Presynaptic [Ca2+] and GCAPs: aspects on the structure and function of photoreceptor ribbon synapses
- Department of Neuroanatomy, Institute for Anatomy and Cell Biology, Medical School Homburg/Saar, Saarland University, Saarland, Germany
Changes in intracellular calcium ions [Ca2+] play important roles in photoreceptor signaling. Consequently, intracellular [Ca2+] levels need to be tightly controlled. In the light-sensitive outer segments (OS) of photoreceptors, Ca2+ regulates the activity of retinal guanylate cyclases thus playing a central role in phototransduction and light-adaptation by restoring light-induced decreases in cGMP. In the synaptic terminals, changes of intracellular Ca2+ trigger various aspects of neurotransmission. Photoreceptors employ tonically active ribbon synapses that encode light-induced, graded changes of membrane potential into modulation of continuous synaptic vesicle exocytosis. The active zones of ribbon synapses contain large electron-dense structures, synaptic ribbons, that are associated with large numbers of synaptic vesicles. Synaptic coding at ribbon synapses differs from synaptic coding at conventional (phasic) synapses. Recent studies revealed new insights how synaptic ribbons are involved in this process. This review focuses on the regulation of [Ca2+] in presynaptic photoreceptor terminals and on the function of a particular Ca2+-regulated protein, the neuronal calcium sensor protein GCAP2 (guanylate cyclase-activating protein-2) in the photoreceptor ribbon synapse. GCAP2, an EF-hand-containing protein plays multiple roles in the OS and in the photoreceptor synapse. In the OS, GCAP2 works as a Ca2+-sensor within a Ca2+-regulated feedback loop that adjusts cGMP levels. In the photoreceptor synapse, GCAP2 binds to RIBEYE, a component of synaptic ribbons, and mediates Ca2+-dependent plasticity at that site. Possible mechanisms are discussed.
Introduction
Presynaptic [Ca2+]: Aspects on the Structure and Function of Photoreceptor Ribbon Synapses
Changes in presynaptic free cytosolic Ca2+ ions trigger important functions in the presynaptic terminals. These include synaptic vesicles exocytosis and the mediation of various forms of synaptic plasticity (Bliss and Collingridge, 1993; Malenka and Nicoll, 1999; Neher and Sakaba, 2008; Kawamoto et al., 2012; Rizo and Südhof, 2012; Catterall et al., 2013; Südhof, 2013). Ca2+ ions also have fundamental functions in photoreceptor synapses. Photoreceptors employ tonically active ribbon synapses that encode light-induced, graded changes of membrane potential into modulation of continuous synaptic vesicle exocytosis to transmit the light-induced signals to the inner retina. The active zones of ribbon synapses contain large electron-dense structures, synaptic ribbons, that are associated with large numbers of synaptic vesicles. Synaptic ribbons have a key importance for signaling at the ribbon synapse. This review focuses on the regulation of [Ca2+] in presynaptic photoreceptor terminals and on the function of a particular Ca2+-regulated protein, the neuronal calcium sensor (NCS) protein GCAP2 (guanylate cyclase-activating protein-2). GCAP2, an EF-hand-containing, Ca2+-binding protein is strongly expressed in photoreceptors and plays multiple roles in the outer segments (OS) and in the photoreceptor synapse.
Photoreceptors
Photoreceptors are the principal light-sensitive neurons in the outer retina. They detect incoming light quanta (photons) and communicate the information to the inner retina for further processing. Rod photoreceptors operate at low light intensities and are capable of single-photon-detection. Cone photoreceptors operate at daylight conditions and mediate color vision (Abramov and Gordon, 1994). Both types of photoreceptors display a bipolar morphology with two processes emanating from opposite ends of the photoreceptor cell body (Figure 1A). (1) An outer process forms the outer segment (OS) that is responsible for phototransduction. During this process, light-sensitive photopigments in the OS transform light quanta into a change of the photoreceptor membrane potential. (2) An inner cell process emerges from the vitread portion of the photoreceptor soma. This inner process ends in a specialized presynaptic terminal that contains synaptic ribbons in their active zones (Figures 1A and 2). At these ribbon-type active zones, signals generated by the absorption of light quanta in the OS are continuously transmitted to the inner retina (for review, see Wässle, 2004; Nassi and Callaway, 2009; Lee et al., 2010).
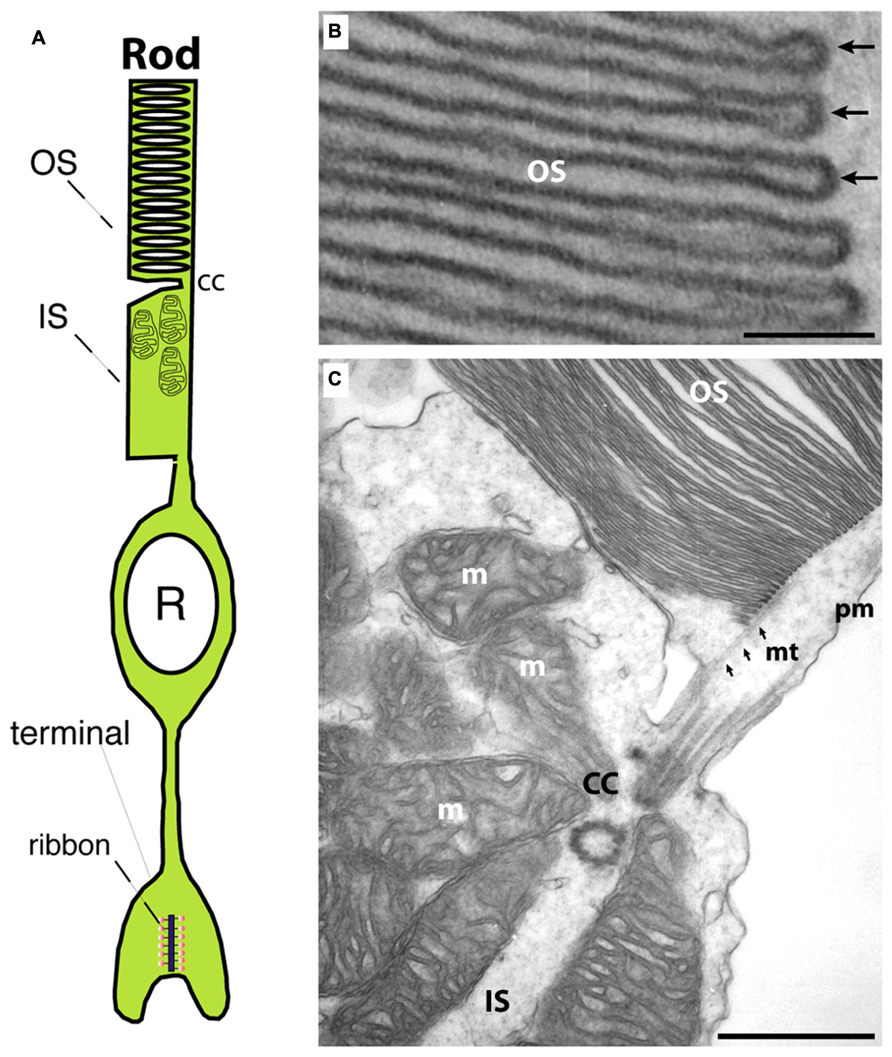
FIGURE 1. (A) Photoreceptors show a bipolar morphology. A distal process forms the outer segment (OS) and the inner segment (IS). The OS is the place where phototransduction takes place. The IS represents the metabolic center of the photoreceptor. An inner, vitread process ends in the presynaptic terminal that contains a synaptic ribbon at the active zone. (B) Transmission electron micrograph of an outer segment (OS). Densely packed arrays of membrane disks can be observed. The arrows in (B) point to individual disks. In the OS, phototransduction occurs and GCAP proteins perform a Ca2+-dependent feedback on guanylate cyclases. (C) Transmission electron micrograph at the junctional zone between OS and IS. OS and IS are connected by the connecting cilum (cc) that organizes mt-dependent vesicle transport into the outer segment (Sung and Chuang, 2010). OS, outer segment; IS, inner segment; m, mitochondrion; cc, connecting cilium linking OS and IS; mt, microtubule; pm, plasma membrane. Scale bars: 50 nm (B), 250 nm (C).
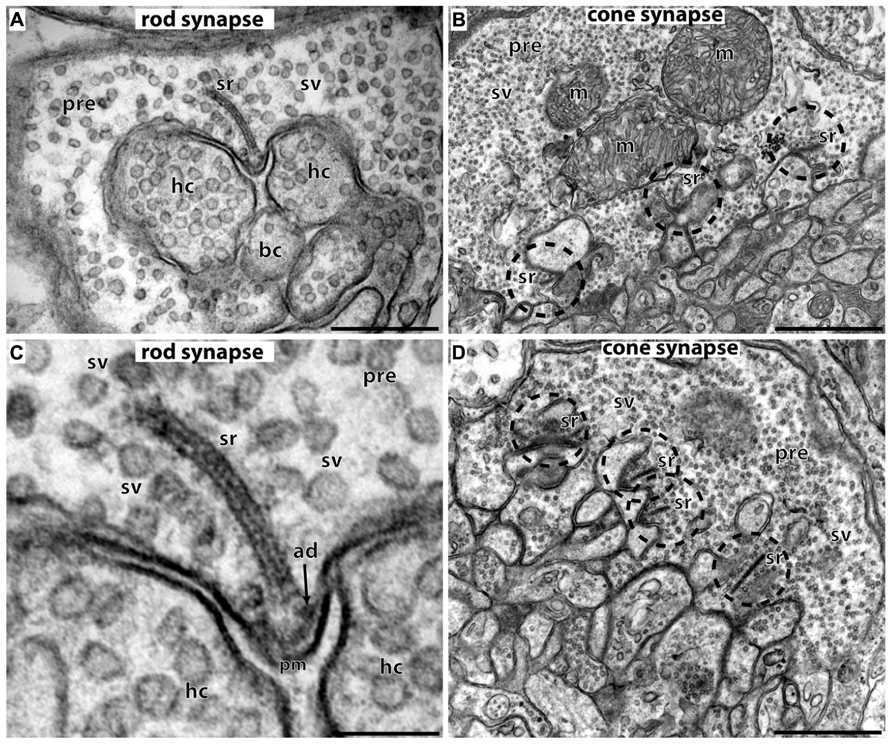
FIGURE 2. Photoreceptor ribbon synapses. In (A,C) rod photoreceptor ribbon synapses are shown by transmission electron microscopy; in (B,D) cone photoreceptor ribbon synapses. (A,B) The large presynaptic terminals are filled with numerous synaptic vesicles (sv). The active zone is characterized by specialized presynaptic densities, the arciform densities (ad). The synaptic ribbon (sr) is anchored to the arciform density. The synaptic ribbon is associated with large numbers of synaptic vesicles. Opposite to the active zones are the dendritic tips of horizontal cells (hc) and bipolar cells (bc) that contain ionotropic and metabotropic glutamate receptors for signaling. The dendritic tips are located within an invagination of the presynaptic terminal. Cone synapses (B,D) are larger in diameter than rod synapses and contain multiple active zones (dashed circles) and multiple synaptic ribbons (sr). sr, synaptic ribbon; sv, synaptic vesicles; ad, arciform density; pre, presynaptic terminal; m, mitochondrium; hc, dendritic tips of horizontal cells; bc, dendritic tips of bipolar cells; pm, presynaptic plasma membrane. Scale bars: 400 nm (A), 1 μm (B), 150 nm (C), 800 nm (D).
Ca2+-Dependent Regulation of Phototransduction in Photoreceptor Outer Segments
The OS contain dense and regular intracellular staples of membrane disks (for rods, Figure 1; for review, see Mustafi et al., 2009). These membrane-specializations are enriched with light-sensitive opsin-containing photopigments (Burns and Baylor, 2001; Chen, 2005). As light quanta are detected by the photopigments in the OS, a signal transduction cascade is initiated that finally leads via G-protein-mediated activation of a phosphodiesterase, phosphodiesterase 6 (PDE6), to the hydrolysis of cGMP (Burns and Baylor, 2001; Chen, 2005; Dell’Orco and Koch, 2010). The drop in cGMP leads to the closure of cyclic nucleotide-gated cation channels (CNG-channels) in the plasma membrane, a non-selective cation channel through which both Na+- and Ca2+-ions can pass from extra- to intracellular space along their electrochemical gradient. The light-induced closure of the CNG-channels generates a hyperpolarization response. The membrane potential ranges from ≈-40 mV in the dark to ≈-70 mV in bright light. The phototransduction system has a high dynamic range that is adjusted – not exclusively, but to a considerable extent – by Ca2+-dependent processes (Arshavsky, 2002; Korenbrot and Rebrik, 2002; Sharma, 2002, 2010; Koch, 2006; Artemyev, 2008; Stephen et al., 2008; Dell’Orco and Koch, 2010; Sharma and Duda, 2012). The light-induced drop of Ca2+ is sensed by Ca2+-binding, EF-hand-containing proteins, the guanylate cyclase-activating proteins (GCAPs; Korenbrot and Rebrik, 2002; Palczewski et al., 2004; Koch, 2006; Sakurai et al., 2011; Sharma and Duda, 2012). GCAPs are members of the family of NCS proteins (Sharma, 2002, 2010; Koch, 2006; Burgoyne, 2007; Baehr and Palczewski, 2009; Koch et al., 2010; Schmitz et al., 2012). GCAP2 (as well as its close relatives GCAP1 and GCAP3; for review, see Palczewski et al., 2004) contains four EF-hands. The first EF-hand is not able to bind Ca2+ due to exchange of critical amino acids (Palczewski et al., 2004; Koch, 2006; Burgoyne, 2007; Schmitz et al., 2012). Thus, under light stimulation, when Ca2+ is low, GCAPs activate the photoreceptor retinal guanylate cyclase (ret-GC) thus restoring cGMP baseline levels (Burns and Baylor, 2001; Sharma, 2002, 2010; Chen, 2005; Sharma and Duda, 2012). In the dark, at elevated Ca2+, GCAPs inhibit ret-GCs activity and prevent over-production of cGMP. These Ca2+-/GCAP-dependent processes set the dynamic range of phototransduction and keep the OS and phototransduction operational at different levels of photon fluxes (Koch, 2006; Stephen et al., 2008; Sharma and Duda, 2012). GCAPs are myristoylated at their N-terminus. Remarkably, in contrast to other members of the NCS family, GCAPs do not perform a Ca2+-dependent myristoyl switch at the N-terminus (Stephen et al., 2007). Instead, the N-terminal myristoyl-group remains buried in the inside of the molecule both in the Ca2+-bound and Ca2+-free state where it is essential to stabilize the protein (Stephen et al., 2007). Unusually, the C-terminus of GCAP2 performs a Ca2+-dependent conformational change (Peshenko et al., 2004).
The Photoreceptor Ribbon Synapse of the Mature Retina: Ultrastructure and Molecular Anatomy of the Synaptic Ribbon Complex
Photoreceptors are non-spiking neurons and communicate light-induced, graded changes of membrane potential into modulations of tonic neurotransmitter release rates at the presynaptic terminals (Heidelberger et al., 2005; Matthews and Fuchs, 2010; Mercer and Thoreson, 2011). For this purpose, photoreceptor terminals are equipped with large active zones to which conspicuous presynaptic specializations, the synaptic ribbons, are attached (for review, see tom Dieck and Brandstätter, 2006; Schmitz, 2009; Matthews and Fuchs, 2010; Mercer and Thoreson, 2011; Schmitz et al., 2012).
The synaptic ribbon is a large, electron-dense structure that is associated with large numbers of synaptic vesicles along its entire surface (Figure 2; see also Schmitz, 2009). In photoreceptor ribbon synapses, the synaptic ribbon usually appears as a bar-shaped structure of several 100 nm in length in the transmission electron microscope (Schmitz, 2009; Mercer and Thoreson, 2011; Schmitz et al., 2012). Three-dimensional reconstructions of the synaptic ribbon revealed that this bar-shaped profile is a cross-section of a plate-shaped structure of the synaptic ribbon (Figures 2 and 3E; Rao-Mirotznik et al., 1995; for review, see also Schmitz, 2009; Mercer and Thoreson, 2011; Schmitz et al., 2012). Furthermore, the synaptic ribbon is bended in a horseshoe-shaped manner along the invaginated presynaptic plasma membrane of the photoreceptor terminal. The horseshoe-shaped synaptic ribbon can have a depth of about 1 μm (Schmitz, 2009; Schmitz et al., 2012). Rod photoreceptor presynaptic terminals usually possess a single active zone and a single synaptic ribbon; cone presynaptic terminals possess multiple active zones and multiple, though typically smaller synaptic ribbons (Figure 2; Wässle, 2004; Schmitz, 2009; Mercer and Thoreson, 2011; Regus-Leidig and Brandstätter, 2012; Schmitz et al., 2012). In photoreceptor ribbon synapses, synaptic ribbons are anchored to the arciform density. The arciform density corresponds to the presynaptic projections of conventional synapses (tom Dieck and Brandstätter, 2006; Schmitz, 2009; Mercer and Thoreson, 2011; Figure 2). At the plasma membrane of the active zone, L-type voltage-gated Ca2+-channels (VGCC) are clustered. Ca2+-influx through these channels triggers release at the photoreceptor synapse (see below). The active zone with the attached synaptic ribbon is opposed by the tips of multiple postsynaptic dendritic endings from horizontal and bipolar cells. These dendritic tips are located in a cavity formed by an invagination of the presynaptic photoreceptor terminal. The dendritic tips contain metabotropic glutamate receptors (in case of invaginating ON bipolar cells) or ionotropic glutamate receptors (in case of horizontal cells and OFF bipolar cells; Nomura et al., 1994; De Vries and Schwartz, 1999; De Vries, 2000; Wässle, 2004; De Vries et al., 2006; for review, see Dhingra and Vardi, 2012). Retinal bipolar cells, photoreceptor-like neurons in the pineal gland, inner ear hair cells, and vestibular hair cells also build ribbon synapses in mammals (Moser et al., 2006; Schmitz, 2009; Matthews and Fuchs, 2010; Mercer and Thoreson, 2011; Safieddine et al., 2012; Schmitz et al., 2012).
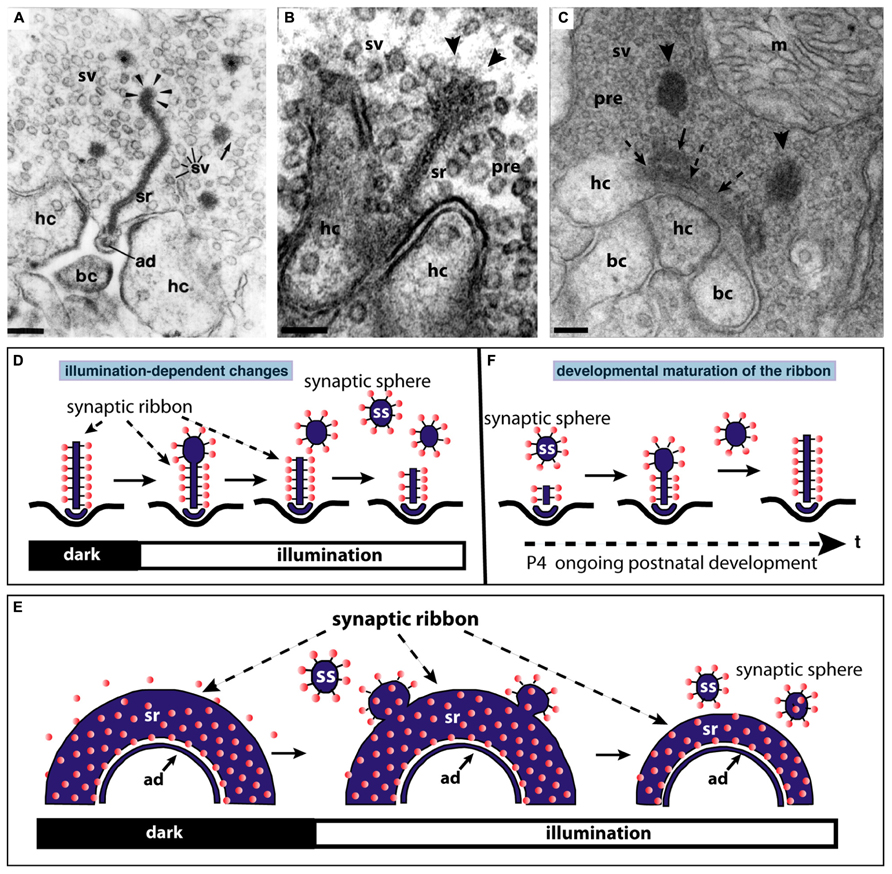
FIGURE 3. The synaptic ribbon is a dynamic structure, both in the mature retina (A,B,D,E) as well as in the immature retina during early postnatal retinal development (C,F). Panels (A,B) show disassemblying synaptic ribbons in the mature retina. The mature, bar-shaped synaptic ribbon disassembles via spherical structures, the synaptic spheres (arrowheads in A,B). Disassembly into synaptic spheres occurs at the cytosolic, non-plasma membrane-anchored end of the synaptic ribbon (arrowheads in A,B). At this end, the synaptic ribbon appears enlarged before the spherical synaptic spheres detach from it (A,B). The spherical synaptic ribbons are still associated with synaptic vesicles via thin connections. But they are no longer anchored to the presynaptic plasma membrane. Panel (A) is modified from Schmitz and Drenckhahn (1993) with permission from Springer-Verlag, Berlin, Heidelberg, Germany. (C) Assembly of the mature, bar-shaped synaptic ribbon during postnatal development. The arciform density (ad) is clearly visible at the active zone (dashed arrowheads in C). In the synapse shown in (C), the assembly of the synaptic ribbon just started. A small piece of the ribbon is already assembled (small black arrow in C) and anchored at the arciform density. Most of the ribbon is still present as immature synaptic spheres (arrowheads) located in vicinity to the small anchored ribbon primer (arrow in C). Later in development, the spherical synaptic ribbons (arrowheads) coalesce with the anchored ribbon primer (small black arrow in C) to form the mature, bar-shaped synaptic ribbon. RIBEYE–RIBEYE interactions might mediate this process. Panel (C) is taken from Schmitz et al. (2006) with permission from PNAS (copyright (2006) National Academy of Sciences, USA). Panels (D,E) schematically depict the illumination-induced disassembly of the synaptic ribbon (see also A,B). Panel (F) summarizes the possible sequence of synaptic ribbon assembly that occurs during postnatal development. sr, synaptic ribbon; ss, synaptic sphere; pre, presynaptic, sv, synaptic vesicle; bc, bipolar cell dendritic tip; hc, horizontal cell dendritic tip; m, mitochondrium; P4, postnatal day 4. Arrowheads in (A,B) point to synaptic spheres forming at the distal, non-membrane-anchored end of the synaptic ribbon. Dashed arrows in (C) point to the arciform density, which is the presynaptic density in ribbon synapses. The spheres colored in red in (D–F) represent synaptic vesicles. Panels (D–F) were re-drawn based on figures by Adly et al. (1999) and Spiwoks-Becker et al. (2004). Scale bars: 100 nm (A–C).
RIBEYE is a unique and major component of synaptic ribbons (Schmitz et al., 2000, 2012; Wan et al., 2005; Schmitz, 2009; Mercer and Thoreson, 2011). RIBEYE is the only known protein specific to synaptic ribbons (Schmitz et al., 2000; Magupalli et al., 2008; for review, see Schmitz, 2009; Mercer and Thoreson, 2011; Schmitz et al., 2012). RIBEYE consists of a unique A-domain that is specific to synaptic ribbons and a B-domain that is largely identical to CtBP2. Using different promoters, RIBEYE and CtBP2 are transcribed from the same gene (Schmitz, 2009). While CtBP2 is expressed in most if not all cells; the expression of RIBEYE expression is limited to cells that form ribbon synapses. Particularly the A-domain contains many interaction sites, at which RIBEYE can bind to neighboring RIBEYE molecules. These multiple RIBEYE–RIBEYE interactions could allow the assembly of multiple RIBEYE molecules into ribbon-like structures (Magupalli et al., 2008; for review, see Schmitz, 2009; Mercer and Thoreson, 2011; Schmitz et al., 2012). These primitive, spherical ribbon precursors could then assemble into mature, bar-/plate-shaped synaptic ribbons in mature photoreceptors (see below). RIBEYE appears to be the main building block of synaptic ribbons. Additional proteins are needed to generate mature, functional synaptic ribbons (see below).
Both RIBEYE(B)-domain and CtBP1/CtBP2 bind NAD(H) (Schmitz et al., 2000). In case of CtBP1/CtBP2, binding of NADH regulates interaction with nuclear proteins (Fjeld et al., 2003). This NAD(H)-regulated interaction can switch on or off distinct target genes relevant, e.g., for cellular migration, adhesion, and apoptosis (Paliwal et al., 2006, 2007, 2012; Chinnadurai, 2009). In the synapse, NAD(H) promotes interaction of RIBEYE with other synaptic proteins, e.g., the NCS protein GCAP2 (Venkatesan et al., 2010; López-del Hoyo et al., 2012). RIBEYE–GCAP2 interaction appears to be relevant for mediating Ca2+-dependent effects on synaptic ribbon dynamics (see below). Other protein–protein interactions of RIBEYE do not depend on NADH, e.g., RIBEYE–Munc119 interaction (Alpadi et al., 2008) or have not been analyzed for their NADH-dependence, e.g., in case of RIBEYE-Bassoon (tom Dieck et al., 2005). While RIBEYE appears to be the only unique and major protein of the synaptic ribbon, there are other ribbon proteins which are also present in the active zone of non-ribbon containing synapses (for review, see tom Dieck and Brandstätter, 2006; Schmitz, 2009; Mercer and Thoreson, 2011; Schmitz et al., 2012). Bassoon is localized in the active zone of photoreceptors, bipolar cells, and hair cells (in addition to the active zone localization of conventional synapses) and is considered as an important anchor for the synaptic ribbon in ribbon synapses. Bassoon knockout mice display non-plasma membrane-anchored “floating” ribbons in photoreceptor and hair cells (Khimich et al., 2005; tom Dieck et al., 2005), though not in retinal bipolar cells. Bassoon is also present in retinal bipolar cells (Deguchi-Tawarada et al., 2006). Therefore, additional anchoring mechanisms for the synaptic ribbon might exist. Bassoon was also been reported to be linked to presynaptic Cav-channels recently (Nishimune et al., 2004, 2012; see also below). Piccolo, like bassoon a very large multidomain-protein, is present both in conventional and ribbon-type synapses. Recently, a splice variant of piccolo was described, named piccolino, that is preferentially expressed at ribbon synapses (Regus-Leidig et al., 2013). Proteins known to be essential for synapse function in conventional synapses are the multi-domain rab3-interacting molecule (RIM) protein family (Wang et al., 1997; Kaeser et al., 2011, 2012; for review, see Südhof, 2013). These proteins have been localized to the synaptic ribbon. The long isoforms of RIM contain a N-terminal Zn-finger, central PDZ-domain and two C2-domains (Südhof, 2012a,b, 2013) that enable them to perform key synaptic functions. RIM proteins control synaptic vesicle docking and vesicle priming as well as Ca2+-channel localization (for review, see Südhof, 2012b, 2013). Their function in the ribbon synapse is less clear. RIM2 appears to be localized at the base of the synaptic ribbon and RIM1 at the body of the synaptic ribbon (tom Dieck et al., 2005). RIM proteins interact with Munc13 proteins in conventional synapses to mediate vesicle priming (Deng et al., 2011). While in conventional synapses, RIMs interact with Munc13-1; Munc13-1 is missing in photoreceptor synapses (Schmitz et al., 2001; Cooper et al., 2012). Instead of Munc13-1, ubMunc13-2 is expressed in photoreceptor synapses (Cooper et al., 2012). Other active zone proteins [e.g., CAST/ELKS; RIM-binding proteins (RIM-BPs)] are also present at photoreceptor ribbon synapses partly differentially distributed in distinct sub-compartments of the synapse (for review, see tom Dieck and Brandstätter, 2006; Mercer and Thoreson, 2011; Schmitz et al., 2012).
Synaptic Ribbons: Functional Considerations
The synaptic ribbon is the most prominent structural feature of photoreceptor ribbon synapses. The function of the synaptic ribbon is still unclear. The synaptic vesicles attached to the synaptic ribbon have been functionally correlated to different synaptic vesicle pools (for review, see Heidelberger et al., 2005; Matthews and Fuchs, 2010; Mercer and Thoreson, 2011). Total internal reflection fluorescence (TIRF) microscopy studies of individual synaptic vesicles demonstrated that the base of the ribbon is a hotspot of synaptic vesicle exocytosis (Zenisek et al., 2000; Chen et al., 2013). The basal row of synaptic vesicles attached to the synaptic ribbon represents the vesicle pool that can be immediately released (the “RRP,” readily releasable pool). Therefore, one role of the ribbon might be to position this basal row of synaptic vesicles in a “strike-distance” for SNARE proteins to rapidly execute Ca2+-triggered exocytosis (Zenisek et al., 2000). The synaptic ribbon seems to be needed for both fast, synchronous release as well as for slow, asynchronous release components (Khimich et al., 2005; Zenisek, 2008; Frank et al., 2010 Snellman et al., 2011).
Exocytosis was frequently observed at ribbon-containing sites if exocytosis was induced by mild stimuli, e.g., weak depolarizations (Chen et al., 2013). If longer depolarizations were applied, exocytosis also occurred at non-ribbon-containing sites, i.e., in some distance from the presynaptic Ca2+-channels (Midorikawa et al., 2007; Zenisek, 2008; Chen et al., 2013). Exocytosis of synaptic vesicles at these non-ribbon-containing sites could contribute to the asynchronous release component (Midorikawa et al., 2007; Zenisek, 2008).
The large dimensions of the synaptic ribbon allow many vesicles to be docked at the base of the synaptic ribbon (Matthews and Fuchs, 2010; Mercer and Thoreson, 2011; Schmitz et al., 2012). This is quite likely necessary to support coordinated multivesicular vesicle fusion at the synaptic ribbon (Glowatzki and Fuchs, 2002; Singer et al., 2004; Khimich et al., 2005; Graydon et al., 2011). The synaptic vesicles attached to the synaptic ribbon in a more distant position (also denoted as “tethered vesicles”; Heidelberger et al., 2005; Matthews and Fuchs, 2010; Mercer and Thoreson, 2011) could contribute to the synaptic vesicle pool that is released with a slower kinetics, the slowly releasable pool (“SRP”). The tethered synaptic vesicles could possibly move down toward the base of the synaptic ribbon before they finally fuse at the active zone (“conveyor belt” hypothesis of the synaptic ribbon; Lenzi and von Gersdorff, 2001; Parsons and Sterling, 2003).
Recently, it was shown that the synaptic ribbon has also an important role in the replenishment of synaptic vesicles (Jackman et al., 2009; Snellman et al., 2011; Chen et al., 2013; see also below). Regulation of synaptic vesicle replenishment could be a major function of synaptic ribbons (Jackman et al., 2009; Chen et al., 2013). It was shown that tonic release at the photoreceptor ribbon synapse leads to vesicle depletion at the base of the ribbon. Based on that suggestion, the ribbon would work as a capacitor for synaptic vesicles that reloads the empty release sites at the base of the synaptic ribbon (Jackman et al., 2009; Snellman et al., 2011; Chen et al., 2013). The ribbon capacitor will be re-charged with synaptic vesicles in the light phase when vesicle exocytosis is low. This recharged ribbon capacitor will provide release-ready vesicles in the dark when the photoreceptor depolarizes (Jackman et al., 2009; Chen et al., 2013). According to this hypothesis, the rate of release is determined by the rate of vesicle replenishment at the synaptic ribbon. In agreement with this assumption, it was recently shown that major endocytic proteins were strongly enriched in the periactive zone around the synaptic ribbon (Wahl et al., 2013).
Synaptic Coding at Ribbon Synapses in Comparison to Coding in Conventional Synapses
Synaptic coding in ribbon synapses differs from coding in conventional synapses. Conventional synapses are typically phasic synapses that initiate synaptic vesicle exocytosis only when a stimulus, usually an action potential, reaches the presynaptic terminal. The depolarization of the presynaptic terminal opens VGCC, typically P-/Q-/N-type calcium channels in phasic synapses (for review, see Catterall et al., 2005; Striessnig et al., 2010; Catterall, 2011; Südhof, 2012a,b, 2013). The subsequent Ca2+-influx initiates synaptic vesicle fusion at the active zone (for review, see Südhof, 2012a,b, 2013). In contrast, ribbon synapses are tonically active synapses that continuously fuse synaptic vesicles with the presynaptic plasma membrane. The basal rate of synaptic vesicle exocytosis is modulated in response to graded changes of the membrane potential. In photoreceptors, exocytosis is high in the dark when the photoreceptor is depolarized. The rate of exocytosis is diminished in the light when the photoreceptor hyperpolarizes (Jackman et al., 2009). Synaptic vesicle exocytosis at ribbon synapses is also initiated by influx of Ca2+ through VGCC. But ribbon synapses typically use other Ca2+-channels than phasic synapses (see below). As mentioned above, exocytosis of synaptic vesicles preferentially occurs at the base of the synaptic ribbon (Zenisek et al., 2000; Chen et al., 2013). Ribbon synapses can fuse several hundreds of synaptic vesicles per second and ribbon (Heidelberger et al., 2005; Schmitz, 2009). Rod photoreceptors maintain continuous exocytosis at physiological membrane potentials indicating a high vesicle release probability in ribbon synapses (Jackman et al., 2009; Chen et al., 2013). How this is achieved is not clear but could be based on a high Ca2+-sensitivity of the release machinery and/or an efficient coupling of the release machinery to the presynaptic L-type Ca2+-channels (see below). In contrast, release probability in conventional, phasic synapses is typically much lower than in ribbon synapses (for review, see Nicoll and Schmitz, 2005; Rusakov, 2006; Fioravante and Regehr, 2011; Regehr, 2012).
At the single vesicle level, using TIRF microscopy it was shown that synaptic vesicles did not fuse immediately after they appeared at the plasma membrane but paused at the base of the ribbon for about 60 ms before fusion finally occurred (Zenisek et al., 2000; Chen et al., 2013). Sixty-millisecond is a relatively long time delay and indicates that the speed of single vesicle exocytosis cannot be the main determinant for synaptic coding and its temporal fidelity at ribbon synapses.
The retrieval of synaptic vesicles has been recently appreciated as an important aspect of signaling at ribbon synapses (see also above). Continuous exocytosis in photoreceptor ribbon synapses caused depletion of synaptic vesicles at the base of the synaptic ribbon at physiological membrane potentials (Jackman et al., 2009; Chen et al., 2013). This depletion of presynaptic release sites during tonic exocytosis at physiological membrane potentials suggested that synaptic vesicle replenishment is rate-limiting for exocytosis and an important aspect for signaling at ribbon synapses (Jackman et al., 2009; Chen et al., 2013). The rate of vesicle replenishment would thus determine how smooth exocytosis could proceed. Gaps in continuous synaptic vesicle exocytosis at the ribbon synapse, e.g., as a result of insufficient vesicle replenishment, could contribute important signaling informations.
Despite the large physiological differences between ribbon synapses and conventional synapses, these synapses do not differ strongly in the composition of the exocytotic machinery (for review, see Schmitz, 2009; Matthews and Fuchs, 2010; Mercer and Thoreson, 2011; Schmitz et al., 2012). In photoreceptor ribbon synapses, syntaxin 1 is replaced by syntaxin 3b (Curtis et al., 2008, 2010); complexin 1/2 by complexin 3/4 (Reim et al., 2005, 2009); Munc13-1 by ubMunc13-2 (Schmitz et al., 2001; Cooper et al., 2012); piccolo by piccolino (Regus-Leidig et al., 2013). But the key players of exocytosis are remarkably conserved.
It should be mentioned that ribbon synapses are not a uniform population of synapses but display both structural and functional differences (Heidelberger et al., 2005; Moser et al., 2006; Matthews and Fuchs, 2010; Mercer and Thoreson, 2011). Exocytosis in ribbon synapses of hair cells in the inner ear operates in a fundamentally different manner and appears to function without neuronal SNARE proteins (Nouvian et al., 2011).
Development of the Synaptic Ribbon Complex
Photoreceptor ribbon synapses of the mouse retina develop postnatally (Olney, 1968; Blanks et al., 1974). The assembly of the synaptic ribbon complex in photoreceptors occurs via distinct steps. Starting around postnatal day 4 (P4) small, spherical ribbon precursors are anchored at the arciform density of the photoreceptor active zone as judged by electron microscopy and immunofluorescence microscopy with antibodies against RIBEYE (Olney, 1968; Hermes et al., 1992; Regus-Leidig et al., 2010a,b; Liu et al., 2013; Zabouri and Haverkamp, 2013). Starting around P10 and proceeding with the time of eye opening, the immature, short spherical ribbons develop into the mature form of the synaptic ribbon which is bar-shaped in cross sections and plate-shaped in three-dimensional representations (Figures 3C,F; Hermes et al., 1992; Vollrath and Spiwoks-Becker, 1996; Adly et al., 1999; Schmitz et al., 2006; Regus-Leidig et al., 2010a,b; Liu et al., 2013).
Recent studies demonstrated that VGCC have a key role for the developmental organization of the presynaptic cytoskeleton including the synaptic ribbons.
Synaptic Ribbons and Voltage-Gated Ca2+ Channels
L-type VGCC are clustered at the basal end of the synaptic ribbon (Heidelberger et al., 2005; Doering et al., 2007; Striessnig et al., 2010; Mercer and Thoreson, 2011; Mercer et al., 2011a; Schmitz et al., 2012). The site is a hotspot of exocytosis (Zenisek et al., 2000). Entry of Ca2+ through L-type VGCC is essential for triggering exocytosis at the photoreceptor synapse (Dacheux and Miller, 1976; Schmitz and Witkovsky, 1997; Witkovsky et al., 1997; Heidelberger et al., 2005). The VGCC at the active zone of photoreceptors consists of a pore-forming transmembrane α1 subunit (Cav1.4, in photoreceptors; Cav1.3 in inner hair cells), an auxiliary β2-subunit and the α2δ4-subunit (Ball et al., 2002, 2011; Catterall et al., 2005; Morgans et al., 2005; Wycisk et al., 2006; Buraei and Yang, 2010; Striessnig et al., 2010; Catterall, 2011; Mercer et al., 2011a; Schmitz et al., 2012; Liu et al., 2013). Few Cav-channels at the base of the synaptic ribbons are sufficient to drive exocytosis (Brandt et al., 2005; Bartoletti et al., 2011). L-type VGCC in photoreceptors are well suited to support tonical exocytosis at ribbon synapses because these channels do not show Ca2+-dependent inactivation (CDI) and little voltage-dependent inactivation (VDI; Singh et al., 2006; Striessnig et al., 2010; Catterall, 2011; Shaltiel et al., 2012). Furthermore, the Ca2+-sensitivity of release is high and exocytosis is triggered by low, submicromolar Ca2+-concentrations (Rieke and Schwartz, 1996; Thoreson et al., 2004; Heidelberger et al., 2005; Sheng et al., 2007; Choi et al., 2008; Jarsky et al., 2010). In addition to L-type channels, retinal bipolar cells also express transient, T-type VGCC (Pan et al., 2001; Ma and Pan, 2003; Hu et al., 2009).
In the development of the outer retina, Cav-channels associate early with synaptic ribbon precursors and synaptic ribbons (Liu et al., 2013; Zabouri and Haverkamp, 2013). Synaptic ribbons do not form properly, if the L-type calcium channels are missing, e.g., in Cav1.4 mouse knockout models (Mansergh et al., 2005; Chang et al., 2006; Raven et al., 2007; Lodha et al., 2010). In the different available Cav1.4 mouse knockout models, the Cav1.4-channel was shown to exert a strong, severe influence on synaptic ribbon localization and assembly. Some quantitative differences were observed in the different available Cav1.4 mouse knockout models (Mansergh et al., 2005; Chang et al., 2006; Raven et al., 2007; Lodha et al., 2010).
Two recent studies (Liu et al., 2013; Zabouri and Haverkamp, 2013) analyzed in detail the relevance of the Cav-channels for distinct steps of synaptic ribbon assembly at the active zone and for the subsequent maturation of the synaptic ribbon complex (Liu et al., 2013; Zabouri and Haverkamp, 2013). Initially, at early stages of development, immature ribbon precursors are clustered in the terminal and probably anchored to the active zone (Zabouri and Haverkamp, 2013) even if the Cav-channels are missing. But – in contrast to the wildtype – these immature ribbon precursors are not aligned and thus functionally linked with postsynaptic glutamate receptors in the Cav1.4 knockout (Liu et al., 2013). Furthermore, without proper channel activity, these immature ribbon precursors do not mature into the bar-shaped synaptic ribbons but remain immature (Liu et al., 2013; Zabouri and Haverkamp, 2013). Interestingly, normal physiological activity of the Cav-channel is needed to transform immature ribbons into mature ribbons. Both loss-of-function mutants as well as gain-of-function mutants (Knoflach et al., 2013; Liu et al., 2013) of the Cav-channels prevented proper maturation of the synaptic ribbon complex (Liu et al., 2013).
Similarly, also knockout models of various proteins linked to the Cav1.4-channel (i.e., β2-subunit of Cav1.4 channels, CaBP4, bassoon, and laminin β2 (for review, see Nishimune, 2012) displayed absent, smaller or non-attached “floating” synaptic ribbons (Libby et al., 1999; Ball et al., 2002; Dick et al., 2003; Haeseleer et al., 2004; Nishimune et al., 2004; Nishimune, 2012). In agreement with these findings, voltage-gated Cav-channels in the inner ear were shown to regulate synaptic ribbon size (Frank et al., 2009, 2010; Sheets et al., 2011, 2012).
Zabouri and Haverkamp (2013) demonstrated that Cav-channels are not only important for the proper development of the synaptic ribbon complex but also for the development of the presynaptic cytoskeleton in photoreceptors. Deletion of Cav1.4 lead to misassembly of many components of the presynaptic photoreceptor cytoskeleton including PSD95, the plasma membrane Ca2+-ATPase (PMCA), Veli3, β-dystroglycan, bassoon, and RIM2. As consequence, the architecture of the synaptic terminal was profoundly altered.
These data argue that the Cav1.4 channel in photoreceptors is a central organizer of the photoreceptor ribbon synapse active zone in general and for the synaptic ribbon complex in particular. Several molecules are candidates that could provide a link between synaptic ribbons and voltage-gated Cav1.4 calcium channels. The RIM multi-domain family of proteins are essential components of the active zones in conventional and ribbon synapses (Wang et al., 1997; Südhof, 2013). In conventional active zones, they are found at presynaptic densities; in ribbon synapses both at the active zone (arciform densities; RIM2; tom Dieck et al., 2005) and also at the proper synaptic ribbon (RIM1, tom Dieck et al., 2005). RIMs are important for various central aspects of synapse function (Deng et al., 2011; Han et al., 2011; Kaeser et al., 2011; for review, see Südhof, 2013). RIM proteins are crucial for anchoring P/Q/N-type VGCC at the active zone in conventional synapses via a direct PDZ-domain interaction (Deng et al., 2011; Han et al., 2011; Kaeser et al., 2011; for review, see Südhof, 2013). RIMs indirectly interact with L-type Ca2+-channels via RIM-BPs (Hibino et al., 2002). Besides immobilizing VGCC, RIMs are essential for vesicle priming via interaction with Munc13 and for synaptic vesicle docking via interaction with rab3/rab27 (Deng et al., 2011; Han et al., 2011; Kaeser et al., 2011; for review, see Südhof, 2013).
RIM1 has also been reported to be associated with the β-subunits (β4, β2a) of VGCC (Kiyonaka et al., 2007; Miki et al., 2007). This interaction was suggested to suppress inactivation of VGCC (Kiyonaka et al., 2007; Miki et al., 2007). Interestingly, a point mutation in the C2A-domain of RIM1 is responsible for a cone rod-dystrophy (CORD7; Johnson et al., 2003). CAST/ELKS proteins that are also present at the synaptic ribbon complex have been recently reported to be also associated with β-subunits of VGCC (Chen et al., 2011; Kiyonaka et al., 2012). Similarly, bassoon has been described to be associated with β-subunits of VGCC (Chen et al., 2011; Nishimune et al., 2012). The binding of bassoon to β-subunits of VGCC is compatible with the finding that the organization of VGCC was disturbed in inner ear ribbon synapses of bassoon-deficient mice (Frank et al., 2010). Thus, the active zone with the synaptic ribbons appears to be intimately associated with VGCC and organized by these channels by a multitude of different protein–protein interactions.
Presynaptic Ca2+, GCAP2, and Synaptic Ribbon Dynamics
In the mature adult mouse retina, synaptic ribbons also underlie changes in size. These changes are illumination-dependent and appear to be regulated by Ca2+ (Vollrath and Spiwoks-Becker, 1996; Adly et al., 1999; Spiwoks-Becker et al., 2004; Schmitz, 2009; Regus-Leidig et al., 2010a). Several studies observed a light-induced reduction in the size of synaptic ribbons in the mouse retina (Adly et al., 1999; Spiwoks-Becker et al., 2004; Regus-Leidig et al., 2010a; Fuchs et al., 2013). The bar-shaped ribbons appear to disassemble (and re-assemble) from the free cytosolic, non-membrane-anchored end (Figure 3). From this end of the bar-shaped ribbon, spherical ribbons, the synaptic spheres, detach (Figure 3). Thus, this illumination-dependent growth and disassembly of the synaptic ribbon appears to employ similar intermediate structures as in development (Figure 3C). These spherical intermediates are similar in appearance as the spherical synaptic ribbons of hair cells in the inner ear (for review, see Moser et al., 2006). The synaptic spheres are still associated with numerous synaptic vesicles (Figures 3A–C). Spherical ribbons were added to remaining ribbons during darkness (Spiwoks-Becker et al., 2004; Regus-Leidig et al., 2010a).
The light-induced reduction of synaptic ribbon size could be mimicked by lowering intracellular Ca2+ (Adly et al., 1999; Spiwoks-Becker et al., 2004) suggesting that Ca2+ is an important factor that regulates ribbon size. In fact, while lowering intracellular Ca2+ lead to a reduction of synaptic ribbon size, increases of intracellular Ca2+ resulted in increased ribbon sizes (Spiwoks-Becker et al., 2004; Regus-Leidig et al., 2010a). The genetic background of animals also influences synaptic ribbon dynamics. Synaptic ribbon size plasticity has been reported to be stronger in albinotic mice than in pigmented mice (Fuchs et al., 2013).
Mechanisms of assembly and disassembly of synaptic ribbons in the mature retina are still largely unknown. The NCS protein GCAP2 is involved in the Ca2+-dependent disassembly of synaptic ribbons (Venkatesan et al., 2010; López-del Hoyo et al., 2012). RIBEYE, the main component of synaptic ribbons, binds the NCS protein GCAP2 (Venkatesan et al., 2010). GCAP2 is the only known Ca2+-binding protein of synaptic ribbons. Interaction between RIBEYE and GCAP2 occurs via the B-domain of RIBEYE and the C-terminal region (CTR) of GCAP2 (Venkatesan et al., 2010; for review, see Schmitz et al., 2012). The CTR of GCAP2 is divergent in sequence between GCAP1 and GCAP2. In agreement, GCAP1 does not bind to RIBEYE while GCAP2 does (Venkatesan et al., 2010). Recent ultrastructural analyses, using postembedding immunogold electron microscopy demonstrated that GCAP2 is a component of synaptic ribbons that is directly localized at the synaptic ribbon body (López-del Hoyo et al., 2012). Viral overexpression of GCAP2 in organotypical retina cultures leads to a disassembly of synaptic ribbons (Venkatesan et al., 2010). Similarly, in GCAP2 – overexpressing transgenic mice, synaptic ribbons also tend to disassemble more easily than in the wildtype retinas (López-del Hoyo et al., 2012) although the synaptic ribbon complex as such is normally formed in GCAP2 knockout mice.
Low intracellular Ca2+-concentrations lead to dimerization and also to conformational changes in the CTR of GCAP2 (Olshevskaya et al., 1999; Peshenko et al., 2004). In particular, low intracellular Ca2+ leads to exposure of the CTR of GCAP2 (Peshenko et al., 2004). In the Ca2+-free form of GCAP2, the CTR is exposed while in the Ca2+-bound state it is not (or much less; Peshenko et al., 2004). Therefore, RIBEYE–GCAP2 interaction is most likely favored by conditions of low intracellular Ca2+, e.g., at illumination. Furthermore, while RIBEYE–GCAP2 interaction is promoted by NAD(H) (Venkatesan et al., 2010), NADH destabilizes the RIBEYE–RIBEYE interaction network (i.e., RE(A)-RE(B) interaction ; Magupalli et al., 2008) which would lead to an overall tendency of reduced RIBEYE–RIBEYE interaction. By this mechanism, depletion of Ca2+ from GCAP2 could contribute to the disassembly of synaptic ribbons in photoreceptor synapses. By this way of thinking, GCAP2 binding to the synaptic ribbon could thus be involved in the regulation of the Ca2+-dependent dynamics of synaptic ribbons.
The conformational change of GCAP2 induced by low Ca2+ could thus lead to a severing and subsequent disassembly of the ribbon body into spherical structures, the synaptic spheres (Figure 4). The immunogold electron microscopic studies by López-del Hoyo et al. (2012) showed that GCAP2 is not homogeneously distributed along the entire ribbon body (as is RIBEYE; Schmitz et al., 2000), but is clustered at hotspots at the synaptic ribbon surface. These hot spots of GCAP2 immunoreactivity on the synaptic ribbon could represent sites of ribbon disassembly (Figure 4).
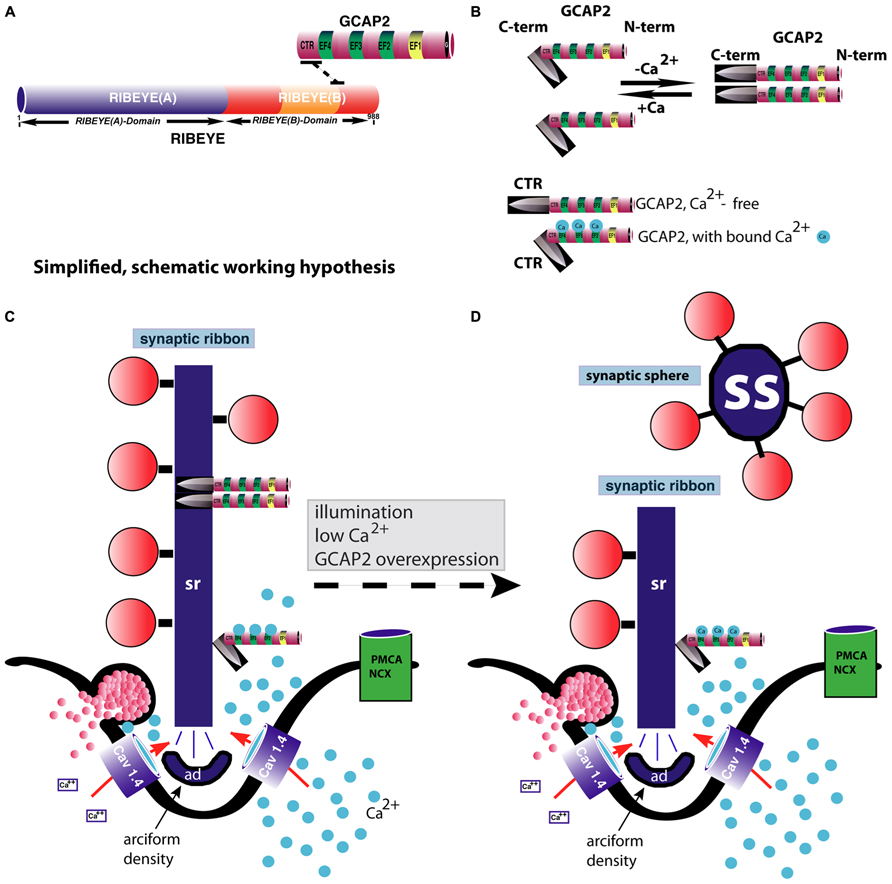
FIGURE 4. GCAP2-mediated disassembly of the synaptic ribbon. (A) Previous studies have shown that GCAP2 interacts with RIBEYE, the main component of synaptic ribbons (Venkatesan et al., 2010). The drawing in (A) schematically summarizes the interaction between GCAP2 and RIBEYE. The C-terminal region (CTR) of GCAP2 interacts with the hinge region of RIBEYE(B)-domain that connects the NAD(H)-binding sub-domain with the substrate-binding sub-domain of RIBEYE (Venkatesan et al., 2010; Schmitz et al., 2012). This interaction is promoted by NAD(H). (B) GCAP2 performs a Ca2+-dependent conformational change of its C-terminal region (CTR). In the Ca2+-free form, the CTR is exposed, whereas in its Ca2+-bound form it is less exposed (Peshenko et al., 2004). In addition, GCAP2 dimerizes at low Ca2+ (Olshevskaya et al., 1999). The CTR is responsible for the binding to RIBEYE (Venkatesan et al., 2010). (C,D) Binding of GCAP2 to RIBEYE of synaptic ribbons might lead to severing of the synaptic ribbon via exposure of the CTR. Exposure of the CTR occurs if Ca2+ is low, e.g., at illumination or if intracellular Ca2+ is buffered away by chelators. As a consequence, ribbons are getting shorter. Spherical ribbons, synaptic spheres, dissociate from the free cytosolic end of the synaptic ribbon. While NAD(H) favors binding of GCAP2 to RIBEYE, it weakens RIBEYE–RIBEYE interactions which might ease disassembly of the synaptic ribbon (Magupalli et al., 2008). For sake of clarity, intracellular membrane system, e.g., ER, and mitochondria which could influence intracellular Ca2+-levels were omitted in the schematic drawing. These compartments can be expected to further shape the Ca2+-signal in the presynaptic terminal. PMCA and NCX-proteins are schematically depicted. The C-terminus (CTR) of GCAP2 that interacts with RIBEYE and which is exposed particularly in the Ca2+-free form of GCAP2 is schematically depicted as a knife-like structure because it is proposed to severe the synaptic ribbon structure. The spheres colored in blue in (B–D) represent Ca2+-ions. sr, synaptic ribbon; ss, synaptic sphere; CTR, C-terminal region of GCAP2; PMCA, plasma membrane Ca2+-ATPases; NCX, Na+/Ca2+-exchanger; ad, arciform density (i.e., the presynaptic density of photoreceptor ribbon synapses); EF, EF-hand.
Intracellular Modulation of Ca2+-Signals in Photoreceptor Terminals
Ca2+ signals generated by influx through VGCC are modulated by various intracellular mechanisms that can subsequently increase or decrease cytosolic Ca2+-concentration. The final Ca2+-signal in the presynaptic terminal is shaped by the temporal and spatial interactions of these mechanisms (Krizaj and Copenhagen, 2002; Szikra and Krizaj, 2006).
The smooth endoplasmic reticulum (ER) is a main intracellular Ca2+-source (Parekh, 2011) and located close to the synaptic ribbon in photoreceptor ribbon synapses as judged by immunolabeling with antibodies against ER components, e.g., the sarco(endo)plasmic reticulum Ca2+-ATPase (SERCA)-2 protein (Johnson et al., 2007; Babai et al., 2010). Ca2+-induced Ca2+-release (CICR) from the ER can amplify Ca2+-signals and enhance signal transmission (Krizaj et al., 1999; Krizaj, 2003; Cadetti et al., 2006; Suryanarayanan and Slaughter, 2006; Szikra and Krizaj, 2007; Szikra et al., 2008, 2009; Babai et al., 2010). Several studies showed that CICR contributes to tonic release at the photoreceptor synapse (Krizaj et al., 1999; Cadetti et al., 2006; Suryanarayanan and Slaughter, 2006; Babai et al., 2010). Both classes of ER-localized receptors responsible for mobilization of Ca2+ from the ER, i.e., ryanodine receptors (RyR) and IP3 receptors (IP3R) have been localized to photoreceptor terminals (Peng et al., 1991; Krizaj et al., 1999; Krizaj, 2003). CICR can also activate Ca2+-activated Cl--channels at the presynaptic plasma membrane. TMEM16B-type of Ca2+-activated Cl--channels have been localized to the photoreceptor terminal (Lalonde et al., 2009; Stöhr et al., 2009; Mercer et al., 2011b). Activation of Ca2+-activated Cl--channels in photoreceptor terminals should lead to depolarization because the membrane potential of about ≈–40 mV at darkness is more negative than the Cl--equilibrium potential of -20 mV in rod photoreceptor synapses (Thoreson et al., 2002; Babai et al., 2010).
If intracellular Ca2+-stores are exhausted, store-operated calcium entry (SOCE) mechanisms can replenish them (for review, see Parekh and Putney, 2005; Lewis, 2011; Soboloff et al., 2012; Gudlur et al., 2013). The ER-localized EF-hand-containing Ca2+-sensor protein STIM activates Ca2+-selective, plasma membrane located Ca2+-release-activated Ca2+ (CRAC)-channels in case of store-depletion. CRAC channels contain Orai-proteins as major components that allow Ca2+ influx through the plasma membrane and subsequent Ca2+ replenishment of the emptied ER store (Lewis, 2011; Soboloff et al., 2012; Gudlur et al., 2013). TRPC1 proteins also contribute to CRAC channel activity (Szikra et al., 2009; Molnar et al., 2012). Silencing of TRPC1 by RNA interference abolished SOCE in rods but not in cones indicating that TRPC1 could be an important component of SOCE in rods (Szikra et al., 2008). STIM1 proteins have been recently localized to the photoreceptor terminal (Szikra et al., 2009). The SERCA2 ATPase (see below) has been suggested to be the “third element” of SOCE that mediates influx of Ca2+ into the ER after it has entered the cytosol via the CRAC channels (Manjarrés et al., 2010, 2011; Alonso et al., 2012). Store-operated Ca2+-channels could contribute to sustained Ca2+ signals and extend the dynamic range of signaling in the tonically active photoreceptor ribbon synapse.
The PMCAs represent a family of transmembrane proteins (Carafoli, 1999; Tempel and Shilling, 2007) that extrudes Ca2+ from the cytosol into the extracellular space in an ATP-dependent manner (Carafoli, 1999; Tempel and Shilling, 2007). The PMCA is immobilized in the plasma membrane of the presynaptic terminal that is scaffolded by PSD95, MPP4, and VELI3 (Aartsen et al., 2006, 2009; Yang et al., 2007; Förster et al., 2009). Proper localization of PMCA in the presynaptic plasma membrane also depends on VGCC (Xing et al., 2012). The PMCA has a strong influence on presynaptic Ca2+-signaling (Duncan et al., 2006; Szikra and Krizaj, 2006) and also on synaptic ribbon length dynamics (Yang et al., 2007; Aartsen et al., 2009). Deletion of the MPP4 gene leads to a loss of PMCA from the plasma membrane of the presynaptic photoreceptor terminals (Yang et al., 2007; Aartsen et al., 2009). The subsequent loss of extrusion activity leads to increased intracellular Ca2+ and enlarged synaptic ribbons (Yang et al., 2007; Aartsen et al., 2009). Na+/Ca2+ exchangers (NCXs) extrude Ca2+ from the cytosol into the extracellular space under physiological conditions (Lytton, 2007). NCX proteins are closely localized to the synaptic ribbon and thus can be expected to strongly influence synaptic transmission and ribbon dynamics (Duncan et al., 2006; Johnson et al., 2007). PMCA appears to be predominantly expressed in rod spherules; NCX is predominantly expressed in cone pedicles (Johnson et al., 2007). The SERCA can lower intracellular Ca2+ by pumping Ca2+ from the cytosol into the ER (Bublitz et al., 2013). In photoreceptor synapses, the SERCA pump has been localized in close proximity to the synaptic ribbon (Yang et al., 2007; Babai et al., 2010). The described Ca2+-extruding systems are important to restore resting levels of Ca2+ after depolarization-induced increases (Duncan et al., 2006; Szikra and Krizaj, 2006; Johnson et al., 2007; Mercer and Thoreson, 2011; Wan et al., 2012; Bublitz et al., 2013). Photoreceptor terminals often contain also mitochondria in proximity to the synaptic ribbon (Johnson et al., 2007). Mitochondria have been suggested to be able to take up large amounts of Ca2+ (Rizzuto et al., 2004; Parekh and Putney, 2005; Rizzuto and Pozzan, 2006). In ribbon terminals, Ca2+-uptake by mitochondria was found to be only functionally relevant if the Ca2+-load was particularly high (Zenisek and Matthews, 2000).
The Synaptic Ribbon Size in Photoreceptor Synapses: A Proxy for Presynaptic Ca2+-Concentration?
As summarized above, there are multiple links between Cav-channels, presynaptic Ca2+-levels, synaptic signaling and the synaptic ribbons. Ca2+-flux through VGCC as well as the subsequent cross-talk to other Ca2+-regulating systems in the presynaptic terminal affect structural plasticity and developmental maturation of the synaptic ribbon in photoreceptor synapses. In the light, when Ca2+ is low in presynaptic photoreceptor terminals (Thoreson et al., 2004; Choi et al., 2005, 2008; Sheng et al., 2007; Jackman et al., 2009), synaptic ribbons appear to be smaller than in the dark (Adly et al., 1999; Balkema et al., 2001; Spiwoks-Becker et al., 2004; Hull et al., 2006; Regus-Leidig et al., 2010a). Similarly, in knockout mouse models in which extrusion of Ca2+ is disturbed and presynaptic Ca2+ increased, synaptic ribbons were longer than in control animals (Yang et al., 2007; Aartsen et al., 2009). On the other hand, in mouse knockout models where Cav-channels were deleted, synaptic ribbons were smaller and did not develop into mature, large synaptic ribbons. Therefore, the size of the synaptic ribbon could appear as a readout of presynaptic Ca2+. A recent publication (Liu et al., 2013) indicated, that the Ca2+-mediated dynamics of synaptic ribbons – at least in development – is more complex. Also gain-of-function mutations of the Cav1.4 channel, which lead to an activation of the Cav1.4 channel already at more negative membrane potentials, displayed shorter than normal synaptic ribbons (Liu et al., 2013). Future investigation need to provide a more detailed picture about the assembly and disassembly of the synaptic ribbon, its regulation and its consequences for signaling at the photoreceptor synapse.
Summary and Outlook
Biochemical and molecular analyses identified the protein composition of synaptic ribbons to a large extent. Surprisingly, most of the ribbon proteins are not only present in ribbon synapses but also in conventional synapses. RIBEYE is the only major building block unique to synaptic ribbons. Still, many questions concerning the structure, assembly, and function of synaptic ribbons remain to be elucidated. How is the assembly of synaptic ribbons regulated? How do immature ribbons develop into mature ribbons? How does Ca2+ exerts its influence on synaptic ribbons? Does Ca2+-dependent phosphorylation of ribbon proteins play a role? Why are there so many links between presynaptic Ca2+-channels and synaptic ribbons? How can very few ribbon synapse-specific components build such a fundamentally different synapse? How are the synaptic ribbon components mechanistically linked to the proposed functions of synaptic ribbons? The application of innovative physiological tools and the analysis of mouse knockout models are needed to answer these questions.
Conflict of Interest Statement
The author declares that the research was conducted in the absence of any commercial or financial relationships that could be construed as a potential conflict of interest.
Acknowledgments
Work of the author was supported by research grants from the German Research Community (DFG) SFB894, GRK1326 and by the Human Frontiers Science Organization HFSP. Thanks to Dr. Jutta Schmitz-Kraemer and Prof. Dr. Doris M. Kraemer (University of Oldenburg) for critically reading the manuscript. The author apologizes that not all relevant original papers could be cited due to limitations in space.
Abbreviations
CNG, cyclic nucleotide-gated channel; CRAC, Ca2+-release-activated Ca2+-channels; GCAP2, guanylate cyclase-activating protein 2; IS, inner segments; NAD(H), designates both the oxidized (NAD+) as well as the reduced form (NADH) of nicotinamide adenine dinucleotide; OS, outer segments; PMCA, plasma membrane Ca2+-ATPases; NCX, Na+/Ca2+-exchanger; RIM, rab3-interacting molecule; RIM-BP, RIM-binding protein; ret-GC, retinal guanylate cyclase; SERCA, sarco/endoplasmic reticulum Ca2+-ATPase; SOCE, store-operated Ca2+-entry; TIRF, total internal reflection fluorescence; VGCC, voltage-gated Ca2+-channels; RE(A), RIBEYE(A)-domain; RE(B), RIBEYE(B)-domain.
References
Aartsen, W. M., Arsanto, J. P., Chauvin, J. P., Vos, R. M., Versteeg, I., Cardozo, B. N., et al. (2009). PSD95b regulates plasma membrane Ca(2+) pump localization at the photoreceptor synapse. Mol. Cell. Neurosci. 41, 156–165. doi: 10.1016/j.mcn.2009.02.003
Aartsen, W. M., Kantardzhieva, A., Klooster, J., van Rossum, A. G., van de Pavert, S. A., Versteeg, I., et al. (2006). MPP4 recruits PSD95 and veli3 towards the photoreceptor synapse. Hum. Mol. Genet. 15, 1291–1302. doi: 10.1093/hmg/ddl047
Abramov, I., and Gordon, J. (1994). Color appearance: on seeing red- or yellow, or green, or blue. Annu. Rev. Psychol. 45, 451–485. doi: 10.1146/annurev.ps.45.020194.002315
Adly, M., Spiwoks-Becker, I., and Vollrath, L. (1999). Ultrastructural changes of photoreceptor synaptic ribbons in relation to time of day and illumination. Invest. Ophthalmol. Vis. Sci. 40, 2165–2172.
Alonso, M. T., Manjarrés, I. M., and García-Sancho, J. (2012). Privileged coupling between Ca2+ entry through plasma membrane store-operated Ca2+ channels and the endoplasmic reticulum Ca2+-pump. Mol. Cell. Endocrinol. 353, 37–44. doi: 10.1016/j.mce.2011.08.021
Alpadi, K., Magupalli, V. G., Käppel, S., Köblitz, L., Schwarz, K., Seigel, G. M., et al. (2008). RIBEYE recruits Munc119, the mammalian ortholog of the Caenorhabditis elegans protein unc119 to synaptic ribbons of photoreceptor synapses. J. Biol. Chem. 283, 26461–26467. doi: 10.1074/jbc.M801625200
Arshavsky, V. Y. (2002). Rhodopsin phosphorylation: from terminating single photon responses to photoreceptor adaptation. Trends Neurosci. 25, 124–126. doi: 10.1016/S0166-2236(00)02094-4
Artemyev, N. O. (2008). Light-dependent compartmentalization of transducin in rod photoreceptors. Mol. Neurobiol. 37, 44–51. doi: 10.1007/s12035-008-8015-2
Babai, N., Morgans, C. W., and Thoreson, W. B. (2010). Calcium-induced calcium release contributes to synaptic release from mouse rod photoreceptors. Neuroscience 165, 1447–1456. doi: 10.1016/j.neuroscience.2009.11.032
Baehr, W., and Palczewski, K. (2009). Focus on molecules: guanylate cyclase-activating proteins (GCAPs). Exp. Eye Res. 89, 2–3. doi: 10.1016/j.exer.2008.12.016
Balkema, G. W., Cusick, K., and Nguyen, T. H. (2001). Diurnal variation in synaptic ribbon length and visual threshold. Vis. Neurosci. 18, 789–792. doi: 10.1017/S0952523801185123
Ball, S. L., McEnery, M. W., Yunker, A. M., Shin, H. S., and Gregg, R. G. (2011). Distribution of voltage gated calcium channel β-subunits in the mouse retina. Brain Res. 1412, 1–8. doi: 10.1016/j.brainres.2011.07.033
Ball, S. L., Powers, P. A., Shin, H. S., Morgans, C. W., Peachey, N. S., and Gregg, R. G. (2002). Role of the β2 subunit of voltage-dependent calcium channels in the retinal outer plexiform layer. Invest. Ophthalmol. Vis. Sci. 43, 1595–1603.
Bartoletti, T. M., Jackman, S. L., Babai, N., Mercer, A. J., Kramer, R. H., and Thoreson, W. B. (2011). Release from the cone ribbon synapse under bright light conditions can be controlled by the opening of few Ca(2+) channels. J. Neurophysiol. 106, 2922–2935. doi: 10.1152/jn.00634.2011
Blanks, J. C., Adinolfi, A. M., and Lolley, R. N. (1974). Synaptogenesis in the photoreceptor terminal of the mouse retina. J. Comp. Neurol. 156, 81–93. doi: 10.1002/cne.901560107
Bliss, T. V., and Collingridge, G. (1993). A synaptic model of memory: long-term potentiation in the hippocampus. Nature 361, 31–39. doi: 10.1038/361031a0
Brandt, A., Khimich, D., and Moser, T. (2005). Few Cav1.3 channels regulate the exocytosis of a synaptic vesicle at the hair cell ribbon synapse. J. Neurosci. 25, 11577–11585. doi: 10.1523/JNEUROSCI.3411-05.2005
Bublitz, M., Musgaard, M., Poulsen, H., Thogersen, L., Olesen, C., Schiott, B., et al. (2013). Ion pathways in the sarcoplasmic reticulum Ca2+ ATPase. J. Biol. Chem. 288, 10759–10765. doi: 10.1074/jbc.R112.436550
Buraei, Z., and Yang, Y. (2010). The β-subunit of Ca2+-channels. Physiol. Rev. 90, 1461–1506. doi: 10.1152/physrev.00057.2009
Burgoyne, R. D. (2007). Neuronal calcium sensor proteins: generating diversity in neuronal Ca2+-signalling. Nat. Rev. Neurosci. 8, 182–193. doi: 10.1038/nrn2093
Burns, M. E., and Baylor, D. E. (2001). Activation, deactivation, and adaptation in vertebrate photoreceptor cells. Annu. Rev. Neurosci. 24, 779–805. doi: 10.1146/annurev.neuro.24.1.779
Cadetti, L., Bryson, E. J., Ciccone, C. A., Rabl, K., and Thoreson, W. B. (2006). Calcium-induced calcium release in rod photoreceptors boosts synaptic transmission during maintained depolarization. Eur. J. Neurosci. 23, 2983–2990. doi: 10.1111/j.1460-9568.2006.04845.x
Carafoli, E. (1999). The plasma membrane calcium pump: structure, function and regulation. Biochem. Soc. Trans. 17, 808–810.
Catterall, W. A. (2011). Voltage-gated calcium channels. Cold Spring Harb. Perspect. Biol. 3, a003947. doi: 10.1101/cshperspect.a003947
Catterall, W. A., Leal, K., and Nanou, E. (2013). Calcium channels and short-term synaptic plasticity. J. Biol. Chem. 288, 10742–10749. doi: 10.1074/jbc.R112.411645
Catterall, W. A., Perez-Reyes, E., Snutch, T. P., and Striessnig, J. (2005). International union of pharmacology. XLVIII. Nomenclature and structure-function relationships of voltage-gated calcium channels. Pharmacol. Rev. 57, 411–425. doi: 10.1124/pr.57.4.5
Chang, B., Heckenlively, J. R., Bayley, P. R., Brecha, N. C., Davisson, M. C., Hawes, N. L., et al. (2006). The nob2 mouse, a null mutation in cacna1f: anatomical and functional abnormalities in the outer retina and their consequences on ganglion cells visual responses. Vis. Neurosci. 23, 11–24. doi: 10.1017/S095252380623102X
Chen, J., Billings, S. E., and Nishimune, H. (2011). Calcium channels link the muscle-derived synapse organizer laminin b2 to bassoon and CAST/ERC2 to organize presynaptic active zones. J. Neurosci. 31, 512–525. doi: 10.1523/JNEUROSCI.3771-10.2011
Chen, K. (2005). The vertebrate phototransduction cascade: amplification and termination mechanisms. Rev. Physiol. Biochem. Pharmacol. 154, 101–121.
Chen, M., van Hook, M., Zenisek, D., and Thoreson, W. B. (2013). Properties of ribbon and non-ribbon release from rod photoreceptors by visualizing individual synaptic vesicles. J. Neurosci. 33, 2071–2086. doi: 10.1523/JNEUROSCI.3426-12.2013
Chinnadurai, G. (2009). The transcriptional corepressor CtBP: a foe of multiple tumor suppressors. Cancer Res. 69, 731–734. doi: 10.1158/0008-5472.CAN-08-3349
Choi, S. Y., Borghuis, B. G., Rea, R., Levitan, E. S., Sterling, P., and Kramer, R. H. (2005). Encoding light intensity by the cone photoreceptor synapse. Neuron 48, 555–562. doi: 10.1016/j.neuron.2005.09.011
Choi, S. Y., Jackman, S., Thoreson, W. B., and Kramer, R. H. (2008). Light regulation of Ca2+ in the cone photoreceptor synaptic terminal. Vis. Neurosci. 25, 693–700. doi: 10.1017/S0952523808080814
Cooper, B., Hemmerlein, M., Ammermüller, J., Imig, C., Reim, K., Lipstein, N., et al. (2012). Munc13-independent vesicle priming at mouse photoreceptor ribbon synapses. J. Neurosci. 32, 8040–8052. doi: 10.1523/JNEUROSCI.4240-11.2012
Curtis, L., Datta, P., Liu, X., Bogdanova, N., Heidelberger, R., and Janz, R. (2010). Syntaxin3b is essential for the exocytosis of synaptic vesicles in ribbon synapses of the retina. Neurosci. 166, 832–841. doi: 10.1016/j.neuroscience.2009.12.075
Curtis, L. B., Doneske, B., Liu, X., Thaller, C., McNew, J. A., and Janz, R. (2008). Syntaxin3b is a t-SNARE specific for ribbon synapses of the retina. J. Comp. Neurol. 510, 550–559. doi: 10.1002/cne.21806
Dacheux, R. F., and Miller, R. F. (1976). Photoreceptor-bipolar cell transmission in the perfused retina eyecup of the mudpuppy. Science 191, 963–964. doi: 10.1126/science.175443
Deguchi-Tawarada, M., Inoue, E., Takao-Rikitsu, E., Inoue, M., Kitajima, I., Ohtsuka, T., et al. (2006). Active zone protein CAST is a component of conventional and ribbon synapses in the mouse retina. J. Comp. Neurol. 495, 480–496. doi: 10.1002/cne.20893
Dell’Orco, D., and Koch, K. W. (2010). Systems biochemistry approaches to vertebrate phototransduction: towards a molecular understanding of disease. Biochem. Soc. Trans. 38, 1275–1280. doi: 10.1042/BST0381275
Deng, L., Kaeser, P. S., Xu, W., and Südhof, T. C. (2011). RIM proteins activate vesicle priming by reversing autoinhibitory homodimerization of Munc13. Neuron 69, 317–331. doi: 10.1016/j.neuron.2011.01.005
De Vries, S. H. (2000). Bipolar cells use kainite and AMPA receptors to filter visual informations into separate channels. Neuron 28, 847–856. doi: 10.1016/S0896-6273(00)00158-6
De Vries, S. H., Li, W., and Saszik, S. (2006). Parallel processing in two transmitter microenvironments at the cone photoreceptor synapse. Neuron 50, 735–748. doi: 10.1016/j.neuron.2006.04.034
De Vries, S. H., and Schwartz, E. A. (1999). Kainate receptors mediate synaptic transmission between cones and “OFF” bipolar cells in a mammalian retina. Nature 397, 157–160. doi: 10.1038/16462
Dhingra, A., and Vardi, N. (2012). “mGlu receptors in the retina” – WIREs membrane transport and signaling. Wiley Interdiscip. Rev. Membr. Transp. Signal. 1, 641–653. doi: 10.1002/wmts.43
Dick, O., tom Dieck, S., Altrock, W. D., Ammermüller, J., Weiler, R., Garner, C. C., et al. (2003). The presynaptic active zone protein bassoon is essential for photoreceptor ribbon synapse formation in the retina. Neuron 37, 775–786. doi: 10.1016/S0896-6273(03)00086-2
Doering, C. J., Peloquin, J. B., and McRory, J. E. (2007). The CaV1.4 channel. Channels 1, 3–10. doi: 10.4161/chan.3938
Duncan, J. L., Yang, H., Doan, T., Silverstein, R. S., Murphy, G. J., Nune, G., et al. (2006). Scotopic visual signalling in the mouse retina is modulated by high-affinity plasma membrane calcium extrusion. J. Neurosci. 26, 7201–7211. doi: 10.1523/JNEUROSCI.5230-05.2006
Fioravante, D., and Regehr, W. G. (2011). Short-term forms of presynaptic plasticity. Curr. Opin. Neurobiol. 21, 269–274. doi: 10.1016/j.conb.2011.02.003
Fjeld, C. C., Birdsong, W. T., and Goodman, R. H. (2003). Differential binding of NAD+ and NADH allows the transcriptional corepressor carboxy-terminal binding protein to serve as a metabolic sensor. Proc. Natl. Acad. Sci. U.S.A. 100, 9202–9207. doi: 10.1073/pnas.1633591100
Förster, J. R., Lochnit, G., and Stöhr, H. (2009). Proteomic analysis of the membrane palmitoylated protein-4 (MPP4)-associated protein complex in the retina. Exp. Eye Res. 88, 39–48. doi: 10.1016/j.exer.2008.09.016
Frank, T., Khimich, D., Neef, A., and Moser, T. (2009). Mechanisms contributing to synaptic Ca2+ signals and their heterogeneity in hair cells. Proc. Natl. Acad. Sci. U.S.A. 106, 4483–4488. doi: 10.1073/pnas.0813213106
Frank, T., Rutherford, M. A., Strenzke, N., Neef, A., Pangrsic, T., Khimich, D., et al. (2010). Bassoon and the synaptic ribbon organize Ca(2+)-channels and vesicles to add release sites and promote refilling. Neuron 68, 724–738. doi: 10.1016/j.neuron.2010.10.027
Fuchs, M., Sendelbeck, A., Atorf, J., Kremers, J., and Brandstätter, J. H. (2013). Strain differences in illumination-dependent structural changes at mouse photoreceptor ribbon synapses. J. Comp. Neurol. 521, 68–78. doi: 10.1002/cne.23161
Glowatzki, E., and Fuchs, P. (2002). Transmitter release at the hair cell ribbon synapse. Nat. Neurosci. 5, 147–154. doi: 10.1038/nn796
Graydon, C. W., Cho, S., Li, G. L., Kachar, B., and von Gersdorff, H. (2011). Sharp Ca2+ nanodomains beneath the ribbon promote highly synchronous multivesicular release at hair cell synapses. J. Neurosci. 31, 16637–16650. doi: 10.1523/JNEUROSCI.1866-11.2011
Gudlur, A., Zhou, Y., and Hogan, P. G. (2013). STIM–ORAI interactions that control the CRAC channel. Curr. Top. Membr. 71, 33–58. doi: 10.1016/B978-0-12-407870-3.00002-0
Haeseleer, F., Imanishi, Y., Maeda, T., Possin, D. E., Maeda, A., Lee, A., et al. (2004). Essential role of Ca2+-binding protein 4, a Cav1.4 channel regulator, in photoreceptor synaptic function. Nat. Neurosci. 7, 1079–1087. doi: 10.1038/nn1320
Han, Y., Kaeser, P. S., Südhof, T. C., and Schneggenburger, R. (2011). RIM determines Ca(2+)-channel density and vesicle docking at the presynaptic active zone. Neuron 69, 304–316. doi: 10.1016/j.neuron.2010.12.014
Heidelberger, R., Thoreson, W. B., and Witkovsky, P. (2005). Synaptic transmission retinal ribbon synapses. Prog. Retin. Eye Res. 24, 682–720. doi: 10.1016/j.preteyeres.2005.04.002
Hermes, B., Reuss, S., and Vollrath, L. (1992). Synaptic ribbons, spheres and intermediate structures in the developing rat retina. Int. J. Dev. Neurosci. 10, 215–223. doi: 10.1016/0736-5748(92)90061-4
Hibino, H., Pironkova, R., Onwumere, O., Vologodkaia, M., Hudspeth, A. J., and Lesage, F. (2002). RIM binding proteins (RBPs) couple Rab3-interacting molecules (RIMs) to voltage-gated Ca2+-channels. Neuron 34, 411–423. doi: 10.1016/S0896-6273(02)00667-0
Hu, C., Bi, A., and Pan, Z. H. (2009). Differential expression of three T-type calcium channels in retinal bipolar cells in rats. Vis. Neurosci. 26, 177–187. doi: 10.1017/S0952523809090026
Hull, C., Studholme, K., Yazulla, S., and von Gersdorff, H. (2006). Diurnal changes in exocytosis and the number of synaptic ribbons at active zones of an ON-type bipolar cell terminal. J. Neurophysiol. 96, 2025–2033. doi: 10.1152/jn.00364.2006
Jackman, S. L., Choi, S. Y., Thoreson, W. B., Rabl, K., Bartoletti, T. M., and Kramer, R. H. (2009). Role of the synaptic ribbon in transmitting the cone light response. Nat. Neurosci. 12, 303–310. doi: 10.1038/nn.2267
Jarsky, T., Tian, M., and Singer, J. H. (2010). Nanodomain control of exocytosis is responsible for the signaling capability of a retinal ribbon synapse. J. Neurosci. 30, 11885–11895. doi: 10.1523/JNEUROSCI.1415-10.2010
Johnson, J. E. Jr., Perkins, G. A., Anand Giddabasappa, C. S., Chaney, S., Xiao, W., White, A. D., et al. (2007). Spatiotemporal regulation of ATP and Ca2+ dynamics in vertebrate rod and cone ribbon synapses. Mol. Vis. 13, 887–919.
Johnson, S., Halford, S., Morris, A. G., Patel, R. J., Wilkie, S. E., Hardcastle, A. J., et al. (2003). Genomic organization and alternative splicing of human RIM1, a gene implicated in autosomal dominant cone-rod dystrophy (CORD7). Genomics 81, 304–314. doi: 10.1016/S0888-7543(03)00010-7
Kaeser, P. S., Deng, L., Fan, M., and Südhof, T. C. (2012). RIM genes differentially contribute to organizing presynaptic release sites. Proc. Natl. Acad. Sci. U.S.A. 109, 11830–11835. doi: 10.1073/pnas.1209318109
Kaeser, P. S., Deng, L., Wang, Y., Dulubova, I., Liu, X., Rizo, J., et al. (2011). RIM tethers Ca2+-channels to presynaptic active zones via a direct PDZ-domain interaction. Cell 144, 282–295. doi: 10.1016/j.cell.2010.12.029
Kawamoto, E. M., Vivar, C., and Camandola, S. (2012). Physiology and pathology of calcium signaling in the brain. Front. Pharmacol. 3:61. doi: 10.3389/fphar.2012.00061
Khimich, D., Nouvian, R., Pujol, R., tom Dieck, S., Egner, A., Gundelfinger, E. D., et al. (2005). Hair cell synaptic ribbons are essential for synchronous auditory signalling. Nature 434, 889–894. doi: 10.1038/nature03418
Kiyonaka, S., Nakajima, H., Takada, Y., Hida, Y., Yoshioka, T., Hagiwara, A., et al. (2012). Physical and functional interaction of the active zone protein CAST/ERC2 and the β-subunit of the voltage-dependent Ca2+-channel. J. Biochem. 152, 149–159. doi: 10.1093/jb/mvs054
Kiyonaka, S., Wakamori, M., Miki, T., Uriu, Y., Nonaka, M., Bito, H., et al. (2007). RIM1 confers sustained activity and neurotransmitter vesicle anchoring to presynaptic Ca2+-channels. Nat. Neurosci. 10, 691–701. doi: 10.1038/nn1904
Knoflach, D., Kerov, V., Sartori, S. B., Obermair, G. J., Schmuckermair, C., Liu, X., et al. (2013). Cav1.4 IT mouse as a model for vision impairment in human congenital stationary night blindness type 2. Channels 7, 6. doi: 10.4161/chan.26368
Koch, K. W. (2006). GCAPs, the classical neuronal calcium sensors in the retina. A Ca2+-relay model of guanylate cyclase activation. Calcium Binding Proteins 1, 3–6.
Koch, K. W., Duda, T., and Sharma, R. K. (2010). Ca2+-modulated vision-linked ROS-GC guanylate cyclase transduction machinery. Mol Cell. Biochem. 334, 105–115. doi: 10.1007/s11010-009-0330-z
Korenbrot, J. I., and Rebrik, T. I. (2002). Tuning outer segment homeostasis to phototransduction in rods and cones. Adv. Exp. Med. Biol. 514, 179–203. doi: 10.1007/978-1-4615-0121-3_11
Krizaj, D., Bao, I. X., Schmitz, Y., Witkovsky, P., and Copenhagen, D. R. (1999). Caffeine-sensitive stores regulate synaptic transmission from retinal rod photoreceptors. J. Neurosci. 19, 7249–7261.
Krizaj, D. (2003). Properties of exocytic response in vertebrate photoreceptors. J. Neurophysiol. 90, 218–225. doi: 10.1152/jn.01025.2002
Krizaj, D., and Copenhagen, D. R. (2002). Calcium regulation in photoreceptors. Front. Biosci. 7:d2023–d2044. doi: 10.2741/krizaj
Krizaj, D., Lai, F. A., and Copenhagen, D. R. (2003). Ryanodine stores and calcium regulation in the inner segments of salamander rods and cones. J. Physiol. (Lond.) 547, 761–774. doi: 10.1113/jphysiol.2002.035683
Lalonde, M. R., Kelly, M. E., and Barnes, S. (2009). Calcium-activated chloride channels in the retina. Channels (Austin) 24, 252–260. doi: 10.4161/chan.2.4.6704
Lee, B. B., Martin, P. R., and Grünert, U. (2010). Retinal connectivity and primate vision. Prog. Retin. Eye Res. 29, 622–639. doi: 10.1016/j.preteyeres.2010.08.004
Lenzi, D., and von Gersdorff, H. (2001). Structure suggests function: the case for synaptic ribbons as exocytotic nanomachines. Bioessays 23, 831–840. doi: 10.1002/bies.1118
Lewis, R. S. (2011). Store-operated calcium channels: new perspectives on mechanism and function. Cold Spring Harb. Perspect. Biol. 3, a003970. doi: 10.1101/cshperspect.a003970
Libby, R. T., Lavallee, C. R., Balkema, G. W., Brunken, W. J., and Hunter, D. D. (1999). Disruption of laminin beta2 chain production causes alterations in morphology and function in the CNS. J. Neurosci. 19, 9399–9411.
Liu, X., Kerov, V., Haeseleer, F., Majumder, A., Artemyev, N., Baker, S. A., et al. (2013). Dysregulation of Cav1.4 channels disrupts the maturation of photoreceptor synaptic ribbons in congenital stationary night blindness type 2. Channels 24, 7.
Lodha, N., Bonfield, S., Orton, N. C., Doering, C. J., McRory, J. E., Mema, S.C., et al. (2010). Congenital stationary night blindness in mice – a tale of two caca1f mutants. Adv. Exp. Med. Biol. 664, 549–558. doi: 10.1007/978-1-4419-1399-9_63
López-del Hoyo, N., Fazioli, L., López-Begines, S., Fernández-Sanchez, L., Cuenca, N., Llorens, J., et al. (2012). Overexpression of guanylate cyclase activating protein 2 in rod photoreceptors in vivo leads to morphological changes at the synaptic Ribbon. PLoS ONE 7:e42994. doi: 10.1371/journal.pone.0042994
Lytton, J. (2007). Na+/Ca2+-exchangers: three mammalian genes families control Ca2+ transport. Biochem. J. 406, 365–382. doi: 10.1042/BJ20070619
Ma, Y. P., and Pan, Z. H. (2003). Spontaneous regenerative activity in mammalian retinal bipolar cells: roles of multiple subtypes of voltage-dependent Ca(2+) channels. Vis. Neurosci. 20, 131–139. doi: 10.1017/S0952523803202042
Magupalli, V. G., Schwarz, K., Alpadi, K., Natarajan, S., Seigel, G. M., and Schmitz, F. (2008). Multiple RIBEYE–RIBEYE interactions create a dynamic scaffold for the formation of synaptic ribbons. J. Neurosci. 28, 7954–7967. doi: 10.1523/JNEUROSCI.1964-08.2008
Malenka, R. C., and Nicoll, R. A. (1999). Long-term potentiation – a decade of progress? Science 285, 1870–1874. doi: 10.1126/science.285.5435.1870
Manjarrés, I. M., Alonso, M. T., and García-Sancho, J. (2011). Calcium entry-calcium refilling (CECR) coupling between store-operated Ca2+ entry and sarco/endoplasmic reticulum ATPase. Cell Calcium 49, 153–161. doi: 10.1016/j.ceca.2011.01.007
Manjarrés, I. M., Rodriguez-Garcia, A., Alonso, M. T., and García-Sancho, J. (2010). The sarco/endoplasmic reticulum Ca(2+)-ATPase (SERCA) is the third element in capacitive calcium entry. Cell Calcium 47, 412–418. doi: 10.1016/j.ceca.2010.03.001
Mansergh, F., Orton, N. C., Vessey, J. P., Lalonde, M. R., Stell, W. K., Tremblay, F., et al. (2005). Mutation of the calcium channel gene Cacna1f disrupts calcium signaling, synaptic transmission and cellular organization in mouse retina. Hum. Mol. Genet. 14, 3035–3046. doi: 10.1093/hmg/ddi336
Matthews, G., and Fuchs, P. (2010). The diverse roles of ribbon synapses in sensory neurotransmission. Nat. Rev. Neurosci. 11, 812–822. doi: 10.1038/nrn2924
Mercer, A. J., Chen, M., and Thoreson, W. B. (2011a). Lateral mobility of presynaptic L-type calcium channels at photoreceptor ribbon synapses. J. Neurosci. 31, 4397–4406. doi: 10.1523/JNEUROSCI.5921-10.2011
Mercer, A. J., Rabl, K., Riccardi, G. E., Brecha, N. C., Stella, S. L. Jr., and Thoreson, W. B. (2011b). Location of release sites and calcium-activated chloride channels relative to calcium channels at the photoreceptor ribbon synapse. J. Neurophysiol. 105, 321–335. doi: 10.1152/jn.00332.2010
Mercer, A. J., and Thoreson, W. B. (2011). The dynamic architecture of photoreceptor ribbon synapses: cytoskeletal, extracellular matrix, and intramembrane proteins. Vis. Neurosci. 28, 453–471. doi: 10.1017/S0952523811000356
Midorikawa, M., Tsukamoto, Y., Berglund, K., Ishii, M., and Tachibana, M. (2007). Different roles of ribbon-associated and ribbon-free active zones in retinal bipolar cells. Nat. Neurosci. 10, 1268–1276. doi: 10.1038/nn1963
Miki, T., Kiyonaka, S., Uriu, Y., deWaard, M., Wakamori, M., Beedle, A. M., et al. (2007). Mutation associated with an autosomal dominant cone-rod dystrophy CORD7 modifies RIM1-mediated modulation of voltage-gated Ca2+-channels. Channels 1, 144–147.
Molnar, T., Barabas, P., Birnbaumer, L., Punzo, C., Kefalov, V., and Krizaj, D. (2012). Store-operated channels regulate intracellular calcium in mammalian rods. J. Physiol. 590, 3465–3481. doi: 10.1113/jphysiol.2012.234641
Morgans, C. W., Bayley, P. R., Oesch, N., Ren, G., Akileswaran, L., and Taylor, W. R. (2005). Photoreceptor calcium channels: insight from night blindness. Vis. Neurosci. 22, 561–568. doi: 10.1017/S0952523805225038
Moser, T., Brandt, A., and Lysakowski, A. (2006). Hair cell ribbon synapses. Cell Tissue Res. 326, 347–359. doi: 10.1007/s00441-006-0276-3
Mustafi, D., Engel, A. H., and Palczewski, K. (2009). Structure of cone photoreceptors. Prog. Retin. Eye Res. 28, 289–302. doi: 10.1016/j.preteyeres.2009.05.003
Nassi, J. J., and Callaway, E. M. (2009). Parallel processing strategies of the primate visual system. Nat. Rev. Neurosci. 10, 360–372. doi: 10.1038/nrn2619
Neher, E., and Sakaba, T. (2008). Multiple roles of calcium ions in the regulation of neurotransmitter release. Neuron 59, 861–872. doi: 10.1016/j.neuron.2008.08.019
Nicoll, R. A., and Schmitz, D. (2005). Synaptic plasticity at hippocampal mossy fibre synapses. Nat. Rev. Neurosci. 6, 863–876. doi: 10.1038/nrn1786
Nishimune, H. (2012). Active zones of mammalian neuromuscular junctions: formation, density, and aging. Ann. N. Y. Acad. Sci. 1274, 24–32. doi: 10.1111/j.1749-6632.2012.06836.x
Nishimune, H., Numata, T., Chen, J., Aoki, Y., Wang, Y., Starr, M. P., et al. (2012). Active zone protein bassoon co-localizes with presynaptic calcium channels, modifies channel function, and recovers from aging related loss by exercise. PLoS ONE 7:e38029. doi: 10.1371/journal.pone.0038029
Nishimune, H., Sanes, J. R., and Carlson, S. S. (2004). A synaptic laminin–calcium channel interaction organizes active zones in motor nerve terminals. Nature 432, 580–587. doi: 10.1038/nature03112
Nomura, A., Shigemoto, R., Nakanishi, Y., Okamoto, N., Mizuno, N., and Nakanishi, S. (1994). Developmentally regulated postsynaptic localization of a metabotropic glutamate receptor in rat rod bipolar cells. Cell 77, 361–369. doi: 10.1016/0092-8674(94)90151-1
Nouvian, R., Neef, J., Bulankina, A., Reisinger, E., Pangrsic, T., Frank, T., et al. (2011). Exocytosis at the hair cell ribbon synapse operates without neuronal SNARE proteins. Nat. Neurosci. 14, 411–413. doi: 10.1038/nn.2774
Olney, J. W. (1968). An electron microscopic study of synapse formation, receptor outer segment development, and other aspects of developing mouse retinas. Invest. Ophthalmol. 7, 250–268.
Olshevskaya, E. V., Ermilov, A. N., and Dizhoor, A. M. (1999). Dimerization of guanylyl cyclase-activating protein and a mechanism of photoreceptor guanylyl cyclase activation. J. Biol. Chem. 274, 25583–25587. doi: 10.1074/jbc.274.36.25583
Palczewski, K., Sokal, I., and Baehr, W. (2004). Guanylate cyclase-activating proteins: structure, function and diversity. Biochem. Biophys. Res. Commun. 322, 1123–1130. doi: 10.1016/j.bbrc.2004.07.122
Paliwal, S., Ho, N., Parker, D., and Grossman, S. R. (2012). CtBP2 promotes human cancer cell migration by transcriptional activation of Tiam1. Genes Cancer 3, 481–490. doi: 10.1177/1947601912463695.
Paliwal, S., Kovi, R., Nath, B., Chen, Y., Lewis, B., and Grossman, S. R. (2007). ARF antagonizes cancer cell migration via interaction with CtBP corepressor. Cancer Res. 67, 9322–9329. doi: 10.1158/0008-5472.CAN-07-1743
Paliwal, S., Pande, S., Kovi, R., Shapless, N., Bardeesy, N., and Grossman, S. R. (2006). Targeting of C-terminal binding protein by ARF results in p53-independent apoptosis. Mol. Cell. Biol. 26, 2360–2372. doi: 10.1128/MCB.26.6.2360-2372.2006
Pan, Z. H., Hu, H. J., Perring, P., and Andrade, R. (2001). T-type Ca2+-channels mediate neurotransmitter release in retinal bipolar cells. Neuron 32, 89–98. doi: 10.1016/S0896-6273(01)00454-8
Parekh, A. B. (2011). Decoding cytoplasmic Ca2+ oscillations. Trends Biochem. Sci. 36, 78–87. doi: 10.1016/j.tibs.2010.07.013
Parekh, A. B., and Putney, J. W. Jr. (2005). Store-operated calcium channels. Physiol. Rev. 85, 757–810. doi: 10.1152/physrev.00057.2003
Parsons, T. D., and Sterling, P. (2003). Synaptic ribbon. Conveyor belt or safety belt? Neuron 37, 379–382. doi: 10.1016/S0896-6273(03)00062-X
Peng, Y. W., Sharp, A. H., Snyder, S. H., and Yau, K. W. (1991). Localization of the inositol 1,4,5-trisphosphate receptor in synaptic terminals in the vertebrate retina. Neuron 6, 525–531. doi: 10.1016/0896-6273(91)90055-5
Peshenko, I. V., Olshevskaya, E. V., and Dizhoor, A. M. (2004). Ca2+-dependent conformational changes in guanylyl cyclase-activating protein 2 (GCAP2) revealed by site-specific phosphorylation and partial proteolysis. J. Biol. Chem. 279, 50342–50349. doi: 10.1074/jbc.M408683200
Rao-Mirotznik, R., Harkins, A. B., Buchsbaum, G., and Sterling, P. (1995). Mammalian rod terminal: architecture of a binary synapse. Neuron 14, 561–569. doi: 10.1016/0896-6273(95)90312-7
Raven, M. A., Orton, N. C., Nassar, H., William, G. A., Stell, W. K., Jacobs, G. H., et al. (2007). Early afferent signaling in the outer plexiform layer regulates the development of horizontal cell morphology. J. Comp. Neurol. 506, 745–758. doi: 10.1002/cne.21526
Regehr, W. G. (2012). Short-term plasticity. Cold Spring Harb. Perspect. Biol. 4, a005702. doi: 10.1101/cshperspect.a005702
Regus-Leidig, H., and Brandstätter, J. H. (2012). Structure and function of a complex sensory synapse. Acta Physiol. (Oxf.) 204, 479–486. doi: 10.1111/j.1748-1716.2011.02355.x
Regus-Leidig, H., Ott, C., Löhner, M., Atorf, J., Fuchs, M., Sedmak, T., et al. (2013). Identification and immunocytochemical characterization of piccolino, a novel splice variant selectively expressed at sensory ribbon synapses of the eye and ear. PLoS ONE 8:e70373. doi: 10.1371/journal.pone.0070373
Regus-Leidig, H., Specht, D., tom Dieck, S., and Brandstätter, J. H. (2010a). Stability of active zone components at the photoreceptor ribbon complex. Mol. Vis. 16, 2690–2770.
Regus-Leidig, H., tom Dieck, S., and Brandstätter, J. H. (2010b). Absence of functional active zone protein bassoon affects assembly and transport of ribbon precursors during early steps of photoreceptor synaptogenesis. Mol. Vis. 16, 2699–2700.
Reim, K., Regus-Leidig, H., Ammermüller, J., El-Kordi, A., Radyushkin, K., Ehrenreich, H., et al. (2009). Aberrant function and structure of retinal ribbon synapses in the absence of complexin 3 and complexin 4. J. Cell Sci. 122, 1352–1361. doi: 10.1242/jcs.045401
Reim, K., Wegmeyer, H., Brandstätter, J. H., Xue, M., Rosenmund, C., Dresbach, T., et al. (2005). Structurally and functionally unique complexins at retinal ribbon synapses. J. Cell Biol. 169, 669–680. doi: 10.1083/jcb.200502115
Rieke, F., and Schwartz, E. A. (1996). Asynchronous transmitter release: control of exocytosis and endocytosis at the salamander rod synapse. J. Physiol. 493, 1–8.
Rizo, J., and Südhof, T. C. (2012). The membrane fusion enigma: SNAREs, sec1/Munc18 proteins, and their complices-guilty as charged? Annu. Rev. Cell Dev. Biol. 28, 279–308. doi: 10.1146/annurev-cellbio-101011-155818
Rizzuto, R., Duchen, M. R., and Pozzan, T. (2004). Flirting in little space: the ER/mitochondria liaison. Sci STKE 215, re1. doi: 10.1126/stke.2152004re1
Rizzuto, R., and Pozzan, T. (2006). Microdomains of intracellular Ca2+: molecular determinants and functional consequences. Physiol. Rev. 86, 369–408. doi: 10.1152/physrev.00004.2005
Rusakov, D. A. (2006). Ca2+-dependent mechanisms of presynaptic control at central synapses. Neuroscientist 12, 317–326. doi: 10.1177/1073858405284672
Safieddine, S., El-Amraoui, A., and Petit, C. (2012). The auditory hair cell ribbon synapse: from assembly to function. Annu. Rev. Neurosci. 35, 509–528. doi: 10.1146/annurev-neuro-061010-113705
Sakurai, K., Chen, J., and Kefalov, V. J. (2011). Role of guanylyl cyclase modulation in mouse cone phototransduction. J. Neurosci. 31, 7991–8000. doi: 10.1523/JNEUROSCI.6650-10.2011
Schmitz, F. (2009). The making of synaptic ribbons: how they are built and what they do. Neuroscientist 15, 611–624. doi: 10.1177/1073858409340253
Schmitz, F., Augustin, I., and Brose, N. (2001). Absence of the synaptic vesicle priming protein Munc13-1 from tonically active ribbon synapses of the rat retina. Brain Res. 895, 258–263. doi: 10.1016/S0006-8993(01)02078-9
Schmitz, F., and Drenckhahn, D. (1993). Intermediate stages in the disassembly of synaptic ribbons in cone photoreceptors of the crucian carp, Carassius carassius. Cell Tissue Res. 272, 487–490. doi: 10.1007/BF00318554
Schmitz, F., Königstorfer, A., and Südhof, T. C. (2000). RIBEYE, a component of synaptic ribbons: a protein’s journey through evolution provides insights into synaptic ribbon function. Neuron 28, 857–872. doi: 10.1016/S0896-6273(00)00159-8
Schmitz, F., Natarajan, N., Venkatesan, J. K., Wahl, S., Schwarz, K., and Grabner, C. P. (2012). EF hand-mediated Ca2+- and cGMP-signaling in photoreceptor synaptic terminals. Front. Mol. Neurosci. 5:26. doi: 10.3389/fnmol.2012.00026
Schmitz, F., Tabares, L., Khimich, D., Strenzke, N., de la Villa-Polo, P., Castellano-Munoz, M., et al. (2006). CSPalpha-deficiency causes massive and rapid photoreceptor degeneration. Proc. Natl. Acad. Sci. U.S.A. 103, 2926–2931. doi: 10.1073/pnas.0510060103
Schmitz, Y., and Witkovsky, P. (1997). Dependence of photoreceptor glutamate release on a dihydropyridine-sensitive calcium channel. Neuroscience 78, 1209–1216. doi: 10.1016/S0306-4522(96)00678-1
Shaltiel, L., Paparizos, C., Fenske, S., Hassan, S., Gruner, C., Rötzer, K., et al. (2012). Complex regulation of voltage-dependent activation and inactivation properties of retinal voltage-gated Cav1.4 L-type Ca2+ channels by Ca2+-binding protein 4 (CaBP4). J. Biol. Chem. 287, 36312–36321. doi: 10.1074/jbc.M112.392811
Sharma, R. K. (2002). Evolution of the membrane guanylate cyclase transduction system. Mol. Cell. Biochem. 230, 3–30. doi: 10.1023/A:1014280410459
Sharma, R. K. (2010). Membrane guanylate cyclase is a beautiful signal transduction machinery. Mol. Cell. Biochem. 334, 3–36. doi: 10.1007/s11010-009-0336-6
Sharma, R. K., and Duda, T. (2012). Ca2+-sensors and ROS-GC: interlocked sensory transduction elements: a review. Front. Mol. Neurosci. 5:42. doi: 10.3389/fnmol.2012.00042
Sheets, L., Kindt, K. S., and Nicolson, T. (2012). Presynaptic CaV1.3 channels regulate synaptic ribbon size and are required for synaptic maintenance in sensory hair cells. J. Neurosci. 28, 17273–17286. doi: 10.1523/JNEUROSCI.3005-12.2012
Sheets, L., Trapani, J. G., Mo, W., Obholzer, N., and Nicolson, T. (2011). RIBEYE is required for presynaptic Ca(V)1.3 channel localization and afferent innervation of sensory hair cells. Development 138, 1309–1319. doi: 10.1242/dev.059451
Sheng, Z., Choi, S. Y., Dharia, A., Li, J., Sterling, P., and Kramer, R. H. (2007). Synaptic Ca2+ in darkness is lower in rods than in cones, causing slower tonic release of vesicles. J. Neurosci. 27, 5033–5042. doi: 10.1523/JNEUROSCI.5386-06.2007
Singer, J. H., Lassová, L., Vardi, N., and Singer, J. S. (2004). Coordinated multivesicular release at a mammalian ribbon synapse. Nat. Neurosci. 7, 826–833. doi: 10.1038/nn1280
Singh, A., Hamedinger, D., Hoda, J. C., Gebhart, M., Koschak, A., Romanin, C., et al. (2006). C-terminal modulator controls Ca2+-dependent gating of Ca(v)1.4 L-type Ca2+ channels. Nat. Neurosci. 9, 1108–1116. doi: 10.1038/nn1751
Snellman, J., Mehta, B., Babai, N., Bartoletti, T. M., Akmentin, W., Francis, A., et al. (2011). Acute destruction of the synaptic ribbon reveals a role for the ribbon in vesicle priming. Nat. Neurosci. 14, 1135–1141. doi: 10.1038/nn.2870
Soboloff, J., Rothberg, B. S., Madesh, M., and Gill, D. L. (2012). STIM proteins: dynamic calcium signal transducers. Nat. Rev. Mol. Cell Biol. 13, 549–565. doi: 10.1038/nrm3414
Spiwoks-Becker, I., Glas, M., Lasarzik, I., and Vollrath, L. (2004). Synaptic ribbons lose and regain material in response to illumination changes. Eur. J. Neurosci. 19, 1559–1571. doi: 10.1111/j.1460-9568.2004.03198.x
Stephen, R., Bereta, G., Golczak, M., Palczewski, K., and Souza, M. C. (2007). Stabilizing function for myristoyl group revealed by the crystal structure of a neuronal calcium sensor, the guanylate cyclase-activating protein1. Structure 15, 1392–1402. doi: 10.1016/j.str.2007.09.013
Stephen, R., Filipek, S., Palczewski, K., and Souza, M. C. (2008). Ca2+-dependent regulation of phototransduction. Photochem. Photobiol. 84, 903–910. doi: 10.1111/j.1751-1097.2008.00323.x
Stöhr, H., Heisig, J. B., Benz, P. M., Schöberl, S., Milenkovic, V. M., Strauss, O., et al. (2009). TMEM16B, a novel protein with calcium-dependent chloride channel activity, associates with a presynaptic protein complex in photoreceptor terminals. J. Neurosci. 29, 6809–6818. doi: 10.1523/JNEUROSCI.5546-08.2009
Striessnig, J., Bolz, H. J., and Koschak, A. (2010). Channelopathies in CaV1.1, CaV1.3, and CaV1.4 voltage-gated L-type Ca2+-channels. Pflügers Arch. 460, 361–374. doi: 10.1007/s00424-010-0800-x
Südhof, T. C. (2012a). Calcium control of neurotransmitter release. Cold Spring Harb. Perspect. Biol. 4, a011353. doi: 10.1101/cshperspect.a011353
Südhof, T. C. (2012b). The presynaptic active zone. Neuron 75, 11–25. doi: 10.1016/j.neuron.2012.06.012
Südhof, T. C. (2013). A molecular machine for neurotransmitter release: synaptotagmin and beyond. Nat. Med. 19, 1227–1231. doi: 10.1038/nm.3338
Sung, C.-H., and Chuang, J. Z. (2010). The cell biology of vision. J. Cell Biol. 190, 953–963. doi: 10.1083/jcb.201006020
Suryanarayanan, A., and Slaughter, M. M. (2006). Synaptic transmission mediated by internal calcium stores in rod photoreceptors. J. Neurosci. 26, 1759–1766. doi: 10.1523/JNEUROSCI.3895-05.2006
Szikra, T., and Krizaj, D. (2006). The dynamic range and domain-specific signals of intracellular calcium in photoreceptors. Neuroscience 141, 143–155. doi: 10.1016/j.neuroscience.2006.03.054
Szikra, T., and Krizaj, D. (2007). Intracellular organelles and calcium homeostasis in rods and cones. Vis. Neurosci. 24, 733–743. doi: 10.1017/S0952523807070587
Szikra, T., Barabas, P., Bartoletti, T. M., Huang, W., Akopian, A., Thoreson, W. B., et al. (2009). Calcium homeostasis and cone signalling are regulated by interactions between calcium stores and plasma membrane ion channels PLoS ONE 4:e6723. doi: 10.1371/journal.pone.0006723
Szikra, T., Cusato, K., Thoreson, W. B., Barabas, P., Bartoletti, T. M., and Krizaj, D. (2008). Depletion of calcium stores regulates calcium influx and signal transmission in rod photoreceptors. J. Physiol. 586, 4859–4875. doi: 10.1113/jphysiol.2008.160051
Tempel, B. L., and Shilling, D. J. (2007). The plasma membrane calcium ATPase and disease. Subcell. Biochem. 45, 365–383. doi: 10.1007/978-1-4020-6191-2_13
Thoreson, W. B., Rabl, K., Townes-Anderson, E., and Heidelberger, R. (2004). A highly Ca2+-sensitive pool of synaptic vesicles contributes to linearity at the rod photoreceptor ribbon synapse. Neuron 42, 595–605. doi: 10.1016/S0896-6273(04)00254-5
Thoreson, W. B., Stella, S. L. Jr., Bryson, E. L., Clements, J., and Witkovsky, P. (2002). D2-like dopamine receptors promote interactions between calcium and chloride channels that diminish rod synaptic transfer in the salamander retina. Vis. Neurosci. 19, 235–247. doi: 10.1017/S0952523802192017
tom Dieck, S., Altrock, W. D., Kessels, M. M., Qualmann, B., Regus, H., Brauner, D., et al. (2005). Molecular dissection of the photoreceptor ribbon synapse: physical interaction of bassoon and RIBEYE is essential for the development of the ribbon complex. J. Cell Biol. 168, 825–836. doi: 10.1083/jcb.200408157
tom Dieck, S., and Brandstätter, J. H. (2006). Ribbon synapses of the retina. Cell Tissue Res. 326, 339–346. doi: 10.1007/s00441-006-0234-0
Venkatesan, J. K., Natarajan, S., Schwarz, K., Mayer, S. I., Alpadi, K., Magupalli, V. G., et al. (2010). Nicotinamide adenine dinucleotide-dependent binding of the neuronal Ca2+ sensor protein GCAP2 to photoreceptor synaptic ribbons. J. Neurosci. 30, 6559–6567. doi: 10.1523/JNEUROSCI.3701-09.2010
Vollrath, L., and Spiwoks-Becker, I. (1996). Plasticity of retinal ribbon synapses. Microsc. Res. Tech. 35, 472–487. doi: 10.1002/(SICI)1097-0029(19961215)35:6<472::AID-JEMT6>3.0.CO;2-K
Wahl, S., Katiyar, R., and Schmitz, F. (2013). A local, periactive zone endocytic machinery at photoreceptor synapses in close vicinity to synaptic ribbons. J. Neurosci. 33, 10278–10300. doi: 10.1523/JNEUROSCI.5048-12.2013
Wan, L., Almers, W., and Chen, W. (2005). Two ribeye genes in teleosts: the role of RIBEYE in ribbon formation and bipolar cell development. J. Neurosci. 25, 941–949. doi: 10.1523/JNEUROSCI.4657-04.2005
Wan, Q. F., Nixon, E., and Heidelberger, R. (2012). Regulation of presynaptic calcium in a mammalian synaptic terminal. J. Neurophysiol. 108, 3059–3067. doi: 10.1152/jn.00213.2012
Wang, Y., Okamoto, M., Schmitz, F., Hofmann, K., and Südhof, T. C. (1997). RIM is a putative effector in regulating synaptic-vesicle fusion. Nature 388, 593–598. doi: 10.1038/41580
Wässle, H. (2004). Parallel processing in the mammalian retina. Nat. Rev. Neurosci. 5, 1–12. doi: 10.1038/nrn1497
Witkovsky, P., Schmitz, Y., Akopian, A., Krizaj, D., and Tranchina, D. (1997). Gain of rod to horizontal cell synaptic transfer: relation to glutamate release and a dihydropyridine-sensitive calcium current. J. Neurosci. 17, 7297–7306.
Wycisk, K. A., Budde, B., Feil, S., Skosyrski, S., Buzzi, F., Neidhardt, J., et al. (2006). Structural and functional abnormalities of retinal ribbon synapses due to cacna2d4 mutations. Invest. Ophthalmol. Vis. Sci. 47, 3523–3530. doi: 10.1167/iovs.06-0271
Xing, W., Akopian, A., and Krizaj, D. (2012). Trafficking of the presynaptic PMCA signaling complexes in mouse photoreceptors requires Cav1.4 β1-subunits. Adv. Exp. Med. Biol. 723, 739–744. doi: 10.1007/978-1-4614-0631-0_94
Yang, J., Pawlyk, B., Wen, X. H., Adamian, M., Soloview, M., Michaud, N., et al. (2007). MPP4 is required for the proper localization of plasma membrane calcium ATPases and maintain calcium homeostasis at the rod photoreceptor synaptic terminal. Hum. Mol. Genet. 16, 1017–1029. doi: 10.1093/hmg/ddm047
Zabouri, N., and Haverkamp, S. (2013). Calcium channel-dependent molecular maturation of photoreceptor synapses. PLoS ONE 8:e63853. doi: 10.1371/journal.pone.0063853
Zenisek, D. (2008). Vesicle association and exocytosis at ribbon and extraribbon sites in retinal bipolar cell presynaptic terminals. Proc. Natl. Acad. Sci. U.S.A. 105, 4922–4927. doi: 10.1073/pnas.0709067105
Zenisek, D., and Matthews, G. (2000). The role of mitochondria in presynaptic calcium handling at a ribbon synapse. Neuron 25, 229–237. doi: 10.1016/S0896-6273(00)80885-5
Keywords: photoreceptor, ribbon synapse, synaptic ribbon, RIBEYE, Ca2+, GCAP2
Citation: Schmitz F (2014) Presynaptic [Ca2+] and GCAPs: aspects on the structure and function of photoreceptor ribbon synapses. Front. Mol. Neurosci. 7:3. doi: 10.3389/fnmol.2014.00003
Received: 30 October 2013; Accepted: 15 January 2014;
Published online: 06 February 2014.
Edited by:
Rameshwar K. Sharma, Salus University, USAReviewed by:
Matthias Kneussel, Zentrum für Molekulare Neurobiologie Hamburg, GermanyNoga Vardi, University of Pennsylvania, USA
Copyright © 2014 Schmitz. This is an open-access article distributed under the terms of the Creative Commons Attribution License (CC BY). The use, distribution or reproduction in other forums is permitted, provided the original author(s) or licensor are credited and that the original publication in this journal is cited, in accordance with accepted academic practice. No use, distribution or reproduction is permitted which does not comply with these terms.
*Correspondence: Frank Schmitz, Department of Neuroanatomy, Institute for Anatomy and Cell Biology, Medical School Homburg/Saar, Saarland University, Kirrbergerstrasse, University Campus, Building 61, Homburg/Saar 66421, Saarland, Germany e-mail: frank.schmitz@uks.eu