Microvascular Changes in Parkinson’s Disease- Focus on the Neurovascular Unit
- 1Translational Neurology Group, Department of Clinical Sciences, Lund University, Lund, Sweden
- 2Department of Neurology, Scania University Hospital, Lund, Sweden
- 3Wallenberg Centre for Molecular Medicine, Lund University, Lund, Sweden
Vascular alterations emerge as a common denominator for several neurodegenerative diseases. In Parkinson’s disease (PD), a number of observations have been made suggesting that the occurrence of vascular pathology is an important pathophysiological aspect of the disease. Specifically, pathological activation of pericytes, blood-brain barrier (BBB) disruption, pathological angiogenesis and vascular regression have been reported. This review summarizes the current evidence for the different vascular alterations in patients with PD and in animal models of PD. We suggest a possible sequence of vascular pathology in PD ranging from early pericyte activation and BBB leakage to an attempt for compensatory angiogenesis and finally vascular rarefication. We highlight different pathogenetic mechanisms that play a role in these vascular alterations including perivascular inflammation and concomitant metabolic disease. Awareness of the contribution of vascular events to the pathogenesis of PD may allow the identification of targets to modulate those mechanisms. In particular the BBB has for decades only been viewed as an obstacle for drug delivery, however, preservation of its integrity and/or modulation of the signaling at this interface between the blood and the brain may prove to be a new avenue to take in order to develop disease-modifying strategies for neurodegenerative disorders.
Brain Vasculature
The brain is a highly oxygen consuming organ and, as a result, has developed a dense network of almost 650 km of microvessels (Pandey et al., 2016). The smallest entity is formed by capillaries that are in close contact with the surrounding parenchyma and allow the gas exchange. This close connection between blood and brain is termed the neurovascular unit (NVU). The NVU consists of endothelial cells, pericytes and the basal lamina forming the microcapillary wall, and cells in the immediate surrounding brain parenchyma including perivascular astrocytes, perivascular microglia and neurons.
Blood Vessels as Adaptors of Blood Flow
Capillaries of the brain are non-fenestrated vessels regulating the influx of nutrients and oxygen according to the changing demands of the brain (Iadecola, 2017).
The adaption to the brains requirements occurs by neurovascular coupling, matching the local blood supply to the neuronal demand by adjustment of the vascular intraluminal diameter (Carmignoto and Gomez-Gonzalo, 2010). Preservation of the highly balanced homeostasis of the brain’s microenvironment, however, is guaranteed by the BBB.
Blood Vessels as Gate Keepers at the Blood-Brain Barrier
The BBB is formed by endothelial cells that require close contact with pericytes in order to form tight junctions, by the basal lamina and by astrocytic endfeet (Zhao et al., 2015). The integrity of the BBB is absolutely vital for normal neuronal function. A leaky BBB enables the uncontrolled entry of pathogens, toxins and inflammatory cells into the brain and leads to inflammatory and immune responses. BBB leakage, whether subtle or severe, ultimately leads to neuronal injury, neurodegeneration and accelerates disease progression (Bell et al., 2010; Sweeney et al., 2018a).
Blood Vessels as Communicators of Signals
As brain capillaries form the contact surface between the blood and the brain, cells at this interface are also the first sensors of systemic changes such as metabolic imbalances, systemic inflammation, circulating pathogens, changes in oxygen tension etc. In particular brain pericytes have been identified as first responders to systemic inflammation mediating signals from the blood onto the neighboring parenchyma cells (Duan et al., 2018). Vascular pathology and changes in cell signaling at and across the BBB may be the interface linking systemic risk factors (e.g., diabetes or chronic inflammation) to neuroinflammation and neurodegeneration (see section “Metabolic Disorders and Vascular Changes in Parkinson’s Disease”).
Vascular Changes in Parkinson’s Disease
Blood vessel alterations, BBB disruption and cerebral blood flow abnormalities are a common denominator of several neurodegenerative disorders and have been described in Alzheimer’s disease (Sweeney et al., 2018b), amyotrophic lateral sclerosis (Zhong et al., 2008; Garbuzova-Davis and Sanberg, 2014; Winkler et al., 2014), Huntington’s disease (Padel et al., 2018) and Parkinson’s disease (PD). There is a growing appreciation that vascular alterations can contribute to disease onset and aggravate the neurodegenerative process as some vascular changes already occur before the onset of neuronal loss or behavioral deficits in animal models of the respective disease (Sagare et al., 2013; Winkler et al., 2014; Padel et al., 2016; Elabi O. et al., 2021).
Here we particularly outline the different histological vascular changes reported in patients with PD and summarize the evidence for vascular alterations from animal models of PD. This minireview does not cover the role of hypoperfusion or white matter lesions in the pathogenesis of PD.
Parkinson’s Disease
Parkinson’s disease (PD) is the second most common neurodegenerative disorder and one of the fastest growing neurological diseases. In 2015, PD affected 6.9 Million people worldwide, a number expected to double by 2040 due to the aging population (Dorsey and Bloem, 2018).
The progressive degeneration of the nigrostriatal system gives rise to the typical clinical symptoms rigidity, bradykinesia and resting tremor (Fearnley and Lees, 1991). In PD, dopaminergic neurons in the substantia nigra pars compacta (SNpc) are degenerating and the histopathological hallmark is the formation of Lewy bodies containing aggregated alpha-synuclein (α-syn) (Spillantini et al., 1997). Although PD is associated with these distinct histological changes, concomitant pathological alterations might sustain or aggravate the neuronal degeneration. This is particularly relevant as there is currently no therapy available that intervenes with the ongoing disease process. In this context, any contributor to the disease is important to elicit.
The Microvascular Environment in Parkinson’s Disease
Almost 90 years ago, it was described that the capillary network in the SNpc is considerably denser than in the adjacent SN zona reticulata (Finley, 1936). Under normal conditions, a distinct tight pattern of neuron-capillary associations is observed in the SNpc. However, in PD, these close contacts between dopaminergic neurons and microvessels are lost leaving an “empty space” (Issidorides, 1971). Based on these early observations it was postulated that modifications of the vascular microenvironment of dopaminergic neurons may alter the availability of nutrients or lead to accumulation of toxic compounds in the immediate vicinity of these cells. Later, vascular alterations in PD were described more in detail ranging from signs of angiogenesis to BBB leakage and vascular regression (Figure 1).
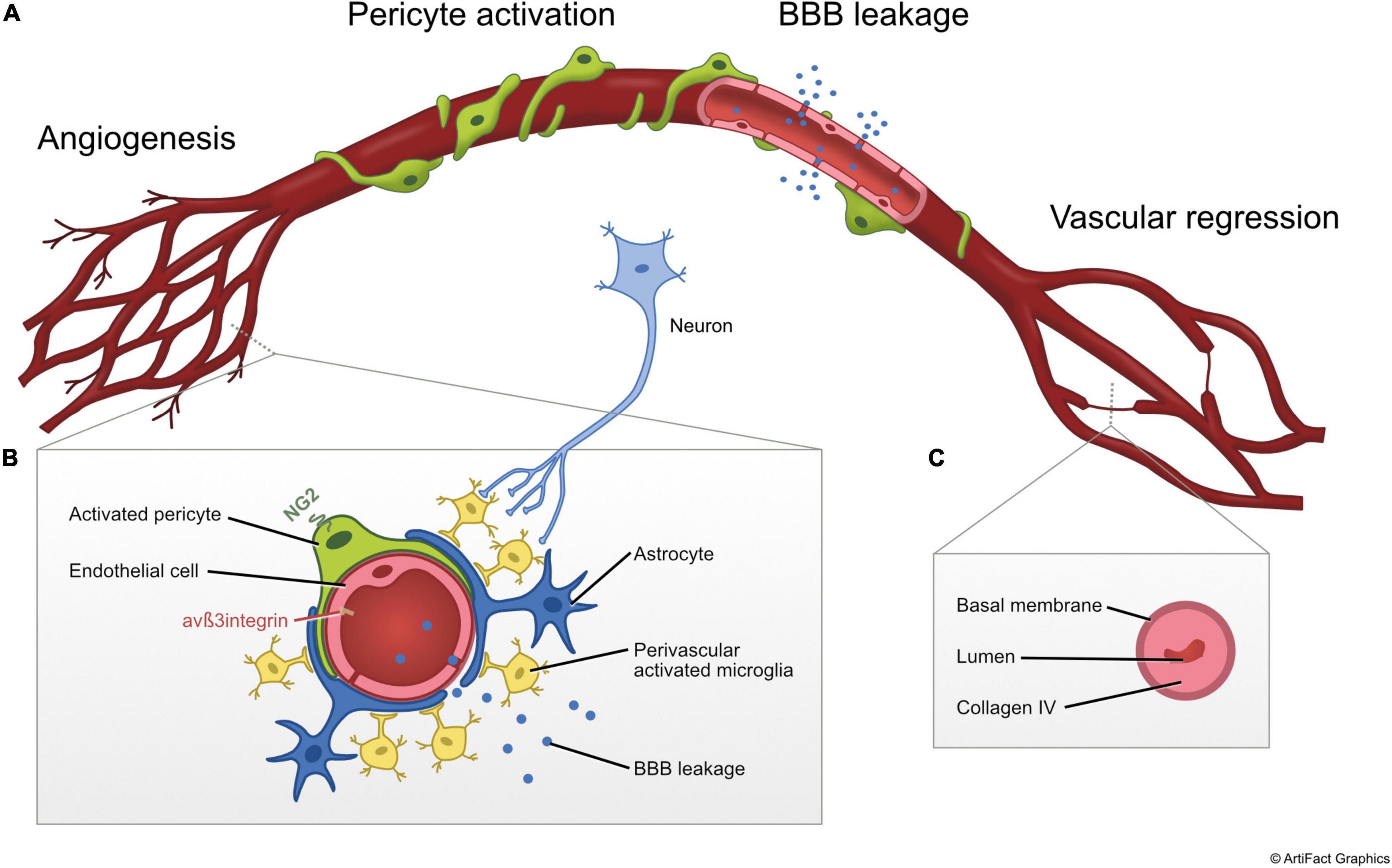
Figure 1. Illustration of vascular changes observed in post mortem tissue and preclinical models of PD. (A) Simplified diagram illustrating the main vascular alterations in non-chronological order including (1) angiogenesis, (2) pericyte activation, (3) BBB leakage, and (4) vascular regression. (B) Cross-sectional diagram of the NVU during the angiogenic response. (C) Cross-sectional diagram of string vessel showing the collapsed basement membrane labeled with collagen-IV and absence of endothelial cells during vascular regression stage. The process of vascular regression includes loss of pericytes and signaling between pericytes and endothelial cells, causing destabilization of endothelial cells and endothelial cell death. NG2, neuron-glial antigen 2.
Evidence for Angiogenesis in Parkinson’s Disease
Angiogenesis refers to the formation of new blood vessels in adulthood. In the adult brain it usually occurs in response to hypoxia or inflammation (Tahergorabi and Khazaei, 2012). Angiogenesis can be recognized by an increase in vascular density and branching points, proliferation of vascular cells, expression of angiogenic markers or an increase in angiogenic molecules in the brain or cerebrospinal fluid (CSF).
Angiogenic Microvessels in Parkinson’s Disease
First evidence for pathological angiogenesis in PD comes from studies in the 90’s observing a 2.5-fold increase in the number of endothelial cells in PD brains (Faucheux et al., 1999) and an increased number of integrin αvβ3-positive vessels, an adhesion molecule that is present on angiogenic vessels (Brooks, 1996) in the SNpc, the locus coeruleus and the putamen, all regions which are affected in PD (Desai Bradaric et al., 2012).
Similar findings reporting angiogenesis in several brain regions were made in the 6-hydroxydopamine (6-OHDA) PD model in rats (Carvey et al., 2005) and mice (Elabi O. F. et al., 2021), in an experimental model of L-DOPA-induced dyskinesias (Westin et al., 2006) and in the 1-methyl-4-phenyl-1,2,3,6-tetrahydropyridine (MPTP) monkey model (Barcia et al., 2005).
Even though toxin-induced models are useful to study several aspects of PD, they do not reflect the slowly progressive nature of PD pathology. Using a human α-syn overexpression mouse model that recapitulates the progressive aggregation of human α-syn (Hansen et al., 2013), we confirmed an increase in vessel density indicating angiogenesis at the moderate stage of the animal model. In late-stage animals, however, the vessel density was significantly reduced suggesting dynamic and stage-dependent vascular changes in PD (Elabi O. et al., 2021).
Pathological Pericyte Activation
Interestingly, at the early stage of α-syn-aggregation, we observed an activation of pericytes that was preceding changes in vessel density and behavioral deficits (Elabi O. et al., 2021). Pericytes line the entire microvasculature of the brain and have an important function in angiogenesis (Stapor et al., 2014). Activation of pericytes leads to changes in morphology and marker expression. Capillary pericytes generally have a flat cell soma with extensive longitudinal and thin processes (Dore-Duffy and Cleary, 2011). However, under pathological conditions, pericytes acquire a more bulging cell soma with shorter processes, typical of activated and migratory pericytes (Dore-Duffy and Cleary, 2011; Ozen et al., 2014). This pattern is predominantly seen following injury and during the early stages of angiogenesis and often associated with expression of markers such as NG2 and/or RGS5 (Ozerdem and Stallcup, 2004; Berger et al., 2005). Angiogenesis requires first pericyte detachment from the vessel wall, allowing endothelial sprouting and then subsequent pericyte recruitment for stabilization and maturation of the vasculature (Kamouchi et al., 2012). We have previously shown that pericytes are activated in the 6-OHDA PD model (Padel et al., 2016), and that pathological pericyte activation is a feature of also other neurodegenerative disorders (Padel et al., 2018).
Pericytes are one of the first responders to brain hypoxia (Gonul et al., 2002; Duz et al., 2007) and to systemic inflammation (Duan et al., 2018). Importantly, pericytes alter their signaling toward a pro-inflammatory secretome when activated (Rustenhoven et al., 2017; Gaceb and Paul, 2018; Gaceb et al., 2018). Interestingly, a direct observation that α-syn can activate pericytes comes from an in vitro study where exposure to monomeric α-syn leads to secretion of high amounts of pro-inflammatory molecules in pericytes that in turn mediated hyperpermeability in endothelial cells resulting in BBB leakage (Dohgu et al., 2019).
Thus, it is conceivable that pericyte activation may form the starting point of vascular alterations and a cascade of pathological signaling events in the NVU in PD.
Angiogenic Molecules
Findings indicating angiogenesis in PD are supported by reports showing an upregulation of the pro-angiogenic molecule Vascular Endothelial Growth Factor (VEGF) in the SNpc of PD patients (Wada et al., 2006; Yasuda et al., 2007; Lan et al., 2021) and non-human primates (Barcia et al., 2005). Increased levels of soluble VEGF receptor-2 and placental growth factor, and lower levels of angiopoietin 2 (an anti-angiogenic molecule) were detected in the CSF of PD patients (Janelidze et al., 2015). In this study, angiogenesis markers in the CSF were associated with microbleeds and white matter lesions on imaging, suggesting abnormal angiogenesis in PD (Janelidze et al., 2015). Further strengthening these findings, a recent study demonstrated CSF changes in miRNAs regulating pathways of angiogenesis and BBB components in PD patients with moderate disease, implying impairment of these pathways as part of the progression of PD (Fowler et al., 2021).
Blood-Brain Barrier Dysfunction in Parkinson’s Disease
Blood-Brain Barrier Dysfunction in Parkinson’s Disease Animal Models
Angiogenesis is a double-edged sword as newly formed vessels are immature and can lead to BBB leakage, especially when pericyte recruitment is impaired.
Indeed, a dysfunctional BBB has been demonstrated in a number of different PD models showing leakage of albumin and other tracers into the brain parenchyma (Carvey et al., 2005, 2009; Westin et al., 2006; Zhao et al., 2007; Chen et al., 2008), increased entry of drugs (Carta et al., 2006; Westin et al., 2006) and infiltration of peripheral immune cells otherwise are prevented from crossing the BBB (Benner et al., 2008; Brochard et al., 2009; Reynolds et al., 2010). Few studies have not been able to confirm BBB leakage, likely due to the methods used (Astradsson et al., 2009; Elabi O. F. et al., 2021).
A study using the A53T PD mouse model showed that the expression of tight junction-related proteins at the BBB decreased leading to increased vascular permeability (Lan et al., 2021). When we examined the temporal dynamics of BBB leakage in another progressive α-syn-PD mouse model (Elabi O. et al., 2021), we detected significant extravascular fibrinogen accumulation already at the early stage preceding behavioral deficits (Elabi O. et al., 2021).
Blood-Brain Barrier Dysfunction in Parkinson’s Disease Post Mortem Tissue
The evidence of an impaired BBB in PD from animal models is validated by compelling post mortem studies in PD using a variety of different methods. Gray and Woulfe (2015) found a sevenfold increase in extravasated erythrocytes, a threefold increase in hemosiderin depositions (often grouped around capillaries), a significant perivascular deposition of hemoglobin (8.6-fold increase) and a 9.4-fold increase in extrasudated fibrinogen in the striatum of PD patients compared to controls. Greater fibrinogen accumulation (Yang et al., 2015), higher IgG leakage and loss of tight junction proteins (Pienaar et al., 2015) were also reported in other autopsy studies.
Similarly, a 10-fold increase of extravascular CD4+ and CD8+ lymphocytes has been shown particularly in the SNpc in post mortem PD brain tissue (Brochard et al., 2009) demonstrating pathological immune cell infiltration across the BBB.
Blood-Brain Barrier Dysfunction Examined in the Cerebrospinal Fluid
In line with post mortem findings, CSF studies have shown BBB leakage as indicated by increased levels of CSF albumin in PD correlating with the severity of the disease (Pisani et al., 2012), or with the level of angiogenic factors in the CSF (Janelidze et al., 2015).
Blood-Brain Barrier Dysfunction Using in vivo-Imaging
Blood-brain barrier dysfunction in PD patients in vivo is more difficult to study. Using positron-emission tomography (PET), progressive BBB impairment has been shown in the midbrain of PD patients as seen by an increased uptake of the tracer 11C-verapamil indicating impairment of the BBB efflux pump P-glycoprotein (Kortekaas et al., 2005; Bartels et al., 2008) and analysis of dynamic contrast-enhanced magnetic resonance images revealed higher BBB leakage in PD patients (Al-Bachari et al., 2020), whereas a study using rubidium-82-PET could not detect BBB leakage in PD patients (Fujita et al., 2021).
Vascular Regression
Angiogenesis and BBB leakage are not the only vascular pathology that has been observed in PD. Signs indicating vascular regression come from reports showing endothelial degeneration, decrease in vessel length and number of branching points and increase in vessel diameter in the SN of PD patients compared to age-matched controls (Guan et al., 2013; Yang et al., 2015). In addition, PD patients had higher numbers, density and total length of “string vessels” when compared to controls (Yang et al., 2015). String vessels are linked to endothelial cell degeneration leaving empty collapsed basal membrane tubes that do not take part in perfusion (Brown, 2010). Using electron microscopy, Farkas et al. (2000) also demonstrated basal membrane thickening in cerebral capillaries in PD.
Vascular regression likely indicates a later stage of vascular pathology in PD. When studying the temporal dynamics of vascular changes, we noted that early pericyte activation and BBB leakage were followed by angiogenesis, whereas vascular rarefication did not occur until the late stage of the PD model (Elabi O. et al., 2021). Thus, the microvasculature in PD might undergo both, an angiogenic and pruning vascular response, whereby occurrence of BBB leakage could be an early event, followed by the attempt for neovascularization at the moderate stage of the disease and vascular degeneration as a sign of late-stage disease. In the α-syn-PD mouse model we observed colocalization of α-syn and phosphorylated α-syn with endothelial cells at all stages, which suggests a direct involvement of α-syn in the vascular pathological mechanism in addition to a pathological stimulation of pericytes (Elabi O. et al., 2021).
Inflammation and Vascular Pathology
Microglia
In PD, neuroinflammation is a well-known pathology as documented by increased numbers of activated microglia in PD brains (Mcgeer et al., 1988; Croisier et al., 2005; Zhang et al., 2005; Whitton, 2007; McGeer and McGeer, 2008) and increased levels of pro-inflammatory molecules in the CSF of PD (Blum-Degen et al., 1995).
Several studies demonstrating an increased activation of microglia also reported BBB disruption in these PD models (Carvey et al., 2005; Zhao et al., 2007; Elabi O. et al., 2021). The interactions between microglia and blood vessels are complex: Microglia are likely activated by the parenchymal leakage of plasma proteins (Merlini et al., 2019), on the other hand, activated microglia may also induce angiogenesis and vascular leakage via the release of inflammatory and pro-angiogenic molecules (Naldini and Carraro, 2005; Ritzel et al., 2015; Haddick et al., 2017; Salter and Stevens, 2017; Chen et al., 2019). The proinflammatory cytokines cause a reduction in the expression of tight junction proteins and increase matrix metalloproteinase-3 and −9, which affect the BBB integrity (Raymond et al., 2016; Bonetti et al., 2019; Edwards et al., 2020).
Recently, we have observed that activated microglia are highly localized particularly in the perivascular space in PD (Elabi O. F. et al., 2021). It has been suggested that perivascular microglia have a dual effect on vessels, maintaining vascular integrity under normal conditions, but phagocytosing the vessel and impairing BBB integrity under prolonged inflammation (Haruwaka et al., 2019). The reason for this increase in activated perivascular microglia in PD is not known, however, we noted the level of perivascular microglia to be strongly associated with the number of pericytes (Elabi O. F. et al., 2021), suggesting a possible interaction of these two cell types at the vascular border.
Other Inflammatory Cell Types
Within the NVU, also astrocytes can release pro-inflammatory cytokines and angiogenic molecules that can affect vascular function (Lee et al., 2010; Barcia et al., 2011; Kam et al., 2020). Several studies have highlighted the role of astrocytes in the control of vascular function, via e.g., cross-talk with microglia (Wang et al., 2014; Ni et al., 2018).
Similarly, pericytes can produce a variety of inflammatory and angiogenic molecules (Gaceb and Paul, 2018; Gaceb et al., 2018). Activation of pericytes specifically via α-syn stimulates release of cytokines and increases expression MMP9 that lead to an increased EC permeability (Dohgu et al., 2019), placing pericytes as mediators between α-syn and BBB disruption.
Metabolic Disorders and Vascular Changes in Parkinson’s Disease
An increasing number of studies suggest an association between neurodegeneration and metabolic diseases. Epidemiological evidence indicates that diabetes is also a risk factor and a negative prognostic factor for PD (Cereda et al., 2011; Pagano et al., 2018; Heinzel et al., 2019; Sergi et al., 2019; Chohan et al., 2021). The link between metabolic dysfunction and neurodegeneration in PD is further strengthened by studies demonstrating a beneficial effect of anti-diabetic medication in PD and PD models (Foltynie and Athauda, 2020). In particular, Exenatide, a glucagon-like peptide-1 (GLP-1) receptor agonist, has shown neuroprotective effects in preclinical models of PD and entered clinical trials (Athauda et al., 2017).
Even though a number of hypotheses has been put forward to what is leading to this aggravation of PD in the presence of diabetes (Sergi et al., 2019), not much attention has been paid to the fact that diabetes and PD both share pathological microvascular alterations in the brain. Similar to the retinal and renal complications, diabetes is associated with signs of cerebral vascular proliferation and progressive BBB disruption (Starr et al., 2003; Huber et al., 2006; Salameh et al., 2016; Machida et al., 2017; Takechi et al., 2017; Rom et al., 2019; Yamamoto et al., 2019). We have examined the interactions of type 2 diabetes (DMT2) and PD at the microvascular interface and shown that DMT2 in combination with a PD lesion resulted in a significant depletion of pericytes, and reduced interactions between microvessels and perivascular microglia which was associated with a lack of the angiogenic response seen in toxin-induced models (Elabi O. F. et al., 2021). It is conceivable that the diabetic state inhibits the attempt of compensatory angiogenesis seen in PD and accelerates the vessel changes toward a later stage of vascular regression.
Discussion and Outlook
Current evidence points to a dynamic evolution of multiple vascular changes in PD (Figure 1). These changes might start with pathological pericyte activation and subtle BBB leakage, continue with compensatory angiogenesis that then fails and cumulates in vascular regression. What constitutes the initiator of these events still remains unknown, but their occurrence is certainly contributing to a disturbed neuronal microenvironment. Interventions stabilizing the vasculature and preventing the progression of BBB dysfunction are clearly indicated. Treatment with platelet-derived growth factor (PDGF-BB), a growth factor required for pericyte recruitment and vessel maturation (Jain and Booth, 2003), not only induced neurorestoration and behavioral recovery in PD animal models (Zachrisson et al., 2011; Padel et al., 2016), but also normalized the number of activated pericytes (Padel et al., 2016) and changed the inflammatory secretome of pericytes toward a trophic factor pattern in vitro (Gaceb et al., 2018). PDGF-BB has shown safety and tolerability in a phase I/IIa clinical trial in PD patients (Paul et al., 2015; Paul and Sullivan, 2019). It now remains to be seen whether approaches targeting vascular pathology, pericyte activation and vascular signaling at the BBB can modify the progression of the disease.
Author Contributions
GP and OE wrote the manuscript. Both authors contributed to the article and approved the submitted version.
Funding
This study was supported by the Swedish Parkinson Foundation (1217/19 and 1357/21) and the Åhlens Foundation (213025 and 193044).
Conflict of Interest
The authors declare that the research was conducted in the absence of any commercial or financial relationships that could be construed as a potential conflict of interest.
Publisher’s Note
All claims expressed in this article are solely those of the authors and do not necessarily represent those of their affiliated organizations, or those of the publisher, the editors and the reviewers. Any product that may be evaluated in this article, or claim that may be made by its manufacturer, is not guaranteed or endorsed by the publisher.
Acknowledgments
We thank Edward Visse at Artifact Graphics for preparing the figure.
References
Al-Bachari, S., Naish, J. H., Parker, G. J. M., Emsley, H. C. A., and Parkes, L. M. (2020). Blood-Brain Barrier Leakage Is Increased in Parkinson’s Disease. Front. Physiol. 11:593026. doi: 10.3389/fphys.2020.593026
Astradsson, A., Jenkins, B. G., Choi, J. K., Hallett, P. J., Levesque, M. A., McDowell, J. S., et al. (2009). The blood-brain barrier is intact after levodopa-induced dyskinesias in parkinsonian primates–evidence from in vivo neuroimaging studies. Neurobiol. Dis. 35, 348–351. doi: 10.1016/j.nbd.2009.05.018
Athauda, D., Maclagan, K., Skene, S. S., Bajwa-Joseph, M., Letchford, D., Chowdhury, K., et al. (2017). Exenatide once weekly versus placebo in Parkinson’s disease: a randomised, double-blind, placebo-controlled trial. Lancet 390, 1664–1675. doi: 10.1016/S0140-6736(17)31585-4
Barcia, C., Bautista, V., Sanchez-Bahillo, A., Fernandez-Villalba, E., Faucheux, B., and Poza y Poza, M. (2005). Changes in vascularization in substantia nigra pars compacta of monkeys rendered parkinsonian. J. Neural Transm. 112, 1237–1248. doi: 10.1007/s00702-004-0256-2
Barcia, C., Ros, C. M., Annese, V., Gomez, A., Ros-Bernal, F., Aguado-Yera, D., et al. (2011). IFN-gamma signaling, with the synergistic contribution of TNF-alpha, mediates cell specific microglial and astroglial activation in experimental models of Parkinson’s disease. Cell Death Dis. 2:e142. doi: 10.1038/cddis.2011.17
Bartels, A. L., Willemsen, A. T. M., Kortekaas, R., de Jong, B. M., de Vries, R., de Klerk, O., et al. (2008). Decreased blood-brain barrier P-glycoprotein function in the progression of Parkinson’s disease, PSP and MSA. J. Neural Transm. 115, 1001–1009. doi: 10.1007/s00702-008-0030-y
Bell, R. D., Winkler, E. A., Sagare, A. P., Singh, I., LaRue, B., Deane, R., et al. (2010). Pericytes control key neurovascular functions and neuronal phenotype in the adult brain and during brain aging. Neuron 68, 409–427. doi: 10.1016/j.neuron.2010.09.043
Benner, E. J., Banerjee, R., Reynolds, A. D., Sherman, S., Pisarev, V. M., Tsiperson, V., et al. (2008). Nitrated alpha-synuclein immunity accelerates degeneration of nigral dopaminergic neurons. PLoS One 3:e1376. doi: 10.1371/journal.pone.0001376
Berger, M., Bergers, G., Arnold, B., Hammerling, G. J., and Ganss, R. (2005). Regulator of G-protein signaling-5 induction in pericytes coincides with active vessel remodeling during neovascularization. Blood 105, 1094–1101. doi: 10.1182/blood-2004-06-2315
Blum-Degen, D., Muller, T., Kuhn, W., Gerlach, M., Przuntek, H., and Riederer, P. (1995). Interleukin-1 beta and interleukin-6 are elevated in the cerebrospinal fluid of Alzheimer’s and de novo Parkinson’s disease patients. Neurosci. Lett. 202, 17–20. doi: 10.1016/0304-3940(95)12192-7
Bonetti, N. R., Diaz-Canestro, C., Liberale, L., Crucet, M., Akhmedov, A., Merlini, M., et al. (2019). Tumour Necrosis Factor-alpha Inhibition Improves Stroke Outcome in a Mouse Model of Rheumatoid Arthritis. Sci. Rep. 9:2173. doi: 10.1038/s41598-019-38670-z
Brochard, V., Combadiere, B., Prigent, A., Laouar, Y., Perrin, A., Beray-Berthat, V., et al. (2009). Infiltration of CD4+ lymphocytes into the brain contributes to neurodegeneration in a mouse model of Parkinson disease. J. Clin. Invest. 119, 182–192. doi: 10.1172/JCI36470
Brooks, P. C. (1996). Role of integrins in angiogenesis. Eur. J. Cancer 32A, 2423–2429. doi: 10.1016/s0959-8049(96)00381-4
Brown, W. R. (2010). A review of string vessels or collapsed, empty basement membrane tubes. J. Alzheimers Dis. 21, 725–739. doi: 10.3233/JAD-2010-100219
Carmignoto, G., and Gomez-Gonzalo, M. (2010). The contribution of astrocyte signalling to neurovascular coupling. Brain Res. Rev. 63, 138–148. doi: 10.1016/j.brainresrev.2009.11.007
Carta, M., Lindgren, H. S., Lundblad, M., Stancampiano, R., Fadda, F., and Cenci, M. A. (2006). Role of striatal L-DOPA in the production of dyskinesia in 6-hydroxydopamine lesioned rats. J. Neurochem. 96, 1718–1727. doi: 10.1111/j.1471-4159.2006.03696.x
Carvey, P. M., Hendey, B., and Monahan, A. J. (2009). The blood-brain barrier in neurodegenerative disease: a rhetorical perspective. J. Neurochem. 111, 291–314. doi: 10.1111/j.1471-4159.2009.06319.x
Carvey, P. M., Zhao, C. H., Hendey, B., Lum, H., Trachtenberg, J., Desai, B. S., et al. (2005). 6-Hydroxydopamine-induced alterations in blood-brain barrier permeability. Eur. J. Neurosci. 22, 1158–1168. doi: 10.1111/j.1460-9568.2005.04281.x
Cereda, E., Barichella, M., Pedrolli, C., Klersy, C., Cassani, E., Caccialanza, R., et al. (2011). Diabetes and risk of Parkinson’s disease: a systematic review and meta-analysis. Diabetes Care 34, 2614–2623. doi: 10.2337/dc11-1584
Chen, A. Q., Fang, Z., Chen, X. L., Yang, S., Zhou, Y. F., Mao, L., et al. (2019). Microglia-derived TNF-alpha mediates endothelial necroptosis aggravating blood brain-barrier disruption after ischemic stroke. Cell Death Dis. 10:487. doi: 10.1038/s41419-019-1716-9
Chen, X., Lan, X., Roche, I., Liu, R., and Geiger, J. D. (2008). Caffeine protects against MPTP-induced blood-brain barrier dysfunction in mouse striatum. J. Neurochem. 107, 1147–1157. doi: 10.1111/j.1471-4159.2008.05697.x
Chohan, H., Senkevich, K., Patel, R. K., Bestwick, J. P., Jacobs, B. M., Bandres Ciga, S., et al. (2021). Type 2 Diabetes as a Determinant of Parkinson’s Disease Risk and Progression. Mov. Disord. 36, 1420–1429. doi: 10.1002/mds.28551
Croisier, E., Moran, L. B., Dexter, D. T., Pearce, R. K. B., and Graeber, M. B. (2005). Microglial inflammation in the parkinsonian substantia nigra: relationship to alpha-synuclein deposition. J. Neuroinflamm. 2:14. doi: 10.1186/1742-2094-2-14
Desai Bradaric, B., Patel, A., Schneider, J. A., Carvey, P. M., and Hendey, B. (2012). Evidence for angiogenesis in Parkinson’s disease, incidental Lewy body disease, and progressive supranuclear palsy. J. Neural Transm. 119, 59–71. doi: 10.1007/s00702-011-0684-8
Dohgu, S., Takata, F., Matsumoto, J., Kimura, I., Yamauchi, A., and Kataoka, Y. (2019). Monomeric alpha-synuclein induces blood-brain barrier dysfunction through activated brain pericytes releasing inflammatory mediators in vitro. Microvasc. Res. 124, 61–66. doi: 10.1016/j.mvr.2019.03.005
Dore-Duffy, P., and Cleary, K. (2011). Morphology and properties of pericytes. Methods Mol. Biol. 686, 49–68. doi: 10.1007/978-1-60761-938-3_2
Dorsey, E. R., and Bloem, B. R. (2018). The Parkinson Pandemic-A Call to Action. JAMA Neurol. 75, 9–10. doi: 10.1001/jamaneurol.2017.3299
Duan, L., Zhang, X. D., Miao, W. Y., Sun, Y. J., Xiong, G., Wu, Q., et al. (2018). PDGFRbeta Cells Rapidly Relay Inflammatory Signal from the Circulatory System to Neurons via Chemokine CCL2. Neuron 100, 183–200.e8. doi: 10.1016/j.neuron.2018.08.030
Duz, B., Oztas, E., Erginay, T., Erdogan, E., and Gonul, E. (2007). The effect of moderate hypothermia in acute ischemic stroke on pericyte migration: an ultrastructural study. Cryobiology 55, 279–284. doi: 10.1016/j.cryobiol.2007.08.009
Edwards, D. N., Salmeron, K., Lukins, D. E., Trout, A. L., Fraser, J. F., and Bix, G. J. (2020). Integrin alpha5beta1 inhibition by ATN-161 reduces neuroinflammation and is neuroprotective in ischemic stroke. J. Cereb. Blood Flow Metab. 40, 1695–1708. doi: 10.1177/0271678X19880161
Elabi, O., Gaceb, A., Carlsson, R., Padel, T., Soylu-Kucharz, R., Cortijo, I., et al. (2021). Human alpha-synuclein overexpression in a mouse model of Parkinson’s disease leads to vascular pathology, blood brain barrier leakage and pericyte activation. Sci. Rep. 11:1120. doi: 10.1038/s41598-020-80889-8
Elabi, O. F., Cunha, J., Gaceb, A., Fex, M., and Paul, G. (2021). High-fat diet-induced diabetes leads to vascular alterations, pericyte reduction, and perivascular depletion of microglia in a 6-OHDA toxin model of Parkinson disease. J. Neuroinflammation 18:175. doi: 10.1186/s12974-021-02218-8
Farkas, E., De Jong, G. I., de Vos, R. A. I., Steur, E. N. H. J., and Luiten, P. G. M. (2000). Pathological features of cerebral cortical capillaries are doubled in Alzheimer’s disease and Parkinson’s disease. Acta Neuropathol. 100, 395–402. doi: 10.1007/s004010000195
Faucheux, B. A., Agid, Y., Hirsch, E. C., and Bonnet, A.-M. (1999). Blood vessels change in the mesencephalon of patients with Parkinson’s disease. Lancet 353, 981–982. doi: 10.1016/s0140-6736(99)00641-8
Fearnley, J. M., and Lees, A. J. (1991). Ageing and Parkinson’s disease: substantia nigra regional selectivity. Brain 114, 2283–2301. doi: 10.1093/brain/114.5.2283
Finley, K. H. (1936). Angio-architecture of the substantia nigra and its pathogenic significance. Arch. Neurol. Psychiatry 36, 118–127. doi: 10.1001/archneurpsyc.1936.02260070126010
Foltynie, T., and Athauda, D. (2020). Repurposing anti-diabetic drugs for the treatment of Parkinson’s disease: rationale and clinical experience. Prog. Brain Res. 252, 493–523. doi: 10.1016/bs.pbr.2019.10.008
Fowler, A. J., Ahn, J., Hebron, M., Chiu, T., Ayoub, R., Mulki, S., et al. (2021). CSF MicroRNAs Reveal Impairment of Angiogenesis and Autophagy in Parkinson Disease. Neurol. Genet. 7:e633. doi: 10.1212/NXG.0000000000000633
Fujita, K., Peng, S., Ma, Y., Tang, C. C., Hellman, M., Feigin, A., et al. (2021). Blood-brain barrier permeability in Parkinson’s disease patients with and without dyskinesia. J. Neurol. 268, 2246–2255. doi: 10.1007/s00415-021-10411-1
Gaceb, A., Ozen, I., Padel, T., Barbariga, M., and Paul, G. (2018). Pericytes secrete pro-regenerative molecules in response to platelet-derived growth factor-BB. J. Cereb. Blood Flow Metab. 38, 45–57. doi: 10.1177/0271678X17719645
Gaceb, A., and Paul, G. (2018). Pericyte Secretome. Adv. Exp. Med. Biol. 1109, 139–163. doi: 10.1007/978-3-030-02601-1_11
Garbuzova-Davis, S., and Sanberg, P. R. (2014). Blood-CNS Barrier Impairment in ALS patients versus an animal model. Front. Cell Neurosci. 8:21. doi: 10.3389/fncel.2014.00021
Gonul, E., Duz, B., Kahraman, S., Kayali, H., Kubar, A., and Timurkaynak, E. (2002). Early pericyte response to brain hypoxia in cats: an ultrastructural study. Microvasc. Res. 64, 116–119. doi: 10.1006/mvre.2002.2413
Gray, M. T., and Woulfe, J. M. (2015). Striatal blood-brain barrier permeability in Parkinson’s disease. J. Cereb. Blood Flow Metab. 35, 747–750. doi: 10.1038/jcbfm.2015.32
Guan, J., Pavlovic, D., Dalkie, N., Waldvogel, H. J., O’Carroll, S. J., Green, C. R., et al. (2013). Vascular degeneration in Parkinson’s disease. Brain Pathol. 23, 154–164. doi: 10.1111/j.1750-3639.2012.00628.x
Haddick, P. C. G., Larson, J. L., Rathore, N., Bhangale, T. R., Phung, Q. T., Srinivasan, K., et al. (2017). A Common Variant of IL-6R is Associated with Elevated IL-6 Pathway Activity in Alzheimer’s Disease Brains. J. Alzheimers Dis. 56, 1037–1054. doi: 10.3233/Jad-160524
Hansen, C., Bjorklund, T., Petit, G. H., Lundblad, M., Murmu, R. P., Brundin, P., et al. (2013). A novel alpha-synuclein-GFP mouse model displays progressive motor impairment, olfactory dysfunction and accumulation of alpha-synuclein-GFP. Neurobiol. Dis. 56, 145–155. doi: 10.1016/j.nbd.2013.04.017
Haruwaka, K., Ikegami, A., Tachibana, Y., Ohno, N., Konishi, H., Hashimoto, A., et al. (2019). Dual microglia effects on blood brain barrier permeability induced by systemic inflammation. Nat. Commun. 10:5816. doi: 10.1038/s41467-019-13812-z
Heinzel, S., Berg, D., Gasser, T., Chen, H., Yao, C., Postuma, R. B., et al. (2019). Update of the MDS research criteria for prodromal Parkinson’s disease. Mov. Disord. 34, 1464–1470. doi: 10.1002/mds.27802
Huber, J. D., VanGilder, R. L., and Houser, K. A. (2006). Streptozotocin-induced diabetes progressively increases blood-brain barrier permeability in specific brain regions in rats. Am. J. Physiol. Heart Circ. Physiol. 291, H2660–H2668. doi: 10.1152/ajpheart.00489.2006
Iadecola, C. (2017). The Neurovascular Unit Coming of Age: a Journey through Neurovascular Coupling in Health and Disease. Neuron 96, 17–42. doi: 10.1016/j.neuron.2017.07.030
Issidorides, M. R. (1971). Neuronal vascular relationships in the zona compacta of normal and parkinsonian substantia nigra. Brain Res. 25, 289–299. doi: 10.1016/0006-8993(71)90439-2
Jain, R. K., and Booth, M. F. (2003). What brings pericytes to tumor vessels? J. Clin. Invest. 112, 1134–1136. doi: 10.1172/JCI20087
Janelidze, S., Lindqvist, D., Francardo, V., Hall, S., Zetterberg, H., Blennow, K., et al. (2015). Increased CSF biomarkers of angiogenesis in Parkinson disease. Neurology 85, 1834–1842. doi: 10.1212/WNL.0000000000002151
Kam, T. I., Hinkle, J. T., Dawson, T. M., and Dawson, V. L. (2020). Microglia and astrocyte dysfunction in parkinson’s disease. Neurobiol. Dis. 144:105028. doi: 10.1016/j.nbd.2020.105028
Kamouchi, M., Ago, T., Kuroda, J., and Kitazono, T. (2012). The possible roles of brain pericytes in brain ischemia and stroke. Cell Mol. Neurobiol. 32, 159–165. doi: 10.1007/s10571-011-9747-5
Kortekaas, R., Leenders, K. L., van Oostrom, J. C. H., Vaalburg, W., Bart, J., Willemsen, A. T. M., et al. (2005). Blood-brain barrier dysfunction in Parkinsonian midbrain in vivo. Ann. Neurol. 57, 176–179. doi: 10.1002/ana.20369
Lan, G., Wang, P., Chan, R. B., Liu, Z., Yu, Z., Liu, X., et al. (2021). Astrocytic VEGFA: an essential mediator in blood-brain-barrier disruption in Parkinson’s disease. Glia 70, 337–353. doi: 10.1002/glia.24109
Lee, H. J., Kim, C., and Lee, S. J. (2010). Alpha-synuclein stimulation of astrocytes Potential role for neuroinflammation and neuroprotection. Oxid. Med. Cell. Longev. 3, 283–287. doi: 10.4161/oxim.3.4.12809
Machida, T., Takata, F., Matsumoto, J., Miyamura, T., Hirata, R., Kimura, I., et al. (2017). Contribution of thrombin-reactive brain pericytes to blood-brain barrier dysfunction in an in vivo mouse model of obesity-associated diabetes and an in vitro rat model. PLoS One 12:e0177447. doi: 10.1371/journal.pone.0177447
Mcgeer, P. L., Itagaki, S., Boyes, B. E., and Mcgeer, E. G. (1988). Reactive Microglia Are Positive for Hla-Dr in the Substantia Nigra of Parkinsons and Alzheimers-Disease Brains. Neurology 38, 1285–1291. doi: 10.1212/Wnl.38.8.1285
McGeer, P. L., and McGeer, E. G. (2008). Glial reactions in Parkinson’s disease. Mov. Disord. 23, 474–483. doi: 10.1002/mds.21751
Merlini, M., Rafalski, V. A., Rios Coronado, P. E., Gill, T. M., Ellisman, M., Muthukumar, G., et al. (2019). Fibrinogen Induces Microglia-Mediated Spine Elimination and Cognitive Impairment in an Alzheimer’s Disease Model. Neuron 101, 1099–1108.e6. doi: 10.1016/j.neuron.2019.01.014
Naldini, A., and Carraro, F. (2005). Role of inflammatory mediators in angiogenesis. Curr. Drug Targets Inflamm. Allergy 4, 3–8. doi: 10.2174/1568010053622830
Ni, P. F., Dong, H. Q., Wang, Y. W., Zhou, Q., Xu, M. M., Qian, Y. N., et al. (2018). IL-17A contributes to perioperative neurocognitive disorders through blood-brain barrier disruption in aged mice. J. Neuroinflammation 15:332. doi: 10.1186/s12974-018-1374-3
Ozen, I., Deierborg, T., Miharada, K., Padel, T., Englund, E., Genove, G., et al. (2014). Brain pericytes acquire a microglial phenotype after stroke. Acta Neuropathol. 128, 381–396. doi: 10.1007/s00401-014-1295-x
Ozerdem, U., and Stallcup, W. B. (2004). Pathological angiogenesis is reduced by targeting pericytes via the NG2 proteoglycan. Angiogenesis 7, 269–276. doi: 10.1007/s10456-004-4182-6
Padel, T., Ozen, I., Boix, J., Barbariga, M., Gaceb, A., Roth, M., et al. (2016). Platelet-derived growth factor-BB has neurorestorative effects and modulates the pericyte response in a partial 6-hydroxydopamine lesion mouse model of Parkinson’s disease. Neurobiol. Dis. 94, 95–105. doi: 10.1016/j.nbd.2016.06.002
Padel, T., Roth, M., Gaceb, A., Li, J. Y., Bjorkqvist, M., and Paul, G. (2018). Brain pericyte activation occurs early in Huntington’s disease. Exp. Neurol. 305, 139–150. doi: 10.1016/j.expneurol.2018.03.015
Pagano, G., Polychronis, S., Wilson, H., Giordano, B., Ferrara, N., Niccolini, F., et al. (2018). Diabetes mellitus and Parkinson disease. Neurology 90, e1654–e1662. doi: 10.1212/WNL.0000000000005475
Pandey, P. K., Sharma, A. K., and Gupta, U. (2016). Blood brain barrier: an overview on strategies in drug delivery, realistic in vitro modeling and in vivo live tracking. Tissue Barriers 4, e1129476. doi: 10.1080/21688370.2015.1129476
Paul, G., and Sullivan, A. M. (2019). Trophic factors for Parkinson’s disease: where are we and where do we go from here? Eur. J. Neurosci. 49, 440–452. doi: 10.1111/ejn.14102
Paul, G., Zachrisson, O., Varrone, A., Almqvist, P., Jerling, M., Lind, G., et al. (2015). Safety and tolerability of intracerebroventricular PDGF-BB in Parkinson’s disease patients. J. Clin. Invest. 125, 1339–1346. doi: 10.1172/Jci79635
Pienaar, I. S., Lee, C. H., Elson, J. L., McGuinness, L., Gentleman, S. M., Kalaria, R. N., et al. (2015). Deep-brain stimulation associates with improved microvascular integrity in the subthalamic nucleus in Parkinson’s disease. Neurobiol. Dis. 74, 392–405. doi: 10.1016/j.nbd.2014.12.006
Pisani, V., Stefani, A., Pierantozzi, M., Natoli, S., Stanzione, P., Franciotta, D., et al. (2012). Increased blood-cerebrospinal fluid transfer of albumin in advanced Parkinson’s disease. J. Neuroinflammation 9:188. doi: 10.1186/1742-2094-9-188
Raymond, A. D., Diaz, P., Chevelon, S., Agudelo, M., Yndart-Arias, A., Ding, H., et al. (2016). Microglia-derived HIV Nef+ exosome impairment of the blood-brain barrier is treatable by nanomedicine-based delivery of Nef peptides. J. Neurovirol. 22, 129–139. doi: 10.1007/s13365-015-0397-0
Reynolds, A. D., Stone, D. K., Hutter, J. A. L., Benner, E. J., Mosley, R. L., and Gendelman, H. E. (2010). Regulatory T Cells Attenuate Th17 Cell-Mediated Nigrostriatal Dopaminergic Neurodegeneration in a Model of Parkinson’s Disease. J. Immunol. 184, 2261–2271. doi: 10.4049/jimmunol.0901852
Ritzel, R. M., Patel, A. R., Grenier, J. M., Crapser, J., Verma, R., Jellison, E. R., et al. (2015). Functional differences between microglia and monocytes after ischemic stroke. J. Neuroinflammation 12:106. doi: 10.1186/s12974-015-0329-1
Rom, S., Zuluaga-Ramirez, V., Gajghate, S., Seliga, A., Winfield, M., Heldt, N. A., et al. (2019). Hyperglycemia-Driven Neuroinflammation Compromises BBB Leading to Memory Loss in Both Diabetes Mellitus (DM) Type 1 and Type 2 Mouse Models. Mol. Neurobiol. 56, 1883–1896. doi: 10.1007/s12035-018-1195-5
Rustenhoven, J., Jansson, D., Smyth, L. C., and Dragunow, M. (2017). Brain Pericytes As Mediators of Neuroinflammation. Trends Pharmacol. Sci. 38, 291–304. doi: 10.1016/j.tips.2016.12.001
Sagare, A. P., Bell, R. D., Zhao, Z., Ma, Q., Winkler, E. A., Ramanathan, A., et al. (2013). Pericyte loss influences Alzheimer-like neurodegeneration in mice. Nat. Commun. 4:2932. doi: 10.1038/ncomms3932
Salameh, T. S., Shah, G. N., Price, T. O., Hayden, M. R., and Banks, W. A. (2016). Blood-Brain Barrier Disruption and Neurovascular Unit Dysfunction in Diabetic Mice: protection with the Mitochondrial Carbonic Anhydrase Inhibitor Topiramate. J. Pharmacol. Exp. Ther. 359, 452–459. doi: 10.1124/jpet.116.237057
Salter, M. W., and Stevens, B. (2017). Microglia emerge as central players in brain disease. Nat. Med. 23, 1018–1027. doi: 10.1038/nm.4397
Sergi, D., Renaud, J., Simola, N., and Martinoli, M. G. (2019). Diabetes, a Contemporary Risk for Parkinson’s Disease: epidemiological and Cellular Evidences. Front. Aging Neurosci. 11:302. doi: 10.3389/fnagi.2019.00302
Spillantini, M. G., Schmidt, M. L., Lee, V. M., Trojanowski, J. Q., Jakes, R., and Goedert, M. (1997). Alpha-synuclein in Lewy bodies. Nature 388, 839–840. doi: 10.1038/42166
Stapor, P. C., Sweat, R. S., Dashti, D. C., Betancourt, A. M., and Murfee, W. L. (2014). Pericyte dynamics during angiogenesis: new insights from new identities. J. Vasc. Res. 51, 163–174. doi: 10.1159/000362276
Starr, J. M., Wardlaw, J., Ferguson, K., MacLullich, A., Deary, I. J., and Marshall, I. (2003). Increased blood-brain barrier permeability in type II diabetes demonstrated by gadolinium magnetic resonance imaging. J. Neurol. Neurosurg. Psychiatry 74, 70–76. doi: 10.1136/jnnp.74.1.70
Sweeney, M. D., Kisler, K., Montagne, A., Toga, A. W., and Zlokovic, B. V. (2018a). The role of brain vasculature in neurodegenerative disorders. Nat. Neurosci. 21, 1318–1331. doi: 10.1038/s41593-018-0234-x
Sweeney, M. D., Sagare, A. P., and Zlokovic, B. V. (2018b). Blood-brain barrier breakdown in Alzheimer disease and other neurodegenerative disorders. Nat. Rev. Neurol. 14, 133–150. doi: 10.1038/nrneurol.2017.188
Tahergorabi, Z., and Khazaei, M. (2012). A review on angiogenesis and its assays. Iran. J. Basic Med. Sci. 15, 1110–1126.
Takechi, R., Lam, V., Brook, E., Giles, C., Fimognari, N., Mooranian, A., et al. (2017). Blood-Brain Barrier Dysfunction Precedes Cognitive Decline and Neurodegeneration in Diabetic Insulin Resistant Mouse Model: an Implication for Causal Link. Front. Aging Neurosci. 9:399. doi: 10.3389/fnagi.2017.00399
Wada, K., Arai, H., Takanashi, M., Fukae, J., Oizumi, H., Yasuda, T., et al. (2006). Expression levels of vascular endothelial growth factor and its receptors in Parkinson’s disease. Neuroreport 17, 705–709. doi: 10.1097/01.wnr.0000215769.71657.65
Wang, Y., Jin, S., Sonobe, Y., Cheng, Y., Horiuchi, H., Parajuli, B., et al. (2014). Interleukin-1 beta Induces Blood-Brain Barrier Disruption by Downregulating Sonic Hedgehog in Astrocytes. PLoS One 9:e110024. doi: 10.1371/journal.pone.0110024
Westin, J. E., Lindgren, H. S., Gardi, J., Nyengaard, J. R., Brundin, P., Mohapel, P., et al. (2006). Endothelial proliferation and increased blood-brain barrier permeability in the basal ganglia in a rat model of 3,4-dihydroxyphenyl-L-alanine-induced dyskinesia. J. Neurosci. 26, 9448–9461. doi: 10.1523/JNEUROSCI.0944-06.2006
Whitton, P. S. (2007). Inflammation as a causative factor in the aetiology of Parkinson’s disease. Br. J. Pharmacol. 150, 963–976. doi: 10.1038/sj.bjp.0707167
Winkler, E. A., Sengillo, J. D., Sagare, A. P., Zhao, Z., Ma, Q., Zuniga, E., et al. (2014). Blood-spinal cord barrier disruption contributes to early motor-neuron degeneration in ALS-model mice. Proc. Natl. Acad. Sci. U. S. A. 111, E1035–E1042. doi: 10.1073/pnas.1401595111
Yamamoto, M., Guo, D. H., Hernandez, C. M., and Stranahan, A. M. (2019). Endothelial Adora2a Activation Promotes Blood-Brain Barrier Breakdown and Cognitive Impairment in Mice with Diet-Induced Insulin Resistance. J. Neurosci. 39, 4179–4192. doi: 10.1523/JNEUROSCI.2506-18.2019
Yang, P., Pavlovic, D., Waldvogel, H., Dragunow, M., Synek, B., Turner, C., et al. (2015). String Vessel Formation is Increased in the Brain of Parkinson Disease. J. Parkinsons Dis. 5, 821–836. doi: 10.3233/JPD-140454
Yasuda, T., Fukuda-Tani, M., Nihira, T., Wada, K., Hattori, N., Mizuno, Y., et al. (2007). Correlation between levels of pigment epithelium-derived factor and vascular endothelial growth factor in the striatum of patients with Parkinson’s disease. Exp. Neurol. 206, 308–317. doi: 10.1016/j.expneurol.2007.05.012
Zachrisson, O., Zhao, M., Andersson, A., Dannaeus, K., Haggblad, J. H., Isacson, R., et al. (2011). Restorative Effects of Platelet Derived Growth Factor-BB in Rodent Models of Parkinson’s Disease. J. Parkinsons Dis. 1, 49–63. doi: 10.3233/Jpd-2011-0003
Zhang, W., Wang, T. G., Pei, Z., Miller, D. S., Wu, X. F., Block, M. L., et al. (2005). Aggregated alpha-synuclein activates microglia: a process leading to disease progression in Parkinson’s disease. FASEB J. 19, 533–542. doi: 10.1096/fj.04-2751com
Zhao, C. H., Ling, Z. D., Newman, M. B., Bhatia, A., and Carvey, P. M. (2007). TNF-alpha knockout and minocycline treatment attenuates blood-brain barrier leakage in MPTP-treated mice. Neurobiol. Dis. 26, 36–46. doi: 10.1016/j.nbd.2006.11.012
Zhao, Z., Nelson, A. R., Betsholtz, C., and Zlokovic, B. V. (2015). Establishment and Dysfunction of the Blood-Brain Barrier. Cell 163, 1064–1078. doi: 10.1016/j.cell.2015.10.067
Keywords: Parkinson’s disease, vasculature, pericytes, angiogenesis, blood-brain barrier, microglia
Citation: Paul G and Elabi OF (2022) Microvascular Changes in Parkinson’s Disease- Focus on the Neurovascular Unit. Front. Aging Neurosci. 14:853372. doi: 10.3389/fnagi.2022.853372
Received: 12 January 2022; Accepted: 11 February 2022;
Published: 10 March 2022.
Edited by:
Axel Montagne, University of Edinburgh, United KingdomReviewed by:
Fumitaka Shimizu, Yamaguchi University School of Medicine, JapanSarah Al-Bachari, Lancaster University, United Kingdom
Copyright © 2022 Paul and Elabi. This is an open-access article distributed under the terms of the Creative Commons Attribution License (CC BY). The use, distribution or reproduction in other forums is permitted, provided the original author(s) and the copyright owner(s) are credited and that the original publication in this journal is cited, in accordance with accepted academic practice. No use, distribution or reproduction is permitted which does not comply with these terms.
*Correspondence: Gesine Paul, gesine.paul-visse@med.lu.se