Deciphering the Link Between ERUPR Signaling and MicroRNA in Pathogenesis of Alzheimer’s Disease
- 1Amity Institute of Biotechnology, Amity University Rajasthan, Jaipur, India
- 2Department of Pharmacology and Toxicology, College of Pharmacy, Umm Al-Qura University, Makkah, Saudi Arabia
- 3Department of Pharmaceutical Chemistry, College of Pharmacy, Taif University, Taif, Saudi Arabia
- 4Addiction and Neuroscience Research Unit, College of Pharmacy, Taif University, Taif, Saudi Arabia
- 5Research and Scientific Studies Unit, College of Nursing and Allied Health Sciences, Jazan University, Jazan, Saudi Arabia
- 6Faculty of Medicine, Bursa Uludağ University, Bursa, Turkey
Alzheimer’s disease (AD) is a neurodegenerative proteinopathic disease. The deposits of misfolded Amyloid β and Tau proteins in the brain of patients with AD suggest an imbalance in endoplasmic reticulum (ER) proteostasis. ER stress is due to accumulation of aberrant proteins in the ER lumen, which then leads to activation of three sensor protein pathways that ultimately evokes the adaptive mechanism of the unfolded protein response (UPR). The UPR mechanism operates via adaptive UPR and the apoptotic UPR. Adaptive UPR tries to restore imbalance in ER hemostasis by decreasing protein production, enhanced chaperone involvement to restore protein folding, misfolded protein decay by proteasome, and suppression of ribosomal translation ultimately relieving the excessive protein load in the ER. Subsequently, apoptotic UPR activated under severe ER stress conditions triggers cell death. MicroRNAs (miRNAs) are small non-coding protein causing dysregulated translational of mRNAs in a sequential manner. They are considered to be critical elements in the maintenance of numerous cellular activities, hemostasis, and developmental processes. Therefore, upregulation or downregulation of miRNA expression is implicated in several pathogenic processes. Evidence from scientific studies suggest a strong correlation between ERUPR signaling and miRNA dysregulation but the research done is still dormant. In this review, we summarized the cross-talk between ER stress, and the UPR signaling processes and their role in AD pathology by scrutinizing and collecting information from original research and review articles.
Introduction
The endoplasmic reticulum (ER) is a cellular organelle with an imperative role in the processes of folding, modification, and trafficking of nascent proteins (Plácido et al., 2014). ER dysfunction leads to an imbalance in the proteostasis that results in accumulation of abnormal (unfolded/misfolded) proteins, consequently, such proteins trigger a stress response called ER stress (Scheper and Hoozemans, 2015; Rahman et al., 2017). ER dysfunctions/ER stress is considered to be involved in several pathological conditions like metabolic disorders, such as diabetes, neurodegenerative diseases, cancer, atherosclerosis, lung, and renal diseases (Lindholm et al., 2017). ER stress in turn triggers unfolded protein response (UPR), an adaptive rescuer mechanism, through upregulation of molecular chaperones (Fu and Gao, 2014; Alshareef et al., 2021). The UPR acts as two-edged sword it can either exhibit cytoprotective action or cytotoxic action. Cytoprotection is exhibited through the revival of proteostatic balance, and cellular adaptations essential for ER protein folding. However, prolonged ER stress-related perturbations might enhance UPR led cytotoxicity and hence apoptosis (Gardner et al., 2013).
Proteostatic defects augment the accretion of misfolded protein, to which neuronal cells are sensitive. Consequently, they survive and evade toxicity by utilizing the ER-induced UPR mechanism (Remondelli and Renna, 2017). Misfolded protein aggregates may cause neuronal toxicity, which is regarded as the crucial step in the pathogenesis of neurodegenerative diseases (Spires-Jones et al., 2017). Alzheimer’s disease (AD) is a type of misfolded-protein disease. AD is an age-related neurodegenerative disease regarded as the most common cause of dementia (Abduljaleel et al., 2014; Scheper and Hoozemans, 2015; Rahman et al., 2018). The prominent neuropathological features of AD include Neuritic plaque (NP) besides neurofibrillary tangle (NFT). NPs are extracellular aggregates of amyloid-β (Aβ) peptides (Labban et al., 2021). Furthermore, dystrophic neurites and neuropil threads along with interneuronal aggregates of hyperphosphorylated Tau proteins in neurofibrillary tangles are other hallmark features of AD (Tanprasertsuk et al., 2019). Katayama et al., in their study, reported that mutation in presenilin-1 (PS1), and induction of high-mobility group A1a (HMGA1a) protein by Presenilin-2 (PS2) isoform, i.e., PS2V, along with the activation of Caspase-4 are all responsible for the neuronal cell death in AD. Eventually, this is attributed to hyperactivity of the protein translation mechanism accompanied by Aβ accumulation in neurons all of which lead to aberrant ER stress (Katayama et al., 2004; Wu Q. et al., 2016; Hashimoto and Saido, 2018).
MicroRNAs (miRNAs) are non-coding 19–25 nucleotides long, single-stranded protein molecules that are endogenously synthesized, evolutionarily conserved (Rahman et al., 2021; Yasmeen et al., 2021). miRNA mediates post-translational gene regulation either by promoting mRNA decay or repressing protein translation (Wang M. et al., 2019). The role of miRNAs is imperative in several cellular processes, such as cellular proliferation, cell differentiation, apoptosis, and in neural cell biology. Local protein synthesis to crucial to maintain neuronal function and synaptic plasticity. The existence of several hundreds of translationally silent mRNAs in axons their activation via stimuli leads to their subsequent protein synthesis specific to that cellular process (Kim and Jung, 2015). miRNAs are located in cell soma, dendrites, and synaptosomes, they control GluA1 or GluA2 subunits of AMPA-type glutamate receptor (AMPAR) along with GABAARs (α1 and γ2) crucial for synaptic coordination and long-term potentiation. Hence, miRNAs are regarded as key regulators of local protein synthesis during synaptic plasticity. In short, neuronal protein translation is also regulated by miRNAs (Smalheiser and Lugli, 2009; Rajgor et al., 2021). Not only this, but they are also implicated in several pathological processes, such as cancer, diabetes, and neurodegenerative diseases (Grasso et al., 2014). The role of miRNAs in regulating ER stress is the foundation to unravel following several studies. However, significant contributions are required to understand the mechanism involved behind this. Numerous revelations are being made in recent times, regarding the influence of miRNA dysregulation in neuronal disease. Several miRNA families for instance miR-485 were found to be dysregulated in AD and other neurological diseases. In this review, we summarize the connection between miRNAs and ERUPR signaling pathways that play a vital role in AD.
ERUPR Signaling
The ER is a membrane-bound organelle that plays an important role in the synthesis, folding, maturation, proper trafficking, quality control, degradation, and post-translational modifications of nascent proteins. It is also involved in several other cellular events, such as calcium homeostasis, cholesterol homeostasis in endosomes, and in cytoplasmic streaming (Almanza et al., 2019). ER quality control helps in preserving proteostasis. ER-associated degradation (ERAD) is a process that helps in disposing of terminally misfolded proteins through ubiquitin–proteasome system (Oku et al., 2021). When the misfolded proteins are overloaded the prototypical ER-stress sensors located at the ER membrane activate UPR. The UPR activation is mediated by an intricate network of three main signaling pathways: inositol-requiring transmembrane kinase/endonucleases (IRE1α and -β), protein kinase RNA (PKR)-like endoplasmic reticulum kinase (PERK), and the activating transcription factor-6 (ATF6α and -β) (Dufey et al., 2014). Under normal physiological conditions, these stress-sensor proteins bind to BiP/GRP78 an ER-resident chaperone forming a complex, which remains dormant (Hoozemans et al., 2012). Whereas, following ER stress this complex dissociates and individual proteins are activated and influence downstream signaling. The signaling pathways involved in UPR are depicted in Figure 1.
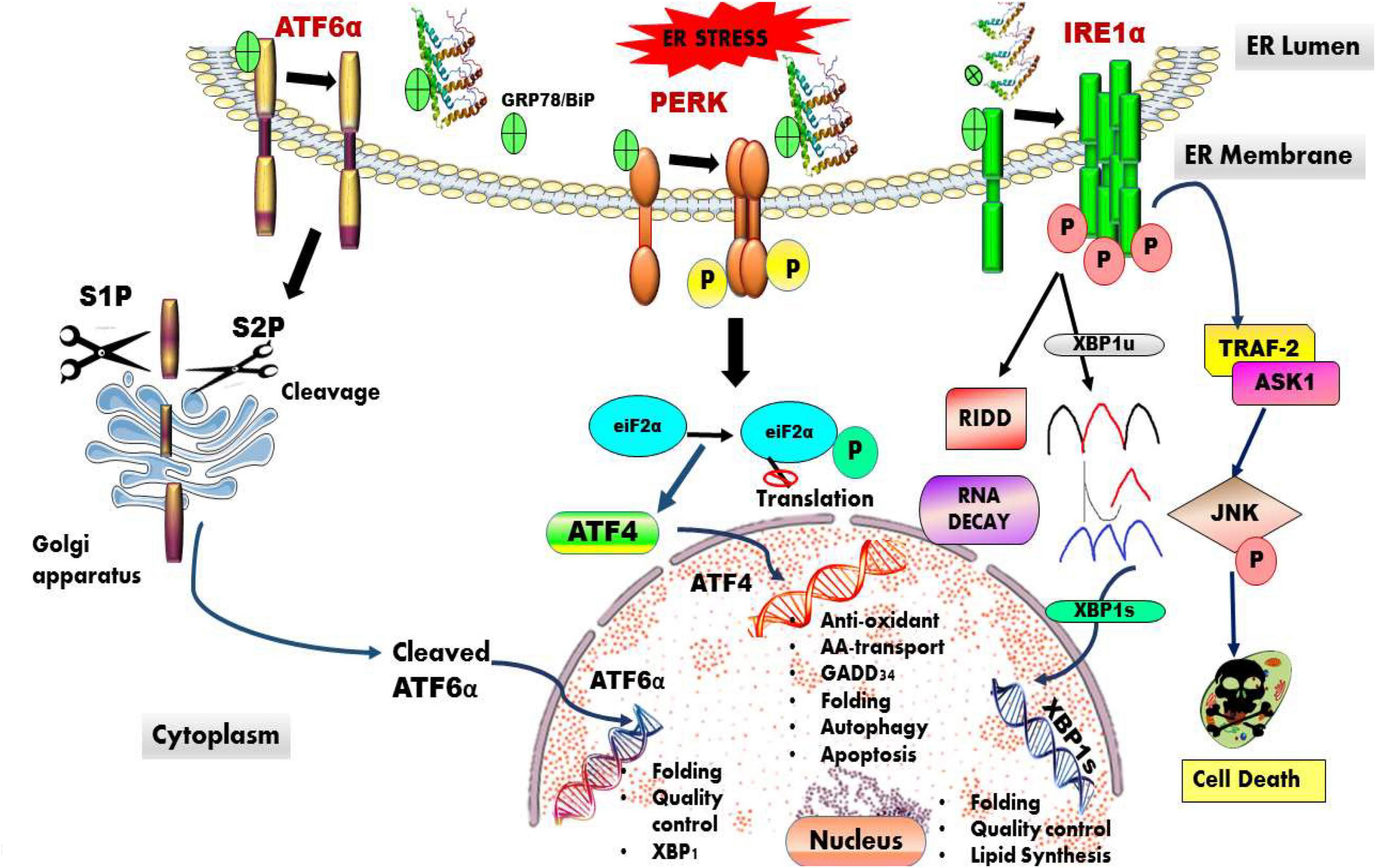
Figure 1. Overview of ER-induced UPR stress signaling pathway. The UPR is an adaptive mechanism activated in response to ER stress. The three UPR stress sensing proteins that are activated are IRE1, PERK, and ATF6, which work independently with an aim to maintain protestatic balance via decreasing the load of misfolded proteins and restoring ER protein homeostasis.
IRE1 Pathway
Inositol-requiring transmembrane kinase/endonucleases 1 (IRE1) is a highly conserved, type I transmembrane protein. In ER-stress conditions, IRE1 self-associates during which BiP/GRP78 chaperone is displaced by Hsp47, from the complex called IRE1 UPRosome and promotes oligomerization (Almanza et al., 2019). The activation of cytosolic kinase domain and IRE1 RNase domain is through conformational change and autophosphorylation. Activation of IRE1 further activates UPR downstream signaling via the splicing of X-box binding protein 1 (Xbp-1) mRNA, which is a basic leucine zipper (bZIP) transcription factor (Gerakis and Hetz, 2018). Spliced XBP1 (sXBP1) mRNA mediates transactivation of genes, such as GRP78/BiP essential for proteostasis, lipid biosynthesis, redox metabolism, and glucose metabolism. Furthermore, the activated RNase domain of IRE1 leads to activation of regulated IRE1-dependent decay (RIDD). RIDD pathway activation degrades mRNAs and miRNAs, decreasing the load of nascent proteins entering ER. Hence, essential for preserving ER homeostasis and cell survival (Siwecka et al., 2021). Under ER stress conditions, IRE1α induces TRAF 2 and ASK1 [tumor necrosis factor (TNF) receptor-associated factor 2; apoptosis signal-regulating kinase 1], respectively. Subsequently, the IRE1α-TRAF2-ASK1 complex formed activates several other pathways, such as JNK (c-Jun N-terminal kinase), MAPK (p38 mitogen-activated protein kinase), and procaspase 12 pathways, which ultimately lead to apoptosis. Hence, IRE1α pathway is regarded as the main trigger of apoptosis (Chen and Brandizzi, 2013; Wu J. et al., 2016; Junjappa et al., 2018).
PERK Pathway
Protein kinase RNA (PKR)-like endoplasmic reticulum kinase is a kinase protein that is ubiquitously expressed lodges ER luminal domain and a cytoplasmic kinase domain. Under ER-stress conditions, the PERK pathway is activated when BiP/GRP78 chaperone and PERK complex dissociates, which leads to oligomerization, additionally, its cytosolic kinase domain is subjected to trans-auto phosphorylation. PERK activation phosphorylates eukaryotic translation initiation factor-2α(eIF2α) on serine 51 causing downstream signaling of eIF2α (Wang et al., 2018). Phosphorylation of eIF2α is involved in regulation of protein synthesis, also inhibits eIF2B activity, which blocks protein translation and hence reduces the protein load. eIF2α phosphorylation translates mRNA having short upstream open reading frames (uORFs) that activates ATF4, which in turn induces GADD34 (growth arrest and DNA damage-inducible34) and C/EBP homologous protein (CHOP) (Pakos-Zebrucka et al., 2016). GADD34 is a regulatory subunit of protein phosphatase (PP1C) complex, it acts as a negative feedback mechanism, as it dephosphorylates eIF2α in the PERK pathway, while CHOP is a proapoptotic factor. Overall PERK/ATF4 signaling is involved in redox homeostasis, protein folding, amino acid biosynthesis, autophagy, and apoptosis. PERK pathway is prosurvival and transitions to proapoptotic under critical ER-stress conditions (Gardner et al., 2013).
ATF6 Pathway
Activating transcription factor 6 a type II transmembrane protein has stress sensor carboxy-terminal luminal domain and an amino-terminal domain, which is a bZip transcription factor (Stauffer et al., 2020). ER-stress conditions activate ATF6, which translocate from the ER to the Golgi, where it is cleaved sequentially by the site-1 and site-2 proteases (S1Pand S2P) releasing the cytosolic amino terminus ATF6-N fragment. ATF6-N later binds to cis-acting ER stress response elements (ERSE) in the nucleus. ATF6 up-regulates gene expression of foldases, chaperones, XBP-1, and ERAD pathway also facilitates cytoprotection, suppressing the UPR-induced apoptotic program (Ibe et al., 2021). Figure 1 depicts the various pathways activated in ER proteotoxic stress conditions.
MicroRNAs Implicated in Alzheimer’s Disease
Alzheimer’s disease is a neurodegenerative disease with dual proteinopathy characterized by deposits of neuritic ß-amyloid (Aß) plaques and deposits of hyper-phosphorylated, microtubule stabilizing protein called tau protein in NFTs (Bejanin et al., 2017; Sultan et al., 2020). Neuropsychiatric symptoms (NPSs) are prevalent hallmark symptoms in patients with AD. Furthermore, AD is characterized by impairment in cognitive, behavioral, and functional domains with symptoms, such as depression, apathy, cognitive decline, delusion, motor disturbance, and agility (Chen M. L. et al., 2021). miRNAs exhibit crucial role in the pathogenesis of several neurodegenerative diseases, such as AD, they may be either upregulated or downregulated in AD. The onset of AD begins with synaptic dysfunction, which is accompanied by the dysregulation of several miRNAs. miRNAs serve as a promising biomarker to monitor initial, pre-symptomatic phases of AD pathogenesis. Evidence from previous studies suggests that several miRNAs are implicated in the AD pathogenesis by dysregulating functions of Aβ production, Cofilin, APP, BACE1, and Tau phosphorylation levels. There are numerous miRNAs implicated in the pathogenesis of AD some of them are elaborated in the following Table 1.
Connection Between ERUPR Signaling Pathways and miRNAs in Alzheimer’s Disease
MiRNAs are proven to be the most efficient modulators of several cellular pathways. They also play a regulatory role in the major ER-stress sensor pathways like IRE1, ATF6, and PERK pathways. miRNAs help in modulation of the cellular stress response, by regulating UPR key component levels. Krammes et al., 2020 reported that miR-34a-5p plays a vital role in the regulation of the IRE1α branch. Its overexpression targets BIP, IRE1α, and XBP1 genes and subsequently leads to a reduction in IRE1α and XBP1s thereby modulating UPR signaling. In another study, it was reported that protection against Aβ-induced injury in SH-SY5Y cells can be enhanced by miR-34a upregulation and IRE1α inhibition (Li et al., 2019). It is also suggested that a plausible link exists between miR expression levels and Aβ-induced ER stress, they reported that upregulation of miR-200c induced by Aβ deposition is mediated via ER stress pathways, such as PERK and eIF2 α pathways, due to the activation of as XBP1, ATF4, and ATF6 transcriptional factors (Wu J. et al., 2016). The findings of a recent study reported significant alterations in the levels of two miR-99b-5p and miR100-5p via Aβ-induced ER-stress modulation along with alteration in the mTOR pathway. This proved the correlation between the ER stress–miRNAs–mTOR” axis in AD pathogenesis (Ye et al., 2015). Figure 2 depicts ER stress and microRNA relation in AD. From the above studies, it is evident that a significant relationship exists between ER stress pathways—miRNAs axis but the exact mechanism still remains elusive and needs further elaborative research to gather substantial evidence.
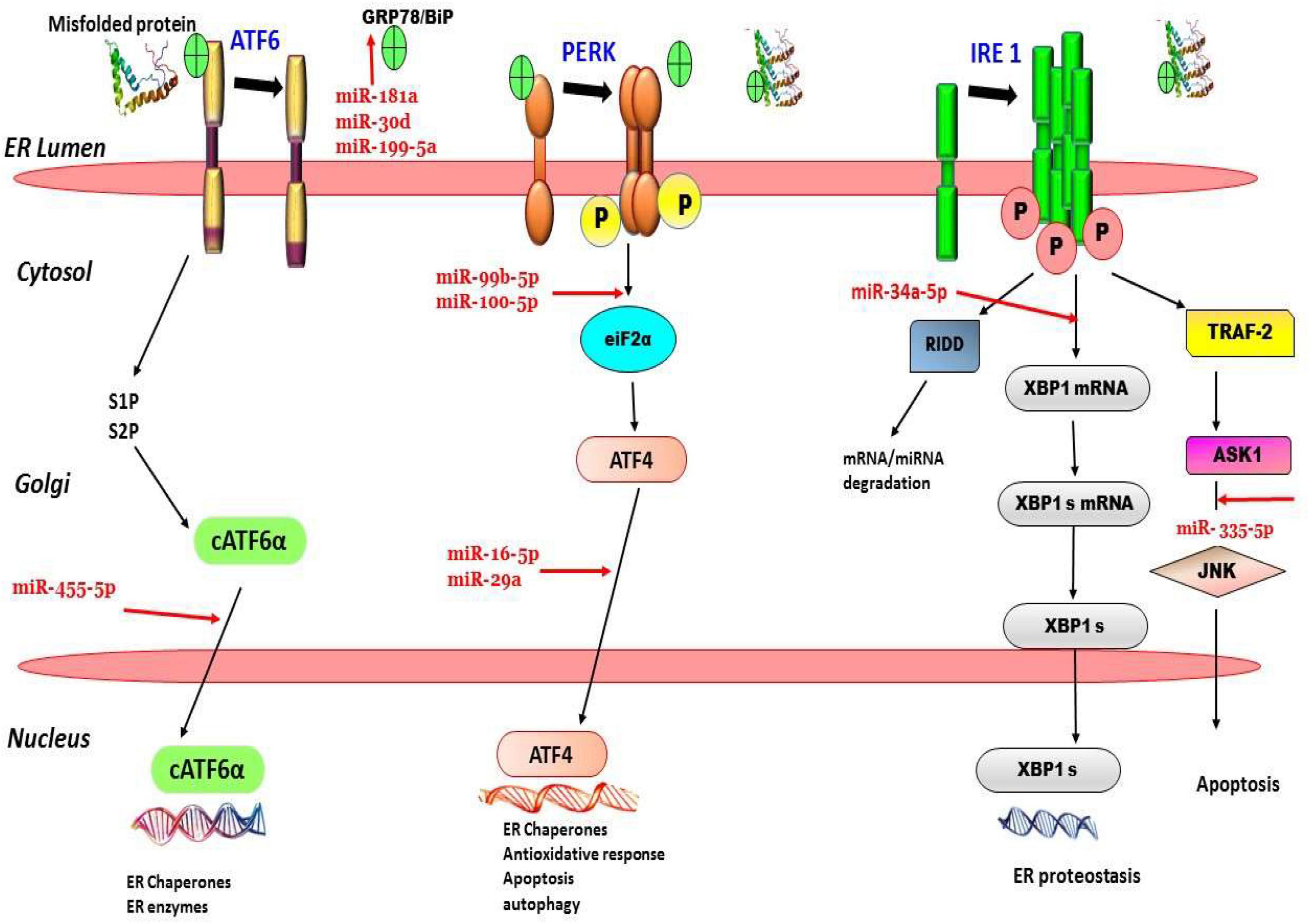
Figure 2. ER stress, UPR, and microRNA relation in Alzheimer’s disease. For instance, evidence suggests that, miR-199-5a, miR-30a and miR-181 suppress GRP78 expression. miR-34a-5p downregulates XBP-1 expression in. miR-335 is involved in activation of JNK pathway leading to apoptosis, etc., can be seen in the figure.
Therapeutic Approaches Against Alzheimer’s Disease Involving the ERUPR Signaling Pathway and MicroRNA
Alzheimer’s disease pathobiology is complex and still elusive. Although, NFT, Aβ, and tau proteins are regarded as the markers of this disease, treatment approaches are dormant. The first drug ever known to treat cognitive symptoms of AD is Tacrine, which is currently withdrawn due to toxicity issues. Later many drugs targeting cholinesterase, NMDA receptors were introduced which could only alleviate symptoms. The year 2021 saw a major breakthrough with the approval of drug, Aducanumab, an IgG1 monoclonal antibody targeted to treat the underlying cause of disease (Riscado et al., 2021). Another drug Verubecestat (MK-8931) targeting beta-secretase 1 (BACE1), was supposedly being investigated to treat AD; however, this was terminated due to no promising results (Kennedy et al., 2016). miRNAs are identified as efficient diagnostic biomarkers for AD, they target multiple genes and multiple pathologic pathways in one stance. Hence, miRNAs are regarded as potential therapeutic targets for several diseases, including AD. However, there are several limitations to develop miRNA-based therapeutic agents, like difficulty in identification of the functional target gene, factors influencing variations in miRNA expression, decay kinetics of miRNA in body fluids, passage across BBB, the delivery options of miRNA in their stable form, toxicity, and immune response (Madadi et al., 2019). Scientists around the world are working tremendously to overcome these hurdles. Currently, encouraging developments are seen targeting diseases, using miRNA mimics, miRNA sponges/decoys, antagomiRs, miRNA antisense nucleotides, antagoNAT and miRNA-based oligonucleotides (Bajan and Hutvagner, 2020). Also, delivery of these miRNA, which is a hurdle, is being worked on using novel targeted delivery approaches, such as conjugated nanoparticle, intra-nasal administration, intracerebroventricular infusion, lipid, and viral vectors (Fu et al., 2019). The first drug developed using miRNA targeting is Miravirsen, miRNA oligonucleotide to treat hepatitis C, which is currently in phase II clinical trials (Baek et al., 2014). Despite such incredible efforts by the scientific fraternity no approved disease-modifying miRNA-based AD therapies 0 exists, no drug have been FDA approved as yet. However, several miRNA-based drugs have managed to reach phase I and phase II clinical trials. These lacunae need to be addressed as early as possible so as to enable AD treatment with novel therapeutic approaches.
Conclusion and Future Perspectives
The endoplasmic reticulum has a very crucial role in several cellular processes with a focus on protein synthesis and transport, proteostasis, redox homeostasis, autophagy, and cellular apoptosis, supposedly disrupted in aberrant conditions ameliorating AD progression. Numerous studies have shown the role of regulatory non-coding proteins miRNA in several pathologies, such as AD. This article reviews the complex, interconnection between non-coding miRNA networks involved in the regulation of ER stress via UPR activation. Hundreds of miRNAs have been discovered and still there is scope for the discovery of miRNAs that are directly involved in altering and regulating ER-induced UPR stress pathways. miRNAs exhibit diversity in their actions, advancements in molecular and RNA sequencing technologies have provided significant insights into the dysregulation in miRNAs in AD and helped us unravel their role as potential diagnostic biomarkers and therapeutic agents. An elaborative analysis of individual miRNAs or clusters of miRNA families targeting ERUPR or ER proteostasis along with miRNA quantification methods must be carried out to precisely know the miRNAs that can serve as excellent biomarkers and therapeutic targets to overcome the disease burden of AD.
Author Contributions
NY, MD, VK, and FA conceptualized the idea of mini-review and were responsible for the entire manuscript in its final form. AA and SH checked the complete manuscript in terms of technical and scientific integrity. All authors contributed to manuscript writing, revision, read, and approved the submitted version.
Funding
The funding was provided by the Deanship of Scientific Research at Umm Al-Qura University for supporting this work by Grant Code: (22UQU4310453DSR01).
Conflict of Interest
The authors declare that the research was conducted in the absence of any commercial or financial relationships that could be construed as a potential conflict of interest.
Publisher’s Note
All claims expressed in this article are solely those of the authors and do not necessarily represent those of their affiliated organizations, or those of the publisher, the editors and the reviewers. Any product that may be evaluated in this article, or claim that may be made by its manufacturer, is not guaranteed or endorsed by the publisher.
Acknowledgments
We would like to thank Director, Amity Institute of Biotechnology, Amity University Rajasthan, Jaipur and the Deanship of Scientific Research at Umm Al-Qura University for providing the needful facilities to write this important manuscript. We also would like to thank the Deanship of Scientific Research at Umm Al-Qura University for supporting this work by Grant Code: (22UQU4310453DSR01).
References
Abduljaleel, Z., Al-Allaf, F. A., Khan, W., Athar, M., Shahzad, N., Taher, M. M., et al. (2014). Evidence of trem2 variant associated with triple risk of Alzheimer’s disease. PLoS One 9:e92648. doi: 10.1371/journal.pone.0092648
Absalon, S., Kochanek, D. M., Raghavan, V., and Krichevsky, A. M. (2013). MiR-26b, upregulated in Alzheimer’s disease, activates cell cycle entry, tau-phosphorylation, and apoptosis in postmitotic neurons. J. Neurosci. 33, 14645–14659. doi: 10.1523/JNEUROSCI.1327-13.2013
Almalki, W. H., Alzahrani, A., Mahmoud El-Daly, M. E., and Fadel Ahmed, A. H. F. (2021). The emerging potential of SIRT-3 in oxidative stress-inflammatory axis associated increased neuroinflammatory component for metabolically impaired neural cell. Chem. Biol. Interact. 333:109328. doi: 10.1016/j.cbi.2020.109328
Almanza, A., Carlesso, A., Chintha, C., Creedican, S., Doultsinos, D., Leuzzi, B., et al. (2019). Endoplasmic reticulum stress signalling–from basic mechanisms to clinical applications. FEBS J. 286, 241–278. doi: 10.1111/febs.14608
Alshareef, M. H., Hartland, E. L., and McCaffrey, K. (2021). Effectors targeting the unfolded protein response during intracellular bacterial infection. Microorganisms 9:705. doi: 10.3390/microorganisms9040705
An, F., Gong, G., Wang, Y., Bian, M., Yu, L., and Wei, C. (2017). MiR-124 acts as a target for Alzheimer’s disease by regulating BACE1. Oncotarget 8, 114065–114071. doi: 10.18632/oncotarget.23119
Baek, J., Kang, S., and Min, H. (2014). MicroRNA-targeting therapeutics for hepatitis C. Arch. Pharm. Res. 37, 299–305. doi: 10.1007/s12272-013-0318-9
Bajan, S., and Hutvagner, G. (2020). RNA-based therapeutics: from antisense oligonucleotides to miRNAs. Cells 9:137. doi: 10.3390/cells9010137
Bejanin, A., Schonhaut, D. R., La Joie, R., Kramer, J. H., Baker, S. L., Sosa, N., et al. (2017). Tau pathology and neurodegeneration contribute to cognitive impairment in Alzheimer’s disease. Brain 140, 3286–3300. doi: 10.1093/brain/awx243
Chen, M. L., Hong, C. G., Yue, T., Li, H. M., Duan, R., Hu, W. B., et al. (2021). Inhibition of miR-331-3p and miR-9-5p ameliorates Alzheimer’s disease by enhancing autophagy. Theranostics 11, 2395–2409. doi: 10.7150/thno.47408
Chen, Y., and Brandizzi, F. (2013). IRE1: ER stress sensor and cell fate executor. Trends Cell Biol. 23, 547–555. doi: 10.1016/j.tcb.2013.06.005
Chen, Y., Dang, M., and Zhang, Z. (2021). Brain mechanisms underlying neuropsychiatric symptoms in Alzheimer’s disease: a systematic review of symptom-general and -specific lesion patterns. Mol. Neurodegen. 16:38. doi: 10.1186/s13024-021-00456-1
Delay, C., Mandemakers, W., and Hébert, S. S. (2012). MicroRNAs in Alzheimer’s disease. Neurobiol. Dis. 46, 285–290. doi: 10.1016/j.nbd.2012.01.003
Du, W., Lei, C., and Dong, Y. (2021). MicroRNA-149 is downregulated in Alzheimer’s disease and inhibits β-amyloid accumulation and ameliorates neuronal viability through targeting BACE1. Genet. Mol. Biol. 44:e20200064. doi: 10.1590/1678-4685-GMB-2020-0064
Dufey, E., Sepúlveda, D., Rojas-Rivera, D., and Hetz, C. (2014). Cellular mechanisms of endoplasmic reticulum stress signaling in health and disease. 1. An overview. Am. J. Physiol. Cell Physiol. 307, C582–C594. doi: 10.1152/ajpcell.00258.2014
Fu, X. L., and Gao, D. S. (2014). Endoplasmic reticulum proteins quality control and the unfolded protein response: the regulative mechanism of organisms against stress injuries. Biofactors 40, 569–585. doi: 10.1002/biof.1194
Fu, Y., Chen, J., and Huang, Z. (2019). Recent progress in microRNA-based delivery systems for the treatment of human disease. ExRNA 1, 1–14. doi: 10.1186/s41544-019-0024-y
Gardner, B. M., Pincus, D., Gotthardt, K., Gallagher, C. M., and Walter, P. (2013). Endoplasmic reticulum stress sensing in the unfolded protein response. Cold Spring Harb. Perspect. Biol. 5:a013169. doi: 10.1101/cshperspect
Gerakis, Y., and Hetz, C. (2018). Emerging roles of ER stress in the etiology and pathogenesis of Alzheimer’s disease. FEBS J. 285, 995–1011. doi: 10.1111/febs.14332
Grasso, M., Piscopo, P., Confaloni, A., and Denti, M. A. (2014). Circulating miRNAs as biomarkers for neurodegenerative disorders. Molecules (Basel, Switzerland) 19, 6891–6910. doi: 10.3390/molecules19056891
Guo, J., Cai, Y., Ye, X., Ma, N., Wang, Y., Yu, B., et al. (2019). MiR-409-5p as a regulator of neurite growth is down regulated in APP/PS1 murine model of Alzheimer’s disease. Front. Neurosci. 13:1264. doi: 10.3389/fnins.2019.01264
Hashimoto, S., and Saido, T. C. (2018). Critical review: involvement of endoplasmic reticulum stress in the aetiology of Alzheimer’s disease. Open Biol. 8:180024. doi: 10.1098/rsob.180024
Hébert, S. S., Horré, K., Nicolaï, L., Papadopoulou, A. S., Mandemakers, W., Silahtaroglu, A. N., et al. (2008). Loss of microRNA cluster miR-29a/b-1 in sporadic Alzheimer’s disease correlates with increased BACE1/beta-secretase expression. Proc. Natl. Acad. Sci. U.S.A. 105, 6415–6420. doi: 10.1073/pnas.0710263105
Higaki, S., Muramatsu, M., Matsuda, A., Matsumoto, K., Satoh, J. I., Michikawa, M., et al. (2018). Defensive effect of microRNA-200b/c against amyloid-beta peptide-induced toxicity in Alzheimer’s disease models. PLoS One 13:e0196929. doi: 10.1371/journal.pone.0196929
Hoozemans, J. J., Van Haastert, E. S., Nijholt, D. A., Rozemuller, A. J., and Scheper, W. (2012). Activation of the unfolded protein response is an early event in Alzheimer’s and Parkinson’s disease. Neurodegener. Dis. 10, 212–215. doi: 10.1159/000334536
Hu, G., Shi, Z., Shao, W., and Xu, B. (2021). MicroRNA-214-5p involves in the protection effect of Dexmedetomidine against neurological injury in Alzheimer’s disease via targeting the suppressor of zest 12. Brain Res. Bull. 178, 164–172. doi: 10.1016/j.brainresbull.2021.10.016
Ibe, N. U., Subramanian, A., and Mukherjee, S. (2021). Non-canonical activation of the ER stress sensor ATF6 by Legionella pneumophila effectors. Life Sci. Alliance 4:e202101247. doi: 10.26508/lsa.202101247
Ji, Q., Wang, X., Cai, J., Du, X., Sun, H., and Zhang, N. (2019). MiR-22-3p regulates amyloid β deposit in mice model of Alzheimer’s disease by targeting mitogen-activated protein kinase 14. Curr. Neurovasc. Res. 16, 473–480. doi: 10.2174/1567202616666191111124516
Ji, Y., Wang, D., Zhang, B., and Lu, H. (2019). MiR-361-3p inhibits β-amyloid accumulation and attenuates cognitive deficits through targeting BACE1 in Alzheimer’s disease. J. Integr. Neurosci. 18, 285–291. doi: 10.31083/j.jin.2019.03.1136
Junjappa, R. P., Patil, P., Bhattarai, K. R., Kim, H. R., and Chae, H. J. (2018). IRE1α implications in endoplasmic reticulum stress-mediated development and pathogenesis of autoimmune diseases. Front. Immunol. 9:1289. doi: 10.3389/fimmu.2018.01289
Katayama, T., Imaizumi, K., Manabe, T., Hitomi, J., Kudo, T., and Tohyama, M. (2004). Induction of neuronal death by ER stress in Alzheimer’s disease. J. Chem. Neuroanat. 28, 67–78. doi: 10.1016/j.jchemneu.2003.12.004
Kennedy, M. E., Stamford, A. W., Chen, X., Cox, K., Cumming, J. N., Dockendorf, M. F., et al. (2016). The BACE1 inhibitor verubecestat (MK-8931) reduces CNS β-amyloid in animal models and in Alzheimer’s disease patients. Sci. Transl. Med. 8:363ra150. doi: 10.1126/scitranslmed.aad9704
Kim, E., and Jung, H. (2015). Local protein synthesis in neuronal axons: why and how we study. BMB Rep. 48:139. doi: 10.5483/BMBRep.2015.48.3.010
Kim, Y. J., Kim, S. H., Park, Y., Park, J., Lee, J. H., Kim, B. C., et al. (2020). miR-16-5p is upregulated by amyloid β deposition in Alzheimer’s disease models and induces neuronal cell apoptosis through direct targeting and suppression of BCL-2. Exp. Gerontol. 136:110954. doi: 10.1016/j.exger.2020.110954
Koh, H. S., Lee, S., Lee, H. J., Min, J. W., Iwatsubo, T., Teunissen, C. E., et al. (2021). Targeting MicroRNA-485-3p blocks Alzheimer’s disease progression. Int. J. Mol. Sci. 22:13136. doi: 10.3390/ijms222313136
Krammes, L., Hart, M., Rheinheimer, S., Diener, C., Menegatti, J., Grässer, F., et al. (2020). Induction of the Endoplasmic-reticulum-stress response: MicroRNA-34a targeting of the IRE1α-branch. Cells 9:1442. doi: 10.3390/cells9061442
Labban, S., Alshehri, F. S., Kurdi, M., Alatawi, Y., and Alghamdi, B. S. (2021). Melatonin improves short-term spatial memory in a mouse model of Alzheimer’s disease. Degener. Neurol. Neuromuscul. Dis. 11, 15–27. doi: 10.2147/DNND.S291172
Li, Q., Liu, T., Yang, S., and Zhang, Z. (2019). Upregulation of miR-34a by Inhibition of IRE1α has protective effect against Aβ-induced injury in SH-SY5Y cells by targeting caspase-2. Oxid. Med. Cell. Longev. 2019:2140427.
Lindholm, D., Korhonen, L., Eriksson, O., and Kõks, S. (2017). Recent insights into the role of unfolded protein response in ER stress in health and disease. Front. Cell Dev. Biol. 5:48. doi: 10.3389/fcell.2017.00048
Liu, C. G., Wang, J. L., Li, L. E. I., and Wang, P. C. (2014). MicroRNA-384 regulates both amyloid precursor protein and β-secretase expression and is a potential biomarker for Alzheimer’s disease. Int. J. Mol. Med. 34, 160–166.
Liu, W., Zhao, J., and Lu, G. (2016). miR-106b inhibits tau phosphorylation at Tyr18 by targeting Fyn in a model of Alzheimer’s disease. Biochem. Biophys. Res. Commun. 478, 852–857.
Long, J. M., Ray, B., and Lahiri, D. K. (2014). MicroRNA-339-5p down-regulates protein expression of β-site amyloid precursor protein-cleaving enzyme 1 (BACE1) in human primary brain cultures and is reduced in brain tissue specimens of Alzheimer disease subjects. J. Biol. Chem. 289, 5184–5198. doi: 10.1074/jbc.M113.518241
Madadi, S., Schwarzenbach, H., Saidijam, M., Mahjub, R., and Soleimani, M. (2019). Potential microRNA-related targets in clearance pathways of amyloid-β: novel therapeutic approach for the treatment of Alzheimer’s disease. Cell Biosci. 9:91. doi: 10.1186/s13578-019-0354-3
Oku, Y., Kariya, M., Fujimura, T., Hoseki, J., and Sakai, Y. (2021). Homeostasis of the ER redox state subsequent to proteasome inhibition. Sci. Rep. 11, 1–9. doi: 10.1038/s41598-021-87944-y
Pakos-Zebrucka, K., Koryga, I., Mnich, K., Ljujic, M., Samali, A., and Gorman, A. M. (2016). The integrated stress response. EMBO Rep. 17, 1374–1395. doi: 10.15252/embr.201642195
Plácido, A. I., Pereira, C. M. F., Duarte, A. I., Candeias, E., Correia, S. C., Santos, R. X., et al. (2014). The role of endoplasmic reticulum in amyloid precursor protein processing and trafficking: implications for Alzheimer’s disease. Biochim. Biophys. Acta 1842, 1444–1453. doi: 10.1016/j.bbadis.2014.05.003
Rahman, S., Archana, A., Jan, A. T., and Minakshi, R. (2018). Dissecting endoplasmic reticulum unfolded protein response (UPRER) in managing clandestine modus operandi of Alzheimer’s disease. Front. Aging Neurosci. 10:30. doi: 10.3389/fnagi.2018.00030
Rahman, S., Jan, A. T., Ayyagari, A., Kim, J., Kim, J., and Minakshi, R. (2017). Entanglement of UPRER in aging driven neurodegenerative diseases. Front. Aging Neurosci. 9:341. doi: 10.3389/fnagi.2017.00341
Rahman, S., Kumar, V., Kumar, A., Abdullah, T. S., Rather, I. A., and Jan, A. T. (2021). Molecular perspective of nanoparticle mediated therapeutic targeting in breast cancer: an odyssey of endoplasmic reticulum unfolded protein response (UPRER) and beyond. Biomedicines 9:635. doi: 10.3390/biomedicines9060635
Rajgor, D., Welle, T. M., and Smith, K. R. (2021). The coordination of local translation, membranous organelle trafficking, and synaptic plasticity in neurons. Front. Cell Dev. Biol. 9:711446. doi: 10.3389/fcell.2021.711446
Remondelli, P., and Renna, M. (2017). The endoplasmic reticulum unfolded protein response in neurodegenerative disorders and its potential therapeutic significance. Front. Mol. Neurosci. 10:187. doi: 10.3389/fnmol.2017.00187
Riscado, M., Baptista, B., and Sousa, F. (2021). New RNA-based breakthroughs in Alzheimer’s disease diagnosis and therapeutics. Pharmaceutics 13:1397. doi: 10.3390/pharmaceutics13091397
Rodriguez-Ortiz, C. J., Prieto, G. A., Martini, A. C., Forner, S., Trujillo-Estrada, L., LaFerla, F. M., et al. (2020). miR-181a negatively modulates synaptic plasticity in hippocampal cultures and its inhibition rescues memory deficits in a mouse model of Alzheimer’s disease. Aging Cell 19:e13118. doi: 10.1111/acel.13118
Scheper, W., and Hoozemans, J. J. (2015). The unfolded protein response in neurodegenerative diseases: a neuropathological perspective. Acta Neuropathol. 130, 315–331. doi: 10.1007/s00401-015-1462-8
Shi, Z., Zhang, K., Zhou, H., Jiang, L., Xie, B., Wang, R., et al. (2020). Increased miR-34c mediates synaptic deficits by targeting synaptotagmin 1 through ROS-JNK-p53 pathway in Alzheimer’s disease. Aging Cell 19:e13125. doi: 10.1111/acel.13125
Siwecka, N., Rozpędek-Kamińska, W., Wawrzynkiewicz, A., Pytel, D., Diehl, J. A., and Majsterek, I. (2021). The structure, activation and signaling of IRE1 and its role in determining cell fate. Biomedicines 9:156. doi: 10.3390/biomedicines9020156
Smalheiser, N. R., and Lugli, G. (2009). microRNA regulation of synaptic plasticity. Neuromol. Med. 11, 133–140. doi: 10.1007/s12017-009-8065-2
Spires-Jones, T. L., Attems, J., and Thal, D. R. (2017). Interactions of pathological proteins in neurodegenerative diseases. Acta Neuropathol. 134, 187–205. doi: 10.1007/s00401-017-1709-7
Stauffer, W. T., Arrieta, A., Blackwood, E. A., and Glembotski, C. C. (2020). Sledgehammer to scalpel: broad challenges to the heart and other tissues yield specific cellular responses via transcriptional regulation of the ER-stress master regulator ATF6α. Int. J. Mol. Sci. 21:1134. doi: 10.3390/ijms21031134
Sultan, S., Taimuri, U., Basnan, S. A., Ai-Orabi, W. K., Awadallah, A., Almowald, F., et al. (2020). Low vitamin D and its association with cognitive impairment and dementia. J. Aging Res. 2020:6097820. doi: 10.1155/2020/6097820
Tanprasertsuk, J., Johnson, E. J., Johnson, M. A., Poon, L. W., Nelson, P. T., Davey, A., et al. (2019). Clinico-neuropathological findings in the oldest old from the georgia centenarian study. J. Alzheimers Dis. 70, 35–49. doi: 10.3233/JAD-181110
Wang, D., Fei, Z., Luo, S., and Wang, H. (2020). MiR-335-5p inhibits β-amyloid (Aβ) accumulation to attenuate cognitive deficits through targeting c-jun-N-terminal kinase 3 in Alzheimer’s disease. Curr. Neurovasc. Res. 17, 93–101. doi: 10.2174/1567202617666200128141938
Wang, L., Liu, J., Wang, Q., Jiang, H., Zeng, L., Li, Z., et al. (2019). MicroRNA-200a-3p mediates neuroprotection in alzheimer-related deficits and attenuates amyloid-beta overproduction and tau hyperphosphorylation via coregulating BACE1 and PRKACB. Front. Pharmacol. 10:806. doi: 10.3389/fphar.2019.00806
Wang, M., Qin, L., and Tang, B. (2019). MicroRNAs in Alzheimer’s disease. Front. Genet. 10:153. doi: 10.3389/fgene.2019.00153
Wang, P., Li, J., Tao, J., and Sha, B. (2018). The luminal domain of the ER stress sensor protein PERK binds misfolded proteins and thereby triggers PERK oligomerization. J. Biol. Chem. 293, 4110–4121. doi: 10.1074/jbc.RA117.001294
Wu, J., He, G. T., Zhang, W. J., Xu, J., and Huang, Q. B. (2016). IRE1α signaling pathways involved in mammalian cell fate determination. Cell Physiol. Biochem. 2016, 847–858. doi: 10.1159/000443039
Wu, Q., Ye, X., Xiong, Y., Zhu, H., Miao, J., Zhang, W., et al. (2016). The protective role of microRNA-200c in Alzheimer’s disease pathologies is induced by beta amyloid-triggered endoplasmic reticulum stress. Front. Mol. Neurosci. 9:140. doi: 10.3389/fnmol.2016.00140
Xiao, G., Chen, Q., and Zhang, X. (2021). MicroRNA-455-5p/CPEB1 pathway mediates Aβ-related learning and memory deficits in a mouse model of Alzheimer’s disease. Brain Res. Bull. 177, 282–294. doi: 10.1016/j.brainresbull.2021.10.008
Yasmeen, N., Kumar, V., and Shaikh, K. D. (2021). “Impact of MicroRNA polymorphisms on breast cancer susceptibility,” in Genetic Polymorphism and Cancer Susceptibility, eds A. S. Sameer, M. Z. Banday, and S. Nissar (Singapore: Springer), 53–77. doi: 10.1007/978-981-33-6699-2_3
Ye, X., Luo, H., Chen, Y., Wu, Q., Xiong, Y., Zhu, J., et al. (2015). MicroRNAs 99b-5p/100-5p regulated by endoplasmic reticulum stress are involved in abeta-induced pathologies. Front. Aging Neurosci. 7:210. doi: 10.3389/fnagi.2015.00210
Keywords: ER stress, unfolded protein response (UPR), microRNA, Alzheimer’s disease, neurodegeneration
Citation: Yasmeen N, Datta M, Kumar V, Alshehri FS, Almalki AH and Haque S (2022) Deciphering the Link Between ERUPR Signaling and MicroRNA in Pathogenesis of Alzheimer’s Disease. Front. Aging Neurosci. 14:880167. doi: 10.3389/fnagi.2022.880167
Received: 21 February 2022; Accepted: 23 March 2022;
Published: 09 May 2022.
Edited by:
Rinki Minakshi, University of Delhi, IndiaReviewed by:
Zeenat Mirza, King Abdulaziz University, Saudi ArabiaNiraj Kumar Jha, Sharda University, India
Copyright © 2022 Yasmeen, Datta, Kumar, Alshehri, Almalki and Haque. This is an open-access article distributed under the terms of the Creative Commons Attribution License (CC BY). The use, distribution or reproduction in other forums is permitted, provided the original author(s) and the copyright owner(s) are credited and that the original publication in this journal is cited, in accordance with accepted academic practice. No use, distribution or reproduction is permitted which does not comply with these terms.
*Correspondence: Vikram Kumar, vikramyadav05@gmail.com; vkumar3@jpr.amity.edu