Synaptic Organizers in Alzheimer’s Disease: A Classification Based on Amyloid-β Sensitivity
- 1Synapse Development and Plasticity Research Unit, Institut de Recherches Cliniques de Montréal (IRCM), Montreal, QC, Canada
- 2Integrated Program in Neuroscience, McGill University, Montreal, QC, Canada
- 3Molecular Biology Program, Université de Montréal, Montréal, QC, Canada
- 4Department of Medicine, Université de Montréal, Montreal, QC, Canada
- 5Division of Experimental Medicine, McGill University, Montreal, QC, Canada
Synaptic pathology is one of the major hallmarks observed from the early stage of Alzheimer’s disease (AD), leading to cognitive and memory impairment characteristic of AD patients. Synaptic connectivity and specificity are regulated by multiple trans-bindings between pre- and post-synaptic organizers, the complex of which exerts synaptogenic activity. Neurexins (NRXs) and Leukocyte common antigen-related receptor protein tyrosine phosphatases (LAR-RPTPs) are the major presynaptic organizers promoting synaptogenesis through their distinct binding to a wide array of postsynaptic organizers. Recent studies have shown that amyloid-β oligomers (AβOs), a major detrimental molecule in AD, interact with NRXs and neuroligin-1, an NRX-binding postsynaptic organizer, to cause synaptic impairment. On the other hand, LAR-RPTPs and their postsynaptic binding partners have no interaction with AβOs, and their synaptogenic activity is maintained even in the presence of AβOs. Here, we review the current evidence regarding the involvement of synaptic organizers in AD, with a focus on Aβ synaptic pathology, to propose a new classification where NRX-based and LAR-RPTP-based synaptic organizing complexes are classified into Aβ-sensitive and Aβ-insensitive synaptic organizers, respectively. We further discuss how their different Aβ sensitivity is involved in Aβ vulnerability and tolerance of synapses for exploring potential therapeutic approaches for AD.
Introduction
Alzheimer’s disease (AD), the most common age-related neurodegenerative disease with progressive cognitive decline including memory loss, has seen a sharp increase in the number of cases and AD-related deaths over the past decades. Although some therapies are clinically applied to AD patients, at best they slightly delay the disease progression and temporarily improve some symptoms (Weller and Budson, 2018; Long and Holtzman, 2019). Thus, a deeper understanding of the mechanisms involved in AD development and progression is indispensable for establishing better treatments for this disease.
There are two major pathohistological hallmarks of the AD brain: extracellular senile plaques and intracellular neurofibrillary tangles (NFT), the major constituents of which are amyloid β (Aβ) peptides and hyper-phosphorylated tau proteins, respectively (Ballard et al., 2011; DeTure and Dickson, 2019). Aβ has been reported to be a key detrimental molecule that plays a major role in AD pathogenesis (Reiss et al., 2018). Aβ is produced from the cleavage of amyloid precursor protein (APP) by β- and γ-secretases (Haass and Selkoe, 2007; O’Brien and Wong, 2011), after which it is secreted to the extracellular space and forms oligomers. Aβ oligomers (AβOs) are thought to be toxic for neurons and their synaptic connections in AD patient brains (Haass and Selkoe, 2007; Sheng et al., 2012). Indeed, many in vitro studies using primary neuron cultures (Parodi et al., 2010; He et al., 2019), brain slices (Hsieh et al., 2006; Shankar et al., 2007; Li et al., 2011) and in vivo studies (Spires-Jones et al., 2007; Hong et al., 2016) using AD model mouse lines overproducing Aβ (e.g., J20 and Tg2576) have supported the toxic effects of AβOs by showing Aβ-induced synaptic loss, decreased presynaptic release probability and impaired postsynaptic long-term potentiation (LTP), which is synaptic plasticity depending on postsynaptic N-Methyl-D-aspartate-type glutamate receptor (NMDAR)-mediated pathways (Nicoll, 2017). According to previous studies, Aβ pathology seems to precede tau pathology and importantly to start even from preclinical AD stage (Jansen et al., 2015; Sasaguri et al., 2017; van der Kant et al., 2020). Furthermore, synapse loss is an early pathological feature of AD and one of the best correlates of cognitive impairment (Scheff and Price, 2003; Sheng et al., 2012). These suggest the importance of understanding the mechanism of Aβ synaptic pathology.
When neurons establish synaptic connections in the brain, many neuronal adhesion molecules mediate physical connections between axons and target neurons (Li and Sheng, 2003; Waites et al., 2005; Dalva et al., 2007). Importantly, a specific subset of the adhesion molecules has a further biological activity called “synaptogenic activity,” by which they promote pre- and/or post-synaptic organization to make synapses functional for neurotransmitter release and reception (Siddiqui and Craig, 2011; Missler et al., 2012). Such synaptogenic adhesion molecules have been called “synaptic organizers.” In general, their trans-synaptic complexes (herein called “synaptic organizing complexes”) drive bidirectional trans-cellular synaptogenic signals: (i) a retrograde signal from the target neuron to trigger the clustering of synaptic vesicles and assembly of the fusion apparatus on the axon; and (ii) an anterograde signal from the axon to trigger postsynaptic clustering of neurotransmitter receptors including α-amino-3-hydroxy-5-methyl-4-isoxazolepropionic acid-type glutamate receptors (AMPARs) and NMDARs and scaffolding molecules on the target neuron (Siddiqui and Craig, 2011; Missler et al., 2012). Such synaptogenic activities can be assessed by an artificial synapse formation assay based on co-culturing primary neurons with non-neuronal cells (e.g., COS-7 and HEK293 cells) transfected with the gene of interest (Craig et al., 2006). Numerous efforts over the years have identified and characterized many synaptic organizers, which can be grouped into two major categories; either neurexin (NRX)-based or Leukocyte common antigen-related receptor protein tyrosine phosphatases (LAR-RPTPs: composed of PTPσ, PTPδ as well as LAR)-based synaptic organizing complexes. NRXs and LAR-RPTPs act as presynaptic molecular hubs to trans-synaptically regulate synapse structure and function by making multiple trans-interactions with their specific postsynaptic organizers, such as NRX-neuroligin (NLGN), NRX-leucine-rich-repeat transmembrane neuronal proteins (LRRTMs), PTPσ-neurotrophin receptor tropomyosin-related kinase C (TrkC), PTPσ/δ-Slit and Trk-like proteins (Slitrks), LAR-netrin-G-ligand 3 (NGL3) and so on (Takahashi and Craig, 2013; Südhof, 2017; Figure 1). The most well-studied synaptic organizing complex is the NRX-NLGN complex, essential for synapse organization, transmission and plasticity as well as genetically linked with cognitive disorders such as autism spectrum disorders (ASD) and schizophrenia (Craig and Kang, 2007; Südhof, 2008, 2017; Kasem et al., 2018). Given the evidence of synaptic impairments in AD, recent studies have been trying to test whether and how Aβ interferes with synaptic organizers because of their pivotal roles in synapse physiology and cognitive function. Interestingly, our recent study has identified NRXs as a direct binding protein of AβOs (Naito et al., 2017b). Other groups have further uncovered the binding of Aβ with NLGN1 (Dinamarca et al., 2011; Brito-Moreira et al., 2017). This highlights the importance of studying the roles of synaptic organizers in the Aβ pathology of AD.
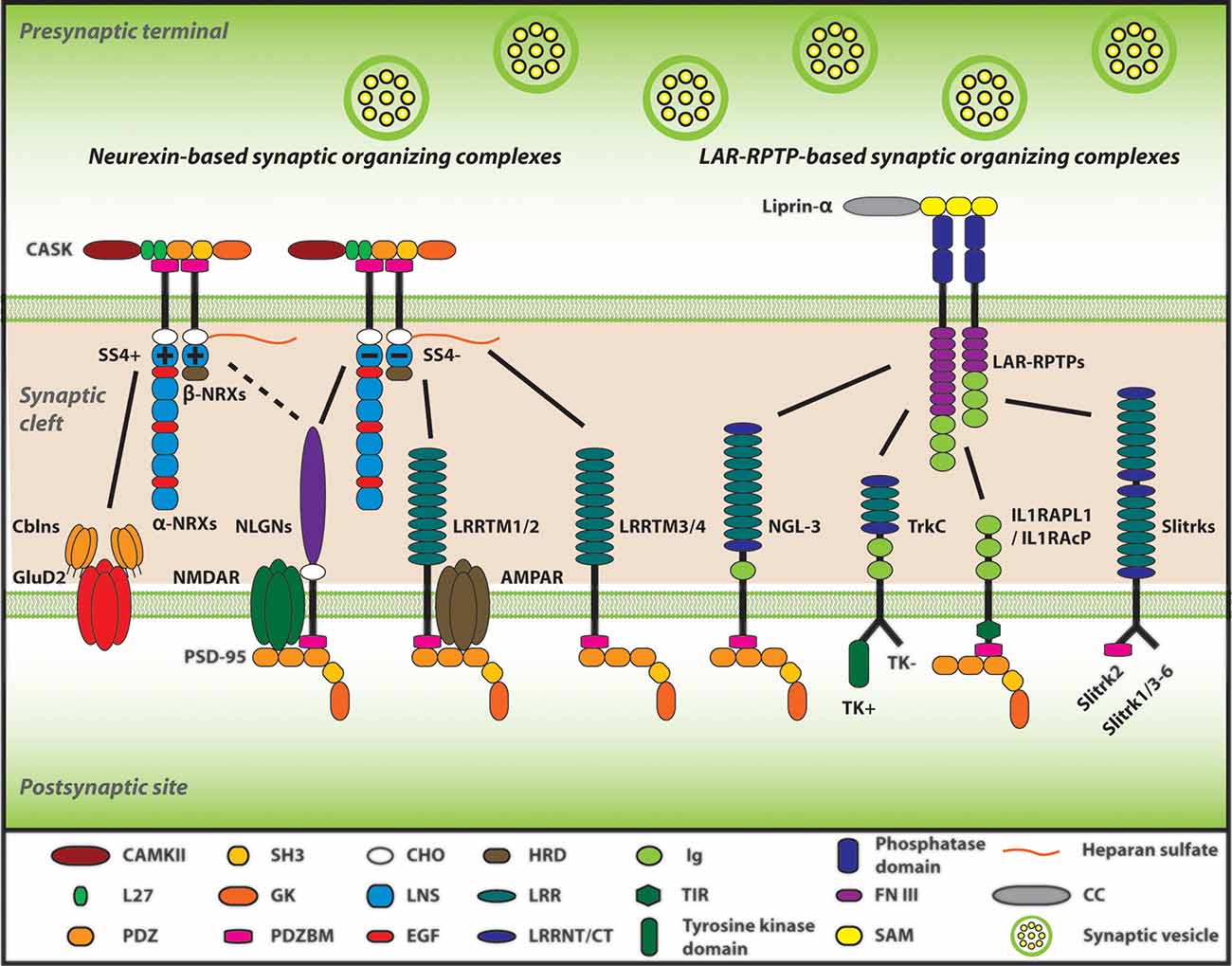
Figure 1. Neurexins (NRXs) and Leukocyte common antigen-related receptor protein tyrosine phosphatases (LAR-RPTPs) serve as presynaptic hubs to orchestrate synapse organization. The trans-interaction between pre- and post-synaptic organizers generate retrograde and anterograde “synaptogenic” signals through the synaptic cleft, resulting in the presynaptic organization, including synaptic vesicles clustering, and in the postsynaptic organization, encompassing the recruitment of neurotransmitter receptors [e.g., AMPA-type and NMDA-type glutamate receptor (AMPAR and NMDAR)] and scaffold proteins (e.g., PSD-95). As presynaptic molecular hubs, NRXs and LAR-RPTPs trans-synaptically interact with multiple specific postsynaptic organizers, for instance, NRXs-neuroligins (NLGNs), NRXs-leucine-rich-repeat transmembrane neuronal proteins (LRRTMs), PTPσ-neurotrophin receptor tropomyosin-related kinase C (TrkC), PTPσ/δ-Slit and Trk-like proteins (Slitrks), along with others. Furthermore, SS4 insertion modulates NRX interactome by regulating its binding properties with its diverse ligands. Solid lines indicate protein interactions, and the dashed line indicates that the insertion of SS4 in NRXs weakens NRX-NLGN interaction.
In this review, we first review the physiological synaptic roles of NRX-based and LAR-RPTP-based synaptic organizing complexes, which are closely relevant to AD synaptic pathology. We then review the emerging evidence of how synaptic organizers are involved in AD pathology, mainly focusing on Aβ pathology. Furthermore, considering their capability of Aβ binding (Dinamarca et al., 2011; Brito-Moreira et al., 2017; Naito et al., 2017b), we propose a new classification of synaptic organizers divided into two groups: Aβ-sensitive and Aβ-insensitive organizers, and discuss their implications in Aβ vulnerability and tolerance of synapses in AD. This would be essential to better understand the mechanisms involved in AD progression and give some insights into the development of novel therapeutic approaches for AD.
Neurexin-Based Synaptic Organizing Complexes
Neurexin
NRX is one of the most well understood presynaptic organizers. NRX is composed of six laminin/neurexin/sex-hormone-binding globulin (LNS) domains and three epidermal growth factor (EGF) domains, and additionally is one of the biggest genes existing in mammals (Missler and Südhof, 1998; Reissner et al., 2013). In mammals, NRX has three isoforms existing in different gene loci; NRXN1, NRXN2, and NRXN3 (Südhof, 2008; Reissner et al., 2013). Moreover, each of these NRXN genes contains two alternative promoters leading to two different sizes: α-NRX, the longer isoform containing all six LNS domains, and β-NRX, the shorter isoform composed of only one LNS domain (identical to the sixth LNS domain of each α-NRX) as well as a unique short amino acid sequence at the N-terminal called histidine-rich domain (HRD; Südhof, 2008; Reissner et al., 2013). Using the common LNS domain, α/β-NRXs trans-synaptically interact with many postsynaptic organizers such as NLGNs and LRRTMs to act as a major presynaptic hub (Reissner et al., 2013; Südhof, 2017; Figure 1). The mRNA coding individual NRXs are broadly expressed in the brain in both overlapping and differential patterns (Uchigashima et al., 2019). For example, in the hippocampus, NRXN1/2/3α and 2β are highly expressed in all CA1/2/3 and dentate gyrus (DG) regions and NRXN1β is highly expressed in these regions except the CA1, although NRXN3β displays modulate and weak expression in the CA1–CA3 and DG, respectively (Uchigashima et al., 2019). At the synapse level, both α-NRXs and β-NRXs are thought to exist in both excitatory (glutamatergic) and inhibitory (GABAergic) synapses (Craig and Kang, 2007; Uchigashima et al., 2019). Although α-NRX has a higher expression level in comparison to β-NRX, β-NRX is more enriched at excitatory synapses (Neupert et al., 2015). Meanwhile, α-NRX and β-NRX expression levels have no significant difference at inhibitory synapses (Neupert et al., 2015). These suggest that both α-NRX and β-NRX play important roles at synapses. Artificial synapse formation assays have shown that β-NRXs can exert synaptogenic activity to induce postsynaptic organization for both excitatory and inhibitory synapses, whereas α-NRXs can induce postsynaptic organization for only inhibitory synapses, suggesting their different roles in synapse organization (Graf et al., 2004; Kang et al., 2008).
Triple-α-NRX knockout (KO) mice show a decrease in the neurotransmitter release from excitatory and inhibitory synapses by impairing presynaptic calcium channel function, despite reducing only inhibitory synapse number (Missler et al., 2003). Behavioral experiments on global KO mice for NRX1α or NRX2α have exhibited cognitive impairments similar to neurological symptoms of ASD and Schizophrenia (Etherton et al., 2009; Grayton et al., 2013; Dachtler et al., 2014, 2015). On the other hand, triple-β-NRX KO decreases excitatory synapse release probability via synaptic endocannabinoid signaling, leading to the impairment of presynaptic LTP and contextual memory (Anderson et al., 2015). These suggest that α/β-NRXs differently regulate synaptic functions and are indispensable for normal cognitive functions.
Also, each NRX has six alternative splicing sites (SS1-6) that regulate its binding properties with its binding partners (Tabuchi and Südhof, 2002; Treutlein et al., 2014; Südhof, 2017). Most of the studies on the NRX splicing sites so far have focused on addressing the roles of SS4. SS4 inclusion in NRX decreases its interaction with NLGN1 and loses its interaction with LRRTM2 (Koehnke et al., 2008; Ko et al., 2009a; Yamagata et al., 2018). On the other hand, at the parallel fiber-Purkinje cell synapses in the cerebellum, SS4 inclusion allows NRX to interact with cerebellin 1 (Cbln1) to make a triad complex with postsynaptic δ2 glutamate receptor (GluD2), which regulates the formation of this type of synapses and motor functions (Matsuda et al., 2010; Uemura et al., 2010). At the hippocampal CA1-subiculum synapses, SS4 insertion in NRX1 enhances NMDAR-mediated response, whereas SS4 insertion in NRX3 suppresses AMPAR-mediated response (Aoto et al., 2013; Dai et al., 2019). Thus, SS4 of NRX1 and NRX3 regulate different synaptic properties, even though NRX1 and NRX3 are supposed to largely share the same binding partners. Taken together, given the exceptional variety of NRX transcript variants expressed from three different genes with two independent promoters and six alternative splicings including SS4 (Reissner et al., 2013; Treutlein et al., 2014), the distinct roles of α/β-NRXs and those of NRX1/3 SS4 splicing have suggested that NRX variety may underlie the diversity and complexity of brain synaptic function and cognitive function.
Neuroligin
NLGN has been well studied as one of the major NRX-interacting postsynaptic organizers (Bemben et al., 2015). NLGN has five subtypes: NLGN1-3, 4X, and 4Y (Bemben et al., 2015). In adult mouse brains, NLGN1/2/3 mRNA is expressed in almost all neuronal populations with a different pattern, in which NLGN2/3 mRNA expression is relatively higher than NLGN1 in the brainstem, hypothalamus, and thalamus (Varoqueaux et al., 2006). In contrast, NLGN4X and 4Y mRNA expression are very low in the human brain (Bolliger et al., 2001; Jamain et al., 2003). At the synapse level, NLGN1 and NLGN2 are mostly localized at excitatory and inhibitory synapses, respectively, whereas NLGN3 is localized at both excitatory and inhibitory synapses (Song et al., 1999; Varoqueaux et al., 2004; Budreck and Scheiffele, 2007). Artificial synapse formation assays have shown that NLGNs have a synaptogenic activity to induce presynaptic organization of excitatory and inhibitory synapses (Scheiffele et al., 2000; Graf et al., 2004; Chubykin et al., 2005; Craig et al., 2006; Naito et al., 2017b) through their trans-interaction with presynaptic NRXs (Ko et al., 2009b; Gokce and Südhof, 2013). Further, a recent study using NLGN1-4 conditional KO mouse brain slices with rescue experiments has shown that the NLGN1 extracellular domain, particularly its trans-interaction with presynaptic NRXs, is crucial for LTP (Wu et al., 2019). The extracellular domain of NLGN, mainly composed of acetylcholinesterase (ACE)-like domain, binds to the LNS6 domain of NRX in a calcium-dependent manner (Nguyen and Südhof, 1997; Südhof, 2008; Bemben et al., 2015). The ACE-like domain of NLGN contains an alternative splicing site that regulates their binding properties with NRXs, with exception to NLGN1 that has two alternative splicing sites (A and B; Chih et al., 2006; Ko et al., 2009a). Also, NLGN1 can form a complex with the major postsynaptic scaffold protein PSD-95 by its intracellular C-terminal tail, and this NLGN1-PSD-95 interaction is thought to be involved in postsynaptic molecular assembly (Irie et al., 1997). Indeed, NMDAR-mediated synaptic transmission is required for the intracellular domain of NLGN1 (Wu et al., 2019). On the other hand, the extracellular domain of NLGN1 also has a capability for postsynaptic recruitment of NMDARs, suggesting molecular and/or functional extracellular interaction between NLGN1 and NMDARs (Budreck et al., 2013). These extracellular and intercellular interactions of NLGN1 have been proposed to be the molecular basis underlying how NLGN1 is involved in synapse formation and function. Both in vitro and in vivo NLGN knockdown (KD) experiments result in a reduction of synapse number (Chih et al., 2005; Shipman et al., 2011; Shipman and Nicoll, 2012), while NLGN overexpression increases it (Prange et al., 2004; Boucard et al., 2005; Chih et al., 2005; Shipman et al., 2011). Also, NLGN1 KO shows LTP impairment in the hippocampus and spatial memory deficit (Blundell et al., 2010). On the other hand, NLGN1-3 triple KO impairs synapse transmission in both excitatory and inhibitory synapses without affecting their number (Chanda et al., 2017). Although the KD and KO studies show controversial results in synapse number, it is evident that NLGNs are crucial for synapse transmission and plasticity.
LRRTM
LRRTM is another NRX-binding postsynaptic organizer. LRRTM family consists of LRRTM1-4 (Roppongi et al., 2017), which have distinct expression patterns in the brain (Laurén et al., 2003). LRRTM1/2 are highly expressed in all the layers of the cerebral cortex except layer 1, the granular layer in the hippocampal DG, and the hippocampal CA1-CA3 pyramidal layers (Laurén et al., 2003; Francks et al., 2007). LRRTM3/4 are highly expressed in the hippocampal DG, the cerebral cortex layer 2 and moderately expressed in the cerebral cortex layers 3–6 (Laurén et al., 2003). LRRTMs can promote the presynaptic organization of excitatory, but not inhibitory, synapses (Ko et al., 2009a; Linhoff et al., 2009; de Wit et al., 2013; Naito et al., 2017b). Interestingly, LRRTM1/2 bind to SS4-negative NRX [NRX SS4(−)], but not SS4-positive NRX [NRX SS4(+)], regardless of α- and β-NRX isoforms (Ko et al., 2009a; Siddiqui et al., 2010). Recently, it was reported that LRRTM3/4 bind to all NRX isoforms at the glycosylated region in the presence of heparan sulfate (HS; Roppongi et al., 2020). These NRX binding codes of LRRTMs may underlie the selective induction of excitatory, but not inhibitory, presynaptic organization by LRRTMs (Roppongi et al., 2017). Indeed, neuronal KD of LRRTM2 causes a significant reduction of excitatory synapses (de Wit et al., 2009). Also, LRRTM1/2 double KO mice show a selective reduction in AMPAR-mediated, but not NMDAR-mediated, synaptic transmission which leads to LTP impairment in hippocampal CA1 pyramidal neurons (Bhouri et al., 2018). This double KO mouse line also displays spatial memory impairment, suggesting that LRRTMs play a crucial role in memory formation by controlling synaptic transmission and plasticity (Bhouri et al., 2018).
LAR-RPTP-Based Synaptic Organizing Complexes
LAR/PTPσ/PTPδ
Besides NRX family members, LAR-RPTPs are the other major presynaptic organizers, consisting of LAR, PTPσ, and PTPδ (Takahashi and Craig, 2013). The mRNAs coding LAR, PTPσ and PTPδ are broadly expressed in various mouse brain areas in overlapping and differential patterns, for instance, in the hippocampal area, LAR is mainly expressed in the DG region, PTPσ is widely expressed in the CA1/2/3 as well as DG regions, and PTPδ is strongly expressed in the DG and the CA2 regions (Kwon et al., 2010). At synapse level, PTPσ is localized at excitatory, but not inhibitory, synaptic sites, whereas PTPδ is localized at inhibitory, rather than excitatory, synaptic sites (Takahashi et al., 2011; Han et al., 2018). According to artificial synapse formation assays, LAR, PTPσ, and PTPδ promote the postsynaptic organization of excitatory synapses, but not that of inhibitory synapses, as an anterograde synaptogenic signal (Woo et al., 2009; Takahashi et al., 2011; Yoshida et al., 2011). Also, as a retrograde synaptogenic signal, LAR, PTPσ and PTPδ mediate presynaptic organization of excitatory and/or inhibitory synapses induced by their postsynaptic binding partners (Woo et al., 2009; Takahashi et al., 2011, 2012; Yoshida et al., 2011; Han et al., 2018; Bomkamp et al., 2019). As a major presynaptic hub other than NRXs, LAR-RPTPs have capabilities to bind with many different postsynaptic binding partners such as TrkC, NGL3, Slitrk1-6, interleukin-1-receptor accessory protein-like 1 (IL1RAPL1) and interleukin-1 receptor accessory protein (IL1RAcP; Kwon et al., 2010; Takahashi et al., 2011, 2012; Yoshida et al., 2011, 2012; Takahashi and Craig, 2013; Um and Ko, 2013; Yim et al., 2013; Han et al., 2018).
Importantly, each of the LAR-RPTPs varies in their binding partner selectivity. For example, NGL3 binds to all the LAR-RPTPs, whereas TrkC binds to only PTPσ, and Slitrks bind to PTPσ/δ, but not LAR (Kwon et al., 2010; Takahashi et al., 2011; Yim et al., 2013). LAR-RPTPs are composed of three immunoglobulin (Ig) domains and eight or four Fibronectin III (FNIII) domains at the extracellular region, which are responsible for trans-synaptic interactions with the above-mentioned postsynaptic organizers (Takahashi and Craig, 2013; Um and Ko, 2013). Intracellularly, LAR-RPTPs bind to the scaffolding protein liprin-α to mediate presynaptic assembly (Dunah et al., 2005; Han et al., 2016a; Xie et al., 2020). These molecular interactions are essential for the anterograde and retrograde synaptogenic signals driven by the LAR-RPTP-based synaptic organizing complexes.
Previous KO mouse studies have revealed the importance of LAR-RPTPs for synaptic and cognitive function. Specifically, PTPσ KO decreases presynaptic release probability and NMDAR-dependent LTP in the hippocampal Schaffer-CA1 synapses and abnormally enhances novel object recognition (Horn et al., 2012; Han et al., 2020a; Kim et al., 2020). In contrast, a previous study by Uetani et al. (2000) showed that PTPδ KO increases release probability and LTP in the same type of synapses and impairs spatial learning and memory. Thus, PTPσ and PTPδ are indispensable for normal synaptic and cognitive functions in a distinct manner, which may be due to their different expression patterns and binding partners. To support this, mutations in PTPσ and PTPδ genes are associated with ASD and/or attention-deficit hyperactivity disorder (ADHD; Takahashi and Craig, 2013). Conversely, however, a more recent study has shown that PTPδ conditional KO does not affect release probability (Han et al., 2020b). Moreover, recent studies on conditional KO of all LAR-RPTPs have shown that they are involved in NMDAR-mediated synaptic transmission and LTP without affecting AMPAR-mediated transmission or synapse number (Sclip and Südhof, 2020). Further studies would be required to explain the apparent discrepancies and to more specifically address the synaptic roles of LAR-RPTPs.
TrkC
TrkC is a member of the tropomyosin-receptor-kinase (Trk) family, which also includes TrkA and TrkB (Barbacid, 1994). The classical role of the Trk family is to recognize neurotrophins (NTs) such as NGF, BDNF, NT-3, and NT-4. TrkC is a specific receptor for NT-3, which promotes both neural crest cell proliferation and neuronal differentiation (Barbacid, 1994; Chao, 2003). TrkC mRNA is substantially expressed in the hippocampus and cortex of adult rat brains (Ringstedt et al., 1993), and TrkC protein is localized at excitatory, but not inhibitory, synapses in rat hippocampal neurons (Takahashi et al., 2011). Among the Trk family, only TrkC has a synaptogenic activity to selectively induce excitatory, but not inhibitory, the presynaptic organization as shown in artificial synapse formation assays and neuronal overexpression experiments (Takahashi et al., 2011; Naito et al., 2017b). While alternative splicing produces two subtypes of TrkC in terms of the presence or absence of an intracellular tyrosine kinase (TK) domain, both subtypes of TrkC contain an identical extracellular region composed of one LRR domain and two Ig domains (Valenzuela et al., 1993; Barbacid, 1994; Naito et al., 2017a). TrkC binds to PTPσ using the LRR and the first Ig domains (Takahashi et al., 2011; Coles et al., 2014) and binds to NT-3 using the second Ig domain (Urfer et al., 1995, 1998), suggesting distinct responsible domains for PTPσ- and NT3-binding and possible simultaneous binding of both PTPσ and NT3 to TrkC (Takahashi and Craig, 2013; Naito et al., 2017a), in which NT-3 may modulate a PTPσ-TrkC complex. To support this, recent studies including our own have revealed that NT-3 enhances the interaction between TrkC and PTPσ and the synaptogenic activity of TrkC, presumably through NT-3-induced dimerization of PTPσ-TrkC complexes (Ammendrup-Johnsen et al., 2015; Han et al., 2016b). Previous studies that characterized TrkC gene in transgenic or mutant mice also support the synaptic roles of TrkC and the involvement of TrkC in normal behaviors. For instance, a TrkC-overexpressing transgenic mouse line displays the elevated excitatory synaptic response in hippocampal CA1 as well as increased anxiety-like behavior and panic reaction (Dierssen et al., 2006; Sahún et al., 2007). Furthermore, TrkC KO mice show a decrease in hippocampal mossy fiber synapses as well as the impairment of synaptic maturation (Martínez et al., 1998; Otal et al., 2005).
Slitrk
Slitrks have six isoforms found in three different chromosomes, and they are composed of two extracellular LRR domains at the extracellular region as well as a transmembrane and an intracellular domain that shares homology with Trks (Aruga and Mikoshiba, 2003; Aruga et al., 2003). An in situ hybridization study has shown different expression levels and patterns for each Slitrk isoform in the brain, especially high expression of Slitrk1/3/5 and moderate expression of Slitrk2/4 in the hippocampus and cortex of young mice (postnatal 10 days; Beaubien and Cloutier, 2009). Previous artificial synapse formation assays have shown that Slitrks have a unique synaptogenic activity, by which Slitrk1/2 induce both excitatory and inhibitory presynaptic organization via presynaptic PTPσ and PTPδ, respectively, while Slitrk3 selectively induces inhibitory, but not excitatory, presynaptic organization via presynaptic PTPδ (Takahashi et al., 2012; Yim et al., 2013). The study characterizing Slitrk3 KO mice has further supported selective involvement of Slitrk3 in inhibitory synapse development (Takahashi et al., 2012) by detecting a decrease in inhibitory synapse number and function as well as seizure behaviors. On the other hand, RNAi-based knockdown studies as well as neuronal overexpression ones have indicated selective involvement of Slitrk1/2 in excitatory synapse number and function (Yim et al., 2013; Schroeder et al., 2018; Han et al., 2019). Also, Slitrk1 KO mice exhibit elevated anxiety behaviors (Katayama et al., 2010), and Slitrk5 KO mice display obsessive-compulsive–like behaviors with decreases in glutamate receptors and excitatory synaptic transmission in cortico-striatum synapses (Shmelkov et al., 2010). Together, each Slitrk isoform plays a distinct role in organizing excitatory or inhibitory synapses for normal cognitive functions.
Synaptic Organizers in AD
Considering the above-mentioned crucial roles of synaptic organizers in physiological synaptic functions, they are expected to be also substantially involved in synaptic dysfunction in AD. Indeed, we have recently uncovered that NRXs interact with AβOs and that this interaction impairs normal trafficking of NRXs on axon surface as well as excitatory presynaptic organization induced by NRX-binding partners such as NLGN1/2 and LRRTM2 (Naito et al., 2017b). Furthermore, given our artificial synapse formation data and cell surface Aβ binding data, we propose a new classification of synaptic organizers into two groups with regards to Aβ pathology: Aβ-sensitive and Aβ-insensitive synaptic organizers as discussed below.
Aβ-Sensitive Synaptic Organizers in AD
Neurexin: A Novel Binding Partner of Aβ Oligomers
Our group has performed an in situ binding assay screen using a non-physiological concentration of AβOs (250 nM, monomer equivalent) to identify synaptic organizers that interact with AβOs. Out of the 19 synaptic organizers that we tested, interestingly, only NRXs were isolated (Naito et al., 2017b). Similarly, another group has also reported that NRX1α and NRX2α bind to AβOs (Brito-Moreira et al., 2017) by performing a plate binding assay using recombinant proteins of NRX1α and NRX2α with AβOs. Our group has further performed a domain analysis and identified that the HRDs of all β-NRXs are responsible for AβO binding (Naito et al., 2017b). Moreover, the oligomeric but not the monomeric form of Aβ has an interaction with NRX1β. Interestingly, the interaction of AβOs with NRX1β does not interfere with its ability to bind to its synaptic partners such as NLGN1 or LRRTM2 (Naito et al., 2017b). To further clarify the AβO influence on β-NRX function in the neurons, we quantified the cell surface expression level of NRX1β on axons by performing time-lapse imaging of NRX1β extracellularly tagged with a pH-sensitive GFP (SEP-NRX1β; Mahon, 2011) transfected in hippocampal primary neurons before and after AβO treatment (Naito et al., 2017b). Interestingly, AβO treatment reduces surface expression of NRX1β on the axons (Naito et al., 2017b). However, SEP-NRX1β lacking the HRD is not affected by AβOs, suggesting that AβOs trigger cell surface reduction of NRX1β by binding to its HRD (Naito et al., 2017b). Currently, the physiological role of HRD in β-NRXs is not well understood, therefore it should be addressed for a better understanding of Aβ-induced synaptic pathology. Taken together, AβOs interact with β-NRXs in an HRD-dependent manner, and this interaction reduces β-NRX expression on the axon surface, presumably through enhanced endocytosis, leading to an impairment in NRX-mediated presynaptic assembly (Figure 2). Further, interestingly, β-NRX conditional triple KO increases tonic endocannabinoid signaling, such as the tonic activation of cannabinoid receptor type 1 (CB1R), to impair excitatory synaptic transmission and LTP (Anderson et al., 2015). Therefore, it is also possible that AβO-induced β-NRX surface reduction may enhance tonic endocannabinoid signaling for synaptic impairment. Indeed, it has been reported that CB1R activity is enhanced in the anterior thalamus in an AD mouse model named 3XTg-AD (Manuel et al., 2016; Basavarajappa et al., 2017). Moreover, the synaptic phenotypes of the β-NRX triple KO are detected in burst-firing, but not regular-firing, subiculum neurons, indicating synapse specificity of β-NRXs at the cellular level. Therefore, it would be interesting to elucidate whether and how Aβ affects the β-NRX-mediated endocannabinoid signaling and the synaptic specificity of β-NRXs in AD.
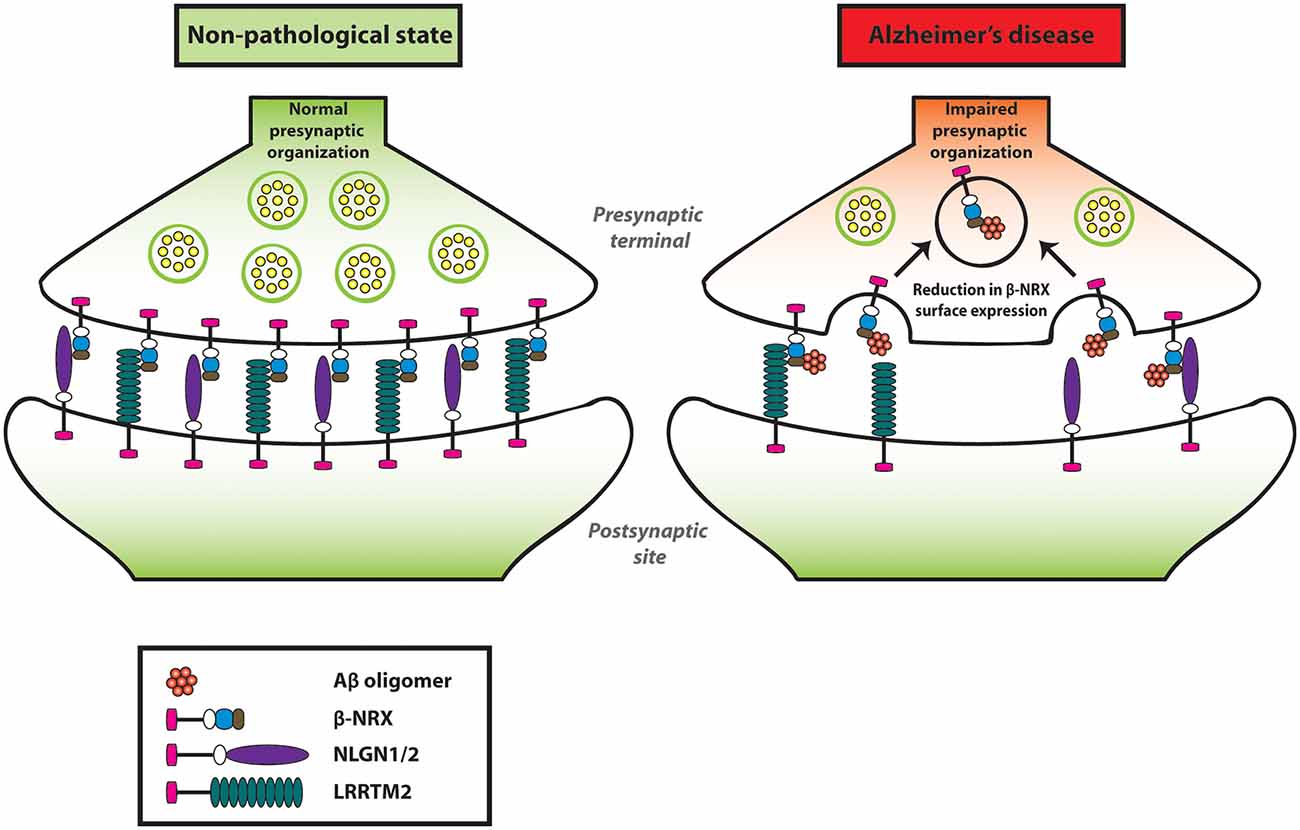
Figure 2. Amyloid-β oligomers (AβOs) impair presynaptic organization by reducing β-NRXs on the axon surface. AβOs bind to the histidine-rich domain (HRD) of β-NRXs. This interaction leads to the reduction of β-NRX surface expression on the axons without interfering with its ability to bind to NLGN1 or LRRTM2. By reducing the surface level of β-NRXs, presumably through enhanced endocytosis, AβOs impair NLGN1/2- and LRRTM2-mediated presynaptic organization.
We also identified that the SS4 of both the α and β isoforms of NRX1/2 are responsible for AβO binding (Naito et al., 2017b). However, the role of AβO binding to the SS4 sites of NRX1/2 remains to be elucidated. Our time-lapse imaging has suggested no effect of the AβO binding to NRX1β SS4 site on NRX1β expression on axon surface (Naito et al., 2017b), suggesting that it may play a different role from the HRD of NRX1β. Given that the SS4 insertion of presynaptic NRX1 increases postsynaptic NMDAR responses and thereby enhances NMDAR-dependent LTP at the hippocampal CA1-subiculum synapses (Dai et al., 2019), it is likely that the AβO binding to NRX1 SS4 site could impact NMDAR-dependent LTP, which is impaired by AβO treatment and in AD model mouse lines with Aβ overproduction (Wang et al., 2004; Hwang et al., 2017; Liu et al., 2019). On the other hand, the SS4 insertion of presynaptic NRX2 does not affect either NMDAR or AMPAR responses in the subiculum. Further studies on NRX2 SS4 as well as NRX1 SS4 are necessary to elucidate their physiological synaptic roles and involvement in Aβ synaptic pathology.
In addition to synaptic dysfunction, AβO binding to NRX could potentially play other roles in Aβ pathology, such as Aβ oligomer formation as an Aβ nucleation factor and/or neuronal AβO uptake as an Aβ receptor. To determine whether NRX can accelerate Aβ oligomerization as well as fibrillar aggregation, the thioflavin T fluorescence assay (Xue et al., 2017), in which Aβ monomers are incubated with/without NRX recombinant proteins, would be useful in further studies. Furthermore, to test whether and how NRXs are involved in neuronal uptake of AβOs, it would be worthy to perform live-cell imaging of NRX KO/KD neurons or control neurons treated with AβOs tagged with pH-sensitive dye [e.g., pHrodo (Han and Burgess, 2010; Mao et al., 2016)] that allows imaging of only internalized AβOs.
Due to the toxic and dysfunctional effects of AD pathology on neurons, the expression level of many genes including NRXs is altered in AD patients compared to healthy controls. A recent study has reported the differentially-expressed genes (DEG) in AD patients’ brains based on published microarray data sets. Interestingly, NRXN3 gene expression is significantly decreased and has the second-highest DEG in AD patients. Moreover, in the hippocampus, NRXN3 gene expression is decreased in both AD- and aging-related groups (Zheng et al., 2018). Similarly, we have reported that synaptosome fractions of J20 mice [Alzheimer’s model mice overproducing Aβ (Mucke et al., 2000)] have a significant reduction in β-NRXs as well as a reduction trend in α-NRXs, compared to their wild-type littermates (Naito et al., 2017b). These reports suggest that the expression levels of NRXs in AD are downregulated. However, it is not fully understood which of the NRX isoforms are mainly affected in AD and which brain regions in AD display changes in NRX expression. Therefore, in situ hybridization for each NRX isoform in AD model mice could provide us with a better understanding of how AD pathology affects NRX-mediated synapses.
Neuroligin: Aβ-Induced Synaptogenic Dysfunction and a Role as Aβ Deposition Stabilizer
Our artificial synapse formation assay has shown that AβO treatment significantly diminishes excitatory, but not inhibitory, presynaptic organization induced by NLGN1 and NLGN2 (Naito et al., 2017b). Given that AβO treatment reduces surface expression of NRX1β on axons, but has no effect on NRX1β-NLGN1 interaction, Aβ impairment of NLGN1-induced presynaptic organization may be due to decreased amount of axonal β-NRXs rather than direct interference with β-NRX-NLGN1 interaction (Naito et al., 2017b; Figure 2). While the artificial synapse formation assay is thus useful to determine Aβ sensitivity of NLGNs by assessing the effect of AβOs on the formation of NRX-NLGN-based synapses, it is also crucial to investigate their effect on the maintenance of NRX-NLGN-based synapses for better understanding of Aβ synaptic pathology. To address this, additional research needs to be carried out by performing artificial synapse formation assays with AβO treatment after synapses have been formed by NLGN-expressing fibroblasts.
A recent study has shown that NRXs are modified with heparan sulfate (HS) and that the synaptogenic activity of NLGN1/2 requires their interaction with the NRX HS chains as well as their protein domain-based NRX interaction (Zhang et al., 2018). Although it remains to be tested whether and how Aβ pathology and NRX HS modification are involved with each other, it has been shown that Aβ can directly interact with HS chains and HS core proteins (Buée et al., 1993; Watson et al., 1997; Cui et al., 2013). Furthermore, neuronal HS deficiency suppresses Aβ deposit in the brain of AD model mice (Liu et al., 2016), suggesting a physical and functional interaction between Aβ and HS-modified proteins, which presumably could include NRXs. Given that NRX HS modification does not affect NRX surface trafficking itself (Zhang et al., 2018), Aβ sensitivity of NRX-NLGN1/2 complexes might depend on not only the Aβ-impaired NRX trafficking on axon surface but also NRX HS modification level in AD condition.
Although our group performed in situ binding assays and concluded that AβOs did not interact with NLGN1 (total four different splicing isoforms), NLGN2 or NLGN3 (Naito et al., 2017b), two independent groups have reported that AβOs interact with at least NLGN1 (Dinamarca et al., 2011; Brito-Moreira et al., 2017). To demonstrate the AβO-NLGN1 interaction, one group performed a plate binding assay using NLGN1 recombinant proteins and AβOs (Brito-Moreira et al., 2017), and the other group used fluorescence spectroscopy to monitor Aβ-induced quenching of intrinsic tryptophan fluorescence from NLGN1 because of the prevalence of tryptophan amino acids in NLGN1, while not at all present in Aβ (Dinamarca et al., 2011). The discrepancy between our results and theirs might come from the sensitivity of the experimental methods. In this sense, a plate binding assay and fluorescence spectroscopy may have higher sensitivity than the in situ binding assays we performed. Also, their study using a thioflavin T fluorescence assay and electron microscopy have suggested that NLGN1 plays a role as a nucleating factor on Aβ aggregation, ultimately facilitating Aβ oligomer formation at the excitatory postsynaptic sites (Dinamarca et al., 2011; Figure 3). Given the three previously described pieces of evidence: (1) NRXs, as well as NLGN1, interact with AβOs; (2) NRXs trans-synaptically interact with NLGNs; and (3) NLGN1 is localized at excitatory synapses, it would be interesting to test whether and how NRXs regulate Aβ aggregation process together with NLGN1 at excitatory synapses as synaptic Aβ nucleating factors.
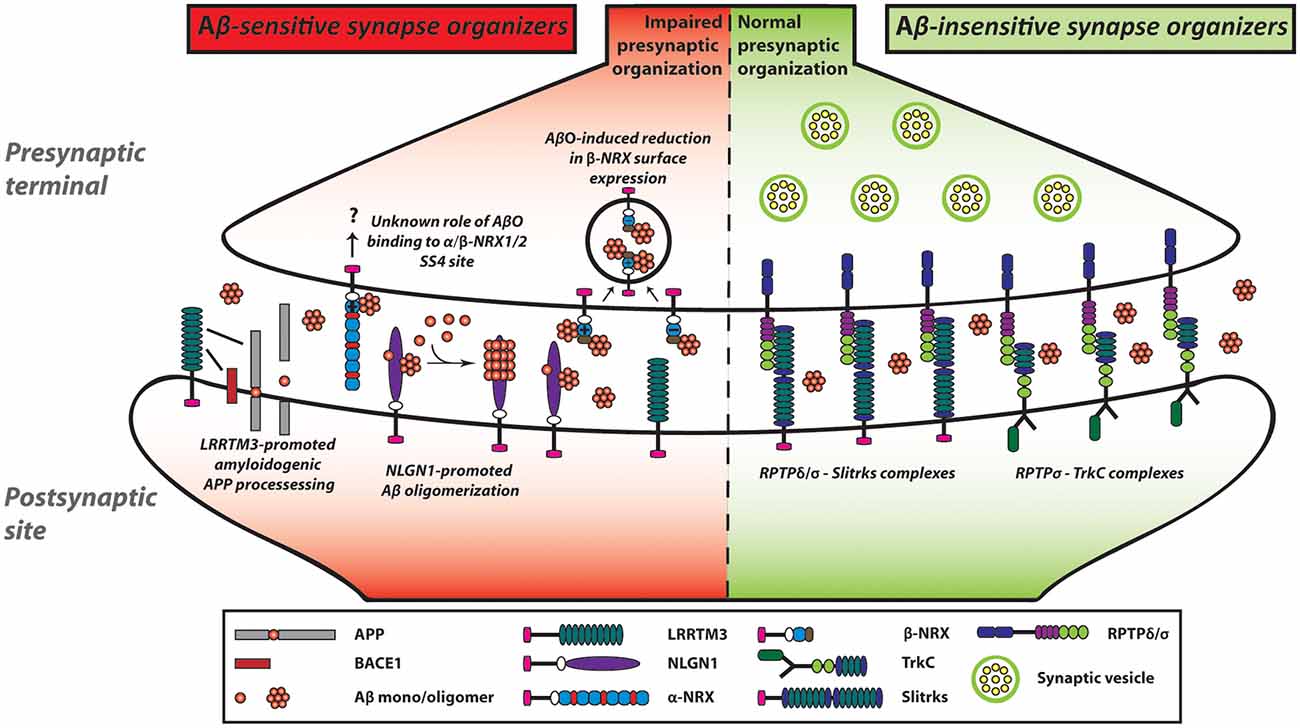
Figure 3. NRX-based and LAR-RPTP-based synaptic organizing complexes display contrasting Aβ sensitivity. AβOs exert a pathological influence on NRX-based synaptic organizing complexes. In contrast, LAR-RPTPs and their post-synaptic binding partners appear to be resistant to AβO-induced deleterious effects on synapses. AβOs bind to the HRD of β-NRXs as well as to the SS4 of NRX1/2. Furthermore, AβOs reduce the expression of β-NRXs on axon surface in an HRD-dependent but SS4-independent manner. However, the role of the NRX SS4 in Aβ synaptic pathology remains to be understood. In addition to NRXs, NLGN1 can interact with Aβ and act as an Aβ deposition stabilizer to accelerate Aβ oligomer formation and fibrillar aggregation. Although AβOs affect not only NRXs but also LRRTMs and NLGNs, we propose that AβO-mediated β-NRX surface reduction may account the most in Aβ-impaired synapse organization. As an additional role of LRRTMs in the Aβ pathway, LRRTM3 interacts with amyloid precursor protein (APP) as well as β-site APP cleaving enzyme 1 (BACE1) and promotes amyloidogenic APP processing to enhance Aβ production. Thus, NRXs and their partners are highly linked with Aβ pathology, which may result in Aβ vulnerability of synapses in Alzheimer’s disease (AD). In contrast, AβOs do not interact with LAR-RPTPs or their postsynaptic partners including TrkC and Slitrks. Accordingly, LAR-RPTP-based complexes may contribute to making synapses more tolerant of Aβ pathology in AD. Solid lines indicate protein interactions.
LRRTM: Aβ-Induced Synaptogenic Dysfunction and a Role in APP Processing
Like the case of NLGN1/2, the synaptogenic activity of LRRTM2 is sensitive to AβOs (Naito et al., 2017b). Specifically, our artificial synapse formation assay has shown that AβO treatment significantly decreases LRRTM2-induced excitatory presynaptic organization in cultured hippocampal neurons. On the other hand, AβO treatment does not affect NRX1β-LRRTM2 binding. Given that AβO treatment reduces the surface expression level of NRXs on the axons (Naito et al., 2017b) and that LRRTM1/2 share same NRX binding code (Siddiqui et al., 2010), AβOs are supposed to dampen the synaptogenic activity of LRRTM1/2 by decreasing the amount of cell surface NRXs on axons (Naito et al., 2017b). More specifically, the AβO-mediated LRRTM1/2 dysfunction may be due to the reduction of axonal expression of β-NRX SS4(−) rather than α-NRX SS4(−) or α/β-NRX SS4(+) for the two reasons: LRRTM1/2 selectively bind to α/β-NRX SS4(−; Siddiqui et al., 2010); and AβOs do not bind to α-NRX SS4(−; Naito et al., 2017b). These suggest that β-NRX SS4(−) may be a key determinant for Aβ sensitivity of LRRTM1/2-mediated excitatory synapses. Importantly, the J20 AD model mouse line shows a more significant reduction in synaptic expression of β-NRX than that of α-NRX (Naito et al., 2017b). Therefore, it would be worthy to analyze the expression of β-NRX SS4(−) and SS4(+) separately and that of LRRTM1/2 in AD animal models and/or in AD patients’ brain for better understanding of Aβ vulnerability of excitatory synapses in vivo condition.
In contrast to LRRTM1/2, LRRTM3/4 bind to all NRX isoforms including NRX1γ, which lacks the LNS domain (Roppongi et al., 2020). So far, no study has tested whether and how AβOs affect the synaptogenic activity of LRRTM3/4, and given that NRX-LRRTM3/4 interaction requires the NRX HS modification, but not the LNS domain (Roppongi et al., 2020), investigating this matter would be helpful to understand how AβOs physically and functionally interact with NRX HS chain.
In addition to the synaptogenic role of LRRTM3, a previous study using a siRNA screen has identified LRRTM3 as a positive modulator of APP processing (Majercak et al., 2006). The siRNA-based LRRTM3 knockdown in SH-SY5Y human neuroblastoma cells reduces Aβ secretion and the production of the intracellular C-terminal fragments (CTFs) by β-secretase (βCTF), suggesting that LRRTM3 positively modulates BACE1 processing of APP (Figure 3). Indeed, LRRTM3 overexpression increases Aβ secretion. A follow-up study has further shown that LRRTM3 interacts with both APP and BACE1 and that LRRTM3 is colocalized with APP in cultured cortical neurons from the Tg2576 AD model mice (Lincoln et al., 2013). On the contrary, another group has reported that LRRTM3 KO in the AD model mouse does not alter the Aβ production, suggesting that LRRTM3 may not be an essential regulator of Aβ production in vivo (Laakso et al., 2012). The authors have pointed out that one possibility for this discrepancy is that LRRTM4, which is the closest paralog of LRRTM3, could compensate for the Aβ production. While the underlying mechanism and the synaptic role of the LRRTM3-dependent modulation of APP processing need to be addressed, these findings suggest that NRX-LRRTM3-mediated synapses may be vulnerable to Aβ due to local Aβ overproduction by LRRTM3 as well as Aβ binding to NRXs at the synapse level.
Influence of Aβ-Insensitive Synaptic Organizers in AD
In addition to the identification of NRXs and their binding partners as Aβ-sensitive synaptic organizers, our recent study has illustrated the potential presence of Aβ-insensitive synaptic organizers (Naito et al., 2017b). The in situ binding screens have demonstrated that except NRXs, the other tested synaptic organizers including LAR-RPTPs and their binding partners, such as TrkC and Slitrk1-6, show no significant binding of AβOs (Figure 3). Consistent with the binding results, AβO treatment does not affect the synaptogenic activity of TrkC and Slitrk2 to induce excitatory presynaptic organization, which is mediated by PTPσ and/or PTPδ (Naito et al., 2017b; Figure 3). Therefore, the LAR-RPTPs and their binding partners could be classified as Aβ-insensitive synaptic organizers. In line with this, a previous postmortem study (Connor et al., 1996) has shown that the expression level of TrkC is unchanged in the hippocampus of AD patients. Specifically, TrkC immunostaining remains high in the granular as well as the pyramidal layers in the hippocampus in both AD and healthy control samples. These suggest that even during AD progression, TrkC may contribute to synapse maintenance by positively regulating synaptic tolerance to Aβ through its Aβ-resistant trans-synaptic bridge with PTPσ. Indeed, some synapses are preserved even at the late stage of AD (Scheff, 2003). To better understand the molecular mechanisms underlying the structural and functional preservation of synapses in AD and the possible correlation between LAR-RPTP-based synaptic organizing complexes and synaptic tolerance to Aβ, it would be important to investigate the expression levels of LAR-RPTPs and their postsynaptic partners in AD brains.
To further validate whether Aβ-insensitive synaptic organizers such as LAR-RPTPs and TrkC have a protective role against Aβ in AD synapses, it would be worth testing whether their KO in AD model mouse brain accelerates synaptic pathology and/or if their overexpression in AD model mouse brain decelerates synaptic pathology. Such studies will be essential to validate the roles of Aβ-insensitive synaptic organizers in Aβ tolerance of synapses and can potentially be approached as a therapeutic strategy.
Moreover, another postmortem study has shown that the expression level of NT-3, a TrkC neurotrophic ligand, is comparable between AD patients and healthy controls in any of the brain regions, although a slight non-significant decrease in NT-3 is detected in the cortex (Durany et al., 2000). Given that NT-3 enhances PTPσ-TrkC interaction and their synaptogenic activity (Ammendrup-Johnsen et al., 2015; Han et al., 2016b), it has also been suggested that for synapse maintenance in AD, NT-3 might reinforce PTPσ-TrkC complex to increase synaptic tolerance to Aβ.
The Role of Synaptic Organizers in Tau Pathology
Besides Aβ pathology, tau pathology is the other major AD hallmark. While there have been very few studies on the involvement of synaptic organizers in tau pathology, one study has reported the involvement of NLGN1 and LRRTM2 in cell-to-cell propagation of tau pathology (Calafate et al., 2015). When NLGN1- or LRRTM2-transfected HEK293 cells are co-cultured with hippocampal neurons expressing human mutant P301L tau, which leads to aggressive tau aggregation, the transfected HEK293 cells enhance tau aggregation in the co-cultured neurons, suggesting that NLGN1 and LRRTM2 mediate cell-to-neuron tau pathology propagation. Moreover, according to tau propagation assays using microfluidic culture devices, neuron-to-neuron propagation of tau pathology via synaptic connections is decreased by NLGN1 KD. Thus, tau propagation between neurons could be facilitated by synaptic connections mediated by synaptic organizing complexes such as NRX-NLGN1 and NRX-LRRTM2.
Given the previous studies showing that Aβ triggers and/or enhances tau pathology (Götz et al., 2004; Bennett et al., 2017; Lee et al., 2017), it would also be interesting to test whether and how AβO binding to NRX influences tau pathology in AD. Notably, NRXs bind to a scaffolding protein called CASK (Hata et al., 1996; LaConte et al., 2016), and the phosphorylation and membrane distribution of CASK are regulated by cyclin-dependent kinase 5 (CDK5), a key player that up-regulates tau hyper-phosphorylation and thereby leads to NFT (Lee and Tsai, 2003; Samuels et al., 2007; Shukla et al., 2012). Furthermore, CASK has been reported as one of the up-regulated biomarkers in the hippocampus of AD patients (Gómez Ravetti et al., 2010). This evidence gives rise to the interesting possibility that NRX might play a role in Aβ-induced tau pathology via CASK/CDK5.
Influence of Other Aβ-Sensitive Cell Adhesion Molecules
Some cell adhesion molecules other than the canonical synapse organizers have also been reported to interact with and be affected by Aβ, such as EphB2 and NCAM2. EphB2 is an ephrin B2 receptor that is localized at the postsynaptic site. A previous study has shown that Aβ interacts with EphB2, reducing the expression of surface and total EphB2 due to enhanced EphB2 degradation, ultimately leading to NMDAR-mediated LTP impairment (Cissé et al., 2011). Similarly, Aβ binds to NCAM2 and reduces NCAM2 expression levels in cultured hippocampal synaptosome (Leshchyns’ka et al., 2015). Also, Aβ affects the number of AMPAR subunit GluRA1-containing glutamatergic synapses in an NCAM2-dependent manner (Leshchyns’ka et al., 2015). Thus, some cell adhesion molecules exhibit Aβ sensitivity and would contribute to further weakening trans-synaptic cell adhesions in AD.
Conclusion and Future Directions
A growing number of studies are accumulating on the roles of synaptic organizers in AD pathology. Among the many different synaptic organizers, it is possible to classify them into two groups with regards to Aβ pathology; Aβ-sensitive and Aβ-insensitive synaptic organizers. Specifically, β-NRX directly binds to AβOs, and this interaction reduces β-NRX expression on axon surface (Naito et al., 2017b), suggesting that β-NRX is a major Aβ-sensitive synaptic organizer. However, given the discrepancy among the studies regarding Aβ binding to NLGN1 (Dinamarca et al., 2011; Brito-Moreira et al., 2017; Naito et al., 2017b), it is also important to confirm whether Aβ-insensitive synaptic organizers including LAR-RPTPs have no Aβ-binding ability by performing multiple independent experimental approaches. Given that NRX-based synaptic organizing complexes are essential for regulating synapse organization, synaptic transmission and synaptic plasticity under physiological conditions and are also required for normal cognitive functions (Südhof, 2017; Kasem et al., 2018), the Aβ-induced dysregulation/dysfunction of NRXs would be a key mechanism underlying synaptic pathology and cognitive decline in AD. On the other hand, Aβ-insensitive synaptic organizers, such as LAR-RPTPs, may contribute to synapse maintenance and preservation in AD and/or compensate for the dysfunctions of Aβ-sensitive synaptic organizers since Aβ-sensitive and Aβ-insensitive synaptic organizers are linked with each other via intracellular protein interactions based on liprin-α (Pulido et al., 1995; Wei et al., 2011; Takahashi and Craig, 2013; LaConte et al., 2016) and share some roles in synapse organization and functions. Together, the Aβ-based classification of synaptic organizers would be useful for a better understanding of the molecular basis determining Aβ vulnerability and tolerance of synapses in AD brains. Since the role of synapse organizers in Aβ binding is still an emerging field, current research has been limited to in vitro studies. Therefore, it will be essential that future studies address the in vivo roles of Aβ binding of synapse organizers to better classify them with regards to Aβ sensitivity and characterize their involvement in AD.
Given no effects of AβOs on inhibitory presynaptic organization induced by NLGN1/2 (Naito et al., 2017b), this review mainly focuses on the roles of synaptic organizers in Aβ impairment of glutamatergic excitatory synapses. However, Aβ also diminishes GABAergic inhibitory synaptic transmission by enhancing GABAA receptor endocytosis (Ulrich, 2015). Given that some synaptic organizers such as NLGN2 and Slitrk3 preferentially regulate inhibitory synapse organization (Poulopoulos et al., 2009; Takahashi et al., 2012; Li et al., 2017), further studies would be also necessary to address whether and how synaptic organizers are involved in Aβ-induced dysfunction of inhibitory synapses and dysregulation of GABAA receptors.
Considering how this evidences regarding synaptic organizers can be translated into AD therapy, we propose that the modification of their Aβ sensitivity to make synapses less vulnerable and/or more tolerant to Aβ would be an interesting and potential approach for alleviating AD synaptic pathology. To modify the Aβ sensitivity, the AβO binding mode of HRD of β-NRX, NRX1/2 SS4, and NLGN1 should be elucidated. This would help predict and screen small molecules and peptides that block AβO-NRX and AβO-NLGN1 interactions and could consequently make synapses less vulnerable to Aβ. Also, determining the amino acid residues responsible for NRX and NLGN1 binding to AβO may allow us to generate Aβ-resistant NRXs and NLGN1 mutants, which might be useful for developing new gene therapeutic approaches to ameliorate Aβ pathology in neuron culture, AD animal models and hopefully in AD patients. On the other hand, to make synapses more tolerant to Aβ, the up-regulation and/or functional enhancement of Aβ-insensitive synaptic organizers, such as TrkC, could be a potential for alternative therapeutic approaches. As mentioned above, NT-3 has been identified as not only TrkC ligand in the canonical neurotrophin pathway (Barbacid, 1994; Chao, 2003) but also a synaptogenic enhancer of PTPσ-TrkC complex for excitatory synapse organization (Ammendrup-Johnsen et al., 2015; Han et al., 2016b; Naito et al., 2017a). Notably, a previous in vitro study has shown that NT-3 application on primary cortical neurons protects them from Aβ-induced toxicity (Lesné et al., 2005). Further studies should be carried out on TrkC and/or NT-3 up-regulation in AD mouse models to validate their beneficial effects on Aβ synaptic pathology in vivo. Thus, targeted manipulation of Aβ sensitivity of synaptic organizers should have great potential in developing novel therapeutic strategies for AD.
Author Contributions
AL and HK structured and wrote the manuscript. NC made figures and wrote figure legends. HT gave structural and contextual input and revised the manuscript.
Funding
This work was supported by the Canadian Institutes of Health Research Project Grant (PTJ-159588), Alzheimer Society Research Program Biomedical Research Grant, Natural Science and Engineering Research Council (NSERC) Discovery Grant (RGPIN-2017-04753), and the Fonds de la recherche du Québec Research Scholars (Senior) to HT, NSERC Doctoral Awards to AL, the 2019-IRCM Emmanuel-Triassi Scholarship to HK, and the IRCM scholarship for the young researcher—2019 Summer to NC.
Conflict of Interest
The authors declare that the research was conducted in the absence of any commercial or financial relationships that could be construed as a potential conflict of interest.
References
Ammendrup-Johnsen, I., Naito, Y., Craig, A. M., and Takahashi, H. (2015). Neurotrophin-3 enhances the synaptic organizing function of TrkC-protein tyrosine phosphatase σ in rat hippocampal neurons. J. Neurosci. 35, 12425–12431. doi: 10.1523/JNEUROSCI.1330-15.2015
Anderson, G. R., Aoto, J., Tabuchi, K., Foldy, C., Covy, J., Yee, A. X., et al. (2015). β-neurexins control neural circuits by regulating synaptic endocannabinoid signaling. Cell 162, 593–606. doi: 10.1016/j.cell.2015.06.056
Aoto, J., Martinelli, D. C., Malenka, R. C., Tabuchi, K., and Südhof, T. C. (2013). Presynaptic neurexin-3 alternative splicing trans-synaptically controls postsynaptic AMPA receptor trafficking. Cell 154, 75–88. doi: 10.1016/j.cell.2013.05.060
Aruga, J., and Mikoshiba, K. (2003). Identification and characterization of Slitrk, a novel neuronal transmembrane protein family controlling neurite outgrowth. Mol. Cell. Neurosci. 24, 117–129. doi: 10.1016/s1044-7431(03)00129-5
Aruga, J., Yokota, N., and Mikoshiba, K. (2003). Human SLITRK family genes: genomic organization and expression profiling in normal brain and brain tumor tissue. Gene 315, 87–94. doi: 10.1016/s0378-1119(03)00715-7
Ballard, C., Gauthier, S., Corbett, A., Brayne, C., Aarsland, D., and Jones, E. (2011). Alzheimer’s disease. Lancet 377, 1019–1031. doi: 10.1016/S0140-6736(10)61349-9
Barbacid, M. (1994). The Trk family of neurotrophin receptors. J. Neurobiol. 25, 1386–1403. doi: 10.1002/neu.480251107
Basavarajappa, B. S., Shivakumar, M., Joshi, V., and Subbanna, S. (2017). Endocannabinoid system in neurodegenerative disorders. J. Neurochem. 142, 624–648. doi: 10.1111/jnc.14098
Beaubien, F., and Cloutier, J. F. (2009). Differential expression of Slitrk family members in the mouse nervous system. Dev. Dyn. 238, 3285–3296. doi: 10.1002/dvdy.22160
Bemben, M. A., Shipman, S. L., Nicoll, R. A., and Roche, K. W. (2015). The cellular and molecular landscape of neuroligins. Trends Neurosci. 38, 496–505. doi: 10.1016/j.tins.2015.06.004
Bennett, R. E., DeVos, S. L., Dujardin, S., Corjuc, B., Gor, R., Gonzalez, J., et al. (2017). Enhanced tau aggregation in the presence of amyloid β. Am. J. Pathol. 187, 1601–1612. doi: 10.1016/j.ajpath.2017.03.011
Bhouri, M., Morishita, W., Temkin, P., Goswami, D., Kawabe, H., Brose, N., et al. (2018). Deletion of LRRTM1 and LRRTM2 in adult mice impairs basal AMPA receptor transmission and LTP in hippocampal CA1 pyramidal neurons. Proc. Natl. Acad. Sci. U S A 115, E5382–E5389. doi: 10.1073/pnas.1803280115
Blundell, J., Blaiss, C. A., Etherton, M. R., Espinosa, F., Tabuchi, K., Walz, C., et al. (2010). Neuroligin-1 deletion results in impaired spatial memory and increased repetitive behavior. J. Neurosci. 30, 2115–2129. doi: 10.1523/JNEUROSCI.4517-09.2010
Bolliger, M. F., Frei, K., Winterhalter, K. H., and Gloor, S. M. (2001). Identification of a novel neuroligin in humans which binds to PSD-95 and has a widespread expression. Biochem. J. 356, 581–588. doi: 10.1042/0264-6021:3560581
Bomkamp, C., Padmanabhan, N., Karimi, B., Ge, Y., Chao, J. T., Loewen, C. J. R., et al. (2019). Mechanisms of PTPσ-mediated presynaptic differentiation. Front. Synaptic. Neurosci. 11:17. doi: 10.3389/fnsyn.2019.00017
Boucard, A. A., Chubykin, A. A., Comoletti, D., Taylor, P., and Südhof, T. C. (2005). A splice code for trans-synaptic cell adhesion mediated by binding of neuroligin 1 to α- and β-neurexins. Neuron 48, 229–236. doi: 10.1016/j.neuron.2005.08.026
Brito-Moreira, J., Lourenco, M. V., Oliveira, M. M., Ribeiro, F. C., Ledo, J. H., Diniz, L. P., et al. (2017). Interaction of amyloid-β (Aβ) oligomers with neurexin 2α and neuroligin 1 mediates synapse damage and memory loss in mice. J. Biol. Chem. 292, 7327–7337. doi: 10.1074/jbc.m116.761189
Budreck, E. C., Kwon, O. B., Jung, J. H., Baudouin, S., Thommen, A., Kim, H. S., et al. (2013). Neuroligin-1 controls synaptic abundance of NMDA-type glutamate receptors through extracellular coupling. Proc. Natl. Acad. Sci. U S A 110, 725–730. doi: 10.1073/pnas.1214718110
Budreck, E. C., and Scheiffele, P. (2007). Neuroligin-3 is a neuronal adhesion protein at GABAergic and glutamatergic synapses. Eur. J. Neurosci. 26, 1738–1748. doi: 10.1111/j.1460-9568.2007.05842.x
Buée, L., Ding, W., Delacourte, A., and Fillit, H. (1993). Binding of secreted human neuroblastoma proteoglycans to the Alzheimer’s amyloid A4 peptide. Brain Res. 601, 154–163. doi: 10.1016/0006-8993(93)91706-x
Calafate, S., Buist, A., Miskiewicz, K., Vijayan, V., Daneels, G., de Strooper, B., et al. (2015). Synaptic contacts enhance cell-to-cell tau pathology propagation. Cell Rep. 11, 1176–1183. doi: 10.1016/j.celrep.2015.04.043
Chanda, S., Hale, W. D., Zhang, B., Wernig, M., and Südhof, T. C. (2017). Unique versus redundant functions of neuroligin genes in shaping excitatory and inhibitory synapse properties. J. Neurosci. 37, 6816–6836. doi: 10.1523/JNEUROSCI.0125-17.2017
Chao, M. V. (2003). Neurotrophins and their receptors: a convergence point for many signalling pathways. Nat. Rev. Neurosci. 4, 299–309. doi: 10.1038/nrn1078
Chih, B., Engelman, H., and Scheiffele, P. (2005). Control of excitatory and inhibitory synapse formation by neuroligins. Science 307, 1324–1328. doi: 10.1126/science.1107470
Chih, B., Gollan, L., and Scheiffele, P. (2006). Alternative splicing controls selective trans-synaptic interactions of the neuroligin-neurexin complex. Neuron 51, 171–178. doi: 10.1016/j.neuron.2006.06.005
Chubykin, A. A., Liu, X., Comoletti, D., Tsigelny, I., Taylor, P., and Südhof, T. C. (2005). Dissection of synapse induction by neuroligins: effect of a neuroligin mutation associated with autism. J. Biol. Chem. 280, 22365–22374. doi: 10.1074/jbc.m410723200
Cissé, M., Halabisky, B., Harris, J., Devidze, N., Dubal, D. B., Sun, B., et al. (2011). Reversing EphB2 depletion rescues cognitive functions in Alzheimer model. Nature 469, 47–52. doi: 10.1038/nature09635
Coles, C. H., Mitakidis, N., Zhang, P., Elegheert, J., Lu, W., Stoker, A. W., et al. (2014). Structural basis for extracellular cis and trans RPTPσ signal competition in synaptogenesis. Nat. Commun. 5:5209. doi: 10.1038/ncomms6209
Connor, B., Young, D., Lawlor, P., Gai, W., Waldvogel, H., Faull, R. L., et al. (1996). Trk receptor alterations in Alzheimer’s disease. Mol. Brain Res. 42, 1–17. doi: 10.1016/s0169-328x(96)00040-x
Craig, A. M., Graf, E. R., and Linhoff, M. W. (2006). How to build a central synapse: clues from cell culture. Trends Neurosci. 29, 8–20. doi: 10.1016/j.tins.2005.11.002
Craig, A. M., and Kang, Y. (2007). Neurexin-neuroligin signaling in synapse development. Curr. Opin. Neurobiol. 17, 43–52. doi: 10.1016/j.conb.2007.01.011
Cui, H., Freeman, C., Jacobson, G. A., and Small, D. H. (2013). Proteoglycans in the central nervous system: role in development, neural repair and Alzheimer’s disease. IUBMB Life 65, 108–120. doi: 10.1002/iub.1118
Dachtler, J., Glasper, J., Cohen, R. N., Ivorra, J. L., Swiffen, D. J., Jackson, A. J., et al. (2014). Deletion of α-neurexin II results in autism-related behaviors in mice. Transl. Psychiatry 4:e484. doi: 10.1038/tp.2014.123
Dachtler, J., Ivorra, J. L., Rowland, T. E., Lever, C., Rodgers, R. J., and Clapcote, S. J. (2015). Heterozygous deletion of α-neurexin I or α-neurexin II results in behaviors relevant to autism and schizophrenia. Behav. Neurosci. 129, 765–776. doi: 10.1037/bne0000108
Dai, J., Aoto, J., and Südhof, T. C. (2019). Alternative splicing of presynaptic neurexins differentially controls postsynaptic NMDA and AMPA receptor responses. Neuron 102, 993.e5–1008.e5. doi: 10.1016/j.neuron.2019.03.032
Dalva, M. B., McClelland, A. C., and Kayser, M. S. (2007). Cell adhesion molecules: signalling functions at the synapse. Nat. Rev. Neurosci. 8, 206–220. doi: 10.1038/nrn2075
de Wit, J., O’Sullivan, M. L., Savas, J. N., Condomitti, G., Caccese, M. C., Vennekens, K. M., et al. (2013). Unbiased discovery of glypican as a receptor for LRRTM4 in regulating excitatory synapse development. Neuron 79, 696–711. doi: 10.1016/j.neuron.2013.06.049
de Wit, J., Sylwestrak, E., O’Sullivan, M. L., Otto, S., Tiglio, K., Savas, J. N., et al. (2009). LRRTM2 interacts with Neurexin1 and regulates excitatory synapse formation. Neuron 64, 799–806. doi: 10.1016/j.neuron.2009.12.019
DeTure, M. A., and Dickson, D. W. (2019). The neuropathological diagnosis of Alzheimer’s disease. Mol. Neurodegener. 14:32. doi: 10.1186/s13024-019-0333-5
Dierssen, M., Gratacos, M., Sahún, I., Martín, M., Gallego, X., Amador-Arjona, A., et al. (2006). Transgenic mice overexpressing the full-length neurotrophin receptor TrkC exhibit increased catecholaminergic neuron density in specific brain areas and increased anxiety-like behavior and panic reaction. Neurobiol. Dis. 24, 403–418. doi: 10.1016/j.nbd.2006.07.015
Dinamarca, M. C., Weinstein, D., Monasterio, O., and Inestrosa, N. C. (2011). The synaptic protein neuroligin-1 interacts with the amyloid β-peptide. Is there a role in Alzheimer’s disease? Biochemistry 50, 8127–8137. doi: 10.1021/bi201246t
Dunah, A. W., Hueske, E., Wyszynski, M., Hoogenraad, C. C., Jaworski, J., Pak, D. T., et al. (2005). LAR receptor protein tyrosine phosphatases in the development and maintenance of excitatory synapses. Nat. Neurosci. 8, 458–467. doi: 10.1038/nn1416
Durany, N., Michel, T., Kurt, J., Cruz-Sánchez, F. F., Cervás-Navarro, J., and Riederer, P. (2000). Brain-derived neurotrophic factor and neurotrophin-3 levels in Alzheimer’s disease brains. Int. J. Dev. Neurosci. 18, 807–813. doi: 10.1016/S0736-5748(00)00046-0
Etherton, M. R., Blaiss, C. A., Powell, C. M., and Südhof, T. C. (2009). Mouse neurexin-1α deletion causes correlated electrophysiological and behavioral changes consistent with cognitive impairments. Proc. Natl. Acad. Sci. U S A 106, 17998–18003. doi: 10.1073/pnas.0910297106
Francks, C., Maegawa, S., Lauren, J., Abrahams, B. S., Velayos-Baeza, A., Medland, S. E., et al. (2007). LRRTM1 on chromosome 2p12 is a maternally suppressed gene that is associated paternally with handedness and schizophrenia. Mol. Psychiatry 12, 1129–1139, 1057. doi: 10.1038/sj.mp.4002053
Gokce, O., and Südhof, T. C. (2013). Membrane-tethered monomeric neurexin LNS-domain triggers synapse formation. J. Neurosci. 33, 14617–14628. doi: 10.1523/JNEUROSCI.1232-13.2013
Gómez Ravetti, M., Rosso, O. A., Berretta, R., and Moscato, P. (2010). Uncovering molecular biomarkers that correlate cognitive decline with the changes of hippocampus’ gene expression profiles in Alzheimer’s disease. PLoS One 5:e10153. doi: 10.1371/journal.pone.0010153
Götz, J., Schild, A., Hoerndli, F., and Pennanen, L. (2004). Amyloid-induced neurofibrillary tangle formation in Alzheimer’s disease: insight from transgenic mouse and tissue-culture models. Int. J. Dev. Neurosci. 22, 453–465. doi: 10.1016/j.ijdevneu.2004.07.013
Graf, E. R., Zhang, X., Jin, S. X., Linhoff, M. W., and Craig, A. M. (2004). Neurexins induce differentiation of GABA and glutamate postsynaptic specializations via neuroligins. Cell 119, 1013–1026. doi: 10.1016/j.cell.2004.11.035
Grayton, H. M., Missler, M., Collier, D. A., and Fernandes, C. (2013). Altered social behaviours in neurexin 1α knockout mice resemble core symptoms in neurodevelopmental disorders. PLoS One 8:e67114. doi: 10.1371/journal.pone.0067114
Haass, C., and Selkoe, D. J. (2007). Soluble protein oligomers in neurodegeneration: lessons from the Alzheimer’s amyloid β-peptide. Nat. Rev. Mol. Cell Biol. 8, 101–112. doi: 10.1038/nrm2101
Han, J., and Burgess, K. (2010). Fluorescent indicators for intracellular pH. Chem. Rev. 110, 2709–2728. doi: 10.1021/cr900249z
Han, K. A., Jeon, S., Um, J. W., and Ko, J. (2016a). Emergent synapse organizers: LAR-RPTPs and their companions. Int. Rev. Cell Mol. Biol. 324, 39–65. doi: 10.1016/bs.ircmb.2016.01.002
Han, K. A., Woo, D., Kim, S., Choii, G., Jeon, S., Won, S. Y., et al. (2016b). Neurotrophin-3 regulates synapse development by modulating TrkC-PTPσ synaptic adhesion and intracellular signaling pathways. J. Neurosci. 36, 4816–4831. doi: 10.1523/JNEUROSCI.4024-15.2016
Han, K. A., Kim, J., Kim, H., Kim, D., Lim, D., Ko, J., et al. (2019). Slitrk2 controls excitatory synapse development via PDZ-mediated protein interactions. Sci. Rep. 9:17094. doi: 10.1038/s41598-019-53519-1
Han, K. A., Ko, J. S., Pramanik, G., Kim, J. Y., Tabuchi, K., Um, J. W., et al. (2018). PTPσ drives excitatory presynaptic assembly via various extracellular and intracellular mechanisms. J. Neurosci. 38, 6700–6721. doi: 10.1523/JNEUROSCI.0672-18.2018
Han, K. A., Lee, H. Y., Lim, D., Shin, J., Yoon, T. H., Lee, C., et al. (2020a). PTPσ controls presynaptic organization of neurotransmitter release machinery at excitatory synapses. iScience 23:101203. doi: 10.1016/j.isci.2020.101203
Han, K. A., Lee, H. Y., Lim, D., Shin, J., Yoon, T. H., Liu, X., et al. (2020b). Receptor protein tyrosine phosphatase δ is not essential for synapse maintenance or transmission at hippocampal synapses. Mol. Brain 13:94. doi: 10.1186/s13041-020-00629-x
Hata, Y., Butz, S., and Südhof, T. C. (1996). CASK: a novel dlg/PSD95 homolog with an N-terminal calmodulin-dependent protein kinase domain identified by interaction with neurexins. J. Neurosci. 16, 2488–2494. doi: 10.1523/JNEUROSCI.16-08-02488.1996
He, Y., Wei, M., Wu, Y., Qin, H., Li, W., Ma, X., et al. (2019). Amyloid β oligomers suppress excitatory transmitter release via presynaptic depletion of phosphatidylinositol-4,5-bisphosphate. Nat. Commun. 10:1193. doi: 10.1038/s41467-019-09114-z
Hong, S., Beja-Glasser, V. F., Nfonoyim, B. M., Frouin, A., Li, S., Ramakrishnan, S., et al. (2016). Complement and microglia mediate early synapse loss in Alzheimer mouse models. Science 352, 712–716. doi: 10.1126/science.aad8373
Horn, K. E., Xu, B., Gobert, D., Hamam, B. N., Thompson, K. M., Wu, C. L., et al. (2012). Receptor protein tyrosine phosphatase σ regulates synapse structure, function and plasticity. J. Neurochem. 122, 147–161. doi: 10.1111/j.1471-4159.2012.07762.x
Hsieh, H., Boehm, J., Sato, C., Iwatsubo, T., Tomita, T., Sisodia, S., et al. (2006). AMPAR removal underlies Aβ-induced synaptic depression and dendritic spine loss. Neuron 52, 831–843. doi: 10.1016/j.neuron.2006.10.035
Hwang, K. D., Bak, M. S., Kim, S. J., Rhee, S., and Lee, Y. S. (2017). Restoring synaptic plasticity and memory in mouse models of Alzheimer’s disease by PKR inhibition. Mol. Brain 10:57. doi: 10.1186/s13041-017-0338-3
Irie, M., Hata, Y., Takeuchi, M., Ichtchenko, K., Toyoda, A., Hirao, K., et al. (1997). Binding of neuroligins to PSD-95. Science 277, 1511–1515. doi: 10.1126/science.277.5331.1511
Jamain, S., Quach, H., Betancur, C., Rastam, M., Colineaux, C., Gillberg, I. C., et al. (2003). Mutations of the X-linked genes encoding neuroligins NLGN3 and NLGN4 are associated with autism. Nat. Genet. 34, 27–29. doi: 10.1038/ng1136
Jansen, W. J., Ossenkoppele, R., Knol, D. L., Tijms, B. M., Scheltens, P., Verhey, F. R., et al. (2015). Prevalence of cerebral amyloid pathology in persons without dementia: a meta-analysis. JAMA 313, 1924–1938. doi: 10.1001/jama.2015.4668
Kang, Y., Zhang, X., Dobie, F., Wu, H., and Craig, A. M. (2008). Induction of GABAergic postsynaptic differentiation by α-neurexins. J. Biol. Chem. 283, 2323–2334. doi: 10.1074/jbc.m703957200
Kasem, E., Kurihara, T., and Tabuchi, K. (2018). Neurexins and neuropsychiatric disorders. Neurosci. Res. 127, 53–60. doi: 10.1016/j.neures.2017.10.012
Katayama, K., Yamada, K., Ornthanalai, V. G., Inoue, T., Ota, M., Murphy, N. P., et al. (2010). Slitrk1-deficient mice display elevated anxiety-like behavior and noradrenergic abnormalities. Mol. Psychiatry 15, 177–184. doi: 10.1038/mp.2008.97
Kim, K., Shin, W., Kang, M., Lee, S., Kim, D., Kang, R., et al. (2020). Presynaptic PTPσ regulates postsynaptic NMDA receptor function through direct adhesion-independent mechanisms. eLife 9:e54224. doi: 10.7554/eLife.54224
Ko, J., Fuccillo, M. V., Malenka, R. C., and Südhof, T. C. (2009a). LRRTM2 functions as a neurexin ligand in promoting excitatory synapse formation. Neuron 64, 791–798. doi: 10.1016/j.neuron.2009.12.012
Ko, J., Zhang, C., Arac, D., Boucard, A. A., Brunger, A. T., and Südhof, T. C. (2009b). Neuroligin-1 performs neurexin-dependent and neurexin-independent functions in synapse validation. EMBO J. 28, 3244–3255. doi: 10.1038/emboj.2009.249
Koehnke, J., Jin, X., Trbovic, N., Katsamba, P. S., Brasch, J., Ahlsen, G., et al. (2008). Crystal structures of β-neurexin 1 and β-neurexin 2 ectodomains and dynamics of splice insertion sequence 4. Structure 16, 410–421. doi: 10.1016/j.str.2007.12.024
Kwon, S. K., Woo, J., Kim, S. Y., Kim, H., and Kim, E. (2010). Trans-synaptic adhesions between netrin-G ligand-3 (NGL-3) and receptor tyrosine phosphatases LAR, protein-tyrosine phosphatase δ (PTPδ) and PTPσ via specific domains regulate excitatory synapse formation. J. Biol. Chem. 285, 13966–13978. doi: 10.1074/jbc.m109.061127
Laakso, T., Muggalla, P., Kysenius, K., Laurén, J., Paatero, A., Huttunen, H. J., et al. (2012). LRRTM3 is dispensable for amyloid-β production in mice. J. Alzheimers Dis. 31, 759–764. doi: 10.3233/jad-2012-120193
LaConte, L. E., Chavan, V., Liang, C., Willis, J., Schonhense, E. M., Schoch, S., et al. (2016). CASK stabilizes neurexin and links it to liprin-α in a neuronal activity-dependent manner. Cell. Mol. Life Sci. 73, 3599–3621. doi: 10.1007/s00018-016-2183-4
Laurén, J., Airaksinen, M. S., Saarma, M., and Timmusk, T. (2003). A novel gene family encoding leucine-rich repeat transmembrane proteins differentially expressed in the nervous system. Genomics 81, 411–421. doi: 10.1016/s0888-7543(03)00030-2
Lee, K. H., Lee, S. J., Lee, H. J., Choi, G. E., Jung, Y. H., Kim, D. I., et al. (2017). Amyloid β1–42 (Aβ1–42) induces the CDK2-mediated phosphorylation of tau through the activation of the mTORC1 signaling pathway while promoting neuronal cell death. Front. Mol. Neurosci. 10:229. doi: 10.3389/fnmol.2017.00229
Lee, M. S., and Tsai, L. H. (2003). Cdk5: one of the links between senile plaques and neurofibrillary tangles? J. Alzheimers Dis. 5, 127–137. doi: 10.3233/jad-2003-5207
Leshchyns’ka, I., Liew, H. T., Shepherd, C., Halliday, G. M., Stevens, C. H., Ke, Y. D., et al. (2015). Aβ-dependent reduction of NCAM2-mediated synaptic adhesion contributes to synapse loss in Alzheimer’s disease. Nat. Commun. 6:8836. doi: 10.1038/ncomms9836
Lesné, S., Gabriel, C., Nelson, D. A., White, E., Mackenzie, E. T., Vivien, D., et al. (2005). Akt-dependent expression of NAIP-1 protects neurons against amyloid-β toxicity. J. Biol. Chem. 280, 24941–24947. doi: 10.1074/jbc.m413495200
Li, J., Han, W., Pelkey, K. A., Duan, J., Mao, X., Wang, Y. X., et al. (2017). Molecular dissection of neuroligin 2 and Slitrk3 reveals an essential framework for GABAergic Synapse development. Neuron 96, 808.e8–826.e8. doi: 10.1016/j.neuron.2017.10.003
Li, S., Jin, M., Koeglsperger, T., Shepardson, N. E., Shankar, G. M., and Selkoe, D. J. (2011). Soluble Aβ oligomers inhibit long-term potentiation through a mechanism involving excessive activation of extrasynaptic NR2B-containing NMDA receptors. J. Neurosci. 31, 6627–6638. doi: 10.1523/JNEUROSCI.0203-11.2011
Li, Z., and Sheng, M. (2003). Some assembly required: the development of neuronal synapses. Nat. Rev. Mol. 4, 833–841. doi: 10.1038/nrm1242
Lincoln, S., Allen, M., Cox, C. L., Walker, L. P., Malphrus, K., Qiu, Y., et al. (2013). LRRTM3 interacts with APP and BACE1 and has variants associating with late-onset Alzheimer’s disease (LOAD). PLoS One 8:e64164. doi: 10.1371/journal.pone.0064164
Linhoff, M. W., Laurén, J., Cassidy, R. M., Dobie, F. A., Takahashi, H., Nygaard, H. B., et al. (2009). An unbiased expression screen for synaptogenic proteins identifies the LRRTM protein family as synaptic organizers. Neuron 61, 734–749. doi: 10.1016/j.neuron.2009.01.017
Liu, J., Chang, L., Song, Y., Li, H., and Wu, Y. (2019). The role of NMDA receptors in Alzheimer’s disease. Front. Neurosci. 13:43. doi: 10.3389/fnins.2019.00043
Liu, C. C., Zhao, N., Yamaguchi, Y., Cirrito, J. R., Kanekiyo, T., Holtzman, D. M., et al. (2016). Neuronal heparan sulfates promote amyloid pathology by modulating brain amyloid-β clearance and aggregation in Alzheimer’s disease. Sci. Transl. Med. 8:332ra344. doi: 10.1126/scitranslmed.aad3650
Long, J. M., and Holtzman, D. M. (2019). Alzheimer disease: an update on pathobiology and treatment strategies. Cell 179, 312–339. doi: 10.1016/j.cell.2019.09.001
Mahon, M. J. (2011). pHluorin2: an enhanced, ratiometric, pH-sensitive green florescent protein. Adv. Biosci. Biotechnol. 2, 132–137. doi: 10.4236/abb.2011.23021
Majercak, J., Ray, W. J., Espeseth, A., Simon, A., Shi, X. P., Wolffe, C., et al. (2006). LRRTM3 promotes processing of amyloid-precursor protein by BACE1 and is a positional candidate gene for late-onset Alzheimer’s disease. Proc. Natl. Acad. Sci. U S A 103, 17967–17972. doi: 10.1073/pnas.0605461103
Manuel, I., Lombardero, L., LaFerla, F. M., Giménez-Llort, L., and Rodríguez-Puertas, R. (2016). Activity of muscarinic, galanin and cannabinoid receptors in the prodromal and advanced stages in the triple transgenic mice model of Alzheimer’s disease. Neuroscience 329, 284–293. doi: 10.1016/j.neuroscience.2016.05.012
Mao, X., Ou, M. T., Karuppagounder, S. S., Kam, T. I., Yin, X., Xiong, Y., et al. (2016). Pathological α-synuclein transmission initiated by binding lymphocyte-activation gene 3. Science 353:aah3374. doi: 10.1126/science.aah3374
Martínez, A., Alcántara, S., Borrell, V., Del Río, J. A., Blasi, J., Otal, R., et al. (1998). TrkB and TrkC signaling are required for maturation and synaptogenesis of hippocampal connections. J. Neurosci. 18, 7336–7350. doi: 10.1523/JNEUROSCI.18-18-07336.1998
Matsuda, K., Miura, E., Miyazaki, T., Kakegawa, W., Emi, K., Narumi, S., et al. (2010). Cbln1 is a ligand for an orphan glutamate receptor δ2, a bidirectional synapse organizer. Science 328, 363–368. doi: 10.1126/science.1185152
Missler, M., and Südhof, T. C. (1998). Neurexins: three genes and 1001 products. Trends Genet. 14, 20–26. doi: 10.1016/s0168-9525(97)01324-3
Missler, M., Südhof, T. C., and Biederer, T. (2012). Synaptic cell adhesion. Cold Spring Harb. Perspect. Biol. 4:a005694. doi: 10.1101/cshperspect.a005694
Missler, M., Zhang, W., Rohlmann, A., Kattenstroth, G., Hammer, R. E., Gottmann, K., et al. (2003). α-neurexins couple Ca2+ channels to synaptic vesicle exocytosis. Nature 423, 939–948. doi: 10.1038/nature01755
Mucke, L., Masliah, E., Yu, G. Q., Mallory, M., Rockenstein, E. M., Tatsuno, G., et al. (2000). High-level neuronal expression of aβ 1–42 in wild-type human amyloid protein precursor transgenic mice: synaptotoxicity without plaque formation. J. Neurosci. 20, 4050–4058. doi: 10.1523/JNEUROSCI.20-11-04050.2000
Naito, Y., Lee, A. K., and Takahashi, H. (2017a). Emerging roles of the neurotrophin receptor TrkC in synapse organization. Neurosci. Res. 116, 10–17. doi: 10.1016/j.neures.2016.09.009
Naito, Y., Tanabe, Y., Lee, A. K., Hamel, E., and Takahashi, H. (2017b). Amyloid-β oligomers interact with neurexin and diminish neurexin-mediated excitatory presynaptic organization. Sci. Rep. 7:42548. doi: 10.1038/srep42548
Neupert, C., Schneider, R., Klatt, O., Reissner, C., Repetto, D., Biermann, B., et al. (2015). Regulatedn. J. Neurosci. 35, 13629–13647. doi: 10.1523/JNEUROSCI.4041-14.2015
Nguyen, T., and Südhof, T. C. (1997). Binding properties of neuroligin 1 and neurexin 1β reveal function as heterophilic cell adhesion molecules. J. Biol. Chem. 272, 26032–26039. doi: 10.1074/jbc.272.41.26032
Nicoll, R. A. (2017). A brief history of long-term potentiation. Neuron 93, 281–290. doi: 10.1016/j.neuron.2016.12.015
O’Brien, R. J., and Wong, P. C. (2011). Amyloid precursor protein processing and Alzheimer’s disease. Annu. Rev. Neurosci. 34, 185–204. doi: 10.1146/annurev-neuro-061010-113613
Otal, R., Martínez, A., and Soriano, E. (2005). Lack of TrkB and TrkC signaling alters the synaptogenesis and maturation of mossy fiber terminals in the hippocampus. Cell Tissue Res. 319, 349–358. doi: 10.1007/s00441-004-1020-5
Parodi, J., Sepúlveda, F. J., Roa, J., Opazo, C., Inestrosa, N. C., and Aguayo, L. G. (2010). β-amyloid causes depletion of synaptic vesicles leading to neurotransmission failure. J. Biol. Chem. 285, 2506–2514. doi: 10.1074/jbc.m109.030023
Poulopoulos, A., Aramuni, G., Meyer, G., Soykan, T., Hoon, M., Papadopoulos, T., et al. (2009). Neuroligin 2 drives postsynaptic assembly at perisomatic inhibitory synapses through gephyrin and collybistin. Neuron 63, 628–642. doi: 10.1016/j.neuron.2009.08.023
Prange, O., Wong, T. P., Gerrow, K., Wang, Y. T., and El-Husseini, A. (2004). A balance between excitatory and inhibitory synapses is controlled by PSD-95 and neuroligin. Proc. Natl. Acad. Sci. U S A 101, 13915–13920. doi: 10.1073/pnas.0405939101
Pulido, R., Serra-Pagès, C., Tang, M., and Streuli, M. (1995). The LAR/PTP δ/PTP σ subfamily of transmembrane protein-tyrosine-phosphatases: multiple human LAR, PTP δ, and PTP σ isoforms are expressed in a tissue-specific manner and associate with the LAR-interacting protein LIP.1. Proc. Natl. Acad. Sci. U S A 92, 11686–11690. doi: 10.1073/pnas.92.25.11686
Reiss, A. B., Arain, H. A., Stecker, M. M., Siegart, N. M., and Kasselman, L. J. (2018). Amyloid toxicity in Alzheimer’s disease. Rev. Neurosci. 29, 613–627. doi: 10.1515/revneuro-2017-0063
Reissner, C., Runkel, F., and Missler, M. (2013). Neurexins. Genome Biol. 14:213. doi: 10.1186/gb-2013-14-9-213
Ringstedt, T., Lagercrantz, H., and Persson, H. (1993). Expression of members of the trk family in the developing postnatal rat brain. Dev. Brain Res. 72, 119–131. doi: 10.1016/0165-3806(93)90165-7
Roppongi, R. T., Dhume, S. H., Padmanabhan, N., Silwal, P., Zahra, N., Karimi, B., et al. (2020). LRRTMs organize synapses through differential engagement of neurexin and PTPσ. Neuron 106, 108.e12–125.e12. doi: 10.1016/j.neuron.2020.01.003
Roppongi, R. T., Karimi, B., and Siddiqui, T. J. (2017). Role of LRRTMs in synapse development and plasticity. Neurosci. Res. 116, 18–28. doi: 10.1016/j.neures.2016.10.003
Sahún, I., Delgado-García, J. M., Amador-Arjona, A., Giralt, A., Alberch, J., Dierssen, M., et al. (2007). Dissociation between CA3-CA1 synaptic plasticity and associative learning in TgNTRK3 transgenic mice. J. Neurosci. 27, 2253–2260. doi: 10.1523/JNEUROSCI.4055-06.2007
Samuels, B. A., Hsueh, Y. P., Shu, T., Liang, H., Tseng, H. C., Hong, C. J., et al. (2007). Cdk5 promotes synaptogenesis by regulating the subcellular distribution of the MAGUK family member CASK. Neuron 56, 823–837. doi: 10.1016/j.neuron.2007.09.035
Sasaguri, H., Nilsson, P., Hashimoto, S., Nagata, K., Saito, T., De Strooper, B., et al. (2017). APP mouse models for Alzheimer’s disease preclinical studies. EMBO J. 36, 2473–2487. doi: 10.15252/embj.201797397
Scheff, S. (2003). Reactive synaptogenesis in aging and Alzheimer’s disease: lessons learned in the Cotman laboratory. Neurochem. Res. 28, 1625–1630. doi: 10.1023/a:1026048619220
Scheff, S. W., and Price, D. A. (2003). Synaptic pathology in Alzheimer’s disease: a review of ultrastructural studies. Neurobiol. Aging 24, 1029–1046. doi: 10.1016/j.neurobiolaging.2003.08.002
Scheiffele, P., Fan, J., Choih, J., Fetter, R., and Serafini, T. (2000). Neuroligin expressed in nonneuronal cells triggers presynaptic development in contacting axons. Cell 101, 657–669. doi: 10.1016/s0092-8674(00)80877-6
Schroeder, A., Vanderlinden, J., Vints, K., Ribeiro, L. F., Vennekens, K. M., Gounko, N. V., et al. (2018). A modular organization of LRR protein-mediated synaptic adhesion defines synapse identity. Neuron 99, 329.e7–344.e7. doi: 10.1016/j.neuron.2018.06.026
Sclip, A., and Südhof, T. C. (2020). LAR receptor phospho-tyrosine phosphatases regulate NMDA-receptor responses. eLife 9:e53406. doi: 10.7554/eLife.53406
Shankar, G. M., Bloodgood, B. L., Townsend, M., Walsh, D. M., Selkoe, D. J., and Sabatini, B. L. (2007). Natural oligomers of the Alzheimer amyloid-β protein induce reversible synapse loss by modulating an NMDA-type glutamate receptor-dependent signaling pathway. J. Neurosci. 27, 2866–2875. doi: 10.1523/JNEUROSCI.4970-06.2007
Sheng, M., Sabatini, B. L., and Südhof, T. C. (2012). Synapses and Alzheimer’s disease. Cold Spring Harb. Perspect. Biol. 4:a005777. doi: 10.1101/cshperspect.a005777
Shipman, S. L., and Nicoll, R. A. (2012). A subtype-specific function for the extracellular domain of neuroligin 1 in hippocampal LTP. Neuron 76, 309–316. doi: 10.1016/j.neuron.2012.07.024
Shipman, S. L., Schnell, E., Hirai, T., Chen, B. S., Roche, K. W., and Nicoll, R. A. (2011). Functional dependence of neuroligin on a new non-PDZ intracellular domain. Nat. Neurosci. 14, 718–726. doi: 10.1038/nn.2825
Shmelkov, S. V., Hormigo, A., Jing, D., Proenca, C. C., Bath, K. G., Milde, T., et al. (2010). Slitrk5 deficiency impairs corticostriatal circuitry and leads to obsessive-compulsive-like behaviors in mice. Nat. Med. 16, 598–602, 591p following 602. doi: 10.1038/nm.2125
Shukla, V., Skuntz, S., and Pant, H. C. (2012). Deregulated Cdk5 activity is involved in inducing Alzheimer’s disease. Arch. Med. Res. 43, 655–662. doi: 10.1016/j.arcmed.2012.10.015
Siddiqui, T. J., and Craig, A. M. (2011). Synaptic organizing complexes. Curr. Opin. Neurobiol. 21, 132–143. doi: 10.1016/j.conb.2010.08.016
Siddiqui, T. J., Pancaroglu, R., Kang, Y., Rooyakkers, A., and Craig, A. M. (2010). LRRTMs and neuroligins bind neurexins with a differential code to cooperate in glutamate synapse development. J. Neurosci. 30, 7495–7506. doi: 10.1523/JNEUROSCI.0470-10.2010
Song, J. Y., Ichtchenko, K., Südhof, T. C., and Brose, N. (1999). Neuroligin 1 is a postsynaptic cell-adhesion molecule of excitatory synapses. Proc. Natl. Acad. Sci. U S A 96, 1100–1105. doi: 10.1073/pnas.96.3.1100
Spires-Jones, T. L., Meyer-Luehmann, M., Osetek, J. D., Jones, P. B., Stern, E. A., Bacskai, B. J., et al. (2007). Impaired spine stability underlies plaque-related spine loss in an Alzheimer’s disease mouse model. Am. J. Pathol. 171, 1304–1311. doi: 10.2353/ajpath.2007.070055
Südhof, T. C. (2008). Neuroligins and neurexins link synaptic function to cognitive disease. Nature 455, 903–911. doi: 10.1038/nature07456
Südhof, T. C. (2017). Synaptic neurexin complexes: a molecular code for the logic of neural circuits. Cell 171, 745–769. doi: 10.1016/j.cell.2017.10.024
Tabuchi, K., and Südhof, T. C. (2002). Structure and evolution of neurexin genes: insight into the mechanism of alternative splicing. Genomics 79, 849–859. doi: 10.1006/geno.2002.6780
Takahashi, H., Arstikaitis, P., Prasad, T., Bartlett, T. E., Wang, Y. T., Murphy, T. H., et al. (2011). Postsynaptic TrkC and presynaptic PTPσ function as a bidirectional excitatory synaptic organizing complex. Neuron 69, 287–303. doi: 10.1016/j.neuron.2010.12.024
Takahashi, H., and Craig, A. M. (2013). Protein tyrosine phosphatases PTPδ, PTPσ, and LAR: presynaptic hubs for synapse organization. Trends Neurosci. 36, 522–534. doi: 10.1016/j.tins.2013.06.002
Takahashi, H., Katayama, K., Sohya, K., Miyamoto, H., Prasad, T., Matsumoto, Y., et al. (2012). Selective control of inhibitory synapse development by Slitrk3-PTPδ trans-synaptic interaction. Nat. Neurosci. 15, 389–398, S1–S2. doi: 10.1038/nn.3040
Treutlein, B., Gokce, O., Quake, S. R., and Südhof, T. C. (2014). Cartography of neurexin alternative splicing mapped by single-molecule long-read mRNA sequencing. Proc. Natl. Acad. Sci. U S A 111, E1291–E1299. doi: 10.1073/pnas.1403244111
Uchigashima, M., Cheung, A., Suh, J., Watanabe, M., and Futai, K. (2019). Differential expression of neurexin genes in the mouse brain. J. Comp. Neurol. 527, 1940–1965. doi: 10.1002/cne.24664
Uemura, T., Lee, S. J., Yasumura, M., Takeuchi, T., Yoshida, T., Ra, M., et al. (2010). Trans-synaptic interaction of GluRδ2 and Neurexin through Cbln1 mediates synapse formation in the cerebellum. Cell 141, 1068–1079. doi: 10.1016/j.cell.2010.04.035
Uetani, N., Kato, K., Ogura, H., Mizuno, K., Kawano, K., Mikoshiba, K., et al. (2000). Impaired learning with enhanced hippocampal long-term potentiation in PTPδ-deficient mice. EMBO J. 19, 2775–2785. doi: 10.1093/emboj/19.12.2775
Ulrich, D. (2015). Amyloid-β impairs synaptic inhibition via GABAA receptor endocytosis. J. Neurosci. 35, 9205–9210. doi: 10.1523/JNEUROSCI.0950-15.2015
Um, J. W., and Ko, J. (2013). LAR-RPTPs: synaptic adhesion molecules that shape synapse development. Trends Cell Biol. 23, 465–475. doi: 10.1016/j.tcb.2013.07.004
Urfer, R., Tsoulfas, P., O’Connell, L., Hongo, J. A., Zhao, W., and Presta, L. G. (1998). High resolution mapping of the binding site of TrkA for nerve growth factor and TrkC for neurotrophin-3 on the second immunoglobulin-like domain of the Trk receptors. J. Biol. Chem. 273, 5829–5840. doi: 10.1074/jbc.273.10.5829
Urfer, R., Tsoulfas, P., O’Connell, L., Shelton, D. L., Parada, L. F., and Presta, L. G. (1995). An immunoglobulin-like domain determines the specificity of neurotrophin receptors. EMBO J. 14, 2795–2805. doi: 10.1002/j.1460-2075.1995.tb07279.x
Valenzuela, D. M., Maisonpierre, P. C., Glass, D. J., Rojas, E., Nunez, L., Kong, Y., et al. (1993). Alternative forms of rat TrkC with different functional capabilities. Neuron 10, 963–974. doi: 10.1016/0896-6273(93)90211-9
van der Kant, R., Goldstein, L. S. B., and Ossenkoppele, R. (2020). Amyloid-β-independent regulators of tau pathology in Alzheimer disease. Nat. Rev. Neurosci. 21, 21–35. doi: 10.1038/s41583-019-0240-3
Varoqueaux, F., Aramuni, G., Rawson, R. L., Mohrmann, R., Missler, M., Gottmann, K., et al. (2006). Neuroligins determine synapse maturation and function. Neuron 51, 741–754. doi: 10.1016/j.neuron.2006.09.003
Varoqueaux, F., Jamain, S., and Brose, N. (2004). Neuroligin 2 is exclusively localized to inhibitory synapses. Eur. J. Cell Biol. 83, 449–456. doi: 10.1078/0171-9335-00410
Waites, C. L., Craig, A. M., and Garner, C. C. (2005). Mechanisms of vertebrate synaptogenesis. Annu. Rev. Neurosci. 28, 251–274. doi: 10.1146/annurev.neuro.27.070203.144336
Wang, Q., Rowan, M. J., and Anwyl, R. (2004). β-amyloid-mediated inhibition of NMDA receptor-dependent long-term potentiation induction involves activation of microglia and stimulation of inducible nitric oxide synthase and superoxide. J. Neurosci. 24, 6049–6056. doi: 10.1523/JNEUROSCI.0233-04.2004
Watson, D. J., Lander, A. D., and Selkoe, D. J. (1997). Heparin-binding properties of the amyloidogenic peptides Aβ and amylin. Dependence on aggregation state and inhibition by Congo red. J. Biol. Chem. 272, 31617–31624. doi: 10.1074/jbc.272.50.31617
Wei, Z., Zheng, S., Spangler, S. A., Yu, C., Hoogenraad, C. C., and Zhang, M. (2011). Liprin-mediated large signaling complex organization revealed by the liprin-α/CASK and liprin-α/liprin-β complex structures. Mol. Cell 43, 586–598. doi: 10.1016/j.molcel.2011.07.021
Weller, J., and Budson, A. (2018). Current understanding of Alzheimer’s disease diagnosis and treatment. F1000Res 7:F1000. doi: 10.12688/f1000research.14506.1
Woo, J., Kwon, S. K., Choi, S., Kim, S., Lee, J. R., Dunah, A. W., et al. (2009). Trans-synaptic adhesion between NGL-3 and LAR regulates the formation of excitatory synapses. Nat. Neurosci. 12, 428–437. doi: 10.1038/nn.2279
Wu, X., Morishita, W. K., Riley, A. M., Hale, W. D., Südhof, T. C., and Malenka, R. C. (2019). Neuroligin-1 signaling controls LTP and NMDA receptors by distinct molecular pathways. Neuron 102, 621.e3–635.e3. doi: 10.1016/j.neuron.2019.02.013
Xie, X., Luo, L., Liang, M., Zhang, W., Zhang, T., Yu, C., et al. (2020). Structural basis of liprin-α-promoted LAR-RPTP clustering for modulation of phosphatase activity. Nat. Commun. 11:169. doi: 10.1038/s41467-019-13949-x
Xue, C., Lin, T. Y., Chang, D., and Guo, Z. (2017). Thioflavin T as an amyloid dye: fibril quantification, optimal concentration and effect on aggregation. R. Soc. Open Sci. 4:160696. doi: 10.1098/rsos.160696
Yamagata, A., Goto-Ito, S., Sato, Y., Shiroshima, T., Maeda, A., Watanabe, M., et al. (2018). Structural insights into modulation and selectivity of transsynaptic neurexin-LRRTM interaction. Nat. Commun. 9:3964. doi: 10.1038/s41467-018-06333-8
Yim, Y. S., Kwon, Y., Nam, J., Yoon, H. I., Lee, K., Kim, D. G., et al. (2013). Slitrks control excitatory and inhibitory synapse formation with LAR receptor protein tyrosine phosphatases. Proc. Natl. Acad. Sci. U S A 110, 4057–4062. doi: 10.1073/pnas.1209881110
Yoshida, T., Shiroshima, T., Lee, S. J., Yasumura, M., Uemura, T., Chen, X., et al. (2012). Interleukin-1 receptor accessory protein organizes neuronal synaptogenesis as a cell adhesion molecule. J. Neurosci. 32, 2588–2600. doi: 10.1523/JNEUROSCI.4637-11.2012
Yoshida, T., Yasumura, M., Uemura, T., Lee, S. J., Ra, M., Taguchi, R., et al. (2011). IL-1 receptor accessory protein-like 1 associated with mental retardation and autism mediates synapse formation by trans-synaptic interaction with protein tyrosine phosphatase δ. J. Neurosci. 31, 13485–13499. doi: 10.1523/JNEUROSCI.2136-11.2011
Zhang, P., Lu, H., Peixoto, R. T., Pines, M. K., Ge, Y., Oku, S., et al. (2018). Heparan sulfate organizes neuronal synapses through neurexin partnerships. Cell 174, 1450.e23–1464.e23. doi: 10.1016/j.cell.2018.07.002
Keywords: Alzheimer’s disease, amyloid-β, synaptic organizers, neurexin, neuroligin, in situ binding assay, artificial synapse formation assay
Citation: Lee AK, Khaled H, Chofflet N and Takahashi H (2020) Synaptic Organizers in Alzheimer’s Disease: A Classification Based on Amyloid-β Sensitivity. Front. Cell. Neurosci. 14:281. doi: 10.3389/fncel.2020.00281
Received: 30 May 2020; Accepted: 10 August 2020;
Published: 02 September 2020.
Edited by:
Masahito Yamagata, Harvard University, United StatesReviewed by:
Jaewon Ko, Daegu Gyeongbuk Institute of Science and Technology (DGIST), South KoreaHisashi Umemori, Harvard Medical School, United States
Copyright © 2020 Lee, Khaled, Chofflet and Takahashi. This is an open-access article distributed under the terms of the Creative Commons Attribution License (CC BY). The use, distribution or reproduction in other forums is permitted, provided the original author(s) and the copyright owner(s) are credited and that the original publication in this journal is cited, in accordance with accepted academic practice. No use, distribution or reproduction is permitted which does not comply with these terms.
*Correspondence: Hideto Takahashi, hideto.takahashi@ircm.qc.ca
† These authors have contributed equally to this work