Advancements in Bioelectricity Generation Through Nanomaterial-Modified Anode Electrodes in Microbial Fuel Cells
- 1Department of Applied Chemistry, Adama Science and Technology University, Adama, Ethiopia
- 2Department of Chemistry, Hawassa University, Hawassa, Ethiopia
The use of nanotechnology in bioelectrochemical systems to recover bioelectricity and metals from waste appears to be a potentially appealing alternative to existing established procedures. This trend exactly characterizes the current renewable energy production technology. Hence, this review focuses on the improvement of the anode electrode by using different functional metal oxide-conducting polymer nanocomposites to enhance microbial fuel cell (MFC) performance. Enhancement of interfacial bioelectrocatalysis between electroactive microorganisms and hierarchical porous nanocomposite materials could enhance cost-effective bioanode materials with superior bioelectrocatalytic activity for MFCs. In this review, improvement in efficiency of MFCs by using iron oxide- and manganese oxide-based polypyrrole hybrid composites as model anode modifiers was discussed. The review also extended to discussing and covering the principles, components, power density, current density, and removal efficiencies of biofuel cell systems. In addition, this research review demonstrates the application of MFCs for renewable energy generation, wastewater treatment, and metal recovery. This is due to having their own unique working principle under mild conditions and using renewable biodegradable organic matter as a direct fuel source.
1 Introduction
Producing a sustainable source of energy to reduce the need for fossil fuels has attracted a lot of attention in green energy generation technology. As a result, several initiatives to enhance the efficiency of renewable energy production through bio-electrochemical energy conversion systems have been launched. This is recommended due to the poor compatibility of precious metal anodes with microorganisms, which is attributable to the lack of surface roughness and can be enhanced by using a conductive polymer. Electronically conducting polymers are distinguished from other materials by their strong electronic conductivity and environmental resilience. Large-scale production of electrodes using flexible and conductive polymers provides a great opportunity to build practical devices for a variety of applications (Mounia and Renouvelables, 2016). Using carbon-free energy sources, particularly renewables, should be pursued aggressively. The current rate of growth in renewable energy production suggests that fossil fuels will not be completely displaced in the foreseeable future (Lueking and Cole, 2017). Better environmental performance is promoted through the reduction of waste and emissions at the source through more efficient use of energy and materials in the areas of nanotechnology and nanoscience (Sagastume Gutiérrez et al., 2018).
Today, light-based photocatalytic fuel cells (Zeng et al., 2018;Khan M. E. et al., 2018) and microorganism-based bioelectrochemical conversion device systems such as electro-microbiology (Ali et al., 2018) or microbial fuel cells (MFCs) have emerged as promising tools for the sustainability of renewable energy generation, simultaneous wastewater treatment, and water purification in the bioenergy sector (Mahrokh et al., 2017;Bakonyi et al., 2018). As a sustainable technology, biofuel cells (BFCs) have recently attracted a great deal of attention due to their unique ability to directly convert stored chemical energy from biodegradable substances to electricity, as shown in Figure 1 (Barelli et al., 2021). Parts of BFCs known as enzymatic biofuel cells (EBFCs) and microorganism-based biofuel cells (MFCs) are the two most important components of the BFC system for such conversion concepts, in which devices with renewable redox enzymes and/or microorganisms are used as catalysts to perform natural oxidation processes at the anode for the release of electrons and the generation of an electrical current (Zhao et al., 2017). Recently, green-synthesized nanocatalyst-modified anode materials played an effective and value-added role in energy conversion systems (Dessie et al., 2021b). Hence, a biosynthesized nanocatalyst hybrid with a conductive polymer matrix-modified electrode has shown novel technological advances in renewable energy production simultaneously with wastewater treatment application (Dessie et al., 2021c).
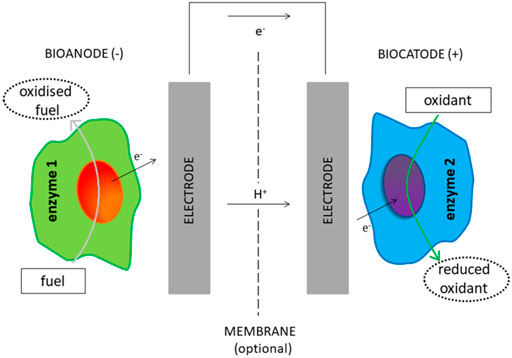
FIGURE 1. Schematic representation of enzymatic biofuel cells (Barelli et al., 2021).
In BFCs, the role of nanomaterials in treating waste or synthesizing the nanomaterial itself from various biowaste materials has been a qualified strategy for the conversion of waste into useful and reusable forms (Samaddar et al., 2018). As a result, improving the efficiency of MFCs using a nanocomposite nanomaterial electrode as a catalyst is critical for low-cost and efficient energy harvesting systems (Kodali et al., 2018). Such improvement has a strong contribution to the assembly of strong nanocomposite materials hybridized from renewable forestry waste (Chen et al., 2018), two dimensional layered nanomaterials (Cao et al., 2018), microscale and nanoscale electrode topography (Champigneux et al., 2018), their dimensional mesoporous electrodes (Bian et al., 2018), smart polymers (Yousefi et al., 2018), and conducting polymers with other nanomaterials as an electrode; in addition, membranes may offer performance improvement in MFC devices due to their synergistic effect (Di Palma et al., 2018;Mulijani et al., 2018). Among nanomaterials, conducting polymers from polyaniline (PANI) and polypyrrole (PPy) were chosen as the basic monomer material to prepare the nanocomposites because of their unique physical, chemical, structural, and optical capabilities. They are selected due to the nature of the nitrogen environment on the redox polymer backbone, which impacts an electrode material’s performance for the MFC device setup (Dumitru et al., 2018).
According to the results of practical study, coating a single bacterial cell with a conjugated polymer/redox polymer results in a high-performance anode for MFC application (Song et al., 2017). These polymers due to their inexpensiveness, biocompatible nature (Singh et al., 2018), and flexibility in designing electrodes (Sushma and S, 2017) can increase efficiency in MFC devices. Stainless steel (SS) is one of the most obvious electrode materials in bioelectrocatalytic systems. However, its smooth surface decreases the deposition of the active bacterial community. In addition, its poor biocompatibility and low corrosion resistance have made it unusable indefinitely. To address these issues, a unique structural SS-based anode for high-performance MFCs was successfully modified by in-situ electrochemical deposition of PPy onto SS (Pu et al., 2018).
2 Bioelectrocatalysis
Application of novel nanomaterials for pollutant degradation has been the recent advanced technology in nanoscience (Zheng et al., 2021). Such nanomaterials in microbial electrochemical technologies have oxidized various types of organic pollutants and can lead to chemical synthesis, bioremediation of polluted matrices, contaminant treatment, and electricity production. These technologies have been based on the presence of electroactive bacteria through metabolic activity in solid-state electrodes (Ramírez-Vargas et al., 2018). In MFCs, interfacial bioelectrocatalysis between electroactive bacteria and hierarchical porous composite materials could improve the cost-effectiveness of anode materials with superior bioelectrocatalytic activity of MFCs (Wu et al., 2018a). Therefore, the performance of MFCs is considerably improved as a result of this interaction (Jiang Q. et al., 2018). Within biological processes, nature has emerged as a roadmap for the creation of microbial electrochemical technologies. Ultimately, it is a consequent use from sustainable energy conversion to bioenvironmental and biosensor technologies (Schröder and Harnisch, 2017; Dessie and Tadesse, 2022). For implementing MFCs, it is critical to reduce the start-up time while also improving the energy-generating performance (Zhong et al., 2018).
2.1 Microbial Fuel Cell
Fuel cells have the potential to become an important energy tool for management, but their technical and economic practicality must be enhanced (Inamuddin et al., 2017). The increasing energy demand and the negative environmental implications of power production from irreversible fossil fuel usage have sparked scientific interest in using biotechnology to harvest energy from various organic waste sources. Converting such renewable organic matter using MFCs has been widely regarded as the most promising source of renewable energy among other microbial technologies (Xia et al., 2018). The technology allows for direct electricity harvesting from organics via anodic microbial catalysis, as shown in Figure 2, and has been utilized to extract electrical energy from organic sewage (Zhao et al., 2017). Energy conversion using MFCs appeared to be a promising technique for the remediation of environmental toxins in terms of efficient removal of different organic chemicals and energy recovery (Zhang Q. et al., 2018). As a result, it is a viable long-term solution to the energy and environmental crises (Saravanan, 2018). Even from organic wastes beyond their bioelectricity production, it is also possible to produce different chemicals (e.g., methane and methanol) using novel microbes through fermentation reactions (Dhiman et al., 2018). Thus, to recover energy from methane, MFCs, on the other hand, can reduce dissolved methane emissions from anaerobic effluents (Chen and Smith, 2018). To fulfill such a requirement, researchers sometimes immobilize microbes on carbon nanotubes (CNTs) to improve the electrode/anodic performance because nanotubes have a long-term electrochemical property effect, which helps enhance power output (Zhang Y. et al., 2018). Other processes such as hydrolysis and acidogenesis can also improve wastewater treatment performance and power generation simultaneously in MFCs by reducing internal losses, i.e., by minimizing the internal resistance of the cell (López Zavala et al., 2018).
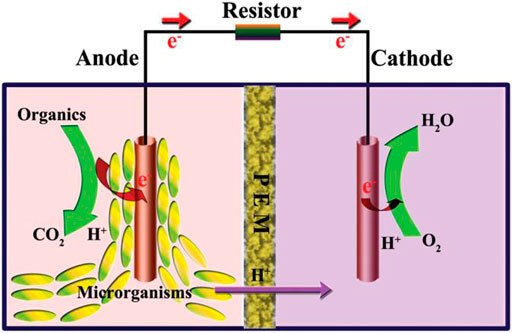
FIGURE 2. Schematics of double chambered microbial fell cells (Zhao et al., 2017).
MFC technology has piqued the interest of scientists over the last half a century due to its ability to convert organic waste directly into electricity using microbially catalyzed anodic and microbial/enzymatic/abiotic cathodic electrochemical reactions under moderate conditions (Santoro et al., 2017). Using bacterial metabolism, the cell device can produce alternating energy from a wide variety of organic substrates under these conditions (Liu et al., 2017). The generated current is mostly created by the metabolic activities of microorganisms. It would be beneficial to learn more about microbial systems’ ability to optimize energy extraction methods in MFCs. The most likely use of this technology appears to be large-scale coupling of such a device with treating wastewater (Krieg et al., 2017). As a result, MFCs have risen to prominence in recent years as a novel technique for converting chemical energy, especially from carbon-rich organic matter, into power generation through a sequence of energy metabolisms mediated by electrochemically active microorganisms (Chandrasekhar and Ahn, 2017). The effluents in the anodic chamber is digested by microorganisms, generating electrons (e-), which are received by the anode and transmitted to the cathode via load resistance in a standard MFC principle and setup, as shown in Figure 3. At the same time, the released protons (H+) created during the metabolism reach the cathode via proton exchange membranes, which are H+ exchangers. An electron acceptor accepts e− and H+ at the cathode, completing the circuit and providing electricity (Capodaglio et al., 2013; Deval et al., 2017). Ion membranes—either cation or anion exchange membranes—are widely used in microbial fuel cells (Jiang S. et al., 2018). Nafion is a commercially available fuel cell ion exchange membrane; however, it has drawbacks such as low conductivity at low humidity and low-stability at high temperatures (Peo-nio-liclo, 2010). In addition to reduced cost, the advancements of electroactive-based carbon nanotube membranes are reviewed in detail (Liu et al., 2020).
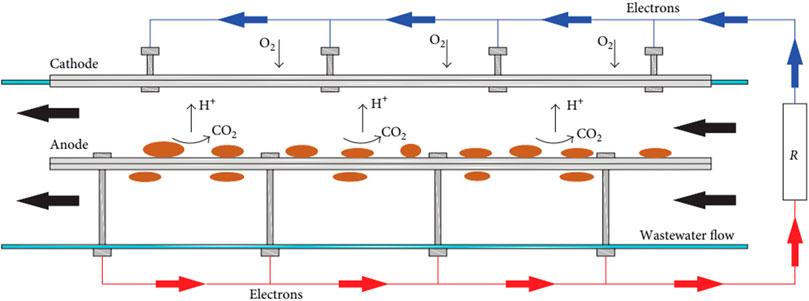
FIGURE 3. Working principle of the MFC prototype (Capodaglio et al., 2013).
For example, the bacterial microorganism Chlorella vulgaris (Huarachi-Olivera et al., 2018), which is a chlorophyte microalga, has been used to cleanse wastewater and create oxygen at the cathode of MFCs, accumulating algal biomass and creating power in bioindustrial settings. In addition to anolytes, the bioelectrochemical performance of MFCs was examined using various catholytes, such as phosphate buffer and anode effluents, in the presence or absence of C. vulgaris (Commault et al., 2017). The presence of exoelectrogenic microorganisms increases the performance of MFCs, which can be further improved by using a nanocomposite cathode catalyst (Kodali et al., 2018). Since ancient times, biomass, a sustainable fuel derived from biological materials, including plant and animal waste, has been utilized as the major energy source (Hoa et al., 2017). Due to enhanced efficiency levels in terms of energy and catholyte production, the use of ceramics as low-cost membrane materials for MFCs is gaining traction. Water or hydrogen peroxide creation from the oxygen reduction reaction in the cathode, water diffusion, and electroosmotic drag through the ion exchange membrane contribute to catholyte production in ceramic MFCs, as shown in Figure 4 (Gajda et al., 2020). Thus, the ceramic membrane’s efficient performance shows its appropriateness as a feasible and economical choice for speeding up the scale-up process and expanding the use of MFC technology.
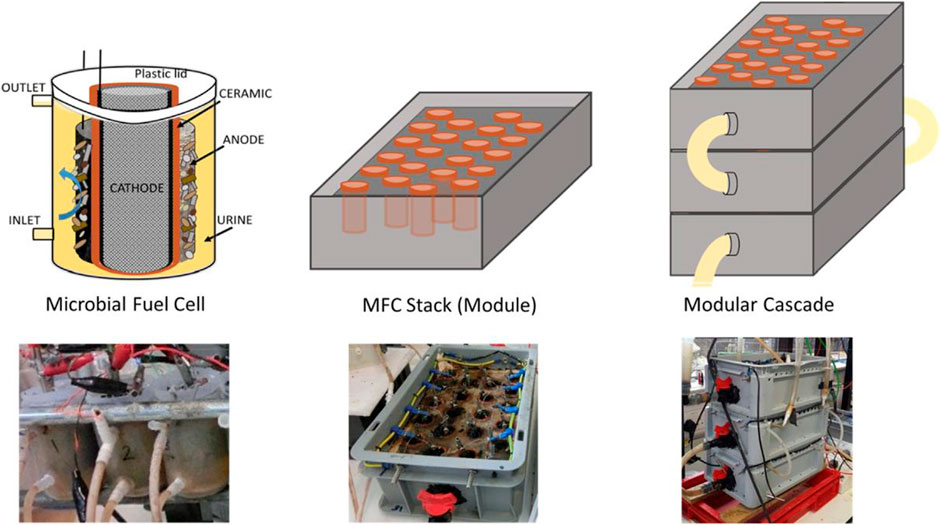
FIGURE 4. (i) Individual MFCs (triplicate), (ii) those assembled in a 22-MFC module (stack), and (iii) those in a 3-module cascade (Gajda et al., 2020).
There is currently a scarcity of data on the elements that influence the power generation of MFCs that use soil organic matter, sewage sludge, marine sediment, garden compost, industrial and home waste, and animal waste as fuel sources (Abbas et al., 2018; On et al., 2017). Integrating all elemental issues in the bioprocesses may be able to boost the overall yield and efficiency of the process. Especially, using an integrated system, energy recovery from water hyacinth can be improved even today (Varanasi et al., 2018). Furthermore, municipal solid waste is employed as a valuable power generator for global energy recovery (Abdel-Shafy and Mansour, 2018). Conversion of environmentally friendly municipal solid wastes into fuels and chemicals is also beneficial to the society (Matsakas et al., 2017). However, enhancing power generation in low-light settings and efficiently collecting electrons from photosynthetic bacteria on the electrode are also key difficulties in the development of a functional photosynthetic microbial fuel cell (PMFC). To address these issues, an anode was developed in a dual-chambered PMFC with an abiotic cathode by casting a nanocomposite matrix that assisted biofilm development of the photo-catalyst Synechococcus sp., surging the bacterial photosystems (PS I and PS II) with suitable light (λ650–750 nm) at a broad excitation spectrum (λ350–644 nm) through fluorescence resonance energy transfer (Kaushik et al., 2017). Researchers have established that an adequate microbial population structure and metabolic activity of the electrode biofilm communities are required for satisfying the performance of microbial electrochemical cells using this conversion technology (Liu et al., 2017).
In air-cathode single-chamber MFCs, electrode improvement and optimization are also ongoing (Wang Y. et al., 2018). Extracellular electron transfer of surface active microbes (Li F. et al., 2018) as well as precise electron carriers events of electron rich microbial species for electron transport functionality (e.g., biofilm formation, electron shuttles, swarming motility, dye decolorization, and bioelectricity generation) to MFCs aids in the generation of more stable and reliable power (Ng et al., 2017). Due to such benefits, MFCs have a lot of practical applications. Some of them are bioremediation and wastewater treatment, removal and recovery of heavy metals, constructed wasteland management, water desalination, biophotovoltaics, biosensors as alternate power tools, and biochemical production via microbial electrosynthesis (Mounia and Renouvelables, 2016). The detailed comparison of MFC performances from previous works using different anodes is clearly summarized and shown in Table 1.
2.1.1 Bioelectricity Generation
A biofilm developed from electron-releasing microorganisms facilitates renewable bioelectricity generation as well as organic carbon removal from waste matter simultaneously, as shown in Figure 5 (Angelaalincy et al., 2018). MFCs are a notable source of sustainable energy due to the degradation in antibiotics and changes in their toxicity (Wang J. et al., 2018) followed by the conversion to power (bioelectricity production) via a biological process. MFCs have been studied for bioelectricity production through organic wastewater degradation by a microbial consortium. For example, methanogenesis/methane (Yamasaki et al., 2018), photosynthesis (Chandra et al., 2017), soil (Li et al., 2019), ceramic (Santoro et al., 2018a), sediment-rich benthos (González-Gamboa et al., 2018), marine benthos (Chen et al., 2017b), novel earthen pot-plants (Regmi et al., 2018a), and xylose-fed mesophiles and thermophiles (Dessì et al., 2018) help plant-assisted MFCs turn solar energy into bioelectricity due to their unique plant–microbe association at the rhizosphere zone of a plant (Regmi et al., 2018b), which are interesting modification devices of MFCs, and they demonstrate how alternative electrodes can be used to boost efficiency. PMFCs are different from regular MFCs, where they produce bioelectricity and biomass in-house rather than relying on external substrates (Nitisoravut and Regmi, 2017). Using a dual chamber, electricity can be generated from the empty fruit bunches of palm oil trees. MFCs are also a typical device system (Ghazali et al., 2017), and they are gaining popularity due to their ability to generate power from a range of substrates by recycling organic wastes. As a result, using animal excrement and leaf mold as a bioelectricity-producing substrate has a lot of promise (Gazali and Moqsud, 2017).
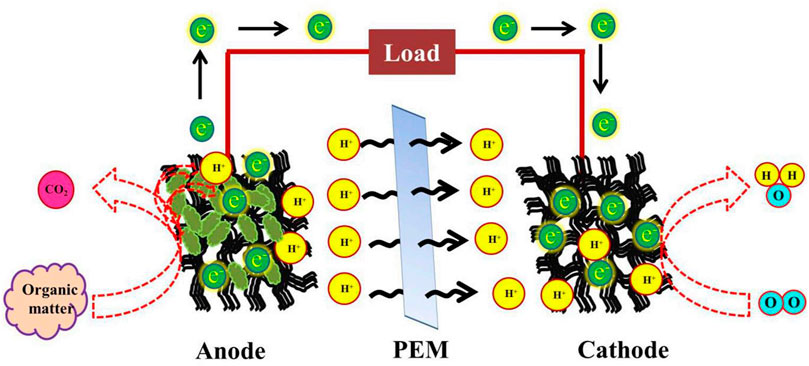
FIGURE 5. Using photosynthetic microorganisms at the anode, an MFC can reduce waste while also producing power (Angelaalincy et al., 2018).
MFC technology is developing as an appealing approach for mitigating pollution in water bodies by combining electricity generation and waste degradation. In this device system, biodegradation of hazardous chemicals using a nanocomposite polymer-coated nanocomposite-based anode can improve dye degradation and power output (Sarma et al., 2018; Dessie et al., 2021a). For example, algae are well-known organisms whose characteristics are prominent in biofuel production and wastewater remediation (Vo Hoang Nhat et al., 2018). Integration of algae and cyanobacteria in MFC bioelectrochemical systems (BESs) can boost energy recovery and nutrient remediation in wastewater treatment dramatically. Algae can contribute to the BES function as an organic feedstock to support bacterial growth by supporting anode bacteria in generating electricity, delivering oxygen through photosynthesis as a cathode electron acceptor, and eliminating nitrogen and phosphorus from effluent water (Luo et al., 2017). It can be concluded that high specific surface area, low cost, strong electrical conductivity, and biocompatibility of anodic materials enhance performance efficiency of MFC systems.
2.1.2 Wastewater Treatment
Because of their high organic matter and nutrient content, wastes from concentrated animal feeding facilities, such as domestic wastes (Han et al., 2018a), plastic waste (Saleem et al., 2018), food waste (Salihoglu et al., 2018; Kibler et al., 2018; Dahiya et al., 2018), agricultural waste (Dai et al., 2018), poultry droppings waste (Oyiwona et al., 2018), fruit waste (Han et al., 2018b), and medical waste (Hong et al., 2018), are difficult to manage. Traditional swine waste treatment methods, such as exposed anaerobic lagoons, produce poor effluent quality and emit greenhouse gases, while modern treatment comes at a significant cost (Amini et al., 2017). Thermal, mechanical, chemical, and biological treatments of asbestos-containing wastes do not completely address the difficulties associated with asbestos fiber release, and they are incompatible with the concepts of sustainable land use, recycling, and material cycle closure (Spasiano and Pirozzi, 2017). Strategies for encouraging the conversion of food waste into bioenergy by anaerobic digestion, in addition to MFCs, may be an additional treatment option (De Clercq et al., 2017).
One of the most prevalent approaches is to use rumen fluid in the production of energy from market waste (such as avocado fruit and tomato wastes) utilizing MFC technology (JM et al., 2018). As a result, MFCs have emerged as a promising technology for self-powered, real-time, on-site wastewater treatment plants in large-scale applications. MFCs, on the other hand, can become a significant instrument for water quality monitoring if their manufacturing costs are drastically reduced, making them accessible even in the poorest and most distant parts of the world (Chouler et al., 2017). Today, platinum group metal-free catalysts (Santoro et al., 2018b), particularly from manganese (Mn), iron (Fe), cobalt (Co), and nickel (Ni), as well as their integration into an air-breathing cathode (Gajda et al., 2018), are used as bio-electrochemical catalysts to treat wastewater and generate electricity (Kodali et al., 2017). In addition to using metal free-catalysts, electrode materials have a huge effect on the performance and cost of MFCs (Zhang Y. et al., 2017). As a result, a unique electrode fabrication using low-cost, stable, and biocompatible metals aids in improving power generation in MFCs (Yamashita and Yokoyama 2018). In the power generation system, active microorganisms can transmit electrons to the anode in wastewater treatment mechanisms in three ways: using exogenous mediators, using mediators created by bacteria, or by directly transferring electrons from respiratory enzymes (i.e., cytochromes) to the electrodes (see Figure 6) (Hassan et al., 2021). In brief, two alternative and simple electrodes, one for each stacked MFC, were used to prove this mechanism: granular graphite and stainless steel mesh. In biocathodes, organic matter was oxidized, ammonium was oxidized to nitrate in an external aerated reactor, and nitrate was reduced to dinitrogen gas (Vilajeliu-Pons et al., 2017).
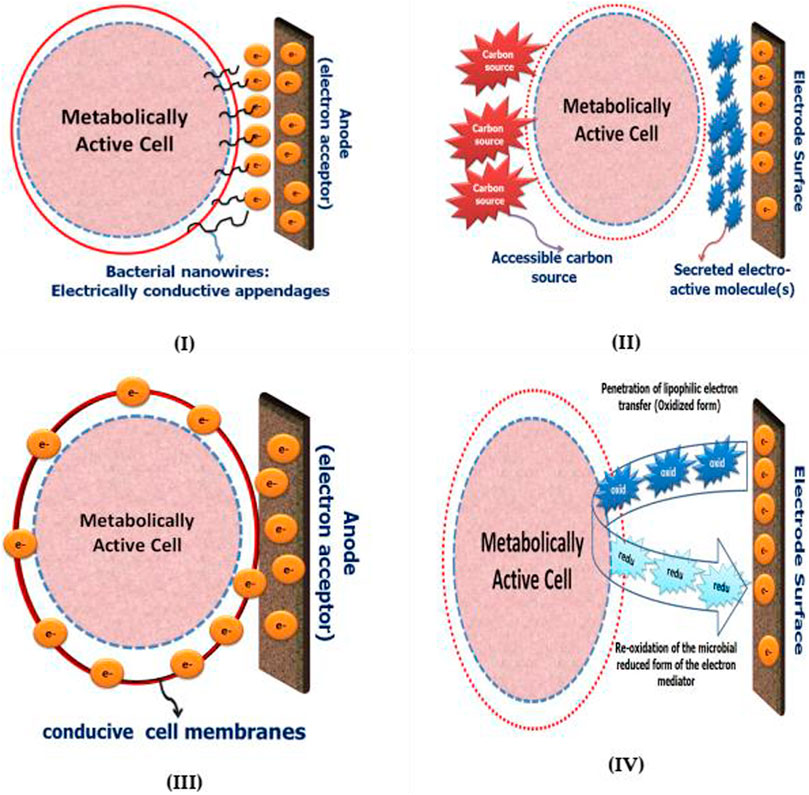
FIGURE 6. Extracellular electron transport in microbial electrochemical systems is depicted. The creation of a conductive layer at the microbial cell wall or the growth of nanowires (I) permits direct microbial electro transfer (DET) (III). The naturally released mediators (II) or chemically used electron redox-shuttles were used in the mediated transfer of electrons (MET) (IV) (Hassan et al., 2021).
BESs have been hailed as a ground-breaking method in pollutant management that is both sustainable and cost-effective (Srikanth et al., 2018). MFCs are one sort of technology that has recently demonstrated renewable energy positive-treated wastewater at a pilot scale. Despite these accomplishments, nothing is known about whether all wastewaters contain significant amounts of energy and, if so, whether MFCs can collect enough energy to counteract electrical power requirements in the wastewater treatment process (Stoll et al., 2018). Hence, to eliminate such limitations, especially wastewater from organo-chlorinated aromatic compounds (Khan N. et al., 2018), chicken processing plants (Gomes et al., 2018), and heavy metal (Zhang et al., 2018a), integration treatment by MFC-coupled wetland construction is a recent and an attractive strategy to treat wastewater (e.g., nitrobenzene-containing one) and generate electricity simultaneously. This inherent relationship between substrate degradation and electricity generation was evaluated by the electronic characteristics of the fabricated electrode materials (Xie et al., 2018). Hence, bioanodes, which are highly rich in conducting biofilms, enhance the degradation of complex wastes. As a result, these electrodes are highly efficient in treating waste water.
2.1.3 Metal Recovery
Heavy metals have emerged as a major inorganic contaminant and hazard to the environment and the human health in recent decades. Only by removing them from metal-laden wastewater before dumping them into aquatic streams can the environment and human health be protected (Saini et al., 2018). Most heavy metals must be considered as highly valued recyclable resources due to their rapid industrialization and long-term sustainability. Advanced oxidation processes, such as photocatalytic degradation, have been evaluated for the effective removal of metal ions for this purpose. In a revolutionary photoelectrochemical cell (PEC), the feasibility of recovering heavy metals from wastewater (e.g., acid mining and electroplating) while also producing electricity is common. However, as BESs point out, its restoration and electricity output are insufficient (Wang D. et al., 2017). To address this issue, a new method combining PECs and MFCs was designed and utilized to effectively remove refractory organic contaminants (such as phenol and aniline) from wastewater while recovering energy for in situ use (Zhang et al., 2019). For example, Pophali et al. (2021) reported reducing Cr(VI) in wastewater by impregnating activated carbon fiber (ACF) photoanodes and photocathodes with cerium oxide (CeO2) and cuprous oxide (Cu2O) nanoparticles and then growing carbon nanofibers (CNFs) on the ACF substrate using catalytic chemical vapor deposition (CVD), as shown in Figure 7. As a result, the constructed MFC reduced COD and Cr (VI) by approximately 94 and 97%, respectively, when operated under visible light irradiation, with a current density of 6,918 mA/m2 and a power density of 1107 mW/m2, and the MFC produced a lot of bioelectricity (Pophali et al., 2021).
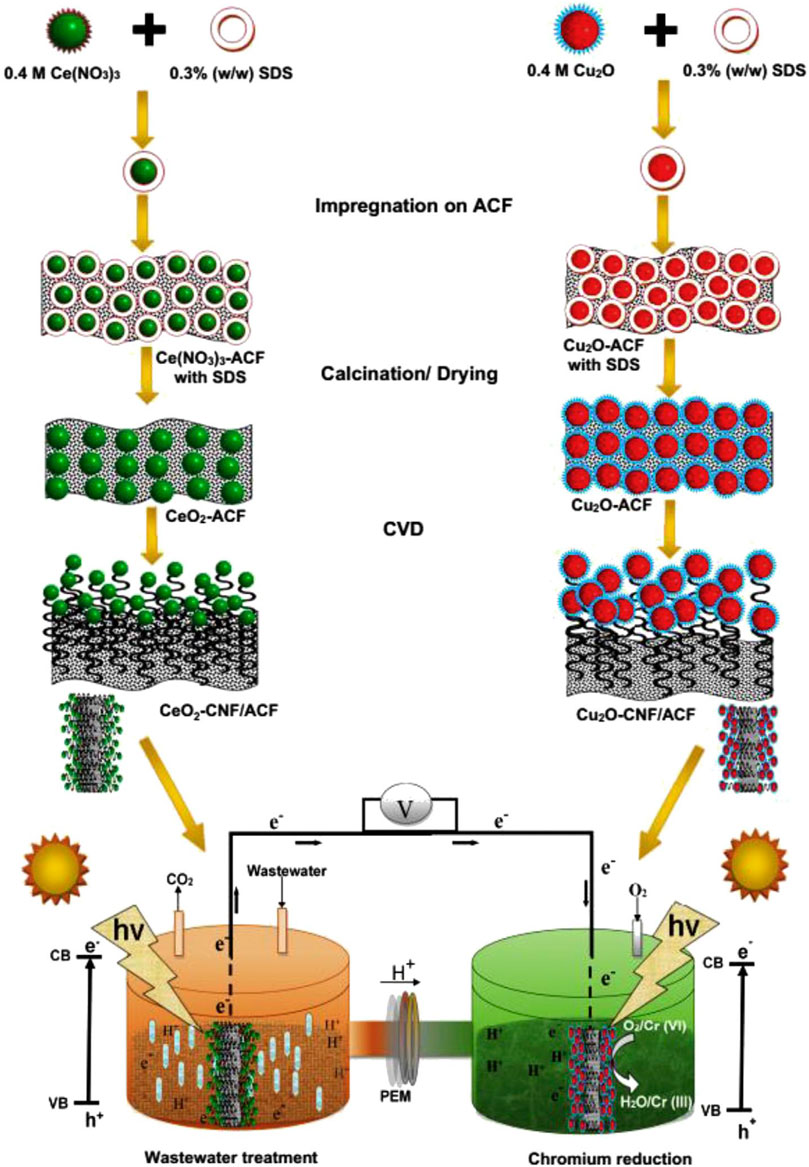
FIGURE 7. The preparation stages for the photoanode anode and cathode, as well as their application in MFC, are shown schematically (Pophali et al., 2021). SDS, sodium dodecyl sulfate.
MFCs also play an important role in the recovery of valuable metals such as silver from wastewater in the form of nanoflakes, which have anti-biofouling properties in a variety of industrial and environmental processes. Maximum power and current densities of 3006 mW/m3 and 34,100 mA/m3, respectively, were found during this recovery, as shown in Figure 8 (Ali et al., 2019). As a result, MFCs are regarded as a revolutionary self-powered wastewater electrolyzer for heavy metal electrocoagulation (Gajda et al., 2017). Hence, MFCs are part of a new technology for producing green energy and reducing the effects of pollutants on the environment (Wang Z. et al., 2017). Cu2+ reduction to copper at the cathode with the anode improved by the rGO/polypyrrole electrode with a COD removal efficiency of 94.5% is also achieved (Rikame et al., 2018).
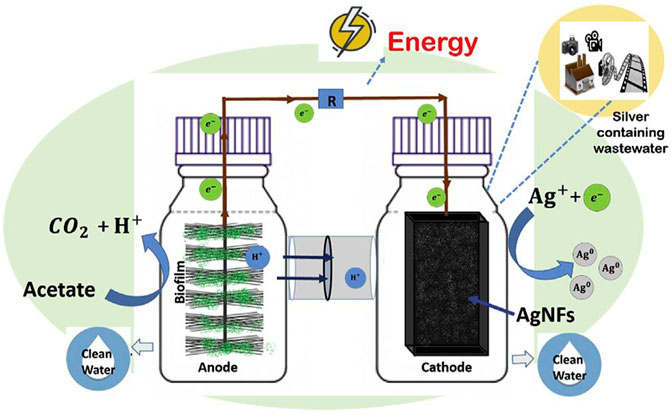
FIGURE 8. The MFC operation principle and components are depicted in a schematic diagram (Ali et al., 2019).
Removal of inorganic heavy metal contaminants such as arsenic (Hernández-Flores et al., 2018), lead (Saini et al., 2018), excessive selenium (He et al., 2018), Cd, Cu, and Cr from various industries provides a very sustainable solution for better living due to their toxicity, harmfulness, and carcinogenicity to human beings (Ahmad and Mirza, 2017). On the other hand, the effect of a new nanocomposite material beyond heavy metal contaminants is a means for waste water treatment from organic dye contaminants; due to this, nanocomposites are considered as key and common to trigger catalytic performance (Arshad et al., 2017). In the subject of environmental engineering, reduction and recovery of precious metals are research hotspots. The transformation and distribution of platinum in MFCs provided a possible strategy to retrieve platinum (Pt) from wastewater with less than 16.88 mg/L platinum by charring biofilms in MFCs and generating the Pt/C catalyst in this field as a support (Liu et al., 2019).
2.2 Bioremediation
Specific combinatorial biobased remediation, including bioremediation, and other approaches are highly effective for sustainable power generation from waste and reduction of heavy metal loads (ROY et al., 2018). Several possible enzymes have been found from various microorganisms that degrade complex chemicals by biodegradation, biostimulation, or bioaugmentation processes, according to recent advances in the environmental remediation of environmental contaminants and their environmental effects (Gaur et al., 2018). As a result, significant progress has been achieved in optimizing MFC performance for both renewable bioenergy generation and environmental remediation. As a result, a concentrated effort has been made to improve MFC performance by improving the reactor structure and electrode architecture, the addition of redox-active electron-donating mediators, biofilm acclimation, feed nutrient correction, and other parameters (Li M. et al., 2018). Significant effort has been taken for the remediation of organically polluted soil, leading to the development of numerous remediation technologies, including physical, bioremediation, and chemical remediation (Zhang H. et al., 2017). Organic-metal co-contamination is also evolving as a major environmental concern worldwide. Such contamination is more difficult to treat than individual contamination because of its great difference in chemical courses and the remediation mechanism of organic and metal pollutants (Chen F. et al., 2017).
The use of heterogeneous catalysts to promote the efficiency (Yuan et al., 2016; Khater et al., 2022) and sustainability of reactions using water as a solvent and the use of solid support (metal nanomaterials and bimetallic and polymetallic nanoparticles; Nascimento et al., 2018) have shown increased stability, reactivity, selectivity, and reusability. An electron-rich polypyrrole hybrid with mercaptoacetic acid (PPy-MAA) composite, which is known to be a very efficient adsorbent in Ag+ removal from an aqueous environment in the cleanup of metal-contaminated water sources, was used for this purpose. In the absence of an additional reducing agent, in situ reduction of Ag+ cations to Ag0 nanoparticles (NPs) was achieved, as well as the silver nanocomposites (AgNCs), which were studied using several optical and electron spectroscopic techniques (Giri et al., 2017). Catalytic reduction of organic pollutants was also achieved using a biosynthesized Ag/C/Fe3O4 nanocomposite made from red water and Caesalpinia gilliesii flower extract (Rostami-Vartooni et al., 2018). In addition to silver nanomaterials, biogenic gold nanoparticles can also modify the anode electrode in the presence of the microbial community for enhanced MFC performance (Wu et al., 2018b). However, both silver and gold are too expensive. Hence, they are not recommended for use as a common electrode for MFC devices at the larger scale. Therefore, this review highlights and discusses the behavior and the way of synthesis of some inexpensive nanocomposite electrode materials.
Nanomaterial-based technologies are gaining traction as a viable approach for removing toxins and completing the environmental cleanup (Wang D. et al., 2018). Synthesizing multifunctional, stable, and regeneratable composite material for cleanup is always seen as a long-term solution for contaminant degradation (Farooq et al., 2018). Such technical aspects have environmental advantages over lignocellulose-based carbon materials (Suganya and Senthil Kumar, 2018). Conventional waste treatment from acid black liquor using the electro-fenton process (Buftia et al., 2018) and an alternative sorbent (activated carbon from kraft lignin) from anthracite (ACCOAL) or coconut (ACBIO) was evaluated using black liquor in the paper pulp industrial process for in situ contaminated sediment remediation (Gustafsson et al., 2017). However, the remediation is not much effective using the sorption method in the presence of active carbon materials. Hence, in this review, we briefly visualize that the listed metal–polymer combinations differ from carbon and its composites for their application.
3 Metal Oxide-Conducting Polymer Nanocomposites
Metal oxide-based electrodes alone in bioelectrochemical conversion device systems as electrodes/anodes are not employed commonly due to their low electrical output (Eyiuche et al., 2017). Analyzing polymers’ fast and reversible redox cycling feature allows them to reversibly transfer electrons and protons, improving the overall electrode functionality (Dessie and Admassie, 2013). As a result, they have a wide range of applications, including those as supercapacitors, mediators for biological processes, and catalysts for electrochemical reactions (Wang and Feng, 2017). Carbon-based polymer nanocomposites (Jafary et al., 2018; Plekhanova et al., 2018) and other semiconductor materials are widely used in environmental remediation applications due to their prominent catalytic applications (Praveen et al., 2018). Therefore, in this review, the role of PPy in iron oxide and manganese oxide as nanocomposites and their scientific relevance will be discussed.
3.1 Iron Oxide/Polypyrrole
One of the most significant obstacles in MFC technology for full-scale application is power output limitation (Gajda et al., 2018). Colloidal magnetic nanocomposite-based iron oxide (Fe3O4, γ-Fe2O3) (Natarov et al., 2018) and its composites (Fe2O3-in-CNT) (Guo et al., 2021) have attracted considerable attention due to a wide range of their applications. Biofilm formation and enrichment of exoelectrogenic bacteria are influenced by iron and iron-doped metal oxide nanoparticle coatings in the anode to boost current production of microbial electrochemical cells. This type of coating has high hydrophilicity and a unique electric characteristic (Muthukumar et al., 2019). For example, bacteria isolated from the soil of rice paddy fields can generate renewable energy (electricity) due to their exoelectrogenic nature (Fakhirruddin et al., 2018). The reaction of microbial populations in anode biofilms to increase concentrations of ferrous iron was more sensitive, according to principal component analysis.
The bulk of the anode biofilm communities of MFCs belongs to Geobacter, which was not the case for the cathode biofilm populations (Liu et al., 2017). A liquid-catalyzed fuel cell is a redox flow fuel cell that converts carbohydrates straight to power. Ferric chloride (FeCl3) was employed as the major catalyst to boost efficiency (Xu et al., 2017).
Anode modification using Fe3O4–PPy composite-modified anodes is most attractive and cost-effective to increase MFC performance. Fe3O4–PPy composite-modified carbon felt anodes, according to the paper, may effectively improve the power production capacity and sewage treatment efficiency of MFCs. Compared to the unmodified anode, the steady-state current density and chemical oxygen demand removal rate were increased by 59.5 and 95.3%, respectively (Fan and Xi, 2021). When this composite was transformed with the addition of MnO2, however, the surface of the anode was altered, resulting in kinetic activities. Because of their large surface area and abundance of electron-donating functional groups and heteroatoms, they have a high surface area. As a result, the PPy-encapsulated MnO2, Fe2O3, and MnO2–Fe2O3 nanocomposite materials act as promising MFC anode electrodes with good electrochemical operations and power densities, according to the findings (Prakash et al., 2020).
In general, immobilization of metal or metal oxide catalysts on the surface of a pure polymer or a functionalized polymer could result in conducting polymer-based nanohybrids (CPNHs) with better catalytic performance and durability. Metal oxides have a lot of surface area and/or porous architectures; hence, they have a lot of synergistic effects with conducting polymers. As a result, CPNHs can provide a stable, environmentally acceptable bio/electro-catalyst with improved catalytic activity and electron-transfer rate. Therefore, blending or direct deposition between CPNHs and metal or metal oxide nanomaterials as anode/cathode and membrane applications in fuel cells (either in direct alcohol fuel cells or microbials) is common today, as shown in Figure 9. The detailed understanding of the electrode materials’ features, changes, and performances is thoroughly reviewed (Ghosh et al., 2020).
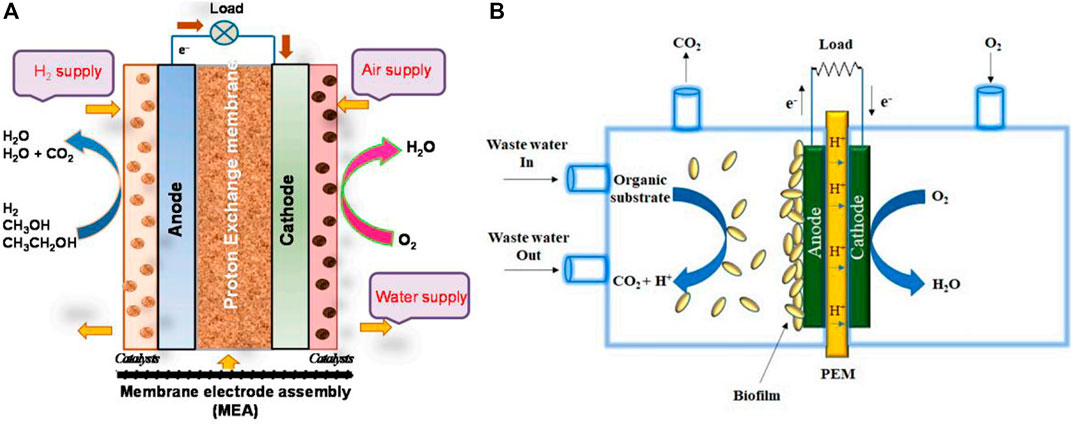
FIGURE 9. The working principle of (A) direct alcohol fuel cells and (B) microbial fuel cells is depicted schematically (Ghosh et al., 2020).
3.2 Manganese Oxide/Polypyrrole
It is vital to use catalysts on the cathode in order to improve MFC performance in real wastewater. Potential electrochemical catalysts for MFCs have to be widely available and should be low-cost materials. Manganese dioxide (MnO2) was also thought to be one of the most effective materials for MFC catalysts because of its inexpensive cost, nontoxic and ecologically benign nature, excellent chemical stability, and catalytic activity (Jiang et al., 2017; Dessie et al., 2020). To enhance electrochemical activities in MFCs, MnO2 electrodeposition on PPy-coated SS presents a promising technology for bioelectricity generation and wastewater treatment (Phonsa et al., 2018). As a result, using in situ chemical polymerization processes to sandwich MnO2 in PPy provides an alternate viable solution for high performance cells, as shown in Figure 10B. This is due to the acquisition of higher electron-storage capacity property of MnO2/PPy composite-modified electrodes, which is clearly shown in Figure 10A. The electron transfer mechanism on MnO2/PPy composite-modified anodes is also shown in Figure 10C. As a result of sandwiching MnO2 in PPy by in situ chemical polymerization, the MnO2/PPy composite-modified anode surface has more microorganisms, according to the results. Manganese ions play an intermediary role in electron transfer and transfers electrons from bacteria to the anode in this case. As a result, the “mosaic” structure causes electrons to reach the anode more efficiently in the redox process due to 1) increased electronic transmission channels between microbes and the anode due to microstructure improvement, 2) manganese ions acting as an electron transfer intermediary to accelerate the electron transfer rate, and 3) the composites’ pseudo-capacitance property, which improves the anode antipolarization performance.
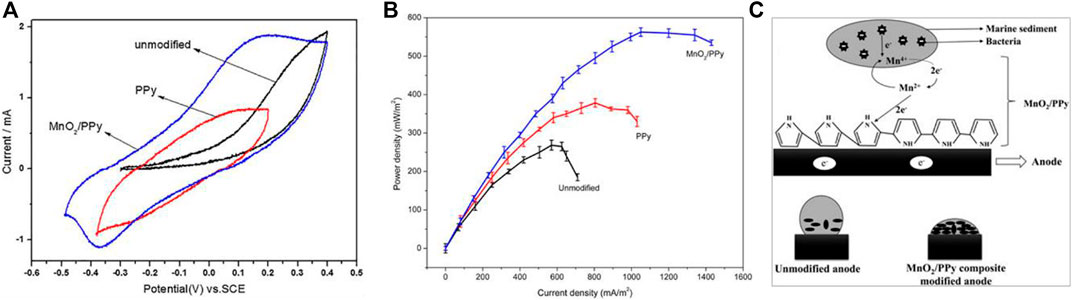
FIGURE 10. (A) Cyclic voltammetry plots, (B) cell power density curves, and (C) electron transfer mechanism of different anodes (Chen et al., 2017b).
4 Future Outlooks and Challenges
Significant advancements have been made on anode modification to increase the performance of MFCs. To succeed in this, various forms of modification techniques and architectural designs are frequently used. However, there are many challenges that need to be addressed in order to modify effective bioelectricity generation in MFC. The type and nature of electrodes, bioreactor design, shape and size, and the types of active electro microorganisms decides performance in MFC applications. However, noble-based nanomaterials are effective in producing electricity even in small device applications of MFCs. However, anodes modified by such nanomaterials are not cost effective in scaling up MFC devices. Hence, to reduce use of such costly nanomaterials, novel, low-cost, biocompatible, and environmentally friendly nanocomposites are used. Although power generation by using nanocomposite-modified anodes yields low power production, factors governing MFC performance will be optimized in the future to advance effective anode materials. Among these factors, electroactive microorganisms/biocatalysts, the type and concentration of fuels (substrates), and pH are critical. In the future, all listed parameters should be optimized to reduce polarization loss, activation losses, concentration losses, and ohmic losses. All the losses will be minimized by fabricating a functional nanocomposite through low-cost metal oxide-conducting polymer integration. As a result, thermodynamically stable, kinetically fast, and bioelectrochemically active nanocomposite-modified anodes can be designed, improving the performance of MFCs with respect to bioelectricity, wastewater treatment, metal recovery, and so forth.
5 Conclusion
Bioelectricity production in microbial fuel cell technology can be achieved in a variety of ways with purposes ranging from energy production to metal recovery (precious noble to heavy metals) and bioremediation. Bioelectricity production from diverse waste discharges via active microorganisms acting on anode electrodes modified with low-cost and suitable nanocomposites can very well operate as a sustainable source of energy, reducing the need for fossil fuels and resulting in green energy. Anaerobic digestion strategies that promote the conversion of various waste sources to bioenergy require an additional treatment procedure and are not cost-effective. Anaerobic digestion of waste products, contaminants, and chemicals in microbial fuel cell systems can be considered a good approach to keep the environment clean while also producing sustainable energy. Generally, promising or renewable energy generation simultaneous with wastewater treatment using MFCs from various waste discharges is intensified and attracts a lot of attention. However, enough power generation has not yet been carried out at the commercial level. Hence, MFCs still need to undergo additional research to scale-up devices in the near future.
Authors Contributions
YD designed the research, wrote the manuscript, and revised the final draft of the manuscript. ST supervised the whole research and reviewed the manuscript.
Conflict of Interest
The authors declare that the research was conducted in the absence of any commercial or financial relationships that could be construed as a potential conflict of interest.
Publisher’s Note
All claims expressed in this article are solely those of the authors and do not necessarily represent those of their affiliated organizations, or those of the publisher, the editors, and the reviewers. Any product that may be evaluated in this article, or claim that may be made by its manufacturer, is not guaranteed or endorsed by the publisher.
Acknowledgments
The authors sincerely acknowledge Adama Science and Technology University for the financial support.
References
Abbas, S. Z., Rafatullah, M., Ismail, N., and Shakoori, F. R. (2018). Electrochemistry and Microbiology of Microbial Fuel Cells Treating Marine Sediments Polluted with Heavy Metals. RSC Adv. 8, 18800–18813. doi:10.1039/C8RA01711E
Abdel-Shafy, H. I., and Mansour, M. S. M. (2018). Solid Waste Issue: Sources, Composition, Disposal, Recycling, and Valorization. Egypt. J. Petroleum 27, 1275–1290. doi:10.1016/j.ejpe.2018.07.003
Ahmad, R., and Mirza, A. (2017). Heavy Metal Remediation by Dextrin-Oxalic acid/Cetyltrimethyl Ammonium Bromide (CTAB) - Montmorillonite (MMT) Nanocomposite. Groundw. Sustain. Dev. 4, 57–65. doi:10.1016/j.gsd.2017.01.001
Ali, J., Sohail, A., Wang, L., Rizwan Haider, M., Mulk, S., and Pan, G. (2018). Electro-microbiology as a Promising Approach towards Renewable Energy and Environmental Sustainability. Energies 11, 1822–1830. doi:10.3390/en11071822
Ali, J., Wang, L., Waseem, H., Sharif, H. M. A., Djellabi, R., Zhang, C., et al. (2019). Bioelectrochemical Recovery of Silver from Wastewater with Sustainable Power Generation and its Reuse for Biofouling Mitigation. J. Clean. Prod. 235, 1425–1437. doi:10.1016/j.jclepro.2019.07.065
Amini, A., Aponte-Morales, V., Wang, M., Dilbeck, M., Lahav, O., Zhang, Q., et al. (2017). Cost-effective Treatment of Swine Wastes through Recovery of Energy and Nutrients. Waste Manag. 69, 508–517. doi:10.1016/j.wasman.2017.08.041
Angelaalincy, M. J., Navanietha Krishnaraj, R., Shakambari, G., Ashokkumar, B., Kathiresan, S., and Varalakshmi, P. (2018). Biofilm Engineering Approaches for Improving the Performance of Microbial Fuel Cells and Bioelectrochemical Systems. Front. Energy Res. 6, 1–12. doi:10.3389/fenrg.2018.00063
Arshad, A., Iqbal, J., Siddiq, M., Ali, M. U., Ali, A., Shabbir, H., et al. (2017). Solar Light Triggered Catalytic Performance of Graphene-CuO Nanocomposite for Waste Water Treatment. Ceram. Int. 43, 10654–10660. doi:10.1016/j.ceramint.2017.03.165
Bakonyi, P., Koók, L., Keller, E., Bélafi-Bakó, K., Rózsenberszki, T., Saratale, G. D., et al. (2018). Development of Bioelectrochemical Systems Using Various Biogas Fermenter Effluents as Inocula and Municipal Waste Liquor as Adapting Substrate. Bioresour. Technol. 259, 75–82. doi:10.1016/j.biortech.2018.03.034
Barelli, L., Bidini, G., Pelosi, D., and Sisani, E. (2021). Enzymatic Biofuel Cells: A Review on Flow Designs. Energies 14, 910. doi:10.3390/en14040910
Bian, B., Wang, C., Hu, M., Yang, Z., Cai, X., Shi, D., et al. (2018). Application of 3D Printed Porous Copper Anode in Microbial Fuel Cells. Front. Energy Res. 6, 1–9. doi:10.3389/fenrg.2018.00050
Buftia, G., Rosales, E., Pazos, M., Lazar, G., and Sanromán, M. A. (2018). Electro-Fenton Process for Implementation of Acid Black Liquor Waste Treatment. Sci. Total Environ. 635, 397–404. doi:10.1016/j.scitotenv.2018.04.139
Cao, X., Halder, A., Tang, Y., Hou, C., Wang, H., Duus, J. Ø., et al. (2018). Engineering Two-Dimensional Layered Nanomaterials for Wearable Biomedical Sensors and Power Devices. Mat. Chem. Front. 2, 1944–1986. doi:10.1039/c8qm00356d
Capodaglio, A. G., Molognoni, D., Dallago, E., Liberale, A., Cella, R., Longoni, P., et al. (2013). Microbial Fuel Cells for Direct Electrical Energy Recovery from Urban Wastewaters. Sci. World J. 2013, 1–8. doi:10.1155/2013/634738
Champigneux, P., Delia, M.-L., and Bergel, A. (2018). Impact of Electrode Micro- and Nano-Scale Topography on the Formation and Performance of Microbial Electrodes. Biosens. Bioelectron. 118, 231–246. doi:10.1016/j.bios.2018.06.059
Chandra, R., Venkata Mohan, S., Roberto, P.-S., Ritmann, B. E., and Cornejo, R. A. S. (2017). “Biophotovoltaics: Conversion of Light Energy to Bioelectricity through Photosynthetic Microbial Fuel Cell Technology,” in Microbial Fuel Cell (Springer), 373–387. doi:10.1007/978-3-319-66793-5_19
Chandrasekhar, K., and Ahn, Y.-H. (2017). Effectiveness of Piggery Waste Treatment Using Microbial Fuel Cells Coupled with Elutriated-Phased Acid Fermentation. Bioresour. Technol. 244, 650–657. doi:10.1016/j.biortech.2017.08.021
Chen, F., Luo, Z., Liu, G., Yang, Y., Zhang, S., and Ma, J. (2017a). Remediation of Electronic Waste Polluted Soil Using a Combination of Persulfate Oxidation and Chemical Washing. J. Environ. Manag. 204, 170–178. doi:10.1016/j.jenvman.2017.08.050
Chen, G.-G., Hu, Y.-J., Peng, F., Bian, J., Li, M.-F., Yao, C.-L., et al. (2018). Fabrication of Strong Nanocomposite Films with Renewable Forestry Waste/montmorillonite/reduction of Graphene Oxide for Fire Retardant. Chem. Eng. J. 337, 436–445. doi:10.1016/j.cej.2017.12.119
Chen, S., and Smith, A. L. (2018). Methane-driven Microbial Fuel Cells Recover Energy and Mitigate Dissolved Methane Emissions from Anaerobic Effluents. Environ. Sci. Water Res. Technol. 4, 67–79. doi:10.1039/c7ew00293a
Chen, W., Liu, Z., Su, G., Fu, Y., Zai, X., Zhou, C., et al. (2017b). Composite-modified Anode by MnO2/polypyrrole in Marine Benthic Microbial Fuel Cells and its Electrochemical Performance. Int. J. Energy Res. 41, 845–853. doi:10.1002/er.3674
Chen, W., Liu, Z., Su, G., Fu, Y., Zai, X., Zhou, C., et al. (2017c). Composite-modified Anode by MnO2/polypyrrole in Marine Benthic Microbial Fuel Cells and its Electrochemical Performance. Int. J. Energy Res. 41, 845–853. doi:10.1002/er.3674
Chen, Z., Lu, X., Yin, R., Luo, Y., Mai, H., Zhang, N., et al. (2018a). Research on Treatment of Wastewater Containing Heavy Metal by Microbial Fuel Cell. IOP Conf. Ser. Earth Environ. Sci. 121, 032022. doi:10.1088/1755-1315/121/3/032022
Chouler, J., Bentley, I., Vaz, F., O’Fee, A., Cameron, P. J., and Di Lorenzo, M. (2017). Exploring the Use of Cost-Effective Membrane Materials for Microbial Fuel Cell Based Sensors. Electrochimica Acta 231, 319–326. doi:10.1016/j.electacta.2017.01.195
Commault, A. S., Laczka, O., Siboni, N., Tamburic, B., Crosswell, J. R., Seymour, J. R., et al. (2017). Electricity and Biomass Production in a Bacteria- Chlorella Based Microbial Fuel Cell Treating Wastewater. J. Power Sources 356, 299–309. doi:10.1016/j.jpowsour.2017.03.097
Dahiya, S., Kumar, A. N., Shanthi Sravan, J., Chatterjee, S., Sarkar, O., and Mohan, S. V. (2018). Food Waste Biorefinery: Sustainable Strategy for Circular Bioeconomy. Bioresour. Technol. 248, 2–12. doi:10.1016/j.biortech.2017.07.176
Dai, Y., Sun, Q., Wang, W., Lu, L., Liu, M., Li, J., et al. (2018). Utilizations of Agricultural Waste as Adsorbent for the Removal of Contaminants: A Review. Chemosphere 211, 235–253. doi:10.1016/j.chemosphere.2018.06.179
De Clercq, D., Wen, Z., Gottfried, O., Schmidt, F., and Fei, F. (2017). A Review of Global Strategies Promoting the Conversion of Food Waste to Bioenergy via Anaerobic Digestion. Renew. Sustain. Energy Rev. 79, 204–221. doi:10.1016/j.rser.2017.05.047
Dessì, P., Porca, E., Haavisto, J., Lakaniemi, A.-M., Collins, G., and Lens, P. N. L. (2018). Composition and Role of the Attached and Planktonic Microbial Communities in Mesophilic and Thermophilic Xylose-Fed Microbial Fuel Cells. RSC Adv. 8, 3069–3080. doi:10.1039/c7ra12316g
Dessie, Y., and Admassie, S. (2013). Electrochemical Study of Conducting Polymer/lignin Composites. Orient. J. Chem. 29, 1359–1369. doi:10.13005/ojc/290411
Dessie, Y., Tadesse, S., and Adimasu, Y. (2022). Improving the Performance of Graphite Anode in a Microbial Fuel Cell via PANI Encapsulated α-MnO2 Composite Modification for Efficient Power Generation and Methyl Red Removal. Chem. Eng. J. Adv. 10, 100283. doi:10.1016/j.ceja.2022.100283
Dessie, Y., Tadesse, S., Eswaramoorthy, R., and Abdisa, E. (2021b). Bimetallic Mn-Ni Oxide Nanoparticles: Green Synthesis, Optimization and its Low-Cost Anode Modifier Catalyst in Microbial Fuel Cell. Nano-Structures Nano-Objects 25, 100663. doi:10.1016/j.nanoso.2020.100663
Dessie, Y., Tadesse, S., Eswaramoorthy, R., and Adimasu, Y. (2021c). Biosynthesized α-MnO2-based Polyaniline Binary Composite as Efficient Bioanode Catalyst for High-Performance Microbial Fuel Cell. All Life 14, 541–568. doi:10.1080/26895293.2021.1934123
Dessie, Y., Tadesse, S., and Eswaramoorthy, R. (2020). Physicochemical Parameter Influences and Their Optimization on the Biosynthesis of MnO2 Nanoparticles Using Vernonia Amygdalina Leaf Extract. Arabian J. Chem. 13, 6472–6492. doi:10.1016/j.arabjc.2020.06.006
Dessie, Y., Tadesse, S., and Eswaramoorthy, R. (2021a). Surface Roughness and Electrochemical Performance Properties of Biosynthesized α-MnO2/NiO-Based Polyaniline Ternary Composites as Efficient Catalysts in Microbial Fuel Cells. J. Nanomater. 2021, 1–21. doi:10.1155/2021/7475902
Dessie, Y., and Tadesse, S. (2022). Optimization of Polyvinyl Alcohol Binder on PANI Coated Pencil Graphite Electrode in Doubled Chamber Microbial Fuel Cell for Glucose Biosensor. Sens. Bio-Sensing Res. 36, 100484. doi:10.1016/j.sbsr.2022.100484
Deval, A. S., Parikh, H. A., Kadier, A., Chandrasekhar, K., Bhagwat, A. M., and Dikshit, A. K. (2017). Sequential Microbial Activities Mediated Bioelectricity Production from Distillery Wastewater Using Bio-Electrochemical System with Simultaneous Waste Remediation. Int. J. Hydrogen Energy 42, 1130–1141. doi:10.1016/j.ijhydene.2016.11.114
Dhiman, S. S., Shrestha, N., David, A., Basotra, N., Johnson, G. R., Chadha, B. S., et al. (2018). Producing Methane, Methanol and Electricity from Organic Waste of Fermentation Reaction Using Novel Microbes. Bioresour. Technol. 258, 270–278. doi:10.1016/j.biortech.2018.02.128
Di Palma, L., Bavasso, I., Sarasini, F., Tirillò, J., Puglia, D., Dominici, F., et al. (2018). Synthesis, Characterization and Performance Evaluation of Fe3O4/PES Nano Composite Membranes for Microbial Fuel Cell. Eur. Polym. J. 99, 222–229. doi:10.1016/j.eurpolymj.2017.12.037
Dumitru, A., Vulpe, S., Radu, A., and Antohe, S. (2018). Influence of Nitrogen Environment on the Performance of Conducting polymers/CNTs Nanocomposites Modified Anodes for Microbial Fuel Cells (MFCs). Rom. J. Phys. 63, 1–11.
Eyiuche, N. J., Asakawa, S., Yamashita, T., Ikeguchi, A., Kitamura, Y., and Yokoyama, H. (2017). Community Analysis of Biofilms on Flame-Oxidized Stainless Steel Anodes in Microbial Fuel Cells Fed with Different Substrates. BMC Microbiol. 17, 1–8. doi:10.1186/s12866-017-1053-z
Fakhirruddin, F., Amid, A., Wan Salim, W. W. A., and Azmi, A. S. (2018). Electricity Generation in Microbial Fuel Cell (MFC) by Bacterium Isolated from Rice Paddy Field Soil. E3S Web Conf. 34, 02036. doi:10.1051/e3sconf/20183402036
Fan, L., and Xi, Y. (2021). Effect of Polypyrrole-Fe3O4 Composite Modified Anode and its Electrodeposition Time on the Performance of Microbial Fuel Cells. Energies 14, 2461. doi:10.3390/en14092461
Farooq, U., Danish, M., Lu, S., Naqvi, M., Qiu, Z., and Sui, Q. (2018). A Step Forward towards Synthesizing a Stable and Regeneratable Nanocomposite for Remediation of Trichloroethene. Chem. Eng. J. 347, 660–668. doi:10.1016/j.cej.2018.04.120
Gajda, I., Greenman, J., Santoro, C., Serov, A., Melhuish, C., Atanassov, P., et al. (2018). Improved Power and Long Term Performance of Microbial Fuel Cell with Fe-N-C Catalyst in Air-Breathing Cathode. Energy 144, 1073–1079. doi:10.1016/j.energy.2017.11.135
Gajda, I., Obata, O., Jose Salar-Garcia, M., Greenman, J., and Ieropoulos, I. A. (2020). Long-term Bio-Power of Ceramic Microbial Fuel Cells in Individual and Stacked Configurations. Bioelectrochemistry 133, 107459. doi:10.1016/j.bioelechem.2020.107459
Gajda, I., Stinchcombe, A., Greenman, J., Melhuish, C., and Ieropoulos, I. (2017). Microbial Fuel Cell - A Novel Self-Powered Wastewater Electrolyser for Electrocoagulation of Heavy Metals. Int. J. Hydrogen Energy 42, 1813–1819. doi:10.1016/j.ijhydene.2016.06.161
Gaur, N., Narasimhulu, K., and Y, P. (2018). Recent Advances in the Bio-Remediation of Persistent Organic Pollutants and its Effect on Environment. J. Clean. Prod. 198, 1602–1631. doi:10.1016/j.jclepro.2018.07.076
Gazali, T. A., and Moqsud, M. A. (2017). The Effectiveness of Animal Dungs and Leaf Mold for Bioelectricity Generation Using Microbial Fuel Cell with Soils. Jsbs 07, 165–181. doi:10.4236/jsbs.2017.74012
Ghazali, N. F., Mahmood, N. A. B. N., Ibrahim, K. A., Muhammad, S. A. F. S., and Amalina, N. S. (2017). Electricity Generation from Palm Oil Tree Empty Fruit Bunch (EFB) Using Dual Chamber Microbial Fuel Cell (MFC). IOP Conf. Ser. Mat. Sci. Eng. 206, 012025. doi:10.1088/1757-899X/206/1/012025
Ghosh, S., Das, S., and Mosquera, M. E. G. (2020). Conducting Polymer-Based Nanohybrids for Fuel Cell Application. Polymers 12, 2993. doi:10.3390/polym12122993
Giri, S., Das, R., van der Westhuyzen, C., and Maity, A. (2017). An Efficient Selective Reduction of Nitroarenes Catalyzed by Reusable Silver-Adsorbed Waste Nanocomposite. Appl. Catal. B Environ. 209, 669–678. doi:10.1016/j.apcatb.2017.03.033
Gomes, A. J. G., Atambo, D. O., Das, K. K., Cocke, D. L., and Das, K. P. (2018). Electrochemical Remediation of Chicken Processing Plant Wastewater. J. Environ. Chem. Eng. 6, 6028–6036. doi:10.1016/j.jece.2018.09.039
González-Gamboa, N., Domínguez-Benetton, X., Pacheco-Catalán, D., Kumar-Kamaraj, S., Valdés-Lozano, D., Domínguez-Maldonado, J., et al. (2018). Effect of Operating Parameters on the Performance Evaluation of Benthic Microbial Fuel Cells Using Sediments from the Bay of Campeche, Mexico. Sustainability 10, 2446. doi:10.3390/su10072446
Guo, D., Liu, Y., Ji, H., Wang, C.-C., Chen, B., Shen, C., et al. (2021). Silicate-Enhanced Heterogeneous Flow-Through Electro-Fenton System Using Iron Oxides under Nanoconfinement. Environ. Sci. Technol. 55, 4045–4053. doi:10.1021/acs.est.1c00349
Gustafsson, Å., Hale, S., Cornelissen, G., Sjöholm, E., and Gunnarsson, J. S. (2017). Activated Carbon from Kraft Lignin: A Sorbent for In Situ Remediation of Contaminated Sediments. Environ. Technol. Innovation 7, 160–168. doi:10.1016/j.eti.2016.11.001
Han, Z., Liu, Y., Zhong, M., Shi, G., Li, Q., Zeng, D., et al. (2018a). Influencing Factors of Domestic Waste Characteristics in Rural Areas of Developing Countries. Waste Manag. 72, 45–54. doi:10.1016/j.wasman.2017.11.039
Han, Z., Park, A., and Su, W. W. (2018b). Valorization of Papaya Fruit Waste through Low-Cost Fractionation and Microbial Conversion of Both Juice and Seed Lipids. RSC Adv. 8, 27963–27972. doi:10.1039/c8ra05539d
Hassan, R. Y. A., Febbraio, F., and Andreescu, S. (2021). Microbial Electrochemical Systems: Principles, Construction and Biosensing Applications. Sensors 21, 1279. doi:10.3390/s21041279
He, Y., Xiang, Y., Zhou, Y., Yang, Y., Zhang, J., Huang, H., et al. (2018). Selenium Contamination, Consequences and Remediation Techniques in Water and Soils: A Review. Environ. Res. 164, 288–301. doi:10.1016/j.envres.2018.02.037
Hernández-Flores, H., Pariona, N., Herrera-Trejo, M., Hdz-García, H. M., and Mtz-Enriquez, A. I. (2018). Concrete/maghemite Nanocomposites as Novel Adsorbents for Arsenic Removal. J. Mol. Struct. 1171, 9–16. doi:10.1016/j.molstruc.2018.05.078
Hoa, L., Vestergaard, M. d., and Tamiya, E. (2017). Carbon-based Nanomaterials in Biomass-Based Fuel-Fed Fuel Cells. Sensors 17, 2587. doi:10.3390/s17112587
Hong, J., Zhan, S., Yu, Z., Hong, J., and Qi, C. (2018). Life-cycle Environmental and Economic Assessment of Medical Waste Treatment. J. Clean. Prod. 174, 65–73. doi:10.1016/j.jclepro.2017.10.206
Huarachi-Olivera, R., Dueñas-Gonza, A., Yapo-Pari, U., Vega, P., Romero-Ugarte, M., Tapia, J., et al. (2018). Bioelectrogenesis with Microbial Fuel Cells (MFCs) Using the Microalga Chlorella Vulgaris and Bacterial Communities. Electron. J. Biotechnol. 31, 34–43. doi:10.1016/j.ejbt.2017.10.013
Inamuddin, ., Mohammad, A., and Asiri, A. M. (2017). Organic-Inorganic Composite Polymer Electrolyte Membranes. Cham, Switzerland: Springer. doi:10.1007/978-3-319-52739-0
Jafary, T., Ghasemi, M., Alam, J., Aljlil, S. A., and Yusup, S. (2018). “Carbon-Based Polymer Nanocomposites as Electrodes for Microbial Fuel Cells,” in Carbon-Based Polymer Nanocomposites for Environmental and Energy Applications (Amsterdam and Cambridge: Microbial Desalination Cell for Brackish Water Desalination and Wastewater Treatment), 361–390. doi:10.1016/b978-0-12-813574-7.00015-0
Jiang, B., Muddemann, T., Kunz, U., Bormann, H., Niedermeiser, M., Haupt, D., et al. (2017). Evaluation of Microbial Fuel Cells with Graphite Plus MnO2and MoS2Paints as Oxygen Reduction Cathode Catalyst. J. Electrochem. Soc. 164, H3083–H3090. doi:10.1149/2.0131703jes
Jiang, Q., Xing, D., Zhang, L., Sun, R., Zhang, J., Zhong, Y., et al. (2018a). Interaction of Bacteria and Archaea in a Microbial Fuel Cell with ITO Anode. RSC Adv. 8, 28487–28495. doi:10.1039/c8ra01207e
Jiang, S., Hagesteijn, K. F. L., Ni, J., and Ladewig, B. P. (2018b). A Scientometric Study of the Research on Ion Exchange Membranes. RSC Adv. 8, 24036–24048. doi:10.1039/c8ra04686g
Jm, K., Dn, M., Jm, M., and Fb, M. (2018). Utilization of Rumen Fluid in Production of Bio-Energy from Market Waste Using Microbial Fuel Cells Technology. Jabb 5, 227–231. doi:10.15406/jabb.2018.05.00142
Kaushik, S., Sarma, M. K., and Goswami, P. (2017). FRET-guided Surging of Cyanobacterial Photosystems Improves and Stabilizes Current in Photosynthetic Microbial Fuel Cell. J. Mat. Chem. A 5, 7885–7895. doi:10.1039/c7ta01137g
Khan, M. E., Khan, M. M., Min, B.-K., and Cho, M. H. (2018a). Microbial Fuel Cell Assisted Band Gap Narrowed TiO2 for Visible Light-Induced Photocatalytic Activities and Power Generation. Sci. Rep. 8, 1–12. doi:10.1038/s41598-018-19617-2
Khan, N., Khan, M. D., Nizami, A.-S., Rehan, M., Shaida, A., Ahmad, A., et al. (2018b). Energy Generation through Bioelectrochemical Degradation of Pentachlorophenol in Microbial Fuel Cell. RSC Adv. 8, 20726–20736. doi:10.1039/c8ra01643g
Khater, D. Z., Amin, R. S., Zhran, M. O., Abd El-Aziz, Z. K., Mahmoud, M., Hassan, H. M., et al. (2022). The Enhancement of Microbial Fuel Cell Performance by Anodic Bacterial Community Adaptation and Cathodic Mixed Nickel-Copper Oxides on a Graphene Electrocatalyst. J. Genet. Eng. Biotechnol. 20, 12. doi:10.1186/s43141-021-00292-2
Kibler, K. M., Reinhart, D., Hawkins, C., Motlagh, A. M., and Wright, J. (2018). Food Waste and the Food-Energy-Water Nexus: A Review of Food Waste Management Alternatives. Waste Manag. 74, 52–62. doi:10.1016/j.wasman.2018.01.014
Kodali, M., Herrera, S., Kabir, S., Serov, A., Santoro, C., Ieropoulos, I., et al. (2018). Enhancement of Microbial Fuel Cell Performance by Introducing a Nano-Composite Cathode Catalyst. Electrochimica Acta 265, 56–64. doi:10.1016/j.electacta.2018.01.118
Kodali, M., Santoro, C., Serov, A., Kabir, S., Artyushkova, K., Matanovic, I., et al. (2017). Air Breathing Cathodes for Microbial Fuel Cell Using Mn-, Fe-, Co- and Ni-Containing Platinum Group Metal-free Catalysts. Electrochimica Acta 231, 115–124. doi:10.1016/j.electacta.2017.02.033
Krieg, T., Enzmann, F., Sell, D., Schrader, J., and Holtmann, D. (2017). Simulation of the Current Generation of a Microbial Fuel Cell in a Laboratory Wastewater Treatment Plant. Appl. Energy 195, 942–949. doi:10.1016/j.apenergy.2017.03.101
Li, F., Li, Y.-X., Cao, Y.-X., Wang, L., Liu, C.-G., Shi, L., et al. (2018a). Modular Engineering to Increase Intracellular NAD(H/+) Promotes Rate of Extracellular Electron Transfer of Shewanella Oneidensis. Nat. Commun. 9, 1–13. doi:10.1038/s41467-018-05995-8
Li, M., Zhou, M., Tian, X., Tan, C., McDaniel, C. T., Hassett, D. J., et al. (2018b). Microbial Fuel Cell (MFC) Power Performance Improvement through Enhanced Microbial Electrogenicity. Biotechnol. Adv. 36, 1316–1327. doi:10.1016/j.biotechadv.2018.04.010
Li, X., Li, Y., Zhang, X., Zhao, X., Sun, Y., Weng, L., et al. (2019). Long-term Effect of Biochar Amendment on the Biodegradation of Petroleum Hydrocarbons in Soil Microbial Fuel Cells. Sci. Total Environ. 651, 796–806. doi:10.1016/j.scitotenv.2018.09.098
Liu, Q., Liu, B., Li, W., Zhao, X., Zuo, W., and Xing, D. (2017). Impact of Ferrous Iron on Microbial Community of the Biofilm in Microbial Fuel Cells. Front. Microbiol. 8, 1–9. doi:10.3389/fmicb.2017.00920
Liu, Y., Liu, F., Ding, N., Hu, X., Shen, C., Li, F., et al. (2020). Recent Advances on Electroactive CNT-Based Membranes for Environmental Applications: The Perfect Match of Electrochemistry and Membrane Separation. Chin. Chem. Lett. 31, 2539–2548. doi:10.1016/j.cclet.2020.03.011
Liu, Y., Song, P., Gai, R., Yan, C., Jiao, Y., Yin, D., et al. (2019). Recovering Platinum from Wastewater by Charring Biofilm of Microbial Fuel Cells (MFCs). J. Saudi Chem. Soc. 23, 338–345. doi:10.1016/j.jscs.2018.08.003
López Zavala, M., Torres Delenne, P., and González Peña, O. (2018). Improvement of Wastewater Treatment Performance and Power Generation in Microbial Fuel Cells by Enhancing Hydrolysis and Acidogenesis, and by Reducing Internal Losses. Energies 11, 2309. doi:10.3390/en11092309
Lueking, A. D., and Cole, M. W. (2017). Energy and Mass Balances Related to Climate Change and Remediation. Sci. Total Environ. 590-591, 416–429. doi:10.1016/j.scitotenv.2016.12.101
Luo, S., Berges, J. A., He, Z., and Young, E. B. (2017). Algal-microbial Community Collaboration for Energy Recovery and Nutrient Remediation from Wastewater in Integrated Photobioelectrochemical Systems. Algal Res. 24, 527–539. doi:10.1016/j.algal.2016.10.006
Mahrokh, L., Ghourchian, H., Nealson, K. H., and Mahrokh, M. (2017). An Efficient Microbial Fuel Cell Using a CNT-RTIL Based Nanocomposite. J. Mat. Chem. A 5, 7979–7991. doi:10.1039/c6ta09766a
Matsakas, L., Gao, Q., Jansson, S., Rova, U., and Christakopoulos, P. (2017). Green Conversion of Municipal Solid Wastes into Fuels and Chemicals. Electron. J. Biotechnol. 26, 69–83. doi:10.1016/j.ejbt.2017.01.004
Mounia, A., and Renouvelables, E. (2016). Microbial Fuel Cell- Plant. Available at: https://www.researchgate.net/publication/276275684_Microbial_Fuel_Cell-_Plant.doi:10.13140/RG.2.1.2208.6568
Mulijani, S., Syahbirin, G., and Wulanawati, A. (2018). "A Novel Approach of Membrane Electrode Assembly for Application in Microbial Fuel Cell ". Rjc 11, 151–154. doi:10.7324/RJC.2018.1111930
Muthukumar, H., Mohammed, S. N., Chandrasekaran, N., Sekar, A. D., Pugazhendhi, A., and Matheswaran, M. (2019). Effect of Iron Doped Zinc Oxide Nanoparticles Coating in the Anode on Current Generation in Microbial Electrochemical Cells. Int. J. Hydrogen Energy 44, 2407–2416. doi:10.1016/j.ijhydene.2018.06.046
Nascimento, M. A., Castro Cruz, J., Dos Reis, M. F., De Carvalho Damasceno, O. I., Lázaro Reis, E., Reis, C., et al. (2018). Synthesis of Polymetallic Nanoparticles from Printed Circuit Board Waste and Application in Textile Dye Remediation. J. Environ. Chem. Eng. 6, 5580–5586. doi:10.1016/j.jece.2018.08.056
Natarov, V., Kotsikau, D., Survilo, V., Gilep, A., and Pankov, V. (2018). Facile Bulk Preparation and Structural Characterization of Agglomerated γ-Fe2O3/SiO2 Nanocomposite Particles for Nucleic Acids Isolation and Analysis. Mater. Chem. Phys. 219, 109–119. doi:10.1016/j.matchemphys.2018.08.011
Ng, I.-S., Hsueh, C.-C., and Chen, B.-Y. (2017). Electron Transport Phenomena of Electroactive Bacteria in Microbial Fuel Cells: a Review of Proteus Hauseri. Bioresour. Bioprocess. 4. doi:10.1186/s40643-017-0183-3
Nitisoravut, R., and Regmi, R. (2017). Plant Microbial Fuel Cells: A Promising Biosystems Engineering. Renew. Sustain. Energy Rev. 76, 81–89. doi:10.1016/j.rser.2017.03.064
On, A. R., Effect, T. H. E., Conductivity, O. F., The, O. N., Of, P., and Fuel, M. (2017). World Journal of Engineering Research and Technology. WJERT 3, 44–55.
Oyiwona, G. E., Ogbonna, J. C., Anyanwu, C. U., and Okabe, S. (2018). Electricity Generation Potential of Poultry Droppings Wastewater in Microbial Fuel Cell Using Rice Husk Charcoal Electrodes. Bioresour. Bioprocess. 5. doi:10.1186/s40643-018-0201-0
Peo-nio-liclo, P. E. (2010). Synthesis, Characterization, and Applications of Polymer Nanocomposites. Spec. Issues 105, 1–7.
Phonsa, S., Sreearunothai, P., Charojrochkul, S., and Sombatmankhong, K. (2018). Electrodeposition of MnO2 on Polypyrrole-Coated Stainless Steel to Enhance Electrochemical Activities in Microbial Fuel Cells. Solid State Ionics 316, 125–134. doi:10.1016/j.ssi.2017.11.022
Plekhanova, Y., Tarasov, S., Kolesov, V., Kuznetsova, I., Signore, M., Quaranta, F., et al. (2018). Effects of Polymer Matrices and Carbon Nanotubes on the Generation of Electric Energy in a Microbial Fuel Cell. Membranes 8, 99. doi:10.3390/membranes8040099
Pophali, A., Singh, S., and Verma, N. (2021). A Dual Photoelectrode-Based Double-Chambered Microbial Fuel Cell Applied for Simultaneous COD and Cr (VI) Reduction in Wastewater. Int. J. Hydrogen Energy 46, 3160–3170. doi:10.1016/j.ijhydene.2020.06.162
Prakash, O., Mungray, A., Chongdar, S., Kailasa, S. K., and Mungray, A. K. (2020). Performance of Polypyrrole Coated Metal Oxide Composite Electrodes for Benthic Microbial Fuel Cell (BMFC). J. Environ. Chem. Eng. 8, 102757. doi:10.1016/j.jece.2018.11.002
Praveen, R., Chandreshia, C. T. B., and Ramaraj, R. (2018). Silicate Sol-Gel Matrix Stabilized ZnO-Ag Nanocomposites Materials and Their Environmental Remediation Applications. J. Environ. Chem. Eng. 6, 3702–3708. doi:10.1016/j.jece.2017.01.048
Pu, K.-B., Ma, Q., Cai, W.-F., Chen, Q.-Y., Wang, Y.-H., and Li, F.-J. (2018). Polypyrrole Modified Stainless Steel as High Performance Anode of Microbial Fuel Cell. Biochem. Eng. J. 132, 255–261. doi:10.1016/j.bej.2018.01.018
Ramírez-Vargas, C., Prado, A., Arias, C., Carvalho, P., Esteve-Núñez, A., and Brix, H. (2018). Microbial Electrochemical Technologies for Wastewater Treatment: Principles and Evolution from Microbial Fuel Cells to Bioelectrochemical-Based Constructed Wetlands. Water 10, 1128–1129. doi:10.3390/w10091128
Regmi, R., Nitisoravut, R., Charoenroongtavee, S., Yimkhaophong, W., and Phanthurat, O. (2018a). Earthen Pot-Plant Microbial Fuel Cell Powered by Vetiver for Bioelectricity Production and Wastewater Treatment. Clean. - Soil, Air, Water 46, 1700193. doi:10.1002/clen.201700193
Regmi, R., Nitisoravut, R., and Ketchaimongkol, J. (2018b). A Decade of Plant-Assisted Microbial Fuel Cells: Looking Back and Moving Forward. Biofuels 9, 605–612. doi:10.1080/17597269.2018.1432272
Rikame, S. S., Mungray, A. A., and Mungray, A. K. (2018). Modification of Anode Electrode in Microbial Fuel Cell for Electrochemical Recovery of Energy and Copper Metal. Electrochimica Acta 275, 8–17. doi:10.1016/j.electacta.2018.04.141
Rostami-Vartooni, A., Moradi-Saadatmand, A., and Mahdavi, M. (2018). Catalytic Reduction of Organic Pollutants Using Biosynthesized Ag/C/Fe3O4 Nanocomposite by Red Water and Caesalpinia Gilliesii Flower Extract. Mater. Chem. Phys. 219, 328–339. doi:10.1016/j.matchemphys.2018.08.026
Roy, M., Roychowdhury, R., and Mukherjee, P. (2018). Remediation of Fly Ash Dumpsites through Bioenergy Crop Plantation and Generation: A Review. Pedosphere 28, 561–580. doi:10.1016/S1002-0160(18)60033-5
Sagastume Gutiérrez, A., Cabello Eras, J. J., Sousa Santos, V., Hernández Herrera, H., Hens, L., and Vandecasteele, C. (2018). Electricity Management in the Production of Lead-Acid Batteries: The Industrial Case of a Production Plant in Colombia. J. Clean. Prod. 198, 1443–1458. doi:10.1016/j.jclepro.2018.07.105
Saini, J., Garg, V. K., and Gupta, R. K. (2020). Green Synthesized SiO2@OPW Nanocomposites for Enhanced Lead (II) Removal from Water. Arabian J. Chem. 13, 2496–2507. doi:10.1016/j.arabjc.2018.06.003
Saleem, J., Adil Riaz, M., and Gordon, M. (2018). Oil Sorbents from Plastic Wastes and Polymers: A Review. J. Hazard. Mater. 341, 424–437. doi:10.1016/j.jhazmat.2017.07.072
Salihoglu, G., Salihoglu, N. K., Ucaroglu, S., and Banar, M. (2018). Food Loss and Waste Management in Turkey. Bioresour. Technol. 248, 88–99. doi:10.1016/j.biortech.2017.06.083
Samaddar, P., Ok, Y. S., Kim, K.-H., Kwon, E. E., and Tsang, D. C. W. (2018). Synthesis of Nanomaterials from Various Wastes and Their New Age Applications. J. Clean. Prod. 197, 1190–1209. doi:10.1016/j.jclepro.2018.06.262
Santoro, C., Arbizzani, C., Erable, B., and Ieropoulos, I. (2017). Microbial Fuel Cells: From Fundamentals to Applications. A Review. J. Power Sources 356, 225–244. doi:10.1016/j.jpowsour.2017.03.109
Santoro, C., Flores-Cadengo, C., Soavi, F., Kodali, M., Merino-Jimenez, I., Gajda, I., et al. (2018a). Ceramic Microbial Fuel Cells Stack: Power Generation in Standard and Supercapacitive Mode. Sci. Rep. 8. doi:10.1038/s41598-018-21404-y
Santoro, C., Kodali, M., Herrera, S., Serov, A., Ieropoulos, I., and Atanassov, P. (2018b). Power Generation in Microbial Fuel Cells Using Platinum Group Metal-free Cathode Catalyst: Effect of the Catalyst Loading on Performance and Costs. J. Power Sources 378, 169–175. doi:10.1016/j.jpowsour.2017.12.017
Saravanan, P. (2018). Microbial Fuel Cell: a Prospective Sustainable Solution for Energy and Environmental Crisis. Ijbsbe 4, 191–193. doi:10.15406/ijbsbe.2018.04.00124
Sarma, M. K., Quadir, M. G. A., Bhaduri, R., Kaushik, S., and Goswami, P. (2018). Composite Polymer Coated Magnetic Nanoparticles Based Anode Enhances Dye Degradation and Power Production in Microbial Fuel Cells. Biosens. Bioelectron. 119, 94–102. doi:10.1016/j.bios.2018.07.065
Schröder, U., and Harnisch, F. (2017). Life Electric-Nature as a Blueprint for the Development of Microbial Electrochemical Technologies. Joule 1, 244–252. doi:10.1016/j.joule.2017.07.010
Singh, S., Bairagi, P. K., and Verma, N. (2018). Candle Soot-Derived Carbon Nanoparticles: An Inexpensive and Efficient Electrode for Microbial Fuel Cells. Electrochimica Acta 264, 119–127. doi:10.1016/j.electacta.2018.01.110
Song, R.-B., Wu, Y., Lin, Z.-Q., Xie, J., Tan, C. H., Loo, J. S. C., et al. (2017). Living and Conducting: Coating Individual Bacterial Cells with In Situ Formed Polypyrrole. Angew. Chem. Int. Ed. 56, 10516–10520. doi:10.1002/anie.201704729
Spasiano, D., and Pirozzi, F. (2017). Treatments of Asbestos Containing Wastes. J. Environ. Manag. 204, 82–91. doi:10.1016/j.jenvman.2017.08.038
Srikanth, S., Kumar, M., and Puri, S. K. (2018). Bio-electrochemical System (BES) as an Innovative Approach for Sustainable Waste Management in Petroleum Industry. Bioresour. Technol. 265, 506–518. doi:10.1016/j.biortech.2018.02.059
Stoll, Z., Dolfing, J., and Xu, P. (2018). Minimum Performance Requirements for Microbial Fuel Cells to Achieve Energy-Neutral Wastewater Treatment. Water 10, 243. doi:10.3390/w10030243
Suganya, S., and Senthil Kumar, P. (2018). Evaluation of Environmental Aspects of Brew Waste-Based Carbon Production and its Disposal Scenario. J. Clean. Prod. 202, 244–252. doi:10.1016/j.jclepro.2018.08.143
Sushma, S., and S, H. A. K. (2017). Designing the Shape of Graphite Anode for Microbial Fuel Cells to Increase its Efficiency. Int. Res. J. Eng. Technol. 4, 553–556.
Varanasi, J. L., Kumari, S., and Das, D. (2018). Improvement of Energy Recovery from Water Hyacinth by Using Integrated System. Int. J. Hydrogen Energy 43, 1303–1318. doi:10.1016/j.ijhydene.2017.11.110
Vilajeliu-Pons, A., Puig, S., Salcedo-Dávila, I., Balaguer, M. D., and Colprim, J. (2017). Long-term Assessment of Six-Stacked Scaled-Up MFCs Treating Swine Manure with Different Electrode Materials. Environ. Sci. Water Res. Technol. 3, 947–959. doi:10.1039/c7ew00079k
Vo Hoang Nhat, P., Ngo, H. H., Guo, W. S., Chang, S. W., Nguyen, D. D., Nguyen, P. D., et al. (2018). Can Algae-Based Technologies Be an Affordable Green Process for Biofuel Production and Wastewater Remediation? Bioresour. Technol. 256, 491–501. doi:10.1016/j.biortech.2018.02.031
Wang, D., Li, Y., Li Puma, G., Lianos, P., Wang, C., and Wang, P. (2017a). Photoelectrochemical Cell for Simultaneous Electricity Generation and Heavy Metals Recovery from Wastewater. J. Hazard. Mater. 323, 681–689. doi:10.1016/j.jhazmat.2016.10.037
Wang, D., Pillai, S. C., Ho, S.-H., Zeng, J., Li, Y., and Dionysiou, D. D. (2018a). Plasmonic-based Nanomaterials for Environmental Remediation. Appl. Catal. B Environ. 237, 721–741. doi:10.1016/j.apcatb.2018.05.094
Wang, G., and Feng, C. (2017). Electrochemical Polymerization of Hydroquinone on Graphite Felt as a Pseudocapacitive Material for Application in a Microbial Fuel Cell. Polymers 9, 220. doi:10.3390/polym9060220
Wang, J., Zhou, B., Ge, R., SongshunYu, T.-s. J., Yu, J., and Xie, J. (2018b). Degradation Characterization and Pathway Analysis of Chlortetracycline and Oxytetracycline in a Microbial Fuel Cell. RSC Adv. 8, 28613–28624. doi:10.1039/c8ra04904a
Wang, Y., Wu, J., Yang, S., Li, H., and Li, X. (2018c). Electrode Modification and Optimization in Air-Cathode Single-Chamber Microbial Fuel Cells. Ijerph 15, 1349. doi:10.3390/ijerph15071349
Wang, Z., Mahadevan, G. D., Wu, Y., and Zhao, F. (2017b). Progress of Air-Breathing Cathode in Microbial Fuel Cells. J. Power Sources 356, 245–255. doi:10.1016/j.jpowsour.2017.02.004
Wu, X., Qiao, Y., Shi, Z., and Li, C. M. (2018a). Enhancement of Interfacial Bioelectrocatalysis in Shewanella Microbial Fuel Cells by a Hierarchical Porous Carbon-Silica Composite Derived from Distiller's Grains. Sustain. Energy Fuels 2, 655–662. doi:10.1039/c7se00560a
Wu, X., Xiong, X., Owens, G., Brunetti, G., Zhou, J., Yong, X., et al. (2018b). Anode Modification by Biogenic Gold Nanoparticles for the Improved Performance of Microbial Fuel Cells and Microbial Community Shift. Bioresour. Technol. 270, 11–19. doi:10.1016/j.biortech.2018.08.092
Xia, C., Zhang, D., Pedrycz, W., Zhu, Y., and Guo, Y. (2018). Models for Microbial Fuel Cells: A Critical Review. J. Power Sources 373, 119–131. doi:10.1016/j.jpowsour.2017.11.001
Xie, T., Jing, Z., Hu, J., Yuan, P., Liu, Y., and Cao, S. (2018). Degradation of Nitrobenzene-Containing Wastewater by a Microbial-Fuel-Cell-Coupled Constructed Wetland. Ecol. Eng. 112, 65–71. doi:10.1016/j.ecoleng.2017.12.018
Xu, F., Li, H., Liu, Y., and Jing, Q. (2017). Advanced Redox Flow Fuel Cell Using Ferric Chloride as Main Catalyst for Complete Conversion from Carbohydrates to Electricity. Sci. Rep. 7, 1–9. doi:10.1038/s41598-017-05535-2
Yamasaki, R., Maeda, T., and Wood, T. K. (2018). Electron Carriers Increase Electricity Production in Methane Microbial Fuel Cells that Reverse Methanogenesis. Biotechnol. Biofuels 11, 1–10. doi:10.1186/s13068-018-1208-7
Yamashita, T., and Yokoyama, H. (2018). Molybdenum Anode: A Novel Electrode for Enhanced Power Generation in Microbial Fuel Cells, Identified via Extensive Screening of Metal Electrodes. Biotechnol. Biofuels 11, 1–13. doi:10.1186/s13068-018-1046-7
Yousefi, V., Mohebbi-Kalhori, D., and Samimi, A. (2018). Application of Layer-By-Layer Assembled Chitosan/montmorillonite Nanocomposite as Oxygen Barrier Film over the Ceramic Separator of the Microbial Fuel Cell. Electrochimica Acta 283, 234–247. doi:10.1016/j.electacta.2018.06.173
Yuan, H., Hou, Y., Abu-Reesh, I. M., Chen, J., and He, Z. (2016). Oxygen Reduction Reaction Catalysts Used in Microbial Fuel Cells for Energy-Efficient Wastewater Treatment: a Review. Mat. Horiz. 3, 382–401. doi:10.1039/C6MH00093B
Zeng, Q., Bai, J., Li, J., Li, L., Xia, L., Zhou, B., et al. (2018). Highly-stable and Efficient Photocatalytic Fuel Cell Based on an Epitaxial TiO2/WO3/W Nanothorn Photoanode and Enhanced Radical Reactions for Simultaneous Electricity Production and Wastewater Treatment. Appl. Energy 220, 127–137. doi:10.1016/j.apenergy.2018.03.042
Zhang, H., Ma, D., Qiu, R., Tang, Y., and Du, C. (2017a). Non-thermal Plasma Technology for Organic Contaminated Soil Remediation: A Review. Chem. Eng. J. 313, 157–170. doi:10.1016/j.cej.2016.12.067
Zhang, M., Wang, Y., Liang, P., Zhao, X., Liang, M., and Zhou, B. (2019). Combined Photoelectrocatalytic Microbial Fuel Cell (PEC-MFC) Degradation of Refractory Organic Pollutants and In-Situ Electricity Utilization. Chemosphere 214, 669–678. doi:10.1016/j.chemosphere.2018.09.085
Zhang, Q., Zhang, L., Wang, H., Jiang, Q., and Zhu, X. (2018b). Simultaneous Efficient Removal of Oxyfluorfen with Electricity Generation in a Microbial Fuel Cell and its Microbial Community Analysis. Bioresour. Technol. 250, 658–665. doi:10.1016/j.biortech.2017.11.091
Zhang, Y., Chen, X., Yuan, Y., Lu, X., Yang, Z., Wang, Y., et al. (2018c). Long-term Effect of Carbon Nanotubes on Electrochemical Properties and Microbial Community of Electrochemically Active Biofilms in Microbial Fuel Cells. Int. J. Hydrogen Energy 43, 16240–16247. doi:10.1016/j.ijhydene.2018.06.144
Zhang, Y., Liu, L., Van Der Bruggen, B., and Yang, F. (2017b). Nanocarbon Based Composite Electrodes and Their Application in Microbial Fuel Cells. J. Mat. Chem. A 5, 12673–12698. doi:10.1039/c7ta01511a
Zhao, C.-e., Gai, P., Song, R., Chen, Y., Zhang, J., and Zhu, J.-J. (2017). Nanostructured Material-Based Biofuel Cells: Recent Advances and Future Prospects. Chem. Soc. Rev. 46, 1545–1564. doi:10.1039/c6cs00044d
Zheng, W., Liu, Y., Liu, W., Ji, H., Li, F., Shen, C., et al. (2021). A Novel Electrocatalytic Filtration System with Carbon Nanotube Supported Nanoscale Zerovalent Copper toward Ultrafast Oxidation of Organic Pollutants. Water Res. 194, 116961. doi:10.1016/j.watres.2021.116961
Keywords: bioelectricity, nanomaterials, anode, recovery, microbial fuel cell
Citation: Dessie Y and Tadesse S (2022) Advancements in Bioelectricity Generation Through Nanomaterial-Modified Anode Electrodes in Microbial Fuel Cells. Front. Nanotechnol. 4:876014. doi: 10.3389/fnano.2022.876014
Received: 14 February 2022; Accepted: 19 April 2022;
Published: 17 May 2022.
Edited by:
Swee Su Lim, National University of Malaysia, MalaysiaReviewed by:
Yanbiao Liu, Donghua University, ChinaMelvin Samuel, University of Wisconsin–Milwaukee, United States
Copyright © 2022 Dessie and Tadesse. This is an open-access article distributed under the terms of the Creative Commons Attribution License (CC BY). The use, distribution or reproduction in other forums is permitted, provided the original author(s) and the copyright owner(s) are credited and that the original publication in this journal is cited, in accordance with accepted academic practice. No use, distribution or reproduction is permitted which does not comply with these terms.
*Correspondence: Yilkal Dessie, yilikaldessie@gmail.com