Researching glutamate – induced cytotoxicity in different cell lines: a comparative/collective analysis/study
- Laboratory of Physiology, Department of Physiology and Pharmacology, School of Medicine, Faculty of Health Sciences, Aristotle University of Thessaloniki, Thessaloniki, Greece
Although glutamate is one of the most important excitatory neurotransmitters of the central nervous system, its excessive extracellular concentration leads to uncontrolled continuous depolarization of neurons, a toxic process called, excitotoxicity. In excitotoxicity glutamate triggers the rise of intracellular Ca2+ levels, followed by up regulation of nNOS, dysfunction of mitochondria, ROS production, ER stress, and release of lysosomal enzymes. Excessive calcium concentration is the key mediator of glutamate toxicity through over activation of ionotropic and metabotropic receptors. In addition, glutamate accumulation can also inhibit cystine (CySS) uptake by reversing the action of the CySS/glutamate antiporter. Reversal of the antiporter action reinforces the aforementioned events by depleting neurons of cysteine and eventually glutathione’s reducing potential. Various cell lines have been employed in the pursuit to understand the mechanism(s) by which excitotoxicity affects the cells leading them ultimately to their demise. In some cell lines glutamate toxicity is exerted mainly through over activation of NMDA, AMPA, or kainate receptors whereas in other cell lines lacking such receptors, the toxicity is due to glutamate induced oxidative stress. However, in the greatest majority of the cell lines ionotropic glutamate receptors are present, co-existing to CySS/glutamate antiporters and metabotropic glutamate receptors, supporting the assumption that excitotoxicity effect in these cells is accumulative. Different cell lines differ in their responses when exposed to glutamate. In this review article the responses of PC12, SH-SY5Y, HT-22, NT-2, OLCs, C6, primary rat cortical neurons, RGC-5, and SCN2.2 cell systems are systematically collected and analyzed.
Introduction
Glutamate is one of the main excitatory neurotransmitters of the CNS, contributing to normal neural transmission, development, differentiation, and plasticity. However, excessive extracellular glutamate concentration can lead to uncontrolled continuous depolarization of neurons, a toxic process called, excitotoxicity, leading eventually to neuronal death. Excitotoxicity is associated with many neurodegenerative conditions such as Huntington’s disease, Alzheimer’s disease, lateral amyotrophic sclerosis, Parkinson’s disease and stroke or traumatic brain injury.
Glutamate both in neurons and glial cells is synthesized through the tricarboxylic acid cycle and additionally in neurons by the glutamate–glutamine cycle, where it is accumulated in vesicles for future release. Glutamate is ligand to post-synaptic either iGluRs or mGluRs. Under pathological stimuli, glutamate release is excessive; GluR over activation ensues, resulting in an augmented intracellular Ca2+ influx.
Increased intracellular Ca2+ concentration disrupts calcium homeostasis and initiates a cascade of signaling pathways, leading to up regulation of nNOS, dysfunction of mitochondria, deregulation of oxidative phosphorylation, ROS production, ER stress, and release of lysosomal enzymes. Excessive calcium concentration is the key mediator of glutamate toxicity through over activation of ionotropic and metabotropic receptors. In addition, glutamate accumulation can also inhibit CySS uptake by reversing the action of the CySS/glutamate antiporter (Xc-). Reversal of Xc- action reinforces the aforementioned events by depleting neurons of CySS and eventually GSH, leading to free radical accumulation. In the absence of glutamate receptors, glutamate toxicity can occur through this antiporter promoting a Ca2+ independent, non-receptor mediated oxidative glutamate toxicity. Glutamate exerts its toxic effects through molecular pathways, which lead to neurodegeneration and cell death, for reviews see (Wang and Qin, 2010; Lai et al., 2014).
In the last three decades various cell models have been used in excitotoxicity studies and different pathways pertaining to cell survival and/or cell death have been reported to be triggered in each cell line. This review summarizes the effect of excitotoxicity on the homeostasis of the cellular organelles, the cell signaling pertaining to survival and cell death and focuses on the cell lines that have been used as models for the study of the excitotoxicity.
Glutamate-Induced Cytotoxicity Triggering and the Effect on Intracellular Organelles
Glutamate Release and Reuptake: the Glutamate–Glutamine Cycle and Xc-
During neurotransmission, glutamate is released by depolarization of pre-synaptic membranes via a Ca2+-dependent process, involving VDCCs (Meldrum, 1994; Anderson and Swanson, 2000). VDCCs are of N, P/Q, R, and L-type characterized by their subunit composition and their inhibition by specific toxins. They mediate glutamate synaptic release in CNS and their distribution among nerve terminals varies. In certain terminals, only one type is present, while others possess more than one (Reid et al., 2003).
Neurotransmission is ended within millisecond by efficient glutamate reuptake via Na+-dependent high affinity glutamate membrane EAATs: EAAT1 (GLAST), EAAT2 (GLT1), EAAT3 (EAAC1), EAAT4, and EAAT5. EAAT2 is commonly expressed in glial cells and EAAT3 in neurons. EAAT2 is believed to play the main role in regulating extracellular glutamate concentration (Danbolt, 2001). In glial cells reuptaken glutamate is converted to glutamine by glutamine synthetase thus ending neurotransmission, offering neuroprotection and preventing excitotoxicity. Glial glutamine is taken up into the presynaptic neuron via Na+-dependent glutamine uptake systems, where it is converted to glutamate by glutaminase thus completing the glutamate–glutamine cycle (Attwell, 2000; Daikhin and Yudkoff, 2000; Hertz et al., 2007; Figure 1).
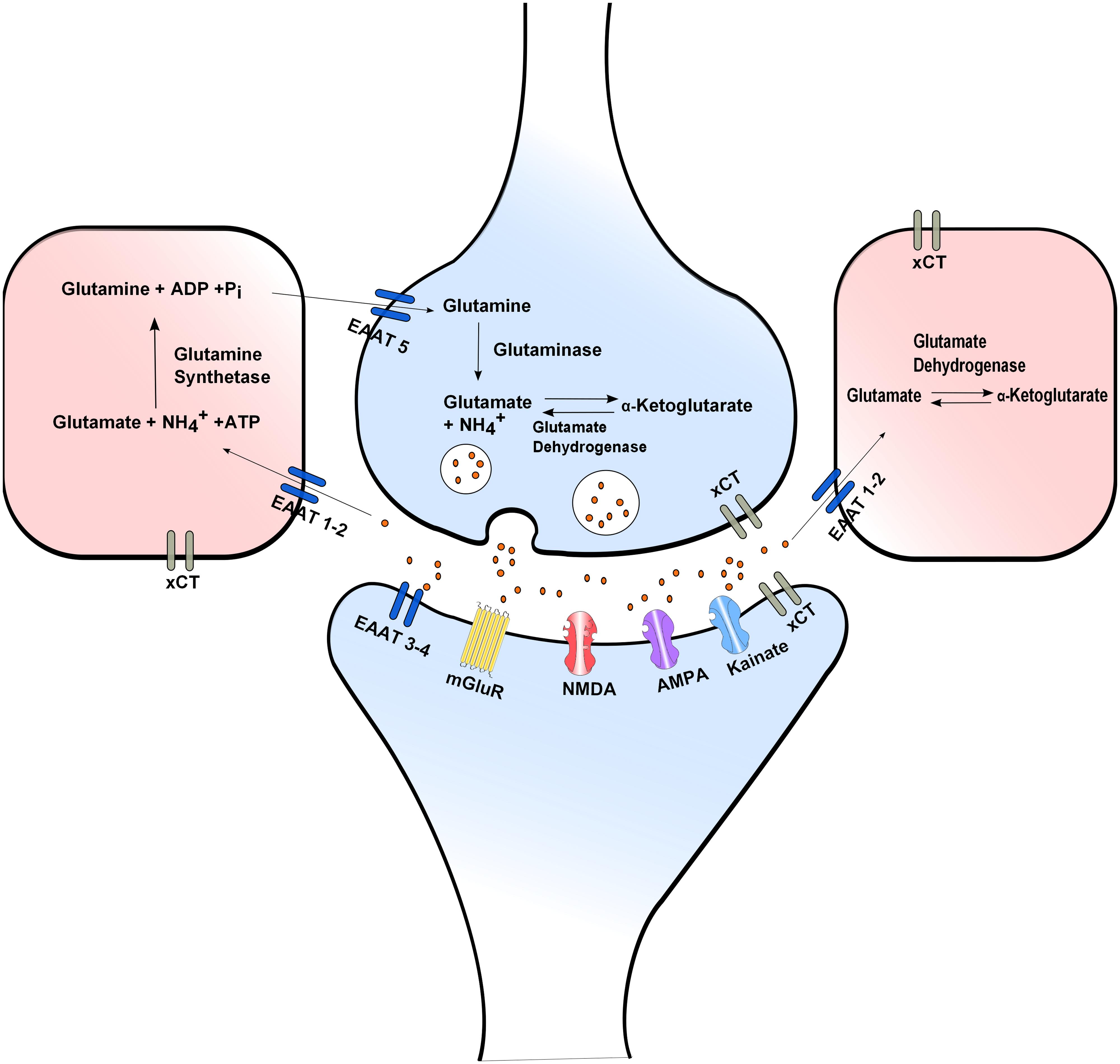
FIGURE 1. Glutamate release and uptake, the Xc- antiporter and the glutamate/glutamine cycle. In glial cells reuptaken glutamate is converted to glutamine by glutamine synthetase. Glial glutamine is taken up into the presynaptic neuron via Na+-dependent glutamine uptake systems, where it is converted to glutamate by glutaminase. Extra- and intra-cellular glutamate concentrations are modulated through the XC- antiporter. Neurotransmission is ended by efficient glutamate reuptake via Na+-dependent high affinity glutamate membrane EAATs.
The Ca2+-independent glutamate release is attributed to reverse action of the aforementioned glutamate transporters. Reverse action of glutamate transporters can occur during depolarization, when the Na+ and K+ gradients are diminished possibly contributing to the regulation of glutamatergic neurotransmission. In hypoxia reduced expression of EAAT1 and 2 contributes to increase extracellular glutamate concentration leading to neuronal overexcitation and excitotoxicity (Rossi et al., 2007; Niciu et al., 2012).
Another important molecule modulating both extra- and intra-cellular glutamate concentrations is the XC-. This is of added importance for the nervous system since L-cystine taken up by the cells can be used for GSH synthesis and protection from oxidative insults, for a review see (Bridges et al., 2012). Excessive extracellular glutamate concentration blocks the uptake of CySS which is essential for biosynthesis of GSH. GSH depletion influences the capacity of cells to scavenge free radicals, a fact that makes cells vulnerable to secondary events such as accumulation of ROS and alteration in Ca2+ homeostatic mechanisms resulting in cell death (Fukui et al., 2009).
Glutamatergic Neurotransmition: the Glutamate Receptors
There are two types of glutamate receptors categorized according to their function. iCluRs functioning upon binding of glutamate as ion channels and mGluRs. mGluRs are G protein coupled receptors, coupled to their associated ion channels via a second messenger cascade. iGluRs are named after their respective agonist, NMDARs, AMPARs, and KARs. They are multimeric assemblies of different protein subunits that form homo or heteromeric complexes of varying subunit combination, resulting to multiple types of ion channels with different properties. iGluRs mediate fast synaptic transmission and are broadly classified in two classes as NMDA and non-NMDA receptors (Keinanen et al., 1990; Monyer et al., 1992; Nakanishi et al., 1994).
N-methyl-D-aspartate receptors are complex structures able to bind glutamate, glycine, Mg2+, Zn2+, and polyamines. Composed from seven subunits (one NR1, four NR2, and two NR3), their function is determined by the combination of NR1 and NR2 subunits. NMDARs form channels that are more permeable to Ca2+ than Na+ and K+. Upon binding of glutamate the magnesium ions, blocking the ion channel, are released and consequently the ion channel is activated allowing the influx of the aforementioned ions into the cytoplasm (Mehta et al., 2012). Kainate and AMPA receptors interact only with glutamate and their specific agonists, and their associated channels are more permeable to Na+ and K+ than Ca2+(Kostandy, 2012; Figure 2).
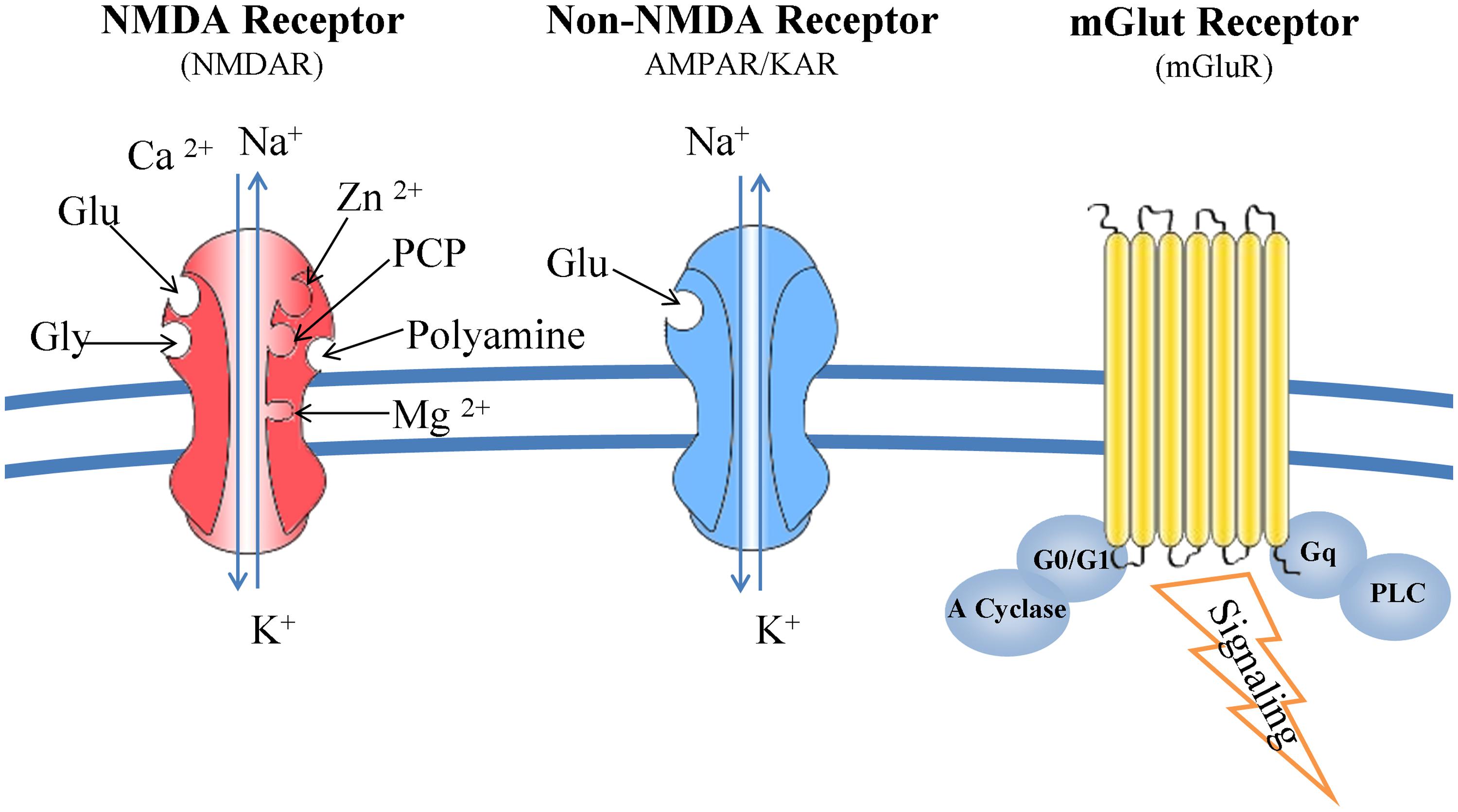
FIGURE 2. Glutamate receptors: structure and function. NMDARs bind glutamate, glycine, Mg2+, Zn2+, and polyamines. Composed from seven subunits (one NR1, four NR2, and two NR3), their function is determined by the combination of NR1 and NR2 subunits. NMDARs form channels that are more permeable to Ca2+ than Na+ and K+. Kainate and AMPA receptors interact only with glutamate and their specific agonists, and their associated channels are more permeable to Na+ and K+ than Ca2+. mGluRs are G-protein coupled receptors and trigger a second messenger cascade. They are found both at the pre- and post-synaptic neurons, subunits of metabotropic receptors are also expressed in microglia.
Metabotropic glutamate receptors are G-protein coupled receptors and trigger a second messenger cascade (Skeberdis et al., 2001a; Lea et al., 2005). They are found both at the pre- and post-synaptic neurons, but also subunits of metabotropic receptors are expressed in microglia (Janssens and Lesage, 2001; Byrnes et al., 2009; Figure 2).
There are eight different types of mGluRs (mGluR1 to mGLUR8) and are classified to three groups according to their structure and physiological activity (groups I, II, and III). Group I (mGluR 1 and 5) are mainly post-synaptic receptors coupled to a Gq heterotrimeric G protein. Upon binding of glutamate, PLC is activated and 1,4,5-inositol triphosphate is produced initiating multiple intracellular responses. Evidence is presented for PKC-dependent and independent pathways potentiating the NMDA responses by mGluRs (Kelso et al., 1992; Ugolini et al., 1997; Skeberdis et al., 2001b). Groups II and III are coupled to Gi/G0 thus inhibiting the action of adenylate cycle and reducing intracellular cAMP levels.
Pertaining to excitotoxicity, NMDARs play the most important role as larger quantities of Ca2+ ions can be moved through them (Bloom, 1994). The role of kainate and AMPA receptors is also important as it has been shown that their activation is directly related to the ER stress. Activation of the iGluRs creates a depolarization (excitatory post-synaptic current) and depending on the number of the receptors that are activated, this current, can lead to an action potential. This current is also essential to the function of NMDARs since it can lift their voltage dependent Mg2+ block (Johnson and Ascher, 1990).
Of the mGluRs class I is positively connected with excitotoxicity by modulating the NMDA receptor activity while classes II and III are negatively related to the phenomenon, through the suppression of intracellular cAMP levels inhibiting the export of potentially neurotoxic glutamate from microglia offering a neuroprotective role (Ambrosini et al., 1995; Faden et al., 1997; Allen et al., 1999; McMullan et al., 2012).
Glutamate Receptors and Cell Death
Both iGluRs and mGluRs mediate the excitotoxic insult via distinct, but not independent pathways. However, key players in the initiation of the exitoxic insult are the iCluRs. The elevated Ca2+ concentration upregulates the activity of nNOS, contributes to mitochondrial activity deregulation and ER stress, leading to cell membrane depolarization and dysfunction of intracellular organelles. As iGluRs are permeable not only to Ca2+ but also to Na+ and K+, their activation leads to depolarization of the cell membrane and osmotic inflow of water.
N-methyl-D-aspartate receptor activity can be modulated by mGluRs. mGluRs do not participate directly in the excitotoxic insult, but rather seem to modulate it. NMDARs can be up regulated by Src kinase mediated tyrosine phosphorylation, leading to increased channel permeability (Salter, 1998; Ali and Salter, 2001). Group I are positively coupled via a Gq-protein to PLC, resulting in the release of Ca2+ from intracellular stores (Skeberdis et al., 2001a). Augmented intracellular Ca2+ concentration can lead to the sequential activation of PKC, Pyk2 and Src, resulting in the tyrosine phosphorylation of NMDARs (Lu et al., 2011). NMDAR phosphorylation via Src family results in its up regulation (Dikic et al., 1996; Salter, 1998) and increases open channel probability (Salter, 1998; Ali and Salter, 2001). PKC and mGluR 1 potentiate NMDARs currents not only by increased open channel probability but also by recruiting new channels to the membrane in cooperation with cytoskeletal proteins (Lan et al., 2001).
Both mGluR1 and mGluR5 lead to the potentiation of NMDA receptor currents via Src-dependent mechanism. Alternatively others (Minakami et al., 1997; Ishikawa et al., 1999; Shinohara et al., 2001) support that both mGluR1 and mGluR5 can interact with calmodulin in a calcium dependent manner, proposing that other molecules besides PKC may be responsible for the mGluR1 mediated activation of Pyk2/Src and the potentiation NMDARs currents (Figure 3). Group II (mGluR2 and 3) and III (mGluR4, 6, 7, and 8) are coupled to Gi/G0 proteins leading to inhibition of adenylate cyclase decreasing the levels of cAMP in the cytoplasm (Conn and Pin, 1997). Subunits of metabotropic receptors (groups I, II, and III) are also expressed in microglia. Groups II and III through the suppression of intracellular cAMP levels inhibit the export of potential neurotoxic glutamate from microglia offering a neuroprotective role (McMullan et al., 2012).
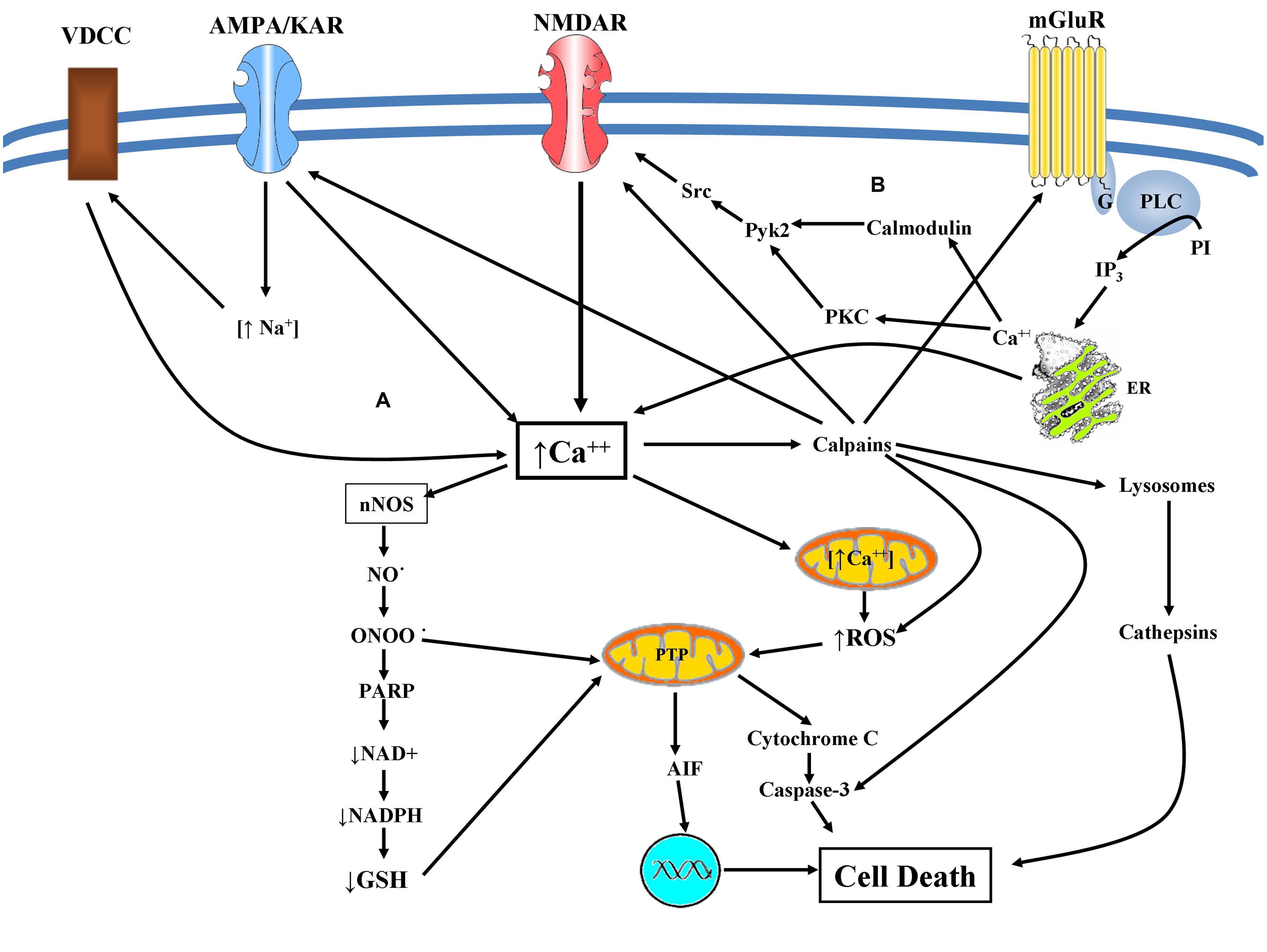
FIGURE 3. (A) Excitotoxicity mediated cell death: glutamate excitotoxicity causes Ca2+ mediated NO production leading to mitochondrial dysfunction resulting in superoxide production. Peroxynitrite is produced causing lipid peroxidation, direct DNA damage, and protein dysfunction. Peroxynitrite inhibits the mitochondrial electron transport chain, cytochrome c normal activity as well as of superoxide dismutase via protein nitration. Activation of ryanodine receptors in conjunction with accumulation of misfolded proteins and depletion of endoplasmic Ca2+ storage, results in ER dysfunction (ER-stress). These facts can provoke caspase mediated cell death and an eventual apoptotic cell death. Alternatively augmented intracellular Ca2+ concentration can lead to calpain activation engaging both calpain dependent and cathepsin dependent cell death. (B) mGluRs and NMDAR crosstalk: both mGluR1 and mGluR5 lead to the potentiation of NMDA receptor currents via Src-dependent mechanism in a PKC or calmodulin depended manner.
Recently it is reported that calpains, proteases activated by elevated intracellular calcium concentration, form a negative feedback loop for controlling calcium influx, by means of cleavage and subsequent inactivation of glutamate receptors. This feedback loop may contribute to a neuroprotective effect against the excitotoxic insult (Doshi and Lynch, 2009; McMullan et al., 2012; Figure 3).
nNOS Up Regulation and Cell Death the Key Player
N-methyl-D-aspartate receptors are linked to neuronal nitric oxide synthase (nNOS), and therefore their activation leads to production of toxic NO-levels. NMDARs and nNOS are connected by the post-synaptic density protein PSD-95. Over activation of NMDARs under excitotoxic conditions provokes Ca2+ influx which results in activation of nNOS via calmodulin (Schrammel et al., 2003; Brown, 2010).
More recently, NO has been shown to interfere with neurotoxicity through an interaction with GAPDH (Hara et al., 2005). GAPDH/Siah 1(an E3 ubiquitin ligase) pathway is a novel pathway that links NO to a form of apoptotic-like death (Leach et al., 1986; Hara et al., 2005). Increased intracellular concentration of NO causes S-nitrosylation of GAPDH which triggers GAPDH binding to Siah 1. GAPDH/Siah1 complex is translocated to the nucleus resulting in the transcription of the proapoptotic factor p53 (Sen et al., 2008). Activation of nuclear proteins such as p53 results in pyknotic nucleus and other morphological characteristics that indicate apoptotic death (Sen et al., 2009). Moreover, GAPDH plays a pivotal role in glycolysis and it is possible that GAPDH-NO interaction leads to loss of function and energy failure (Vedia et al., 1992).
Glutamate excitotoxicity causes Ca2+ mediated NO production leading to mitochondrial dysfunction resulting in superoxide production, which is released via voltage dependent anion channels into the cytosol. NO-superoxide interaction can produce peroxynitrite an oxidative molecule that can cause lipid peroxidation, direct DNA damage and protein dysfunction (Radi et al., 1991a,b; Salgo et al., 1995). Peroxynitrite has many deleterious effects, such as the inhibition of mitochondrial electron transport chain (Radi et al., 1994), cytochrome c normal activity (Nakagawa et al., 2001) as well as of superoxide dismutase via protein nitration (Yamakura et al., 1998). These facts can provoke caspase mediated cell death and an eventual apoptotic cell death (Szab, 2003; Figure 3).
In addition NO activated soluble guanylate cyclase increase levels of cGMP. The three principal targets of cGMP are protein kinase G (PKG), cyclic-nucleotide gated channels (CNGCs), and cyclic-nucleotide phosphodiesterase (PDE). Of these, PKG provides the broadest means for controlling ion-channel function modulating this way neuronal excitability (Denninger and Marletta, 1999; Ahern et al., 2002).
Deregulation of Intracellular Organelles-Mitochondria, ER, Lysosomes
Deregulation of cell signaling due to excitotoxicity leads to altered function of intracellular organelles. Mitochondria, ER, and lysosomes are mostly affected by the increased Ca2+ influx, and their degeneration plays a pivotal role in neuronal death.
Mitochondria produce not only ATP, but also ROS and regulate Ca2+ homeostasis. Normally Ca2+ intake controls the activity of three dehydrogenases: pyruvate, isocitrate and ketoglutaric acid dehydrogenase, as well as ATP synthase. However, the increased influx of Ca2+ leads to mitochondrial Ca2+ overload and depolarization of mitochondrial membrane. The consequences of this overload is: (a) the activation of mitochondrial permeability transition pore, (b) phospholipase A2 and xanthine oxidase up-regulation, (c) inhibition of respiratory chain enzymes and (d) deactivation of catalase, superoxide dismutase, and GSH peroxidase (Yang et al., 2011; Cheng et al., 2012). The deregulation of respiratory chain enzymes firstly decreases ATP synthesis and secondly overproduces ROS, which cannot be neutralized by the cell. ATP depletion leads to neuronal bioenergetic failure and neurodegeneration. ROS react with biological molecules (lipids, proteins, nucleic acids, carbohydrates), producing new oxidative species, which trigger oxidative chain reactions of other macromolecules. In this way ROS bind to DNA evoking its fragmentation. Mitochondrial DNA, which lacks on histones, is especially vulnerable to ROS oxidation. The above in combination to PLC up-regulation, lead to membrane lipid peroxidation with the consequent membrane destabilization (Nicholls and Budd, 1998).
The result of all these factors is synaptic dysfunction, impaired neuronal plasticity and cell death via apoptosis, necrosis and/or autophagy. A central player in the potential driven mitochondrial Ca2+ uptake, is the mitochondrial Ca2+ uniporter (MCU), whose gene has been recently characterized (Luetjens et al., 2000; Pivovarova et al., 2004). In excitotoxicity Ca2+ uniporter acts as a mediator of death-signal, induced by loss of mitochondrial membrane potential (MMP), but can also serve a pro-survival role through neuroprotective Ca2+ signaling stemming from synaptic activity (Qiu et al., 2013; Figure 3).
Endoplasmic reticulum is an important cell organelle responsible for correct folding and sorting, translation, and post-translational modification of proteins and serves as an intracellular Ca2+ storage. ER is functionally connected to mitochondria through intracellular Ca2+ flow between them. ER functions can be disturbed by different insults such as accumulation of unfolded proteins and changes in Ca2+ homeostasis. Overstimulation of AMPA receptors results in inordinate Ca2+ concentration which leads to activation of ryanodine receptors (RyRs) located in ER (Ruiz et al., 2010; Mehta et al., 2012).
Activation of RyRs in conjunction with accumulation of misfolded proteins and depletion of endoplasmic Ca2+ storage, results in ER dysfunction (ER-stress). Cell response to ER-stress is called unfolded protein response (UPR; Boyce and Yuan, 2006) and consists of two repair mechanisms: activation of proteasome and ubiquitinization of dysfunctional proteins and induced expression of molecular chaperones (Verkhratsky, 2005; Boyce and Yuan, 2006; Ruiz et al., 2010).
Lysosomes are organelles which contain hydrolytic enzymes (proteases, nucleases, and lipases) necessary for intracellular digestion. Under excitotoxic conditions the number of lysosomes is increased because of enhanced induction of autophagy. It has been reported that NMDARs channeling in rat cerebellar granule neurons in culture, increased phaghosomes and their conjugation with lysosomes (Sadasivan et al., 2010). Moreover, several lines of evidence support a cross-talk between apoptosis and autophagy, since certain caspases can directly or indirectly activate cathepsins (Hsieh et al., 2009). Mitochondrial dysfunction leads eventually to activation of caspases which results in the release of cathepsins. The latter activates authophagy through release of lysosomal contents into the cytoplasm (Nixon et al., 2001; Terman et al., 2006).
Autophagy is a natural cell function in CNS since it plays a pivotal role in neuroprotection. It has been reported that traumatic brain injury is followed by enhanced autophagic processes. Activation of NR2B via CaMKII kinases contributes to the release of Beclin-1 which induces autophagy, while NR2B antagonists prevent excitotoxic-induced autophagy. Over activation of lysosomes and uncontrolled release of lysosomal enzymes caused by excitotoxicity contributes to neuronal death and brain pathology (Sadasivan et al., 2010; Figure 3).
Cell Lines Models Utilized for the Study of Glutamate-Induced Cytotoxicity
Based in bibliographic search we concluded that nine cell lines are widely used in researching in vitro excitotoxicity: PC12-rat pheochromocytoma, SH-SY5Y-human neuroblastoma, HT-22-immortalized mouse hippocampal cell line, Ntera /D1-NT-2 human teratocarcinoma, oligodendroglial lineage cells (OLCs), C6 -rat glioma, primary cortical rat neurons (PCRNs), RGC-5-mouse retinal ganglion cells, and SCN2.2 -hypothalamic suprachiasmatic nucleus (SCN) rat cell line. Below we summarize the methodology employed and the findings with respect to the signaling pathways activated in each cell line while researching excitotoxicity.
PC12
PC12 is a cell line derived from rat adrenal medulla pheochromocytoma, they synthesize dopamine and glutamate and can be induced to differentiate by NGF to a sympathetic phenotype expressing neurites and excitability (Greene and Tischler, 1976). PC12 cell line has been extensively used as a tool for studying the function of neurons, neuronal differentiation, and neurotoxicity.
Glutamate exerts its toxic effects on PC12 in a dose and time dependent manner. Its toxic concentration varies between 0.01 and 10 mM (Pereira and Oliveira, 1997, 2000; Penugonda et al., 2006; Pourzitaki et al., 2007, 2008, 2009; Lu et al., 2011) while time varies from 30 min to 3-12-24-48 h of incubation.
Even though mRNA for NMDA receptor subunits is expressed by PC12 cells (Schubert et al., 1992; Leclerc et al., 1995) there are contradictory findings, reported in the literature, concerning the receptor functionality, and presence of receptor protein. According to Sucher et al. (1993) only trace amounts of the receptor protein are present in PC12 cells and no functional NMDA-operated channels exist in this cells line. This has also been supported recently from in vitro findings in PC12 cell studies (Said et al., 1998; Vazhappilly and Sucher, 2002; Edwards et al., 2007). On the other hand, others support both the presence and functionality of the NMDARs in PC12 cells (Casado et al., 1996; Penugonda et al., 2006; Pourzitaki et al., 2007, 2008, 2009; Figure 3).
In addition to excitotoxicity, glutamate appears to exert a cytotoxic action at very high extracellular concentrations (5–10 mM; Murphy et al., 1990). This glutamate-induced cytotoxicity is independent of NMDARs and is mediated through the inhibition of CySS uptake leading to depletion of GSH and oxidative glutamate toxicity (Tyurin et al., 1998; Penugonda et al., 2006; Figure 4 and Table 1).
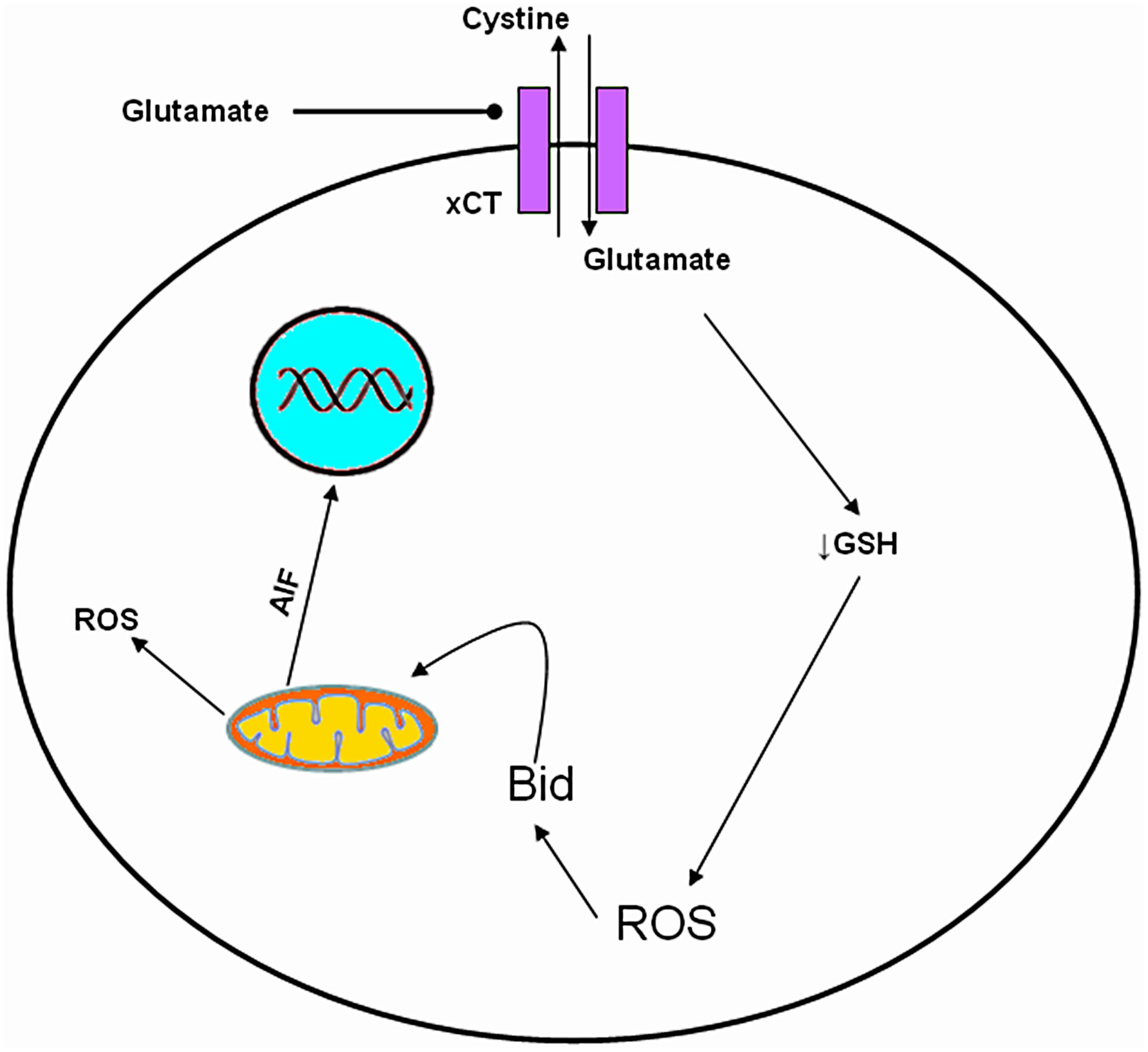
FIGURE 4. Molecular mechanism of oxidative glutamate toxicity. Increased extracellular glutamate concentration leads to reverse action of Cys glutamate antiporter. GSH depletion, follows due to decrease of intracellular Cys influencing the capacity of cells to scavenge free radicals, rendering them vulnerable mitochondrial dysfunction and to secondary events such as accumulation of ROS, production of Bid and AIF, resulting in cell death.
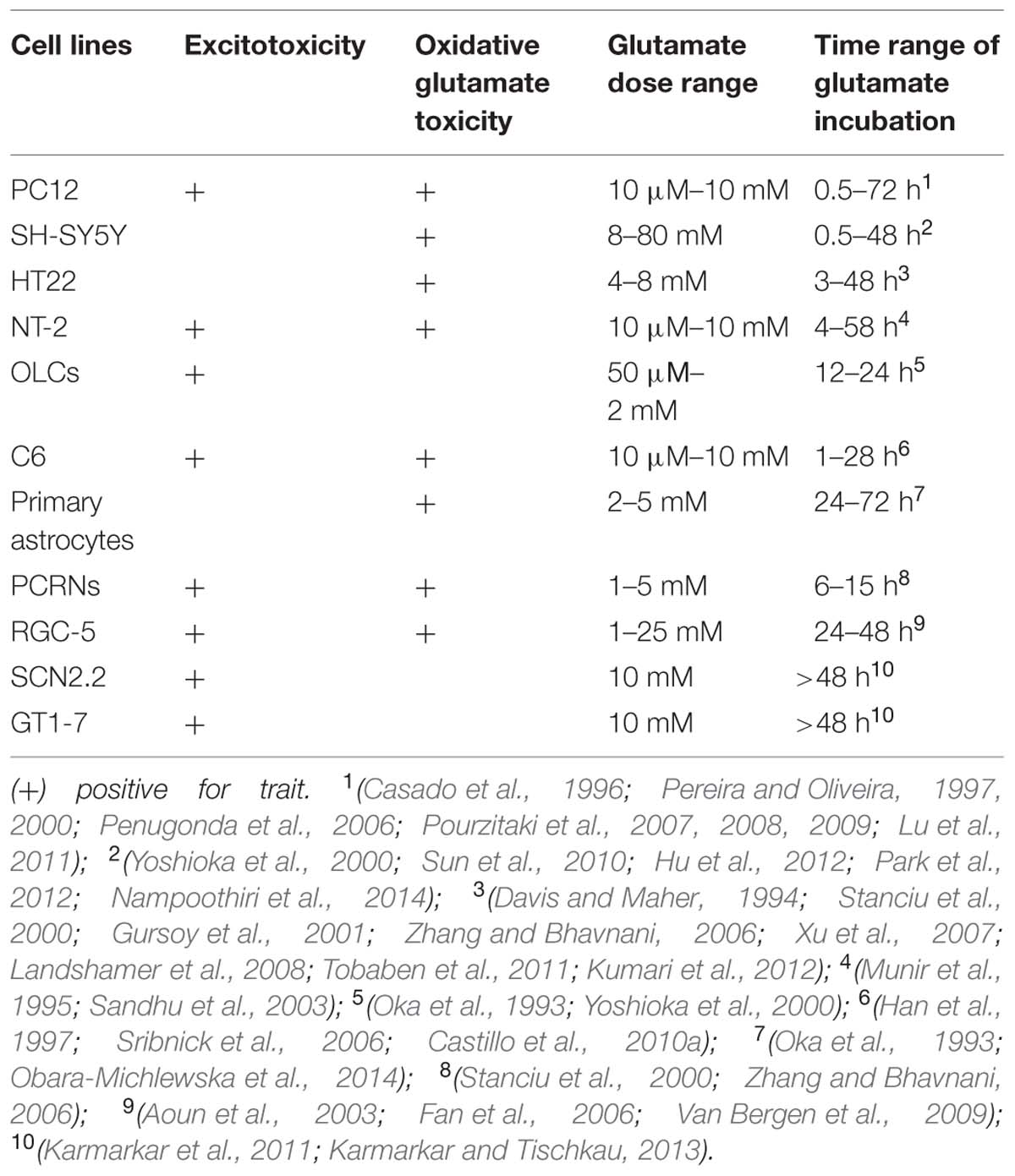
TABLE 1. Reported responses of individual cell lines to excitotoxicity and oxidative glutamate toxicity.
The above two mechanisms of glutamate-mediated toxicity can act cooperatively on PC12 cell death (Ma et al., 2012), while other investigators support that cell death on PC12 is exclusively due to NMDARs over activation (Penugonda et al., 2006; Pourzitaki et al., 2007, 2008, 2009) or inhibition of glu/cys antiporter without NMDAR implication (Pereira and Oliveira, 1997, 2000). We propose that these conflicting reports can be attributed to researchers studying cell death triggered simultaneously by two different events: increased intracellular Ca2+ concentration and GSH depletion. At glutamate concentrations greater than 20 μM the Xc- antiporter begins the reverse CySS transport thus depriving the cells of their ROS inactivation potential (Seib et al., 2011).
In relation to the above, there are reports that excitotoxic cell death in PC12 cells, can be effected by apoptosis and/or necrosis (Bal-Price and Brown, 2000; Lu et al., 2011; Ma et al., 2012), while others support a caspase independent calpain mediated cell death (Roth et al., 2000; Pourzitaki et al., 2007, 2009) probably necroptosis by activation of AIF (Shang et al., 2014). Investigating hypoxia in a PC12 oxygen glucose deprivation model (Kritis et al., 2011), showed that cathepsin D inhibition protects from cell death suggesting the implication of the autophagic processes. This is important in view of excitotoxicity following ischemic insults due to excessive cell death and uncontrolled release of glutamate (Lai et al., 2014).
Neuroprotective mechanisms of PC12 cells include the expression of GRP78, which suppresses oxidative stress and stabilizes Ca2+ homeostasis (Yu et al., 1999). Protein kinase B/Akt exhibits prosurvival and antiapoptotic activities and is involved in growth factor-mediated neuronal protection. Akt deactivation characterizes both caspase-dependent and -independent cell death (Luo et al., 2003). Additionally PI3K/Akt pathway may preferentially regulate both NGF and BDNF-mediated cell survival (Nguyen et al., 2010; Table 2).
Human Neuroblastoma SH-SY5Y Cell Line
Human brain neuroblastoma SH-SY5Y differentiated cell line is derived from bone marrow and is extensively used as a model for the study of oxidative stress pertaining to neuronal death. SH-SY5Y cells exhibit many characteristics of dopaminergic neurons, they have the ability to synthesize dopamine and norepinephrine and they express dopamine transporter. Upon treatment with a variety of agents, including RA (Singh and Kaur, 2009) phorbol ester 12-O-tetradecanoylphorbol-13-acetate (TPA; Pahlman et al., 1981), BDNF (Cernaianu et al., 2008), dibutyryl cAMP (Kume et al., 2008), purine or staurosporine (Mollereau et al., 2007). SH-SY5Y cells are differentiated and exhibit neuronal like characteristics (Cheng et al., 1995; Ismail et al., 2012). This cell line serves as a neuronal model for Parkinson’s disease research (Xie et al., 2010).
Several studies in this cell line have detected the expression of both iCluRs and mGluRs (Naarala et al., 1993; Nair et al., 1996; Sun and Murali, 1998; Naarala et al., 2002; Akundi et al., 2003), while others support that SH-SY5Y cells are deficient in NMDARs or that NMDARs have no function (Cheng et al., 1995; Sun et al., 2010; Xie et al., 2010; Ismail et al., 2012). Sun et al. (2010) support that increased cytoplasmic Ca2+ after glutamate treatment is independent of glutamate receptors (both NMDA and metabotropic) in SH-SY5Y cells. In this cell line glutamate induced cytotoxicity could be mediated by oxidative stress through CySS/glutamate antiporter, depletion of GSH, down regulation of SOD activity leading to apoptosis (oxidative glutamate toxicity; Figure 4 and Table 1).
Others (Fallarini et al., 2009; Beske and Jackson, 2012) use the SH-SY5Y cell line for investigating hypoxia employing protocol of oxygen glucose deprivation (OGD). Experimental evidence suggests that glutamate-induced apoptotic cell death involves the Rac-NADPH oxidase-mediated ROS formation in SH-SY5Y cells (Nikolova et al., 2005; Table 2). One of the downstream targets of NADPH oxidase-derived superoxide radicals is the transcription factor NF-κB which regulates the expression of many genes involved in cell survival and inflammation. NF-κB is also a key factor in regulating NADPH oxidase expression and it is possible that there is a positive feedback loop in which NF-κB activation by oxidative stress leads to further radical production via NADPH oxidase (Nikolova et al., 2005; Anrather et al., 2006).
HT-22 Immortalized Hippocampal Cell Line
HT-22 cell line is an immortalized mouse hippocampal cell line that is extensively used to study the non-receptor mediated oxidative glutamate toxicity (Murphy et al., 1989, 1990; Davis and Maher, 1994). These cells lack iCluRs but are still sensitive to high concentrations of extracellular glutamate (Tables 1 and 2). Glutamate evokes oxidative death in HT22 in a time- and dose-dependent manner involving both necrotic and apoptotic processes (Tan et al., 2001; Fukui et al., 2009; Xu et al., 2010). Recent data shows that glutamate induces mainly necrosis at early time points (before 12 h), but predominantly induces apoptosis at latter ones (12–24 h; Thomas and Huganir, 2004; Fukui et al., 2009). This toxicity is exerted by reduction in GSH production through the CySS/glutamate exchanger (Tan et al., 1998; Zaulyanov et al., 1999; Xu et al., 2007; Grohm et al., 2010; Chen et al., 2011; Tobaben et al., 2011; Poteet et al., 2012; Figure 4). Mitochondrial oxidative stress and dysfunction are important preceding events promoting glutamate induced cell death in HT-22 (Suh et al., 2007; Gliyazova et al., 2013; Shah et al., 2014). Treatment with glutamate (i) alters MMP (ii) induces mitochondrial cytochrome c release (iii) release of mitochondrial AIF, which catalyzes DNA fragmentation and apoptosis. Oxidative stress activates the c-jun N-terminal kinase (JNK) and p38 mitogen activated kinase (Yang et al., 2012) pathways via apoptosis signal regulating kinase-1 (ASK1), leading to apoptosis.
The exact mechanism of glutamate induced excitotoxicity is not clear, and some believe that this process may be mediated through the activation of MAPKs and inhibition of the PI3K/Akt pathway.
In vitro evidence support that glutamate treated HT22 cells, exhibit a delayed and persistent activation of ERKs which contributes to oxidative toxicity (Stanciu et al., 2000). In HT-22 cell line glutamate significantly up regulates the phosphorylation of ERK1/2 while decreasing Erk3 (Gliyazova et al., 2013). Furthermore glutamate treated HT22 increase intracellular Ca2+ levels by means of activation of cobalt-sensitive channels (Finkbeiner and Greenberg, 1996). It is noteworthy that cell death in HT-22 is characterized as necroptosis (Nikoletopoulou et al., 2013). Moreover, increasing evidence suggests that glutamate treated HT22 cells lack caspase activation and glutamate induced cell death proceeds independently of the bcl-2 family proteins so in this cell line glutamate induced apoptosis is mediated via the caspase independent pathway which involves calpain and AIF and is accompanied by DNA ladder formation but not chromatin condensation (Zhang and Bhavnani, 2006). AIF translocation from mitochondria to the nucleus has been identified as the final step of caspase independent mitochondrial death signaling in neurons (Kinney and Slater, 1993; Rahman and Neuman, 1996; Holohean et al., 1999; Culmsee et al., 2005; Landshamer et al., 2008; Poteet et al., 2012).
Human Teratocarcinoma Cell Line – Ntera /D1 (NT-2)
Ntera-2 cell line is derived from a pluripotent embryonal testicular carcinoma. Upon treatment with RA, NT2 precursors are differentiated into NT2N cells, which are identical to neurons, and the majority of them express iCluRs (Perovic et al., 1996; Yoshioka et al., 1997; Paquet-Durand and Bicker, 2004; Paquet-Durand et al., 2006; Podrygajlo et al., 2009). NT2 cells are immunoreactive to the cholinergic markers choline acetyl-transferase, vesicular acetylcholine transporter, and the non-phosphorylated form of neurofilament H, all indicative of motor neurons. The NT2 system may thus be well-suited for research related to motor neuron diseases (Podrygajlo et al., 2009). Additionally NT-2N cells are able to secrete amyloid precursor protein constituting a model cell line for Alzheimer’s disease studies (Di et al., 2010).
Both NMDA and non-NMDA glutamate receptor channels have been identified electrophysiologically and mRNAs for several subtypes of glutamate receptors have been detected (Younkin et al., 1993).NT-2N cells are susceptible to both NMDA and non-NMDA mediated excitotoxicity (Younkin et al., 1993; Munir et al., 1995; Figure 3). High concentrations of glutamate on NT-2N can also inhibit the CySS/glutamate antiporter (Xc-), diminish GSH, thus causing oxidative glutamate toxicity (Munir et al., 1995; Sandhu et al., 2002; Figure 4).
According to Munir et al. (1995), these cells exhibit an excitotoxic response characterized by the absence of NOS and NADPH activity, while Podrygajlo et al. (2009), support that NO is involved in the excitotoxic response of NT-2. Regularly, astrocytes are the main source of NO, however, it has been shown that NT2 neurons can produce NO and therefore increase levels of cGMP (Denninger and Marletta, 1999). In this cell line hypoxia is studied in relation to excitotoxicity and it has been shown that NMDA antagonist (MK801) can offer protection against cell death thus directly connecting hypoxia and excitotoxicity NT2 cells are mainly used to study the neuroprotective potential of glutamate antagonists (Paquet-Durand et al., 2006).
Cell death in NT-2 cell does not seem to be effected by means of caspase 3 activation alone, since addition of the NMDA antagonist MK801 results in synergistic protection (Hanko et al., 2006; Table 2).
Oligodendroglial Lineage Cells
Oligodendroglial lineage cells are derived from CG-4-immortalized rat O-2A progenitor cells and are established as an in vitro model for oligodendroglial cell studies. Failure of MK-801, to attenuate kainate-induced cell damage under excitotoxic conditions has led to the conclusion that OLCs do not express NMDARs (Yoshioka et al., 1995) and that excitotoxicity in oligodendrocytes is mediated through non-NMDA glutamatergic receptors (Yoshioka et al., 1995, 2000; Yamaya et al., 2002; Itoh et al., 2003). In vitro evidence support that although both CG-4 and non-immortalized rat OLCs transcribe the NMDA GluR subunit genes NMDAR1 and NMDAR2D they do not translate NMDAR1 GluR protein (Peterson and Moore, 1980).
Excitotoxic stimuli can damage oligodendroglial cells by means of Ca2+ entry which leads to Ca2+ dyshomeostasis and mitochondrial membrane alterations. These changes can lead to release of cytochrome c and AIF (Susin et al., 1999) exhibiting a proapoptotic action. Death of oligodentroglial cells can be either caspase-depended or caspase- independent (Alberdi et al., 2002; Sanchez-Gomez et al., 2003; Ness et al., 2004). The molecular events observed after glutamate excitotoxicity are shown in Figure 3. Excitotoxic stimuli results in increased production of ROS, depolarization of the mitochondrial membrane and release of caspase-activating proapoptotic factors. Whether cell demise is mediated via necrosis or apoptosis, it is determined through the relative contribution of the above events (Ankarcrona et al., 1995; Sanchez-Gomez et al., 2003). Consequently, cells die via apoptosis or necrosis, as is observed in excitotoxic models (Bonfoco et al., 1995). Overexpression of Bcl2 and Bcl-xl can prevent cell death in cases of mild excitotoxic insults, whereas other members of this family such as Bad or Bax have a proapoptotic role and are involved in excitotoxicity induced mitochondrial dysfunction (Xiang et al., 1998; Table 2).
C6 Cell Line
Although it has been shown that C6 rat glioma cells express iGluRs (Singh and Kaur, 2009; Tsuchioka et al., 2011; Veenman et al., 2012), it seems that excitotoxicity in this cell line is mediated by mGluRs (Albasanz et al., 1997; Viwatpinyo and Chongthammakun, 2009; Castillo et al., 2010a,b; Piers et al., 2012; Sun et al., 2012). Concurrently studied C6 and PRCNs cells, showed that in PCRNs cells mGluRs levels are down regulated and the death rate is increased, after treatment with glutamate, compared to C6 cells which were resistant to excitotoxicity, and after 24/48 h the levels of mGluRs were increased. GSH depletion seems to be responsible for the development of oxidative stress in C6 cells (Han et al., 1997; Sun et al., 2012; Figure 4).
Zhu et al. (2012) showed, that oxidized extracellular Cys/CySS (CySS) redox state in C6 glial cells, induced a significant increase in mGluR5-mediated phosphorylation of ERK kinases. Cys/CySS redox could be blocked by U0126 an inhibitor of MEK/MAPK and a specific mGluR5 antagonist, 2-methyl-6-(phenylethynyl) pyridine (MPEP). Activation of mGluR5 by oxidized extracellular Cys/CySS affected the expression of NF-κB and inducible iNOS (Zhu et al., 2012).
Moreover A1-mediated adenylyl cyclase inhibition and A2A-mediated adenylyl cyclase stimulation were, respectively, increased and decreased after glutamate exposure (Castillo et al., 2010b).
Neuroprotective responses activated due to loss of neural network and cell death were, the increased expression of the stress protein HSP70 (Du et al., 2012), chaperone GRP78 (Suyama et al., 2011), and of BDNF (Viwatpinyo and Chongthammakun, 2009; Hirata et al., 2012).
Primary Rat Astrocytes
Primary rat astrocytes are widely used to investigate the protective effect of glial cells against excitotoxic neuronal trauma, specifically via astrocytic glutamate transporters GLT-1 (EAAT2) and GLAST (EAAT1), which are the main glutamate transporters in the CNS, taking up most of excess glutamate from the synaptic cleft to prevent excitotoxic neuronal death (Lu et al., 2008; Fang et al., 2012; Lane and Lawen, 2013; Foran et al., 2014; Karki et al., 2014; Obara-Michlewska et al., 2014; Sulkowski et al., 2014). Although astrocytes appear to be capable of expressing all five of the known EAAT isoforms (i.e., termed EAATs 1–5) in humans, the rodent glutamate aspartate transporter GLAST; ortholog of human EAAT1, and the glutamate transporter 1 GLT-1; ortholog of human EAAT2, are thought to be the predominantly expressed glutamate transporters in rodent astrocytes (Lane and Lawen, 2013; Karki et al., 2014) in their effort to prove that raloxifene (RX) upregulates glutamate transporters in rat primary astrocytes, found that this happens through the activation of multiple signaling pathways including ERK, EGFR, and CREB mediated by estrogen receptors (ERs) ER-a, ER-b, and GPR30. At the transcriptional level, NF-κB played a critical role in RX-induced GLT-1 expression, while TNF-a reduced GLT-1 promoter activity, mRNA and protein levels in primary astrocytes.
Ionotropic glutamate receptor or mGluRs are known to be expressed in cultured astrocytes. Although kainate receptor activation was not directly assessed, clear evidence for a functional expression of these iGluRs in astrocytes is lacking. Groups II and III mGluRs do not appear to be expressed by rodent astrocytes under standard culture conditions (Lane and Lawen, 2013).
Furthermore, potassium channels Kir4.1 abundantly expressed in astrocytes contribute to K+ spatial buffering, a fundamental mechanism in maintaining neuronal excitability and synaptic transmission and are implicated in the regulation of cell volume. Down regulation of Kir4.1 channels expression has been reported to decrease glutamate (Glu) uptake in cultured astrocytes. (Obara-Michlewska et al., 2014) have shown that over-activation of astroglial NMDA receptors, is a primary cause of the reduction of Kir4.1 expression in CNS disorders associated with increased exposure to Glu, giving a new insight to the excitotoxic contribution of NMDARs.
Primary Cortical Rat Neurons
Despite of all the disadvantages pertaining to their culture, PRCNs are widely used for excitotoxicity experiments, because they present all the characteristics of living neurons activated by iGluRs agonists (Chen et al., 2013). Additionally the activation of NMDARs was verified after incubation with NMDA (Antonelli et al., 2004; Smialowska et al., 2012; Voulgari-Kokota et al., 2012; Yang et al., 2012).
Kim et al. (2012) in an effort to prove the anti-inflammatory effect of Vitis amurensis found out that excitotoxicity was induced not only by NMDARs but also due to the depletion of GSH levels and lipid peroxidation. MAPKs, cyclooxygenase-2 (COX-2), and pro-apoptotic proteins were also found to be active in these neurons.
Yang et al. (2012) supported the involvement of MAPK pathways in NMDAR-induced apoptosis of rat cortical neurons. Current studies show that treatment of cortical neurons with glutamate resulted in an increase in Bax with a decrease in Bcl-2 proteins, loss of the MMP followed by a release of cytochrome c, and activation of caspase-9, representing the classical mitochondrial apoptotic pathway (Figure 3).
Concerning neuroprotection, adenosine A2A receptor subtype stimulation induced the activation of Akt-GSK-3b signaling pathway. The blockade of this signaling pathway with specific inhibitors abolished the increase of BDNF production, possibly via modulation of ERK1/2- CREB pathway. The physiological roles of A2A receptor-induced BDNF production was demonstrated by the protection of neurons from the excitotoxicity and increased neurite extension, as well as synapse formation from immature and mature neurons. Taken together, activation of A2A receptor regulates BDNF production in rat cortical neurons, which provides a neuroprotective action (Jeon et al., 2011; Table 1).
RGC-5 (Retinal Ganglion Cells)
Retinal ganglion cell (RGC-5) line is used widely in glaucoma research. This cell line simulates retinal ganglion cells as it is designated positive for certain characteristics of retinal ganglia, including Thy-1 and Brn-3C expression, and for sensitivity to glutamate excitotoxicity upon neurotrophin withdrawal. RGC-5 cell line has been widely used to study the Xc- antiporter because retina is extremely vulnerable to oxidative stress (Figure 4). It must be noted that there is controversy concerning the origin of the RGC-5 cell line. Van Bergen et al. (2009) have shown utilizing mitochondrial and nuclear DNA analysis that the cell line is of mouse origin (Mus musculus) and not of rat origin as originally was thought. Furthermore, recent evidence has surfaced from Krishnamoorthy et al. (2013) which point out that the RGC-5 cell line may have been mischaracterized and actually be is cell line 661W, a mouse SV-40 T antigen transformed photoreceptor cell line. Thus, caution is advised in drawing conclusions from data extrapolated from experiments using RGC-5 cell line as well as using the aforementioned cell line as a retinal ganglion cell line.
Retinal ganglion cells express σ1-receptor, which is believed to induce neuroprotection against excitotoxicity. Hayashi and Su have shown that σ1 receptors are localized both in the ER and on the plasma membrane in many organs including the eye. The receptor is mainly localized in ER, with two transmembrane regions that have the ability to translocate to the plasma membrane upon agonist stimulation or under stressful conditions. This translocation probably gives σ1 receptor the ability to reach plasma membrane and regulate membrane channels including voltage-gated and ligand-gated Ca2+, K+, Na+, Cl-, and SK ion channels (Hayashi and Su, 2003; Tchedre and Yorio, 2008). In vitro exposure of cultured rat brain neurons to selective σ-receptor ligands protects cells against glutamate or NMDA exposure (Lesage et al., 1995). Both excitotoxicity and oxidative glutamate toxicity have been proposed as possible mechanisms of RGC injury and cell death (Almasieh et al., 2012).
Moreover, in vitro glutamate treatment increased BDNF mRNA and protein expression and also caused a release of BDNF in the culture media (Jeon et al., 2011). NF-κB activation was observed in response to glutamate treatment and it is postulated that increased BDNF expression is stimulated through NF-κB activation (Fan et al., 2006). Also CaMKII inhibitor, AIP, may play a neuroprotective role by enhancing the release of BDNF in glutamate treated RGC-5 cells. The BDNF/TrkB signaling pathway plays a pivotal role in RGC survival (Dahlmann-Noor et al., 2010; Almasieh et al., 2012). Decline in the BDNF/TrkB signaling is an important observation in the RGCs in glaucoma disease (Gupta et al., 2013). Over activation of glutamate receptors and the resulting Ca2+ overload stimulates calpains which target cytoskeletal proteins, kinases and phosphatases, membrane receptors and transporters (Xu et al., 2009; Figure 3). Concerning the TrkB receptors, it is well-established that the trkB gene encodes a full length receptor tyrosine kinase (TrkB.FL; Tsuchioka et al., 2011). Under excitotoxic conditions calpain mediates TrkB.FL cleavage results in up regulation of the truncated TrkB isoform (TrkB.T). This is pivotal for cell survival since it results in the inactivation of RhoA-GTPase and downstream inhibition of pro-death pathways of p38/MAPK and JNK/c-Jun signaling. BDNF stimulation of TrkB receptors results in the activation of intracellular signaling pathways of Akt and the MAPKs extracellular signal-regulated kinases 1 and 2 (ERK 1/2), thus promoting cell survival (Klocker et al., 2000; Cheng et al., 2002).
SCN2.2 Cell Line
The hypothalamic SCN is a brain region that controls circadian rhythms and is endogenously resistant to excitotoxicity in vivo (Ebling, 1996). This in vivo resistance was first established in the 1980s (Peterson et al., 1989; Reynolds and Hastings, 1995). In vitro studies of the ability of these cells to resist the toxic effects of glutamate would probably be the first step for excitotoxicity treatment.
It has been demonstrated that SCN2.2 (immortalized SCN cell line derived from rat SCN) retains resistance to glutamate toxicity under conditions that are toxic to other immortalized cell lines. This ability may provide insights into signaling pathways that offer endogenous neuroprotection in SCN. SCN2.2 cells retain the ability to respond to glutamate since in vitro studies have proved the presence of functional NMDAR subunits NR2A, NR2B, and NR2D. This fact enforces the perception that lack of excitotoxicity in SCN2.2 is not because of incapability to respond to the glutamate stimuli (Bottum et al., 2010). Bottum et al. (2010), supported that excitotoxic resistance in SCN2.2 is an endogenous ability and is not due to glutamate uptake by glia. In addition, experimental evidence support that SCN2.2 have higher levels of AkT activity than other cell lines (Marchetti et al., 2004). Activation of Akt pathway (PKB/Akt/PI3) is correlated with neuroprotection since Akt pathway stimulates the expression of many neuroprotective factors such as: estrogens, brain-derived neurotrophic factor (Jeon et al., 2011), insulin-like growth factor and tumor necrosis growth factor (Marchetti et al., 2004; Johnson-Farley et al., 2007; De et al., 2008; Bourque et al., 2009).
In vitro, SCN2.2 excitotoxic resistance was evaluated in comparison with GT1-7 (a hypothalamic cell line derived from embryonic GnRH) which served as a neuronal control and has been widely used in neurotoxicity studies (Mellon et al., 1990; Bonfoco et al., 1996; Karmarkar et al., 2011). Glutamate treated SCN2.2 and GT1-7 (10 mmol/L) resulted in activation of different signaling pathways. SCN2.2 exposure to glutamate resulted in activation of the anti-apoptotic ERK/MAPK pathway without affecting the pro-apoptotic p38/MAPK pathway, whereas in GT1-7 was noted an increase in p38/MAPK pathway and decrease in ERK/MAPK pathway (Karmarkar et al., 2011; Table 1).
Moreover in GT1-7 cells glutamate treatment resulted in increased levels of caspase-3 and BID protein, with subsequent DNA damage and cell death, while in SCN2.2 glutamate didn’t affect caspase-3 activity. Increased caspase 3 activity and cell death in SCN2.2 was noticed only after pretreatment with PD98059 (inhibitor of ERK/MAPK) which resulted in NMDAR-mediated death via an apoptotic pathway (Karmarkar et al., 2011).These results support the connection between activation of ERK/MAPK pathway and cell survival (Villalba et al., 1997; Persons et al., 1999; Yazlovitskaya et al., 1999). More specifically, activation of ERK/MAPK pathway stimulates the release of molecules such as (a) CREB (Cao et al., 2008) and (b) mammalian target of rapamycin (Hay and Sonenberg, 2004). The activation of the above intracellular molecules through ERK/MAPK pathway plays a key role to cell survival. It is worth mentioning that treatment with BDNF also up regulates ERK/MAPK pathway (Hetman et al., 1999; Han and Holtzman, 2000; Hetman and Kharebava, 2006).
Conclusion
From the information presented above becomes evident that excitotoxicity is a multifactorial and complex phenomenon. Depending on the extracellular glutamate concentration (below 20 μM) the glutamate receptors alone come into play increasing the intracellular Ca2+ concentration. In higher extracellular glutamate concentrations, besides the action of glutamate receptors, the antiporter XC- exchanges intracellular CySS for glutamate, thus eventually depleting the cells of their GSH reducing potential. In addition in certain conditions the reverse action of glutamate transporters (EAATs) contribute to increased extracellular glutamate concentration.
Different cell lines differ in their responses when exposed to glutamate. In some cell lines glutamate toxicity is exerted through over activation of NMDA, AMPA, or kainate receptors whereas in other cell lines lacking such receptors, the toxicity is due to glutamate induced oxidative stress (Table 2). Another point worth mentioning is the differentiation of temporal characteristics of the signaling cascades in different cell lines, such as time and extent of activation, that play a pivotal role in promoting either cell survival or cell death. Different researchers used different exposure times and varying concentrations of glutamate to study excitotoxicity in their cell model systems.
The evolving understanding of cell death mechanisms also contributed in part to the hazy picture of excitotoxic cell death. Today, we understand many types of cell death, necrosis and programmed cell death. Programmed cell death can be caspase dependent (apoptosis), caspase independent calpain dependent (necroptosis or apoptosis like programmed cell death) and caspase independent cathepsin dependent (autophagic cell death; Nikoletopoulou et al., 2013). The study of excitotoxicity should take into consideration these facts. We recognized the need of a well-defined system that can distinguish excitotoxicity from oxidative glutamate toxicity and take into account our current view of necrosis or programmed cell death in order to decipher the response of the nerve cell to increased extracellular glutamate concentration.
The response of the cells under such conditions can be addressed by a concerted proteomics approach in order to assess the end result of the activation of the multiple intracellular signaling cascades initiated by the excitotoxic insult. It is also of great interest to examine the kinetics of the key pathways involved in order to assess their implication in cell survival or cell death under the stimulus of glutamate toxicity.
Herein are collected and presented the cell models, used to study excitotoxicity and the findings reported so far with respect to the defense and cell death mechanisms, elicited by elevated extracellular glutamate concentration.
Author Contributions
AK contributed to the conception of the theme-axis of this review and to the interpretation of the collected information. ES contributed to the conception of the work and also to analysis and interpretation of the information TV contributed also to the acquisition and analysis. KP contributed to the collection and analysis of the data. All the aforementioned authors cooperated for the drafting of the review, its final approval, and agreed to be accountable for all aspects of the work in ensuring that questions related to the accuracy or integrity of any part of the work are appropriately investigated and resolved.
Abbreviations
AIF, apoptosis inducing factor; AMPAR, α-amino-3-hydroxy-5-methyl-4-isoxazolepropionic acid receptor; BDNF, brain derived neuron factor; BID, BH3 interacting domain death agonist; cAMP, cyclic adenosine monophosphate; CNS, central nervous system; CREB, cAMP response element binding protein; Cys, cysteine; CySS, cystine; EAAT, excitatory amino-acid transporter; ER, endoplasmic reticulum; GAPDH, glyceraldehyde 3-phosphate dehydrogenase; GSH, glutathione; iGluR, ionotropic glutamate receptor; KARs, kainic acid receptors; MAPK, mitogen-activated protein kinase; mGluRs, metabotropic glutamate receptors; NADPH, nicotinamide adenine dinucleotide phosphate; NGF, nerve growth factor; NMDAR, N-methyl-D-aspartate receptor; NOS, nitric oxide synthase; PKC, protein kinase C; PLC, phospholipase C; PRCNs, primary rat cortical neurons; RA, retinoic acid; Src, proto-oncogene tyrosine-protein kinase; VDCC, voltage-dependent calcium channel; XC-, glutamate/cystine antiporter.
Conflict of Interest Statement
The authors declare that the research was conducted in the absence of any commercial or financial relationships that could be construed as a potential conflict of interest.
References
Ahern, G. P., Klyachko, V. A., and Jackson, M. B. (2002). cGMP and S-nitrosylation: two routes for modulation of neuronal excitability by NO. Trends Neurosci. 25, 510–517. doi: 10.1016/S0166-2236(02)02254-3
PubMed Abstract | Full Text | CrossRef Full Text | Google Scholar
Akundi, R. S., Hüll, M., Clement, H. W., and Fiebich, B. L. (2003). 1-trichloromethyl-1,2,3,4-tetrahydro-beta-carboline (TaClo) induces apoptosis in human neuroblastoma cell lines. Ann. N. Y. Acad. Sci. 1010, 304–306. doi: 10.1196/annals.1299.053
Albasanz, J. L., Ros, M., and Martin, M. (1997). Characterization of metabotropic glutamate receptors in rat C6 glioma cells. Eur. J. Pharmacol. 326, 85–91. doi: 10.1016/S0014-2999(97)00154-4
PubMed Abstract | Full Text | CrossRef Full Text | Google Scholar
Alberdi, E., Sánchez-Gómez, M. V., Marino, A., and Matute, C. (2002). Ca(2+) influx through AMPA or kainate receptors alone is sufficient to initiate excitotoxicity in cultured oligodendrocytes. Neurobiol. Dis. 9, 234–243. doi: 10.1006/nbdi.2001.0457
PubMed Abstract | Full Text | CrossRef Full Text | Google Scholar
Ali, D. W., and Salter, M. W. (2001). NMDA receptor regulation by Src kinase signalling in excitatory synaptic transmission and plasticity. Curr. Opin. Neurobiol. 11, 336–342. doi: 10.1016/S0959-4388(00)00216-6
PubMed Abstract | Full Text | CrossRef Full Text | Google Scholar
Allen, J. W., Ivanova, S. A., Fan, L., Espey, M. G., Basile, A. S., and Faden, A. I. (1999). Group II metabotropic glutamate receptor activation attenuates traumatic neuronal injury and improves neurological recovery after traumatic brain injury. J. Pharmacol. Exp. Ther. 290, 112–120.
Almasieh, M., Wilson, A. M., Morquette, B., Cueva Vargas, J. L., and Di, P. A. (2012). The molecular basis of retinal ganglion cell death in glaucoma. Prog. Retin. Eye Res. 31, 152–181. doi: 10.1016/j.preteyeres.2011.11.002
PubMed Abstract | Full Text | CrossRef Full Text | Google Scholar
Ambrosini, A., Bresciani, L., Fracchia, S., Brunello, N., and Racagni, G. (1995). Metabotropic glutamate receptors negatively coupled to adenylate cyclase inhibit N-methyl-D-aspartate receptor activity and prevent neurotoxicity in mesencephalic neurons in vitro. Mol. Pharmacol. 47, 1057–1064.
Anderson, C. M., and Swanson, R. A. (2000). Astrocyte glutamate transport: review of properties, regulation, and physiological functions. Glia 32, 1–14. doi: 10.1002/1098-1136(200010)32:1<1::AID-GLIA10>3.0.CO;2-W
PubMed Abstract | Full Text | CrossRef Full Text | Google Scholar
Ankarcrona, M., Dypbukt, J. M., Bonfoco, E., Zhivotovsky, B., Orrenius, S., Lipton, S. A.,et al. (1995). Glutamate-induced neuronal death: a succession of necrosis or apoptosis depending on mitochondrial function. Neuron 15, 961–973. doi: 10.1016/0896-6273(95)90186-8
Anrather, J., Racchumi, G., and Iadecola, C. (2006). NF-kappaB regulates phagocytic NADPH oxidase by inducing the expression of gp91phox. J. Biol. Chem. 281, 5657–5667. doi: 10.1074/jbc.M506172200
PubMed Abstract | Full Text | CrossRef Full Text | Google Scholar
Antonelli, T., Ferraro, L., Fuxe, K., Finetti, S., Fournier, J., Tanganelli, S.,et al. (2004). Neurotensin enhances endogenous extracellular glutamate levels in primary cultures of rat cortical neurons: involvement of neurotensin receptor in NMDA induced excitotoxicity. Cereb. cortex 14, 466–473. doi: 10.1093/cercor/bhh008
PubMed Abstract | Full Text | CrossRef Full Text | Google Scholar
Aoun, P., Simpkins, J. W., and Agarwal, N. (2003). Role of PPAR-gamma ligands in neuroprotection against glutamate-induced cytotoxicity in retinal ganglion cells. Invest. Ophthalmol. Vis. Sci. 44, 2999–3004. doi: 10.1167/iovs.02-1060
PubMed Abstract | Full Text | CrossRef Full Text | Google Scholar
Bal-Price, A., and Brown, G. C. (2000). Nitric-oxide-induced necrosis and apoptosis in PC12 cells mediated by mitochondria. J. Neurochem. 75, 1455–1464. doi: 10.1046/j.1471-4159.2000.0751455.x
PubMed Abstract | Full Text | CrossRef Full Text | Google Scholar
Beske, P. H., and Jackson, D. A. (2012). NADPH oxidase mediates the oxygen-glucose deprivation/reperfusion-induced increase in the tyrosine phosphorylation of the N-methyl-D-aspartate receptor NR2A subunit in retinoic acid differentiated SH-SY5Y Cells. J. Mol. Signal. 7:15. doi: 10.1186/1750-2187-7-15
PubMed Abstract | Full Text | CrossRef Full Text | Google Scholar
Bonfoco, E., Krainc, D., Ankarcrona, M., Nicotera, P., and Lipton, S. A. (1995). Apoptosis and necrosis: two distinct events induced, respectively, by mild and intense insults with N-methyl-D-aspartate or nitric oxide/superoxide in cortical cell cultures. Proc. Natl. Acad. Sci. U.S.A. 92, 7162–7166. doi: 10.1073/pnas.92.16.7162
Bonfoco, E., Zhivotovsky, B., Rossi, A. D., Aguilar-Santelises, M., Orrenius, S., Lipton, S. A.,et al. (1996). BCL-2 delay apoptosis and PARP cleavage induced by NO donors in GT1-7 cells. Neuroreport 8, 273–276. doi: 10.1097/00001756-199612200-00054
PubMed Abstract | Full Text | CrossRef Full Text | Google Scholar
Bottum, K., Poon, E., Haley, B., Karmarkar, S., and Tischkau, S. A. (2010). Suprachiasmatic nucleus neurons display endogenous resistance to excitotoxicity. Exp. Biol. Med. (Maywood) 235, 237–246. doi: 10.1258/ebm.2009.009244
PubMed Abstract | Full Text | CrossRef Full Text | Google Scholar
Bourque, M., Dluzen, D. E., and Di, P. T. (2009). Neuroprotective actions of sex steroids in Parkinson’s disease. Front. Neuroendocrinol. 30:142–157. doi: 10.1016/j.yfrne.2009.04.014
PubMed Abstract | Full Text | CrossRef Full Text | Google Scholar
Boyce, M., and Yuan, J. (2006). Cellular response to endoplasmic reticulum stress: a matter of life or death. Cell Death Differ. 13, 363–373. doi: 10.1038/sj.cdd.4401817
PubMed Abstract | Full Text | CrossRef Full Text | Google Scholar
Bridges, R. J., Natale, N. R., and Patel, S. A. (2012). System xc(-) cystine/glutamate antiporter: an update on molecular pharmacology and roles within the CNS. Br. J. Pharmacol. 165, 20–34. doi: 10.1111/j.1476-5381.2011.01480.x
PubMed Abstract | Full Text | CrossRef Full Text | Google Scholar
Brown, G. C. (2010). Nitric oxide and neuronal death. Nitric Oxide 23, 153–165. doi: 10.1016/j.niox.2010.06.001
PubMed Abstract | Full Text | CrossRef Full Text | Google Scholar
Byrnes, K. R., Loane, D. J., and Faden, A. I. (2009). Metabotropic glutamate receptors as targets for multipotential treatment of neurological disorders. Neurotherapeutics 6, 94–107. doi: 10.1016/j.nurt.2008.10.038
PubMed Abstract | Full Text | CrossRef Full Text | Google Scholar
Cao, R., Lee, B., Cho, H. Y., Saklayen, S., and Obrietan, K. (2008). Photic regulation of the mTOR signaling pathway in the suprachiasmatic circadian clock. Mol. Cell. Neurosci. 38, 312–324. doi: 10.1016/j.mcn.2008.03.005
Casado, M., Lopez-Guajardo, A., Mellstrom, B., Naranjo, J. R., and Lerma, J. (1996). Functional N-methyl-D-aspartate receptors in clonal rat phaeochromocytoma cells. J. Physiol. 490, 391–404. doi: 10.1113/jphysiol.1996.sp021153
Castillo, C. A., Leon, D. A., Ballesteros-Yanez, I., Iglesias, I., Martin, M., and Albasanz, J. L. (2010a). Glutamate differently modulates metabotropic glutamate receptors in neuronal and glial cells. Neurochem. Res. 35, 1050–1063. doi: 10.1007/s11064-010-0154-y
PubMed Abstract | Full Text | CrossRef Full Text | Google Scholar
Castillo, C. A., León, D. A., Ballesteros-Yáñez, I., Albasanz, J. L., and Martín, M. (2010b). Glutamate differently modulates excitatory and inhibitory adenosine receptors in neuronal and glial cells. Neurochem. Int. 57, 33–42. doi: 10.1016/j.neuint.2010.04.008
PubMed Abstract | Full Text | CrossRef Full Text | Google Scholar
Cernaianu, G., Brandmaier, P., Scholz, G., Ackermann, O. P., Alt, R., Rothe, K.,et al. (2008). All-trans retinoic acid arrests neuroblastoma cells in a dormant state. Subsequent nerve growth factor/brain-derived neurotrophic factor treatment adds modest benefit. J. pediatr. Surg. 43, 1284–1294. doi: 10.1016/j.jpedsurg.2008.01.007
PubMed Abstract | Full Text | CrossRef Full Text | Google Scholar
Chen, J., Chua, K. W., Chua, C. C., Yu, H., Pei, A., Chua, B. H.,et al. (2011). Antioxidant activity of 7,8-dihydroxyflavone provides neuroprotection against glutamate-induced toxicity. Neurosci. Lett. 499, 181–185. doi: 10.1016/j.neulet.2011.05.054
PubMed Abstract | Full Text | CrossRef Full Text | Google Scholar
Chen, M. J., Ng, J. M., Peng, Z. F., Manikandan, J., Yap, Y. W., Llanos, R. M.,et al. (2013). Gene profiling identifies commonalities in neuronal pathways in excitotoxicity: evidence favouring cell cycle re-activation in concert with oxidative stress. Neurochem. Int. 62, 719–730. doi: 10.1016/j.neuint.2012.12.015
PubMed Abstract | Full Text | CrossRef Full Text | Google Scholar
Cheng, B., Martinez, A. A., Morado, J., Scofield, V., Roberts, J. L., and Maffi, S. K. (2012). Retinoic acid protects against proteasome inhibition associated cell death in SH-SY5Y cells via the AKT pathway. Neurochem. Int. 62, 31–42. doi: 10.1016/j.neuint.2012.10.014
PubMed Abstract | Full Text | CrossRef Full Text | Google Scholar
Cheng, J., Standifer, K. M., Tublin, P. R., Su, W., and Pasternak, G. W. (1995). Demonstration of kappa 3-opioid receptors in the SH-SY5Y human neuroblastoma cell line. J. Neurochem. 65, 170–175. doi: 10.1046/j.1471-4159.1995.65010170.x
Cheng, L., Sapieha, P., Kittlerova, P., Hauswirth, W. W., and Di, P. A. (2002). TrkB gene transfer protects retinal ganglion cells from axotomy-induced death in vivo. J. Neurosci. 22, 3977–3986.
Conn, P. J., and Pin, J. P. (1997). Pharmacology and functions of metabotropic glutamate receptors. Annu. Rev. Pharmacol. Toxicol. 37, 205–237. doi: 10.1146/annurev.pharmtox.37.1.205
Culmsee, C., Zhu, C., Landshamer, S., Becattini, B., Wagner, E., Pellecchia, M.,et al. (2005). Apoptosis-inducing factor triggered by poly(ADP-ribose) polymerase and bid mediates neuronal cell death after oxygen-glucose deprivation and focal cerebral ischemia. J. Neurosci. 25, 10262–10272. doi: 10.1523/JNEUROSCI.2818-05.2005
PubMed Abstract | Full Text | CrossRef Full Text | Google Scholar
Dahlmann-Noor, A. H., Vijay, S., Limb, G. A., and Khaw, P. T. (2010). Strategies for optic nerve rescue and regeneration in glaucoma and other optic neuropathies. Drug Discov. Today 15, 287–299. doi: 10.1016/j.drudis.2010.02.007
PubMed Abstract | Full Text | CrossRef Full Text | Google Scholar
Daikhin, Y., and Yudkoff, M. (2000). Compartmentation of brain glutamate metabolism in neurons and glia. J. Nutr. 130, 1026S–1031S.
Danbolt, N. C. (2001). Glutamate uptake. Prog. Neurobiol. 65, 1–105. doi: 10.1016/S0301-0082(00)00067-8
Davis, J. B., and Maher, P. (1994). Protein kinase C activation inhibits glutamate-induced cytotoxicity in a neuronal cell line. Brain Res. 652, 169–173. doi: 10.1016/0006-8993(94)90334-4
PubMed Abstract | Full Text | CrossRef Full Text | Google Scholar
De, P. C., Falcone, S., Panzeri, C., Radice, S., Bassi, M. T., and Clementi, E. (2008). Endothelial nitric oxide synthase overexpression by neuronal cells in neurodegeneration: a link between inflammation and neuroprotection. J. Neurochem. 106, 193–204. doi: 10.1111/j.1471-4159.2008.05351.x
PubMed Abstract | Full Text | CrossRef Full Text | Google Scholar
Denninger, J. W., and Marletta, M. A. (1999). Guanylate cyclase and the.NO/cGMP signaling pathway. Biochim. Biophys. Acta 1411, 334–350. doi: 10.1016/S0005-2728(99)00024-9
Di, X., Yan, J., Zhao, Y., Zhang, J., Shi, Z., Chang, Y.,et al. (2010). L-theanine protects the APP (Swedish mutation) transgenic SH-SY5Y cell against glutamate-induced excitotoxicity via inhibition of the NMDA receptor pathway. Neuroscience 168, 778–786. doi: 10.1016/j.neuroscience.2010.04.019
PubMed Abstract | Full Text | CrossRef Full Text | Google Scholar
Dikic, I., Tokiwa, G., Lev, S., Courtneidge, S. A., and Schlessinger, J. (1996). A role for Pyk2 and Src in linking G-protein-coupled receptors with MAP kinase activation. Nature 383, 547–550. doi: 10.1038/383547a0
PubMed Abstract | Full Text | CrossRef Full Text | Google Scholar
Doshi, S., and Lynch, D. R. (2009). Calpain and the glutamatergic synapse. Front. Biosci. (Schol. Ed.) 1:466–476.
Du, P., Hu, S., Cheng, Y., Li, F., Li, M., Li, J.,et al. (2012). Photodynamic therapy leads to death of C6 glioma cells partly through AMPAR. Brain Res. 1433, 153–159. doi: 10.1016/j.brainres.2011.11.048
PubMed Abstract | Full Text | CrossRef Full Text | Google Scholar
Ebling, F. J. (1996). The role of glutamate in the photic regulation of the suprachiasmatic nucleus. Prog. Neurobiol. 50, 109–132. doi: 10.1016/S0301-0082(96)00032-9
Edwards, M. A., Loxley, R. A., Williams, A. J., Connor, M., and Phillips, J. K. (2007). Lack of functional expression of NMDA receptors in PC12 cells. Neurotoxicology 28, 876–885. doi: 10.1016/j.neuro.2007.04.006
PubMed Abstract | Full Text | CrossRef Full Text | Google Scholar
Faden, A. I., Ivanova, S. A., Yakovlev, A. G., and Mukhin, A. G. (1997). Neuroprotective effects of group III mGluR in traumatic neuronal injury. J. Neurotrauma 14, 885–895. doi: 10.1089/neu.1997.14.885
Fallarini, S., Miglio, G., Paoletti, T., Minassi, A., Amoruso, A., Bardelli, C.,et al. (2009). Clovamide and rosmarinic acid induce neuroprotective effects in in vitro models of neuronal death. Br. J. Pharmacol. 157, 1072–1084. doi: 10.1111/j.1476-5381.2009.00213.x
PubMed Abstract | Full Text | CrossRef Full Text | Google Scholar
Fan, W., Agarwal, N., and Cooper, N. G. (2006). The role of CaMKII in BDNF-mediated neuroprotection of retinal ganglion cells (RGC-5). Brain Res. 1067, 48–57. doi: 10.1016/j.brainres.2005.10.030
PubMed Abstract | Full Text | CrossRef Full Text | Google Scholar
Fang, J., Han, D., Hong, J., Tan, Q., and Tian, Y. (2012). The chemokine, macrophage inflammatory protein-2gamma, reduces the expression of glutamate transporter-1 on astrocytes and increases neuronal sensitivity to glutamate excitotoxicity. J. Neuroinflammation 9:267. doi: 10.1186/1742-2094-9-267
Finkbeiner, S., and Greenberg, M. E. (1996). Ca(2+)-dependent routes to ras: mechanisms for neuronal survival, differentiation, and plasticity? Neuron 16, 233–236. doi: 10.1016/S0896-6273(00)80040-9
Foran, E., Rosenblum, L., Bogush, A., Pasinelli, P., and Trotti, D. (2014). Sumoylation of the astroglial glutamate transporter EAAT2 governs its intracellular compartmentalization. Glia 62, 1241–1253. doi: 10.1002/glia.22677
PubMed Abstract | Full Text | CrossRef Full Text | Google Scholar
Fukui, M., Song, J. H., Choi, J., Choi, H. J., and Zhu, B. T. (2009). Mechanism of glutamate-induced neurotoxicity in HT22 mouse hippocampal cells. Eur. J. Pharmacol. 617, 1–11. doi: 10.1016/j.ejphar.2009.06.059
PubMed Abstract | Full Text | CrossRef Full Text | Google Scholar
Gliyazova, N. S., Huh, E. Y., and Ibeanu, G. C. (2013). A novel phenoxy thiophene sulphonamide molecule protects against glutamate evoked oxidative injury in a neuronal cell model. BMC Neurosci. 14:93. doi: 10.1186/1471-2202-14-93
PubMed Abstract | Full Text | CrossRef Full Text | Google Scholar
Greene, L. A., and Tischler, A. S. (1976). Establishment of a noradrenergic clonal line of rat adrenal pheochromocytoma cells which respond to nerve growth factor. Proc. Natl. Acad. Sci. U.S.A. 73, 2424–2428. doi: 10.1073/pnas.73.7.2424
Grohm, J., Plesnila, N., and Culmsee, C. (2010). Bid mediates fission, membrane permeabilization and peri-nuclear accumulation of mitochondria as a prerequisite for oxidative neuronal cell death. Brain Behav. Immun. 24, 831–838. doi: 10.1016/j.bbi.2009.11.015
PubMed Abstract | Full Text | CrossRef Full Text | Google Scholar
Gupta, V. K., You, Y., Li, J. C., Klistorner, A., and Graham, S. L. (2013). Protective effects of 7,8-dihydroxyflavone on retinal ganglion and RGC-5 cells against excitotoxic and oxidative stress. J. Mol. Neurosci. 49, 96–104. doi: 10.1007/s12031-012-9899-x
PubMed Abstract | Full Text | CrossRef Full Text | Google Scholar
Gursoy, E., Cardounel, A., and Kalimi, M. (2001). Pregnenolone protects mouse hippocampal (HT-22) cells against glutamate and amyloid beta protein toxicity. Neurochem. Res. 26, 15–21. doi: 10.1023/A:1007668213330
PubMed Abstract | Full Text | CrossRef Full Text | Google Scholar
Han, B. H., and Holtzman, D. M. (2000). BDNF protects the neonatal brain from hypoxic-ischemic injury in vivo via the ERK pathway. J. Neurosci. 20, 5775–5781.
Han, D., Sen, C. K., Roy, S., Kobayashi, M. S., Tritschler, H. J., and Packer, L. (1997). Protection against glutamate-induced cytotoxicity in C6 glial cells by thiol antioxidants. Am. J. Physiol. 273, R1771–R1778.
Hanko, E., Hansen, T. W., Almaas, R., Paulsen, R., and Rootwelt, T. (2006). Synergistic protection of a general caspase inhibitor and MK-801 in bilirubin-induced cell death in human NT2-N neurons. Pediatr. Res. 59, 72–77. doi: 10.1203/01.pdr.0000191135.63586.08
PubMed Abstract | Full Text | CrossRef Full Text | Google Scholar
Hara, M. R., Agrawal, N., Kim, S. F., Cascio, M. B., Fujimuro, M., Ozeki, Y.,et al. (2005). S-nitrosylated GAPDH initiates apoptotic cell death by nuclear translocation following Siah1 binding. Nat. Cell Biol. 7, 665–674. doi: 10.1038/ncb1268
PubMed Abstract | Full Text | CrossRef Full Text | Google Scholar
Hay, N., and Sonenberg, N. (2004). Upstream and downstream of mTOR. Genes Dev. 18, 1926–1945. doi: 10.1101/gad.1212704
PubMed Abstract | Full Text | CrossRef Full Text | Google Scholar
Hayashi, T., and Su, T. P. (2003). Sigma-1 receptors (sigma(1) binding sites) form raft-like microdomains and target lipid droplets on the endoplasmic reticulum: roles in endoplasmic reticulum lipid compartmentalization and export. J. Pharmacol. Exp. Ther. 306, 718–725. doi: 10.1124/jpet.103.051284
PubMed Abstract | Full Text | CrossRef Full Text | Google Scholar
Hertz, L., Peng, L., and Dienel, G. A. (2007). Energy metabolism in astrocytes: high rate of oxidative metabolism and spatiotemporal dependence on glycolysis/glycogenolysis. J. Cereb. Blood Flow Metab. 27, 219–249. doi: 10.1038/sj.jcbfm.9600343
PubMed Abstract | Full Text | CrossRef Full Text | Google Scholar
Hetman, M., Kanning, K., Cavanaugh, J. E., and Xia, Z. (1999). Neuroprotection by brain-derived neurotrophic factor is mediated by extracellular signal-regulated kinase and phosphatidylinositol 3-kinase. J. Biol. Chem. 274, 22569–22580. doi: 10.1074/jbc.274.32.22569
Hetman, M., and Kharebava, G. (2006). Survival signaling pathways activated by NMDA receptors. Curr. Top. Med. Chem. 6, 787–799. doi: 10.2174/156802606777057553
Hirata, Y., Furuta, K., Suzuki, M., Oh-hashi, K., Ueno, Y., and Kiuchi, K. (2012). Neuroprotective cyclopentenone prostaglandins up-regulate neurotrophic factors in C6 glioma cells. Brain Res. 1482, 91–100. doi: 10.1016/j.brainres.2012.09.008
PubMed Abstract | Full Text | CrossRef Full Text | Google Scholar
Holohean, A. M., Hackman, J. C., and Davidoff, R. A. (1999). Mechanisms involved in the metabotropic glutamate receptor-enhancement of NMDA-mediated motoneurone responses in frog spinal cord. Br. J. Pharmacol. 126, 333–341. doi: 10.1038/sj.bjp.0702263
PubMed Abstract | Full Text | CrossRef Full Text | Google Scholar
Hsieh, Y. C., Athar, M., and Chaudry, I. H. (2009). When apoptosis meets autophagy: deciding cell fate after trauma and sepsis. Trends Mol. Med. 15, 129–138. doi: 10.1016/j.molmed.2009.01.002
PubMed Abstract | Full Text | CrossRef Full Text | Google Scholar
Hu, Y., Li, J., Liu, P., Chen, X., Guo, D. H., Li, Q. S.,et al. (2012). Protection of SH-SY5Y neuronal cells from glutamate-induced apoptosis by 3,6’-disinapoyl sucrose, a bioactive compound isolated from Radix Polygala. J. Biomed. Biotechnol. 2012, 1–5. doi: 10.1155/2012/728342
PubMed Abstract | Full Text | CrossRef Full Text | Google Scholar
Ishikawa, K., Nash, S. R., Nishimune, A., Neki, A., Kaneko, S., and Nakanishi, S. (1999). Competitive interaction of seven in absentia homolog-1A and Ca2+/calmodulin with the cytoplasmic tail of group 1 metabotropic glutamate receptors. Genes Cells 4, 381–390. doi: 10.1046/j.1365-2443.1999.00269.x
Ismail, N., Ismail, M., Fathy, S. F., Musa, S. N., Imam, M. U., Foo, J. B.,et al. (2012). Neuroprotective effects of germinated brown rice against hydrogen peroxide induced cell death in human SH-SY5Y cells. Int. J. Mol. Sci. 13, 9692–9708. doi: 10.3390/ijms13089692
PubMed Abstract | Full Text | CrossRef Full Text | Google Scholar
Itoh, T., Itoh, A., and Pleasure, D. (2003). Bcl-2-related protein family gene expression during oligodendroglial differentiation. J. Neurochem. 85, 1500–1512. doi: 10.1046/j.1471-4159.2003.01795.x
PubMed Abstract | Full Text | CrossRef Full Text | Google Scholar
Janssens, N., and Lesage, A. S. (2001). Glutamate receptor subunit expression in primary neuronal and secondary glial cultures. J. Neurochem. 77, 1457–1474. doi: 10.1046/j.1471-4159.2001.00369.x
Jeon, S. J., Rhee, S. Y., Ryu, J. H., Cheong, J. H., Kwon, K., Yang, S. I.,et al. (2011). Activation of adenosine A2A receptor up-regulates BDNF expression in rat primary cortical neurons. Neurochem. Res. 36, 2259–2269. doi: 10.1007/s11064-011-0550-y
PubMed Abstract | Full Text | CrossRef Full Text | Google Scholar
Johnson, J. W., and Ascher, P. (1990). Voltage-dependent block by intracellular Mg2+ of N-methyl-D-aspartate-activated channels. Biophys. J. 57, 1085–1090. doi: 10.1016/S0006-3495(90)82626-6
Johnson-Farley, N. N., Patel, K., Kim, D., and Cowen, D. S. (2007). Interaction of FGF-2 with IGF-1 and BDNF in stimulating Akt, ERK, and neuronal survival in hippocampal cultures. Brain Res. 1154, 40–49. doi: 10.1016/j.brainres.2007.04.026
PubMed Abstract | Full Text | CrossRef Full Text | Google Scholar
Karki, P., Webb, A., Zerguine, A., Choi, J., Son, D. S., and Lee, E. (2014). Mechanism of raloxifene-induced upregulation of glutamate transporters in rat primary astrocytes. Glia 62, 1270–1283. doi: 10.1002/glia.22679
PubMed Abstract | Full Text | CrossRef Full Text | Google Scholar
Karmarkar, S. W., Bottum, K. M., Krager, S. L., and Tischkau, S. A. (2011). ERK/MAPK is essential for endogenous neuroprotection in SCN2.2 Cells. PLoS ONE 6:e23493. doi: 10.1371/journal.pone.0023493
PubMed Abstract | Full Text | CrossRef Full Text | Google Scholar
Karmarkar, S. W., and Tischkau, S. (2013). Influences of the circadian clock on neuronal susceptibility to excitotoxicity. Front. Physiol. 4:313. doi: 10.3389/fphys.2013.00313
PubMed Abstract | Full Text | CrossRef Full Text | Google Scholar
Keinanen, K., Wisden, W., Sommer, B., Werner, P., Herb, A., Verdoorn, T. A.,et al. (1990). A family of AMPA-selective glutamate receptors. Science 249, 556–560. doi: 10.1126/science.2166337
Kelso, S. R., Nelson, T. E., and Leonard, J. P. (1992). Protein kinase C-mediated enhancement of NMDA currents by metabotropic glutamate receptors in Xenopus oocytes. J. Physiol. 449, 705–718. doi: 10.1113/jphysiol.1992.sp019110
PubMed Abstract | Full Text | CrossRef Full Text | Google Scholar
Kim, T. H., Kim, J., Kim, Z., Huang, R. B., and Wang, R. S. (2012). Khz (fusion of Ganoderma lucidum and Polyporus umbellatus mycelia) induces apoptosis by increasing intracellular calcium levels and activating JNK and NADPH oxidase-dependent generation of reactive oxygen species. PLoS ONE 7:e46208. doi: 10.1371/journal.pone.0046208
PubMed Abstract | Full Text | CrossRef Full Text | Google Scholar
Kinney, G. A., and Slater, N. T. (1993). Potentiation of NMDA receptor-mediated transmission in turtle cerebellar granule cells by activation of metabotropic glutamate receptors. J. Neurophysiol. 69, 585–594.
Klocker, N., Kermer, P., Weishaupt, J. H., Labes, M., Ankerhold, R., and Bahr, M. (2000). Brain-derived neurotrophic factor-mediated neuroprotection of adult rat retinal ganglion cells in vivo does not exclusively depend on phosphatidyl-inositol-3’-kinase/protein kinase B signaling. J. Neurosci. 20, 6962–6967.
Kostandy, B. B. (2012). The role of glutamate in neuronal ischemic injury: the role of spark in fire. Neurol. Sci. 33, 223–237. doi: 10.1007/s10072-011-0828-5
PubMed Abstract | Full Text | CrossRef Full Text | Google Scholar
Krishnamoorthy, R. R., Clark, A. F., Daudt, D., Vishwanatha, J. K., and Yorio, T. (2013). A forensic path to RGC-5 cell line identification: lessons learned. Invest. Ophthalmol. Vis. Sci. 54, 5712–5719. doi: 10.1167/iovs.13-12085
PubMed Abstract | Full Text | CrossRef Full Text | Google Scholar
Kritis, A., Pourzitaki, C., Klagas, I., Chourdakis, M., and Albani, M. (2011). Proteases inhibition assessment on PC12 and NGF treated cells after oxygen and glucose deprivation reveals a distinct role for aspartyl proteases. PLoS ONE 6:e25950. doi: 10.1371/journal.pone.0025950
PubMed Abstract | Full Text | CrossRef Full Text | Google Scholar
Kumari, S., Mehta, S. L., and Li, P. A. (2012). Glutamate induces mitochondrial dynamic imbalance and autophagy activation: preventive effects of selenium. PLoS ONE 7:e39382. doi: 10.1371/journal.pone.0039382
PubMed Abstract | Full Text | CrossRef Full Text | Google Scholar
Kume, T., Kawato, Y., Osakada, F., Izumi, Y., Katsuki, H., Nakagawa, T.,et al. (2008). Dibutyryl cyclic AMP induces differentiation of human neuroblastoma SH-SY5Y cells into a noradrenergic phenotype. Neurosci. Lett. 443, 199–203. doi: 10.1016/j.neulet.2008.07.079
PubMed Abstract | Full Text | CrossRef Full Text | Google Scholar
Lai, T. W., Zhang, S., and Wang, Y. T. (2014). Excitotoxicity and stroke: identifying novel targets for neuroprotection. Prog. Neurobiol. 115, 157–188. doi: 10.1016/j.pneurobio.2013.11.006
PubMed Abstract | Full Text | CrossRef Full Text | Google Scholar
Lan, J. Y., Skeberdis, V. A., Jover, T., Zheng, X., Bennett, M. V., and Zukin, R. S. (2001). Activation of metabotropic glutamate receptor 1 accelerates NMDA receptor trafficking. J. Neurosci. 21, 6058–6068.
Landshamer, S., Hoehn, M., Barth, N., Duvezin-Caubet, S., Schwake, G., Tobaben, S.,et al. (2008). Bid-induced release of AIF from mitochondria causes immediate neuronal cell death. Cell Death Differ. 15, 1553–1563. doi: 10.1038/cdd.2008.78
PubMed Abstract | Full Text | CrossRef Full Text | Google Scholar
Lane, D. J., and Lawen, A. (2013). The glutamate aspartate transporter (GLAST) mediates L-glutamate-stimulated ascorbate-release via swelling-activated anion channels in cultured neonatal rodent astrocytes. Cell Biochem. Biophys. 65, 107–119. doi: 10.1007/s12013-012-9404-8
PubMed Abstract | Full Text | CrossRef Full Text | Google Scholar
Lea, P. M., Movsesyan, V. A., and Faden, A. I. (2005). Neuroprotective activity of the mGluR5 antagonists MPEP and MTEP against acute excitotoxicity differs and does not reflect actions at mGluR5 receptors. Br. J. Pharmacol. 145, 527–534. doi: 10.1038/sj.bjp.0706219
PubMed Abstract | Full Text | CrossRef Full Text | Google Scholar
Leach, M. J., Marden, C. M., and Miller, A. A. (1986). Pharmacological studies on lamotrigine, a novel potential antiepileptic drug: II. Neurochemical studies on the mechanism of action. Epilepsia 27, 490–497. doi: 10.1111/j.1528-1157.1986.tb03573.x
PubMed Abstract | Full Text | CrossRef Full Text | Google Scholar
Leclerc, C. L., Chi, C. L., Awobuluyi, M., and Sucher, N. J. (1995). Expression of N-methyl-D-aspartate receptor subunit mRNAs in the rat pheochromocytoma cell line PC12. Neurosci. Lett. 201, 103–106. doi: 10.1016/0304-3940(95)12145-5
Lesage, A. S., De Loore, K. L., Peeters, L., and Leysen, J. E. (1995). Neuroprotective sigma ligands interfere with the glutamate-activated NOS pathway in hippocampal cell culture. Synapse 20, 156–164. doi: 10.1002/syn.890200210
PubMed Abstract | Full Text | CrossRef Full Text | Google Scholar
Lu, M., Hu, L. F., Hu, G., and Bian, J. S. (2008). Hydrogen sulfide protects astrocytes against H(2)O(2)-induced neural injury via enhancing glutamate uptake. Free Radic. Biol. Med. 45, 1705–1713. doi: 10.1016/j.freeradbiomed.2008.09.014
PubMed Abstract | Full Text | CrossRef Full Text | Google Scholar
Lu, S., Lu, C., Han, Q., Li, J., Du, Z., Liao, L.,et al. (2011). Adipose-derived mesenchymal stem cells protect PC12 cells from glutamate excitotoxicity-induced apoptosis by upregulation of XIAP through PI3-K/Akt activation. Toxicology 279, 189–195. doi: 10.1016/j.tox.2010.10.011
PubMed Abstract | Full Text | CrossRef Full Text | Google Scholar
Luetjens, C. M., Bui, N. T., Sengpiel, B., Munstermann, G., Poppe, M., Krohn, A. J.,et al. (2000). Delayed mitochondrial dysfunction in excitotoxic neuron death: cytochrome c release and a secondary increase in superoxide production. J. Neurosci. 20, 5715–5723.
Luo, H. R., Hattori, H., Hossain, M. A., Hester, L., Huang, Y., Lee-Kwon, W.,et al. (2003). Akt as a mediator of cell death. Proc. Natl. Acad. Sci. U.S.A. 100, 11712–11717. doi: 10.1073/pnas.1634990100
PubMed Abstract | Full Text | CrossRef Full Text | Google Scholar
Ma, S., Liu, H., Jiao, H., Wang, L., Chen, L., Liang, J.,et al. (2012). Neuroprotective effect of ginkgolide K on glutamate-induced cytotoxicity in PC 12 cells via inhibition of ROS generation and Ca2+ influx. Neurotoxicology 33, 59–69. doi: 10.1016/j.neuro.2011.11.003
PubMed Abstract | Full Text | CrossRef Full Text | Google Scholar
Marchetti, L., Klein, M., Schlett, K., Pfizenmaier, K., and Eisel, U. L. (2004). Tumor necrosis factor (TNF)-mediated neuroprotection against glutamate-induced excitotoxicity is enhanced by N-methyl-D-aspartate receptor activation. Essential role of a TNF receptor 2-mediated phosphatidylinositol 3-kinase-dependent NF-kappa B pathway. J. Biol. Chem. 279, 32869–32881. doi: 10.1074/jbc.M311766200
PubMed Abstract | Full Text | CrossRef Full Text | Google Scholar
McMullan, S. M., Phanavanh, B., Li, G. G., and Barger, S. W. (2012). Metabotropic glutamate receptors inhibit microglial glutamate release. ASN Neuro 4:e00094. doi: 10.1042/AN20120044
PubMed Abstract | Full Text | CrossRef Full Text | Google Scholar
Mehta, A., Prabhakar, M., Kumar, P., Deshmukh, R., and Sharma, P. L. (2012). Excitotoxicity: bridge to various triggers in neurodegenerative disorders. Eur. J. Pharmacol. 698, 6–18. doi: 10.1016/j.ejphar.2012.10.032
PubMed Abstract | Full Text | CrossRef Full Text | Google Scholar
Meldrum, B. S. (1994). The role of glutamate in epilepsy and other CNS disorders. Neurology 44, S14–S23.
Mellon, P. L., Windle, J. J., Goldsmith, P. C., Padula, C. A., Roberts, J. L., and Weiner, R. I. (1990). Immortalization of hypothalamic GnRH neurons by genetically targeted tumorigenesis. Neuron 5, 1–10. doi: 10.1016/0896-6273(90)90028-E
PubMed Abstract | Full Text | CrossRef Full Text | Google Scholar
Minakami, R., Jinnai, N., and Sugiyama, H. (1997). Phosphorylation and calmodulin binding of the metabotropic glutamate receptor subtype 5 (mGluR5) are antagonistic in vitro. J. Biol. Chem. 272, 20291–20298. doi: 10.1074/jbc.272.32.20291
PubMed Abstract | Full Text | CrossRef Full Text | Google Scholar
Mollereau, C., Zajac, J. M., and Roumy, M. (2007). Staurosporine differentiation of NPFF2 receptor-transfected SH-SY5Y neuroblastoma cells induces selectivity of NPFF activity towards opioid receptors. Peptides 28, 1125–1128. doi: 10.1016/j.peptides.2007.03.001
PubMed Abstract | Full Text | CrossRef Full Text | Google Scholar
Monyer, H., Sprengel, R., Schoepfer, R., Herb, A., Higuchi, M., Lomeli, H.,et al. (1992). Heteromeric NMDA receptors: molecular and functional distinction of subtypes. Science 256, 1217–1221. doi: 10.1126/science.256.5060.1217
PubMed Abstract | Full Text | CrossRef Full Text | Google Scholar
Munir, M., Lu, L., and Mcgonigle, P. (1995). Excitotoxic cell death and delayed rescue in human neurons derived from NT2 cells. J. Neurosci. 15, 7847–7860.
Murphy, T. H., Miyamoto, M., Sastre, A., Schnaar, R. L., and Coyle, J. T. (1989). Glutamate toxicity in a neuronal cell line involves inhibition of cystine transport leading to oxidative stress. Neuron 2, 1547–1558. doi: 10.1016/0896-6273(89)90043-3
PubMed Abstract | Full Text | CrossRef Full Text | Google Scholar
Murphy, T. H., Schnaar, R. L., and Coyle, J. T. (1990). Immature cortical neurons are uniquely sensitive to glutamate toxicity by inhibition of cystine uptake. FASEB J. 4, 1624–1633.
Naarala, J., Nykvist, P., Tuomala, M., and Savolainen, K. (1993). Excitatory amino acid-induced slow biphasic responses of free intracellular calcium in human neuroblastoma cells. FEBS Lett. 330, 222–226. doi: 10.1016/0014-5793(93)80278-3
PubMed Abstract | Full Text | CrossRef Full Text | Google Scholar
Naarala, J., Tervo, P., Loikkanen, J., and Savolainen, K. (2002). Blocking of carbacholGANinduced calcium mobilization by glutamate receptor antagonists. Neurosci. Res. Commun. 30, 1–6. doi: 10.1002/nrc.10012
Nair, V. D., Niznik, H. B., and Mishra, R. K. (1996). Interaction of NMDA and dopamine D2L receptors in human neuroblastoma SH-SY5Y cells. J. Neurochem. 66, 2390–2393. doi: 10.1046/j.1471-4159.1996.66062390.x
Nakagawa, D., Ohshima, Y., Takusagawa, M., Ikota, N., Takahashi, Y., Shimizu, S.,et al. (2001). Functional modification of cytochrome c by peroxynitrite in an electron transfer reaction. Chem. Pharm. Bull. (Tokyo) 49, 1547–1554. doi: 10.1248/cpb.49.1547
PubMed Abstract | Full Text | CrossRef Full Text | Google Scholar
Nakanishi, S., Masu, M., Bessho, Y., Nakajima, Y., Hayashi, Y., and Shigemoto, R. (1994). Molecular diversity of glutamate receptors and their physiological functions. EXS 71, 71–80.
Nampoothiri, M., Reddy, N. D., John, J., Kumar, N., Nampurath, G. K., and Chamallamudi, M. R. (2014). Insulin blocks glutamate-induced neurotoxicity in differentiated SH-SY5Y neuronal cells. Behav. Neurol. 2014:674164. doi: 10.1155/2014/674164
PubMed Abstract | Full Text | CrossRef Full Text | Google Scholar
Ness, J. K., Scaduto, R. C., and Wood, T. L. (2004). IGF-I prevents glutamate-mediated bax translocation and cytochrome C release in O4+ oligodendrocyte progenitors. Glia 46, 183–194. doi: 10.1002/glia.10360
PubMed Abstract | Full Text | CrossRef Full Text | Google Scholar
Nguyen, T. L., Kim, C. K., Cho, J. H., Lee, K. H., and Ahn, J. Y. (2010). Neuroprotection signaling pathway of nerve growth factor and brain-derived neurotrophic factor against staurosporine induced apoptosis in hippocampal H19-7 cells. Exp. Mol. Med. 42, 583–595. doi: 10.3858/emm.2010.42.8.060
PubMed Abstract | Full Text | CrossRef Full Text | Google Scholar
Nicholls, D. G., and Budd, S. L. (1998). Neuronal excitotoxicity: the role of mitochondria. Biofactors 8, 287–299. doi: 10.1002/biof.5520080317
Niciu, M. J., Kelmendi, B., and Sanacora, G. (2012). Overview of glutamatergic neurotransmission in the nervous system. Pharmacol. Biochem. Behav. 100, 656–664. doi: 10.1016/j.pbb.2011.08.008
PubMed Abstract | Full Text | CrossRef Full Text | Google Scholar
Nikoletopoulou, V., Markaki, M., Palikaras, K., and Tavernarakis, N. (2013). Crosstalk between apoptosis, necrosis and autophagy. Biochim. Biophys. Acta 1833, 3448–3459. doi: 10.1016/j.bbamcr.2013.06.001
PubMed Abstract | Full Text | CrossRef Full Text | Google Scholar
Nikolova, S., Lee, Y. S., Lee, Y. S., and Kim, J. A. (2005). Rac1-NADPH oxidase-regulated generation of reactive oxygen species mediates glutamate-induced apoptosis in SH-SY5Y human neuroblastoma cells. Free Radic. Res. 39, 1295–1304. doi: 10.1080/10715760500176866
PubMed Abstract | Full Text | CrossRef Full Text | Google Scholar
Nixon, R. A., Mathews, P. M., and Cataldo, A. M. (2001). The neuronal endosomal-lysosomal system in Alzheimer’s disease. J. Alzheimers Dis. 3, 97–107.
Obara-Michlewska, M., Ruszkiewicz, J., Zielinska, M., Verkhratsky, A., and Albrecht, J. (2014). Astroglial NMDA receptors inhibit expression of K4.1 channels in glutamate-overexposed astrocytes in vitro and in the brain of rats with acute liver failure. Neurochem. Int. doi: 10.1016/j.neuint.2014.10.006 [Epub ahead of print].
Oka, A., Belliveau, M. J., Rosenberg, P. A., and Volpe, J. J. (1993). Vulnerability of oligodendroglia to glutamate - pharmacology, mechanisms, and prevention. J. Neurosci. 13, 1441–1453.
Pahlman, S., Odelstad, L., Larsson, E., Grotte, G., and Nilsson, K. (1981). Phenotypic changes of human neuroblastoma cells in culture induced by 12-O-tetradecanoyl-phorbol-13-acetate. Int. J. cancer 28, 583–589. doi: 10.1002/ijc.2910280509
Park, K. W., Nozell, S. E., and Benveniste, E. N. (2012). Protective role of STAT3 in NMDA and glutamate-induced neuronal death: negative regulatory effect of SOCS3. PLoS ONE 7:e50874. doi: 10.1371/journal.pone.0050874
PubMed Abstract | Full Text | CrossRef Full Text | Google Scholar
Paquet-Durand, F., and Bicker, G. (2004). Hypoxic/ischaemic cell damage in cultured human NT-2 neurons. Brain Res. 1011, 33–47. doi: 10.1016/j.brainres.2004.02.060
PubMed Abstract | Full Text | CrossRef Full Text | Google Scholar
Paquet-Durand, F., Gierse, A., and Bicker, G. (2006). Diltiazem protects human NT-2 neurons against excitotoxic damage in a model of simulated ischemia. Brain Res. 1124, 45–54. doi: 10.1016/j.brainres.2006.09.077
PubMed Abstract | Full Text | CrossRef Full Text | Google Scholar
Penugonda, S., Mare, S., Lutz, P., Banks, W. A., and Ercal, N. (2006). Potentiation of lead-induced cell death in PC12 cells by glutamate: protection by N-acetylcysteine amide (NACA), a novel thiol antioxidant. Toxicol. Appl. Pharmacol. 216, 197–205. doi: 10.1016/j.taap.2006.05.002
PubMed Abstract | Full Text | CrossRef Full Text | Google Scholar
Pereira, C. M., and Oliveira, C. R. (1997). Glutamate toxicity on a PC12 cell line involves glutathione (GSH) depletion and oxidative stress. Free Radic. Biol. Med. 23, 637–647. doi: 10.1016/S0891-5849(97)00020-8
PubMed Abstract | Full Text | CrossRef Full Text | Google Scholar
Pereira, C. F., and Oliveira, C. R. (2000). Oxidative glutamate toxicity involves mitochondrial dysfunction and perturbation of intracellular Ca2+ homeostasis. Neurosci. Res. 37, 227–236. doi: 10.1016/S0168-0102(00)00124-3
Perovic, S., Pialoglou, P., Schroder, H. C., Pergande, G., and Muller, W. E. (1996). Flupirtine increases the levels of glutathione and Bc1-2 in hNT (human Ntera/D1) neurons: mode of action of the drug-mediated anti-apoptotic effect. Eur. J. Pharmacol. 317, 157–164. doi: 10.1016/S0014-2999(96)00712-1
Persons, D. L., Yazlovitskaya, E. M., Cui, W., and Pelling, J. C. (1999). Cisplatin-induced activation of mitogen-activated protein kinases in ovarian carcinoma cells: inhibition of extracellular signal-regulated kinase activity increases sensitivity to cisplatin. Clin. Cancer Res. 5, 1007–1014.
Peterson, C., Neal, J. H., and Cotman, C. W. (1989). Development of N-methyl-D-aspartate excitotoxicity in cultured hippocampal neurons. Brain Res. Dev. Brain Res. 48, 187–195. doi: 10.1016/0165-3806(89)90075-8
Peterson, G. M., and Moore, R. Y. (1980). Selective effects of kainic acid on diencephalic neurons. Brain Res. 202, 165–182. doi: 10.1016/S0006-8993(80)80042-4
Piers, T. M., Kim, D. H., Kim, B. C., Regan, P., Whitcomb, D. J., and Cho, K. (2012). Translational concepts of mGluR5 in synaptic diseases of the brain. Front. Pharmacol. 3:199. doi: 10.3389/fphar.2012.00199
PubMed Abstract | Full Text | CrossRef Full Text | Google Scholar
Pivovarova, N. B., Nguyen, H. V., Winters, C. A., Brantner, C. A., Smith, C. L., and Andrews, S. B. (2004). Excitotoxic calcium overload in a subpopulation of mitochondria triggers delayed death in hippocampal neurons. J. Neurosci. 24, 5611–5622. doi: 10.1523/JNEUROSCI.0531-04.2004
PubMed Abstract | Full Text | CrossRef Full Text | Google Scholar
Podrygajlo, G., Tegenge, M. A., Gierse, A., Paquet-Durand, F., Tan, S., Bicker, G.,et al. (2009). Cellular phenotypes of human model neurons (NT2) after differentiation in aggregate culture. Cell Tissue Res. 336, 439–452. doi: 10.1007/s00441-009-0783-0
PubMed Abstract | Full Text | CrossRef Full Text | Google Scholar
Poteet, E., Winters, A., Yan, L. J., Shufelt, K., Green, K. N., Simpkins, J. W.,et al. (2012). Neuroprotective actions of methylene blue and its derivatives. PLoS ONE 7:e48279. doi: 10.1371/journal.pone.0048279
PubMed Abstract | Full Text | CrossRef Full Text | Google Scholar
Pourzitaki, C., Kanellos, G., Klagas, I., and Kritis, A. (2008). Combined treatment of aspartyl protease inhibitor and Nmda antagonist in Pc12 cells after glutamate excitotoxicity. Rev. Clin. Pharmacol. Pharmacokinet. Int. Ed. 22, 304–307.
Pourzitaki, C., Klagas, I., Kaidoglou, A., Tzimagiorgis, G., and Kritis, A. (2007). Caspase dependent and independent cell death in na+ψve and NGF treated PC12 cells after glutamate induced exitotoxicity. Epitheor. Klin. Farmakol. Farmakokinet. 25, 94–97.
Pourzitaki, C., Klagas, I., Kanellos, G., and Kritis, A. (2009). Calpaine mediated cell death in naive and NGF treated PC12 cells after glutamate induced exitotoxicity. Epitheor. Klin. Farmakol. Farmakokinet. 27, 79–82.
Qiu, J., Tan, Y. W., Hagenston, A. M., Martel, M. A., Kneisel, N., Skehel, P. A.,et al. (2013). Mitochondrial calcium uniporter Mcu controls excitotoxicity and is transcriptionally repressed by neuroprotective nuclear calcium signals. Nat. Commun. 4:2034. doi: 10.1038/ncomms3034
PubMed Abstract | Full Text | CrossRef Full Text | Google Scholar
Radi, R., Beckman, J. S., Bush, K. M., and Freeman, B. A. (1991a). Peroxynitrite oxidation of sulfhydryls. The cytotoxic potential of superoxide and nitric oxide. J. Biol. Chem. 266, 4244–4250.
Radi, R., Beckman, J. S., Bush, K. M., and Freeman, B. A. (1991b). Peroxynitrite-induced membrane lipid peroxidation: the cytotoxic potential of superoxide and nitric oxide. Arch. Biochem. Biophys. 288, 481–487. doi: 10.1016/0003-9861(91)90224-7
PubMed Abstract | Full Text | CrossRef Full Text | Google Scholar
Radi, R., Rodriguez, M., Castro, L., and Telleri, R. (1994). Inhibition of mitochondrial electron transport by peroxynitrite. Arch. Biochem. Biophys. 308, 89–95. doi: 10.1006/abbi.1994.1013
PubMed Abstract | Full Text | CrossRef Full Text | Google Scholar
Rahman, S., and Neuman, R. S. (1996). Characterization of metabotropic glutamate receptor-mediated facilitation of N-methyl-D-aspartate depolarization of neocortical neurones. Br. J. Pharmacol. 117, 675–683. doi: 10.1111/j.1476-5381.1996.tb15243.x
Reid, C. A., Bekkers, J. M., and Clements, J. D. (2003). Presynaptic Ca2+ channels: a functional patchwork. Trends Neurosci. 26, 683–687. doi: 10.1016/j.tins.2003.10.003
PubMed Abstract | Full Text | CrossRef Full Text | Google Scholar
Reynolds, I. J., and Hastings, T. G. (1995). Glutamate induces the production of reactive oxygen species in cultured forebrain neurons following NMDA receptor activation. J. Neurosci. 15, 3318–3327.
Rossi, D. J., Brady, J. D., and Mohr, C. (2007). Astrocyte metabolism and signaling during brain ischemia. Nat. Neurosci. 10, 1377–1386. doi: 10.1038/nn2004
PubMed Abstract | Full Text | CrossRef Full Text | Google Scholar
Roth, J. A., Feng, L., Walowitz, J., and Browne, R. W. (2000). Manganese-induced rat pheochromocytoma (PC12) cell death is independent of caspase activation. J. Neurosci. Res. 61, 162–171. doi: 10.1002/1097-4547(20000715)61:2<162::AID-JNR7>3.0.CO;2-G
PubMed Abstract | Full Text | CrossRef Full Text | Google Scholar
Ruiz, A., Matute, C., and Alberdi, E. (2010). Intracellular Ca2+ release through ryanodine receptors contributes to AMPA receptor-mediated mitochondrial dysfunction and ER stress in oligodendrocytes. Cell Death Dis. 1:e54. doi: 10.1038/cddis.2010.31
PubMed Abstract | Full Text | CrossRef Full Text | Google Scholar
Sadasivan, S., Zhang, Z., Larner, S. F., Liu, M. C., Zheng, W., Kobeissy, F. H.,et al. (2010). Acute NMDA toxicity in cultured rat cerebellar granule neurons is accompanied by autophagy induction and late onset autophagic cell death phenotype. BMC Neurosci. 11:21. doi: 10.1186/1471-2202-11-21
PubMed Abstract | Full Text | CrossRef Full Text | Google Scholar
Said, S. I., Dickman, K., Dey, R. D., Bandyopadhyay, A., De Stefanis, P., Raza, S.,et al. (1998). Glutamate toxicity in the lung and neuronal cells: prevention or attenuation by VIP and PACAP. Ann. N. Y. Acad. Sci. 865, 226–237. doi: 10.1111/j.1749-6632.1998.tb11182.x
Salgo, M. G., Stone, K., Squadrito, G. L., Battista, J. R., and Pryor, W. A. (1995). Peroxynitrite causes DNA nicks in plasmid pBR322. Biochem. Biophys. Res. Commun. 210, 1025–1030. doi: 10.1006/bbrc.1995.1759
PubMed Abstract | Full Text | CrossRef Full Text | Google Scholar
Salter, M. W. (1998). Src, N-methyl-D-aspartate (n.d.) receptors, and synaptic plasticity. Biochem. Pharmacol. 56, 789–798. doi: 10.1016/S0006-2952(98)00124-5
Sanchez-Gomez, M. V., Alberdi, E., Ibarretxe, G., Torre, I., and Matute, C. (2003). Caspase-dependent and caspase-independent oligodendrocyte death mediated by AMPA and kainate receptors. J. Neurosci. 23, 9519–9528.
Sandhu, J. K., Pandey, S., Ribecco-Lutkiewicz, M., Monette, R., Borowy-Borowski, H., Walker, P. R.,et al. (2003). Molecular mechanisms of glutamate neurotoxicity in mixed cultures of NT2-derived neurons and astrocytes: protective effects of coenzyme Q(10). J. Neurosci. Res. 72, 691–703. doi: 10.1002/jnr.10579
PubMed Abstract | Full Text | CrossRef Full Text | Google Scholar
Sandhu, J. K., Sikorska, M., and Walker, P. R. (2002). Characterization of astrocytes derived from human NTera-2/D1 embryonal carcinoma cells. J. Neurosci. Res. 68, 604–614. doi: 10.1002/jnr.10236
Schrammel, A., Gorren, A. C., Schmidt, K., Pfeiffer, S., and Mayer, B. (2003). S-nitrosation of glutathione by nitric oxide, peroxynitrite, and (*)NO/O(2)(*-). Free Radic. Biol. Med. 34, 1078–1088. doi: 10.1016/S0891-5849(03)00038-8
Schubert, D., Kimura, H., and Maher, P. (1992). Growth factors and vitamin E modify neuronal glutamate toxicity. Proc. Natl. Acad. Sci. U.S.A. 89, 8264–8267. doi: 10.1073/pnas.89.17.8264
PubMed Abstract | Full Text | CrossRef Full Text | Google Scholar
Seib, T. M., Patel, S. A., and Bridges, R. J. (2011). Regulation of the system x(C)- cystine/glutamate exchanger by intracellular glutathione levels in rat astrocyte primary cultures. Glia 59, 1387–1401. doi: 10.1002/glia.21176
PubMed Abstract | Full Text | CrossRef Full Text | Google Scholar
Sen, N., Hara, M. R., Ahmad, A. S., Cascio, M. B., Kamiya, A., Ehmsen, J. T.,et al. (2009). GOSPEL: a neuroprotective protein that binds to GAPDH upon S-nitrosylation. Neuron 63, 81–91. doi: 10.1016/j.neuron.2009.05.024
PubMed Abstract | Full Text | CrossRef Full Text | Google Scholar
Sen, N., Hara, M. R., Kornberg, M. D., Cascio, M. B., Bae, B. I., Shahani, N.,et al. (2008). Nitric oxide-induced nuclear GAPDH activates p300/CBP and mediates apoptosis. Nat. Cell Biol. 10, 866–873. doi: 10.1038/ncb1747
PubMed Abstract | Full Text | CrossRef Full Text | Google Scholar
Shah, S. A., Lee, H. Y., Bressan, R. A., Yun, D. J., and Kim, M. O. (2014). Novel osmotin attenuates glutamate-induced synaptic dysfunction and neurodegeneration via the JNK/PI3K/Akt pathway in postnatal rat brain. Cell death dis. 5:e1026.
Shang, L., Huang, J. F., Ding, W., Chen, S., Xue, L. X., Ma, R. F.,et al. (2014). Calpain: a molecule to induce AIF-mediated necroptosis in RGC-5 following elevated hydrostatic pressure. BMC Neurosci. 15:63. doi: 10.1186/1471-2202-15-63
PubMed Abstract | Full Text | CrossRef Full Text | Google Scholar
Shinohara, Y., Nakajima, Y., and Nakanishi, S. (2001). Glutamate induces focal adhesion kinase tyrosine phosphorylation and actin rearrangement in heterologous mGluR1-expressing CHO cells via calcium/calmodulin signaling. J. Neurochem. 78, 365–373. doi: 10.1046/j.1471-4159.2001.00415.x
PubMed Abstract | Full Text | CrossRef Full Text | Google Scholar
Singh, J., and Kaur, G. (2009). Transcriptional regulation of PSA-NCAM expression by NMDA receptor activation in RA-differentiated C6 glioma cultures. Brain Res. Bull. 79, 157–168. doi: 10.1016/j.brainresbull.2009.02.005
Skeberdis, V. A., Lan, J., Opitz, T., Zheng, X., Bennett, M. V., and Zukin, R. S. (2001a). mGluR1-mediated potentiation of NMDA receptors involves a rise in intracellular calcium and activation of protein kinase C. Neuropharmacology 40, 856–865. doi: 10.1016/S0028-3908(01)00005-3
PubMed Abstract | Full Text | CrossRef Full Text | Google Scholar
Skeberdis, V. A., Lan, J., Zheng, X., Zukin, R. S., and Bennett, M. V. (2001b). Insulin promotes rapid delivery of N-methyl-D- aspartate receptors to the cell surface by exocytosis. Proc. Natl. Acad. Sci. U.S.A. 98, 3561–3566. doi: 10.1073/pnas.051634698
PubMed Abstract | Full Text | CrossRef Full Text | Google Scholar
Smialowska, M., Golembiowska, K., Kajta, M., Zieba, B., Dziubina, A., and Domin, H. (2012). Selective mGluR1 antagonist EMQMCM inhibits the kainate-induced excitotoxicity in primary neuronal cultures and in the rat hippocampus. Neurotox. Res. 21, 379–392. doi: 10.1007/s12640-011-9293-4
PubMed Abstract | Full Text | CrossRef Full Text | Google Scholar
Sribnick, E. A., Ray, S. K., and Banik, N. L. (2006). Estrogen prevents glutamate-induced apoptosis in C6 glioma cells by a receptor-mediated mechanism. Neuroscience 137, 197–209. doi: 10.1016/j.neuroscience.2005.08.074
PubMed Abstract | Full Text | CrossRef Full Text | Google Scholar
Stanciu, M., Wang, Y., Kentor, R., Burke, N., Watkins, S., Kress, G.,et al. (2000). Persistent activation of ERK contributes to glutamate-induced oxidative toxicity in a neuronal cell line and primary cortical neuron cultures. J. Biol. Chem. 275, 12200–12206. doi: 10.1074/jbc.275.16.12200
PubMed Abstract | Full Text | CrossRef Full Text | Google Scholar
Sucher, N. J., Brose, N., Deitcher, D. L., Awobuluyi, M., Gasic, G. P., Bading, H.,et al. (1993). Expression of endogenous NMDAR1 transcripts without receptor protein suggests post-transcriptional control in PC12 cells. J. Biol. Chem. 268, 22299–22304.
Suh, H. W., Kang, S., and Kwon, K. S. (2007). Curcumin attenuates glutamate-induced HT22 cell death by suppressing MAP kinase signaling. Mol. Cell. Biochem. 298, 187–194. doi: 10.1007/s11010-006-9365-6
PubMed Abstract | Full Text | CrossRef Full Text | Google Scholar
Sulkowski, G., Dabrowska-Bouta, B., Salinska, E., and Struzynska, L. (2014). Modulation of glutamate transport and receptor binding by glutamate receptor antagonists in EAE rat brain. PLoS ONE 9:e113954. doi: 10.1371/journal.pone.0113954
PubMed Abstract | Full Text | CrossRef Full Text | Google Scholar
Sun, D., and Murali, S. G. (1998). Stimulation of Na+-K+-2Cl- cotransporter in neuronal cells by excitatory neurotransmitter glutamate. Am. J. Physiol. 275, C772–C779.
Sun, L., Gu, L., Wang, S., Yuan, J., Yang, H., Zhu, J.,et al. (2012). N-acetylcysteine protects against apoptosis through modulation of group I metabotropic glutamate receptor activity. PLoS ONE 7:e32503. doi: 10.1371/journal.pone.0032503
PubMed Abstract | Full Text | CrossRef Full Text | Google Scholar
Sun, Z. W., Zhang, L., Zhu, S. J., Chen, W. C., and Mei, B. (2010). Excitotoxicity effects of glutamate on human neuroblastoma SH-SY5Y cells via oxidative damage. Neurosci. Bull. 26, 8–16. doi: 10.1007/s12264-010-0813-7
PubMed Abstract | Full Text | CrossRef Full Text | Google Scholar
Susin, S. A., Lorenzo, H. K., Zamzami, N., Marzo, I., Snow, B. E., Brothers, G. M.,et al. (1999). Molecular characterization of mitochondrial apoptosis-inducing factor. Nature 397, 441–446. doi: 10.1038/17135
PubMed Abstract | Full Text | CrossRef Full Text | Google Scholar
Suyama, K., Watanabe, M., Sakabe, K., Okada, Y., Matsuyama, D., Kuroiwa, M.,et al. (2011). Overexpression of GRP78 protects glial cells from endoplasmic reticulum stress. Neurosci. Lett. 504, 271–276. doi: 10.1016/j.neulet.2011.09.045
PubMed Abstract | Full Text | CrossRef Full Text | Google Scholar
Szab, C. (2003). Multiple pathways of peroxynitrite cytotoxicity. Toxicol. Lett. 140–141, 105–112. doi: 10.1016/S0378-4274(02)00507-6
Tan, S., Schubert, D., and Maher, P. (2001). Oxytosis: a novel form of programmed cell death. Curr. Top. Med. Chem. 1, 497–506. doi: 10.2174/1568026013394741
PubMed Abstract | Full Text | CrossRef Full Text | Google Scholar
Tan, S., Wood, M., and Maher, P. (1998). Oxidative stress induces a form of programmed cell death with characteristics of both apoptosis and necrosis in neuronal cells. J. Neurochem. 71, 95–105. doi: 10.1046/j.1471-4159.1998.71010095.x
PubMed Abstract | Full Text | CrossRef Full Text | Google Scholar
Tchedre, K. T., and Yorio, T. (2008). sigma-1 receptors protect RGC-5 cells from apoptosis by regulating intracellular calcium, Bax levels, and caspase-3 activation. Invest. Ophthalmol. Vis. Sci. 49, 2577–2588. doi: 10.1167/iovs.07-1101
Terman, A., Gustafsson, B., and Brunk, U. T. (2006). The lysosomalGAϒ mitochondrial axis theory of postmitotic aging and cell death. Chem. Biol. Interact. 163, 29–37. doi: 10.1016/j.cbi.2006.04.013
PubMed Abstract | Full Text | CrossRef Full Text | Google Scholar
Thomas, G. M., and Huganir, R. L. (2004). MAPK cascade signalling and synaptic plasticity. Nat. Rev. Neurosci. 5, 173–183. doi: 10.1038/nrn1346
PubMed Abstract | Full Text | CrossRef Full Text | Google Scholar
Tobaben, S., Grohm, J., Seiler, A., Conrad, M., Plesnila, N., and Culmsee, C. (2011). Bid-mediated mitochondrial damage is a key mechanism in glutamate-induced oxidative stress and AIF-dependent cell death in immortalized HT-22 hippocampal neurons. Cell Death Differ. 18, 282–292. doi: 10.1038/cdd.2010.92
PubMed Abstract | Full Text | CrossRef Full Text | Google Scholar
Tsuchioka, M., Hisaoka, K., Yano, R., Shibasaki, C., Kajiatani, N., and Takebayashi, M. (2011). Riluzole-induced glial cell line-derived neurotrophic factor production is regulated through fibroblast growth factor receptor signaling in rat C6 glioma cells. Brain Res. 1384, 1–8. doi: 10.1016/j.brainres.2011.01.100
PubMed Abstract | Full Text | CrossRef Full Text | Google Scholar
Tyurin, V. A., Tyurina, Y. Y., Quinn, P. J., Schor, N. F., Balachandran, R., Day, B. W.,et al. (1998). Glutamate-induced cytotoxicity in PC12 pheochromocytoma cells: role of oxidation of phospholipids, glutathione and protein sulfhydryls revealed by bcl-2 transfection. Brain Res. Mol.Brain Res. 60, 270–281. doi: 10.1016/S0169-328X(98)00181-8
PubMed Abstract | Full Text | CrossRef Full Text | Google Scholar
Ugolini, A., Corsi, M., and Bordi, F. (1997). Potentiation of NMDA and AMPA responses by group I mGluR in spinal cord motoneurons. Neuropharmacology 36, 1047–1055. doi: 10.1016/S0028-3908(97)00103-2
PubMed Abstract | Full Text | CrossRef Full Text | Google Scholar
Van Bergen, N. J., Wood, J. P., Chidlow, G., Trounce, I. A., Casson, R. J., Ju, W. K.,et al. (2009). Recharacterization of the RGC-5 retinal ganglion cell line. Invest. Ophthalmol. Vis. Sci. 50, 4267–4272. doi: 10.1167/iovs.09-3484
PubMed Abstract | Full Text | CrossRef Full Text | Google Scholar
Vazhappilly, R., and Sucher, N. J. (2002). Turnover analysis of N-methyl-D-aspartate receptor subunit NR1 protein in PC12 cells. Neurosci. Lett. 318, 153–157. doi: 10.1016/S0304-3940(01)02482-X
Vedia, L., McDonald, B., Reep, B., Brune, B., Di, S. M., Billiar, T. R.,et al. (1992). Nitric oxide-induced S-nitrosylation of glyceraldehyde-3-phosphate dehydrogenase inhibits enzymatic activity and increases endogenous ADP-ribosylation. J. Biol. Chem. 267, 24929–24932.
Veenman, L., Bode, J., Gaitner, M., Caballero, B., Pe’er, Y., Zeno, S.,et al. (2012). Effects of 18-kDa translocator protein knockdown on gene expression of glutamate receptors, transporters, and metabolism, and on cell viability affected by glutamate. Pharmacogenet. Genomics 22, 606–619. doi: 10.1097/FPC.0b013e3283544531
PubMed Abstract | Full Text | CrossRef Full Text | Google Scholar
Verkhratsky, A. (2005). Physiology and pathophysiology of the calcium store in the endoplasmic reticulum of neurons. Physiol. Rev. 85, 201–279. doi: 10.1152/physrev.00004.2004
PubMed Abstract | Full Text | CrossRef Full Text | Google Scholar
Villalba, M., Bockaert, J., and Journot, L. (1997). Pituitary adenylate cyclase-activating polypeptide (PACAP-38) protects cerebellar granule neurons from apoptosis by activating the mitogen-activated protein kinase (MAP kinase) pathway. J. Neurosci. 17, 83–90.
Viwatpinyo, K., and Chongthammakun, S. (2009). Activation of group I metabotropic glutamate receptors leads to brain-derived neurotrophic factor expression in rat C6 cells. Neurosci. Lett. 467, 127–130. doi: 10.1016/j.neulet.2009.10.020
PubMed Abstract | Full Text | CrossRef Full Text | Google Scholar
Voulgari-Kokota, A., Fairless, R., Karamita, M., Kyrargyri, V., Tseveleki, V., Evangelidou, M.,et al. (2012). Mesenchymal stem cells protect CNS neurons against glutamate excitotoxicity by inhibiting glutamate receptor expression and function. Exp. Neurol. 236, 161–170. doi: 10.1016/j.expneurol.2012.04.011
PubMed Abstract | Full Text | CrossRef Full Text | Google Scholar
Wang, Y., and Qin, Z. H. (2010). Molecular and cellular mechanisms of excitotoxic neuronal death. Apoptosis 15, 1382–1402. doi: 10.1007/s10495-010-0481-0
PubMed Abstract | Full Text | CrossRef Full Text | Google Scholar
Xiang, H., Kinoshita, Y., Knudson, C. M., Korsmeyer, S. J., Schwartzkroin, P. A., and Morrison, R. S. (1998). Bax involvement in p53-mediated neuronal cell death. J. Neurosci. 18, 1363–1373.
Xie, H. R., Hu, L. S., and Li, G. Y. (2010). SH-SY5Y human neuroblastoma cell line: in vitro cell model of dopaminergic neurons in Parkinson’s disease. Chin. Med. J. 123, 1086–1092.
Xu, J., Kurup, P., Zhang, Y., Goebel-Goody, S. M., Wu, P. H., Hawasli, A. H.,et al. (2009). Extrasynaptic NMDA receptors couple preferentially to excitotoxicity via calpain-mediated cleavage of STEP. J. Neurosci. 29, 9330–9343. doi: 10.1523/JNEUROSCI.2212-09.2009
Xu, X., Chua, C. C., Kong, J., Kostrzewa, R. M., Kumaraguru, U., Hamdy, R. C.,et al. (2007). Necrostatin-1 protects against glutamate-induced glutathione depletion and caspase-independent cell death in HT-22 cells. J. Neurochem. 103, 2004–2014. doi: 10.1111/j.1471-4159.2007.04884.x
PubMed Abstract | Full Text | CrossRef Full Text | Google Scholar
Xu, X., Chua, C. C., Zhang, M., Geng, D., Liu, C. F., Hamdy, R. C.,et al. (2010). The role of PARP activation in glutamate-induced necroptosis in HT-22 cells. Brain Res. 1343, 206–212. doi: 10.1016/j.brainres.2010.04.080
PubMed Abstract | Full Text | CrossRef Full Text | Google Scholar
Yamakura, F., Taka, H., Fujimura, T., and Murayama, K. (1998). Inactivation of human manganese-superoxide dismutase by peroxynitrite is caused by exclusive nitration of tyrosine 34 to 3-nitrotyrosine. J. Biol. Chem. 273, 14085–14089. doi: 10.1074/jbc.273.23.14085
PubMed Abstract | Full Text | CrossRef Full Text | Google Scholar
Yamaya, Y., Yoshioka, A., Saiki, S., Yuki, N., Hirose, G., and Pleasure, D. (2002). Type-2 astrocyte-like cells are more resistant than oligodendrocyte-like cells against non-N-methyl-D-aspartate glutamate receptor-mediated excitotoxicity. J. Neurosci. Res. 70, 588–598. doi: 10.1002/jnr.10425
PubMed Abstract | Full Text | CrossRef Full Text | Google Scholar
Yang, J. L., Sykora, P., Wilson, D. M. III, Mattson, M. P., and Bohr, V. A. (2011). The excitatory neurotransmitter glutamate stimulates DNA repair to increase neuronal resiliency. Mech. Ageing Dev. 132, 405–411. doi: 10.1016/j.mad.2011.06.005
PubMed Abstract | Full Text | CrossRef Full Text | Google Scholar
Yang, X. R., Sun, P., Qin, H. P., Si, P. P., Sun, X. F., and Zhang, C. (2012). Involvement of MAPK pathways in NMDA-induced apoptosis of rat cortical neurons. Sheng Li Xue Bao 64, 609–616.
Yazlovitskaya, E. M., Pelling, J. C., and Persons, D. L. (1999). Association of apoptosis with the inhibition of extracellular signal-regulated protein kinase activity in the tumor necrosis factor alpha-resistant ovarian carcinoma cell line UCI 101. Mol. Carcinog. 25, 14–20. doi: 10.1002/(SICI)1098-2744(199905)25:1<14::AID-MC2>3.0.CO;2-V
PubMed Abstract | Full Text | CrossRef Full Text | Google Scholar
Yoshioka, A., Hardy, M., Younkin, D. P., Grinspan, J. B., Stern, J. L., and Pleasure, D. (1995). Alpha-amino-3-hydroxy-5-methyl-4-isoxazolepropionate (AMPA) receptors mediate excitotoxicity in the oligodendroglial lineage. J. Neurochem. 64, 2442–2448. doi: 10.1046/j.1471-4159.1995.64062442.x
Yoshioka, A., Yamaya, Y., Saiki, S., Kanemoto, M., Hirose, G., Beesley, J.,et al. (2000). Non-N-methyl-D-aspartate glutamate receptors mediate oxygen–glucose deprivation-induced oligodendroglial injury. Brain Res. 854, 207–215. doi: 10.1016/S0006-8993(99)02359-8
Yoshioka, A., Yudkoff, M., and Pleasure, D. (1997). Expression of glutamic acid decarboxylase during human neuronal differentiation: studies using the NTera-2 culture system. Brain Res. 767, 333–339. doi: 10.1016/S0006-8993(97)00627-6
PubMed Abstract | Full Text | CrossRef Full Text | Google Scholar
Younkin, D. P., Tang, C. M., Hardy, M., Reddy, U. R., Shi, Q. Y., Pleasure, S. J.,et al. (1993). Inducible expression of neuronal glutamate receptor channels in the NT2 human cell line. Proc. Natl. Acad. Sci. U.S.A. 90, 2174–2178. doi: 10.1073/pnas.90.6.2174
Yu, Z., Luo, H., Fu, W., and Mattson, M. P. (1999). The endoplasmic reticulum stress-responsive protein GRP78 protects neurons against excitotoxicity and apoptosis: suppression of oxidative swtress and stabilization of calcium homeostasis. Exp. Neurol. 155, 302–314. doi: 10.1006/exnr.1998.7002
PubMed Abstract | Full Text | CrossRef Full Text | Google Scholar
Zaulyanov, L. L., Green, P. S., and Simpkins, J. W. (1999). Glutamate receptor requirement for neuronal death from anoxia-reoxygenation: an in Vitro model for assessment of the neuroprotective effects of estrogens. Cell. Mol. Neurobiol. 19, 705–718. doi: 10.1023/A:1006948921855
PubMed Abstract | Full Text | CrossRef Full Text | Google Scholar
Zhang, Y., and Bhavnani, B. R. (2006). Glutamate-induced apoptosis in neuronal cells is mediated via caspase-dependent and independent mechanisms involving calpain and caspase-3 proteases as well as apoptosis inducing factor (AIF) and this process is inhibited by equine estrogens. BMC Neurosci. 7:49. doi: 10.1186/1471-2202-7-49
PubMed Abstract | Full Text | CrossRef Full Text | Google Scholar
Zhu, J. W., Yuan, J. F., Yang, H. M., Wang, S. T., Zhang, C. G., Sun, L. L.,et al. (2012). Extracellular cysteine (Cys)/cystine (CySS) redox regulates metabotropic glutamate receptor 5 activity. Biochimie 94, 617–627. doi: 10.1016/j.biochi.2011.09.013
PubMed Abstract | Full Text | CrossRef Full Text | Google Scholar
Keywords: excitotoxicity, glutamate oxidative toxicity, PC12, SH-SY5Y, HT-22, NT-2, RGC-5, SCN2.2
Citation: Kritis AA, Stamoula EG, Paniskaki KA and Vavilis TD (2015) Researching glutamate – induced cytotoxicity in different cell lines: a comparative/collective analysis/study. Front. Cell. Neurosci. 9:91. doi: 10.3389/fncel.2015.00091
Received: 13 January 2015; Accepted: 26 February 2015;
Published online: 17 March 2015
Edited by:
Rena Li, Roskamp Institute, USAReviewed by:
Tibor Kristian, University of Maryland School of Medicine, USAShaohua Yang, University of North Texas Health Science Center, USA
Copyright © 2015 Kritis, Stamoula, Paniskaki and Vavilis. This is an open-access article distributed under the terms of the Creative Commons Attribution License (CC BY). The use, distribution or reproduction in other forums is permitted, provided the original author(s) or licensor are credited and that the original publication in this journal is cited, in accordance with accepted academic practice. No use, distribution or reproduction is permitted which does not comply with these terms.
*Correspondence: Aristeidis A. Kritis, Laboratory of Physiology, Department of Physiology and Pharmacology, School of Medicine, Faculty of Health Sciences, Aristotle University of Thessaloniki, University Campus, 54124 Thessaloniki, Greece kritis@med.auth.gr