Causal Role of Neural Signals Transmitted From the Frontal Eye Field to the Superior Colliculus in Saccade Generation
- 1Division of Biomedical Science, Faculty of Medicine, University of Tsukuba, Tsukuba, Japan
- 2Graduate School of Comprehensive Human Sciences, University of Tsukuba, Tsukuba, Japan
- 3Transborder Medical Research Center, University of Tsukuba, Tsukuba, Japan
- 4Systems Neuroscience Section, Primate Research Institute, Kyoto University, Inuyama, Japan
- 5Precursory Research for Embryonic Science and Technology (PRESTO), Japan Science and Technology Agency (JST), Kawaguchi, Japan
The frontal eye field (FEF) and superior colliculus (SC) are major and well-studied components of the oculomotor system. The FEF sends strong projections to the SC directly, and neurons in these brain regions transmit a variety of signals related to saccadic eye movements. Electrical microstimulation and pharmacological manipulation targeting the FEF or SC affect saccadic eye movements. These data suggest the causal contribution of each region to saccade generation. To understand how the brain generates behavior, however, it is critical not only to identify the structures and functions of individual regions, but also to elucidate how they interact with each other. In this review article, we first survey previous works that aimed at investigating whether and how the FEF and SC interact to regulate saccadic eye movements using electrophysiological and pharmacological techniques. These works have reported what signals FEF neurons transmit to the SC and what roles such signals play in regulating oculomotor behavior. We then highlight a recent attempt of our own that has applied an optogenetic approach to stimulate the neural pathway from the FEF to the SC in nonhuman primates. This study has shown that optogenetic stimulation of the FEF-SC pathway is sufficiently effective not only to modulate SC neuron activity, but also to evoke saccadic eye movements. Although the oculomotor system is a complex neural network composed of numbers of cortical and subcortical regions, the optogenetic approach will provide a powerful strategy for elucidating the role of each neural pathway constituting this network.
Introduction
The oculomotor system is composed of numbers of cortical and subcortical regions that form a complex neural network. The frontal eye field (FEF) and the superior colliculus (SC) are major components of this system. The roles of the FEF and SC in regulating oculomotor behavior have long been investigated in macaque monkeys in which the oculomotor system is substantially developed. Electrophysiological recording studies have reported that neurons in both the FEF and the SC transmit a variety of signals related to saccadic eye movements, ranging from visual responses evoked by saccadic targets to burst firing before and after the execution of the eye movements (Bizzi, 1968; Schiller and Koerner, 1971; Wurtz and Goldberg, 1971; Mohler et al., 1973; Bruce and Goldberg, 1985). Electrical microstimulation of the FEF or SC elicits saccadic eye movements (Robinson and Fuchs, 1969; Robinson, 1972; Schiller and Stryker, 1972; Bruce et al., 1985), while pharmacological inactivation of either region severely disrupts the eye movements (Hikosaka and Wurtz, 1985; Dias et al., 1995; Sommer and Tehovnik, 1997; Dias and Segraves, 1999). Notably, inactivation of the FEF more severely disrupts saccadic eye movements than that of other cortical oculomotor areas (Sommer and Tehovnik, 1999; Chafee and Goldman-Rakic, 2000). These works suggest the strong causal contribution of the FEF and SC to saccade generation.
Understanding how the brain generates behavior, however, requires more than just analyzing the roles of individual regions. It is critical to determine how particular regions interact with each other and how their interaction contributes to behavior. Since the FEF and SC are mutually connected in a manner that the former projects directly to the latter (Fries, 1984; Komatsu and Suzuki, 1985; Stanton et al., 1988b), while the latter sends signals indirectly to the former via the mediodorsal nucleus of the thalamus (MD; Sommer and Wurtz, 2002, 2004a,b), these regions could interact to regulate saccadic eye movements. Here we first review prior electrophysiological and pharmacological studies that aimed at investigating what signals FEF neurons transmit to the SC and what roles these signals play in regulating oculomotor behavior. Evidence accumulated by these studies indicates that the FEF is largely involved in saccade generation by conveying oculomotor signals to the SC.
The optogenetic methodology that has been used to stimulate signal transmission connecting two given brain regions (Bernstein and Boyden, 2011; Tye and Deisseroth, 2012) allows us to directly address what roles the neural signals transmitted from the FEF to the SC play in regulating saccadic eye movements. Such optogenetic methodology has brought substantial success in modulating behaviors in rodents (Stuber et al., 2011; Tye et al., 2011; Stamatakis and Stuber, 2012; Warden et al., 2012; Ahmari et al., 2013; Miyamoto et al., 2016), and has advanced our understanding of the roles of particular neural circuits in behaviors. After reviewing the electrophysiological and pharmacological studies, we highlight a recent attempt of our own that has applied an optogenetic approach to stimulate the neural pathway from the FEF to the SC in macaque monkeys. This study has shown that optogenetic stimulation of the FEF-SC pathway is sufficiently effective not only to modulate SC neuron activity, but also to evoke saccadic eye movements. The same procedure will be relevant to elucidating other neural network functions as well and will provide significant advances in understanding of brain mechanisms underlying behavioral control in nonhuman primates.
Roles of the FEF-SC Pathway in Saccade Generation: Electrophysiological and Pharmacological Studies
In order to consider how the FEF and SC interact to regulate saccadic eye movements, it is crucial to determine what signals FEF neurons transmit to the SC. To address this issue, previous studies identified FEF neurons projecting to the SC and recorded the activity of these neurons in macaque monkeys performing a visually- or memory-guided saccade task (Segraves and Goldberg, 1987; Sommer and Wurtz, 2000, 2001). To identify the SC-projecting FEF neurons, they were antidromically activated by electrical microstimulation of the SC. Sommer and Wurtz (2000) found that the corticotectal neurons transmitted a variety of signals related to saccadic eye movements. These neurons exhibited visual responses to saccadic targets, tonic discharges during the delay period of the memory-guided saccade task, and/or burst firing before and after the execution of the eye movements. In addition, some corticotectal neurons showed discharges during fixation. Thus, the FEF relays visual-, memory-, motor- and even fixation-related signals to the SC (Figure 1). Since all these signals are observed in a broad population of FEF neurons as well, the FEF is likely to provide the SC with non-biased, general signals rather than any specific signals.
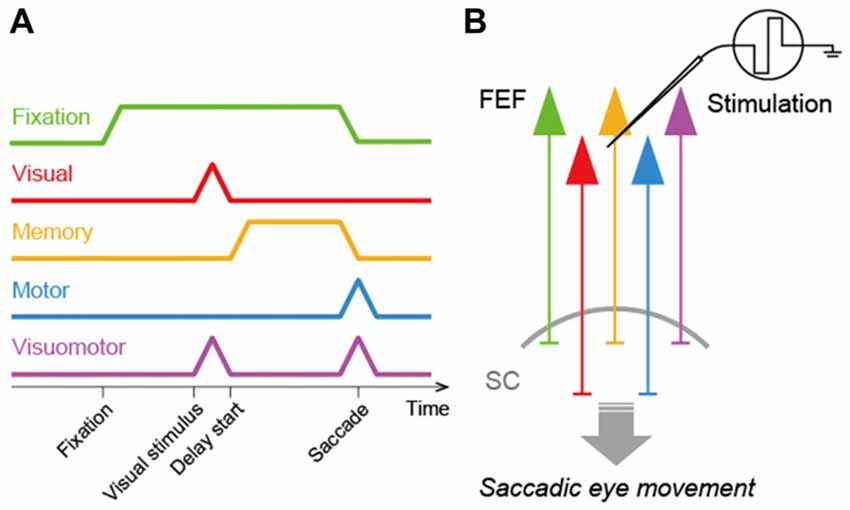
Figure 1. Signal transmission in the frontal eye field-superior colliculus (FEF-SC) pathway. (A) Diagram of signals transmitted from the FEF to the SC. The FEF transmits fixation- (green), visual- (red), memory- (orange), motor- (blue) and visuomotor- (purple) related signals to the SC. (B) Schema of the FEF-SC pathway. The effect of FEF stimulation on saccadic eye movements is predominantly mediated by this pathway.
In contrast with the above observations, however, Segraves and Goldberg (1987) reported that SC-projecting FEF neurons preferentially transmitted motor-related signals. They found that the corticotectal neurons had little or no response to visual stimuli, but exhibited very strong activity before both visually- and memory-guided saccadic eye movements. Sommer and Wurtz (2000) discussed why these two studies reached the contradictory results. The most critical reason may be related to the topographic distribution of responsive neurons. Although Sommer and Wurtz (2000) found that the corticotectal neurons conveyed a variety of signals, neuronal populations with distinct signals were distributed differently in the FEF. In fact, the neurons with motor-related signals tended to be located in the medial part of the FEF, and tended to project to the caudal level of the SC. If Segraves and Goldberg (1987) focused their recordings on the medial FEF or their stimulations on the caudal SC, this could account for why they observed a larger proportion of motor-related neurons than other neuron types. Consistent with the observations in the study of Sommer and Wurtz (2000); Everling and Munoz (2000) found that the antidromically-identified corticotectal neurons in the FEF transmitted not only motor-, but also visual-related signals during the performance of a pro- or an anti-saccade task.
The FEF sends projections not only to the SC, but also to other oculomotor structures in the brainstem (Stanton et al., 1988b). Therefore, the FEF might contribute to saccade generation via its output pathway to the brainstem that bypasses the SC. To test whether FEF signals transmitted to the SC participate in saccade generation, Hanes and Wurtz (2001) pharmacologically inactivated the SC in macaque monkeys and examined the effect of electrical microstimulation of the FEF on saccadic eye movements in the animals. In their experimental condition, the FEF-SC pathway was disrupted but the FEF-brainstem pathway was kept intact. If the FEF-brainstem pathway is more responsible for saccade generation than the FEF-SC pathway, then the FEF microstimulation is supposed to evoke saccadic eye movements even in SC-inactivated animals. Contrary to this supposition, they found that the SC inactivation eliminated saccadic eye movements evoked by the FEF microstimulation. Notably, this effect was observed when the stimulation site in the FEF and the inactivation site in the SC topographically overlapped. These findings suggest that the FEF-brainstem pathway is not sufficiently operative to generate saccadic eye movements on their own, and that FEF signals transmitted to the SC contribute to saccade generation. It should be noted, however, that bilateral lesions of the SC do not completely disrupt saccade generation. Schiller et al. (1980) reported that monkeys can produce voluntary saccadic eye movements even only 4 days after bilateral SC lesions, whereas paired lesions of the FEF and SC cause drastic deficits in the eye movements. These findings suggest that the FEF may possess functional routes that bypass the SC to generate saccadic eye movements. Such routes might not work in normal animals, but become active in the absence of the SC.
Roles of The FEF-SC Pathway in Saccade Generation: Optogenetic Approach
As mentioned above, Hanes and Wurtz (2001) showed the causal contribution of the FEF signals transmitted to the SC to saccade generation. However, the FEF not only directly projects to the SC, but also indirectly sends signals to the SC via other brain structures, such as the basal ganglia (for details, see the “Future Directions” section). It remains unclear whether the causal contribution is mediated by the direct or indirect pathway from the FEF to the SC. Optogenetics has provided a powerful tool to address these issues. For instance, neurons in a particular brain region are genetically modified to express channelrhodopsin-2 (ChR2), a blue-light-sensitive cation channel, by injecting a viral vector thereinto. Then, an optical fiber is placed into another region that receives projections from the infected region. Photo-stimulation of the ChR2 expressed on axon terminals induces a synaptic response to evoke signal transmission connecting the two regions. This methodology has brought substantial success in modulating behaviors in rodents (Stuber et al., 2011; Tye et al., 2011; Stamatakis and Stuber, 2012; Warden et al., 2012; Ahmari et al., 2013; Miyamoto et al., 2016), and has advanced our understanding of the roles of particular neural pathways in behaviors. However, the use of this methodology has greatly been restricted to small animals, and its application to primates which have much larger brains than rodents has so far been limited.
In our recent study (Inoue et al., 2015), we made an attempt to apply the optogenetic methodology to the primate oculomotor system that is substantially developed, as compared to the rodent system. Using this methodology, we stimulated the pathway from the FEF to the SC in macaque monkeys and analyzed the causal role of FEF signals transmitted to the SC in saccade generation (Figure 2A). An adeno-associated virus type 2 vector (AAV2-CMV-ChR2-EYFP) was injected unilaterally into the FEF, and, consequently, ChR2-positive axon terminals were observed in the ipsilateral SC, but not in the contralateral SC (Figure 2B). The axon terminals were stimulated by illuminating laser light into the SC. Through the optical stimulation of FEF axon terminals in the ipsilateral SC, many of recorded SC neurons exhibited an excitation that was sustained during laser light emission (left in Figure 2C), while only a few of them displayed an inhibition (right in Figure 2C). Thus, the direct stimulation of FEF signals transmitted to the SC can modulate SC neuron activity.
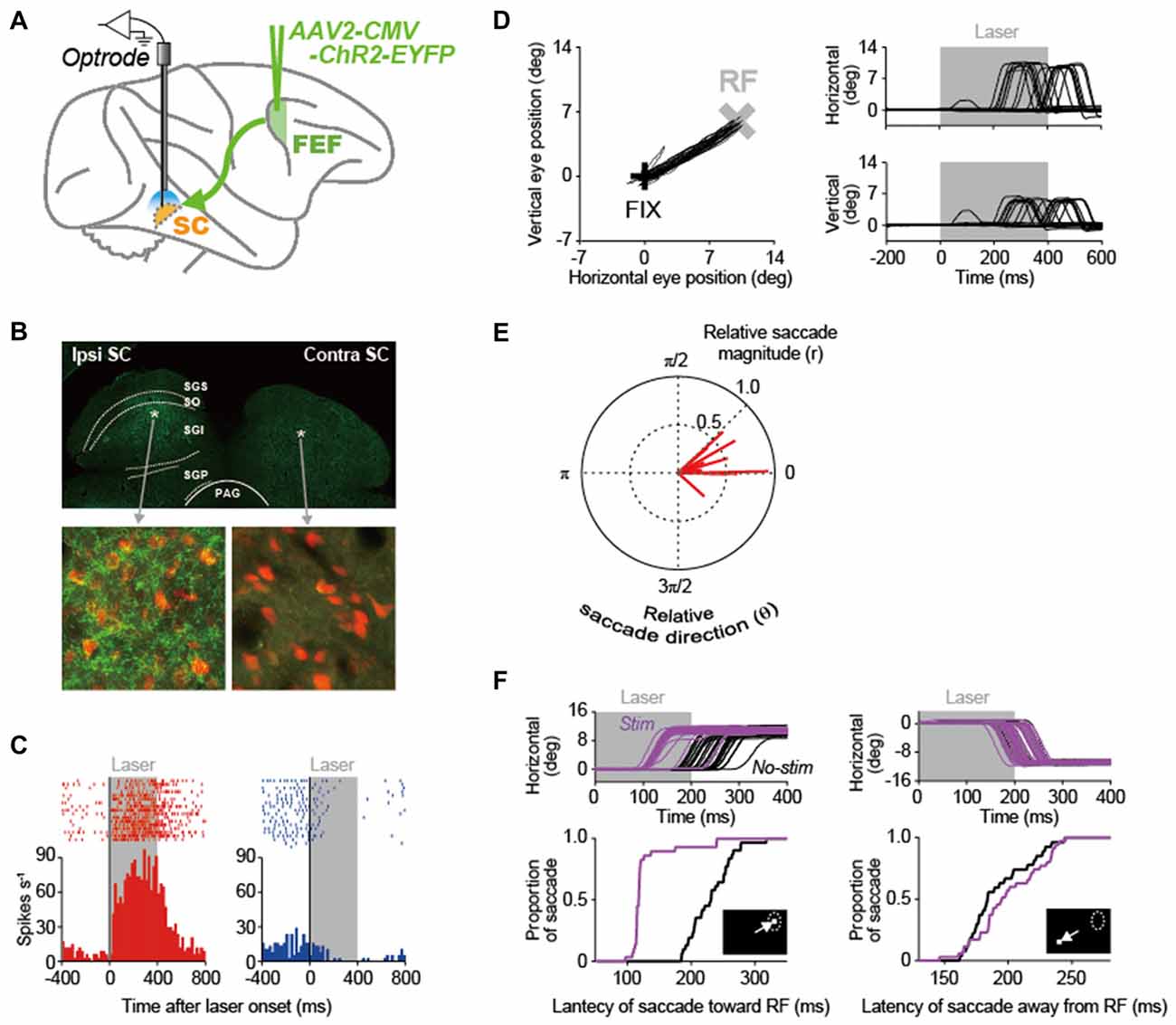
Figure 2. Optogenetic approach to elucidating the role of the FEF-SC pathway in oculomotor behavior. (A) Experimental design. In order to deliver the channelrhodopsin-2 (ChR2) gene into FEF neurons, adeno-associated virus type 2 vector (AAV2-CMV-ChR2-EYFP) was injected unilaterally into the FEF. Using optrodes (an optic fiber attached to a recording electrode), 473-nm blue laser light was emitted into the SC and single-unit activity was simultaneously recorded. (B) ChR2-EYFP expression in the SC. Top, wide-field immunofluorescent image of a coronal section through the SC. Bottom, immunofluorescence of YFP-expressing axons in the ipsilateral and contralateral SC. NeuN-expressing SC neurons are shown in red. Asterisks in the above panel indicate the locations of the ipsilateral and contralateral images in the SC. PAG, periaqueductal gray; SGI, stratum griseum intermediale; SGP, stratum griseum profundum; SGS, stratum griseum superficiale; SO, stratum opticum. (C) Activity of two neuron examples that were excited (left) and inhibited (right) during laser light emission in the ipsilateral SC. Gray areas indicate the period of laser light emission. (D) Saccadic eye movements evoked by optical stimulation at a representative site in the ipsilateral SC. Left, trajectories of eye positions. Black and gray crosses indicate the fixation point (FIX) and the center of the response field (RF) at the stimulation site in the SC. The FIX was kept on during laser light emission. Right, horizontal and vertical eye traces. (E) Polar plot of the magnitude (r) and direction (θ) of evoked saccades relative to the RF center of the stimulation sites. Red lines indicate the averaged vector of evoked saccades at each stimulation site (n = 15). Saccade toward the RF center is denoted with (r, θ) = (1.0, 0). (F) Effect of optical stimulation at a representative site in the ipsilateral SC on saccade latency. Top, horizontal eye traces. Bottom, cumulative distribution of the latency of saccades toward the RF (left) and those away from the RF (right). Purple and black curves indicate stimulated and non-stimulated saccades, respectively. Reproduced with permission from Inoue et al. (2015).
A pivotal issue of this research is whether the optogenetic methodology could induce behavioral modulations in primates. The optical stimulation of FEF axon terminals often evoked saccadic eye movements toward the response fields (RFs) corresponding to the stimulation sites in the SC (for a representative stimulation site, see Figure 2D). However, as a population, the magnitude of evoked saccadic eye movements was smaller than the eccentricity of the RF center (Figure 2E), suggesting that the intensity of optical stimulation was not enough to evoke full saccades that reach the eccentricity. The stimulation also modulated the latency of saccadic eye movements in a visually-guided saccade task in which the optical stimulation started simultaneously with the onset of a saccadic target that was presented inside or outside the RF. The stimulation decreased the latency of saccades toward the RF (for a representative stimulation site, see left in Figure 2F) and increased it away from the RF (see right in Figure 2F). These data indicate that stimulating the FEF-SC pathway, among the complex oculomotor network, is effective not only to modulate SC neuron activity, but also to initiate saccadic eye movements in macaque monkeys. The same optogenetic approach will be relevant to elucidating the roles of other neural pathways constituting the oculomotor network such as the pathway from the FEF to the lateral intraparietal area (LIP) and that from the LIP to the SC.
Future Directions
Here, we have introduced the previous studies reporting what signals FEF neurons transmit to the SC and the causal contribution of these signals to saccade generation. However, it is not yet completely clear how the FEF and SC interact to generate saccadic eye movements. For instance, although FEF neurons seem to transmit visual-, memory- and motor-related signals to the SC, it remains to be determined which of these signals are involved in saccade generation. A recent study applied an optogenetic approach to the FEF in macaque monkeys and suppressed the activity of FEF neurons at any given timing in a memory-guided saccade task (Acker et al., 2016). This study found that suppression of FEF neurons during the visual, delay (i.e., memory), or movement periods altered the pattern of saccadic eye movements, suggesting that all of the FEF signals contribute to memory-guided saccades. By applying the circuit-level manipulation to their study, the role of each FEF signal transmitted to the SC in saccade generation will be determined.
The FEF is known to transmit signals related not only to simple saccade generation, but also to several executive functions, such as visual attention (Kodaka et al., 1997; Schall, 2004; Thompson et al., 2005), saccadic response inhibition (Hanes et al., 1998), and working memory (Sommer and Wurtz, 2001; Umeno and Goldberg, 2001). Some of these signals have been shown to be transmitted to the SC (Everling and Munoz, 2000; Sommer and Wurtz, 2001). For instance, Everling and Munoz (2000) identified FEF neurons projecting to the SC using antidromic stimulation in monkeys, and recorded their activity while the monkey was performing a pro- or an anti-saccade task. They found that saccade-related corticotectal neurons were inhibited in the context in which the monkey was required to inhibit a reflexive saccadic eye movement toward a visual stimulus (i.e., anti-saccade task) compared with the context in which the animal was simply required to make the reflexive eye movement (i.e., pro-saccade task). Their findings suggest that the FEF transmits signals involved in the executive control of oculomotor behavior to the SC. It remains to be determined, however, what roles such corticotectal signals play in regulating executive functions. The electrophysiological, pharmacological and optogenetic approaches that we have introduced above will be applicable to address this issue.
Neurons in the FEF exert their effects on the SC not only through the direct projection to the SC, especially to its deeper layers, but also by way of the basal ganglia. The caudate nucleus and the rostral part of the putamen, input stations of the basal ganglia, receive projections from the FEF (Stanton et al., 1988a; Parthasarathy et al., 1992; Neggers et al., 2015) and, in turn, send projections to the substantia nigra pars reticulata (SNr; Parent et al., 1984;François et al., 1987), an output station of the basal ganglia. Then, the SNr sends projections to the deeper layers of the SC (Beckstead et al., 1981; Francois et al., 1984). Thus, the basal ganglia appear to mediate signal transmission from the FEF to the SC. Notably, since the SNr sends GABAergic projections to the SC (Vincent et al., 1978; Di Chiara et al., 1979), the SNr is most likely to exert a strong inhibitory effect on the activity of SC neurons (Hikosaka and Wurtz, 1983). In particular, it has been proposed that the SNr contributes to saccade generation by disinhibiting SC neuron activity (Hikosaka et al., 2000), although the exact role of the FEF-basal ganglia-SC pathway is still unclear. It remains to be determined how the two parallel pathways, i.e., the FEF-SC and FEF-basal ganglia-SC pathways, cooperate in regulating saccadic eye movements.
There are other brain structures than the basal ganglia that connect the FEF and SC. Among them, the cerebellum has attracted attention for its crucial roles in voluntary eye movements (Robinson and Fuchs, 2001). The anatomical organization of cerebro-cerebellar circuits has been established especially by Strick and colleagues (Lynch et al., 1994; Kelly and Strick, 2003; Strick et al., 2009). At least part of the cerebellum projects directly to the SC (May et al., 1990). The cerebellum also communicates with the basal ganglia multisynaptically (Hoshi et al., 2005; Bostan et al., 2010).
We have so far introduced prior attempts that investigated the role of signals transmitted from the FEF to the SC. On the other hand, the SC sends back signals to the FEF via the MD (Sommer and Wurtz, 2002, 2004a,b). It has previously been shown that the SC-MD-FEF pathway transmit signals related to the corollary discharge (or internal copy) of oculomotor command, and that pharmacological inactivation of the MD impairs sequential eye movements consistent with the loss of the corollary discharge (Sommer and Wurtz, 2002, 2004b). Understanding how the entire oculomotor network including the FEF, SC, basal ganglia, cerebellum and MD generates oculomotor behavior is a challenging issue. Optogenetic techniques applicable to primates could have advantages to address this issue.
Author Contributions
MM conceived of the review topic and all authors wrote the review article.
Funding
This work was supported by Grants-in-Aid for Scientific Research (15H05879 and 17H05565 to KI, 16H02454 to MT and 26120707, 26710001 and 16H06567 to MM) from the Ministry of Education, Science, Sports, Culture and Technology of Japan; PREST (JPMJPR1683) from the Japan Science and Technology Agency (JST) to KI; Funding Program for Next Generation World-Leading Researchers (LS074) to MM from Cabinet Office, Government of Japan; the Takeda Science Foundation to MM; the Inamori Foundation to MM; and the Uehara Memorial Foundation to MM.
Conflict of Interest Statement
The authors declare that the research was conducted in the absence of any commercial or financial relationships that could be construed as a potential conflict of interest.
References
Acker, L., Pino, E. N., Boyden, E. S., and Desimone, R. (2016). FEF inactivation with improved optogenetic methods. Proc. Natl. Acad. Sci. U S A 113, E7297–E7306. doi: 10.1073/pnas.1610784113
Ahmari, S. E., Spellman, T., Douglass, N. L., Kheirbek, M. A., Simpson, H. B., Deisseroth, K., et al. (2013). Repeated cortico-striatal stimulation generates persistent OCD-like behavior. Science 340, 1234–1239. doi: 10.1126/science.1234733
Beckstead, R. M., Edwards, S. B., and Frankfurter, A. (1981). A comparison of the intranigral distribution of nigrotectal neurons labeled with horseradish peroxidase in the monkey, cat and rat. J. Neurosci. 1, 121–125. doi: 10.1523/JNEUROSCI.01-02-00121.1981
Bernstein, J. G., and Boyden, E. S. (2011). Optogenetic tools for analyzing the neural circuits of behavior. Trends Cogn. Sci. 15, 592–600. doi: 10.1016/j.tics.2011.10.003
Bizzi, E. (1968). Discharge of frontal eye field neurons during saccadic and following eye movements in unanesthetized monkeys. Exp. Brain Res. 6, 69–80. doi: 10.1007/bf00235447
Bostan, A. C., Dum, R. P., and Strick, P. L. (2010). The basal ganglia communicate with the cerebellum. Proc. Natl. Acad. Sci. U S A 107, 8452–8456. doi: 10.1073/pnas.1000496107
Bruce, C. J., and Goldberg, M. E. (1985). Primate frontal eye fields. I. Single neurons discharging before saccades. J. Neurophysiol. 53, 603–635. doi: 10.1152/jn.1985.53.3.603
Bruce, C. J., Goldberg, M. E., Bushnell, M. C., and Stanton, G. B. (1985). Primate frontal eye fields. II. Physiological and anatomical correlates of electrically evoked eye movements. J. Neurophysiol. 54, 714–734. doi: 10.1152/jn.1985.54.3.714
Chafee, M. V., and Goldman-Rakic, P. S. (2000). Inactivation of parietal and prefrontal cortex reveals interdependence of neural activity during memory-guided saccades. J. Neurophysiol. 83, 1550–1566. doi: 10.1152/jn.2000.83.3.1550
Di Chiara, G., Porceddu, M. L., Morelli, M., Mulas, M. L., and Gessa, G. L. (1979). Evidence for a GABAergic projection from the substantia nigra to the ventromedial thalamus and to the superior colliculus of the rat. Brain Res. 176, 273–284. doi: 10.1016/0006-8993(79)90983-1
Dias, E. C., Kiesau, M., and Segraves, M. A. (1995). Acute activation and inactivation of macaque frontal eye field with GABA-related drugs. J. Neurophysiol. 74, 2744–2748. doi: 10.1152/jn.1995.74.6.2744
Dias, E. C., and Segraves, M. A. (1999). Muscimol-induced inactivation of monkey frontal eye field: effects on visually and memory-guided saccades. J. Neurophysiol. 81, 2191–2214. doi: 10.1152/jn.1999.81.5.2191
Everling, S., and Munoz, D. P. (2000). Neuronal correlates for preparatory set associated with pro-saccades and anti-saccades in the primate frontal eye field. J. Neurosci. 20, 387–400. doi: 10.1523/JNEUROSCI.20-01-00387.2000
Francois, C., Percheron, G., and Yelnik, J. (1984). Localization of nigrostriatal, nigrothalamic and nigrotectal neurons in ventricular coordinates in macaques. Neuroscience 13, 61–76. doi: 10.1016/0306-4522(84)90259-8
François, C., Yelnik, J., and Percheron, G. (1987). Golgi study of the primate substantia nigra. II. Spatial organization of dendritic arborizations in relation to the cytoarchitectonic boundaries and to the striatonigral bundle. J. Comp. Neurol. 265, 473–493. doi: 10.1002/cne.902650403
Fries, W. (1984). Cortical projections to the superior colliculus in the macaque monkey: a retrograde study using horseradish peroxidase. J. Comp. Neurol. 230, 55–76. doi: 10.1002/cne.902300106
Hanes, D. P., Patterson, W. F. II., and Schall, J. D. (1998). Role of frontal eye fields in countermanding saccades: visual, movement and fixation activity. J. Neurophysiol. 79, 817–834. doi: 10.1152/jn.1998.79.2.817
Hanes, D. P., and Wurtz, R. H. (2001). Interaction of the frontal eye field and superior colliculus for saccade generation. J. Neurophysiol. 85, 804–815. doi: 10.1152/jn.2001.85.2.804
Hikosaka, O., and Wurtz, R. H. (1983). Visual and oculomotor functions of monkey substantia nigra pars reticulata. IV. Relation of substantia nigra to superior colliculus. J. Neurophysiol. 49, 1285–1301. doi: 10.1152/jn.1983.49.5.1285
Hikosaka, O., and Wurtz, R. H. (1985). Modification of saccadic eye movements by GABA-related substances. I. Effect of muscimol and bicuculline in monkey superior colliculus. J. Neurophysiol. 53, 266–291. doi: 10.1152/jn.1985.53.1.266
Hikosaka, O., Takikawa, Y., and Kawagoe, R. (2000). Role of the basal ganglia in the control of purposive saccadic eye movements. Physiol. Rev. 80, 953–978. doi: 10.1152/physrev.2000.80.3.953
Hoshi, E., Tremblay, L., Féger, J., Carras, P. L., and Strick, P. L. (2005). The cerebellum communicates with the basal ganglia. Nat. Neurosci. 8, 1491–1493. doi: 10.1038/nn1544
Inoue, K., Takada, M., and Matsumoto, M. (2015). Neuronal and behavioural modulations by pathway-selective optogenetic stimulation of the primate oculomotor system. Nat. Commun. 6:8378. doi: 10.1038/ncomms9378
Kelly, R. M., and Strick, P. L. (2003). Cerebellar loops with motor cortex and prefrontal cortex of a nonhuman primate. J. Neurosci. 23, 8432–8444. doi: 10.1523/JNEUROSCI.23-23-08432.2003
Kodaka, Y., Mikami, A., and Kubota, K. (1997). Neuronal activity in the frontal eye field of the monkey is modulated while attention is focused on to a stimulus in the peripheral visual field, irrespective of eye movement. Neurosci. Res. 28, 291–298. doi: 10.1016/s0168-0102(97)00055-2
Komatsu, H., and Suzuki, H. (1985). Projections from the functional subdivisions of the frontal eye field to the superior colliculus in the monkey. Brain Res. 327, 324–327. doi: 10.1016/0006-8993(85)91528-8
Lynch, J. C., Hoover, J. E., and Strick, P. L. (1994). Input to the primate frontal eye field from the substantia nigra, superior colliculus and dentate nucleus demonstrated by transneuronal transport. Exp. Brain Res. 100, 181–186. doi: 10.1007/bf00227293
May, P. J., Hartwich-Young, R., Nelson, J., Sparks, D. L., and Porter, J. D. (1990). Cerebellotectal pathways in the macaque: implications for collicular generation of saccades. Neuroscience 36, 305–324. doi: 10.1016/0306-4522(90)90428-7
Miyamoto, D., Hirai, D., Fung, C. C., Inutsuka, A., Odagawa, M., Suzuki, T., et al. (2016). Top-down cortical input during NREM sleep consolidates perceptual memory. Science 352, 1315–1318. doi: 10.1126/science.aaf0902
Mohler, C. W., Goldberg, M. E., and Wurtz, R. H. (1973). Visual receptive fields of frontal eye field neurons. Brain Res. 61, 385–389. doi: 10.1016/0006-8993(73)90543-x
Neggers, S. F., Zandbelt, B. B., Schall, M. S., and Schall, J. D. (2015). Comparative diffusion tractography of corticostriatal motor pathways reveals differences between humans and macaques. J. Neurophysiol. 113, 2164–2172. doi: 10.1152/jn.00569.2014
Parent, A., Bouchard, C., and Smith, Y. (1984). The striatopallidal and striatonigral projections: two distinct fiber systems in primate. Brain Res. 303, 385–390. doi: 10.1016/0006-8993(84)91224-1
Parthasarathy, H. B., Schall, J. D., and Graybiel, A. M. (1992). Distributed but convergent ordering of corticostriatal projections: analysis of the frontal eye field and the supplementary eye field in the macaque monkey. J. Neurosci. 12, 4468–4488. doi: 10.1523/JNEUROSCI.12-11-04468.1992
Robinson, D. A. (1972). Eye movements evoked by collicular stimulation in the alert monkey. Vision Res. 12, 1795–1808. doi: 10.1016/0042-6989(72)90070-3
Robinson, D. A., and Fuchs, A. F. (1969). Eye movements evoked by stimulation of frontal eye fields. J. Neurophysiol. 32, 637–648. doi: 10.1152/jn.1969.32.5.637
Robinson, F. R., and Fuchs, A. F. (2001). The role of the cerebellum in voluntary eye movements. Annu. Rev. Neurosci. 24, 981–1004. doi: 10.1146/annurev.neuro.24.1.981
Schall, J. D. (2004). On the role of frontal eye field in guiding attention and saccades. Vision Res. 44, 1453–1467. doi: 10.1016/j.visres.2003.10.025
Schiller, P. H., and Koerner, F. (1971). Discharge characteristics of single units in superior colliculus of the alert rhesus monkey. J. Neurophysiol. 34, 920–936. doi: 10.1152/jn.1971.34.5.920
Schiller, P. H., and Stryker, M. (1972). Single-unit recording and stimulation in superior colliculus of the alert rhesus monkey. J. Neurophysiol. 35, 915–924. doi: 10.1152/jn.1972.35.6.915
Schiller, P. H., True, S. D., and Conway, J. L. (1980). Deficits in eye movements following frontal eye-field and superior colliculus ablations. J. Neurophysiol. 44, 1175–1189. doi: 10.1152/jn.1980.44.6.1175
Segraves, M. A., and Goldberg, M. E. (1987). Functional properties of corticotectal neurons in the monkey’s frontal eye field. J. Neurophysiol. 58, 1387–1419. doi: 10.1152/jn.1987.58.6.1387
Sommer, M. A., and Tehovnik, E. J. (1997). Reversible inactivation of macaque frontal eye field. Exp. Brain Res. 116, 229–249. doi: 10.1007/pl00005752
Sommer, M. A., and Tehovnik, E. J. (1999). Reversible inactivation of macaque dorsomedial frontal cortex: effects on saccades and fixations. Exp. Brain Res. 124, 429–446. doi: 10.1007/s002210050639
Sommer, M. A., and Wurtz, R. H. (2000). Composition and topographic organization of signals sent from the frontal eye field to the superior colliculus. J. Neurophysiol. 83, 1979–2001. doi: 10.1152/jn.2000.83.4.1979
Sommer, M. A., and Wurtz, R. H. (2001). Frontal eye field sends delay activity related to movement, memory and vision to the superior colliculus. J. Neurophysiol. 85, 1673–1685. doi: 10.1152/jn.2001.85.4.1673
Sommer, M. A., and Wurtz, R. H. (2002). A pathway in primate brain for internal monitoring of movements. Science 296, 1480–1482. doi: 10.1126/science.1069590
Sommer, M. A., and Wurtz, R. H. (2004a). What the brain stem tells the frontal cortex. I. Oculomotor signals sent from superior colliculus to frontal eye field via mediodorsal thalamus. J. Neurophysiol. 91, 1381–1402. doi: 10.1152/jn.00738.2003
Sommer, M. A., and Wurtz, R. H. (2004b). What the brain stem tells the frontal cortex. II. Role of the SC-MD-FEF pathway in corollary discharge. J. Neurophysiol. 91, 1403–1423. doi: 10.1152/jn.00740.2003
Stamatakis, A. M., and Stuber, G. D. (2012). Activation of lateral habenula inputs to the ventral midbrain promotes behavioral avoidance. Nat. Neurosci. 15, 1105–1107. doi: 10.1038/nn.3145
Stanton, G. B., Goldberg, M. E., and Bruce, C. J. (1988a). Frontal eye field efferents in the macaque monkey: I. Subcortical pathways and topography of striatal and thalamic terminal fields. J. Comp. Neurol. 271, 473–492. doi: 10.1002/cne.902710402
Stanton, G. B., Goldberg, M. E., and Bruce, C. J. (1988b). Frontal eye field efferents in the macaque monkey: II. Topography of terminal fields in midbrain and pons. J. Comp. Neurol. 271, 493–506. doi: 10.1002/cne.902710403
Strick, P. L., Dum, R. P., and Fiez, J. A. (2009). Cerebellum and nonmotor function. Annu. Rev. Neurosci. 32, 413–434. doi: 10.1146/annurev.neuro.31.060407.125606
Stuber, G. D., Sparta, D. R., Stamatakis, A. M., van Leeuwen, W. A., Hardjoprajitno, J. E., Cho, S., et al. (2011). Excitatory transmission from the amygdala to nucleus accumbens facilitates reward seeking. Nature 475, 377–380. doi: 10.1038/nature10194
Thompson, K. G., Biscoe, K. L., and Sato, T. R. (2005). Neuronal basis of covert spatial attention in the frontal eye field. J. Neurosci. 25, 9479–9487. doi: 10.1523/JNEUROSCI.0741-05.2005
Tye, K. M., and Deisseroth, K. (2012). Optogenetic investigation of neural circuits underlying brain disease in animal models. Nat. Rev. Neurosci. 13, 251–266. doi: 10.1038/nrn3171
Tye, K. M., Prakash, R., Kim, S. Y., Fenno, L. E., Grosenick, L., Zarabi, H., et al. (2011). Amygdala circuitry mediating reversible and bidirectional control of anxiety. Nature 471, 358–362. doi: 10.1038/nature09820
Umeno, M. M., and Goldberg, M. E. (2001). Spatial processing in the monkey frontal eye field. II. Memory responses. J. Neurophysiol. 86, 2344–2352. doi: 10.1152/jn.2001.86.5.2344
Vincent, S. R., Hattori, T., and McGeer, E. G. (1978). The nigrotectal projection: a biochemical and ultrastructural characterization. Brain Res. 151, 159–164. doi: 10.1016/0006-8993(78)90959-9
Warden, M. R., Selimbeyoglu, A., Mirzabekov, J. J., Lo, M., Thompson, K. R., Kim, S. Y., et al. (2012). A prefrontal cortex-brainstem neuronal projection that controls response to behavioural challenge. Nature 492, 428–432. doi: 10.1038/nature11617
Keywords: frontal eye field, superior colliculus, saccade, optogenetics, macaque monkey
Citation: Matsumoto M, Inoue K and Takada M (2018) Causal Role of Neural Signals Transmitted From the Frontal Eye Field to the Superior Colliculus in Saccade Generation. Front. Neural Circuits 12:69. doi: 10.3389/fncir.2018.00069
Received: 26 February 2018; Accepted: 07 August 2018;
Published: 28 August 2018.
Edited by:
Tadashi Isa, Kyoto University, JapanReviewed by:
Marc A. Sommer, Duke University, United StatesLaurent Petit, Centre National de la Recherche Scientifique (CNRS), France
Ziad M. Hafed, Universität Tübingen, Germany
Copyright © 2018 Matsumoto, Inoue and Takada. This is an open-access article distributed under the terms of the Creative Commons Attribution License (CC BY). The use, distribution or reproduction in other forums is permitted, provided the original author(s) and the copyright owner(s) are credited and that the original publication in this journal is cited, in accordance with accepted academic practice. No use, distribution or reproduction is permitted which does not comply with these terms.
*Correspondence: Masayuki Matsumoto, mmatsumoto@md.tsukuba.ac.jp