Multiple Functions of Draxin/Netrin-1 Signaling in the Development of Neural Circuits in the Spinal Cord and the Brain
- 1Department of Neuroscience and Pharmacology, The University of Texas Southwestern Medical Center, Dallas, TX, United States
- 2Department of Medical Neuroscience, Graduate School of Medical Sciences, Kanazawa University, Kanazawa, Japan
Axon guidance proteins play key roles in the formation of neural circuits during development. We previously identified an axon guidance cue, named draxin, that has no homology with other axon guidance proteins. Draxin is essential for the development of various neural circuits including the spinal cord commissure, corpus callosum, and thalamocortical projections. Draxin has been shown to not only control axon guidance through netrin-1 receptors, deleted in colorectal cancer (Dcc), and neogenin (Neo1) but also modulate netrin-1-mediated axon guidance and fasciculation. In this review, we summarize the multifaceted functions of draxin and netrin-1 signaling in neural circuit formation in the central nervous system. Furthermore, because recent studies suggest that the distributions and functions of axon guidance cues are highly regulated by glycoproteins such as Dystroglycan and Heparan sulfate proteoglycans, we discuss a possible function of glycoproteins in draxin/netrin-1-mediated axon guidance.
Introduction
The proper function of the nervous system relies on appropriate patterns of connectivity among an enormous number of neurons. A key process in the formation of neural circuits is the navigation of neuronal axons to their targets, which is regulated by axon guidance molecules (Tessier-Lavigne and Goodman, 1996; Dickson, 2002). In the 1990s, four major families of axon guidance proteins were identified: netrins, semaphorins, ephrins, and slits (Raper and Kapfhammer, 1990; Rothberg et al., 1990; Kolodkin et al., 1993; Luo et al., 1993; Kennedy et al., 1994; Serafini et al., 1994; Cheng et al., 1995; Drescher et al., 1995; Brose et al., 1999; Kidd et al., 1999). In addition, three other families of secreted signaling molecules have been shown to act as axon guidance cues: the Wingless/Wnt, Hedgehog (Hh), and transforming growth factor-β/bone morphogenic protein (BMP) families (Charron and Tessier-Lavigne, 2007; Zou and Lyuksyutova, 2007; Yam and Charron, 2013). Numerous studies of these axon guidance cues have revealed the molecular basis of neural circuit formation, in which axons expressing guidance receptors navigate to their targets by detecting a variety of attractive and repulsive cues presented by cells in the environment. However, many guidance processes during brain development cannot be explained by the function of these molecules alone. It has been shown that interactions of multiple guidance signals diversify the possible responses to a limited number of guidance cues (Dudanova and Klein, 2013). Another potential mechanism is that more unidentified molecules controlling axon guidance and/or regulating known guidance signaling participate in the formation of the complex nervous system.
We previously identified a novel axon guidance cue, draxin, that is highly expressed in the developing central nervous system (CNS) (Islam et al., 2009). We showed that draxin controls axon guidance through netrin-1 receptors, Deleted in colorectal cancer (Dcc) and Neogenin (Neo1) (Ahmed et al., 2011; Shinmyo et al., 2015). Draxin has also been shown to modulate netrin-1-mediated axon guidance and fasciculation (Gao et al., 2015; Liu et al., 2018; Meijers et al., 2020). In this review, we highlight possible roles of draxin/netrin-1 signaling in the development of spinal commissural axons, corpus callosum, and thalamocortical axons. Furthermore, we discuss a possible contribution of glycoproteins to the distribution and function of draxin and netrin-1 in the developing spinal cord and brain.
Draxin and Netrin-1 Signaling in Axon Guidance
Draxin is a secreted protein that has a cysteine-rich domain in its carboxyl terminal region. Although the positions of 10 cysteine residues in the cysteine-rich domain of draxin are similar to those of members of the Dickkopf (Dkk) family, draxin shares no homology with any known proteins (Islam et al., 2009; Miyake et al., 2009). Draxin homologs are found in vertebrate genomes but not in invertebrate genomes. We and others reported that draxin regulates the outgrowth of axons originating from various types of neurons in vitro (Islam et al., 2009; Naser et al., 2009; Ahmed et al., 2010, 2011; Chen et al., 2013; Meli et al., 2015; Shinmyo et al., 2015). In addition, draxin knockout (KO) mice show defects in many axonal tracts including the spinal cord commissure, anterior commissure, corpus callosum, internal capsule (thalamocortical and corticofugal tracts), and hippocampal commissure (Islam et al., 2009; Shinmyo et al., 2015). Thus, draxin controls the development of neural circuits in the CNS via a vertebrate-specific mechanism.
The neuronal network defects in draxin KO mice clearly demonstrate that draxin plays an essential role in axon guidance in the spinal cord and the brain. Yet, how draxin regulates axon guidance in vivo is still poorly understood. Through cell binding assays, we previously showed that draxin binds the netrin-1 receptors Dcc, Neo1, Unc5s, and Down’s syndrome cell adhesion molecule (DSCAM) (Ahmed et al., 2011). Based on draxin and Dcc KO mouse phenotype similarities, we analyzed interaction between draxin and Dcc further and found that draxin co-immunoprecipitated immunoglobulin (Ig) domain of Dcc, which is separate from the netrin-1-binding domain (Figure 1). Furthermore, draxin-mediated axonal outgrowth in neurons from the neocortex (Ahmed et al., 2011; Meli et al., 2015; Shinmyo et al., 2015), thalamus (Shinmyo et al., 2015), and dorsal horn of the spinal cord (Chen et al., 2013) requires Dcc and Neo1. A recent study showed draxin directly binds netrin-1 and acts as a netrin-1 antagonist by preventing netrin-1 from binding to the Dcc receptor (Gao et al., 2015). On the other hand, structural studies demonstrated that draxin can interact with both netrin-1 and Dcc simultaneously (Liu et al., 2018). Draxin contains the 22-residue conserved hydrophobic sequence that binds netrin-1 at the EGF-3 domain and an approximately 90-residue cysteine knot domain at the C terminus that binds Dcc at the Ig4 domain (Figure 1). Netrin-1 utilizes its laminin VI, EGF-1, EGF-2, and EGF-3 domains to interact with the fibronectin (FN) domain of Dcc (Figure 1; Finci et al., 2014; Xu et al., 2014). From the binding configurations of these three molecules, Liu et al. (2018) have proposed that the draxin/netrin-1 complex can build a bridge between two axons decorated with Dcc, promoting adhesion, and fasciculation between these axons. Thus, it is likely that draxin not only controls axon guidance through the Dcc/Neo1 receptors but also modulates netrin-1-mediated axon guidance and fasciculation. In contrast, it is unknown whether Unc5s and DSCAM mediate draxin functions in axon guidance and fasciculation.
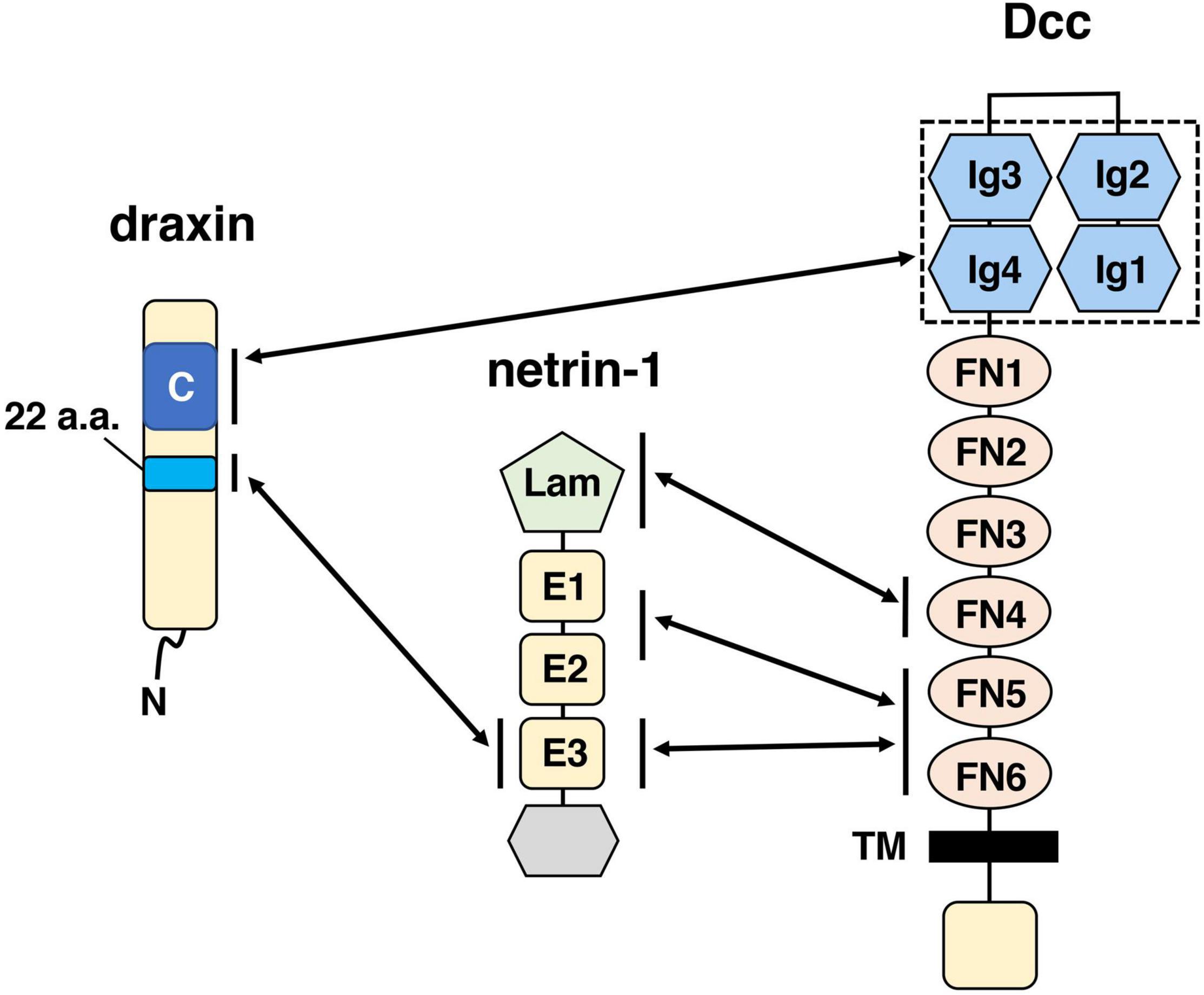
Figure 1. Schematic drawings of draxin and its interacting proteins, netrin-1, and Dcc. The C-terminal domain of draxin interacts with the Ig domain of Dcc. A 22-residue sequence of draxin interacts with the EGF-3 (E3) domain of netrin-1. Netrin-1 interacts with Dcc at the fibronectin (FN) domain through three binding sites: the laminin VI domain (Lam), EGF-1/EGF-2 (E1/E2) domain, and E3 domain. It should be noted that in addition to Dcc, Neo1 is also a functionally important receptor for draxin. Because Dcc and Neo1 are structurally very similar proteins, the C-terminal domain of draxin may interact with the Ig domain of Neo1.
Spinal Commissural Axons
Commissural axon guidance has been extensively studied in the developing spinal cord (Tessier-Lavigne and Goodman, 1996; Dickson, 2002; Kolodkin and Tessier-Lavigne, 2011). Commissural neurons are generated in the dorsal spinal cord and extend their axons ventrally toward the floor plate (FP), turning away from the roof plate (RP) and lying close to the pial surface (Figure 2A). Once they cross the ventral midline, most of the commissural axons turn rostrally toward the brain.
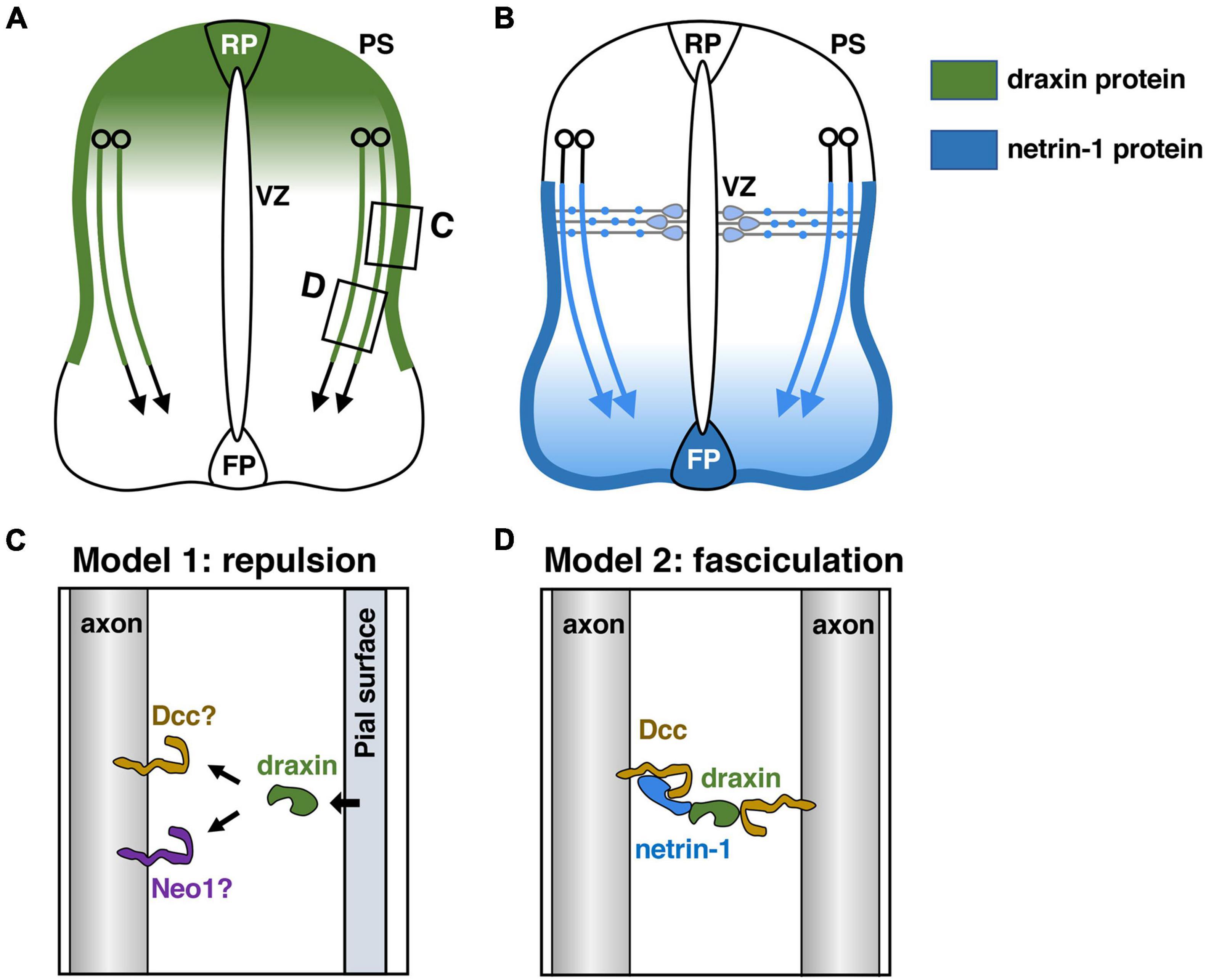
Figure 2. Draxin and netrin-1 signaling in the guidance of spinal commissural axons. (A) Draxin mRNA is expressed in the dorsal spinal cord including the roof plate (RP), and its protein is deposited along the pial surface of the dorsal spinal cord and commissural axons. VZ, ventricular zone; PS, pial surface. (B) Netrin-1 produced by progenitor cells in the ventricular zone and the floor plate (FP) cells acts synergistically to guide commissural axons in the spinal cord. Note that netrin-1 is deposited along the pial surface of the ventral spinal cord and commissural axons. (C,D) Two models for draxin functions in the guidance of spinal commissural axons. The areas within the boxes in panel (A) were magnified and are shown in panels (C,D). We have proposed that draxin on the pial surface acts as a repulsive barrier for commissural axons (C). Liu et al. (2018) have proposed a model in which draxin mediates the fasciculation of Dcc-expressing axons by creating a Dcc-draxin-netrin-1-Dcc bridge-like configuration between two axons (D).
The RP is the source of diffusible repellents that guide the early trajectory of spinal commissural axons (Augsburger et al., 1999). It was shown that Growth/differentiation factor 7 (Gdf7): Bmp7 heterodimers mediate the RP-derived chemorepellent activity for spinal commissural axons (Augsburger et al., 1999; Butler and Dodd, 2003). Indeed, commissural axons extend aberrantly toward or into the RP in Bmp7 and Gdf7 mutant mice (Butler and Dodd, 2003). Since many commissural axons in Bmp7 and Gdf7 doubly mutant mice recover their correct projection pattern at later developmental stages, it has also been postulated that the RP may express other repellents (Butler and Dodd, 2003). Draxin mRNA is expressed in the dorsal spinal cord including the RP (Islam et al., 2009). In vivo experiments with chicken spinal cord as well as in vitro neurite outgrowth experiments showed that draxin has repulsive activity for spinal commissural axons (Islam et al., 2009). Thus, draxin is most likely an additional repellent protein from the dorsal spinal cord that orients commissural axons (Figure 2A). It should be noted that draxin KO mice do not show medial misrouting of commissural axons (Islam et al., 2009). This may reflect functional redundancy with Bmp7 and Gdf7 expressed by the RP. Further analyses with double or triple mutant mice for these factors would help to clarify this point.
In contrast to the RP, the FP was shown by in vitro explant assays to be the source of chemoattractants for commissural axons (Tessier-Lavigne et al., 1988; Placzek et al., 1990). So far, three FP-derived chemoattractants have been identified: netrin-1 (Kennedy et al., 1994; Serafini et al., 1994), sonic hedgehog (Shh) (Charron et al., 2003), and vascular endothelial growth factor (VEGF) (Ruiz de Almodovar et al., 2011). In the mouse spinal cord, netrin-1 is expressed in not only FP cells but also neural progenitor cells (NPCs) of the ventricular zone (VZ) (Serafini et al., 1996). Recently, conditional knockout approaches have been used to investigate the roles of netrin-1 derived from these two sources in commissural axon guidance. Removal of netrin-1 from the FP results in the defasciculation and misrouting of commissural axons in the ventral spinal cord, supporting the classical model that FP-derived netrin-1 guides commissural axons at long range (Figure 2B; Moreno-Bravo et al., 2019; Wu et al., 2019). Furthermore, removal of both FP-netrin-1 and the Shh receptor Boc results in additive guidance defects, suggesting that netrin-1 from the FP collaborates with Shh in commissural axon guidance in the ventral spinal cord (Wu et al., 2019). Interestingly, draxin and netrin-1 proteins form reciprocal gradients along the dorsoventral axis of the spinal cord. Gao et al. (2015) have proposed that draxin may sharpen the extracellular distribution of active netrin-1 by acting as a netrin-1 antagonist.
Although removal of netrin-1 from the FP causes the axon guidance defects mentioned above, many commissural axons reach the midline in the spinal cord. This is in contrast to the phenotype in netrin-1 hypomorphs (Serafini et al., 1996) and netrin-1-null mutants (Bin et al., 2015; Yung et al., 2015), in which the number of commissural axons reaching the FP is substantially reduced. Interestingly, several recent studies demonstrated that netrin-1 from the VZ, rather than the FP, is the key source of netrin-1 that guides commissural axons to the FP in the spinal cord and the hindbrain (Dominici et al., 2017; Varadarajan et al., 2017; Yamauchi et al., 2017). In the spinal cord, removal of netrin-1 from the VZ results in many guidance defects of commissural axons, which aberrantly cross the dorsal midline and invade the VZ and motor neuron domain (Dominici et al., 2017; Varadarajan et al., 2017). This result suggests that VZ-supplied netrin-1 is crucial for commissural axon guidance to the midline (Figure 2B).
How does VZ-derived netrin-1 guide commissural axons to the ventral midline? While netrin-1 mRNA is expressed in NPCs of the VZ, its protein is present on the pial surface and commissural axons (Figure 2B; Kennedy et al., 2006; Varadarajan et al., 2017). It was suggested that netrin-1 produced by NPCs is transported via their radial processes to the basement membrane, where their end-feet contact the pial surface (Varadarajan et al., 2017). Thus, netrin-1 deposited on the pial surface is likely to function as a short-range cue for commissural axons, which grow alongside it (Figure 2B). One current model suggests that the pial-netrin-1 substrate acts by haptotaxis to promote commissural axon extension and direct axons toward the ventral midline. Other excellent papers detailed the functions of netrin-1 in guiding the commissural axons to the ventral midline (Morales, 2018; Chedotal, 2019; Comer et al., 2019; Ducuing et al., 2019). Importantly, draxin is also heavily deposited in the basement membrane along the dorsal and lateral sides of the spinal cord (Figure 2A; Islam et al., 2009), suggesting a role of pial-draxin in commissural axon guidance. In draxin KO mice, commissural axons project in a defasciculated manner toward the FP (Islam et al., 2009). In addition, commissural axons along the basement membrane are observed more frequently in draxin KO mice than in control mice (Islam et al., 2009). Based on these results, we have proposed that draxin on the pial surface acts as a repulsive barrier for commissural axons (Figure 2C). In this context, it will be interesting to determine how Dcc and/or other receptors mediate signaling cascades for draxin and netrin-1 from the pial surface, especially because these two ligands have opposite activities on spinal cord commissural axons. Another potential explanation for this phenotype is that draxin promotes the fasciculation of commissural axons by interacting with Dcc and netrin-1 (Liu et al., 2018; Meijers et al., 2020). Liu et al. (2018) have proposed a model in which draxin mediates the fasciculation of Dcc-expressing axons by creating a Dcc-draxin-netrin-1-Dcc bridge-like configuration between two axons (Figure 2D; Liu et al., 2018; Meijers et al., 2020). This model is supported by observations that these three proteins are present on commissural axons and that the accumulation of netrin-1 protein is greatly reduced in axons of Dcc mutants (Varadarajan et al., 2017).
It is important to note that draxin also interacts with the canonical Wnt receptor LRP6 and antagonizes canonical Wnt signaling (Miyake et al., 2009). It was shown that canonical Wnt signaling is required for postcrossing commissural axon guidance in the spinal cord (Aviles and Stoeckli, 2016). Although postcrossing commissural axon projections seemed to be not affected in draxin KO mice (Islam et al., 2009), draxin might be involved in postcrossing commissural axon guidance by modulating canonical Wnt signaling.
The Corpus Callosum
The corpus callosum is the major axonal tract that connects and coordinates information between the two cerebral hemispheres. Complete absence (agenesis) of the corpus callosum in humans is associated with various neurological disorders such as language dysfunction and abnormalities in social interaction (Paul et al., 2007; Edwards et al., 2014; Brown and Paul, 2019). A large number of genes including netrin-1, draxin and Dcc have been shown to be linked to malformations of the corpus callosum in humans and mice (Richards et al., 2004; Paul et al., 2007; Edwards et al., 2014). Loss-of-function mutations of DCC in humans cause dysgenesis of the corpus callosum (Morcom et al., 2021b). Although pathogenic variants in DRAXIN and NETRIN-1 have not yet been reported in humans with dysgenesis of the corpus callosum, in mice, mutant forms of draxin, netrin-1, and Dcc have each been shown to cause agenesis of the corpus callosum with similar misprojection of callosal axons (Serafini et al., 1996; Fazeli et al., 1997; Ren et al., 2007; Islam et al., 2009; Fothergill et al., 2014). In these mutants, callosal axons do not cross the midline; instead, they form ipsilateral “Probst” bundles that run parallel to the midline. These facts suggest that these three molecules may act in a similar manner to control midline crossing of callosal axons.
During brain development, callosal pioneering axons originate in the cingulate cortex, and cross the midline in a region initially separated by the interhemispheric fissure (IHF) (Rakic and Yakovlev, 1968; Silver et al., 1982; Gobius et al., 2016). Subsequently, these pioneering axons guide the midline crossing of neocortical callosal axons projecting from mainly layers II/III and V. Two distinct developmental mechanisms have been shown to be critical for midline crossing of callosal axons. First, the remodeling of the IHF mediated by specialized astroglial cells known as midline zipper glia (MZG) is necessary to form a permissive substrate for callosal pioneering axons to cross the midline (Gobius et al., 2016). Second, midline glial structures that express axon guidance cues including netrin-1, draxin, and Slit2 play an essential role in the guidance of callosal axons at the midline (Lindwall et al., 2007; Nishikimi et al., 2013; Fothergill et al., 2014). Recently, Morcom et al. (2021a; 2021b) reported that draxin, netrin-1 and Dcc are key players in the development of astroglial cells during IHF remodeling. It is therefore plausible that draxin/netrin-1 signaling has multiple roles in corpus callosum formation including IHF remodeling and the guidance of callosal axons.
Interhemispheric fissure remodeling has been shown to consist of several key processes including generation and specification of the MZG from radial glia, somal translocation of the MZG to the IHF and differentiation of the MZG into multipolar MZG cells that degrade the IHF and intercalate across the midline (Figure 3A; Gobius et al., 2016; Morcom et al., 2021a,b). A previous study with neuroimaging showed that agenesis of the corpus callosum is remarkably correlated with retention of the IHF in humans (Gobius et al., 2016). Furthermore, a more recent study showed that IHF remodeling is disrupted in humans with DCC pathogenic variants that display agenesis of the corpus callosum (Morcom et al., 2021b). Thus, developmental defects in IHF remodeling are likely to be a primary etiology leading to human callosal agenesis (Gobius et al., 2016). Consistent with human subjects with DCC mutations, both netrin-1 and Dcc mutant mice display impaired remodeling of the IHF (Hakanen and Salminen, 2015; Morcom et al., 2021b). Dcc mutant mice show irregular morphology of the MZG and reduced somal translocation to the IHF. Taken together with the fact that netrin-1 and Dcc are expressed in the MZG during IHF remodeling, these results suggest that netrin-1/Dcc signaling facilitates IHF remodeling by regulating MZG morphology and migration to the IHF (Morcom et al., 2021b).
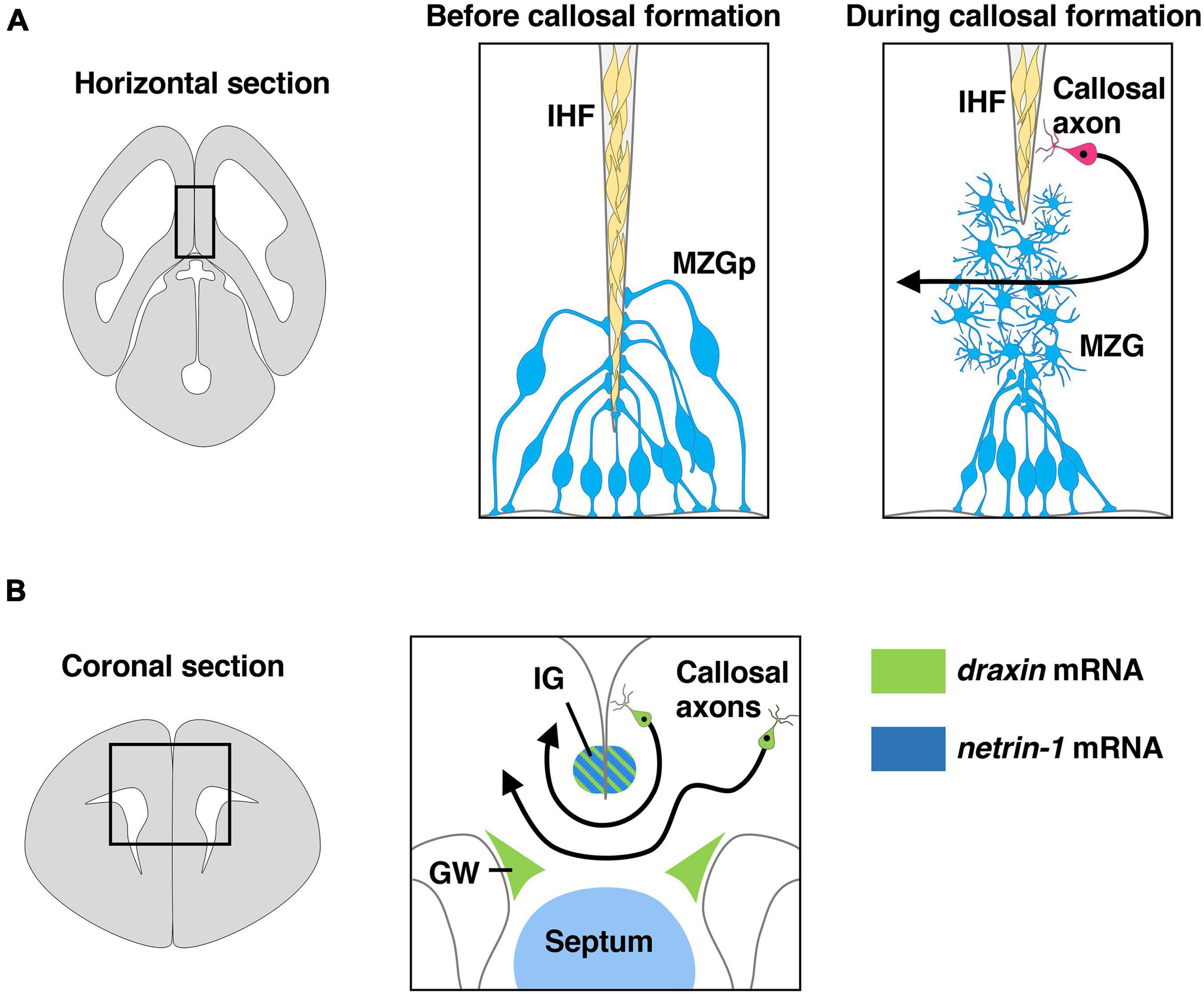
Figure 3. Draxin and netrin-1 signaling in the development of corpus callosum. (A) The remodeling of the interhemispheric fissure (IHF) mediated by midline zipper glia (MZG) is necessary to form a permissive substrate for callosal axons to cross the midline. The area within the box in the left panel is shown in the middle panel and the right panel. Draxin, netrin-1, and Dcc are key players in IHF remodeling. MZGp, midline zipper glia progenitors. (B) Midline structures that express draxin and netrin-1 paly an essential role in guidance of callosal axons at the midline. The area within the box in the left panel is shown in the right panel. Draxin mRNA is expressed in the indusium griseum (IG) and glial wedge (GW), while netrin-1 mRNA is expressed in IG and septum. Draxin mRNA is also expressed in callosal neurons.
It remains unclear whether IHF remodeling is disrupted in draxin KO mice. Recently, however, an eight base-pair deletion in the draxin gene was identified in BTBR mouse strains, which have a severe commissural phenotype including dysgenesis of the corpus callosum (Morcom et al., 2021a). This deletion results in a premature strop codon in exon 2 of the draxin gene, creating a truncated protein lacking domains that bind netrin-1 and Dcc. Importantly, the severity of corpus callosum dysgenesis in BTBR mice was shown to be strongly associated with abnormal retention of the IHF, suggesting that a failure of IHF remodeling caused by loss of draxin function results in corpus callosum dysgenesis in these mice. Draxin is highly expressed in MZG progenitors, and its protein is widely distributed on various components of the interhemispheric midline including the MZG, leptomeninges, and callosal axons. Interestingly, BTBR mice show increased somal translocation of the MZG to the IHF, which is the opposite phenotype of Dcc mutant mice. In this context, draxin might antagonize netrin-1/Dcc signaling that stimulates somal translocation of the MZG to the IHF during normal IHF remodeling (Morcom et al., 2021a). Furthermore, BTBR mice show an increase in the proliferation of both MZG cells and leptomeningeal cells. This phenotype is not observed in Dcc and netrin-1 mutants, suggesting that draxin may regulate the proliferation of these cells independently of netrin-1/Dcc signaling (Morcom et al., 2021a). Thus, draxin may be involved in multiple processes of IHF remodeling by interacting with not only netrin/Dcc signaling but also other signaling molecules (Morcom et al., 2021a).
Midline crossing of callosal axons is thought to be controlled by axon guidance cues expressed in midline guidepost structures, including the glial wedge (GW) and indusium griseum (IG) (Figure 3B; Lindwall et al., 2007; Nishikimi et al., 2013; Fothergill et al., 2014). Netrin-1 is expressed in the septum and the IG region (Figure 3B), and Dcc is expressed by callosal axons during midline crossing of callosal axons (Fothergill et al., 2014; Hakanen and Salminen, 2015). In vitro experiments showed that netrin-1 acts as a chemoattractant for callosal pioneering axons derived from the cingulate cortex but is not attractive for neocortical callosal axons, suggesting that netrin-1 attracts callosal pioneering axons toward the midline (Fothergill et al., 2014). Furthermore, netrin-1/Dcc signaling was suggested to attenuate Slit2-mediated repulsion of pre-crossing axons from the GW and the IG, allowing them to approach and cross the midline (Fothergill et al., 2014). During midline crossing of callosal axons, they must decrease responsiveness to attractive cues from midline guidepost structures and increase responsiveness to repulsive cues from these structures to leave the midline and enter the contralateral hemisphere. Importantly, Dcc expression is downregulated on post-crossing callosal axons. This leads to the cancelation of netrin-1-regulated attenuation of Slit2-mediated repulsion, thereby allowing the callosal axons to leave the midline (Fothergill et al., 2014).
We previously showed that draxin regulates axonal growth of neocortical neurons in a concentration-dependent manner and that this effect is mediated through the Dcc and Neo1 receptors (Shinmyo et al., 2015). Low concentrations of draxin stimulate neurite outgrowth of neocortical neurons, while higher concentrations of draxin inhibit neurite outgrowth. This result suggests that the bimodal effects of draxin might be critical for midline crossing of callosal axons. Similar bimodal responses depending on concentration were reported for ephrin-A2 (Hansen et al., 2004) and Shh (Kolpak et al., 2005) on retinal ganglion cells. Ephrin-A2 inhibits retinal axon outgrowth at high concentrations but promotes growth at lower concentrations. This concentration-dependent activity of ephrin-A2 has been proposed to be involved in topographic map formation in the mouse visual system (Hansen et al., 2004). During corpus callosum development, draxin is expressed in midline glial structures including the GW and the IG (Figure 3B). It may be possible that draxin from the midline glial structures initially attracts callosal axons toward the midline and later acts as a repellent when callosal axons are exposed to higher concentrations of draxin at the midline. Taken together, the current evidence suggests that draxin from the midline structures is likely to serve critical roles in the development of the corpus callosum through the regulation of IHF remodeling and the guidance of callosal axons. However, it is also possible that draxin secreted from other regions contributes to the guidance of callosal axons. It should be noted that draxin is also expressed in callosal neurons of the cingulate cortex and the neocortex (Figure 3B), suggesting that draxin might regulate midline crossing of callosal axons in an autocrine manner. Conditional strategies that enable the spatial and temporal manipulation of draxin expression are necessary to determine the exact roles of draxin in the development of the corpus callosum.
Thalamocortical Projections
Thalamocortical axons originate in the dorsal thalamus and carry sensory information to the neocortex (Lopez-Bendito and Molnar, 2003; Leyva-Diaz and Lopez-Bendito, 2013; Garel and Lopez-Bendito, 2014). In mice, developing thalamocortical axons first exit the thalamus and then turn dorsolaterally at the diencephalic–telencephalic boundary (DTB) to enter the internal capsule by embryonic day 13 (E13) (Figure 4). Thalamic axons continue to extend through the subpallium and reach the pallial-subpallial boundary (PSPB), where they meet corticofugal axons, at around E14 (Figure 4). Thalamic axons advance through the intermediate zone to reach the developing cortex and arrive at the appropriate cortical regions around E16. Thalamic axons wait in the subplate for 2 or 3 days and finally invade the cortical plate, forming synapses onto neurons of the appropriate layers.
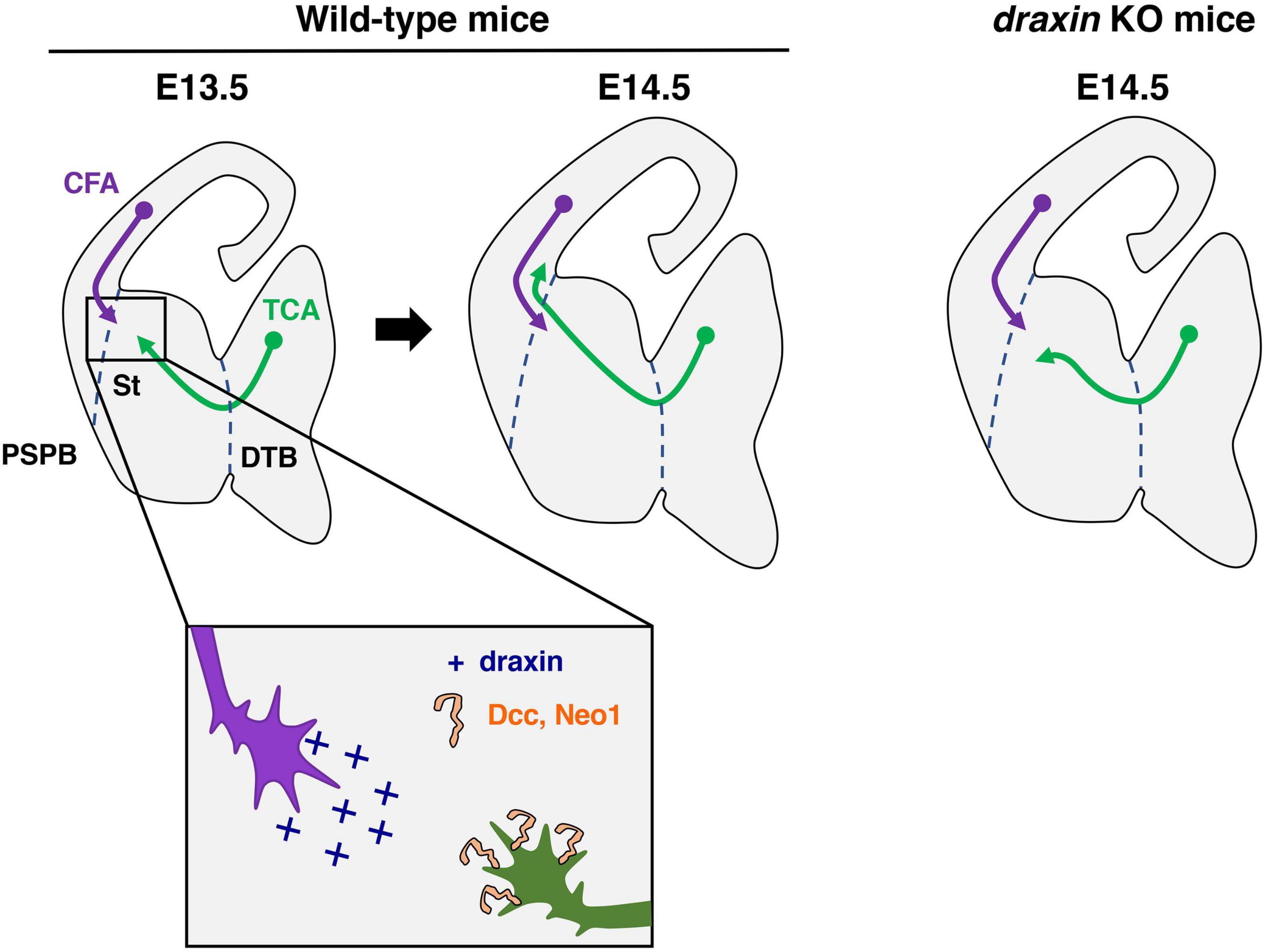
Figure 4. Draxin signaling in the development of thalamocortical axons. Thalamocortical axons (TCA) and corticofugal axons (CFA) meet in the striatum (St) to form the internal capsule around E13.5. At E14.5, and TCA progress through the pallial-subpallial boundary (PSPB) to enter the neocortex in wild-type mice. In draxin KO mice, TCA do not cross the PSPB, while early CFA projected normally toward the internal capsule. We have proposed a model, in which draxin from CFA promotes the growth of TCA from the internal capsule to the neocortex, and this effect of draxin is mediated through the Dcc and Neo1 receptors. DTB, diencephalic/telencephalic boundary.
It was shown over two decades that the guidance of thalamocortical axons is dependent on neocortical subplate neurons, which pioneer the corticofugal pathway from the neocortex to the internal capsule (McConnell et al., 1989; Ghosh et al., 1990; Ghosh and Shatz, 1993). The handshake hypothesis, proposed by Molnar and colleagues, postulates that thalamocortical projections rely on the interaction of corticofugal and thalamocortical axons (Molnar et al., 1998). This hypothesis is supported by previous results obtained with conditional genetic experiments. Indeed, it was shown in conditional mutant mice lacking corticofugal axons that these axons are required for the guidance of thalamocortical axons across the PSPB (Chen et al., 2012). In a complementary study using conditional ablation of the embryonic thalamus, thalamocortical axons were shown to be necessary to guide corticothalamic axons into the corridor and toward the thalamus (Deck et al., 2013). However, the molecular mechanisms underlying this reciprocal interaction are not fully understood.
Importantly, we previously showed that draxin controls the guidance of thalamocortical axons by regulating the interaction of thalamocortical and corticofugal axons (Shinmyo et al., 2015). Draxin KO mice display severe defects in thalamocortical and corticofugal projections. Thalamocortical axons of draxin KO mice grow into the internal capsule, but the majority of them do not enter the cortex and instead either stall or turn laterally toward the external capsule. This phenotype is similar to that in conditional mutant mice lacking corticofugal axons, suggesting that draxin could mediate the interaction of thalamocortical and corticofugal axons. In draxin KO mice, corticofugal axons project normally toward the internal capsule at E14.5, while thalamocortical axons have already misrouted toward the external capsule at this stage (Figure 4). This result indicates that the pathfinding errors of thalamocortical axons precede those of corticofugal axons in draxin KO mice. Draxin is widely expressed in developing brains including in corticofugal neurons, while it is not expressed in thalamic neurons. The thalamocortical phenotype in draxin KO mice is rescued by the transgenic expression of draxin in the neocortex. These results suggest that draxin secreted from corticofugal axons is necessary for guidance of thalamocortical projections from the internal capsule to the neocortex.
Similar to the growth of neocortical axons, that of thalamic axons is promoted by low concentrations of draxin but inhibited by higher concentrations of draxin. The growth-promoting effect of draxin is absent in thalamic neurons from Dcc and Neo1 double mutants. Consistently, Dcc and Neo1 double mutants display a severe thalamocortical phenotype similar to that observed in draxin KO mice. Therefore, we have proposed that draxin from corticofugal axons promotes the growth of thalamocortical axons from the internal capsule to the neocortex and this effect of draxin is mediated through Dcc and Neo1 receptors (Figure 4; Shinmyo et al., 2015). It was shown that netrin-1 is not expressed in neocortical neurons and that no obvious thalamocortical projection defects are observed in the internal capsule of netrin-1 mutants (Braisted et al., 2000). Thus, draxin is likely to be a main player in Neo1- and Dcc-mediated guidance of thalamocortical projections into the neocortex. In our model, corticofugal axons are assumed to attract thalamocortical axons toward the PSPB. It is important to note that this model seems to be inconsistent with previous observations that neocortical and thalamic axons repel each other in vitro (Bagnard et al., 2001), and that bundles of thalamic and neocortical axons are segregated in the internal capsule and the intermediate zone. These facts have raised an intriguing possibility that thalamocortical axons might change their response to corticofugal axons from attraction to repulsion when they encounter each other in the internal capsule. This possibility might be explained by the concentration-dependent effects of draxin on thalamocortical axons. Further investigations are needed to clarify the molecular mechanisms governing reciprocal interactions between corticofugal and thalamocortical axons. It is interesting to note that commissural axons originated from the two hemispheres meet at the midline and continue to extend in opposite directions to reach their targets. This configuration seems to be similar to that of thalamocortical and corticofugal axons in the internal capsule. Since draxin is expressed in commissural neurons of the spinal cord and the neocortex, draxin might regulate axon-axon interactions of commissural neurons around the midline.
Thalamocortical axons originating from distinct nuclei in the dorsal thalamus project to the appropriate cortical areas through the internal capsule (Vanderhaeghen and Polleux, 2004; Garel and Lopez-Bendito, 2014). For instance, ventroanterior/ventrolateral axons project to the primary motor area, ventroposterior medial axons to the primary somatosensory area and dorsolateral geniculate nucleus axons project to the primary visual cortex. This topography is regulated by gradients of axon guidance cues including netrin-1, Slit1 and ephrin-A5 in the corridor and the striatum (Dufour et al., 2003; Bonnin et al., 2007; Powell et al., 2008; Bielle et al., 2011). Netrin-1 mRNA is expressed in a rostral-high to caudal-low gradient in these structures (Bonnin et al., 2007; Powell et al., 2008). This graded expression of netrin-1 has been proposed to play a dual role in attracting rostral thalamic axons in a Dcc-dependent manner and repelling caudal thalamocortical axons in a Dcc-Unc5 receptor-dependent manner (Bonnin et al., 2007; Powell et al., 2008). Draxin mRNA is also expressed in the corridor and the striatum during thalamocortical development (Shinmyo et al., 2015), suggesting that draxin might interact with netrin-1 signaling to generate the topographic organization of thalamocortical projections.
Glycoproteins and Draxin/Netrin-1 Signaling
We have proposed that draxin on the pial surface is critical for the guidance of commissural axons (Figure 2). Glycoproteins such as Dystroglycan and Heparan sulfate proteoglycans (HSPGs) may regulate the localization of draxin on the pial surface. Dystroglycan functions as a scaffold for extracellular matrix (ECM) proteins, which control distributions of secreted proteins (Wright et al., 2012; Lindenmaier et al., 2019). Indeed, ECM proteins that comprise the basement membrane show a fragmented and discontinuous pattern in a mutant of β-1,3-N-acetyl-glucosaminyltransferase-1 (B3gnt1), which is essential for glycosylation of Dystroglycan, suggesting that Dystroglycan is required for basement membrane integrity in the developing spinal cord. Furthermore, localization of Slit in the basement membrane and the FP requires the interaction between Dystroglycan and the C-terminal region of Slit (Wright et al., 2012). In the B3gnt1 mutant, binding of alkaline phosphatase (AP)-tagged Slit2 to the basement membrane is absent. Thus, it is possible that Dystroglycan directly or indirectly regulates the distribution of draxin protein in the basement membrane of the spinal cord. HSPGs, components of the basement membrane, are also possible candidates to regulate the distribution of draxin protein. HSPGs were shown to interact with extracellular ligands such as those of the Wingless/Wnt and HH families and control their distributions (Lee and Chien, 2004; Masu, 2016; De Pasquale and Pavone, 2019). We previously reported that AP-tagged draxin binds many brain regions including the basement membrane in the cerebrum (Islam et al., 2009; Shinmyo et al., 2015). Addition of heparin drastically inhibited binding of draxin to the basement membrane (our unpublished data), suggesting that heparan sulfate (HS) mediates this binding. Therefore, it is plausible that glycoproteins could play crucial roles as regulators of the distribution of draxin on the basement membrane. Interestingly, draxin was shown to regulate basement membrane remodeling during epithelial-to-mesenchymal transition of cranical neural crest cells (Hutchins and Bronner, 2019). This finding might suggest that draxin itself regulates basement membrane integrity in the spinal cord and contributes to the localization of other axon guidance cues in the basement membrane.
It has also been shown that HSPGs play essential roles in modulating the action of axon-guidance ligands and receptors (Lee and Chien, 2004; Masu, 2016). There are several lines of evidence implicating HS and netrin-1/Dcc signaling. Heparin can bind both netrin-1 and Dcc (Serafini et al., 1994; Bennett et al., 1997). In addition, in vitro experiments suggested interaction of HS with Dcc is important for netrin-1 dependent commissural axon outgrowth (Keino-Masu et al., 1996; Bennett et al., 1997). Definitive evidence for a cell-autonomous role of HS in netrin-1-mediated axon guidance was demonstrated by using conditional knockout mice for Ext1, which encodes an enzyme essential for HS synthesis (Zak et al., 2002; Matsumoto et al., 2007). Ablation of Ext1 in the dorsal spinal cord results in commissural axon pathfinding defects that share similarities with those of netrin-1 and Dcc knockout mice. Consistently, Ext1-deficient dorsal spinal cord explants do not respond to netrin-1 in vitro. Furthermore, Ext1-deficient commissural neurons are incapable of inducing intracellular signaling downstream of netrin-1 and Dcc. Thus, expression of HS in commissural neurons is necessary for netrin-1/Dcc-mediated axon guidance.
Although the contribution of HS to draxin-dependent axon guidance is unclear, we suggest that HS might be involved in the concentration-dependent effects of draxin on cortical and thalamic axons (Shinmyo et al., 2015). Neurite outgrowth from cortical and thalamic neurons is promoted by draxin at low concentrations and inhibited at higher concentrations. The growth-promoting effect of draxin is absent in these neurons from Neo1 and Dcc double-deficient mice, suggesting that this effect of draxin is mediated by Dcc and Neo1 receptors. Importantly, the inhibitory effect of draxin is not completely abolished in these neurons from Neo1 and Dcc double-deficient mice, suggesting that, in addition to Dcc and Neo1, other receptors may be necessary for the inhibitory effect of draxin. Our preliminary data demonstrated that the inhibitory effect of draxin on cortical axons was absent following treatment with heparinase III, an enzyme that digests HS (Our unpublished data). This fact suggests that HSPGs might serve as low affinity receptors for draxin and participate in the conversion of draxin-induced attraction into repulsion.
Intracellular Mechanisms of Draxin Signaling
It was shown that mice deficient for MAP1B, which encodes a microtubule-associated protein, have axon guidance defects in the corpus callosum and the hippocampal commissure (Meixner et al., 2000). These phenotypes are similar to those in draxin KO mice (Islam et al., 2009). Meli et al. (2015) demonstrated that draxin-induced growth cone collapse and inhibition of neurite outgrowth are suppressed in MAP1B-deficient cortical neurons. In addition, they showed draxin causes growth cone collapse through interaction with Dcc, inhibition of the phosphatidylinositol 3-kinase (PI3K)/Akt signaling pathway and activation of glycogen synthase kinase-3β (GSK-3β). Thus, it is likely that GSK-3β dependent MAP1B pathway is essential for repulsive draxin signaling. In contrast to draxin repulsion, molecular events in draxin attraction are still unclear. Further investigations are needed to elucidate the intracellular mechanisms underlying the bimodal effects of draxin.
Draxin Signaling in Hippocampal Development
Our phenotypic analyses of draxin KO mice suggest that draxin has multiple roles in hippocampal development (Zhang et al., 2010; Tawarayama et al., 2018a, b, 2020). Importantly, loss of draxin leads to a reduction in the volume of the dentate gyrus, in addition to abnormal projections of the hippocampal commissure and mossy fibers. Consistently, enhanced apoptosis was observed in the developing dentate gyrus of draxin KO mice. Immunostaining with granule cell lineage markers revealed that the number of apoptotic neuroblasts was markedly increased in the dentate gyrus of draxin KO mice. It is known that Dcc belongs to the family of dependence receptors, which trigger apoptosis in the absence of ligands (Mehlen and Thibert, 2004). Indeed, Dcc is mainly expressed in neuroblasts of the dentate gyrus. Furthermore, our in vitro assays suggest that draxin suppresses Dcc-induced apoptosis in differentiating hippocampal neural cells. Thus, it is likely that draxin act as a dependent receptor ligand for Dcc to maintain and promote survival of neuroblasts in the dentate gyrus (Tawarayama, 2018; Tawarayama et al., 2018a).
Conclusion and Perspectives
In this review, we discussed many possible roles of draxin and netrin-1 signaling in the development of neural circuits in the spinal cord and the brain. Further investigations are required to dissect these possibilities. In particular, spatially and temporally confined deletion of the draxin and/or netrin-1 genes will be important to determine the precise roles of these molecules at multiple choice points for axon guidance in the developing CNS.
Studies of axon guidance signaling are critical to advance not only our knowledge of axon guidance mechanisms in the developing CNS, but also our understanding of human neurological disorders caused by axon guidance defects. Multiple human neurological disorders such as intellectual disability, autism spectrum disorders, and schizophrenia are known to be associated with developmental errors in axonal pathfinding (Nugent et al., 2012). It was suggested that mutations in the NETRIN-1 or DCC gene cause congential mirror movements (CMM) because of inappropriate ipsilateral projections of the corticospinal tract from the hindbrain (Srour et al., 2010; Depienne et al., 2011; Meneret et al., 2017). CMM are involuntary symmetrical movements of one side of the body that mirror voluntary movements of the other side. Importantly, draxin KO mice are viable and fertile, even though they have drastic axon guidance defects in the brain. It will therefore be of great interest to investigate whether mutations in the DRAXIN gene are directly linked to human neurological disorders in future studies.
Author Contributions
Both authors listed have made a substantial, direct, and intellectual contribution to the work, and approved it for publication.
Funding
This work was supported by Grant-in-Aid for Scientific Research from the Ministry of Education, Culture, Sports, Science and Technology (MEXT).
Conflict of Interest
The authors declare that the research was conducted in the absence of any commercial or financial relationships that could be construed as a potential conflict of interest.
Publisher’s Note
All claims expressed in this article are solely those of the authors and do not necessarily represent those of their affiliated organizations, or those of the publisher, the editors and the reviewers. Any product that may be evaluated in this article, or claim that may be made by its manufacturer, is not guaranteed or endorsed by the publisher.
Acknowledgments
We apologize to all of the researchers whose work could not be cited due to space limitation. We are grateful to Hiroshi Kawasaki (Kanazawa University), Hirohide Takebayashi (Niigata University), Kunimasa Ohta (Kyushu University), and late Hideaki Tanaka for their continuous encouragement. We thank Toshihide Hamabe-Horiike for helpful discussions and comments on the manuscript.
References
Ahmed, G., Shinmyo, Y., Naser, I. B., Hossain, M., Song, X., and Tanaka, H. (2010). Olfactory bulb axonal outgrowth is inhibited by draxin. Biochem. Biophys. Res. Commun. 398, 730–734. doi: 10.1016/j.bbrc.2010.07.010
Ahmed, G., Shinmyo, Y., Ohta, K., Islam, S. M., Hossain, M., Naser, I. B., et al. (2011). Draxin inhibits axonal outgrowth through the netrin receptor DCC. J. Neurosci. 31, 14018–14023. doi: 10.1523/JNEUROSCI.0943-11.2011
Augsburger, A., Schuchardt, A., Hoskins, S., Dodd, J., and Butler, S. (1999). BMPs as mediators of roof plate repulsion of commissural neurons. Neuron 24, 127–141. doi: 10.1016/s0896-6273(00)80827-2
Aviles, E. C., and Stoeckli, E. T. (2016). Canonical wnt signaling is required for commissural axon guidance. Dev. Neurobiol. 76, 190–208. doi: 10.1002/dneu.22307
Bagnard, D., Chounlamountri, N., Puschel, A. W., and Bolz, J. (2001). Axonal surface molecules act in combination with semaphorin 3a during the establishment of corticothalamic projections. Cereb. Cortex 11, 278–285. doi: 10.1093/cercor/11.3.278
Bennett, K. L., Bradshaw, J., Youngman, T., Rodgers, J., Greenfield, B., Aruffo, A., et al. (1997). Deleted in colorectal carcinoma (DCC) binds heparin via its fifth fibronectin type III domain. J. Biol. Chem. 272, 26940–26946. doi: 10.1074/jbc.272.43.26940
Bielle, F., Marcos-Mondejar, P., Keita, M., Mailhes, C., Verney, C., Nguyen Ba-Charvet, K., et al. (2011). Slit2 activity in the migration of guidepost neurons shapes thalamic projections during development and evolution. Neuron 69, 1085–1098. doi: 10.1016/j.neuron.2011.02.026
Bin, J. M., Han, D., Lai Wing Sun, K., Croteau, L. P., Dumontier, E., Cloutier, J. F., et al. (2015). Complete loss of Netrin-1 results in embryonic lethality and severe axon guidance defects without increased neural cell death. Cell Rep. 12, 1099–1106. doi: 10.1016/j.celrep.2015.07.028
Bonnin, A., Torii, M., Wang, L., Rakic, P., and Levitt, P. (2007). Serotonin modulates the response of embryonic thalamocortical axons to netrin-1. Nat. Neurosci. 10, 588–597. doi: 10.1038/nn1896
Braisted, J. E., Catalano, S. M., Stimac, R., Kennedy, T. E., Tessier-Lavigne, M., Shatz, C. J., et al. (2000). Netrin-1 promotes thalamic axon growth and is required for proper development of the thalamocortical projection. J. Neurosci. 20, 5792–5801. doi: 10.1523/JNEUROSCI.20-15-05792.2000
Brose, K., Bland, K. S., Wang, K. H., Arnott, D., Henzel, W., Goodman, C. S., et al. (1999). Slit proteins bind Robo receptors and have an evolutionarily conserved role in repulsive axon guidance. Cell 96, 795–806. doi: 10.1016/s0092-8674(00)80590-5
Brown, W. S., and Paul, L. K. (2019). The neuropsychological syndrome of agenesis of the corpus callosum. J. Int. Neuropsychol. Soc. 25, 324–330. doi: 10.1017/S135561771800111X
Butler, S. J., and Dodd, J. (2003). A role for BMP heterodimers in roof plate-mediated repulsion of commissural axons. Neuron 38, 389–401. doi: 10.1016/s0896-6273(03)00254-x
Charron, F., Stein, E., Jeong, J., McMahon, A. P., and Tessier-Lavigne, M. (2003). The morphogen sonic hedgehog is an axonal chemoattractant that collaborates with netrin-1 in midline axon guidance. Cell 113, 11–23. doi: 10.1016/s0092-8674(03)00199-5
Charron, F., and Tessier-Lavigne, M. (2007). The Hedgehog, TGF-beta/BMP and Wnt families of morphogens in axon guidance. Adv. Exp. Med. Biol. 621, 116–133. doi: 10.1007/978-0-387-76715-4_9
Chedotal, A. (2019). Roles of axon guidance molecules in neuronal wiring in the developing spinal cord. Nat. Rev. Neurosci. 20, 380–396. doi: 10.1038/s41583-019-0168-7
Chen, Q., Sun, X., Zhou, X. H., Liu, J. H., Wu, J., Zhang, Y., et al. (2013). N-terminal horseshoe conformation of DCC is functionally required for axon guidance and might be shared by other neural receptors. J. Cell Sci. 126, 186–195. doi: 10.1242/jcs.111278
Chen, Y., Magnani, D., Theil, T., Pratt, T., and Price, D. J. (2012). Evidence that descending cortical axons are essential for thalamocortical axons to cross the pallial-subpallial boundary in the embryonic forebrain. PLoS One 7:e33105. doi: 10.1371/journal.pone.0033105
Cheng, H. J., Nakamoto, M., Bergemann, A. D., and Flanagan, J. G. (1995). Complementary gradients in expression and binding of ELF-1 and Mek4 in development of the topographic retinotectal projection map. Cell 82, 371–381. doi: 10.1016/0092-8674(95)90426-3
Comer, J. D., Alvarez, S., Butler, S. J., and Kaltschmidt, J. A. (2019). Commissural axon guidance in the developing spinal cord: from Cajal to the present day. Neural Dev. 14:9. doi: 10.1186/s13064-019-0133-1
De Pasquale, V., and Pavone, L. M. (2019). Heparan sulfate proteoglycans: the sweet side of development turns sour in mucopolysaccharidoses. Biochim. Biophys. Acta Mol. Basis Dis. 1865:165539. doi: 10.1016/j.bbadis.2019.165539
Deck, M., Lokmane, L., Chauvet, S., Mailhes, C., Keita, M., Niquille, M., et al. (2013). Pathfinding of corticothalamic axons relies on a rendezvous with thalamic projections. Neuron 77, 472–484. doi: 10.1016/j.neuron.2012.11.031
Depienne, C., Cincotta, M., Billot, S., Bouteiller, D., Groppa, S., Brochard, V., et al. (2011). A novel DCC mutation and genetic heterogeneity in congenital mirror movements. Neurology 76, 260–264. doi: 10.1212/WNL.0b013e318207b1e0
Dickson, B. J. (2002). Molecular mechanisms of axon guidance. Science 298, 1959–1964. doi: 10.1126/science.1072165
Dominici, C., Moreno-Bravo, J. A., Puiggros, S. R., Rappeneau, Q., Rama, N., Vieugue, P., et al. (2017). Floor-plate-derived netrin-1 is dispensable for commissural axon guidance. Nature 545, 350–354. doi: 10.1038/nature22331
Drescher, U., Kremoser, C., Handwerker, C., Loschinger, J., Noda, M., and Bonhoeffer, F. (1995). In vitro guidance of retinal ganglion cell axons by RAGS, a 25 kDa tectal protein related to ligands for Eph receptor tyrosine kinases. Cell 82, 359–370. doi: 10.1016/0092-8674(95)90425-5
Ducuing, H., Gardette, T., Pignata, A., Tauszig-Delamasure, S., and Castellani, V. (2019). Commissural axon navigation in the spinal cord: a repertoire of repulsive forces is in command. Semin. Cell Dev. Biol. 85, 3–12. doi: 10.1016/j.semcdb.2017.12.010
Dudanova, I., and Klein, R. (2013). Integration of guidance cues: parallel signaling and crosstalk. Trends Neurosci. 36, 295–304. doi: 10.1016/j.tins.2013.01.007
Dufour, A., Seibt, J., Passante, L., Depaepe, V., Ciossek, T., Frisen, J., et al. (2003). Area specificity and topography of thalamocortical projections are controlled by ephrin/Eph genes. Neuron 39, 453–465. doi: 10.1016/s0896-6273(03)00440-9
Edwards, T. J., Sherr, E. H., Barkovich, A. J., and Richards, L. J. (2014). Clinical, genetic and imaging findings identify new causes for corpus callosum development syndromes. Brain 137, 1579–1613. doi: 10.1093/brain/awt358
Fazeli, A., Dickinson, S. L., Hermiston, M. L., Tighe, R. V., Steen, R. G., Small, C. G., et al. (1997). Phenotype of mice lacking functional Deleted in colorectal cancer (Dcc) gene. Nature 386, 796–804. doi: 10.1038/386796a0
Finci, L. I., Kruger, N., Sun, X., Zhang, J., Chegkazi, M., Wu, Y., et al. (2014). The crystal structure of netrin-1 in complex with DCC reveals the bifunctionality of netrin-1 as a guidance cue. Neuron 83, 839–849. doi: 10.1016/j.neuron.2014.07.010
Fothergill, T., Donahoo, A. L., Douglass, A., Zalucki, O., Yuan, J., Shu, T., et al. (2014). Netrin-DCC signaling regulates corpus callosum formation through attraction of pioneering axons and by modulating Slit2-mediated repulsion. Cereb. Cortex 24, 1138–1151. doi: 10.1093/cercor/bhs395
Gao, X., Metzger, U., Panza, P., Mahalwar, P., Alsheimer, S., Geiger, H., et al. (2015). A floor-plate extracellular protein-protein interaction screen identifies Draxin as a secreted Netrin-1 antagonist. Cell Rep. 12, 694–708. doi: 10.1016/j.celrep.2015.06.047
Garel, S., and Lopez-Bendito, G. (2014). Inputs from the thalamocortical system on axon pathfinding mechanisms. Curr. Opin. Neurobiol. 27, 143–150. doi: 10.1016/j.conb.2014.03.013
Ghosh, A., Antonini, A., McConnell, S. K., and Shatz, C. J. (1990). Requirement for subplate neurons in the formation of thalamocortical connections. Nature 347, 179–181. doi: 10.1038/347179a0
Ghosh, A., and Shatz, C. J. (1993). A role for subplate neurons in the patterning of connections from thalamus to neocortex. Development 117, 1031–1047. doi: 10.1242/dev.117.3.1031
Gobius, I., Morcom, L., Suarez, R., Bunt, J., Bukshpun, P., Reardon, W., et al. (2016). Astroglial-mediated remodeling of the interhemispheric midline is required for the formation of the corpus callosum. Cell Rep. 17, 735–747. doi: 10.1016/j.celrep.2016.09.033
Hakanen, J., and Salminen, M. (2015). Defects in neural guidepost structures and failure to remove leptomeningeal cells from the septal midline behind the interhemispheric fusion defects in Netrin1 deficient mice. Int. J. Dev. Neurosci. 47, 206–215. doi: 10.1016/j.ijdevneu.2015.08.005
Hansen, M. J., Dallal, G. E., and Flanagan, J. G. (2004). Retinal axon response to ephrin-as shows a graded, concentration-dependent transition from growth promotion to inhibition. Neuron 42, 717–730. doi: 10.1016/j.neuron.2004.05.009
Hutchins, E. J., and Bronner, M. E. (2019). Draxin alters laminin organization during basement membrane remodeling to control cranial neural crest EMT. Dev. Biol. 446, 151–158. doi: 10.1016/j.ydbio.2018.12.021
Islam, S. M., Shinmyo, Y., Okafuji, T., Su, Y., Naser, I. B., Ahmed, G., et al. (2009). Draxin, a repulsive guidance protein for spinal cord and forebrain commissures. Science 323, 388–393. doi: 10.1126/science.1165187
Keino-Masu, K., Masu, M., Hinck, L., Leonardo, E. D., Chan, S. S., Culotti, J. G., et al. (1996). Deleted in Colorectal Cancer (DCC) encodes a netrin receptor. Cell 87, 175–185. doi: 10.1016/s0092-8674(00)81336-7
Kennedy, T. E., Serafini, T., de la Torre, J. R., and Tessier-Lavigne, M. (1994). Netrins are diffusible chemotropic factors for commissural axons in the embryonic spinal cord. Cell 78, 425–435. doi: 10.1016/0092-8674(94)90421-9
Kennedy, T. E., Wang, H., Marshall, W., and Tessier-Lavigne, M. (2006). Axon guidance by diffusible chemoattractants: a gradient of netrin protein in the developing spinal cord. J. Neurosci. 26, 8866–8874. doi: 10.1523/JNEUROSCI.5191-05.2006
Kidd, T., Bland, K. S., and Goodman, C. S. (1999). Slit is the midline repellent for the robo receptor in Drosophila. Cell 96, 785–794. doi: 10.1016/s0092-8674(00)80589-9
Kolodkin, A. L., Matthes, D. J., and Goodman, C. S. (1993). The semaphorin genes encode a family of transmembrane and secreted growth cone guidance molecules. Cell 75, 1389–1399. doi: 10.1016/0092-8674(93)90625-z
Kolodkin, A. L., and Tessier-Lavigne, M. (2011). Mechanisms and molecules of neuronal wiring: a primer. Cold Spring Harb. Perspect. Biol. 3:a001727. doi: 10.1101/cshperspect.a001727
Kolpak, A., Zhang, J., and Bao, Z. Z. (2005). Sonic hedgehog has a dual effect on the growth of retinal ganglion axons depending on its concentration. J. Neurosci. 25, 3432–3441. doi: 10.1523/JNEUROSCI.4938-04.2005
Lee, J. S., and Chien, C. B. (2004). When sugars guide axons: insights from heparan sulphate proteoglycan mutants. Nat. Rev. Genet. 5, 923–935. doi: 10.1038/nrg1490
Leyva-Diaz, E., and Lopez-Bendito, G. (2013). In and out from the cortex: development of major forebrain connections. Neuroscience 254, 26–44. doi: 10.1016/j.neuroscience.2013.08.070
Lindenmaier, L. B., Parmentier, N., Guo, C., Tissir, F., and Wright, K. M. (2019). Dystroglycan is a scaffold for extracellular axon guidance decisions. eLife 8:e42143. doi: 10.7554/eLife.42143
Lindwall, C., Fothergill, T., and Richards, L. J. (2007). Commissure formation in the mammalian forebrain. Curr. Opin. Neurobiol. 17, 3–14. doi: 10.1016/j.conb.2007.01.008
Liu, Y., Bhowmick, T., Liu, Y., Gao, X., Mertens, H. D. T., Svergun, D. I., et al. (2018). Structural basis for Draxin-modulated axon guidance and fasciculation by Netrin-1 through DCC. Neuron 97, 1261–1267.e4. doi: 10.1016/j.neuron.2018.02.010
Lopez-Bendito, G., and Molnar, Z. (2003). Thalamocortical development: how are we going to get there? Nat. Rev. Neurosci. 4, 276–289. doi: 10.1038/nrn1075
Luo, Y., Raible, D., and Raper, J. A. (1993). Collapsin: a protein in brain that induces the collapse and paralysis of neuronal growth cones. Cell 75, 217–227. doi: 10.1016/0092-8674(93)80064-l
Masu, M. (2016). Proteoglycans and axon guidance: a new relationship between old partners. J. Neurochem. 139, 58–75. doi: 10.1111/jnc.13508
Matsumoto, Y., Irie, F., Inatani, M., Tessier-Lavigne, M., and Yamaguchi, Y. (2007). Netrin-1/DCC signaling in commissural axon guidance requires cell-autonomous expression of heparan sulfate. J. Neurosci. 27, 4342–4350. doi: 10.1523/JNEUROSCI.0700-07.2007
McConnell, S. K., Ghosh, A., and Shatz, C. J. (1989). Subplate neurons pioneer the first axon pathway from the cerebral cortex. Science 245, 978–982. doi: 10.1126/science.2475909
Mehlen, P., and Thibert, C. (2004). Dependence receptors: between life and death. Cell. Mol. Life Sci. 61, 1854–1866. doi: 10.1007/s00018-004-3467-7
Meijers, R., Smock, R. G., Zhang, Y., and Wang, J. H. (2020). Netrin synergizes signaling and adhesion through DCC. Trends Biochem. Sci. 45, 6–12. doi: 10.1016/j.tibs.2019.10.005
Meixner, A., Haverkamp, S., Wassle, H., Fuhrer, S., Thalhammer, J., Kropf, N., et al. (2000). MAP1B is required for axon guidance and Is involved in the development of the central and peripheral nervous system. J. Cell Biol. 151, 1169–1178. doi: 10.1083/jcb.151.6.1169
Meli, R., Weisova, P., and Propst, F. (2015). Repulsive axon guidance by Draxin is mediated by protein Kinase B (Akt), glycogen synthase kinase-3beta (GSK-3beta) and microtubule-associated protein 1B. PLoS One 10:e0119524. doi: 10.1371/journal.pone.0119524
Meneret, A., Franz, E. A., Trouillard, O., Oliver, T. C., Zagar, Y., Robertson, S. P., et al. (2017). Mutations in the netrin-1 gene cause congenital mirror movements. J. Clin. Invest. 127, 3923–3936. doi: 10.1172/JCI95442
Miyake, A., Takahashi, Y., Miwa, H., Shimada, A., Konishi, M., and Itoh, N. (2009). Neucrin is a novel neural-specific secreted antagonist to canonical Wnt signaling. Biochem. Biophys. Res. Commun. 390, 1051–1055. doi: 10.1016/j.bbrc.2009.10.113
Molnar, Z., Adams, R., Goffinet, A. M., and Blakemore, C. (1998). The role of the first postmitotic cortical cells in the development of thalamocortical innervation in the reeler mouse. J. Neurosci. 18, 5746–5765. doi: 10.1523/JNEUROSCI.18-15-05746.1998
Morales, D. (2018). A new model for netrin1 in commissural axon guidance. J. Neurosci. Res. 96, 247–252. doi: 10.1002/jnr.24117
Morcom, L., Gobius, I., Marsh, A. P., Suarez, R., Lim, J. W., Bridges, C., et al. (2021b). DCC regulates astroglial development essential for telencephalic morphogenesis and corpus callosum formation. eLife 10:e61769. doi: 10.7554/eLife.61769
Morcom, L., Edwards, T. J., Rider, E., Jones-Davis, D., Lim, J. W., Chen, K. S., et al. (2021a). DRAXIN regulates interhemispheric fissure remodelling to influence the extent of corpus callosum formation. eLife 10:e61618. doi: 10.7554/eLife.61618
Moreno-Bravo, J. A., Roig Puiggros, S., Mehlen, P., and Chedotal, A. (2019). Synergistic activity of floor-plate- and ventricular-zone-derived Netrin-1 in spinal cord commissural axon guidance. Neuron 101, 625–634.e3. doi: 10.1016/j.neuron.2018.12.024
Naser, I. B., Su, Y., Islam, S. M., Shinmyo, Y., Zhang, S., Ahmed, G., et al. (2009). Analysis of a repulsive axon guidance molecule, draxin, on ventrally directed axon projection in chick early embryonic midbrain. Dev. Biol. 332, 351–359. doi: 10.1016/j.ydbio.2009.06.004
Nishikimi, M., Oishi, K., and Nakajima, K. (2013). Axon guidance mechanisms for establishment of callosal connections. Neural Plast. 2013:149060. doi: 10.1155/2013/149060
Nugent, A. A., Kolpak, A. L., and Engle, E. C. (2012). Human disorders of axon guidance. Curr. Opin. Neurobiol. 22, 837–843. doi: 10.1016/j.conb.2012.02.006
Paul, L. K., Brown, W. S., Adolphs, R., Tyszka, J. M., Richards, L. J., Mukherjee, P., et al. (2007). Agenesis of the corpus callosum: genetic, developmental and functional aspects of connectivity. Nat. Rev. Neurosci. 8, 287–299. doi: 10.1038/nrn2107
Placzek, M., Tessier-Lavigne, M., Jessell, T., and Dodd, J. (1990). Orientation of commissural axons in vitro in response to a floor plate-derived chemoattractant. Development 110, 19–30. doi: 10.1242/dev.110.1.19
Powell, A. W., Sassa, T., Wu, Y., Tessier-Lavigne, M., and Polleux, F. (2008). Topography of thalamic projections requires attractive and repulsive functions of Netrin-1 in the ventral telencephalon. PLoS Biol. 6:e116. doi: 10.1371/journal.pbio.0060116
Rakic, P., and Yakovlev, P. I. (1968). Development of the corpus callosum and cavum septi in man. J. Comp. Neurol. 132, 45–72. doi: 10.1002/cne.901320103
Raper, J. A., and Kapfhammer, J. P. (1990). The enrichment of a neuronal growth cone collapsing activity from embryonic chick brain. Neuron 4, 21–29. doi: 10.1016/0896-6273(90)90440-q
Ren, T., Zhang, J., Plachez, C., Mori, S., and Richards, L. J. (2007). Diffusion tensor magnetic resonance imaging and tract-tracing analysis of Probst bundle structure in Netrin1- and DCC-deficient mice. J. Neurosci. 27, 10345–10349. doi: 10.1523/JNEUROSCI.2787-07.2007
Richards, L. J., Plachez, C., and Ren, T. (2004). Mechanisms regulating the development of the corpus callosum and its agenesis in mouse and human. Clin. Genet. 66, 276–289. doi: 10.1111/j.1399-0004.2004.00354.x
Rothberg, J. M., Jacobs, J. R., Goodman, C. S., and Artavanis-Tsakonas, S. (1990). slit: an extracellular protein necessary for development of midline glia and commissural axon pathways contains both EGF and LRR domains. Genes Dev. 4, 2169–2187. doi: 10.1101/gad.4.12a.2169
Ruiz de Almodovar, C., Fabre, P. J., Knevels, E., Coulon, C., Segura, I., Haddick, P. C., et al. (2011). VEGF mediates commissural axon chemoattraction through its receptor Flk1. Neuron 70, 966–978. doi: 10.1016/j.neuron.2011.04.014
Serafini, T., Colamarino, S. A., Leonardo, E. D., Wang, H., Beddington, R., Skarnes, W. C., et al. (1996). Netrin-1 is required for commissural axon guidance in the developing vertebrate nervous system. Cell 87, 1001–1014. doi: 10.1016/s0092-8674(00)81795-x
Serafini, T., Kennedy, T. E., Galko, M. J., Mirzayan, C., Jessell, T. M., and Tessier-Lavigne, M. (1994). The netrins define a family of axon outgrowth-promoting proteins homologous to C. elegans UNC-6. Cell 78, 409–424. doi: 10.1016/0092-8674(94)90420-0
Shinmyo, Y., Asrafuzzaman Riyadh, M., Ahmed, G., Bin Naser, I., Hossain, M., Takebayashi, H., et al. (2015). Draxin from neocortical neurons controls the guidance of thalamocortical projections into the neocortex. Nat. Commun. 6:10232. doi: 10.1038/ncomms10232
Silver, J., Lorenz, S. E., Wahlsten, D., and Coughlin, J. (1982). Axonal guidance during development of the great cerebral commissures: descriptive and experimental studies, in vivo, on the role of preformed glial pathways. J. Comp. Neurol. 210, 10–29. doi: 10.1002/cne.902100103
Srour, M., Riviere, J. B., Pham, J. M., Dube, M. P., Girard, S., Morin, S., et al. (2010). Mutations in DCC cause congenital mirror movements. Science 328:592. doi: 10.1126/science.1186463
Tawarayama, H. (2018). Novel function of the chemorepellent draxin as a regulator for hippocampal neurogenesis. Neural Regen. Res. 13, 799–800. doi: 10.4103/1673-5374.232465
Tawarayama, H., Yamada, H., Amin, R., Morita-Fujimura, Y., Cooper, H. M., Shinmyo, Y., et al. (2018a). Draxin regulates hippocampal neurogenesis in the postnatal dentate gyrus by inhibiting DCC-induced apoptosis. Sci. Rep. 8:840. doi: 10.1038/s41598-018-19346-6
Tawarayama, H., Yamada, H., Shinmyo, Y., Tanaka, H., and Ikawa, S. (2018b). The chemorepellent draxin is involved in hippocampal mossy fiber projection. Biochem. Biophys. Res. Commun. 500, 217–223. doi: 10.1016/j.bbrc.2018.04.043
Tawarayama, H., Yamada, H., Amin, R., Morita-Fujimura, Y., Cooper, H. M., Shinmyo, Y., et al. (2020). Draxin-mediated regulation of granule cell progenitor differentiation in the postnatal hippocampal dentate gyrus. Neuroscience 431, 184–192. doi: 10.1016/j.neuroscience.2020.02.005
Tessier-Lavigne, M., and Goodman, C. S. (1996). The molecular biology of axon guidance. Science 274, 1123–1133. doi: 10.1126/science.274.5290.1123
Tessier-Lavigne, M., Placzek, M., Lumsden, A. G., Dodd, J., and Jessell, T. M. (1988). Chemotropic guidance of developing axons in the mammalian central nervous system. Nature 336, 775–778. doi: 10.1038/336775a0
Vanderhaeghen, P., and Polleux, F. (2004). Developmental mechanisms patterning thalamocortical projections: intrinsic, extrinsic and in between. Trends Neurosci. 27, 384–391. doi: 10.1016/j.tins.2004.05.009
Varadarajan, S. G., Kong, J. H., Phan, K. D., Kao, T. J., Panaitof, S. C., Cardin, J., et al. (2017). Netrin1 produced by neural progenitors, not floor pate cells, is required for axon guidance in the spinal cord. Neuron 94, 790–799.e3. doi: 10.1016/j.neuron.2017.03.007
Wright, K. M., Lyon, K. A., Leung, H., Leahy, D. J., Ma, L., and Ginty, D. D. (2012). Dystroglycan organizes axon guidance cue localization and axonal pathfinding. Neuron 76, 931–944. doi: 10.1016/j.neuron.2012.10.009
Wu, Z., Makihara, S., Yam, P. T., Teo, S., Renier, N., Balekoglu, N., et al. (2019). Long-range guidance of spinal commissural axons by Netrin1 and Sonic hedgehog from midline floor plate cells. Neuron 101, 635–647.e4. doi: 10.1016/j.neuron.2018.12.025
Xu, K., Wu, Z., Renier, N., Antipenko, A., Tzvetkova-Robev, D., Xu, Y., et al. (2014). Neural migration. Structures of netrin-1 bound to two receptors provide insight into its axon guidance mechanism. Science 344, 1275–1279. doi: 10.1126/science.1255149
Yam, P. T., and Charron, F. (2013). Signaling mechanisms of non-conventional axon guidance cues: the Shh, BMP and Wnt morphogens. Curr. Opin. Neurobiol. 23, 965–973. doi: 10.1016/j.conb.2013.09.002
Yamauchi, K., Yamazaki, M., Abe, M., Sakimura, K., Lickert, H., Kawasaki, T., et al. (2017). Netrin-1 derived from the ventricular zone, but not the floor plate, directs hindbrain commissural axons to the ventral midline. Sci. Rep. 7:11992. doi: 10.1038/s41598-017-12269-8
Yung, A. R., Nishitani, A. M., and Goodrich, L. V. (2015). Phenotypic analysis of mice completely lacking netrin 1. Development 142, 3686–3691. doi: 10.1242/dev.128942
Zak, B. M., Crawford, B. E., and Esko, J. D. (2002). Hereditary multiple exostoses and heparan sulfate polymerization. Biochim. Biophys. Acta 1573, 346–355. doi: 10.1016/s0304-4165(02)00402-6
Zhang, S., Su, Y., Shinmyo, Y., Islam, S. M., Naser, I. B., Ahmed, G., et al. (2010). Draxin, a repulsive axon guidance protein, is involved in hippocampal development. Neurosci. Res. 66, 53–61. doi: 10.1016/j.neures.2009.09.1710
Keywords: axon guidance, draxin, netrin-1, spinal commissural axons, corpus callosum, thalamocortical projections, glycoproteins
Citation: Ahmed G and Shinmyo Y (2021) Multiple Functions of Draxin/Netrin-1 Signaling in the Development of Neural Circuits in the Spinal Cord and the Brain. Front. Neuroanat. 15:766911. doi: 10.3389/fnana.2021.766911
Received: 30 August 2021; Accepted: 22 October 2021;
Published: 25 November 2021.
Edited by:
Satoru Yamagishi, Hamamatsu University School of Medicine, JapanReviewed by:
Artur Kania, Montreal Clinical Research Institute (IRCM), CanadaJoaquim Egea, Biomedical Research Institute of Lleida, Spain
Copyright © 2021 Ahmed and Shinmyo. This is an open-access article distributed under the terms of the Creative Commons Attribution License (CC BY). The use, distribution or reproduction in other forums is permitted, provided the original author(s) and the copyright owner(s) are credited and that the original publication in this journal is cited, in accordance with accepted academic practice. No use, distribution or reproduction is permitted which does not comply with these terms.
*Correspondence: Yohei Shinmyo, shinmyo@med.kanazawa-u.ac.jp