic acid on matriglycan. Glycobiology 30 (10), 817–829. doi:10.1093/glycob/cwaa024
Shimojima, M., and Kawaoka, Y. (2012). Cell surface molecules involved in infection mediated by lymphocytic choriomeningitis virus glycoprotein. J. veterinary Med. Sci./Jpn. Soc. Veterinary Sci. 74 (10), 1363–1366. doi:10.1292/jvms.12-0176
Shimojima, M., Ströher, U., Ebihara, H., Feldmann, H., and Kawaoka, Y. (2012). Identification of cell surface molecules involved in dystroglycan-independent lassa virus cell entry. J. virology 86 (4), 2067–2078. doi:10.1128/JVI.06451-11
Shimon, A., Shani, O., and Diskin, R. (2017). Structural basis for receptor selectivity by the whitewater arroyo mammarenavirus. J. Mol. Biol. 429 (18), 2825–2839. doi:10.1016/j.jmb.2017.07.011
Shulman, A., Katz, M., Cohen-Dvashi, H., Greenblatt, H. M., Levy, Y., and Diskin, R. (2019). Variations in core packing of GP2 from old world mammarenaviruses in their post-fusion conformations affect membrane-fusion efficiencies. J. Mol. Biol. 431 (11), 2095–2111. doi:10.1016/j.jmb.2019.04.012
Shurer, C. R., Kuo, J. C. H., Roberts, L. M., Gandhi, J. G., Colville, M. J., Enoki, T. A., et al. (2019). Physical principles of membrane shape regulation by the glycocalyx. Cell 177 (7), 1757–1770. doi:10.1016/j.cell.2019.04.017
Smelt, S. C., Borrow, P., Kunz, S., Cao, W., Tishon, A., Lewicki, H., et al. (2001). Differences in affinity of binding of lymphocytic choriomeningitis virus strains to the cellular receptor alpha-dystroglycan correlate with viral tropism and disease kinetics. J. virology 75 (1), 448–457. doi:10.1128/JVI.75.1.448-457.2001
Soares, M. M., King, S. W., and Thorpe, P. E. (2008). Targeting inside-out phosphatidylserine as a therapeutic strategy for viral diseases. Nat. Med. 14 (12), 1357–1362. doi:10.1038/nm.1885
Sommerstein, R., Flatz, L., Remy, M. M., Malinge, P., Magistrelli, G., Fischer, N., et al. (2015). Arenavirus glycan shield promotes neutralizing antibody evasion and protracted infection. PLoS Pathog. 11 (11), 10052766–e1005325. doi:10.1371/journal.ppat.1005276
Southern, P. J., and Bishop, D. H. L. (1987). Sequence comparison among arenaviruses. Curr. Top. Microbiol. Immunol. 133, 19–39. doi:10.1007/978-3-642-71683-6_3
Spillmann, D. (2001). Heparan sulfate: anchor for viral intruders? Biochimie 83 (8), 811–817. doi:10.1016/s0300-9084(01)01290-1
Spiropoulou, C. F., Kunz, S., Rollin, P. E., Campbell, K. P., and Oldstone, M. B. A. (2002). New World arenavirus clade C, but not clade A and B viruses, utilizes alpha-dystroglycan as its major receptor. J. virology 76 (10), 5140–5146. doi:10.1128/jvi.76.10.5140-5146.2002
Stephenson, E. H., Larson, E. W., and Dominik, J. W. (1984). Effect of environmental factors on aerosol-induced lassa virus infection. J. Med. virology 14 (4), 295–303. doi:10.1002/jmv.1890140402
Summerford, C., and Samulski, R. J. (1998). Membrane-associated heparan sulfate proteoglycan is a receptor for adeno-associated virus type 2 virions. J. virology 72 (2), 1438–1445. doi:10.1128/JVI.72.2.1438-1445.1998
Suprunenko, T., and Hofer, M. J. (2019). Complexities of type I interferon biology: lessons from LCMV. Viruses 11 (2), 172. doi:10.3390/v11020172
Taujale, R., Venkat, A., Huang, L. C., Zhou, Z., Yeung, W., Rasheed, K. M., et al. (2020). Deep evolutionary analysis reveals the design principles of fold A glycosyltransferases. eLife 9, e54532. doi:10.7554/eLife.54532
Teng, M. N., Borrow, P., Oldstone, M. B., and de la Torre, J. C. (1996). A single amino acid change in the glycoprotein of lymphocytic choriomeningitis virus is associated with the ability to cause growth hormone deficiency syndrome. J. virology 70 (12), 8438–8443. doi:10.1128/JVI.70.12.8438-8443.1996
Thakkar, N., Yadavalli, T., Jaishankar, D., and Shukla, D. (2017). Emerging roles of heparanase in viral pathogenesis. Pathogens 6 (3), 43. doi:10.3390/pathogens6030043
Vincent, M. J., Sanchez, A. J., Erickson, B. R., Basak, A., Chretien, M., Seidah, N. G., et al. (2003). Crimean-Congo hemorrhagic fever virus glycoprotein proteolytic processing by subtilase SKI-1. J. virology 77 (16), 8640–8649. doi:10.1128/jvi.77.16.8640-8649.2003
Volland, A., Lohmüller, M., Heilmann, E., Kimpel, J., Herzog, S., and von Laer, D. (2021). Heparan sulfate proteoglycans serve as alternative receptors for low affinity LCMV variants. PLoS Pathog. 17 (10), 10099966–e1010022. doi:10.1371/journal.ppat.1009996
Walimbe, A. S., Okuma, H., Joseph, S., Yang, T., Yonekawa, T., Hord, J. M., et al. (2020). POMK regulates dystroglycan function via LARGE1-mediated elongation of matriglycan. eLife 9, e61388. doi:10.7554/eLife.61388
Watanabe, Y., Raghwani, J., Allen, J. D., Seabright, G. E., Li, S., Moser, F., et al. (2018). Structure of the Lassa virus glycan shield provides a model for immunological resistance. Proc. Natl. Acad. Sci. 115 (28), 7320–7325. doi:10.1073/pnas.1803990115
Willer, T., Inamori, K. I., Venzke, D., Harvey, C., Morgensen, G., Hara, Y., et al. (2014). The glucuronyltransferase B4GAT1 is required for initiation of LARGE-mediated α-dystroglycan functional glycosylation. eLife 3, e03941. doi:10.7554/eLife.03941
Xu, D., and Esko, J. D. (2014). Demystifying heparan sulfate–protein interactions. Annu. Rev. Biochem. 83 (1), 129–157. doi:10.1146/annurev-biochem-060713-035314
Xu, R., Zhu, X., McBride, R., Nycholat, C. M., Yu, W., Paulson, J. C., et al. (2012). Functional balance of the hemagglutinin and neuraminidase activities accompanies the emergence of the 2009 H1N1 influenza pandemic. J. virology 86 (17), 9221–9232. doi:10.1128/JVI.00697-12
Yang, T., Chandel, I., Gonzales, M., Okuma, H., Prouty, S. J., Zarei, S., et al. (2023). Identification of a short, single site matriglycan that maintains neuromuscular function in the mouse. bioRxiv. doi:10.1101/2023.12.20.572361
Yaro, C. A., Kogi, E., Opara, K. N., Batiha, G. E. S., Baty, R. S., Albrakati, A., et al. (2021). Infection pattern, case fatality rate and spread of Lassa virus in Nigeria. BMC Infect. Dis. 21 (1), 149. doi:10.1186/s12879-021-05837-x
Yoshida-Moriguchi, T., and Campbell, K. P. (2015). Matriglycan: a novel polysaccharide that links dystroglycan to the basement membrane. Glycobiology 25 (7), 702–713. doi:10.1093/glycob/cwv021
Yoshida-Moriguchi, T., Willer, T., Anderson, M. E., Venzke, D., Whyte, T., Muntoni, F., et al. (2013). SGK196 is a glycosylation-specific O-mannose kinase required for dystroglycan function. Science 341 (6148), 896–899. doi:10.1126/science.1239951
Yoshida-Moriguchi, T., Yu, L., Stalnaker, S. H., Davis, S., Kunz, S., Madson, M., et al. (2010). O-mannosyl phosphorylation of alpha-dystroglycan is required for laminin binding. Science 327 (5961), 88–92. doi:10.1126/science.1180512
Keywords: lassa virus, arenaviruses, spike complex, LARGE1, receptor recognition, matriglycan
Citation: Katz M and Diskin R (2024) The underlying mechanisms of arenaviral entry through matriglycan. Front. Mol. Biosci. 11:1371551. doi: 10.3389/fmolb.2024.1371551
Received: 16 January 2024; Accepted: 15 February 2024;
Published: 07 March 2024.
Edited by:
Bulmaro Cisneros, National Polytechnic Institute of Mexico (CINVESTAV), MexicoReviewed by:
Lisa Parsons, United States Food and Drug Administration, United StatesCopyright © 2024 Katz and Diskin. This is an open-access article distributed under the terms of the Creative Commons Attribution License (CC BY). The use, distribution or reproduction in other forums is permitted, provided the original author(s) and the copyright owner(s) are credited and that the original publication in this journal is cited, in accordance with accepted academic practice. No use, distribution or reproduction is permitted which does not comply with these terms.
*Correspondence: Ron Diskin, ron.diskin@weizmann.ac.il