Emerging Roles of Neuronal Ca2+ Sensor-1 in Cardiac and Neuronal Tissues: A Mini Review
- 1Department of Molecular Physiology, National Cerebral and Cardiovascular Center Research Institute, Suita, Japan
- 2Department of Biomedical Sciences, College of Life Sciences, Ritsumeikan University, Kusatsu, Japan
- 3Department of Pharmacology, Osaka Medical College, Takatsuki, Japan
The EF-hand calcium (Ca2+)-binding protein, neuronal Ca2+ sensor-1 (NCS-1/frequenin), is predominantly expressed in neuronal tissues and plays a crucial role in neuronal functions, including synaptic transmission and plasticity. NCS-1 has diverse functional roles, as elucidated in the past 15 years, which include the regulation of phosphatidylinositol 4-kinase IIIβ (PI-4K-β) and several ion channels such as voltage-gated K+ and Ca2+ channels, the D2 dopamine receptors, and inositol 1,4,5-trisphosphate receptors (InsP3Rs). Functional analyses demonstrated that NCS-1 enhances exocytosis and neuronal survival after injury, as well as promotes learning and memory in mice. NCS-1 is also expressed in the heart including the Purkinje fibers (PFs) of the conduction system. NCS-1 interacts with KV4 K+ channels together with dipeptidyl peptidase-like protein-6 (DPP-6), and this macromolecule then composes the transient outward current in PFs and contributes to the repolarization of PF action potential, thus being responsible for idiopathic arrhythmia. Moreover, NCS-1 expression was reported to be significantly high at the immature stage and at hypertrophy in adults. That report demonstrated that NCS-1 positively regulates cardiac contraction in immature hearts by increasing intracellular Ca2+ signals through interaction with InsP3Rs. With the related signals, NCS-1 activates nuclear Ca2+ signals, which would be a mechanism underlying hormone-induced cardiac hypertrophy. Furthermore, NCS-1 contributes to stress tolerance in cardiomyocytes by activating mitochondrial detoxification pathways, with a key role in Ca2+-dependent pathways. In this review, we will discuss recent findings supporting the functional significance of NCS-1 in the brain and heart and will address possible underlying molecular mechanisms.
NCS-1 and its Interacting Proteins
Intracellular calcium (Ca2+) is a versatile second messenger that regulates diverse cellular processes, including neurotransmission, muscle contraction, and signal transduction. Changes in intracellular Ca2+ are transduced by multiple proteins, with a key role of a large family of EF-hand Ca2+-binding proteins that act as Ca2+ sensors or Ca2+ buffers. Ca2+-buffer proteins chelate Ca2+ and often terminate Ca2+ signals (Ikura, 1996). In contrast, the binding of Ca2+ to Ca2+-sensor proteins causes a large conformational change, which consequently transduces the Ca2+ signal into various cellular functional changes, by regulating specific target proteins. Calmodulin is one of the best-characterized Ca2+-sensor proteins and is involved in many aspects of Ca2+ signaling. Neuronal calcium sensor-1 (NCS-1) is the mammalian homolog of the Drosophila frequenin protein, which belongs to the larger NCS protein family that includes NCS-1, visinin-like proteins, recoverin, guanylate cyclase-activating proteins, and potassium channel-interacting proteins (KChIPs). The structure of NCS-1 is shown in the Figure 1A, indicating that it is a small (22 kDa) Ca2+-binding protein containing 4 EF-hand motifs; of these, 3 (EF2-4) bind to Ca2+. Unlike ubiquitously expressed calmodulin, NCS-1 is predominantly expressed in the brain and cardiac tissues, suggesting its specialized roles in these tissues. The Ca2+-binding affinity of NCS-1 is significantly higher than that of calmodulin (Kd values of ~300 nM vs. ~10 μM, respectively). Although both Ca2+-binding proteins can operate within the physiological range of Ca2+ levels (~100 nM to ~1–5 μM), the above data suggest that NCS-1 may be more sensitive to small changes in intracellular Ca2+.
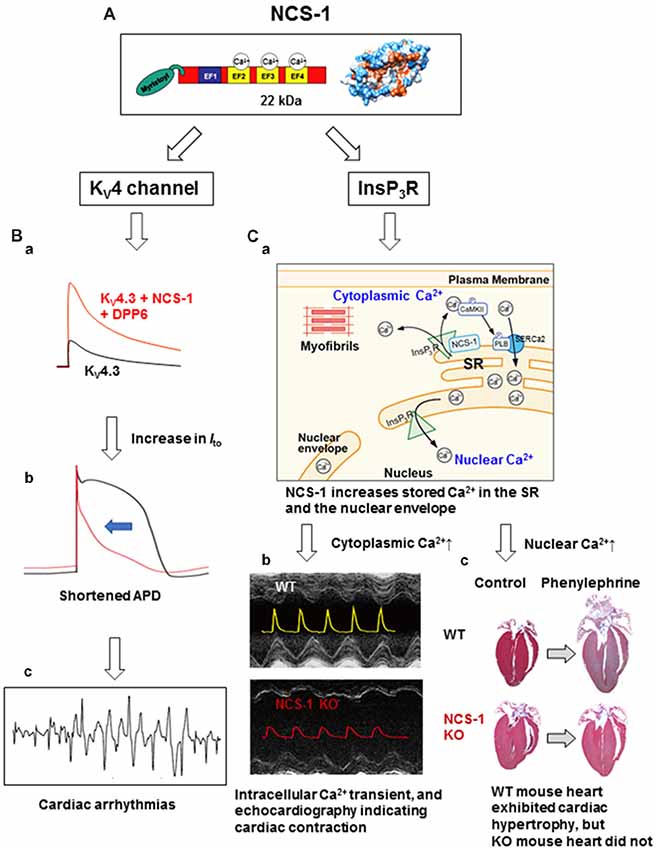
Figure 1. The structure and cardiac functions of NCS-1. (A) The structure of NCS-1 (PDB: 1G8I). (B) Cartoons demonstrate that NCS-1 and DPP6, both are auxiliary subunits of KV4 channels in Purkinje fiber (PF), slows inactivation kinetics of KV4 current and increases the current amplitude, respectively, thus increase Ito-mediated K+ efflux (Ba). This would accelerate PF repolarization and shortening of APD (Bb), and may lead to cardiac arrhythmias (Bc). The same concepts of the traces in (B) were originally reported by Xiao et al. (2013). (C) NCS-1 also interacts with IP3Rs on the SR and increases local Ca2+. This activates CaMKII, followed by CaMKII-dependent phosphorylation of PLB that enhances the Ca2+-pump activity of SERCa2, resulting in the increase in SR Ca2+ content (Ca). This increases the global Ca2+ transient and contraction in the immature heart. NCS-1 deficiency results in a smaller Ca2+-transient and contraction (Cb; the composite figure of echocardiograms and Ca2+ transients are based on data from Nakamura et al., 2011). NCS-1 also increases nuclear Ca2+ levels because the SR and the nuclear envelope are interconnected (Ca). NCS-1-mediated increase in nuclear Ca2+ signal can promote hormone-induced cardiac hypertrophy, whereas NCS-1 deficiency prevents progression of hypertrophy (Cc; adapted from Nakamura et al., 2011). Phenylephrine is an agonist of α1-adrenergic receptor. For further details, please refer to the text. APD, action potential duration; CaMKII, calcium/calmodulin-dependent protein kinase II; DPP6, dipeptidyl peptidase-like protein 6; EF, EF-hand; InsP3R, inositol 3,4,5-trisphosphate receptor; Ito, transient outward K+ current; KO, knock-out; KV, voltage-dependent potassium channel; NCS-1, neuronal Ca2+ sensor-1; PLB, phospholamban; SERCa2, sarcoplasmic/endoplasmic reticulum calcium ATPase 2; SR, sarcoplasmic reticulum; WT, wild type.
The functional roles of NCS-1 are still being elucidated. Currently, known functions include the regulation of diverse target proteins, including phosphatidylinositol 4-kinase (PI-4K), voltage- and ligand-gated ion channels, and interleukin-1 receptor accessory protein like-1 (IL1RAPL1). Here, we summarize the current understanding of NCS-1 regulation of some of these target proteins and how it affects brain and cardiac functions (Table 1). Even though NCS-1 has a well-characterized function in these organ systems, it should be pointed out that NCS-1 may have more diverse functions in human physiology and disease, such as a potential role in oncogenesis (Jerng et al., 2004). Such roles should be the focus of future studies.
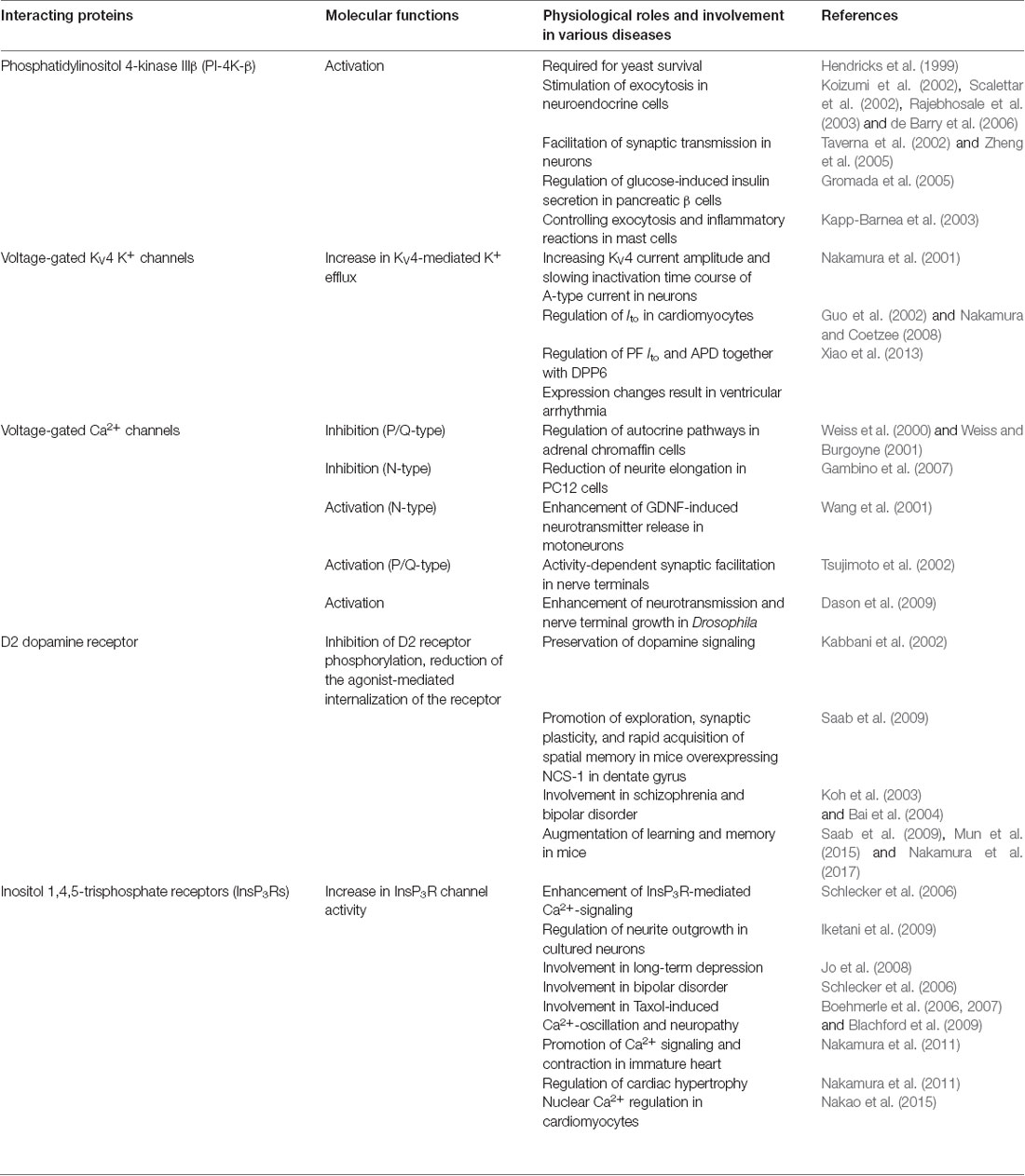
Table 1. Neuronal Ca2+ sensor-1 (NCS-1) interacting proteins, known functions of NCS-1 mediated by those proteins and involvement in various diseases.
Phosphatidylinositol 4-Kinase
Role in Exocytosis and Secretion
PI-4K IIIβ (PI-4K-β) catalyzes the synthesis of phosphatidylinositol 4-phosphate, which is a late limiting step in the synthesis of phosphatidylinositol 4,5-bisphosphate, an important lipid regulator of many cellular functions including exocytosis. A yeast homolog of NCS-1 and PI-4-K interact, and NCS-1-induced activation of PI-4-K is required for yeast survival (Hendricks et al., 1999). Structural support for interaction was obtained from a recent NMR structure of Ca2+-bound yeast NCS-1 (Ncs1) in complex with an N-terminal yeast PI-4-K (Pik1) fragment (Strahl et al., 2007). This interaction was also detected in neuroendocrine cells (Koizumi et al., 2002; Scalettar et al., 2002; Rajebhosale et al., 2003; de Barry et al., 2006), neurons (Taverna et al., 2002; Zheng et al., 2005) and other cell types including pancreatic beta cells (Gromada et al., 2005) and mast cells (Kapp-Barnea et al., 2003), and was shown to facilitate exocytosis and secretion in these cells (Table 1). However, previous reports have suggested no direct interaction in neurons (Bartlett et al., 2000). This contradiction may be explained by the presence of newly discovered PI-4-Kβ regulators, calneurons. While calneurons interact with PI-4K-β at low Ca2+ levels to inhibit its enzyme activity, NCS-1 binds to PI-4K-β at high Ca2+ levels to activate it (Mikhaylova et al., 2009), suggesting that calneurons and NCS-1 compete for PI-4-K-β interaction depending on intracellular Ca2+ levels. Thus, when the intracellular Ca2+ level is low, its interaction might be difficult to be detected.
Voltage-Gated KV4 K+ Channels
Regulation of Excitability in the Brain
The V7 Drosophila mutant that overexpresses NCS-1 has a phenotype of hyperactivity, which results in the proposal that NCS-1 facilitates neurotransmission, possibly by regulating the activities of ion channels (Pongs et al., 1993; Poulain et al., 1994). Indeed, we found that NCS-1 is a Ca2+-sensitive regulatory component of a native K+ current (Nakamura et al., 2001; Table 1). In the brain and heart, rapidly inactivating (A-type) voltage-gated K+ currents control cellular excitability. Although the pore-forming alpha-subunits of these channels are considered to be KV4 channels (Serôdio et al., 1994; Fiset et al., 1997; Nakamura et al., 1997), the kinetic properties of KV4 channels differ from native A-type currents, suggesting the presence of regulatory subunits. KChIPs, a member of the NCS protein subfamily, were initially reported as a specific KV4 regulatory subunit (An et al., 2000). Because NCS-1 modulates KV4 currents similar to KChIPs, by increasing current amplitude and slowing the inactivation time course, and NCS-1 physically interacts with KV4.2 in mouse brain, it was identified as a regulator of A-type K+ currents in neurons (Nakamura et al., 2001; Table 1).
Involvement in Cardiac Arrhythmia
This interaction and activation also occurs in adult mouse cardiomyocytes (Guo et al., 2002) and in zebrafish heart (Nakamura and Coetzee, 2008), which lacks KChIPs. The differential regulation of KV4 channels by NCS-1 and KChIPs in specific tissues and cell types was an unaddressed topic, and this was clearly demonstrated in the report by Nattel’s group (Xiao et al., 2013). Purkinje fibers (PFs) show an unusual form of transient outward K+ current Ito with slow recovery kinetics and TEA sensitivity compared with ventricular Ito, suggesting a distinct molecular composition. This group found that NCS-1 and DPP6, which were also reported to be auxiliary subunits of KV4 K+ channels (Jerng et al., 2004), are preferentially enriched in PFs, while KChIP2, an essential subunit of ventricular KV4.3 is weakly expressed. Moreover, NCS-1 slowed inactivation kinetics of KV4.3, while DPP6 increased its current amplitude, thus increasing the Ito-mediated K+ efflux (Figure 1Ba), which would accelerate PF repolarization and shortening of action potentials (Figure 1Bb; similar computer simulation was reported by Xiao et al., 2013). Thus, overexpression of KV4 auxiliary subunits may result in steep transmural repolarization gradients in PFs with adjacent ventricular tissues that induces coupled ectopic activity, and potentially leads to lethal arrhythmias (Figure 1Bc). NCS-1 also interacts with the anti-cancer drug taxol (Boehmerle et al., 2006), and is involved in the regulation of taxol-induced cardiac arrhythmia (Zhang et al., 2010). Thus, NCS-1 can be a potential target for anti-arrhythmic therapy.
Voltage-Gated Ca2+ Channels
Neurotransmitter Release and Neurite Elongation
NCS-1 regulates voltage-gated Ca2+ channels. Published data, however, are somewhat inconsistent with reports demonstrating both positive and negative effects (Table 1). NCS-1 was described to inhibit P/Q-type Ca2+ channels an regulates autocrine pathways in adrenal chromaffin cells (Weiss et al., 2000; Weiss and Burgoyne, 2001) and N-type Ca2+ channels in PC12 cells, which reduces neurite elongation (Gambino et al., 2007). Other studies, in contrast, have demonstrated positive regulation of N-type Ca2+ channels, causing glial cell line-derived neurotrophic factor (GDNF)-induced enhancement of neurotransmitter release in motoneurons (Wang et al., 2001). Activation of P/Q-type Ca2+ channels by NCS-1 causes activity-dependent synaptic facilitation in nerve terminals (Tsujimoto et al., 2002). In Drosophila, NCS-1 enhances neurotransmission and nerve terminal growth, by functionally interacting with the α1 subunit of the voltage-gated Ca2+ channel (Dason et al., 2009). The possible reason for the apparent contradictory findings is that the effects may be cell type-specific and/or mediated by accessory proteins, such as a β-subunit (Rousset et al., 2003), or dependent on other interacting proteins, such as IL1RAPL1 that cooperatively regulates the N-type Ca2+ channel via NCS-1 (Gambino et al., 2007). In addition, regulation of the Ca2+ channel by Ca2+ influx through the channel should be considered. Well-characterized examples are Ca2+-dependent inactivation (Standen and Stanfield, 1982) and facilitation (Dolphin, 1996) of Ca2+ channels regulated by other Ca2+-binding proteins, such as calmodulin (Budde et al., 2002; Christel and Lee, 2012). Future research should aim to understand the regulatory mechanisms of Ca2+ channels that involve NCS-1.
D2 Dopamine Receptor
Role in Synaptic Plasticity and Psychiatric Illness
Dopamine plays an important role in the reward system of the brain. Disorders of the dopamine system result in several psychiatric and neurological conditions. Dopamine transmission is regulated by dopamine receptor-interacting proteins (DRIP), including NCS-1, calcyon, and DARPP-32. NCS-1 directly interacts with the D2 dopamine receptor, inhibits D2 receptor phosphorylation, and reduces the agonist-mediated internalization of the receptor (Kabbani et al., 2002), indicating that NCS-1 preserves dopamine signaling (Table 1). Indeed, modest NCS-1 overexpression in the dentate gyrus in mice promotes exploration, synaptic plasticity, and rapid acquisition of spatial memory (Saab et al., 2009). NCS-1 is upregulated in the prefrontal cortex of patients with schizophrenia and bipolar disorder (Koh et al., 2003; Bai et al., 2004). Because the levels of other DRIPs were also changed in patients with schizophrenia (Bai et al., 2004; Souza et al., 2008), DRIP signaling is possibly involved in psychiatric disorders. Furthermore, recent findings indicate the N-terminal 60 residues of NCS-1 are responsible for binding to the D2 receptor (Woll et al., 2011). Such knowledge would provide an opportunity to screen for drugs that can specifically interrupt the NCS-1-D2 dopamine receptor interaction and thus prevent psychiatric disorders.
Role in Learning and Memory and Possible Mechanism
Several studies have demonstrated that NCS-1 modulates learning and memory. For example, deletion or reduction of NCS-1 resulted in dysfunction of learning and memory in Caenorhabditis elegans (Gomez et al., 2001), as well as in mice (Mun et al., 2015), whereas mice overexpressing NCS-1 rapidly acquire spatial memory (Saab et al., 2009). Thus, NCS-1 affects neurophysiology, possibly through various interacting proteins. A mechanism underlying NCS-1-mediated learning and memory was further investigated (Nakamura et al., 2017). Ncs1−/− mice exhibited impaired spatial learning and memory function in the Morris Water Maze test, with slight changes in their exercise activity or a structural change in the hippocampus. However, the levels of brain-derived neurotrophic factor (BDNF), a key regulator of memory function, and dopamine were decreased. Furthermore, phosphorylation of Ca2+/calmodulin-dependent protein kinase II-α (CaMKII-α), which regulates long-term potentiation, and BDNF levels were decreased, suggesting that CaMKII-α signaling that increases BDNF production is at least partly involved in NCS-1-mediated learning and memory function.
Inositol 1,4,5-Trisphosphate Receptors
Role in Neuronal Pathogenesis
Ca2+ signaling via inositol 1,4,5-trisphosphate receptors (InsP3Rs) regulates cellular function and is involved in pathogenesis (Table 1). NCS-1 physically interacts with InsP3R1 and enhances InsP3R-mediated Ca2+ signaling in rat brains. Indeed, physical/functional interaction of these proteins was directly demonstrated in an in vitro experiment showing that the addition of NCS-1 to InsP3R1 in the lipid bilayer increased InsP3R channel activity (Schlecker et al., 2006). This interaction was also detected at the growth cone region of neurites in cultured neurons, and indicated to be crucial for neurite outgrowth (Iketani et al., 2009). Metabotropic glutamate receptor-mediated cis also mediated by NCS-1/InsP3R interaction (Jo et al., 2008). Pathologically, NCS-1/InsP3R1 interaction is believed to be involved in bipolar disorder (Schlecker et al., 2006) because lithium, a medical drug for bipolar disorder, inhibited the NCS-1-induced enhancement of InsP3R function. NCS-1/InsP3R interaction is also considered to mediate neuropathy (Boehmerle et al., 2006, 2007; Blachford et al., 2009), as paclitaxel (taxol), a chemotherapeutic agent used for the treatment of solid cancers, modulates the expression/function of NCS-1, and hence InsP3R1-mediated Ca2+ signaling.
Enhancement of Immature Heart Contraction and Hypertrophy
NCS-1/InsP3R interaction is also detected in the heart and is crucial for contraction at the immature stage and cardiac hypertrophy in adult (Nakamura et al., 2011; Table 1 and Figure 1C). A high expression of NCS-1 was found in the immature heart (Nakamura et al., 2003, 2011), but its function at this stage was unknown. Using Ncs1−/− mice, Nakamura et al demonstrated that NCS-1 contributes to an increase in contraction and Ca2+ signaling, specifically at the immature stage (Figure 1Cb). Intracellular Ca2+ levels and the sarcoplasmic reticulum (SR) Ca2+ content were significantly lower in Ncs1−/− myocytes at the neonatal stage than in wild-type cells. Mechanistically, the interaction of NCS-1 with InsP3R increases InsP3R-dependent Ca2+ signaling, followed by the activation of CaMKII-dependent pathways, and promotes SR Ca2+ pump via the phosphorylation of phospholamban (PLB), which ultimately induce increase in the SR Ca2+ content and global Ca2+ transient, thus cardiomyocyte contraction (Figures 1Ca,b). The importance of crosstalk among NCS-1, InsP3Rs, and CaMKII in the immature hearts was evident by the high expression of all three proteins in immature hearts (Nakamura et al., 2011). In the neonatal mouse heart, the structure and function of SR are immature. Nonetheless, it is considered a primary source of Ca2+ necessary for muscle contractions, suggesting the existence of factors missing during development and promoting SR-dependent excitation-contraction (E-C) coupling in the postnatal stages. NCS-1 may act as one of these missing factors. Numbers of molecules which levels are high at the immature stage are often up-regulated in the disease conditions, such as cardiac hypertrophy. NCS-1 is also highly expressed during the early stages of hypertrophy in the adult heart and promotes the progression of hypertrophy, at least in part, through InsP3R activation (Nakamura et al., 2011; Figure 1Cc). A possible molecular mechanism is suggested in the next section.
Regulation of Nuclear Ca2+ Signals
The aforementioned data indicate that NCS-1 can discretely regulate different types of Ca2+ signaling pathways in the heart (i.e., regulation of E-C coupling in immature heart and changes in gene expression in the adult heart). Recent evidence suggests that gene transcription is regulated by nuclear Ca2+ signals. However, the mechanisms underlying nuclear Ca2+ regulation and its relationship to cytoplasmic Ca2+ regulation have not been completely solved. Using a subcellular-specific, fluorescent protein-based Ca2+ indicator GECO (Zhao et al., 2011; Nakao et al., 2015) confirmed the following: (1) nuclear Ca2+ transients were elicited by both electrical and receptor stimulations (with insulin-like growth factor-1, IGF-1) in neonatal mouse ventricular myocytes; and (2) receptor stimulation-elicited nuclear Ca2+ transients were mainly mediated by InsP3Rs. Furthermore, based on the evidence that IGF-1-elicited nuclear Ca2+ transient was significantly diminished in Ncs1−/− cardiomyocytes, NCS-1 is involved in the receptor stimulation-induced nuclear Ca2+ regulation through interaction with InsP3Rs (Nakao et al., 2015; Table 1). This may contribute to NCS-1-mediated hypertrophy, which was described above. A possible mechanism underlying a dual effect of NCS-1 on nuclear Ca2+ signals and E-C coupling is that NCS-1 increases the Ca2+ content of SR (Nakamura et al., 2011) that is interconnected to the nuclear envelope (Wu and Bers, 2006) and consequently may increase nuclear Ca2+ (Nakao et al., 2015; Figure 1Ca).
Other Functions of NCS-1 With Unknown Interacting Proteins
Enhancement of Neuronal Survival After Injury
Physical or chemical injury and genetic abnormalities can result in neuronal degeneration, which may underlie human neurodegenerative disorders, such as Alzheimer’s disease and Parkinson’s disease. Both intrinsic and extrinsic factors, including neurotrophic factors, can activate the anti-apoptotic process to rescue neuronal cell death. The signaling pathway leading to cell survival remains unresolved. In this regard, NCS-1 was found to be a novel Ca2+-dependent survival-promoting factor upregulated in injured neurons (Nakamura et al., 2006), based on the following observations. (1) NCS-1 expression increases in injured neurons; (2) NCS-1 overexpression diminished various stress-induced neuronal cell death in culture; and (3) the dominant negative EF-hand NCS-1 mutant (E120Q) accelerated cell death. Mechanistically, the expression level of NCS-1 in neuron is increased by GDNF, a neurotrophic factor upregulated by neuronal injury, and NCS-1 mediates GDNF survival signaling via the activation of the Akt pathway.
Role in Stress Tolerance in Cardiomyocytes
Not only in neurons, NCS-1 also plays a key role in protecting cardiomyocytes against stress through the activation of mitochondrial detoxification pathways (Nakamura et al., 2016). Excessive stress induces cytosolic Ca2+ overload and cell death. In contrast, mild forms of stress lead to physiologically relevant changes in Ca2+, which activate Ca2+-dependent survival pathways by binding to Ca2+-sensor proteins. As one such protein, NCS-1 was found to play important roles in Ca2+-dependent survival signaling. Ncs1−/− myocytes were more susceptible to oxidative and metabolic stress, and cellular ATP levels, mitochondrial respiration and biosynthesis were significantly reduced in these cells. In wild-type myocytes, mild oxidative stress increased the mitochondrial proton leak, which exerted a protective effect by inhibiting the production of reactive oxygen species. However, this response was diminished in Ncs1−/− cardiomyocytes, thus resulting in cell death. Similar susceptibility was also observed in Ncs1−/− hearts subjected to ischemia-reperfusion injury. In these hearts, molecules regulating Ca2+-dependent survival pathways, such as Akt and PGC-1α, which promote mitochondrial biogenesis and function, were significantly downregulated compared to wild-type hearts. These data demonstrate a novel role of NCS-1 that contributes to stress tolerance in cardiomyocytes, partly by the activation of Ca2+-dependent survival pathways. NCS-1 may also participate in cardioprotection by mediating receptor-signaling pathways. For example, NCS-1 associates with, and modulates, adenosine receptor activity (Navarro et al., 2012). Given the central role of adenosine in mediating the protective effects of ischemic preconditioning (Cohen and Downey, 2008), it is entirely possible that the cardioprotective effects of NCS-1 is partially mediated by this pathway.
Conclusion
Recently, studies have elucidated new roles of NCS-1 in physiology and pathophysiology. In this review, we have mainly focused on NCS-1 in the neuronal system and heart. Our particular interest is the emerging theme that NCS-1 directly regulates the function of several ion channels that permeate Ca2+ (e.g., several types of voltage-gated Ca2+ channels, ionotropic dopamine receptors, and InsP3Rs), suggesting a general role of Ca2+ influx via the channel that binds to NCS-1 and consequently regulates channel functions and/or downstream Ca2+-dependent signaling, which affect various neuronal and cardiac functions. Furthermore, many established roles of NCS-1 are related to protective responses of cells against exogenous stress that leads to mild increases in cytosolic Ca2+. This suggest that intracellular Ca2+ as a determinant of cell survival and cell death, and Ca2+-sensor proteins, such as NCS-1, may serve as a switch to proceed the signal. We believe that this review provides intriguing observations and compels researchers to conduct detailed investigations and extend their studies on NCS-1 and its important regulatory proteins.
Author Contributions
TN conducted most part of research, wrote, organized, and finalized the article. SN did some experiments and wrote some part of the article. SW contributed to the discussion on all part of the study and wrote some part of the manuscript. SN and SW wrote and edited some parts of the article.
Funding
This work was supported by Grant Number JP24590293, 15K08200 JSPS KAKENHI, The Naito Science and Engineering Foundation #J004, and the Salt Science Research Foundation (Grant No. 1547 and 1643), and Intramural Research Fund for Cardiovascular Disease of the National Cerebral and Cardiovascular Center #22-2-3 obtained by TN; Grant Number JP26460312, JSPS KAKENHI obtained by SW; Grant Number JP25860174, JSPS KAKENHI obtained by SN.
Conflict of Interest Statement
The authors declare that the research was conducted in the absence of any commercial or financial relationships that could be construed as a potential conflict of interest.
References
An, W. F., Bowlby, M. R., Betty, M., Cao, J., Ling, H. P., Mendoza, G., et al. (2000). Modulation of A-type potassium channels by a family of calcium sensors. Nature 403, 553–556. doi: 10.1038/35000592
Bai, J., He, F., Novikova, S. I., Undie, A. S., Dracheva, S., Haroutunian, V., et al. (2004). Abnormalities in the dopamine system in schizophrenia may lie in altered levels of dopamine receptor-interacting proteins. Biol. Psychiatry 56, 427–440. doi: 10.1016/j.biopsych.2004.06.022
Bartlett, S. E., Reynolds, A. J., Weible, M., Jeromin, A., Roder, J., and Hendry, I. A. (2000). PtdIns 4-kinaseβ and neuronal calcium sensor-1 co-localize but may not directly associate in mammalian neurons. J. Neurosci. Res. 62, 216–224. doi: 10.1002/1097-4547(20001015)62:2<216::AID-JNR6>3.0.CO;2-A
Blachford, C., Celić, A., Petri, E. T., and Ehrlich, B. E. (2009). Discrete proteolysis of neuronal calcium sensor-1 (NCS-1) by mu-calpain disrupts calcium binding. Cell Calcium 46, 257–262. doi: 10.1016/j.ceca.2009.08.002
Boehmerle, W., Splittgerber, U., Lazarus, M. B., Mckenzie, K. M., Johnston, D. G., Austin, D. J., et al. (2006). Paclitaxel induces calcium oscillations via an inositol 1,4,5-trisphosphate receptor and neuronal calcium sensor 1-dependent mechanism. Proc. Natl. Acad. Sci. U S A 103, 18356–18361. doi: 10.1073/pnas.0607240103
Boehmerle, W., Zhang, K., Sivula, M., Heidrich, F. M., Lee, Y., Jordt, S. E., et al. (2007). Chronic exposure to paclitaxel diminishes phosphoinositide signaling by calpain-mediated neuronal calcium sensor-1 degradation. Proc. Natl. Acad. Sci. U S A 104, 11103–11108. doi: 10.1073/pnas.0701546104
Budde, T., Meuth, S., and Pape, H. C. (2002). Calcium-dependent inactivation of neuronal calcium channels. Nat. Rev. Neurosci. 3, 873–883. doi: 10.1038/nrn959
Christel, C., and Lee, A. (2012). Ca2+-dependent modulation of voltage-gated Ca2+ channels. Biochim. Biophys. Acta 1820, 1243–1252. doi: 10.1016/j.bbagen.2011.12.012
Cohen, M. V., and Downey, J. M. (2008). Adenosine: trigger and mediator of cardioprotection. Basic Res. Cardiol. 103, 203–215. doi: 10.1007/s00395-007-0687-7
Dason, J. S., Romero-Pozuelo, J., Marin, L., Iyengar, B. G., Klose, M. K., Ferrus, A., et al. (2009). Frequenin/NCS-1 and the Ca2+-channel α1-subunit co-regulate synaptic transmission and nerve-terminal growth. J. Cell Sci. 122, 4109–4121. doi: 10.1242/jcs.055095
de Barry, J., Janoshazi, A., Dupont, J. L., Procksch, O., Chasserot-Golaz, S., Jeromin, A., et al. (2006). Functional implication of neuronal calcium sensor-1 and phosphoinositol 4-kinase-β interaction in regulated exocytosis of PC12 cells. J. Biol. Chem. 281, 18098–18111. doi: 10.1074/jbc.m509842200
Dolphin, A. C. (1996). Facilitation of Ca2+ current in excitable cells. Trends Neurosci. 19, 35–43. doi: 10.1016/0166-2236(96)81865-0
Fiset, C., Clark, R. B., Shimoni, Y., and Giles, W. R. (1997). Shal-type channels contribute to the Ca2+-independent transient outward K+ current in rat ventricle. J. Physiol. 500, 51–64. doi: 10.1113/jphysiol.1997.sp021998
Gambino, F., Pavlowsky, A., Begle, A., Dupont, J. L., Bahi, N., Courjaret, R., et al. (2007). IL1-receptor accessory protein-like 1 (IL1RAPL1), a protein involved in cognitive functions, regulates N-type Ca2+-channel and neurite elongation. Proc. Natl. Acad. Sci. U S A 104, 9063–9068. doi: 10.1073/pnas.0701133104
Gomez, M., De Castro, E., Guarin, E., Sasakura, H., Kuhara, A., Mori, I., et al. (2001). Ca2+ signaling via the neuronal calcium sensor-1 regulates associative learning and memory in C. elegans. Neuron 30, 241–248. doi: 10.1016/s0896-6273(01)00276-8
Gromada, J., Bark, C., Smidt, K., Efanov, A. M., Janson, J., Mandic, S. A., et al. (2005). Neuronal calcium sensor-1 potentiates glucose-dependent exocytosis in pancreatic β cells through activation of phosphatidylinositol 4-kinase β. Proc. Natl. Acad. Sci. U S A 102, 10303–10308. doi: 10.1073/pnas.0504487102
Guo, W., Malin, S. A., Johns, D. C., Jeromin, A., and Nerbonne, J. M. (2002). Modulation of Kv4-encoded K+ currents in the mammalian myocardium by neuronal calcium sensor-1. J. Biol. Chem. 277, 26436–26443. doi: 10.1074/jbc.m201431200
Hendricks, K. B., Wang, B. Q., Schnieders, E. A., and Thorner, J. (1999). Yeast homologue of neuronal frequenin is a regulator of phosphatidylinositol-4-OH kinase. Nat. Cell Biol. 1, 234–241. doi: 10.1038/12058
Iketani, M., Imaizumi, C., Nakamura, F., Jeromin, A., Mikoshiba, K., Goshima, Y., et al. (2009). Regulation of neurite outgrowth mediated by neuronal calcium sensor-1 and inositol 1,4,5-trisphosphate receptor in nerve growth cones. Neuroscience 161, 743–752. doi: 10.1016/j.neuroscience.2009.04.019
Ikura, M. (1996). Calcium binding and conformational response in EF-hand proteins. Trends Biochem. Sci. 21, 14–17. doi: 10.1016/s0968-0004(06)80021-6
Jerng, H. H., Pfaffinger, P. J., and Covarrubias, M. (2004). Molecular physiology and modulation of somatodendritic A-type potassium channels. Mol. Cell. Neurosci. 27, 343–369. doi: 10.1016/j.mcn.2004.06.011
Jo, J., Heon, S., Kim, M. J., Son, G. H., Park, Y., Henley, J. M., et al. (2008). Metabotropic glutamate receptor-mediated LTD involves two interacting Ca2+ sensors, NCS-1 and PICK1. Neuron 60, 1095–1111. doi: 10.1016/j.neuron.2008.10.050
Kabbani, N., Negyessy, L., Lin, R., Goldman-Rakic, P., and Levenson, R. (2002). Interaction with neuronal calcium sensor NCS-1 mediates desensitization of the D2 dopamine receptor. J. Neurosci. 22, 8476–8486. doi: 10.1523/jneurosci.22-19-08476.2002
Kapp-Barnea, Y., Melnikov, S., Shefler, I., Jeromin, A., and Sagi-Eisenberg, R. (2003). Neuronal calcium sensor-1 and phosphatidylinositol 4-kinase β regulate IgE receptor-triggered exocytosis in cultured mast cells. J. Immunol. 171, 5320–5327. doi: 10.4049/jimmunol.171.10.5320
Koh, P. O., Undie, A. S., Kabbani, N., Levenson, R., Goldman-Rakic, P. S., and Lidow, M. S. (2003). Up-regulation of neuronal calcium sensor-1 (NCS-1) in the prefrontal cortex of schizophrenic and bipolar patients. Proc. Natl. Acad. Sci. U S A 100, 313–317. doi: 10.1073/pnas.232693499
Koizumi, S., Rosa, P., Willars, G. B., Challiss, R. A., Taverna, E., Francolini, M., et al. (2002). Mechanisms underlying the neuronal calcium sensor-1-evoked enhancement of exocytosis in PC12 cells. J. Biol. Chem. 277, 30315–30324. doi: 10.1074/jbc.m201132200
Mikhaylova, M., Reddy, P. P., Munsch, T., Landgraf, P., Suman, S. K., Smalla, K. H., et al. (2009). Calneurons provide a calcium threshold for trans-Golgi network to plasma membrane trafficking. Proc. Natl. Acad. Sci. U S A 106, 9093–9098. doi: 10.1073/pnas.0903001106
Mun, H. S., Saab, B. J., Ng, E., Mcgirr, A., Lipina, T. V., Gondo, Y., et al. (2015). Self-directed exploration provides a Ncs1-dependent learning bonus. Sci. Rep. 5:17697. doi: 10.1038/srep17697
Nakamura, T. Y., and Coetzee, W. A. (2008). Functional and pharmacological characterization of a Shal-related K+ channel subunit in Zebrafish. BMC Physiol. 8:2. doi: 10.1186/1472-6793-8-2
Nakamura, T. Y., Coetzee, W. A., Vega-Saenz De Miera, E., Artman, M., and Rudy, B. (1997). Modulation of KV4 channels, key components of rat ventricular transient outward K+ current, by PKC. Am. J. Physiol. 273, H1775–H1786. doi: 10.1152/ajpheart.1997.273.4.h1775
Nakamura, T. Y., Jeromin, A., Mikoshiba, K., and Wakabayashi, S. (2011). Neuronal calcium sensor-1 promotes immature heart function and hypertrophy by enhancing Ca2+ signals. Cir. Res. 109, 512–523. doi: 10.1161/CIRCRESAHA.111.248864
Nakamura, T. Y., Jeromin, A., Smith, G., Kurushima, H., Koga, H., Nakabeppu, Y., et al. (2006). Novel role of neuronal Ca2+ sensor-1 as a survival factor up-regulated in injured neurons. J. Cell Biol. 172, 1081–1091. doi: 10.1083/jcb.200508156
Nakamura, T. Y., Nakao, S., and Wakabayashi, S. (2016). Neuronal Ca2+ sensor-1 contributes to stress tolerance in cardiomyocytes via activation of mitochondrial detoxification pathways. J. Mol. Cell. Cardiol. 99, 23–34. doi: 10.1016/j.yjmcc.2016.08.013
Nakamura, T. Y., Nakao, S., Nakajo, Y., Takahashi, J. C., Wakabayashi, S., and Yanamoto, H. (2017). Possible signaling pathways mediating neuronal calcium sensor-1-dependent spatial learning and memory in mice. PLoS One 12:e0170829. doi: 10.1371/journal.pone.0170829
Nakamura, T. Y., Pountney, D. J., Ozaita, A., Nandi, S., Ueda, S., Rudy, B., et al. (2001). A role for frequenin, a Ca2+-binding protein, as a regulator of KV4 K+-currents. Proc. Natl. Acad. Sci. U S A 98, 12808–12813. doi: 10.1073/pnas.221168498
Nakamura, T. Y., Sturm, E., Pountney, D. J., Orenzoff, B., Artman, M., and Coetzee, W. A. (2003). Developmental expression of NCS-1 (frequenin), a regulator of KV4 K+ channels, in mouse heart. Pediatr. Res. 53, 554–557. doi: 10.1203/01.PDR.0000057203.72435.C9
Nakao, S., Wakabayashi, S., and Nakamura, T. Y. (2015). Stimulus-dependent regulation of nuclear Ca2+ signaling in cardiomyocytes: a role of neuronal calcium sensor-1. PLoS One 10:e0125050. doi: 10.1371/journal.pone.0125050
Navarro, G., Hradsky, J., Lluís, C., Casado, V., McCormick, P. J., Kreutz, M. R., et al. (2012). NCS-1 associates with adenosine A2A receptors and modulates receptor function. Front. Mol. Neurosci. 5:53. doi: 10.3389/fnmol.2012.00053
Pongs, O., Lindemeier, J., Zhu, X. R., Theil, T., Engelkamp, D., Krah-Jentgens, I., et al. (1993). Frequenin-a novel calcium-binding protein that modulates synaptic efficacy in the Drosophila nervous system. Neuron 11, 15–28. doi: 10.1016/0896-6273(93)90267-u
Poulain, C., Ferrús, A., and Mallart, A. (1994). Modulation of type A K+ current in Drosophila larval muscle by internal Ca2+; effects of the overexpression of frequenin. Pflugers Arch. 427, 71–79. doi: 10.1007/bf00585944
Rajebhosale, M., Greenwood, S., Vidugiriene, J., Jeromin, A., and Hilfiker, S. (2003). Phosphatidylinositol 4-OH kinase is a downstream target of neuronal calcium sensor-1 in enhancing exocytosis in neuroendocrine cells. J. Biol. Chem. 278, 6075–6084. doi: 10.1074/jbc.M204702200
Rousset, M., Cens, T., Gavarini, S., Jeromin, A., and Charnet, P. (2003). Down-regulation of voltage-gated Ca2+ channels by neuronal calcium sensor-1 is β subunit-specific. J. Biol. Chem. 278, 7019–7026. doi: 10.1074/jbc.M209537200
Saab, B. J., Georgiou, J., Nath, A., Lee, F. J., Wang, M., Michalon, A., et al. (2009). NCS-1 in the dentate gyrus promotes exploration, synaptic plasticity, and rapid acquisition of spatial memory. Neuron 63, 643–656. doi: 10.1016/j.neuron.2009.08.014
Scalettar, B. A., Rosa, P., Taverna, E., Francolini, M., Tsuboi, T., Terakawa, S., et al. (2002). Neuronal calcium sensor-1 binds to regulated secretory organelles and functions in basal and stimulated exocytosis in PC12 cells. J. Cell Sci. 115, 2399–2412.
Schlecker, C., Boehmerle, W., Jeromin, A., DeGray, B., Varshney, A., Sharma, Y., et al. (2006). Neuronal calcium sensor-1 enhancement of InsP3 receptor activity is inhibited by therapeutic levels of lithium. J. Clin. Invest. 116, 1668–1674. doi: 10.1172/jci22466
Serôdio, P., Kentros, C., and Rudy, B. (1994). Identification of molecular components of A-type channels activating at subthreshold potentials. J. Neurophysiol. 72, 1516–1529. doi: 10.1152/jn.1994.72.4.1516
Souza, B. R., Motta, B. S., Rosa, D. V., Torres, K. C., Castro, A. A., Comim, C. M., et al. (2008). DARPP-32 and NCS-1 expression is not altered in brains of rats treated with typical or atypical antipsychotics. Neurochem. Res. 33, 533–538. doi: 10.1007/s11064-007-9470-2
Standen, N. B., and Stanfield, P. R. (1982). A binding-site model for calcium-channel inactivation that depends on calcium entry. Proc. R. Soc. Lond. B Biol. Sci. 217, 101–110. doi: 10.1098/rspb.1982.0097
Strahl, T., Huttner, I. G., Lusin, J. D., Osawa, M., King, D., Thorner, J., et al. (2007). Structural insights into activation of phosphatidylinositol 4-kinase (Pik1) by yeast frequenin (Frq1). J. Biol. Chem. 282, 30949–30959. doi: 10.1074/jbc.M705499200
Taverna, E., Francolini, M., Jeromin, A., Hilfiker, S., Roder, J., and Rosa, P. (2002). Neuronal calcium sensor 1 and phosphatidylinositol 4-OH kinase β interact in neuronal cells and are translocated to membranes during nucleotide-evoked exocytosis. J. Cell Sci. 115, 3909–3922. doi: 10.1242/jcs.00072
Tsujimoto, T., Jeromin, A., Saitoh, N., Roder, J. C., and Takahashi, T. (2002). Neuronal calcium sensor 1 and activity-dependent facilitation of P/Q-type calcium currents at presynaptic nerve terminals. Science 295, 2276–2279. doi: 10.1126/science.1068278
Wang, C. Y., Yang, F., He, X., Chow, A., Du, J., Russell, J. T., et al. (2001). Ca2+ binding protein frequenin mediates GDNF-induced potentiation of Ca2+ channels and transmitter release. Neuron 32, 99–112. doi: 10.1016/s0896-6273(01)00434-2
Weiss, J. L., Archer, D. A., and Burgoyne, R. D. (2000). Neuronal Ca2+ sensor-1/frequenin functions in an autocrine pathway regulating Ca2+ channels in bovine adrenal chromaffin cells. J. Biol. Chem. 275, 40082–40087. doi: 10.1074/jbc.M008603200
Weiss, J. L., and Burgoyne, R. D. (2001). Voltage-independent inhibition of P/Q-type Ca2+ channels in adrenal chromaffin cells via a neuronal Ca2+ sensor-1-dependent pathway involves Src family tyrosine kinase. J. Biol. Chem. 276, 44804–44811. doi: 10.1074/jbc.m103262200
Woll, M. P., De Cotiis, D. A., Bewley, M. C., Tacelosky, D. M., Levenson, R., and Flanagan, J. M. (2011). Interaction between the D2 dopamine receptor and neuronal calcium sensor-1 analyzed by fluorescence anisotropy. Biochemistry 50, 8780–8791. doi: 10.1021/bi200637e
Wu, X., and Bers, D. M. (2006). Sarcoplasmic reticulum and nuclear envelope are one highly interconnected Ca2+ store throughout cardiac myocyte. Circ. Res. 99, 283–291. doi: 10.1161/01.res.0000233386.02708.72
Xiao, L., Koopmann, T. T., Ördög, B., Postema, P. G., Verkerk, A. O., Iyer, V., et al. (2013). Unique cardiac purkinje fiber transient outward current β-subunit composition a potential molecular link to idiopathic ventricular fibrillation. Circ. Res. 112, 1310–1322. doi: 10.1161/circresaha.112.300227
Zhang, K., Heidrich, F. M., Degray, B., Boehmerle, W., and Ehrlich, B. E. (2010). Paclitaxel accelerates spontaneous calcium oscillations in cardiomyocytes by interacting with NCS-1 and the InsP3R. J. Mol. Cell. Cardiol. 49, 829–835. doi: 10.1016/j.yjmcc.2010.08.018
Zhao, Y. X., Araki, S., Wu, J., Teramoto, T., Chang, Y. F., Nakano, M., et al. (2011). An expanded palette of genetically encoded Ca2+ indicators. Science 333, 1888–1891. doi: 10.1126/science.1208592
Keywords: neuronal calcium sensor-1, frequenin, ion channel, survival, immature heart contraction, hypertrophy, nuclear Ca2+ signaling, learning and memory
Citation: Nakamura TY, Nakao S and Wakabayashi S (2019) Emerging Roles of Neuronal Ca2+ Sensor-1 in Cardiac and Neuronal Tissues: A Mini Review. Front. Mol. Neurosci. 12:56. doi: 10.3389/fnmol.2019.00056
Received: 18 October 2018; Accepted: 15 February 2019;
Published: 04 March 2019.
Edited by:
Daniele Dell’Orco, University of Verona, ItalyReviewed by:
Jeanne M. Nerbonne, Washington University in St. Louis, United StatesWilliam A. Coetzee, New York University, United States
Copyright © 2019 Nakamura, Nakao and Wakabayashi. This is an open-access article distributed under the terms of the Creative Commons Attribution License (CC BY). The use, distribution or reproduction in other forums is permitted, provided the original author(s) and the copyright owner(s) are credited and that the original publication in this journal is cited, in accordance with accepted academic practice. No use, distribution or reproduction is permitted which does not comply with these terms.
*Correspondence: Tomoe Y. Nakamura, tomoen@ncvc.go.jp