Microglial Implication in Parkinson’s Disease: Loss of Beneficial Physiological Roles or Gain of Inflammatory Functions?
- 1Axe Neurosciences, Centre de Recherche du CHU de Québec, Université Laval, Quebec, QC, Canada
- 2Faculté de Pharmacie, Université Laval, Quebec, QC, Canada
- 3Integrated Program of Neuroscience, Faculty of Medicine, McGill University, Montréal, QC, Canada
- 4Département de Chirurgie, Faculté de Médecine, Université Laval, Quebec, QC, Canada
- 5CERVO Brain Research Centre, Quebec, QC, Canada
- 6Département de Psychiatrie et Neurosciences, Faculté de Médecine, Université Laval, Quebec, QC, Canada
- 7Département de Médecine Moléculaire, Faculté de Médecine, Université Laval, Quebec, QC, Canada
Microglia, often described as the brain-resident macrophages, play crucial roles in central nervous system development, maintenance, plasticity, and adaptation to the environment. Both aging and chronic stress promote microglial morphological and functional changes, which can lead to the development of brain pathologies including Parkinson’s disease (PD). Indeed, aging, and chronic stress represent main environmental risk factors for PD. In these conditions, microglia are known to undergo different morphological and functional changes. Inflammation is an important component of PD and disequilibrium between pro- and anti-inflammatory microglial functions might constitute a crucial component of PD onset and progression. Cumulated data also suggest that, during PD, microglia might lose beneficial functions and gain detrimental ones, in addition to mediating inflammation. In this mini-review, we aim to summarize the literature discussing the functional and morphological changes that microglia undergo in PD pathophysiology and upon exposure to its two main environmental risk factors, aging, and chronic stress.
Introduction
Parkinson’s disease (PD) affects one to two individuals per 1000 (Tysnes and Storstein, 2017), making it the most common neurodegenerative movement disorder (Morin et al., 2014). The diagnosis is based on four clinical cardinal signs: rigidity, bradykinesia, resting tremors, and postural instability (Jankovic, 2008). PD is often preceded by a prodromal stage, which includes non-motor symptoms like mood and sleep disorders (Poewe, 2008). In PD, motor symptoms arise from the progressive degeneration of dopaminergic (DA) neurons in the substantia nigra (SN) pars compacta. DA neurons innervate the striatum and their degeneration is associated with a significant decrease of DA striatal content (Morin et al., 2014; Tysnes and Storstein, 2017). DA neurons loss is often associated with an accumulation of Lewy bodies (LB), which are formed by the aggregation of misfolded α-synuclein, mainly in the SN, but also across several brain regions (Tansey and Goldberg, 2010). PD pathogenesis is associated with genetic variations and environmental risk factors that mainly comprise aging and chronic psychological stress, as well as infection, brain trauma, and exposure to pesticides or herbicides (Tansey and Goldberg, 2010; Schapira and Jenner, 2011; Vyas et al., 2016; Niraula et al., 2017). Levodopa (L-DOPA) is the gold-standard symptomatic treatment for PD, as no DA agonist demonstrates an equal efficacy on motors symptoms. However, adverse effects limit its chronic use. Within 5–10 years of treatment, most patients experience motor complications including L-DOPA-induced dyskinesia (LID), abnormal involuntary movements that can be more debilitating than the disease itself (Mercuri and Bernardi, 2005).
Inflammation, among the central nervous system (CNS) and periphery, is also a main hallmark of PD (Vawter et al., 1996; Nagatsu et al., 2000; Imamura et al., 2003; Mount et al., 2007; Litteljohn and Hayley, 2012; Doorn et al., 2014). In the CNS, microglia which are known as the resident immune cells were proposed to mediate the inflammatory response in PD. The two main environmental risk factors for PD, aging, and chronic stress, are linked to increased levels of pro-inflammatory mediators in the CNS and periphery (Vyas et al., 2016; Niraula et al., 2017; Tian et al., 2017). Nevertheless, the implication of microglia in the development and progression of PD is still unclear, and it remains undetermined whether their alterations are a cause or consequence of DA neurons loss (Le et al., 2016). Microglia were recently shown to exert throughout the lifespan crucial physiological roles (Tay et al., 2018), which could become compromised and contribute to PD pathophysiology. Transcriptomic studies also shed light on the complex signature of microglia, defining several phenotypes across contexts of health and disease (Butovsky et al., 2014; Gosselin et al., 2017; Hanamsagar et al., 2017; Wlodarczyk et al., 2017). In the present mini-review, we aim to summarize microglial functions in health and their potential implications in PD.
Diverse Roles of Microglia in Health
The origin of microglia has long been a subject of debate until elegantly designed in vivo lineage studies in mice identified erythromyeloid cells from the embryonic yolk sac as their progenitors (Ginhoux et al., 2010; Schulz et al., 2012; Kierdorf and Prinz, 2013; Gomez et al., 2015; Hoeffel et al., 2015). These progenitors colonize the brain during the first trimester of fetal development, in both rodents, and humans, then mature into microglia (Kierdorf and Prinz, 2013). Thereafter, microglial pools are maintained by self-renewal, at least under normal physiological conditions (Hashimoto et al., 2013; Askew et al., 2017).
Mature microglia which display a ramified morphology, referred to as homeostatic microglia, constantly survey the CNS environment and contribute to its maintenance and plasticity through specific molecular pathways (Tremblay et al., 2011; Kierdorf and Prinz, 2013; Nayak et al., 2014; Schafer and Stevens, 2015; Tay et al., 2017). In particular, homeostatic microglia contribute to synaptogenesis, synaptic pruning, and myelination (Schafer and Stevens, 2015; Kaur et al., 2017; Li and Barres, 2017; Paolicelli and Ferretti, 2017; Tay et al., 2017; Low and Ginhoux, 2018). Microglia are also required for the adaptation of the brain and behavior to the living environment (Tremblay et al., 2011; Schafer and Stevens, 2015; Tay et al., 2017, 2018). Upon injury or infection, and even chronic psychological stress, microglia undergo various morphological and functional changes often designated as microglial “activation” or reactivity to pathological or traumatic challenges (Nayak et al., 2014; Tay et al., 2017). Morphological and functional changes of microglia also occur during aging where these cells become “senescent,” i.e., impaired in their surveillance and response to injury (Streit et al., 2014). Considering that changes in microglial density and morphology profoundly impact on their functions (summarized in Figure 1), these findings indicate that microglia could play an important role in PD.
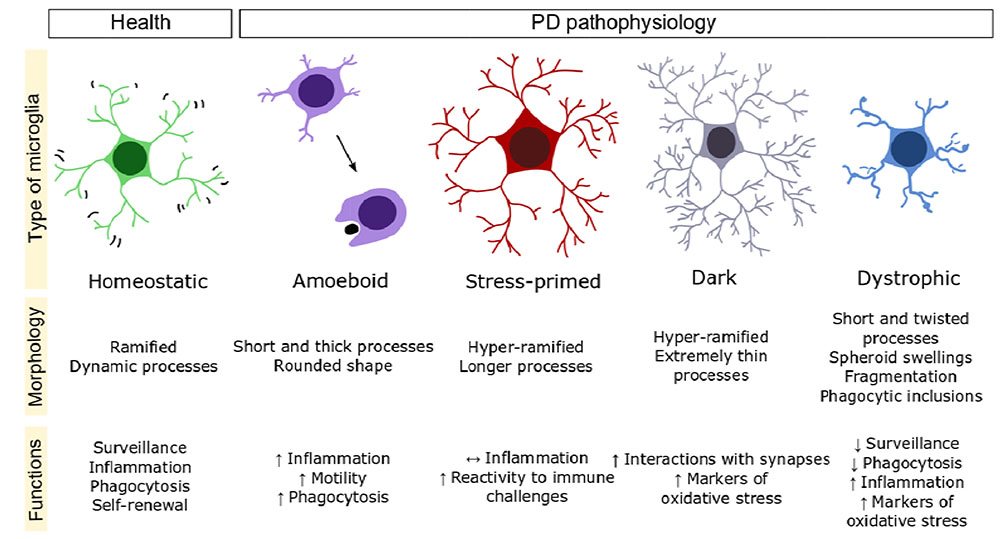
FIGURE 1. Microglial phenotypes that could be differently implicated in PD. Schematized morphologies are represented and their main characteristics summarized. The following symbols are used; ↑, increased; ↔, unchanged; ↓, decreased.
Microglia in Parkinson’s Disease Pathophysiology
In macaque monkeys treated with the neurotoxin 1-methyl-4-phenyl-1,2,3,6-tetrahydropyridine (MPTP), microglia immunopositive (+) for major histocompatibility complex (MHC) class II were found to be highly heterogeneous. They showed ramified, amoeboid or multinucleated morphologies, in the SN, nigrostriatal tract, and globus pallidus. Of note, MHC class II is considered a marker of antigen presentation (Weenink and Gautam, 1997). However, in the striatum of macaque monkeys receiving MPTP, microglia mainly displayed a ramified morphology with little evidence of active phagocytosis associated with the accumulation of fat granules in their processes (Hurley et al., 2003). By contrast, amoeboid microglia were observed in both the SN and striatum of mice exposed to MPTP (Kurkowska-Jastrzebska et al., 1999; Wu et al., 2002). Differences in MPTP administration paradigms or between species could explain this apparent discrepancy (Hurley et al., 2003). In fact, PD progression in humans has been shown to be best recapitulated in non-human primates (Table 1 summarizes different animal models used in PD research) (Grow et al., 2016).
Various brain regions (e.g., pons, basal ganglia, striatum, frontal, and temporal cortices) of PD patients also showed increased binding of the radiotracer 11C-(R)-PK11195 compared to age-matched healthy controls by positron emission tomography (Ouchi et al., 2005; Gerhard et al., 2006). The radiotracer 11C-(R)-PK11195 binds to 18-kDa translocator protein (TSPO) expressed mainly by microglia, in association with inflammatory stimuli (Mondelli et al., 2017). In the SN of post-mortem PD samples, MHC class II+ microglia were first described in 1988 (McGeer et al., 1988). Since then, other studies confirmed the presence of reactive microglia in the SN of PD patients (Hirsch and Hunot, 2009). Besides MHC class II, microglia were shown to express intracellular adhesion molecule (ICAM)-1, integrin receptors CD11a, the lysosomal activity marker CD68, and the scavenger receptor TLR2 in the SN, putamen, and hippocampus of PD patients (Imamura et al., 2003; Doorn et al., 2014). Microglia also stained positively for pro-inflammatory cytokines such as tumor necrosis factor (TNF)α and interleukin (IL)-6 in the striatum of PD patients (Imamura et al., 2003). Other investigators nevertheless failed to observe evidence of microglial reactivity in the same region of PD patients (Knott et al., 1999; Mirza et al., 2000). Cytokines such as IL-1β, IL-2, IL-4, IL-6, TNFα, transforming growth factor (TGF)α, and TGFβ1 were also increased at the protein level in the striatum, and in the ventricular and lumbar cerebrospinal fluid of PD patients (Vawter et al., 1996; Nagatsu et al., 2000). Additionally, high levels of interferon (IFN)γ were measured in blood plasma from PD patients (Mount et al., 2007). Taken together, this data suggests that PD patients possess an increased brain inflammatory status. Neurotoxic reactive species that microglia can produce, such as superoxide and nitric oxide, were proposed to induce cellular stress and, in turn, contribute to neuronal loss in PD (Block et al., 2007; Le et al., 2016). Moreover, the cerebrospinal fluid of PD patients was shown to be toxic to DA neurons in vitro due to the high concentration of cytokines and auto-antibodies against quinone proteins altered by DA oxidation (He et al., 2002; Nagatsu and Sawada, 2005).
Loss of Beneficial Physiological Functions
Signaling between the microglial complement receptor 3 (CR3) and its ligand, the complement component C3, enriched at synapses, plays a key role in synaptic pruning during brain circuits refinement (Schafer et al., 2012). In rats chronically receiving rotenone, a pesticide acting as a mitochondrial complex I inhibitor, CR3+ microglia were more abundant in the striatum and the SN. They also possessed an enlarged cell body with shorter, stubby processes in these two regions, contrary to the cerebral cortex, suggesting an exacerbated phagocytic activity (Sherer et al., 2003). Microglia release neurotrophic and anti-inflammatory factors that promote neuronal survival (Le et al., 2016). These cells can also modulate the formation of dendritic spines through the release of brain-derived neurotrophic factor (BDNF) in mouse primary motor cortex, a role that was required for motor learning and procedural memory (Parkhurst et al., 2013). In PD, BDNF levels were reduced in the nigrostriatal region and/or cerebrospinal fluid of PD patients and animal models, notably exposed to MPTP or 6-hydroxydopamine (6-OHDA) (Nagatsu and Sawada, 2005). Furthermore, glial-derived neurotrophic factor (GDNF) was shown to protect and rescue DA neurons from degeneration in models, including rats exposed to methyl-4-phenylpyridinium (MPP+), the active metabolite of MPTP (Ding et al., 2004; Nam et al., 2015). Additionally, in a mouse model overexpressing human mutant α-synuclein, within neurons mostly of the spinal cord, an increase in ionized calcium binding adaptor molecule 1 (IBA1)+ microglial staining was measured in this region alongside an increased co-expression of AXL (Fourgeaud et al., 2016). With TYRO3 and MER, AXL is part of the TAM receptor family of tyrosine kinases that regulates microglial phagocytic removal of apoptotic cells, notably during adult neurogenesis. In the α-synuclein transgenic mouse, loss of both receptors modestly prolonged the lifespan (Fourgeaud et al., 2016). The authors speculated that microglia might remove distressed motor neurons in PD, through TAM receptor-mediated “phagoptosis” of living neurons causing their death (Brown and Neher, 2012), thus accelerating PD progression (Fourgeaud et al., 2016). In this case, a beneficial microglial function was proposed to become detrimental upon disease.
Gain of Detrimental Inflammatory Functions
Midbrain DA neurons may be particularly vulnerable to detrimental microglial functions due to the abundance of these cells in this region (Lawson et al., 1990). This susceptibility is also conferred by the enrichment of DA neurons with iron, a redox active element, associated with antioxidant glutathione deficiency and monoamine oxidase activity, which all contribute to DA oxidation resulting in the production of reactive species (Block et al., 2007; Tansey and Goldberg, 2010; Wang and Michaelis, 2010). This susceptibility of the SN was further emphasized by the finding that local lipopolysaccharide (LPS; a bacterial component) injection into the SN, hippocampus or cerebral cortex of wild-type rats induced neuronal loss only in the SN (Kim et al., 2000). Further in vitro characterization of neuron-glial cultures identified a key role for microglia in the regional sensitivity to LPS. Indeed, supplementation of microglia into cortical neuron-glial cultures was sufficient to promote LPS-induced neurotoxicity (Kim et al., 2000). Microglia might over-produce pro-inflammatory mediators and reactive species, notably when performing phagocytosis, which could lead to neuronal damage and in turn contribute to sustaining inflammation in PD (Whitton, 2007; Tansey and Goldberg, 2010). In rat primary neuron-glial cell cultures, the exogenous application of α-synuclein aggregates induced microglial transformation into amoeboid cells, which produced reactive species resulting in DA neurons loss (Zhang et al., 2005). In vitro, neuromelanin (NM), a dark pigment formed by melanin that is found in catecholaminergic neurons (containing DA or norepinephrine) (Fedorow et al., 2005), induced loss of DA neurons when added to human primary mesencephalic neuron-glia cultures (Zhang et al., 2011). The phagocytic clearance of NM by microglia also induced the production of superoxide, nitric oxide, hydrogen peroxide and pro-inflammatory TNFα and IL-6, which could be prevented by genetic deletion of CR3 in vitro (Zhang et al., 2011). Besides NM, matrix metalloproteinase 3 (MMP3) and α-synuclein, which are released by degenerating DA neurons, promote microglial production of reactive species (Block et al., 2007).
Microglia in Aging and Chronic Stress
Normal Aging and Microglial Alterations
Aging is associated with an increased expression level of pro-inflammatory cytokines (e.g., IL-1β, IL-6, and TNFα) as well as decreased expression level of anti-inflammatory cytokines (e.g., IL-10) and anti-oxidants (e.g., glutathione levels) in rodent brain (Frank et al., 2006; Sierra et al., 2007; Ritzel et al., 2015). Furthermore, during aging, LPS-immune-challenged mice display an exacerbated inflammatory response (Godbout et al., 2005; Sierra et al., 2007; Njie et al., 2012). With relevance to PD, analyses of IBA1+ microglia in the SN and striatum of wild-type mice, from birth until 24 months of age, revealed that cellular density is decreased while clustering is increased after 18 months of age in both regions (Sharaf et al., 2013). Moreover, microglia underwent dystrophic morphological changes in the SN (see Figure 1), such as reduced ramifications, starting at 12 months (Sharaf et al., 2013). In aged rhesus monkeys, an increase in human leukocyte antigen-DR (HLA-DR), a component of MHC class II+ microglia, and similar morphological alterations were observed in the SN, together with an increased prevalence of amoeboid-shaped microglia, upon administration of the MPTP toxin (Kanaan et al., 2008).
Chronic Stress and Pathological Aging
Aging can induce important alterations of brain homeostasis notably through its effects on microglia. In addition, aging might become “pathological” under the influence of an environmental risk factor, such as chronic psychological stress, thus triggering disease onset and progression (Streit et al., 2014).
Chronic psychological stress can accelerate cellular aging by acting on both oxidative stress and inflammation (Tay et al., 2017; Tian et al., 2017). Upon stress, microglia become “primed” and show exaggerated response to a subsequent challenge (Cunningham et al., 2005; Frank et al., 2007). Other than the neuroinflammation changes, chronic restraint stress in otherwise healthy rodents was associated with a loss of neurons expressing tyrosine hydroxylase (TH), the enzyme that converts L-DOPA into DA, in the SN (Sugama et al., 2016; Ong et al., 2017). This TH+ neuronal loss correlated with an increase of insoluble α-synuclein monomers leading to the formation of aggregates and decreased numbers of IBA1+ microglia in the SN (Ong et al., 2017). Furthermore, when MPTP was administered after inducing stress, using the same paradigm, the loss of TH+ neurons in the SN was found to be more important in stressed rats than in unstressed littermates, which also displayed motor learning deficits (assessed with the rotarod) (Lauretti et al., 2016). However, different outcomes of stress on DA neurons were reported according to the type of stressor used and the brain region examined (Belujon and Grace, 2015). The overall evidence nevertheless suggests a close relationship between chronic stress and PD, which highlights the importance of investigating microglial changes as a contributing factor to PD pathophysiology.
Another subset of microglia, “dark microglia” identified by electron microscopy, was observed in adult mice exposed to maternal immune activation (Hui et al., 2018), chronic unpredictable stress, or aging (Bisht et al., 2016). Dark microglia display several markers of oxidative stress including a condensed cytoplasm and nucleoplasm, which led to their name, accompanied by dilation of the endoplasmic reticulum and Golgi apparatus, as well as mitochondrial alteration. They are highly ramified (see Figure 1) with their processes extensively encircling excitatory synapses and making direct contacts with synaptic clefts (Bisht et al., 2016) suggesting an involvement in synaptic remodeling under pathological or traumatic conditions. The involvement of dark microglia in PD still remains unknown, however.
Conclusion
Overall, various studies using in vivo and in vitro approaches have improved our knowledge of microglial involvement in PD despite the differences in paradigms and species used. Aging and chronic stress, two main environmental risk factors for PD, exacerbated inflammation and altered microglial functions. These changes might trigger pathological pathways notably in vulnerable CNS regions, such as the SN. Microglia produce both pro- and anti-inflammatory mediators, but upon neurodegeneration this tight equilibrium might get disrupted and become mainly pro-inflammatory. The death of DA neurons and associated reactive species production lead to pro-inflammatory and “phagoptotic” microglial phenotypes. Considering the inflammatory component of PD, it is important to study microglial implication with its onset, progression, and symptomatic treatment using L-DOPA for a translation to human patients. A better comprehension of the roles of different microglial phenotypes in PD might one day help to find anti-parkinsonian and/or anti-dyskinetic drugs targeting microglia.
Author Contributions
CL, MB, and M-ÈT designed the review outline and wrote a first version of the manuscript. MP, LC, and TD revised the manuscript and contributed to the subsequent versions.
Funding
CL and MB are recipients of master and doctoral training awards, respectively, from Fonds de Recherche du Québec – Santé (FRQS). MP, LC, TD, and M-ÈT are funded by the Canadian Institutes of Health Research (CIHR; #341846). M-ÈT is a Canada Research Chair (Tier 2) in Neuroimmune plasticity in health and therapy.
Conflict of Interest Statement
The authors declare that the research was conducted in the absence of any commercial or financial relationships that could be construed as a potential conflict of interest.
Acknowledgments
We are grateful to Dr. Giamal Luheshi for his critical revision of the manuscript.
References
Askew, K., Li, K., Olmos-Alonso, A., Garcia-Moreno, F., Liang, Y., Richardson, P., et al. (2017). Coupled proliferation and apoptosis maintain the rapid turnover of microglia in the adult brain. Cell Rep. 18, 391–405. doi: 10.1016/j.celrep.2016.12.041
Belujon, P., and Grace, A. A. (2015). Regulation of dopamine system responsivity and its adaptive and pathological response to stress. Proc. Soc. Biol. 282, 20142516. doi: 10.1098/rspb.2014.2516
Bisht, K., Sharma, K. P., Lecours, C., Gabriela Sánchez, M., El Hajj, H., Milior, G., et al. (2016). Dark microglia: a new phenotype predominantly associated with pathological states. Glia 64, 826–839. doi: 10.1002/glia.22966
Blesa, J., and Przedborski, S. (2014). Parkinson’s disease: animal models and dopaminergic cell vulnerability. Front. Neuroanat. 8:155. doi: 10.3389/fnana.2014.00155
Block, M. L., Zecca, L., and Hong, J.-S. (2007). Microglia-mediated neurotoxicity: uncovering the molecular mechanisms. Nat. Rev. Neurosci. 8, 57–69. doi: 10.1038/nrn2038
Brown, G. C., and Neher, J. J. (2012). Eaten alive! cell death by primary phagocytosis: ‘phagoptosis.’. Trends Biochem. Sci. 37, 325–332. doi: 10.1016/j.tibs.2012.05.002
Butovsky, O., Jedrychowski, M. P., Moore, C. S., Cialic, R., Lanser, A. J., Gabriely, G., et al. (2014). Identification of a unique TGF-β dependent molecular and functional signature in microglia. Nat. Neurosci. 17, 131–143. doi: 10.1038/nn.3599
Cunningham, C., Wilcockson, D. C., Campion, S., Lunnon, K., and Perry, V. H. (2005). Central and systemic endotoxin challenges exacerbate the local inflammatory response and increase neuronal death during chronic neurodegeneration. J. Neurosci. 25, 9275–9284. doi: 10.1523/JNEUROSCI.2614-05.2005
Ding, Y. M., Jaumotte, J. D., Signore, A. P., and Zigmond, M. J. (2004). Effects of 6-hydroxydopamine on primary cultures of substantia nigra: specific damage to dopamine neurons and the impact of glial cell line-derived neurotrophic factor. J. Neurochem. 89, 776–787. doi: 10.1111/j.1471-4159.2004.02415.x
Doorn, K. J., Moors, T., Drukarch, B., van de Berg, W. D., Lucassen, P. J., and van Dam, A.-M. (2014). Microglial phenotypes and toll-like receptor 2 in the substantia nigra and hippocampus of incidental Lewy body disease cases and Parkinson’s disease patients. Acta Neuropathol. Commun. 2:90. doi: 10.1186/s40478-014-0090-1
Fedorow, H., Tribl, F., Halliday, G., Gerlach, M., Riederer, P., and Double, K. L. (2005). Neuromelanin in human dopamine neurons: comparison with peripheral melanins and relevance to Parkinson’s disease. Prog. Neurobiol. 75, 109–124. doi: 10.1016/j.pneurobio.2005.02.001
Fourgeaud, L., Través, P. G., Tufail, Y., Leal-Bailey, H., Lew, E. D., Burrola, P. G., et al. (2016). TAM receptors regulate multiple features of microglial physiology. Nature 532, 240. doi: 10.1038/nature17630
Frank, M. G., Baratta, M. V., Sprunger, D. B., Watkins, L. R., and Maier, S. F. (2007). Microglia serve as a neuroimmune substrate for stress-induced potentiation of CNS pro-inflammatory cytokine responses. Brain. Behav. Immun. 21, 47–59. doi: 10.1016/j.bbi.2006.03.005
Frank, M. G., Barrientos, R. M., Biedenkapp, J. C., Rudy, J. W., Watkins, L. R., and Maier, S. F. (2006). mRNA up-regulation of MHC II and pivotal pro-inflammatory genes in normal brain aging. Neurobiol. Aging 27, 717–722. doi: 10.1016/j.neurobiolaging.2005.03.013
Gerhard, A., Pavese, N., Hotton, G., Turkheimer, F., Es, M., Hammers, A., et al. (2006). In vivo imaging of microglial activation with [11C](R)-PK11195 PET in idiopathic Parkinson’s disease. Neurobiol. Dis. 21, 404–412. doi: 10.1016/j.nbd.2005.08.002
Ginhoux, F., Greter, M., Leboeuf, M., Nandi, S., See, P., Gokhan, S., et al. (2010). Fate Mapping Analysis Reveals That Adult Microglia Derive from Primitive Macrophages. Science 330, 841–845. doi: 10.1126/science.1194637
Godbout, J. P., Chen, J., Abraham, J., Richwine, A. F., Berg, B. M., Kelley, K. W., et al. (2005). Exaggerated neuroinflammation and sickness behavior in aged mice following activation of the peripheral innate immune system. FASEB J. 19, 1329–1331. doi: 10.1096/fj.05-3776fje
Gomez, P. E., Klapproth, K., Schulz, C., Busch, K., Azzoni, E., Crozet, L., et al. (2015). Tissue-resident macrophages originate from yolk-sac-derived erythro-myeloid progenitors. Nature 518, 547–551. doi: 10.1038/nature13989
Gosselin, D., Skola, D., Coufal, N. G., Holtman, I. R., Schlachetzki, J. C. M., Sajti, E., et al. (2017). An environment-dependent transcriptional network specifies human microglia identity. Science 356:eaal3222. doi: 10.1126/science.aal3222
Grow, D. A., McCarrey, J. R., and Navara, C. S. (2016). Advantages of nonhuman primates as preclinical models for evaluating stem cell-based therapies for Parkinson’s disease. Stem Cell Res. 17, 352–366. doi: 10.1016/j.scr.2016.08.013
Hanamsagar, R., Alter, M. D., Block Carina, S., Sullivan, H., Bolton, J. L., and Bilbo Staci, D. (2017). Generation of a microglial developmental index in mice and in humans reveals a sex difference in maturation and immune reactivity. Glia 65, 1504–1520. doi: 10.1002/glia.23176
Hashimoto, D., Chow, A., Noizat, C., Teo, P., Beasley, M. B., Leboeuf, M., et al. (2013). Tissue resident macrophages self-maintain locally throughout adult life with minimal contribution from circulating monocytes. Immunity 38, 792–804. doi: 10.1016/j.immuni.2013.04.004
He, Y., Le, W.-D., and Appel, S. H. (2002). Role of Fcgamma receptors in nigral cell injury induced by Parkinson disease immunoglobulin injection into mouse substantia nigra. Exp. Neurol. 176, 322–327.
Hirsch, E. C., and Hunot, S. (2009). Neuroinflammation in Parkinson’s disease: a target for neuroprotection? Lancet Neurol. 8, 382–397. doi: 10.1016/S1474-4422(09)70062-6
Hoeffel, G., Chen, J., Lavin, Y., Low, D., Almeida, F. F., See, P., et al. (2015). C-Myb+ Erythro-Myeloid progenitor-derived fetal monocytes give rise to adult tissue-resident macrophages. Immunity 42, 665–678. doi: 10.1016/j.immuni.2015.03.011
Hui, C. W., St-Pierre, A., El Hajj, H., Remy, Y., Hébert, S. S., Luheshi, G. N., et al. (2018). Prenatal immune challenge in mice leads to partly sex-dependent behavioral, microglial, and molecular abnormalities associated with schizophrenia. Front. Mol. Neurosci. 11:13. doi: 10.3389/fnmol.2018.00013
Hurley, S. D., O’Banion, M. K., Song, D. D., Arana, F. S., Olschowka, J. A., and Haber, S. N. (2003). Microglial response is poorly correlated with neurodegeneration following chronic, low-dose MPTP administration in monkeys. Exp. Neurol. 184, 659–668. doi: 10.1016/S0014-4886(03)00273-5
Imamura, K., Hishikawa, N., Sawada, M., Nagatsu, T., Yoshida, M., and Hashizume, Y. (2003). Distribution of major histocompatibility complex class II-positive microglia and cytokine profile of Parkinson’s disease brains. Acta Neuropathol. 106, 518–526. doi: 10.1007/s00401-003-0766-2
Jagmag, S. A., Tripathi, N., Shukla, S. D., Maiti, S., and Khurana, S. (2016). Evaluation of models of Parkinson’s disease. Front. Neurosci. 9:503. doi: 10.3389/fnins.2015.00503
Jankovic, J. (2008). Parkinson’s disease: clinical features and diagnosis. J. Neurol. Neurosurg. Psychiatry 79, 368–376. doi: 10.1136/jnnp.2007.131045
Kanaan, N. M., Kordower, J. H., and Collier, T. J. (2008). Age and region-specific responses of microglia, but not astrocytes, suggest a role in selective vulnerability of dopamine neurons after 1-methyl-4-phenyl-1,2,3,6-tetrahydropyridine exposure in monkeys. Glia 56, 1199–1214. doi: 10.1002/glia.20690
Kaur, C., Rathnasamy, G., and Ling, E.-A. (2017). Biology of Microglia in the Developing Brain. J. Neuropathol. Exp. Neurol. 76, 736–753. doi: 10.1093/jnen/nlx056
Kierdorf, K., and Prinz, M. (2013). Factors regulating microglia activation. Front. Cell. Neurosci. 7:44. doi: 10.3389/fncel.2013.00044
Kim, W. G., Mohney, R. P., Wilson, B., Jeohn, G. H., Liu, B., and Hong, J. S. (2000). Regional difference in susceptibility to lipopolysaccharide-induced neurotoxicity in the rat brain: role of microglia. J. Neurosci 20,6309–6316.
Knott, C., Wilkin, G. P., and Stern, G. (1999). Astrocytes and microglia in the substantia nigra and caudate-putamen in Parkinson’s disease. Parkinsonism Relat. Disord. 5, 115–122.
Kurkowska-Jastrzebska, I., Wrońska, A., Kohutnicka, M., Członkowski, A., and Członkowska, A. (1999). The inflammatory reaction following 1-methyl-4-phenyl-1,2,3, 6-tetrahydropyridine intoxication in mouse. Exp. Neurol. 156, 50–61. doi: 10.1006/exnr.1998.6993
Lauretti, E., Di Meco, A., Merali, S., and Praticò, D. (2016). Chronic behavioral stress exaggerates motor deficit and neuroinflammation in the MPTP mouse model of Parkinson’s disease. Transl. Psychiatry 6:e733. doi: 10.1038/tp.2016.1
Lawson, L. J., Perry, V. H., Dri, P., and Gordon, S. (1990). Heterogeneity in the distribution and morphology of microglia in the normal adult mouse brain. Neuroscience 39, 151–170.
Le, W., Wu, J., and Tang, Y. (2016). Protective microglia and their regulation in Parkinson’s disease. Front. Mol. Neurosci. 9:89. doi: 10.3389/fnmol.2016.00089
Li, Q., and Barres, B. A. (2017). Microglia and macrophages in brain homeostasis and disease. Nat. Rev. Immunol 18, 225–242. doi: 10.1038/nri.2017.125
Litteljohn, D., and Hayley, S. (2012). Cytokines as Potential Biomarkers for Parkinson’s Disease: a multiplex approach. Methods Mol. Biol. 934, 121–144. doi: 10.1007/978-1-62703-071-7_7
Low, D., and Ginhoux, F. (2018). Recent advances in the understanding of microglial development and homeostasis. Cell. Immunol doi: 10.1016/j.cellimm.2018.01.004
McGeer, P. L., Itagaki, S., Boyes, B. E., and McGeer, E. G. (1988). Reactive microglia are positive for HLA-DR in the substantia nigra of Parkinson’s and Alzheimer’s disease brains. Neurology 38, 1285–1291.
Mercuri, N. B., and Bernardi, G. (2005). The “magic” of L-dopa: why is it the gold standard Parkinson’s disease therapy? Trends Pharmacol. Sci. 26, 341–344. doi: 10.1016/j.tips.2005.05.002
Mirza, B., Hadberg, H., Thomsen, P., and Moos, T. (2000). The absence of reactive astrocytosis is indicative of a unique inflammatory process in Parkinson’s disease. Neuroscience 95, 425–432.
Mondelli, V., Vernon, A. C., Turkheimer, F., Dazzan, P., and Pariante, C. M. (2017). Brain microglia in psychiatric disorders. Lancet Psychiatry 4, 563–572. doi: 10.1016/S2215-0366(17)30101-3
Morin, N., Jourdain, V. A., and Di Paolo, T. (2014). Modeling dyskinesia in animal models of Parkinson disease. Exp. Neurol. 256, 105–116. doi: 10.1016/j.expneurol.2013.01.024
Morissette, M., and Di Paolo, T. (2018). Non-human primate models of PD to test novel therapies. J. Neural Transm. 1996, 291–324. doi: 10.1007/s00702-017-1722-y
Mount, M. P., Lira, A., Grimes, D., Smith, P. D., Faucher, S., Slack, R., et al. (2007). Involvement of interferon-gamma in microglial-mediated loss of dopaminergic neurons. J. Neurosci. 27, 3328–3337. doi: 10.1523/JNEUROSCI.5321-06.2007
Nagatsu, T., Mogi, M., Ichinose, H., and Togari, A. (2000). Cytokines in Parkinson’s Disease Advances in Research on Neurodegeneration. Vienna: Springer, 143–151. doi: 10.1007/978-3-7091-6284-2_12
Nagatsu, T., and Sawada, M. (2005). Inflammatory process in Parkinson’s disease: role for cytokines. Curr. Pharm. Des. 11, 999–1016.
Nam, J. H., Leem, E., Jeon, M.-T., Jeong, K. H., Park, J.-W., Jung, U. J., et al. (2015). Induction of GDNF and BDNF by hRheb(S16H) transduction of SNpc neurons: neuroprotective mechanisms of hRheb(S16H) in a model of Parkinson’s disease. Mol. Neurobiol. 51, 487–499. doi: 10.1007/s12035-014-8729-2
Nayak, D., Roth, T. L., and McGavern, D. B. (2014). Microglia development and function. Annu. Rev. Immunol. 32, 367–402. doi: 10.1146/annurev-immunol-032713-120240
Niraula, A., Sheridan, J. F., and Godbout, J. P. (2017). Microglia priming with aging and stress. Neuropsychopharmacology 42, 318–333. doi: 10.1038/npp.2016.185
Njie, E. G., Boelen, E., Stassen, F. R., Steinbusch, H. W. M., Borchelt, D. R., and Streit, W. J. (2012). Ex vivo cultures of microglia from young and aged rodent brain reveal age-related changes in microglial function. Neurobiol. Aging 33, 195.e1–195.e12. doi: 10.1016/j.neurobiolaging.2010.05.008
Ong, L. K., Zhao, Z., Kluge, M., TeBay, C., Zalewska, K., Dickson, P. W., et al. (2017). Reconsidering the role of glial cells in chronic stress-induced dopaminergic neurons loss within the substantia nigra? Friend or foe? Brain. Behav. Immun. 60, 117–125. doi: 10.1016/j.bbi.2016.10.001
Ouchi, Y., Yoshikawa, E., Sekine, Y., Futatsubashi, M., Kanno, T., Ogusu, T., et al. (2005). Microglial activation and dopamine terminal loss in early Parkinson’s disease. Ann. Neurol. 57, 168–175. doi: 10.1002/ana.20338
Paolicelli, R. C., and Ferretti, M. T. (2017). Function and dysfunction of microglia during brain development: consequences for synapses and neural circuits. Front. Synaptic Neurosci. 9:9. doi: 10.3389/fnsyn.2017.00009
Parkhurst, C. N., Yang, G., Ninan, I., Savas, J. N., Yates, J. R., Lafaille, J. J., et al. (2013). Microglia promote learning-dependent synapse formation through BDNF. Cell 155, 1596–1609. doi: 10.1016/j.cell.2013.11.030
Poewe, W. (2008). Non-motor symptoms in Parkinson’s disease. Eur. J. Neurol. 15(Suppl. 1), 14–20. doi: 10.1111/j.1468-1331.2008.02056.x
Ritzel, R. M., Patel, A. R., Pan, S., Crapser, J., Hammond, M., Jellison, E., et al. (2015). Age- and location-related changes in microglial function. Neurobiol. Aging 36, 2153–2163. doi: 10.1016/j.neurobiolaging.2015.02.016
Schafer, D. P., Lehrman, E. K., Kautzman, A. G., Koyama, R., Mardinly, A. R., Yamasaki, R., et al. (2012). Microglia sculpt postnatal neural circuits in an activity and complement-dependent manner. Neuron 74, 691–705. doi: 10.1016/j.neuron.2012.03.026
Schafer, D. P., and Stevens, B. (2015). Microglia function in central nervous system development and plasticity. Cold Spring Harb. Perspect. Biol. 7:a020545. doi: 10.1101/cshperspect.a020545
Schapira, A. H., and Jenner, P. (2011). Etiology and pathogenesis of Parkinson’s disease. Mov. Disord. 26, 1049–1055. doi: 10.1002/mds.23732
Schulz, C., Perdiguero, E. G., Chorro, L., Szabo-Rogers, H., Cagnard, N., Kierdorf, K., et al. (2012). A lineage of myeloid cells independent of Myb and hematopoietic stem cells. Science 336, 86–90. doi: 10.1126/science.1219179
Sharaf, A., Krieglstein, K., and Spittau, B. (2013). Distribution of microglia in the postnatal murine nigrostriatal system. Cell Tissue Res. 351, 373–382. doi: 10.1007/s00441-012-1537-y
Sherer, T. B., Betarbet, R., Kim, J. H., and Greenamyre, J. T. (2003). Selective microglial activation in the rat rotenone model of Parkinson’s disease. Neurosci. Lett. 341, 87–90.
Sierra, A., Gottfried-Blackmore, A. C., McEwen, B. S., and Bulloch, K. (2007). Microglia derived from aging mice exhibit an altered inflammatory profile. Glia 55, 412–424. doi: 10.1002/glia.20468
Streit, W. J., Xue, Q.-S., Tischer, J., and Bechmann, I. (2014). Microglial pathology. Acta Neuropathol. Commun. 2:142. doi: 10.1186/s40478-014-0142-6
Sugama, S., Sekiyama, K., Kodama, T., Takamatsu, Y., Takenouchi, T., Hashimoto, M., et al. (2016). Chronic restraint stress triggers dopaminergic and noradrenergic neurodegeneration: possible role of chronic stress in the onset of Parkinson’s disease. Brain. Behav. Immun. 51, 39–46. doi: 10.1016/j.bbi.2015.08.015
Tansey, M. G., and Goldberg, M. S. (2010). Neuroinflammation in Parkinson’s disease: its role in neuronal death and implications for therapeutic intervention. Neurobiol. Dis. 37, 510–518. doi: 10.1016/j.nbd.2009.11.004
Tay, T. L., Béchade, C., D’Andrea, I., St-Pierre, M.-K., Henry, M. S., Roumier, A., et al. (2018). Microglia gone rogue: impacts on psychiatric disorders across the lifespan. Front. Mol. Neurosci. 10:421. doi: 10.3389/fnmol.2017.00421
Tay, T. L., Savage, J. C., Hui, C. W., Bisht, K., and Tremblay, M. (2017). Microglia across the lifespan: from origin to function in brain development, plasticity and cognition. J. Physiol. 595, 1929–1945. doi: 10.1113/JP272134
Tian, L., Hui, C. W., Bisht, K., Tan, Y., Sharma, K., Chen, S., et al. (2017). Microglia under psychosocial stressors along the aging trajectory: consequences on neuronal circuits, behavior, and brain diseases. Prog. Neuropsychopharmacol. Biol. Psychiatry 79, 27–39. doi: 10.1016/j.pnpbp.2017.01.007
Tieu, K. (2011). A guide to neurotoxic Animal models of Parkinson’s disease. Cold Spring Harb. Perspect. Med. 1:a009316. doi: 10.1101/cshperspect.a009316
Tremblay, M. -È, Stevens, B., Sierra, A., Wake, H., Bessis, A., and Nimmerjahn, A. (2011). The role of microglia in the healthy brain. J. Neurosci. 31, 16064–16069. doi: 10.1523/JNEUROSCI.4158-11.2011
Tysnes, O.-B., and Storstein, A. (2017). Epidemiology of Parkinson’s disease. J. Neural Transm. 124, 901–905. doi: 10.1007/s00702-017-1686-y
Vawter, M. P., Dillon-Carter, O., Tourtellotte, W. W., Carvey, P., and Freed, W. J. (1996). TGFbeta1 and TGFbeta2 concentrations are elevated in Parkinson’s disease in ventricular cerebrospinal fluid. Exp. Neurol. 142, 313–322. doi: 10.1006/exnr.1996.0200
Vyas, S., Rodrigues, A. J., Silva, J. M., Tronche, F., Almeida, O. F. X., Sousa, N., et al. (2016). Chronic stress and glucocorticoids: from neuronal plasticity to neurodegeneration. Neural Plast. 2016, 6391686. doi: 10.1155/2016/6391686
Wang, X., and Michaelis, E. K. (2010). Selective neuronal vulnerability to oxidative stress in the brain. Front. Aging Neurosci. 2:12. doi: 10.3389/fnagi.2010.00012
Weenink, S. M., and Gautam, A. M. (1997). Antigen presentation by MHC class II molecules. Immunol. Cell Biol. 75, 69–81. doi: 10.1038/icb.1997.11
Whitton, P. S. (2007). Inflammation as a causative factor in the aetiology of Parkinson’s disease. Br. J. Pharmacol. 150, 963. doi: 10.1038/sj.bjp.0707167
Wlodarczyk, A., Holtman, I. R., Krueger, M., Yogev, N., Bruttger, J., Khorooshi, R., et al. (2017). A novel microglial subset plays a key role in myelinogenesis in developing brain. EMBO J. 36, 3292–3308. doi: 10.15252/embj.201696056
Wu, D. C., Jackson-Lewis, V., Vila, M., Tieu, K., Teismann, P., Vadseth, C., et al. (2002). Blockade of microglial activation is neuroprotective in the 1-methyl-4-phenyl-1,2,3,6-tetrahydropyridine mouse model of Parkinson disease. J. Neurosci. 22, 1763–1771.
Zhang, W., Phillips, K., Wielgus, A. R., Liu, J., Albertini, A., Zucca, F. A., et al. (2011). Neuromelanin activates microglia and induces degeneration of dopaminergic neurons: implications for progression of Parkinson’s disease. Neurotox. Res. 19, 63–72. doi: 10.1007/s12640-009-9140-z
Keywords: aging, chronic stress, inflammation, microglia, phagocytosis, Parkinson’s disease
Citation: Lecours C, Bordeleau M, Cantin L, Parent M, Di Paolo T and Tremblay M-È (2018) Microglial Implication in Parkinson’s Disease: Loss of Beneficial Physiological Roles or Gain of Inflammatory Functions? Front. Cell. Neurosci. 12:282. doi: 10.3389/fncel.2018.00282
Received: 31 May 2018; Accepted: 09 August 2018;
Published: 30 August 2018.
Edited by:
Jose Luis Venero, Universidad de Sevilla, SpainCopyright © 2018 Lecours, Bordeleau, Cantin, Parent, Di Paolo and Tremblay. This is an open-access article distributed under the terms of the Creative Commons Attribution License (CC BY). The use, distribution or reproduction in other forums is permitted, provided the original author(s) and the copyright owner(s) are credited and that the original publication in this journal is cited, in accordance with accepted academic practice. No use, distribution or reproduction is permitted which does not comply with these terms.
*Correspondence: Marie-Ève Tremblay, tremblay.marie-eve@crchudequebec.ulaval.ca; tremblay0202@gmail.com